- Plant Physiology and Ecology Laboratory, Department of Ecology, College of Life Sciences, Zhejiang University, Hangzhou, China
The limited availability of nitrogen (N) is a fundamental challenge for many crop plants. We have hypothesized that the relative crop photosynthetic rate (P) is exponentially constrained by certain plant-specific enzyme activities, such as ribulose-1,5-bisphosphate carboxylase/oxygenase (Rubisco), NADP-glyceraldehyde-3-phosphate dehydrogenase (NADP-G3PDH), 3-phosphoglyceric acid (PGA) kinase, and chloroplast fructose-1,6-bisphosphatase (cpFBPase), in Triticum aestivum and Oryza sativa. We conducted a literature search to compile information from previous studies on C3 and C4 crop plants, to examine the photosynthetic rate responses to limited leaf [N] levels. We found that in Zea mays, NADP-malic enzyme (NADP-ME), PEP carboxykinase (PCK), and Rubisco activities were positively correlated with P. A positive correlation was also observed between both phosphoenolpyruvate carboxylase (PEPC) and Rubisco activity with leaf [N] in Sorghum bicolor. Key enzyme activities responded differently to P in C3 and C4 plants, suggesting that other factors, such as leaf [N] and the stage of leaf growth, also limited specific enzyme activities. The relationships followed the best fitting exponential relationships between key enzymes and the P rate in both C3 and C4 plants. It was found that C4 species absorbed less leaf [N] but had higher [N] assimilation rates (Arate) and higher maximum photosynthesis rates (Pmax), i.e., they were able to utilize and invest more [N] to sustain higher carbon gains. All C3 species studied herein had higher [N] storage (Nstore) and higher absorption of [N], when compared with the C4 species. Nstore was the main [N] source used for maintaining photosynthetic capacity and leaf expansion. Of the nine C3 species assessed, rice had the greatest Pmax, thereby absorbing more leaf [N]. Elevated CO2 (eCO2) was also found to reduce the leaf [N] and Pmax in rice but enhanced the leaf [N] and N use efficiency of photosynthesis in maize. We concluded that eCO2 affects [N] allocation, which directly or indirectly affects Pmax. These results highlight the need to further study these physiological and biochemical processes, to better predict how crops will respond to eCO2 concentrations and limited [N].
Introduction
Insufficient levels of important chemical elements, such as nitrogen (N), can result in constraints on the metabolic fluxes required to produce enzymes in plants (Baudouin-Cornu et al., 2001). N resource allocation and its constraints specifically, have marked impacts on the assimilation rates of CO2 (Arate) (Von Caemmerer, 2000). The Michaelis-Menten equation (MME) (Michaelis and Menten, 1913) was derived to describe the relationship between metabolism and limiting resources (Carr et al., 1997; Aguiar-González et al., 2012), and it can also explain a plants’ ability to live and grow for long periods when its resources are limited. Generally, C3 and C4 photosynthesis represents a balancing act between the Calvin-Benson cycle enzymes and N resource allocation. This shows that crop plants optimally allocate their nutrients to obtain a “functional equilibrium” for fitness. Therefore, resource availability or the demands of metabolic scaling, depend on the capacity of the species (Glazier, 2018) and of the plant leaves to efficiently utilize their resources (P’yankov et al., 2001). The availability of limited resources and the demand of each species are scaled differently, creating variations in the leaf traits that govern leaf economy. The leaf economic traits that are related to the carbon (C) and N concentrations of the plant have a strong influence on the leaf photosynthetic traits, both among and within species (Hu et al., 2015). However, as greater growth rates require enhanced N levels, N can become the more limiting nutrient in soils of terrestrial ecosystems (Sardans and Peñuelas, 2015). Leaf traits such as N allocation and photosynthetic capacity, may differ significantly among various crop plants; hence, an improved understanding of the various scalings of leaf trait relationships would be valuable for the fields of ecology, plant biology, and crop science. For example, the various scalings of the leaf traits related to leaf functional traits, including the photosynthetic rate, N concentration, and CO2 concentration, are the main drivers of leaf trait variation. The study of leaf trait variations in different groups of plants has previously been the focus when trying to understand plant adaptations to limited N concentrations and low and elevated CO2 concentrations. Based on these ideas, we developed a novel enzyme-driven model (EDM) that hypothesizes that the photosynthetic rate has an exponential relationship with basic enzymes, and that the photosynthetic rate is dependent on effective N sources.
This investigation focused on the effects that the N content of leaves has on the photosynthetic rates of C3 and C4 plants. N is an essential plant nutrient in both agricultural and natural environments as every plant species requires it for growth (Evans, 1983; LeBauer and Treseder, 2008), and if it is limited, there may be negative consequences such as reduced crop yields (Xu et al., 2012). N significantly affects growth, because a large N investment is required for the assimilation of C (Evans, 1989; Hohmann-Marriott and Blankenship, 2011), and consequently, leaf N determines a plant’s growth potential. N is one of the main elements in the photosynthetic apparatus and understanding the relationship between photosynthesis and leaf N is critical for optimizing C production and identifying the mechanisms that regulate photosynthesis. Plants invest a huge amount of N into their photosynthetic machinery (Ghannoum et al., 2005), and so, leaf N has a positive correlation with photosynthesis (Paponov and Engels, 2003) and various N components in the allocation of leaf N (Uribelarrea et al., 2009). Evans (1989) revealed that the relationship between leaf N and photosynthetic capacity varied among the different types of plants. When integrating information on the anatomy with the mechanical properties, the nutrient and light availabilities were found to possibly scale leaf traits, meaning that they could alter the properties of the leaf morphology and structure (Onoda et al., 2008). The photosynthesis rates and N concentrations increase when moving from the shade to the sun (Niinemets et al., 2015), as light is important for the partitioning of N in photosynthesis (Ishimaru et al., 2001; Yamori et al., 2011); hence, light absorption influences the photosynthetic transport chain and further enhances yields and photosynthetic productivity (Ye et al., 2013). Therefore, both nutrient and light availabilities affect the activity of Rubisco and PEP carboxylase (PEPC) (Usuda, 1984; Meinzer and Saliendra, 1997). Various other leaf traits are also affected in N-limited conditions (Makino and Ueno, 2018). Consequently, N limitations affect the photosynthetic machinery (Evans, 1983), reduce chloroplast size (Laza et al., 1993; Bondada and Syvertsen, 2005; Kelly, 2018), and markedly influence plant growth and nutrient cycles (Osnas et al., 2018). Strong correlations were found between the limiting enzymes and leaf N content in relation to photosynthesis under low and high partial pressures of CO2 (Makino et al., 2003).
