- 1School of Agriculture, Food and Wine, The University of Adelaide, Adelaide, SA, Australia
- 2Australian Plant Phenomics Facility, The Plant Accelerator, School of Agriculture, Food & Wine, The University of Adelaide, Adelaide, SA, Australia
Wheat (Triticum aestivum L.) production is increasingly challenged by simultaneous drought and heatwaves. We assessed the effect of both stresses combined on whole plant water use and carbohydrate partitioning in eight bread wheat genotypes that showed contrasting tolerance. Plant water use was monitored throughout growth, and water-soluble carbohydrates (WSC) and starch were measured following a 3-day heat treatment during drought. Final grain yield was increasingly associated with aboveground biomass and total water use with increasing stress intensity. Combined drought and heat stress immediately reduced daily water use in some genotypes and altered transpiration response to vapor pressure deficit during grain filling, compared to drought only. In grains, glucose and fructose concentrations measured 12 days after anthesis explained 43 and 40% of variation in final grain weight in the main spike, respectively. Starch concentrations in grains offset the reduction in WSC following drought or combined drought and heat stress in some genotypes, while in other genotypes both stresses altered the balance between WSC and starch concentrations. WSC were predominantly allocated to the spike in modern Australian varieties (28–50% of total WSC in the main stem), whereas the stem contained most WSC in older genotypes (67–87%). Drought and combined drought and heat stress increased WSC partitioning to the spike in older genotypes but not in the modern varieties. Ability to maintain transpiration, especially following combined drought and heat stress, appears essential for maintaining wheat productivity.
Introduction
Recent decades have witnessed severe drought and heatwaves worldwide, including in major wheat producing regions such as India, the United States, Russia, Western Europe, and Australia. These climatic conditions have a significant impact on global wheat production, with dramatic social and economic consequences (Van Dijk et al., 2013). Current climate projections predict drought and heatwaves will become more common and more intense in the future (Rosenzweig et al., 2014). One way to limit the impact of weather variability on productivity is to develop wheat varieties better adapted to the changing climate. This can be assisted by understanding the mechanisms underlying plants’ responses to complex stresses so as to identify the traits that characterize stress tolerant varieties for breeding. A small number of studies document the impact of combined drought and high temperature on wheat productivity and biological processes, especially during the reproductive developmental stage. The effect of combined stresses is more detrimental than the effect of an individual stress (Mittler, 2006). Both drought and high temperatures reduce expansive growth, accelerate flowering and shorten grain filling duration, resulting in a low grain set, size and weight (Asana and Williams, 1965; Pradhan et al., 2012). In combination, drought and high temperature impair the photosynthetic system, reduce stomatal conductance and gas exchange, and disrupt plants’ water relations (Machado and Paulsen, 2001; Shah and Paulsen, 2003). These additive alterations of morphological, physiological and cellular processes result in severe reductions in final grain weight. Although the major impact of combined drought and high temperature on wheat productivity is well-described, there is scarce information on the mechanisms that determine the ability to maintain grain weight in these unfavorable environments (also called tolerance).
Wheat harvested grain mass consists of 85% carbohydrates, of which ∼80% is starch (Stone and Morell, 2009). During grain filling, water-soluble carbohydrates (WSC) are delivered to grains either from current photosynthesis in photosynthesizing organs or from remobilization of WSC stored during the vegetative stage (Borrell et al., 1989; Schnyder, 1993). Abiotic stress after anthesis can limit gas exchange and damage the photosynthetic system, in which case stored carbohydrates become a major source of carbon for grain filling (Blum, 1998). In addition to the contribution from stem reserves, spike organs, especially awns, are thought to contribute to the grain filling process due to their active photosynthesis, especially in dry environments (Evans et al., 1972; Rebetzke et al., 2016). Carbohydrate synthesis and transport are closely related to water movements in plants. Open stomata are necessary for carbon capture and plants trade-off between maximizing carbon assimilation and limiting water loss through transpiration under adverse conditions such as drought. Carbohydrate transport via the phloem and distribution throughout the plant rely on water exchange with adjacent xylem (Hölttä et al., 2009), and the impact of water shortage on xylem water transport also impairs phloem function (Sevanto, 2018). Soluble carbohydrates also play an important role during drought by acting as compatible osmolytes to maintain cell turgor and favorable plant water status, thereby sustaining biological processes and soil water uptake (Blum, 2017). Maintaining plant hydration and enhancing carbohydrate remobilization to grains are considered key factors for crop productivity in limiting environments (Blum, 2006), and the interplay between plant water relations and carbohydrate metabolism and distribution highlights the importance of studying both mechanisms together.
Wheat genetic diversity is a valuable resource for the identification of new stress-adaptive mechanisms to environmental stress (Lopes et al., 2015). In this work, we describe the impact of combined drought and heat stress on whole plant water use and carbohydrate partitioning during grain filling in diverse wheat genotypes. We hypothesized that the combination of both stresses would alter plant water use and carbohydrate partitioning in the stem and spike, and that WSC availability would be a limiting factor for optimal grain weight under combined drought and heat stress.
Materials and Methods
Experiment 1
Genetic Material and Growth Conditions
Eight bread wheat (Triticum aestivum L.) genotypes were selected from a diverse panel of 534 wheat accessions from 44 countries described in Garcia et al. (2019). The diversity panel was previously subjected to post-anthesis drought and combined drought and heat stress in a pilot experiment and evaluated for plant total grain weight (yield) at harvest (data not shown). The selected genotypes contrasted for grain weight following drought or combined drought and heat stress, and consisted of three Australian older varieties (Currawa, Koda, Mendos), three Australian modern commercial varieties (Frame, Young, Gladius), one synthetic line from CIMMYT (Synthetic W7984) and one landrace from Ethiopia (Odessa ES19565) (Supplementary Table S1). The selected genotypes were released between 1912 and 2007. In this study, Frame, Gladius, and Young were considered as modern genotypes; the remaining genotypes were considered as older genotypes. Single seeds were sown in 40 cm × 15 cm round pots containing 8.2 kg of a mixture of 1:1:1 (v/v/v) clay/loam:UC Davis mix:cocopeat mix. Seeds were sown on 11 August 2016, late winter in the southern hemisphere. From 13 days after sowing (DAS) until the end of the experiment, plants were grown in a glasshouse (34°58′17.8′′ S, 138°38′23.4′′ E) on a gravimetric platform (Droughtspotter, Phenospex, Heerlen, Netherlands) that automatically irrigated to the pre-defined pot weight and recorded weights and water added (details in Water use and transpiration below). The 168 pots (6–7 pots per genotype and per treatment) were randomized to 168 Droughtspotter cells using a factorial, randomized complete block design, such that each block comprised one replicate of each Genotype–Treatment combination, except in three blocks that contained one empty pot each to estimate soil evaporation. The three treatment groups comprised well-watered (WW), drought (D) and combined drought & heat stress (D&H). In particular, all plants were well-watered [soil water potential = −0.3 MPa, gravimetric soil water content = 20% (g/g)] and grown in temperate conditions (22°C/15°C day/night) until anthesis of the main spike. Anthesis date was the first day anthers were observed on the main spike. One third of the plants (WW) were maintained in well-watered, cool conditions until harvest. The remaining plants (D, D&H) were subject to a 6 days drought treatment [soil water potential = −0.6 MPa, gravimetric soil water content = 12% (g/g)] starting 3 days after anthesis on the main spike of each individual; this was followed, in half of these plants (D&H), by a 3 days heat treatment at 37°C/27°C day/night (n = 7 for each accession in each treatment). Heat treatment was imposed in an adjacent glasshouse where plants were watered to weight manually. Drought was maintained until harvest in the D and D&H groups. LED lights (400 μE/m2/s) were installed above plants to minimize variations due to light intensity. A graphical representation of the experimental design is shown in Supplementary Figure S1. Environmental data are shown in Supplementary Figure S2.
Water Use and Transpiration
The gravimetric platform was configured to weigh each pot at regular time intervals. All weight and water values were automatically logged and water usage estimated hourly for each pot throughout the experiment. During the heat treatment in an adjacent glasshouse, plants were watered to weight manually at similar times as the drought treatment and weights recorded. Pots were watered at least six times daily (6 and 10 am, 12, 2, 4, and 10 pm). Pots containing soil only were weighed to estimate non-transpirational water loss under WW, D, and D&H treatments. The water usage is a combination of plant transpiration and evaporation from the soil surface, which was negligible in all treatments as estimated from pots containing soil only.
Carbohydrates Quantification
The main stem and spike of three plants per genotype per treatment were sampled 12 days after anthesis (DAA), i.e., 1 day after heat treatment in drought and heat stressed plants, and stored at −80°C for further analysis. Measurements were conducted separately on the stem, flag leaf sheath, covered peduncle, exposed peduncle, rachis, grains, palea, lemma, awns, and glumes. Dry weight was obtained by weighing the samples after freeze-drying. Total WSC in each tissue were determined using the anthrone method (Yemm and Willis, 1954) with some modifications: soluble sugars were extracted with 80% ethanol at 80°C for 1 h, then extracted with distilled water at 60°C for 1 h. The extraction was repeated as many times as needed until no coloration was observed. Supernatants were combined in the same tube for colorimetric assay. Starch content in grains was measured using the Megazyme Total Starch HK (K-TSHK 08/18, Megazyme, Bray, Ireland) according to the manufacturer’s instructions. Individual WSC measurements in grains were performed in four genotypes (Frame, Odessa, Synthetic, and Young). As plant morphology and grain number varied greatly between the genotypes, WSC and starch contents were expressed as g/g DW to allow for comparison between genotypes. Glucose, fructose and sucrose were analyzed in the same samples used for total WSC analysis using high performance anion exchange chromatography with pulsed amperometric detection HPAEC-PAD (Dionex ICS-5000; Thermo Fisher Scientific, Sunnyvale, United States). Separations were performed at 30°C and the flow rate was 0.5 mL/min. A 25 μL sample was injected on a Guard CarboPac PA20 (3 mm × 30 mm) in series with an analytical CarboPac PA20 (3 mm × 150 mm). The elution program consisted of 0.1M NaOH from 0 to 2 min, followed by increasing 1M sodium acetate concentration up to 20% from 2 min to 35 min, followed by increasing 1M sodium acetate concentration up to 100% from 35 to 36.5 min, a steady concentration from 36.5 to 37.5 min, followed by a 0.1M NaOH wash until return to equilibrium. Glucose, fructose and sucrose were identified based on glucose, fructose and sucrose standards. Fructans were identified by acid hydrolysis. Two WSC samples from the stem and awns were incubated with 0.2M trifluoroacetic acid (TFA) at 80°C for 30 min together with untreated samples. Treated and untreated samples were analyzed using HPAEC-PAD as described above. Glucose, fructose, and sucrose were quantified using external standards and peak areas determined using the instrument’s Chromeleon software. Fructans were quantified using peak areas.