The rate of CO2 assimilation in relation to the N content is known as the photosynthetic nitrogen use efficiency (PNUE). Different molecular and physiological factors cause variations in the PNUE (Rotundo and Cipriotti, 2017), and as a result, there are large differences between plant species. Accordingly, C4 plants have a 50% greater photosynthetic rate than C3 plants with the same N concentration (Evans and von Caemmerer, 2000). Consequently, a higher NUE was found in the C4 pathway than in the C3 pathway (Kelly, 2018). The increased NUE of the C4 species compared with that in the C3 species shows that the availability of N had a positive role in their evolution (Vogan and Sage, 2011). Furthermore, C4 plants exhibit two times higher Rubisco activity, compared with C3 plants (Sage, 2002); hence, lower Rubisco concentrations may enhance the photosynthetic rates of C4 plants (Makino et al., 2003). Due to the reduction in photorespiration, C4 plants show higher photosynthesis rates (Sage and Pearcy, 1987). In addition, higher N uptake capacity has been correlated with photorespiration (Dellero et al., 2015; Busch et al., 2018), raising the question as to whether CO2 concentrations affect plant N uptake.
To investigate the responses of C3 and C4 crop plants to atmospheric CO2 (atmCO2), an in-depth study at the leaf level is required. However, a previous investigation on elevated CO2 (eCO2) showed positive physiological feedback responses in crops (Sakai et al., 2006). Furthermore, CO2 plays an integral role in plant photosynthesis, thereby affecting plant metabolism. An improved understanding of the plant responses when there is limited N to atmCO2 is required in order to predict future changes in their leaf photosynthetic properties (Osada et al., 2010), as well as their physiological and morphological changes (Ainsworth and Long, 2005), and photosynthetic capacities (Ghannoum et al., 2000). To understand the total N content and the N allocations to the photosynthetic machinery, which contribute to the diversity of various photosynthetic capacities, a great deal of research is required. The reduction in leaf N mostly aggravates photosynthetic acclimation to eCO2 (Halpern et al., 2019), while low N availability reduces the photosynthetic capacity by reducing the C assimilation proteins, as well as Rubisco (Cohen et al., 2019b). Finally, both low leaf N and eCO2 may have adverse impacts on the expression of Rubisco, which stimulates eCO2 (Cohen et al., 2019a). Global atmCO2 concentrations have been increasing, and the magnitude of these enhancements due to CO2 enrichment, varies with species and other limiting environmental conditions. Limited N availability may constrain the stimulation of plant growth by eCO2 (Bloom et al., 2014), which raises the question of how atmCO2 affects and constrains leaf N content and the response of photosynthesis in crops. To understand the responses of the crop photosynthetic rates to low and elevated [CO2], crop plants receiving N at the leaf level and the responses of their half-photosynthesis constants (Kp) to the effective limited N sources were questioned.
The Calvin-Benson cycle carries out C assimilation, which produces carbohydrates from atmCO2 using ATP and NADPH in photochemical reactions (Calvin, 1989; Benson, 2002). The Calvin cycle of C3 plants fixes the C in mesophyll cells, and the Rubisco enzyme further catalyzes it. In C4 plants there are two types of cells, known as mesophyll cells and bundle sheath (BS) cells, and they fix CO2 with phosphoenolpyruvate, catalyzed by PEPC, which has a higher affinity for CO2 than Rubisco. The photosynthetic rate changes during leaf development, which explains why such changes occur via the activities of the Calvin cycle enzymes. In general, the activities of the Rubisco enzyme decrease at a faster rate during leaf senescence (Nakano et al., 1995; Crafts-Brandner et al., 1998; Ishizuka et al., 2004). Like C3 plants, the main CO2 limitations occur due to Rubisco in C4 plants (Von Caemmerer et al., 1997). Increasing the amount of N enhances the PEPC activities relative to Rubisco (Sugiyama et al., 1984). The higher expression of Rubisco in N-limited plants shows that a reduction in Rubisco could reduce leaf N, due to the reallocation of the N to younger leaves in the N-limited plants (Nie et al., 1995). In addition, allocations of leaf N to the PEPC and PEPC to Rubisco were reduced under limited N conditions (Sage et al., 1987). Our EDM identified a relationship between photosynthesis and important plant enzymes, such as ribulose-1,5-bisphosphate carboxylase/oxygenase (Rubisco), NADP-glyceraldehyde-3-phosphate dehydrogenase (NADP-G3PDH), 3-phosphoglyceric acid (PGA) kinase, and chloroplast fructose-1,6-bisphosphatase (cpFBPase) in wheat and rice (C3 plants). While in C4 plants, NADP-malic enzyme (NADP-ME), PEP carboxykinase (PCK), Rubisco (Zea mays for C4 plant), and Rubisco and PEPC (Sorghum bicolor, C4 plant) were shown to be involved with photosynthesis. In this published literature study, we selected major C3 and C4 crop plants, and predicted that their photosynthetic rates would exponentially increase with the plant enzyme activities. In addition, we predict that the log-photosynthesis rates were dependent on the resource levels at the leaf level. We hypothesized that along with the key enzymes and resources, other factors (leaf trait variation, PNUE, low and high CO2, and N allocation) also played important roles in plant photosynthesis.