Harvest Data at Maturity
Four plants per genotype per treatment were harvested at maturity to measure grain yield components. Total grain weight was determined for the main spike and for the whole plant. Seed number was counted using an automatic seed counter (Contador, Pfeuffer GmbH, Germany). Biomass weight included tillers, leaves and spikes but excluded grains. Plant height was measured from the base of the main stem to the top of the highest spike excluding awns. Biomass water use efficiency (bWUE) was calculated as the ratio of total aboveground biomass to total water use per plant. Grain water use efficiency (gWUE) was calculated as the ratio of total grain weight to total water use per plant.
Experiment 2
In order to test the reproducibility of water use results, the 2016 experiment described above was replicated in an independent experiment with four genotypes (Currawa, Synthetic W7984, Mendos, and Young) in 2017 with the same settings used for plant growth and treatments, except that plants were sown 1 month earlier. Plant water use was recorded using the gravimetric platform and three plants per genotype and per treatment were harvested at maturity and measured as before.
Statistical Analyses
The data were analyzed by two-way ANOVA with genotype and treatment as fixed factors for all measured yield component and biomass traits and for the analysis of carbohydrates within each tissue. Treatment means within genotypes were compared using Tukey’s HSD (honestly significant difference) test at p = < 0.1. Statistical analyses (ANOVA, Tukey’s tests, regression analyses) and graphical representation were performed using R software (version 3.4.4, R Core Team, 2019) and ASReml-R (Butler et al., 2009).
The recorded water use data were used to identify genotype and treatment effects on hourly transpiration rate (TR, mL/hr) and specific transpiration rate (STR, mL/hr/g biomass), with the proviso that soil evaporation and plant transpiration water losses were indistinguishable.
VPD was computed hourly from vapor capacity (VC, kPa) using the following formula:
where T is temperature and RH is relative humidity. It was further decided to model TR or STR (henceforth denoted y) as a simple linear function of VPD with (a) genotype × treatment interaction incorporated into the VPD slope and intercept parameters, and (b) error variance modeled as a function of treatment (but not genotype). The resulting model comprises 2 × 8 × 3 = 48 fixed effects of interest (i.e., slope and intercept parameters) and 3 variance estimates, represented symbolically as
where (a) yij is the TR or STR value for Pot i on hour j, (b) Poti is a random-effects term for variation between pots, and (c) spatial comprises fixed-effects terms for spatial variation within the greenhouse. The model was fitted separately to each of TR and STR using the R package ASReml-R4 (Butler et al., 2017). Analysis of the effect of 3 days heat stress on transpiration was narrowed to 30 days [0 to 30 days after treatment (DAT)] after heat stress, while all genotypes were still using water in the well-watered treatment, to limit the effects of intrinsic differences of grain-filling duration on transpiration and distinguish the effects of treatments.
Results
Combined Drought and Heat Stress Differentially Reduced Total Grain Weight at Harvest
The effect of drought and combined drought and heat stress (D&H) on total grain weight per plant at harvest (per plant yield) depended on genotype (Table 1). Drought reduced per plant yield in Currawa, Odessa, Frame, Young, and Gladius (Figure 1A). Interaction of drought with high temperature further reduced per plant yield in Odessa, Mendos, and Young. In contrast, heat stress did not exacerbate the effect of drought on yield in Currawa, Frame, and Gladius. Per plant yield in Koda was not sensitive to either drought or D&H stress. Overall, the combination of drought and high temperature was more detrimental to per plant yield in some genotypes, but not all (Figure 1A). In order to assess the impact of drought and combined D&H on grain filling, grain dry weight in the main spike was measured at 12 days after anthesis (DAA) and at harvest. At 12 DAA, total grain weight in the main spike was different among genotypes (Figure 1B) but there was no effect of the treatments or genotype × treatment interaction (Table 1). At harvest, total grain weight in the main spike was reduced by drought in Gladius, and reduced by combined D&H in Odessa, Koda, Mendos, and Frame (Figure 1C). There was no effect of drought or combined D&H on the main spike total grain weight at harvest in Currawa, Synthetic W7984 and Young.
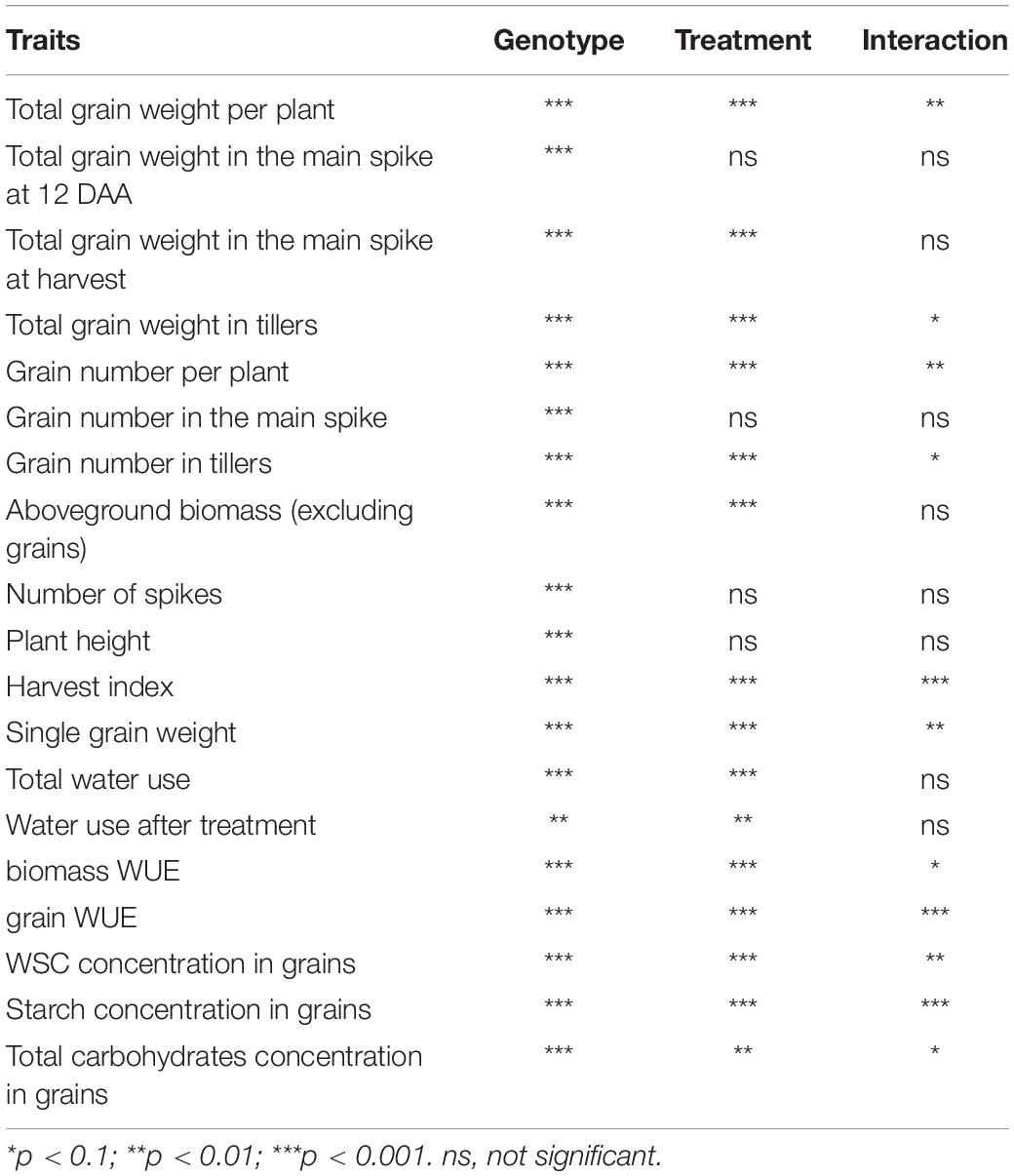
Table 1. Analysis of variance (ANOVA) showing the statistical significance of the traits measured for genotype, treatment and interaction between genotype and treatment.
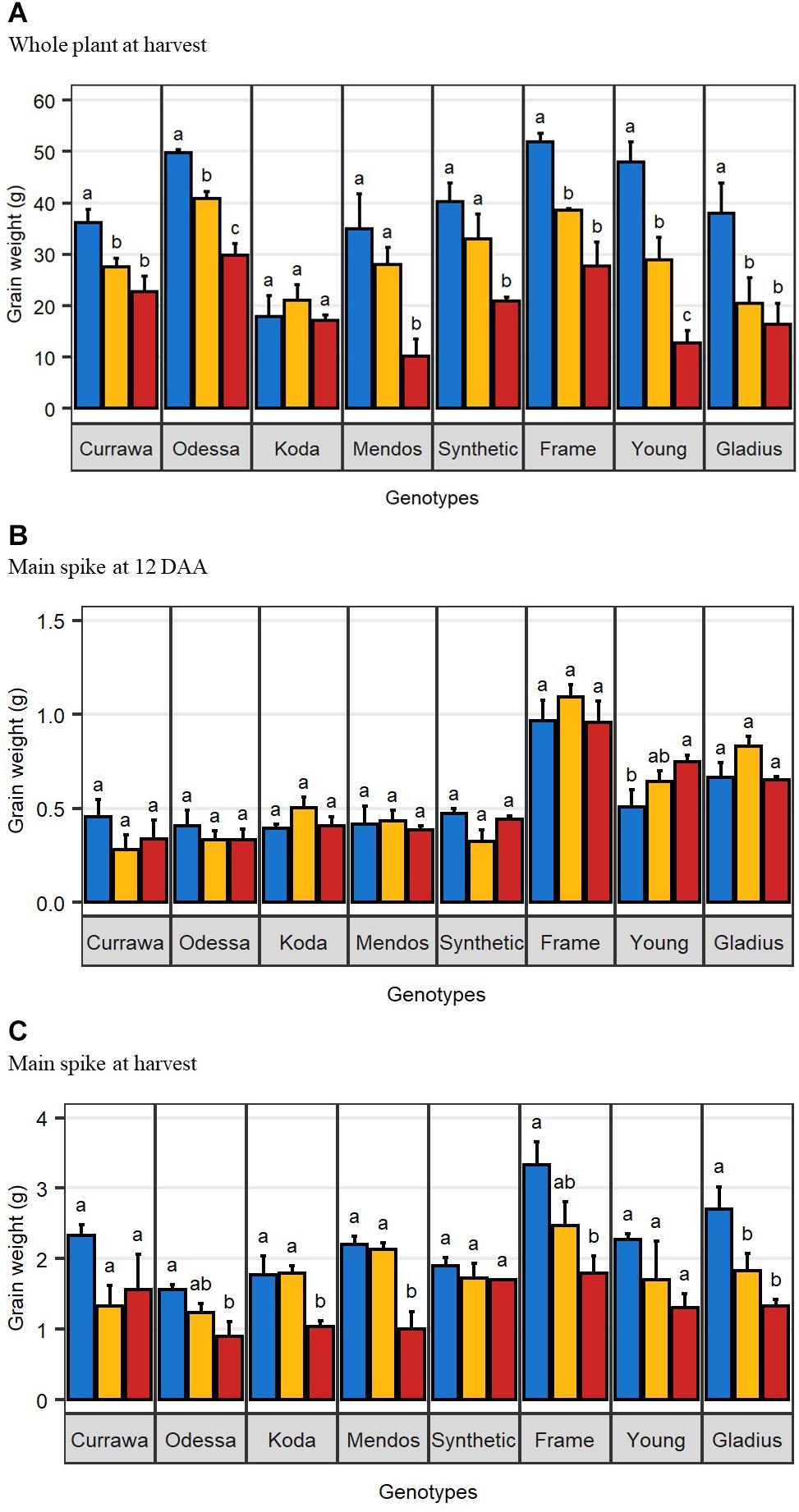
Figure 1. Combined drought and heat stress differentially reduced final grain weight. (A) Mean total grain weight per plant at harvest (n = 4). (B) Mean total grain weight in the main spike at 12 days after anthesis (n = 3). (C) Mean total grain weight in the main spike at harvest (n = 4). Error bars are standard error. Letters indicate the results of Tukey’s test comparing treatment effect within each genotype (p < 0.1). Plants were grown under well-watered conditions (blue), drought (orange), or combined drought and heat (red).