Materials and Methods
Data Sources
A search of the published literature from 1980 to 2018 was conducted to identify studies on the photosynthetic responses of important C3 and C4 crop plants to limited plant-specific enzyme activities, limited leaf N content, and partial pressures of [CO2]. We searched for the following six key terms alone using the ISI Web of Science and Google Scholar: “leaf N,” “specific enzymes,” “low partial pressure of [CO2],” “high partial pressure of [CO2],” “low and high partial pressure of [CO2],”, and “assimilation rate.” We then searched for the six key terms again using the ISI Web of Science and Google Scholar, but this time, each in combination with the following three terms individually: “C3 photosynthesis rates,” “C4 photosynthesis rates,” and “C3 and C4 photosynthesis rates.” This yielded 12 and 48 studies, respectively. The published articles identified with these search terms were then further screened using the following principles: 1) the study organisms were C3 or C4 crop species of interest; 2) the responses of photosynthesis in the C3 and C4 plants were measured; 3) the response variables under limited leaf N were reported; and 4) the responses of photosynthesis to the current and elevated partial pressures of the (CO2) were reported in figures and tables. As a result of this screening procedure, we ultimately selected 47 published articles for the analysis. Fifteen of the articles involved C4 crops: five on Sorghum bicolor (sorghum), and ten on Zea mays (maize). Fourteen of the articles involved C3 cereal crops: six for Triticum aestivum (wheat), seven for Oryza sativa (rice), and one for Hordeum vulgare (barley). Twelve of the articles involved C3 dicotyledonous crops: four for Glycine max (soybean), six for Helianthus annuus (sunflower), and two on Solanum tuberosum (potato). Finally, there were four publications relating to C3 trees: one for Citrus sinensis (Citrus orange), one for Malus domestica (apple), and two for Prunus persica (peach). Furthermore, 30 of the publications were related to photosynthesis, 40 to leaf N (leaf N content, 20 publications; N limitation, four publications; N response, four publications; N distribution, two publications; and N availability, 10 publications), three to enzyme activities, one to partial pressure of [CO2], and six to NUE.
All the data for our analysis were obtained from the figures and tables of the 47 papers by using the software GetData Graph Digitizer 2.22. For each dataset, we used one-way ANOVA and Tukey tests to assess each of the parameters (mentioned in figures, tables, and statistical analysis), using Origin 9.0 software (Data analysis and Graphing software). For all figures, the sources for the data (see Dataset_S1) and references presented are given in Figure legends and Dataset S1. We analyzed the relationships between the enzyme activities (µmol m-2 s-1) and the photosynthetic rates (µmol CO2 m-2 s-1) in the young leaves of various C3 (wheat and rice) and C4 (maize and sorghum) plants. The methods for quantifying the roles of the enzymes via the photosynthetic rates are explained in the subsection section “Model Background”; the literature and data utilized for this concept can also be extracted from Figure 1 and Table 1. We also tested the goodness of fit statistics (R2 and Akaike information criterion, AIC) of exponential and linear model for photosynthetic rate and various enzyme activities (see Supplementary Material for Supplementary Table 1). Additionally, we select data as a line-symbol for photosynthesis and leaf N content (0.05, 0.2, 0.4, or 0.6 g N) relationships in Sorghum bicolor (see Supplementary Material for Supplementary Figure 1); sources were obtained from Makino and Ueno (2018). The data analyzed to determine the relationship between the light-saturated logarithmic-photosynthetic rates (nmol m-2 s-1 CO2 nmol PAR-1) and the N content (g N m-2) in the leaves of the C3 and C4 plants can be found in Figure 2 and Dataset S1. Here, we used only the logarithmic-photosynthetic rates to study the effects of the leaf N content on the leaf photosynthetic capacity. The comparisons between the logarithmic-assimilation rates (µmol m-2 s-1) and the same amount of leaf N content per unit area (mmol m-2) in the C3 (rice) and C4 (maize) plants were also determined (Figure 3); the sources were obtained from Evans and von Caemmerer (2000). Ignoring P0 in Eq. 3 (a detailed explanation is given in “Model Background”) allowed for the responses of the logarithmic-assimilation rates at the leaf level to the same amount of leaf N content per unit area to be identified. A comparison between the C3 and C4 plants at 36 and 100 Pa, their logarithmic-photosynthesis rates (µmol CO2 m-2 s-1), and their leaf N content (mmol m-2) in the young leaves were obtained and digitized from the figures (Figure 4, Table 2), and the specific data sources are given in Dataset S1. Additionally, we further tested the line-graph analysis for positive correlation between photosynthesis rates and leaf N content by showing that N deficiency causes areduction in the photosynthetic rate and intercellular CO2 concentrations (Ci) (see Supplementary Figure 2 in Supplementary Material); the sources were obtained from Zhao et al. (2005). Furthermore, Eq. 3 also allowed for the identification of the plant responses at the leaf level to the eCO2 concentrations, which are essential for predicting the structural changes and biochemical dynamics in plants.
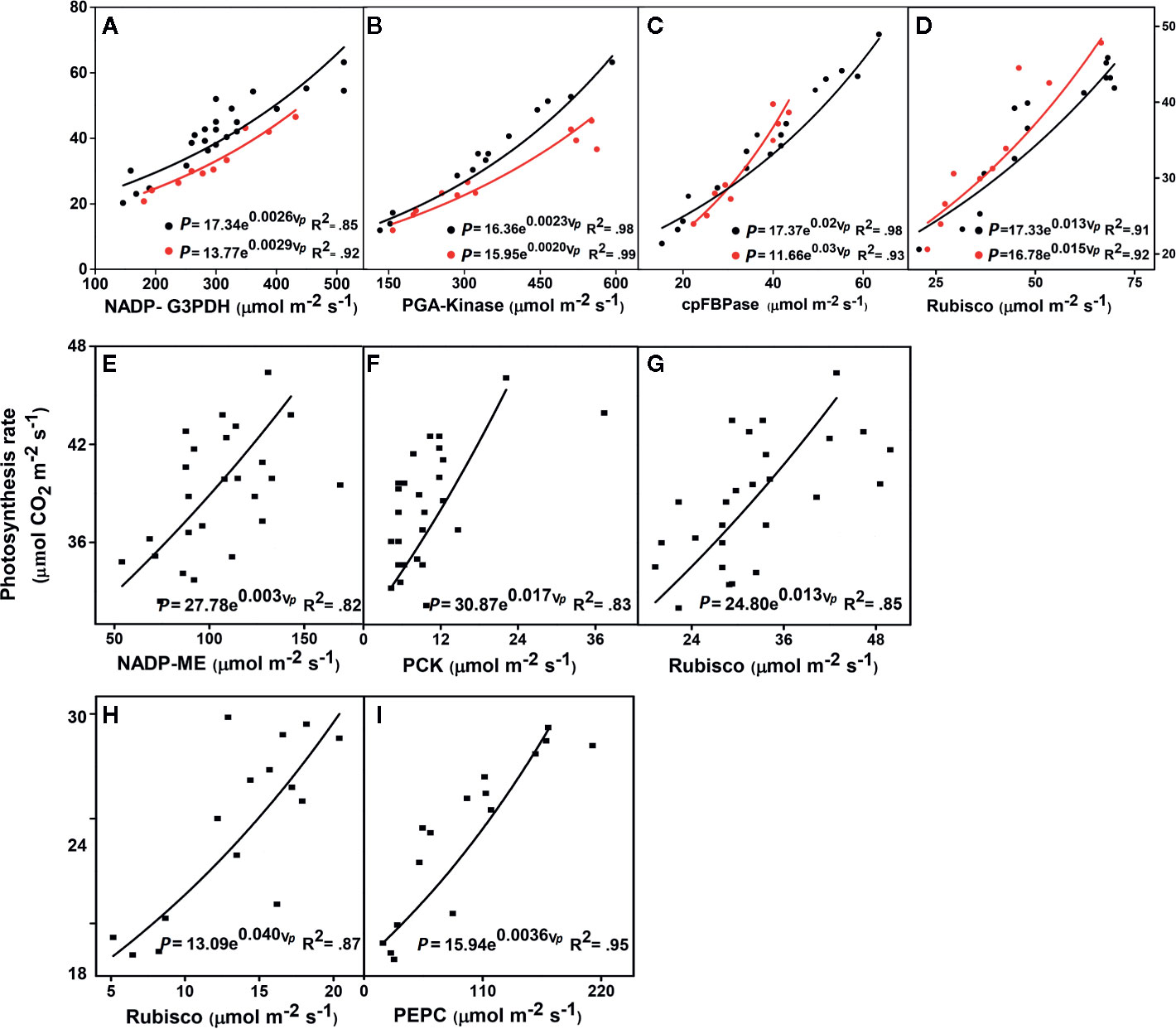
Figure 1 Relationships between the leaf photosynthesis rates and key enzyme activities. (A–D) Triticum aestivum (wheat, black circles) and Oryza sativa (rice, red circles), both C3 plants. (E–G) Zea mays (maize), a C4 plant. (H, I) Sorghum bicolor (sorghum), a C4 plant. NADP-G3PDH, NADP-glyceraldehyde-3-phosphate dehydrogenase; PGA-kinase, 3-phosphoglyceric acid kinase; cpFBPase, chloroplast fructose-1,6-bisphosphatase; Rubisco, ribulose-1,5-bisphosphate carboxylase/oxygenase; NADP-ME, NADP-malic enzyme; PCK, PEP carboxykinase; PEPC, phosphoenolpyruvate carboxylase; P, photosynthetic rate; Vp, enzyme activities. P-values arising from one-way ANOVA (Tukey test) are presented, significance at (P < 0.05). All parameter values are given in Table 1. The data for Triticum aestivum and Oryza sativa were taken from Sudo et al., 2003, for Zea mays were from Yabiku and Ueno, 2017, and for Sorghum bicolor were from Makino and Ueno, 2018. The complete detailed mentioned in Materials and Methods, Data Sources.
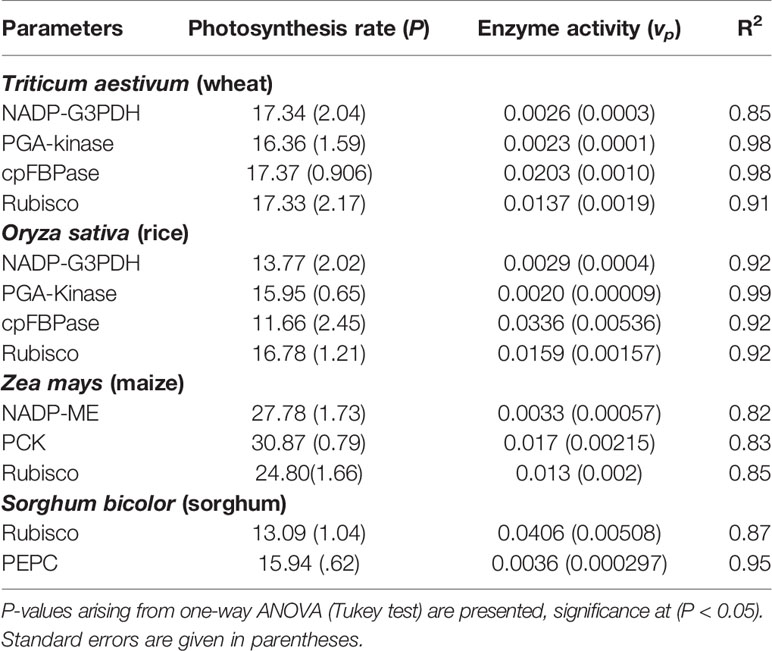
Table 1 Effect and the relationships between the photosynthetic rates (µmol CO2 m-2 s-1) and enzyme activities (µmol m-2 s-1) in the young leaves of C3 and C4 plants.