The Correlation Between Aboveground Biomass, Water Use and Plant Yield Increased With Increasing Stress Intensity
There was a significant interaction between genotype and treatment for both harvest index (grain weight/biomass) and water productivity (grain weight/water used) (Table 1). Plant yield was associated with aboveground vegetative biomass and total water use to different degrees depending on treatments (Figure 2). Total grain weight was linearly related to aboveground biomass and total water used by the plant throughout the experiment; these coefficients increased with stress intensity from r2 = 0.2 and r2 = 0.31 in well-watered conditions (WW), to r2 = 0.35 and r2 = 0.56 under drought, and r2 = 0.46 and r2 = 0.67 under combined D&H, respectively (Figure 2C). This dependence of plant yield on total water use and interaction with treatment was confirmed in a repeated experiment (Supplementary Figure S3). During the 3 days heat treatment, plants generally used similar amounts of water as compared to well-watered conditions (Figure 3), although soil water potential was halved. When comparing modern Australian genotypes (Frame, Young, and Gladius) to older genotypes (Currawa, Odessa, Koda, Mendos, and Synthetic W7984), the relationship between per plant yield and aboveground biomass was similar in both groups (r2 = 0.48 and r2 = 0.46, p < 0.001, respectively). Despite this, the slope of the regression for modern varieties, i.e., harvest index, was higher compared to older genotypes (a = 0.8 and a = 0.3, respectively, Figure 2A). The higher slope for modern genotypes was explained by the lower biomass required to produce similar grain weight compared to older genotypes under well-watered conditions and reflects the high harvest index of modern genotypes in favorable conditions. The dependence of plant yield on total water used was higher in modern genotypes compared to older genotypes (r2 = 0.80 and r2 = 0.63, p < 0.001, respectively).
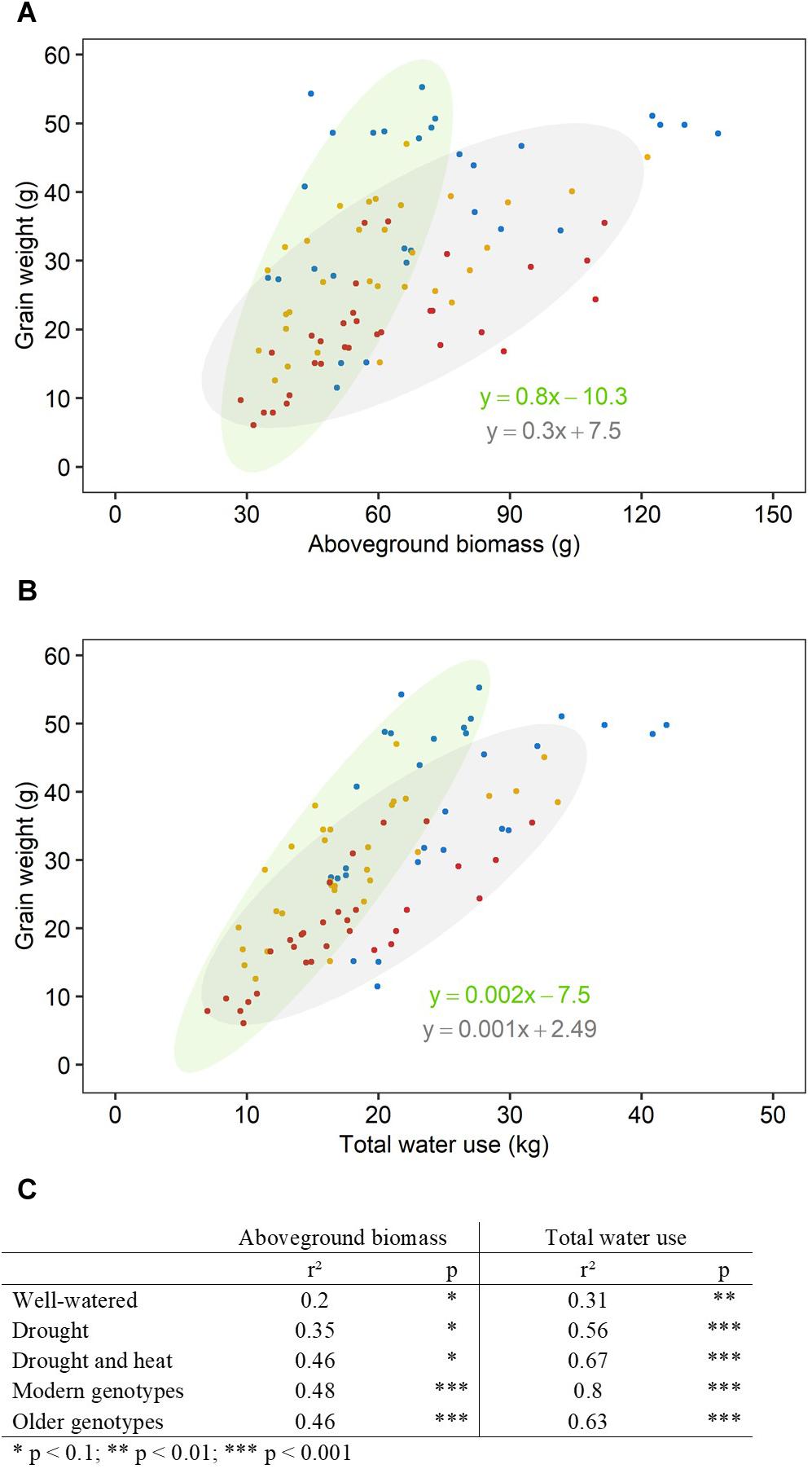
Figure 2. Aboveground biomass and water use explained more of the variation in grain weight under increasing stress intensity than in well-watered conditions. Relationships between final grain weight per plant and (A) final aboveground biomass excluding grains, (B) total water use. Each point represents one plant, grown under well-watered conditions (blue), drought (orange) or combined drought and heat stress (red). Ellipses circle modern genotypes (in green) and older genotypes (in gray). Linear regression equations correspond to linear regression for modern genotypes (in green) and older genotypes (in gray). (C) Table shows r2 and p-value of linear regressions (*p < 0.1, **p < 0.01, ***p < 0.001) for each treatment (well-watered, drought, combined drought, and heat stress) and genotype group (modern, older).
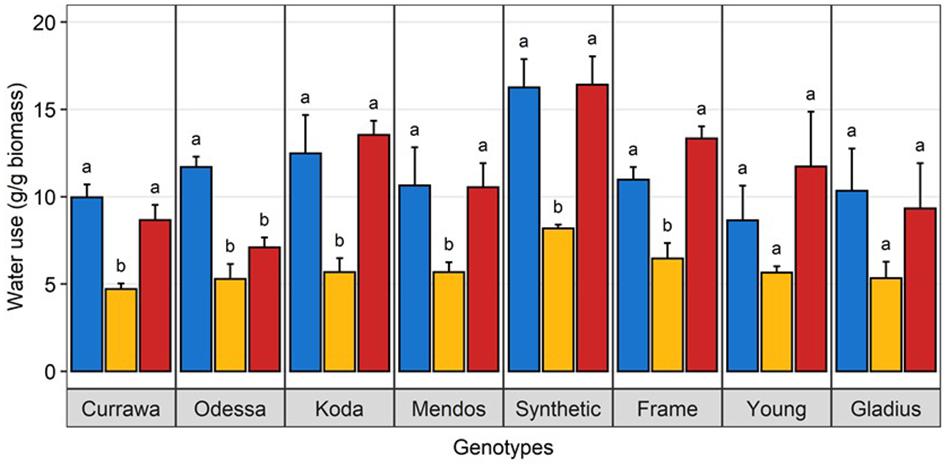
Figure 3. Water use under heat stress was similar to well-watered plants. Water use per plant during the 3-day heat treatment, normalized to aboveground biomass. Plants grown in well-watered conditions (blue), drought (orange) or combined drought and heat stress (blue). Values are means and standard error of four replicates (n = 4). Data were subjected to two-way ANOVA. Letters indicate the results of Tukey’s test comparing treatment effect within each genotype (p < 0.1).
Although in modern genotypes, spikes were longer and grain number was higher compared to older genotypes in all treatments, single grain weight was higher in older genotypes (Figure 4A). The difference in HI between modern and older types was reduced by the drought treatment and disappeared in the combined drought and heat treatment (Figure 4B). This was due to a greater reduction in single grain weight from developing tillers, post-anthesis of the main spike, in more modern types that were more sensitive to reduction in biomass and particularly water use (Figure 2C). There was little genotypic difference in biomass water-use efficiency (bWUE, Figure 4C) as most of biomass was produced before treatment in WW conditions and water use was highly dependent on biomass (Supplementary Figure S4). bWUE averaged 4.4 mg/g water in WW conditions, 5.1 mg/g water under drought and 4.7 mg/g water under combined D&H. In contrast, there was high genotypic variation in grain water-use efficiency (gWUE) under WW conditions, modern genotypes having the highest gWUE (Figure 4D). The difference in gWUE was reduced by the drought treatment and disappeared in the combined drought and heat treatment.
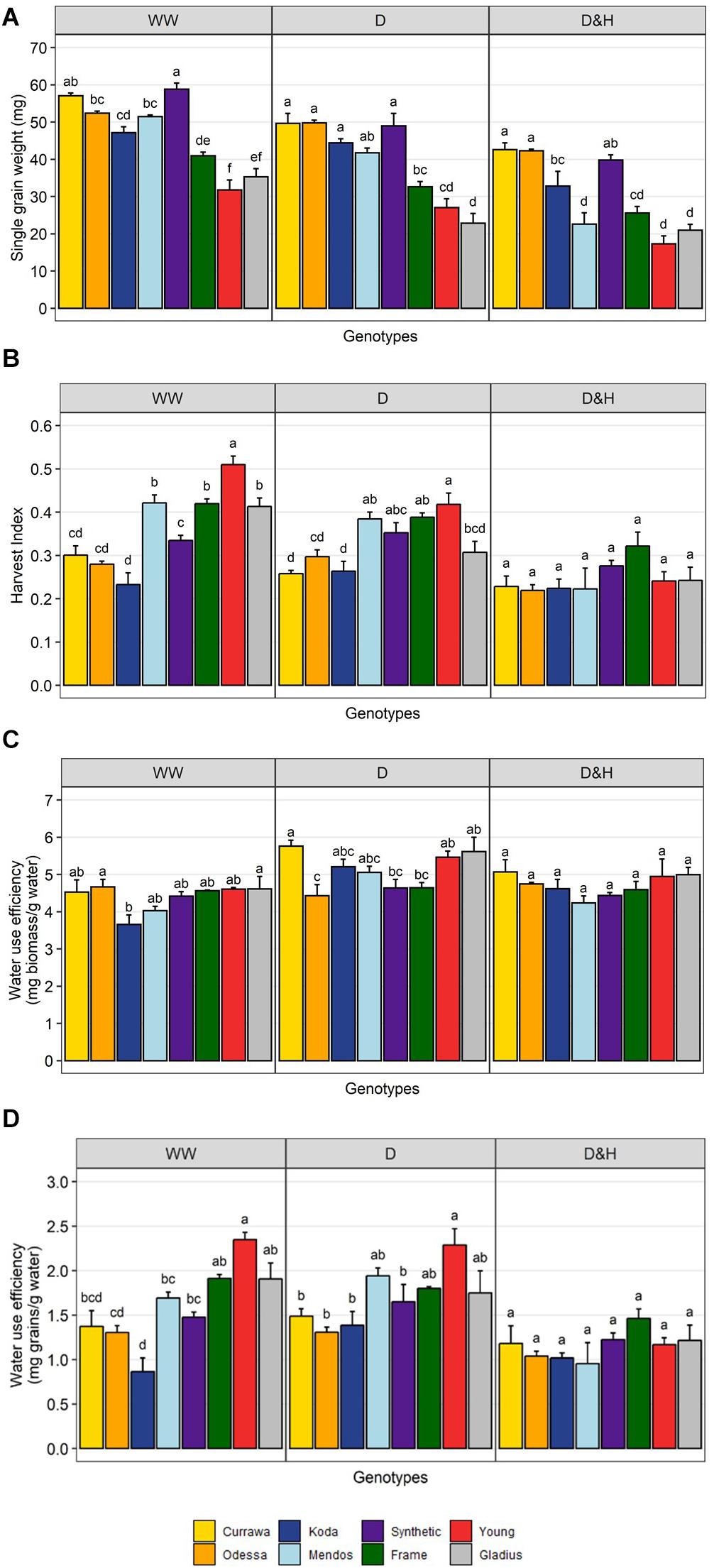
Figure 4. Reduction in yield per plant was due to reduction in grain weight post-anthesis. Genotypic differences in single grain weight (A), harvest index (B), biomass water-use efficiency (C), and grain water-use efficiency (D). Plants grown in well-watered conditions (WW), drought (D), or combined drought and heat stress (D&H). Values are means and standard error of four replicates (n = 4). Values represent average per plant. Data were subjected to two-way ANOVA. Letters indicate the results of Tukey’s test comparing genotype effect within each treatment (p < 0.1).
Combined Drought and Heat Stress Differentially Reduced Transpiration Response to Vapor Pressure Deficit
As total water use was strongly dependent on plant biomass, water use was normalized to the final aboveground biomass and expressed as unit of water per unit of biomass to allow for comparison between plants. Water use differed between genotypes following combined D&H when all treated plants (D and D&H replicates) were in the same droughted conditions (Figure 5A). Interaction of drought and 3 days high temperature reduced daily water use in Odessa, Koda, Mendos, and Young for the subsequent 30 days, whereas daily water use following combined D&H was similar to D alone in Currawa, Frame, Synthetic W7984, and Gladius. Plant water use is the summation of transpiration, which is driven by changes in vapor pressure deficit (VPD). Transpiration response to VPD was differentially altered by the 3 day high temperature treatment depending on genotype over the same grain-filling period when D and D&H plants were in the same droughted conditions (Figure 5B). For both transpiration rate (TR) and specific transpiration rate (STR), statistical significance was confirmed (p < 0.05) for the genotype × treatment interaction component of the VPD slope parameter. That is, the VPD effect on transpiration exhibited genotype × treatment interaction both before and after normalization to final aboveground biomass. Heat stress reduced subsequent transpiration rate at VPD > 0.5 kPa in Odessa, at VPD > 0.7 kPa in Young and at VPD > 1.0 kPa in Koda and Mendos (Figure 5B). In contrast, transpiration response to VPD was not altered following combined D&H stress in Currawa, Frame, Synthetic W7984, and Gladius. Transpiration response to VPD was affected in the same genotypes where daily water use was reduced by combined D&H. During drought, Young had the highest transpiration rate at VPD > 1.5 kPa whereas Currawa and Frame had the lowest transpiration rates. Following combined D&H, Synthetic W7984 had the highest transpiration rate at VPD > 1.5 kPa whereas Currawa and Odessa had the lowest transpiration rates (Supplementary Figure S5B).
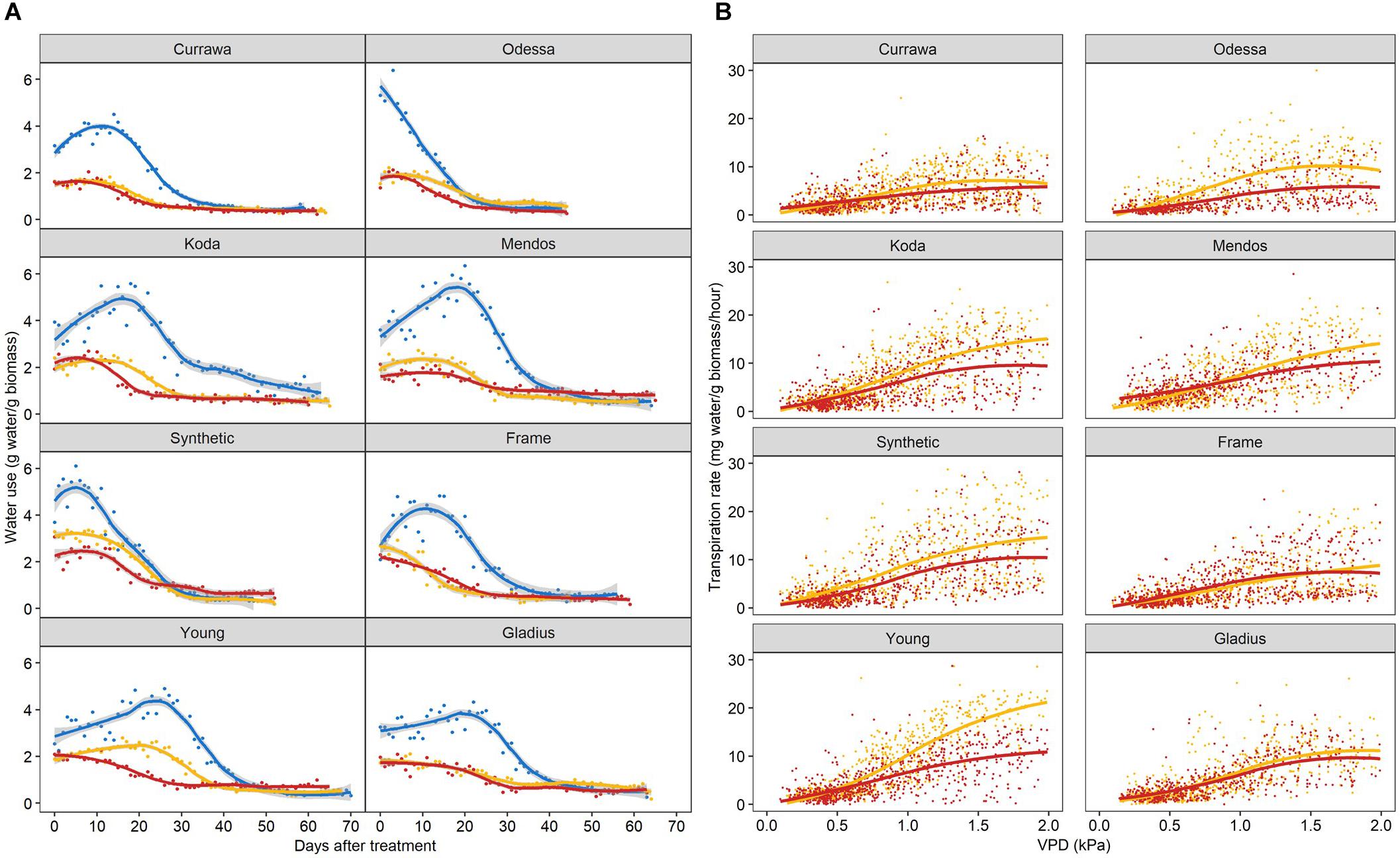
Figure 5. Interaction of high temperature and drought differentially reduced daily water use and transpiration response to vapor pressure deficit. (A) Daily water use per plant estimated as total irrigation per day, normalized to aboveground biomass. Plants grown in well-watered conditions (blue), drought (orange), or in drought following 3-day heat stress (red). 0 DAT is the first day post-heat treatment (12 days after anthesis). Trend lines are loess regressions. Values are means of four replicates (n = 4). The confidence interval (0.95) is displayed around smoothed regressions in gray. (B) Hourly transpiration rate response to VPD normalized to aboveground biomass. Plants grown in drought (orange) or in drought following 3-day heat stress (red). Graphs include data from 0 to 30 DAT. Trend lines are smooth regression lines.