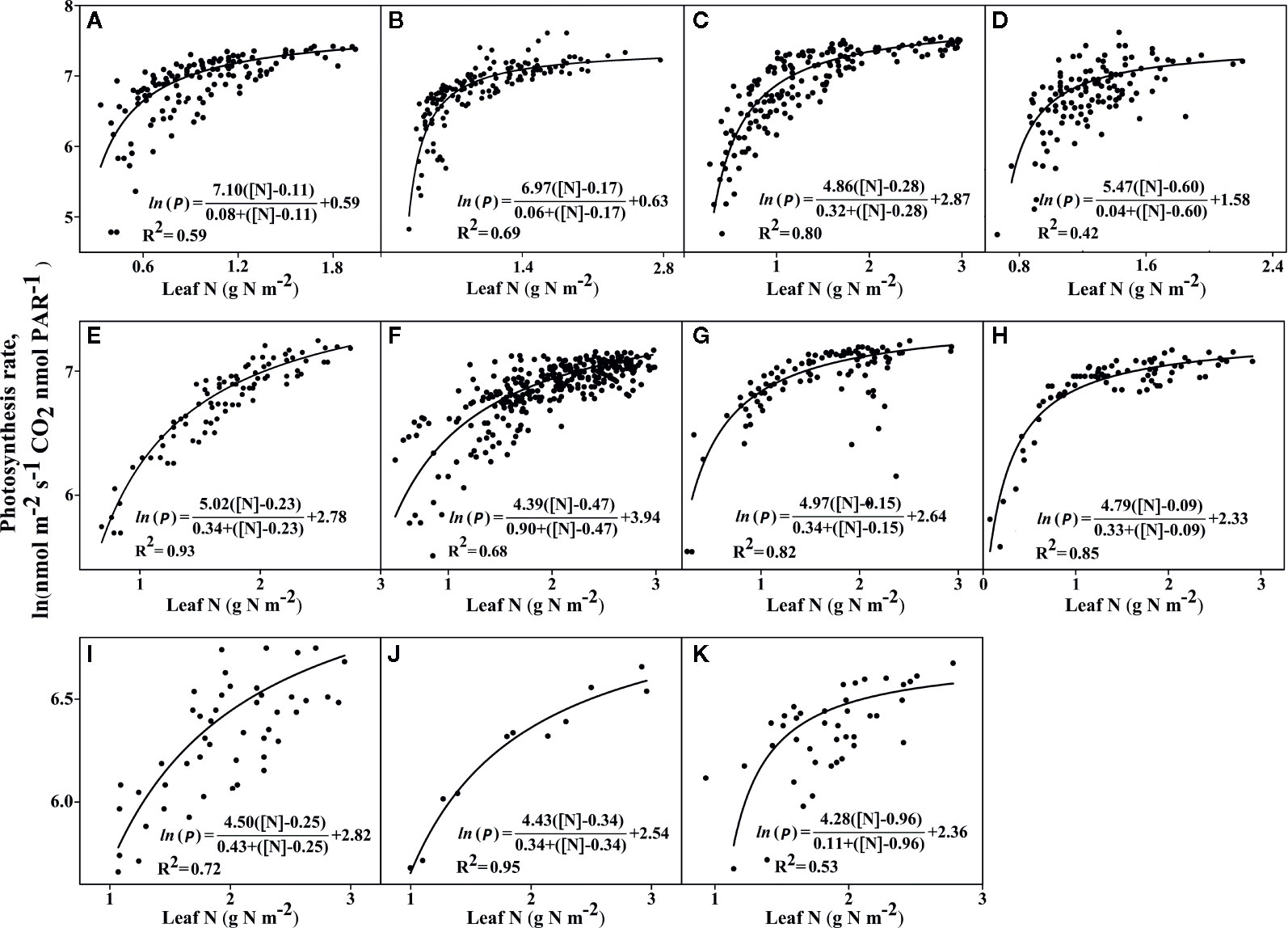
Figure 2 The photosynthetic rates in light-saturated conditions, which are dependent on leaf nitrogen [N] levels. Two C4 plants: (A) Sorghum bicolor and (B) Zea mays, and nine C3 plants (C) Triticum aestivum, (D) Oryza sativa, (E) Hordeum vulgare, (F) Glycine max, (G) Helianthus annuus, (H) Solanum tuberosum, (I) Citrus sinensis, (J) Malus domestica, and (K) Prunus persica. All parameters were determined using a best-fit non-linear regression line, and the R2 and P-values were determined using one-way ANOVA (Tukey’s test). Statistical significance was defined as P < 0.05. The sources for the data and references presented are given in Dataset_S1. The complete detailed mentioned in Materials and Methods, Data Sources.
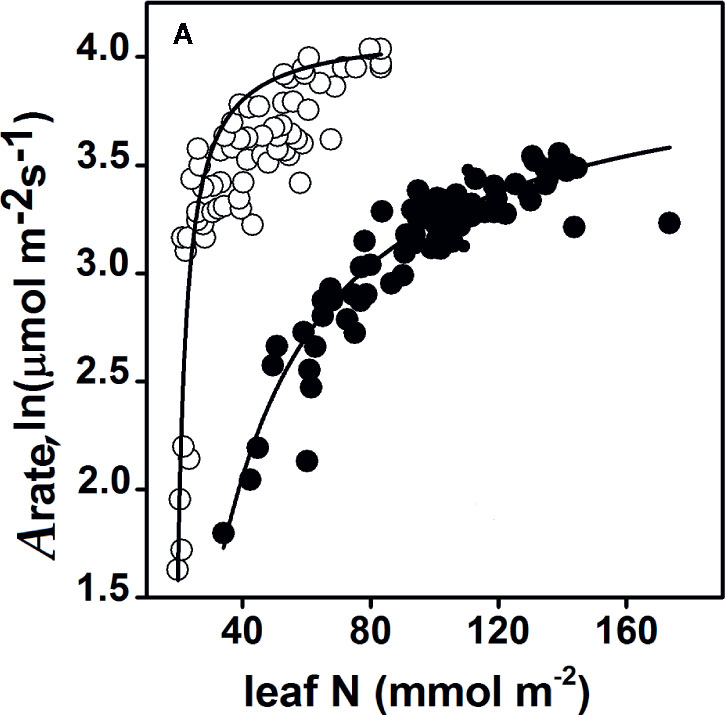
Figure 3 Response of the CO2 assimilation rate (Arate) to the same nitrogen content per unit leaf area in Zea mays (maize) (C4, white circle) and Oryza sativa (rice) (C3, black circle) plants. For the response of the nitrogen assimilation rate, Arate = 4.12, leaf N = 18.55, and R2 = 0.78 (maize); Arate = 4.05, leaf N = 18.75, and R2 = 0.91 (rice). The data collected for maize were from Sinclair and Horie, 1989 and Wong et al., 1985, and the data collected for rice were from Cook and Evans, 1983 and Makino et al., 1988. All responses were significant, and P-values arising from one-way ANOVA (Tukey’s test) analyses are presented. Statistical significance was defined as P < 0.05. The complete detailed mentioned in Materials and Methods, Data Sources.