Drought and Combined Drought and Heat Stress Altered WSC and Starch Balance in Grains
In order to quantify WSC availability for starch synthesis, WSC and starch concentrations were measured in grains 12 DAA, immediately following D&H treatments. There was a significant interaction between genotype and treatment for WSC and starch concentrations in grains (Table 1). Drought significantly reduced WSC concentration in grains in the Synthetic type and heat stress did not exacerbate the effect of drought (Figure 6). WSC concentration in grains was reduced by combined D&H in Odessa and Koda compared to WW and was specifically reduced by combined D&H in Currawa, Young, and Gladius. Starch concentrations offset the reduction in WSC concentration in Currawa, Odessa and Synthetic W7984, resulting in a similar total non-structural carbohydrates (NSC) concentration in grains in all conditions. The balance between WSC and starch concentrations was altered in Koda, Young, and Gladius (Figure 6). Total NSC concentration was reduced under drought in Koda and Young, and combined D&H reduced total NSC in Gladius. There was no significant effect of drought and combined D&H on total NSC concentration in grains in Currawa, Odessa, Mendos, and Frame. Overall, there was a significant interaction between genotypes and treatments for total carbohydrates concentration in grains (Table 1) that was mainly driven by interaction between genotypes and treatments for WSC concentration.
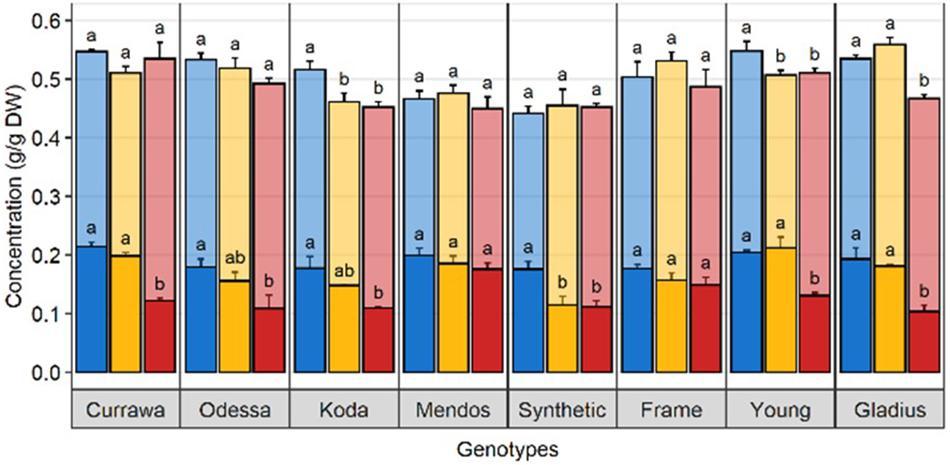
Figure 6. Drought and combined drought and heat stress altered WSC and starch balance in grains at 12 DAA. Water-soluble carbohydrates (solid) and starch (transparent) concentration in grains at 12 DAA from plants grown under well-watered conditions (blue), drought (orange), or combined drought and heat stress (red). The sum of WSC and starch concentrations constitutes the non-structural carbohydrate (NSC). Values are means of three replicates (± SE). Letters (top: NSC, bottom: WSC) indicate the results of Tukey’s test comparing treatment effects within each genotype (p < 0.1).
The Relationships Between WSC Concentrations and Plant Yield Depended on Plant Organ, Individual Carbohydrate and the Date of Release of the Variety
The relationship between WSC concentration at 12 DAA and plant yield depended on tissue and date of variety release. Total WSC concentration in grains at 12 DAA was positively related with plant yield in older genotypes (r2 = 0.41) whereas there was no relationship in modern genotypes (r2 = 0.13) (Table 2 and Figure 7A). Similarly, total WSC concentration in awns at 12 DAA was positively related with plant yield in the two, awned older genotypes (r2 = 0.85) whereas there was no relationship in modern genotypes (r2 = 0.06, Figure 7B). In contrast, total WSC concentration in the stem at 12 DAA was positively related with per plant yield in modern genotypes (r2 = 0.53) whereas there was no significant regression observed in older genotypes (r2 = 0.1) (Table 2 and Figure 7C). To determine whether individual WSC varied similarly to total WSC, we quantified glucose, fructose and sucrose concentrations in the stem, awns and grains. In grains, glucose and fructose concentrations were positively related with total grain weight at harvest (r2 = 0.43 and 0.40, respectively – Figure 7A). Sucrose concentrations at 12 DAA were low compared to glucose and fructose concentrations (Figure 7A). There was no relationship between starch concentrations at 12 DAA and plant yield (Figure 7A). In awns, glucose, fructose and sucrose concentrations at 12 DAA were each negatively related with plant yield in the two, awned older genotypes (r2 = 0.84, 0.81, and 0.67, respectively) but there were no significant relationships between these sugars and plant yield in more modern types (Table 2 and Figure 7B). In the stem, a similar contrast was observed for individual WSC between older and modern varieties as was observed for total WSC (Table 2 and Figure 7C). Glucose and sucrose concentrations in the stem at 12 DAA were positively related with plant yield in modern varieties (r2 = 0.34 and 0.56, respectively). Stem fructose concentrations at 12 DAA were negatively related to plant yield in older varieties (r2 = 0.31). Two (unknown) fructans also appeared important for plant yield in modern varieties: fructan 1 in awns (r2 = 0.47) and fructan 2 in the stem (r2 = 0.73). In contrast with other sugars in the awns, fructan 1 concentrations at 12 DAA and yield per plant were positively related.
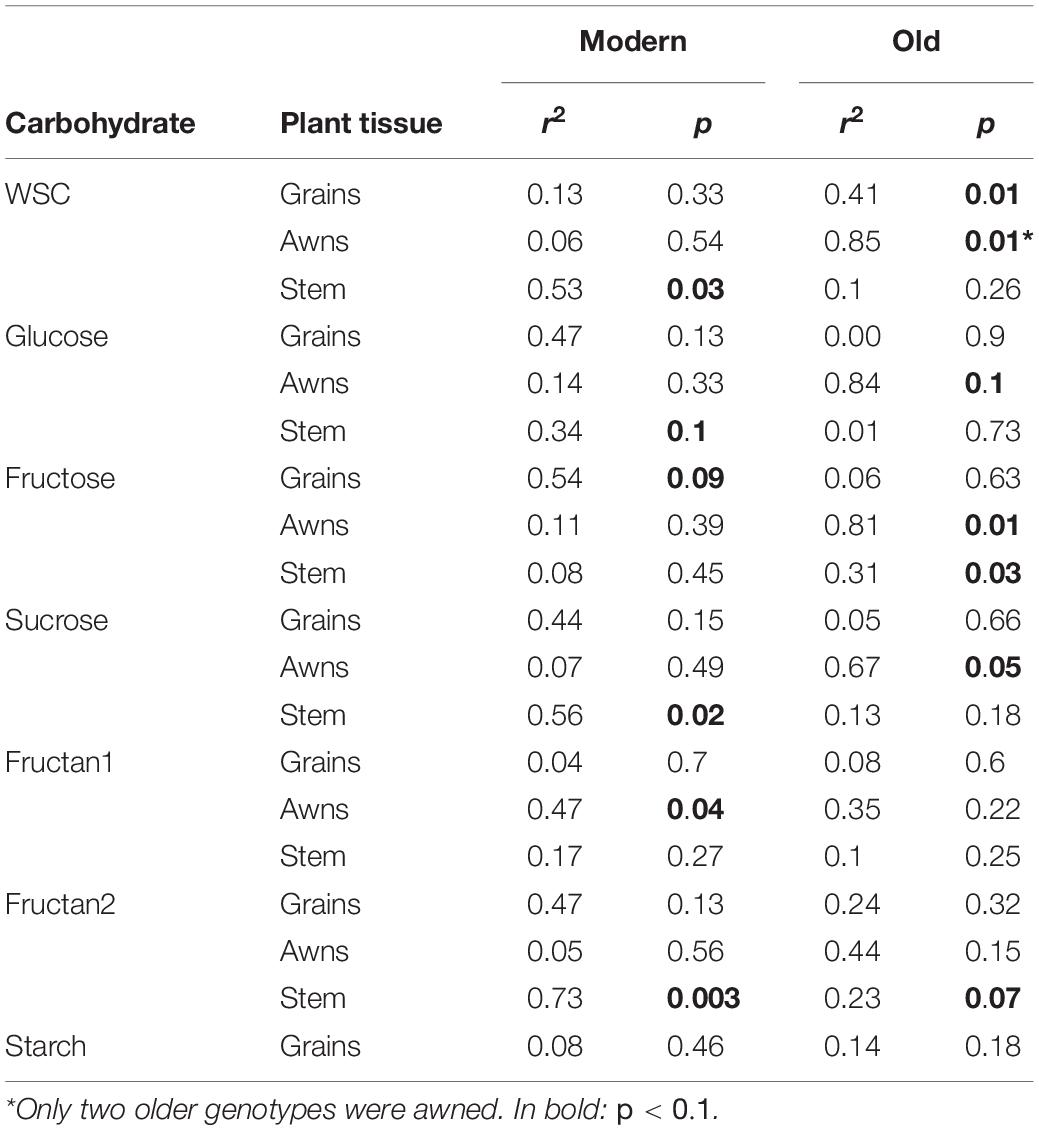
Table 2. r2 and p-values of linear regressions between individual carbohydrates’ concentrations in different plant organs at 12 DAA and final grain weight per plant, in modern or older genotypes.
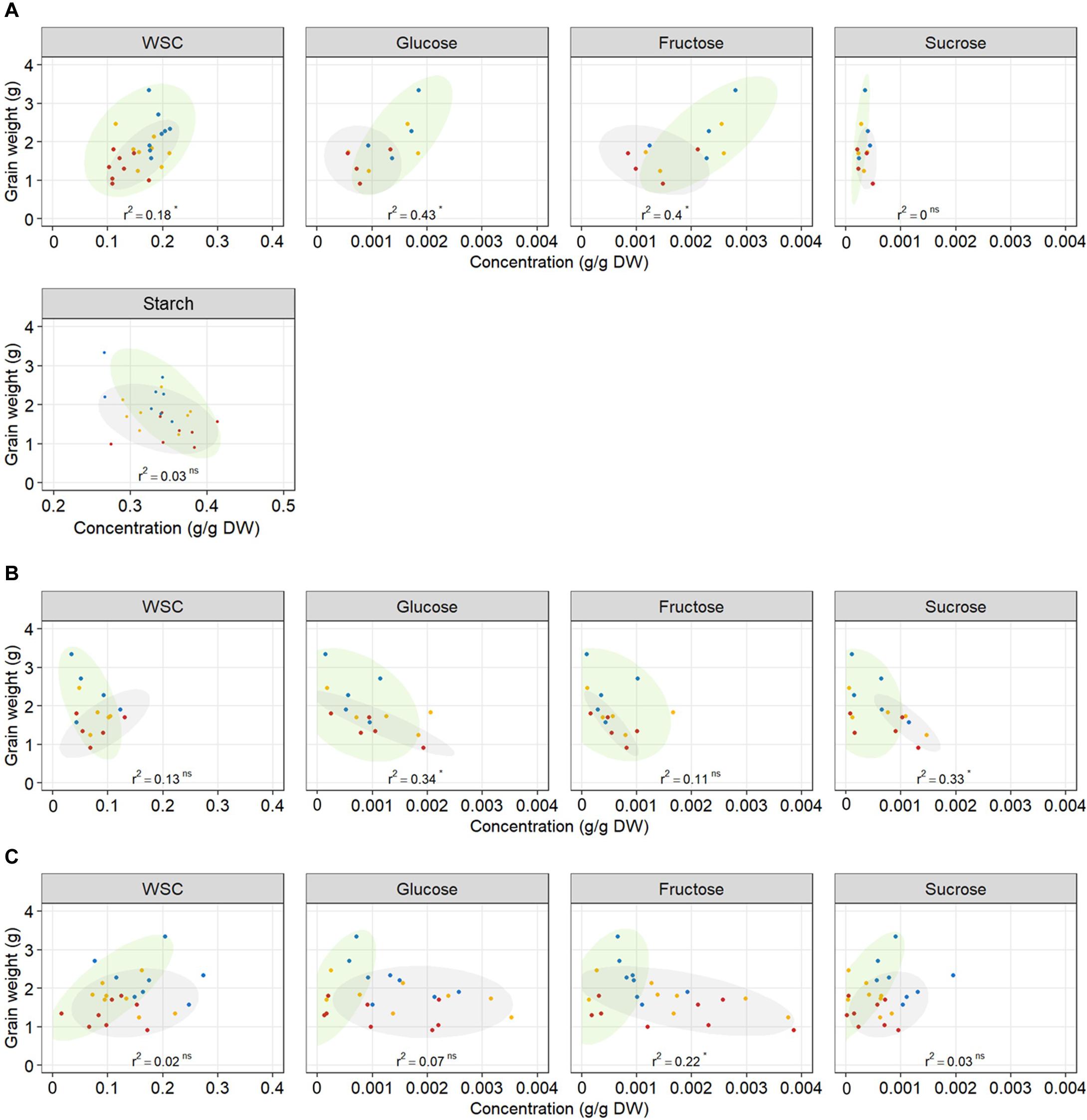
Figure 7. Relationship between total WSC, glucose, fructose, sucrose, and starch concentrations in (A) grains, (B) awns, (C) the stem at 12 DAA, and final grain weight per plant. Each point represents an average of carbohydrate concentration (n = 3) in the main tiller for one genotype and one treatment. Each regression line includes the whole dataset. r2 and p-value of linear regressions (‘ns’ not significant, *p < 0.05) are indicated. Ellipses circle old genotypes (gray) and modern genotypes (green). Plants grown under well-watered conditions (blue), drought (orange), or combined drought and heat stress (red).
Combined Drought and Heat Stress Increased WSC Partitioning to the Spike in Old Genotypes, but Not in Modern Varieties
Water-soluble carbohydrates were quantified in the main stem and the spike tissues following all treatments 12 DAA, i.e., immediately following the heat stress for the D&H replicates. There was a clear contrast between older genotypes (Currawa, Odessa, Koda, Mendos, Synthetic W7984) and more modern varieties (Frame, Young, Gladius) for WSC partitioning (defined as the percentage of the total WSC content) in stem parts compared with the main spike (Figure 8). In well-watered conditions, stem parts contained 67–87% of total WSC in older wheat genotypes compared to 28–50% in modern varieties. The spike tissues (excluding grains) contained 49–71% of total WSC in modern varieties, whereas WSC in the spike were 12–33% of total WSC in older genotypes. Drought and combined D&H differentially affected WSC distribution in the main stem and spike depending on genotype (Figure 8). Drought significantly increased WSC partitioning into the spike in Currawa and Odessa and reduced the WSC fraction in the stem in Frame (Supplementary Table S2). Combined D&H significantly increased WSC partitioning into the spike in older genotypes, whereas there was no change in WSC partitioning in modern varieties in both treatments. Changes in WSC allocation to the spike following combined drought and heat stress did not affect WSC partitioning to grains, except in the Synthetic genotype where WSC partitioning to grains was significantly increased by combined D&H compared to drought only.
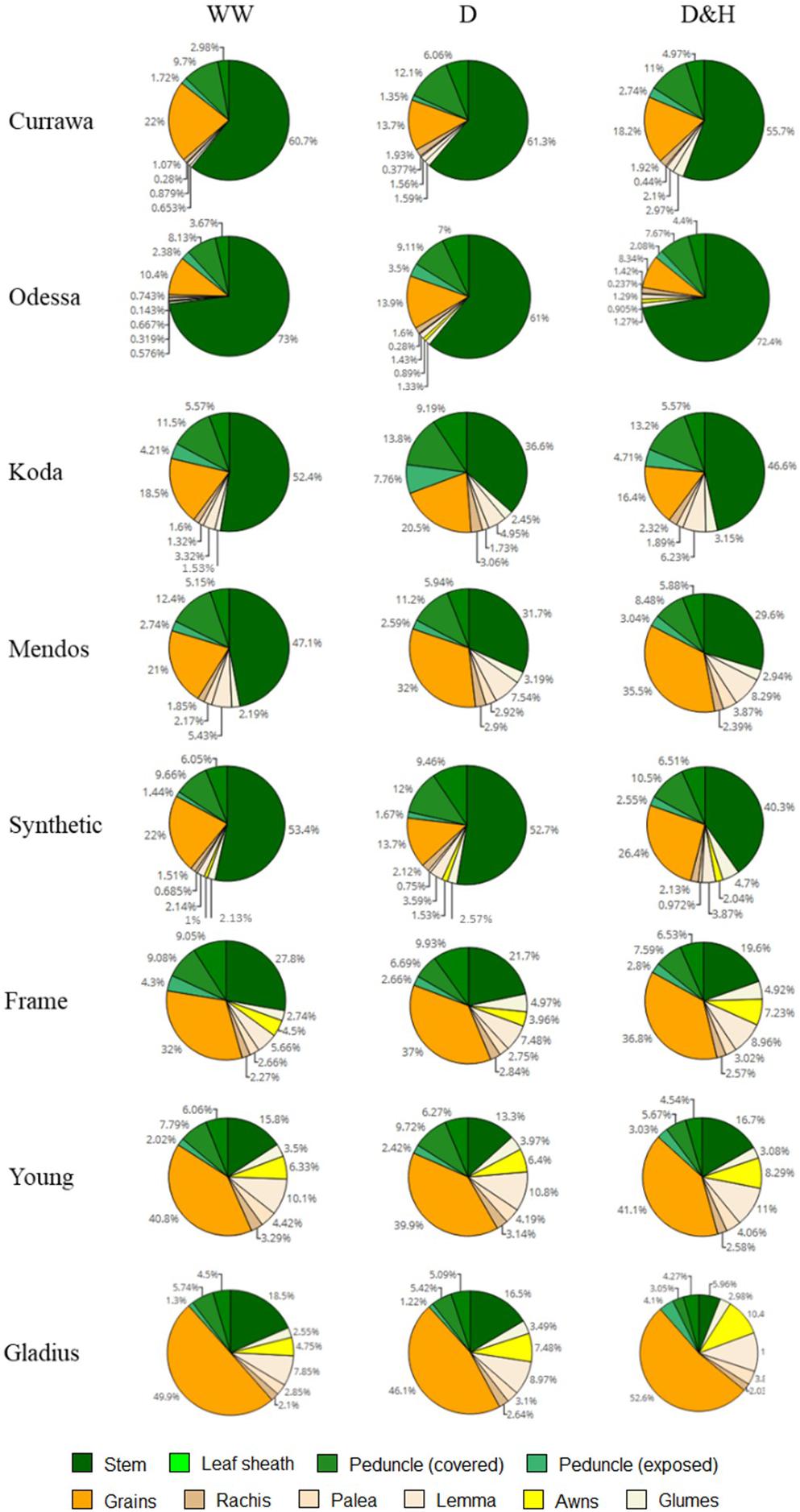
Figure 8. Combined drought and heat stress increased WSC partitioning to the spike in old genotypes, not in modern varieties. Genotypes are shown in order of date of release (top – bottom, oldest = Currawa to newest = Gladius). Total water-soluble carbohydrates (WSC) in different organs of plants as a percentage of total WSC (n = 3). Plant organs are color-coded as shown in the legend.
Discussion
Higher Water Use and Responsiveness to Evaporative Demand Are Indicators of Higher Yield Under Combined Drought and Heat Stress
In this study, the impact of drought and combined D&H on total grain weight at harvest was assessed in eight diverse wheat genotypes released between 1912 and 2007. The detrimental effect of heat stress combined with drought depended on genotype, illustrating genetic variation in grain weight response to combined D&H in wheat. Per plant yield was increasingly dependent on both aboveground biomass and total water use with increasing stress intensity (drought, then additional heat stress) (Figure 2), highlighting the important relationships between biomass, water use and grain weight under stress previously reported (Reynolds et al., 2006; Blum, 2009; Reynolds and Langridge, 2016). Biomass and water use are linearly related (de Wit, 1958) and mutually dependent during the plant’s lifecycle. During the vegetative stage, transpiration drives biomass accumulation, which in turn results in high water use during grain filling when water is available. The maintenance of water use ensures the favorable water status of plant tissues and assimilate transport to grains. We observed a high association between per plant yield and total water used in two independent experiments (Figure 2B and Supplementary Figure S3) regardless of transpiration sensitivity to heat stress: the higher the water use, the higher the total grain weight at harvest. This indicated that maintaining transpiration following heat stress was a desirable trait in our conditions, confirming the strong relationship between plant transpiration and yield (de Wit, 1958; Fischer and Turner, 1978; Sinclair et al., 2005). The amount of water used in transpiration is driven by the evaporative demand in the atmosphere (Grantz, 1990). Our work illustrated the genetic variation in transpiration response to VPD previously observed in diverse wheat genotypes grown under well-watered and water-limited conditions (Schoppach and Sadok, 2012; Schoppach et al., 2016; Medina et al., 2019). In addition, we identified genetic variation in transpiration response to combined D&H (Figure 5B). A 3-day heat treatment altered transpiration response to VPD in the subsequent drought-only treatment in some genotypes. As transpiration largely depends on green leaf area, the dynamics of senescence in response to heat stress could potentially have explained genotypic differences in transpiration response to heat stress; water use would be quickly reduced in genotypes with faster heat-induced senescence compared to genotypes with slower senescence rate following heat stress. However, no significant differences or genetic variation for drought and heat stress-induced chlorophyll content (greenness) in comparison with drought were found in these genotypes in repeated experiments (Schmidt et al., 2020). The combination of high evaporative demand and water scarcity can lead to the disruption of the water column in the xylem and cause cavitation. Cavitation damage might explain the lack of recovery in water use following heat stress observed in some genotypes in our experiments.