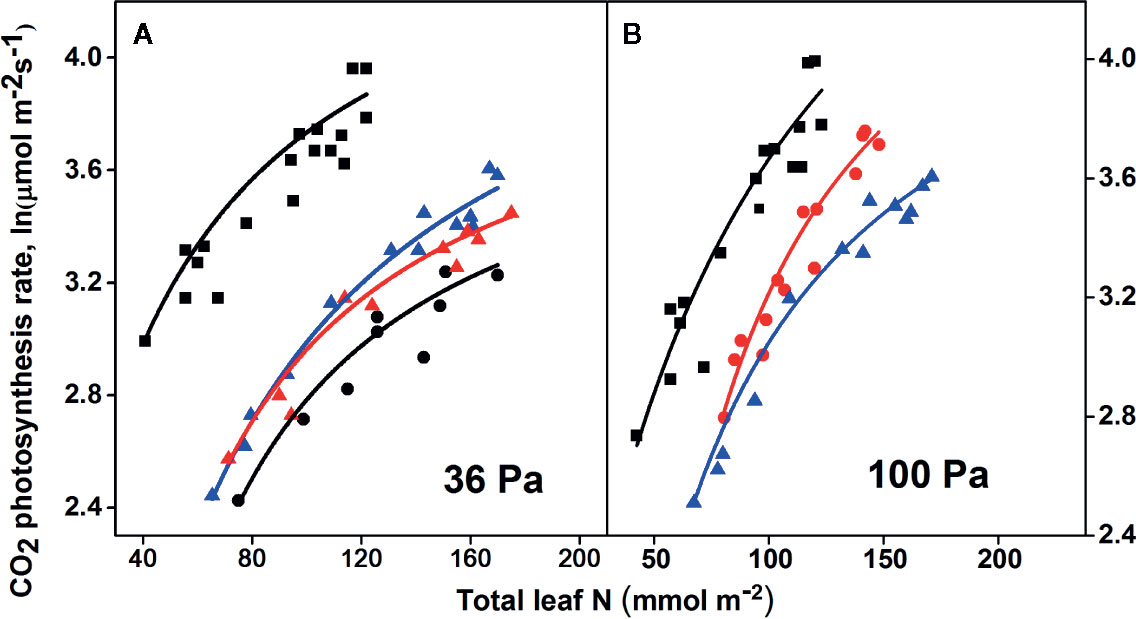
Figure 4 The response of the logarithmic-maximum photosynthetic rates to current (36 Pa) and elevated partial pressure (100 PaCO2) when the total leaf N was limited in the young leaves of C3 and C4 plants: (A) Zea mays (black squares, C4), Oryza sativa (blue triangle, C3), Spinacia oleracea (red triangle, C3), and Phaseolus vulgaris (black circles, C3); (B) Zea mays (black squares, C4), anti-rbcS 77 which is rbcS antisense rice with 65% wild-type Rubisco (red circles, C3), and O. sativa (blue triangles, C3). One-way ANOVA represents statistical differences (P < 0.05) by Tukey’s test and best R2. All the parameters are given in Table 2. The data were collected from Makino et al., 2003, and at 36 Pa for rice were from Makino et al., 1997 and at 100 Pa for rice including rbcS antisense rice from Makino et al., 1997. The complete detailed mentioned in Materials and Methods, Data Sources.

Table 2 Variations in the logarithmic-photosynthesis rates (lnPmax) of C3 and C4 species, at 36 and 100 partial pressure, under limited leaf N content (R – R0).
Model Background
Following the enzyme-kinetic model and MME (Michaelis and Menten, 1913), we developed a novel enzyme-driven model (EDM) to determine how individual leaf photosynthesis responds to limited nutrients for both the C3 and C4 crop plants. It was tested using the data of the leaf photosynthetic rate responses to the [N]. Therefore, the relative changes of individual photosynthesis (𝜕P) should be constrained by the activity of the various basic enzymes (𝜕vp) of photosynthesis in crop plants at the leaf level (Nagaraj et al., 2017), at a time when enzyme activities were a limiting factor and the other conditions were constant:
where P represents the photosynthetic rate of the crops plants, vp denotes the activities of the enzymes associated with the photosynthetic rate of the crop plants, 𝜕P represents the differential of P, while 𝜕vp represents the differential of the enzymatic activity of photosynthesis. Hence, the relationships between the photosynthesis and enzyme activities were determined by combining this information with Eq. 1 as follows:
where the coefficient of the transformation is represented by α, and b shows the potential of the enzyme activities (vp) in the crop plants. Therefore, Eq. 2 predicted that the photosynthetic rate increased exponentially with the enzymatic activity. Here, logarithmic (log) photosynthesis rates (ln P) and effective limited N sources (R – R0) were determined by applying log into Eq. 2, following the MME and letting the concentration of the nitrogen substrate ([S]) be proportional to the effective limited N resources (R – R0),
where ln Pmax = bVmax and [S] = R – R0. Kp is the half Michael’s constant (ln P = P_max/2), R represents the limiting N concentration, and R0 presents the value of R, where ln P = ln P0, while P0 is the coefficient of transformation (α) in Eq. 2, which is the photosynthetic rate when the effective resource (R – R0) is 0. This effective resource was presented in a lowest amount of stored [N] presented by the (P0 or Pstore) and used in the photosynthetic process of leaf production. Equation 3 predicts that the logarithm of the photosynthetic rate is dependent on the limited effective N content. Furthermore, by ignoring P0 in Eq. 3, the response of the assimilation rates to the leaf [N] in the C3 and C4 plants (Figure 3) and the response of the photosynthesis rates to the current and high partial pressures of [CO2] with regard to the leaf N contents in the C4 and C3 plants, were also tested (Figure 4).
Statistical Analysis
All statistical analyses were implemented in Origin 9.0 software (Data analysis and Graphing software). All the data (figures and tables) were tested using one-way ANOVA and Tukey’s test to assess each of the parameters. Data were log-transformed before analysis with Eq. 3. Most data were obtained from the supplementary resources of previous publications. These data sources were collected to verify our predictions by fitting exponential regressions between individual photosynthesis rates and the enzyme activities of the C3 and C4 plants in young leaves (Figure 1). Standard errors are given in parentheses (Table 1). A nonlinear correlation was used (Eq. 3) to evaluate the relationships between the various C3 and C4 plants. Furthermore, the assimilation rates for the same amount of leaf N were tested in C4 (maize) and C3 (rice) plants. Finally, the rates of photosynthesis per unit leaf N content in the young leaves of C4 and C3 plants, at low and elevated atmospheric partial pressures of CO2 (36 and 100 Pa of CO2) were investigated.