Wheat yield potential in water-limited environments is driven by water use, water use efficiency and harvest index (Passioura, 1977) with the theoretical possibility that, if genetic variation for each of these factors is independent from the others, yield may be increased by selection of favorable genotypes for any one factor (Reynolds and Tuberosa, 2008). The transpiration driven water use changes observed here following heat stress during grain-filling, when plants were all subjected to drought, influenced final grain weight. This trait – ability to maintain transpiration following heat stress under drought – separated more from less tolerant types. Tolerant genotypes may use more water by opening stomata at cooler times of the day or be more resistant to xylem cavitation, allowing continuous water flow through the plant and more favorable water status under stress.
In contrast, there was no clear genetic variation within the limited number of genotypes used in our experiments for either whole plant water-use efficiency or harvest index following the heat stress while under drought. There were interactions between treatments and genotypes for both biomass WUE and grain WUE, but no significant differences between genotypes once heat stress was applied. Despite the limited number of genotypes, in well-watered conditions we observed clear increased grain weight per unit of biomass, as well as per unit of water, in more modern compared with older genotypes reflecting the improved yield driven by the higher harvest index of modern types. Although harvest index was generally still higher in modern than in older genotypes under drought, this was not the case following heat stress which abolished harvest index difference between modern, semi-dwarf types compared with older, tall types. These results suggest that the responses of WUE and harvest index under combined drought and heat stress are independent from the water use response and that their contributions may be limited by the relative sensitivity of modern types to stress-induced decrease in biomass following heat stress.
WSC Availability in Grains Rather Than Grain Capacity Limited Grain Weight Under Stress
Starch is the main component of final grain mass. It is synthesized from stored WSC or produced from current photosynthesis. Drought and heat stress alter WSC supply to grains, either by limiting carbon assimilation through photosynthesis or by interrupting assimilate remobilization. Thus WSC availability in grains might be a limiting factor for starch synthesis and grain filling (Jurgens et al., 1978). In a field study on wheat genotypes grown in well-watered conditions, Fahy et al. (2018) quantified WSC and starch content and key starch biosynthesis enzyme activity in grains at different grain developmental stages. They did not find any correlation between carbohydrate content in grains and final grain yield, suggesting that assimilate availability for starch synthesis is not a limiting factor for grain filling in wheat in favorable growing conditions. These findings are in accordance with our results in well-watered conditions where there was no relationship between WSC and starch content and plant yield. However, WSC concentration was reduced with increased stress intensity, and grains with relatively higher WSC concentrations at 12 DAA had higher yield (Figure 7A). Glucose and fructose are the first substrates in the starch biosynthesis pathway (Emes et al., 2003). Genotypes with higher glucose and fructose concentrations in grains at 12 DAA had higher yield, implying that shortage in glucose and fructose might have limited starch biosynthesis later during grain filling and consequently final grain weight. Accelerated starch biosynthesis under stress depleted glucose and fructose in grains without any increase in sucrose content, indicating that insufficient sucrose supply to grains probably limited starch biosynthesis under stress. Many studies propose sink strength (grain capacity) is the limiting factor for starch accumulation and grain filling in favorable environments (Borrás et al., 2004; Borrill et al., 2015; Fahy et al., 2018). In our study, genotypes with higher grain capacity, represented by grain dry weight at 12 DAA, had a higher yield in the well-watered treatment (Figures 1A,B) suggesting that grain sink strength was a major determinant of grain weight at harvest when conditions were favorable. With D&H stress, however, genotypes with higher grain capacity did not have higher yield, suggesting that high grain capacity was not sufficient to determine grain weight at harvest under stress, as has also been observed in barley (Savin and Nicolas, 1996). In our experiments, drought and combined D&H did not immediately reduce grain weight at 12 DAA. Reduction in grain filling occurred after 12 DAA, which corresponds to the end of cell enlargement and beginning of carbohydrate accumulation (Emes et al., 2003). Reduced grain weight under stress was due to altered grain filling, probably as a consequence of limited WSC supply to grains.
The Spike Is the Main Storage Tissue for WSC in the More Modern Wheat Varieties
Excess assimilates that are not used for growth and defence may be stored for further use during reproductive stages. The stem is considered an important source of stored WSC for grain filling, and the ability to store and remobilize stem reserves is regarded as a beneficial trait for wheat productivity under stress (Bidinger et al., 1977; Blum, 1998; Rebetzke et al., 2008). At 12 DAA, WSC content in the stem is at its peak (Zhang et al., 2015; Shirdelmoghanloo et al., 2016). Our results showed that the stem was the main storage organ for WSC in tall genotypes in which the stem was the largest organ by weight, but not in more modern varieties where stems are much shorter as a consequence of the introduction of semi-dwarfing Rht genes (Borrell et al., 1993). More recent varieties partitioned more of the biomass to spikes, and the reproductive organ was also the major store of WSC in modern varieties (Figure 8). Interestingly, in our experiment, a positive relationship between WSC concentration in the stem and total grain weight in the main spike at harvest was only observed in modern genotypes, which suggests that the important contribution of WSC content stored in the stem to grain filling may be a consequence of the introduction of semi-dwarfing genes (Richards, 1992; Miralles et al., 1998). Alternatively, it might suggest that plant breeders have selected for varieties that partition more of their WSC to spike tissues in the hot and dry conditions of South Eastern Australia, the origin of the more modern varieties. Older genotypes had large reserves of WSC in the stem for a limited sink in the spike (lower grain number), which could explain the absence of a relationship between both traits as stored WSC in the stem may not have been used (Borrell et al., 1993). In contrast with older types, more modern varieties had relatively low WSC concentration in the stem, indicating an opportunity to increase stem capacity for WSC storage in modern varieties. Dreccer et al. (2014) demonstrated in a recombinant inbred line population that proportionately high stem WSC types were also proportionately high spike WSC types. They also showed that these WSC were associated with higher grain weight and recently fixed carbon under heat stress, i.e., high WSC types (stem and spike) remobilized proportionately more WSC. Recently, Liu et al. (2020) showed a gradient of WSC and remobilization efficiency along the stem toward the spike under drought stress, contributing to grain filling. Taken together, these results suggest that there could be capacity to increase stem WSC in future varieties that would be remobilized to increase stress tolerance.
Conclusion
Drought and heat stress have rarely been studied together, despite their co-occurrence being a common scenario in wheat-growing regions. This work illustrated the effect of morphological changes introduced in wheat over a century on plant water use and carbohydrates partitioning. Results showed that heat stress occurring during grain filling, while plants were suffering from water stress, changed subsequent water use immediately so that some genotypes were unable to recover. Sensitivity to increased stress intensity was associated with low transpiration response to high VPD following heat stress and to genetic variation in transpiration. Reduced availability of WSC in grains following combined D&H was also identified and important for final grain weight. This suggested that measurements of transpiration and WSC content in grains following heat stress might be used to identify genetic variation for tolerance of combined drought and heat stress.
Data Availability Statement
All datasets generated for this study are included in the article/Supplementary Material.
Author Contributions
AE, DF, TG, and PT conceived and designed the experiments. AE performed the experiments, analyzed and interpreted the data. NJ analyzed and interpreted data. All authors drafted the manuscript.
Funding
This work was supported by the Australian Research Council Industrial Transformation Research Hub for wheat in a hot and dry climate (IH130200027).
Conflict of Interest
The authors declare that the research was conducted in the absence of any commercial or financial relationships that could be construed as a potential conflict of interest.
Acknowledgments
We thank and acknowledge the use of the facilities and scientific and technical assistance of the Australian Plant Phenomics Facility, which is supported by the Australian Government’s National Collaborative Research Infrastructure Strategy (NCRIS). In particular, we thank Chris Brien of the APPF for his constructive comments and advise on analysis of these data. We thank Priyanka Kalambettu, Coline de l’Hommeau, and Pauline Perrodin for technical assistance. We acknowledge and thank Vincent Bulone, Helen Collins and Jelle Lahnstein for helpful comments and assistance with the carbohydrates analysis.
Supplementary Material
The Supplementary Material for this article can be found online at: https://www.frontiersin.org/articles/10.3389/fpls.2020.568693/full#supplementary-material
References
Asana, R., and Williams, R. (1965). The effect of temperature stress on grain development in wheat. Austr. J. Agric. Res. 16, 1–13. doi: 10.1071/AR9650001
Bidinger, F., Musgrave, R. B., and Fischer, R. A. (1977). Contribution of stored pre-anthesis assimilate to grain yield in wheat and barley. Nature 270, 431–433. doi: 10.1038/270431a0
Blum, A. (1998). Improving wheat grain filling under stress by stem reserve mobilisation. Euphytica 100, 77–83. doi: 10.1023/a:1018303922482
Blum, A. (2006). “Drought adaptation in cereal crops: a prologue,” in Drought Adaptation in Cereals, ed. J. M. Ribaut (Binghamton: Food Products Press), 21–42.
Blum, A. (2009). Effective use of water (EUW) and not water-use efficiency (WUE) is the target of crop yield improvement under drought stress. Field Crops Res. 112, 119–123. doi: 10.1016/j.fcr.2009.03.009
Blum, A. (2017). Osmotic adjustment is a prime drought stress adaptive engine in support of plant production. Plant Cell Environ. 40, 4–10. doi: 10.1111/pce.12800
Borrás, L., Slafer, G. A., and Otegui, M. A. E. (2004). Seed dry weight response to source-sink manipulations in wheat, maize and soybean: a quantitative reappraisal. Field Crops Res. 86, 131–146. doi: 10.1016/j.fcr.2003.08.002
Borrell, A. K., Incoll, L. D., and Dalling, M. J. (1993). The influence of the Rht1 and Rht2 alleles on the deposition and use of stem reserves in wheat. Ann. Bot. 71, 317–326. doi: 10.1006/anbo.1993.1041
Borrell, A. K., Incoll, L. D., Simpson, R. J., and Dalling, M. J. (1989). Partitioning of dry matter and the deposition and use of stem reserves in a semi-dwarf wheat crop. Ann. Bot. 63, 527–539. doi: 10.1093/oxfordjournals.aob.a087778
Borrill, P., Fahy, B., Smith, A. M., and Uauy, C. (2015). Wheat grain filling is limited by grain filling capacity rather than the duration of flag leaf photosynthesis: a case study using NAM RNAi plants. PLoS One 10:e0134947. doi: 10.1371/journal.pone.0134947
Butler, D. G., Cullis, B. R., Gilmour, A. R., Gogel, B. G., and Thompson, R. (2017). ASReml-R Reference Manual Version 4. Hemel Hempstead: VSN International Ltd.
Butler, D. G., Cullis, B. R., Gilmour, A. R., and Gogel, B. J. (2009). ASReml-R Reference Manual. Brisbane: DPI Publications.
de Wit, C. T. (1958). Transpiration and Crop Yields: Agricultural Research Reports 64.6. Wageningen: PUDOC.