Results
The Photosynthetic Rate Increased Exponentially With the Limiting Enzymatic Rate in Young Leaves
We applied our model on published literature to understand the response of photosynthesis to enzymes activity. Our model also explained the response of light saturated photosynthetic assimilation to changes in leaf nitrogen in various C3 and C4 plants. We also tested the crops plant to the leaf N content under low and elevated CO2 concentration.
Our predicted exponential model (Eq. 2) was the best fitting model, shown by the lowest Akaike’s information criterion (Akaike, 1973; Posada and Buckley, 2004) and the best R2, when compared with the linear equation (see Supplementary Table 1 in Supplementary Material). Furthermore, the linear equation did not show the best feedback to photosynthesis, in comparison with our predicted exponential equation (EDM, Eq. 2). All the data were obtained under constant [N] conditions to show that the activities of certain key enzymes exponentially increased with the photosynthetic rate (P) at the leaf scale in C3 (wheat and rice) and C4 plants (maize and sorghum) (Figure 1) (see all data source in Dataset_S1). Conversely, the respective values for P were higher and had decreased enzymatic activities in wheat when compared with rice (Figures 1A–D). The lower enzyme activities increased P and indicated that along with the limiting enzymes, other limiting factors also affected the enzyme activities and P level. In contrast, the increased activity of PGA-kinase (Figure 1B) significantly enhanced P in wheat compared with rice. In addition (Figure 1D), lower activities of Rubisco also enhanced P in wheat than in rice. These results clearly showed that the Calvin cycle enzymes changed their activities in young leaves of different crops plant, which is also dependent on the leaf trait (Makino and Ueno, 2018) and leaf age (Yamaoka et al., 2016). The response of enzyme activities increased exponentially with P in young maize leaves. It appears that higher plants have a complicated mechanism, and the activity levels of the Calvin cycle enzymes are limited by other factors. Of these, the activity of three enzymes from the maize leaf, PCK, and NADP-ME, initially influenced P, whereas Rubisco activity initially enhanced and then reduced P when compared with effects of other enzymes such as PCK and NADP-ME (Figures 1E–G). Our exponential model showed that all the three enzymes exhibited a significant correlation with P (P < 0.05) compared to linear model (see in Supplementary Material; Supplementary Table 1). Compared with Rubisco, the PEPC displayed an increased P, whereas the Rubisco contributed to the higher enzymatic activities in sorghum (Figures 1H, I). The results posit that PEPC had a higher affinity to Rubisco, which led to an increased P in the sorghum leaf. Therefore, the data used for sorghum were taken from low to high N sources (Makino and Ueno, 2017; see Supplementary Material for Supplementary Figure 1), which showed that PEPC and Rubisco decreased their activities by reducing the concentrations of N. The results revealed that reducing the N supplies resulted in a reduced P, as shown in sorghum (see in Supplementary Material for Supplementary Figure 1). Along with the limited Calvin cycle enzyme activities and N limitations, some biochemical and physiological traits are indirectly involved in the interspecific differences of the P.
Photosynthetic Rate Dependence on Leaf [N] at the Leaf Scale
We assessed 11 species, including nine C3 plants and C4 grasses (two C4 plants: sorghum and maize) for their photosynthetic capacities, to explain the higher use of photosynthetic N at the leaf level (Figure 2). Under saturated light conditions, the amount of leaf [N] was lower in both the C4 plants (two C4 plants) compared to C3 plants (nine C3 plants). However, the C4 plants had the highest maximum CO2 photosynthesis rate (Pmax) but the lowest amount of stored [N] (P0), when compared with the C3 plants (Figures 2A–K). Across all 11 plant species, the photosynthetic capacity was the highest in the C4 plants (Figures 2A, B) and the lowest in the C3 plants (Figures 2C–K). However, even when there were low N uptake concentrations (higher Pmax needs less N absorption), C4 plants had greater photosynthesis rates (Pmax) when compared with C3 plants (higher Pmax needs more N absorption). For instance, rice, maize, and sorghum showed the highest affinity (half-saturation constant, Kp, for leaf [N]), in decreasing order. However, both maize and sorghum exhibited the lowest P0 (half to the highest Pmax) across all 11 plants. P0 is the minimum amount of stored [N], mainly used for leaf expansion and photosynthetic capacity. Rice had the highest amount of leaf [N] or uptake after Prunus persica, and thus achieved the highest Pmax rate of all nine C3 species tested. Therefore, with the same amount of leaf N content, maize (C4) showed a higher Arate relative to rice (C3) (Figure 3). Interestingly, Prunus persica achieved the highest leaf [N] but still showed the lowest Pmax for all 11 plants. These results showed that the allocation of N to the photosynthetic machinery decreased, but still enhanced the Pmax rate when compared with all the C3 plants.
The Shift of the EDM From Low to Elevated Pa [CO2]
The responses of the C3 and C4 plants to the leaf N contents under 36 and 100 Pa [CO2] and the effects of the CO2 on the maximum photosynthetic rate (Pmax) and the uptake of the leaf N content were examined (Figures 4A, B). The results demonstrated that under both Pa [CO2] conditions, the maize plants maintained the maximum Pmax rate compared with the C3 plants. Interestingly, maize showed higher Pmax and absorption of leaf N content under elevated Pa [CO2] (Figure 4B). In the maize plant, the response of the Kp (half-photosynthesis constant) to the leaf N content showed a higher affinity under elevated CO2 (Figure 4B). Although while transgenic anti-rbcS 77 (wild rice) had 65% wild-type Rubisco, it still had a lower Pmax when compared with the maize under elevated Pa. Although these results suggest the suppression of Pmax and leaf N content under the elevated Pa when compared with the low Pa due to the enrichment of the CO2 in the rice plants (Figures 4A, B), the results suggest that long-term CO2 decreases the initial stimulation of photosynthesis and then down-regulates it; this finding suggests a decrease in the Rubisco content in plants. These results revealed that the leaf N content and Rubisco were closely related, which further directly or indirectly affects photosynthesis. Many researchers have suggested a positive correlation between photosynthesis rates and leaf N content, and we have validated this by showing that N deficiency causes a reduction in the photosynthetic rate and intercellular CO2 concentrations (Ci) (see Supplementary Figure 2 in Supplementary Materials). Thus, N deficiency and CO2 concentrations are important for crops in the future, both physiologically and morphologically.