Dreccer, M. F., Wockner, K. B., Palta, J. A., McIntyre, C. L., Borgognone, M. G., Bourgault, M., et al. (2014). More fertile florets and grains per spike can be achieved at higher temperature in wheat lines with high spike biomass and sugar content at booting. Funct. Plant Biol. 41, 482–495. doi: 10.1071/FP13232
Emes, M. J., Bowsher, C. G., Hedley, C., Burrell, M. M., Scrase-Field, E. S. F., and Tetlow, I. J. (2003). Starch synthesis and carbon partitioning in developing endosperm. J. Exper. Bot. 54, 569–575. doi: 10.1093/jxb/erg089
Evans, L. T., Bingham, J., Jackson, P., and Sutherland, J. (1972). Effect of awns and drought on the supply of photosynthate and its distribution within wheat ears. Ann. Appl. Biol. 70, 67–76. doi: 10.1111/j.1744-7348.1972.tb04689.x
Fahy, B., Siddiqui, H., David, L. C., Powers, S. J., Borrill, P., Uauy, C., et al. (2018). Final grain weight is not limited by the activity of key starch-synthesising enzymes during grain filling in wheat. J. Exper. Bot. 69, 5461–5475. doi: 10.1093/jxb/ery314
Fischer, R. A., and Turner, N. C. (1978). Plant productivity in the arid and semiarid zones. Annu. Rev. Plant Physiol. 29, 277–317. doi: 10.1146/annurev.pp.29.060178.001425
Garcia, M., Eckermann, P., Haefele, S., Satija, S., Sznajder, B., Timmins, A., et al. (2019). Genome-wide association mapping of grain yield in a diverse collection of spring wheat (Triticum aestivum L.) evaluated in southern Australia. PLoS One 14:e0211730. doi: 10.1371/journal.pone.0211730
Grantz, D. A. (1990). Plant response to atmospheric humidity. Plant Cell Environ. 13, 667–679. doi: 10.1111/j.1365-3040.1990.tb01082.x
Hölttä, T., Mencuccini, M., and Nikinmaa, E. (2009). Linking phloem function to structure: analysis with a coupled xylem-phloem transport model. J. Theoret. Biol. 259, 325–337. doi: 10.1016/j.jtbi.2009.03.039
Jurgens, S. K., Johnson, R. R., and Boyer, J. S. (1978). Dry matter production and translocation in maize subjected to drought during grain fill. Agron. J. 70, 678–682. doi: 10.2134/agronj1978.00021962007000040036x
Liu, Y., Zhang, P., Li, M., Chang, L., Cheng, H., Chai, S., et al. (2020). Dynamic responses of accumulation and remobilization of water soluble carbohydrates in wheat stem to drought stress. Plant Physiol. Biochem. 155, 262–270. doi: 10.1016/j.plaphy.2020.07.024
Lopes, M. S., El-Basyoni, I., Baenziger, P. S., Singh, S., Royo, C., Ozbek, K., et al. (2015). Exploiting genetic diversity from landraces in wheat breeding for adaptation to climate change. J. Exper. Bot. 66, 3477–3486. doi: 10.1093/jxb/erv122
Machado, S., and Paulsen, G. M. (2001). Combined effects of drought and high temperature on water relations of wheat and sorghum. Plant Soil 233, 179–187. doi: 10.1023/a:1010346601643
Medina, S., Vicente, R., Nieto-Taladriz, M. T., Aparicio, N., Chairi, F., Vergara-Diaz, O., et al. (2019). The plant-transpiration response to vapor pressure deficit (VPD) in durum wheat is associated with differential yield performance and specific expression of genes involved in primary metabolism and water transport. Front. Plant Sci. 9:1994. doi: 10.3389/fpls.2018.01994
Miralles, D. J., Katz, S. D., Colloca, A., and Slafer, G. A. (1998). Floret development in near isogenic wheat lines differing in plant height. Field Crops Res. 59, 21–30. doi: 10.1016/S0378-4290(98)00103-8
Mittler, R. (2006). Abiotic stress, the field environment and stress combination. Trends Plant Sci. 11, 15–19. doi: 10.1016/j.tplants.2005.11.002
Passioura, J. B. (1977). Grain yield, harvest index and water use of wheat. J. Austr. Instit. Agric. Sci. 43, 117–120.
Pradhan, G. P., Prasad, P. V. V., Fritz, A. K., Kirkham, M. B., and Gill, B. S. (2012). Effects of drought and high temperature stress on synthetic hexaploid wheat. Funct. Plant Biol. 39, 190–198. doi: 10.1071/FP11245
R Core Team (2019). R: A Language and Environment for Statistical Computing. Vienna: R Foundation for Statistical Computing.
Rebetzke, G. J., Bonnett, D. G., and Reynolds, M. P. (2016). Awns reduce grain number to increase grain size and harvestable yield in irrigated and rainfed spring wheat. J. Exper. Bot. 67, 2573–2586. doi: 10.1093/jxb/erw081
Rebetzke, G. J., Van Herwaarden, A. F., Jenkins, C., Weiss, M., Lewis, D., Ruuska, S., et al. (2008). Quantitative trait loci for water-soluble carbohydrates and associations with agronomic traits in wheat. Austr. J. Agric. Res. 59, 891–905. doi: 10.1071/AR08067
Reynolds, M., Dreccer, F., and Trethowan, R. (2006). Drought-adaptive traits derived from wheat wild relatives and landraces. J. Exper. Bot. 58, 177–186. doi: 10.1093/jxb/erl250
Reynolds, M., and Langridge, P. (2016). Physiological breeding. Curr. Opin. Plant Biol. 31, 162–171. doi: 10.1016/j.pbi.2016.04.005
Reynolds, M., and Tuberosa, R. (2008). Translational research impacting on crop productivity in drought-prone environments. Curr. Opin. Plant Biol. 11, 171–179. doi: 10.1016/j.pbi.2008.02.005
Richards, R. (1992). The effect of dwarfing genes in spring wheat in dry environments. I. Agronomic characteristics. Austr. J. Agric. Res. 43, 517–527. doi: 10.1071/AR9920517
Rosenzweig, C., Elliott, J., Deryng, D., Ruane, A. C., Müller, C., Arneth, A., et al. (2014). Assessing agricultural risks of climate change in the 21st century in a global gridded crop model intercomparison. Proc. Natl. Acad. Sci. 111, 3268–3273. doi: 10.1073/pnas.1222463110
Savin, R., and Nicolas, M. E. (1996). Effects of short periods of drought and high temperature on grain growth and starch accumulation of two malting barley cultivars. Funct. Plant Biol. 23, 201–210. doi: 10.1071/PP9960201
Schmidt, J., Tricker, P. J., Eckermann, P., Kalambettu, P., Garcia, M., and Fleury, D. (2020). Novel alleles for combined drought and heat stress tolerance in wheat. Front. Plant Sci. 10:1800. doi: 10.3389/fpls.2019.01800
Schnyder, H. (1993). The role of carbohydrate storage and redistribution in the source-sink relations of wheat and barley during grain filling — a review. New Phytol. 123, 233–245. doi: 10.1111/j.1469-8137.1993.tb03731.x
Schoppach, R., and Sadok, W. (2012). Differential sensitivities of transpiration to evaporative demand and soil water deficit among wheat elite cultivars indicate different strategies for drought tolerance. Environ. Exper. Bot. 84, 1–10. doi: 10.1016/j.envexpbot.2012.04.016
Schoppach, R., Taylor, J. D., Majerus, E., Claverie, E., Baumann, U., Suchecki, R., et al. (2016). High resolution mapping of traits related to whole-plant transpiration under increasing evaporative demand in wheat. J. Exper. Bot. 67, 2847–2860. doi: 10.1093/jxb/erw125
Sevanto, S. (2018). Drought impacts on phloem transport. Curr. Opin. Plant Biol. 43, 76–81. doi: 10.1016/j.pbi.2018.01.002
Shah, N. H., and Paulsen, G. M. (2003). Interaction of drought and high temperature on photosynthesis and grain-filling of wheat. Plant Soil 257, 219–226. doi: 10.1023/A:1026237816578
Shirdelmoghanloo, H., Cozzolino, D., Lohraseb, I., and Collins, N. C. (2016). Truncation of grain filling in wheat (Triticum aestivum) triggered by brief heat stress during early grain filling: association with senescence responses and reductions in stem reserves. Funct. Plant Biol. 43, 919–930. doi: 10.1071/FP15384
Sinclair, T. R., Hammer, G. L., and Van Oosterom, E. J. (2005). Potential yield and water-use efficiency benefits in sorghum from limited maximum transpiration rate. Funct. Plant Biol. 32, 945–952. doi: 10.1071/FP05047
Stone, B., and Morell, M. K. (2009). “Carbohydrates,” in Wheat, 4 Edn, eds K. Khan and P. R. Shewry (London: AACC International Press), 299–362. doi: 10.1016/B978-1-891127-55-7.50016-1
Van Dijk, A. I. J. M., Beck, H. E., Crosbie, R. S., De Jeu, R. A. M., Liu, Y. Y., Podger, G. M., et al. (2013). The millennium drought in southeast australia (2001-2009): natural and human causes and implications for water resources, ecosystems, economy, and society. Water Resour. Res. 49, 1040–1057. doi: 10.1002/wrcr.20123
Yemm, E. W., and Willis, A. J. (1954). The estimation of carbohydrates in plant extracts by anthrone. Biochem. J. 57, 508–514. doi: 10.1042/bj0570508
Keywords: crops, water use, carbohydrate partitioning, Triticum aestivum, drought and heat stress
Citation: El Habti A, Fleury D, Jewell N, Garnett T and Tricker PJ (2020) Tolerance of Combined Drought and Heat Stress Is Associated With Transpiration Maintenance and Water Soluble Carbohydrates in Wheat Grains. Front. Plant Sci. 11:568693. doi: 10.3389/fpls.2020.568693
Received: 01 June 2020; Accepted: 22 September 2020;
Published: 15 October 2020.
Edited by:
Raul Antonio Sperotto, Universidade do Vale do Taquari (Univates), BrazilReviewed by:
Dejan Bogdan Dejan, Maize Research Institute Zemun Polje, SerbiaAlejandro Del Pozo, University of Talca, Chile
Yin-Gang Hu, Northwest A&F University, China
Copyright © 2020 El Habti, Fleury, Jewell, Garnett and Tricker. This is an open-access article distributed under the terms of the Creative Commons Attribution License (CC BY). The use, distribution or reproduction in other forums is permitted, provided the original author(s) and the copyright owner(s) are credited and that the original publication in this journal is cited, in accordance with accepted academic practice. No use, distribution or reproduction is permitted which does not comply with these terms.
*Correspondence: Abdeljalil El Habti, abdeljalil.elhabti@adelaide.edu.au
†Present address: Trevor Garnett, Grains Research and Development Corporation, Dulwich, SA, Australia