Discussion
Photosynthesis Rate Showed Exponential Response to Enzyme Activities
We made a simple prediction, that the photosynthetic rate (P) of various plant species would be scaled non-linearly in relation to their enzyme dynamics and limited leaf [N]. Previous studies showed that activities of the Calvin cycle enzymes changed slightly from the young to the mature leaves of rice (Yamaoka et al., 2016), and in wheat (Suzuki et al., 1987). In addition, there were other limiting factors that inhibited enzyme activities and affected P in the crop plants. In our model, we found lower activities of cpFBPase still enhanced higher P in wheat than in higher activities of cpFBPase with lower P in rice (Figure 1C). This higher linear correlation of cpFBPase with the CO2 photosynthesis rate in wheat, had previously been reported (Sudo et al., 2003). A positive correlation was also found between the Calvin cycle enzymes such as cpFBPase and the photosynthesis rates (Miyagawa et al., 2001). While Rubisco showed lower activity, it still enhanced P in wheat than in rice. This showed that wheat exhibited a higher capacity for the assimilation of C when compared with rice. Earlier reports mentioned that changes in the amount of N supplied directly, enhanced the rate of leaf expansion progressively and therefore enhanced the capacity for C assimilation, which correlated with the increased levels of key photosynthetic enzymes (Huber et al., 1989). In certain conditions, rice was revealed to have higher photosynthetic rates, due to the higher conductance of CO2 through the cell wall, chloroplast thickness, and carbonic anhydrase activity, when compared with wheat (Makino et al., 1988). Moreover, the synthesis of Rubisco decreased with advancing leaf maturity, which was closely interlinked with decreases in the N influx into the leaf, as demonstrated by Imai et al. (2005). This suggests a higher RUBP regeneration capacity in wheat when compared with rice. The results (Figures 1A–D) revealed that the Calvin cycle enzymes in wheat and rice plants responded differently, clearly showing variations with leaf traits and developmental stages. Rubisco synthesis was completed during the leaf expansion, while the concentrations of the Rubisco regulated the levels of protein degradation during the leaf senescence (Mae et al., 1984: Makino et al., 1984: Suzuki et al., 2001). This indicates that the synthesis of Rubisco could be stimulated if a greater N concentration was present in the senescing leaf, because N influx drops during leaf senescence and is closely associated with the synthesis of Rubisco (Imai et al., 2008). These variations in the activities of the limited Calvin cycle enzymes depend on leaf age (Prasad et al., 2009), and leaf ontogeny (Sesták, 2012). Similar results were reported and explained for various key enzymes that were correlated with the photosynthetic activities of C4 species (Usuda et al., 1984) and the changes associated with leaf age in maize (Usuda, 1984), and various levels of N (Sugiyama et al., 1984). Therefore, we tested our exponential model where the enzyme activities showed higher significance (P value and R2) and lowest AIC values compared to the linear model (See Supplementary Material for Supplementary Table 1).
On the contrary, P showed positive exponential correlations with the activities of NADP-ME, PCK, and Rubisco (Figures 1E–G). Similarly, a positive correlation was also found for the photosynthesis rate and activity of NADP-ME (Nose et al., 1994). Furthermore, PCK activity increased, leading to higher photosynthesis rates and a higher exponential correlation with P (Figure 1F). The PCK enzyme is highly expressed in the BS cells, where oxaloacetate releases CO2, which is fixed through Rubisco. Although, NADP-ME activity showed the lowest activity, it still enhanced the P in maize. Previous results reports the reason that in the C4 cycle, NADP-ME was involved in short steps of the enzyme and amino acid pathways (Kanai and Edwards, 1999), and tended to have higher PNUE and NUE (Ghannoum et al., 2005). Therefore, various characteristic traits play important roles in the photosynthetic rate and could be regulated by the electron transport rates in maize. Some studies like NADP-ME in maize, and Rubisco were also involved in the re-fixation or regenerate phosphoenolpyruate carboxylase (PEPC), and as a result Rubsico is a rate-limiting enzyme (Yabiku and Ueno, 2017), which has positive correlations with the photosynthetic rates (Baer and Schrader, 1985). PEPC did not show a correlation with the photosynthesis in maize (Yabiku and Ueno, 2017), but showed a significant relationship in sorghum (Makino and Ueno, 2018). Various studies suggest that higher NUE occurred due to various genetic factors that were closely related to the N content in maize (Yabiku and Ueno, 2017). However, our model suggest that the exponential relationship between NADP-ME activity and P possibly be differ among species.
The highest positive correlation with P was in the leaves of sorghum; however, it had higher Rubisco enzyme activity (Figures 1H–I). PEPC exhibited higher affinity and was strongly correlated to P in C4 plants, when compared with the C3 plants, and followed the EDM. As the data were taken from low to high N sources, the ratio of the PEPC and Rubisco declined with the reducing N contents. Compared with the Rubisco, the PEPC showed greater reductions in line with the reduced N content at the leaf level (Makino and Ueno, 2018), and similar responses were also found in Amaranthus retroflexus (Sage et al., 1987) and maize (Sugiyama et al., 1984). Therefore, C4 plants with lower enzyme levels revealed a higher affinity of PEPC for CO2 to achieve higher P. A strong positive correlation is commonly observed between both PEPC and Rubisco activity and maximum photosynthetic rates (Von Caemmerer et al., 1997). Similarly, in maize, it was reported that N was partitioned into PEPC, which may function as a storage reservoir for excess leaf N (Uribelarrea et al., 2009), and consequently, the optimum maximum growth was exceeded (Sugiyama et al., 1984; Makino et al., 2003). In response to the supply of N (Suzuki et al., 1994), the PEPC activity increased with the N supply.
Therefore, N plays a huge role alongside enzymes in increasing the P. Similarly, reducing the N supply resulted in a reduced photosynthesis, N content, chlorophyll content, and PEPC and Rubisco carboxylase/oxygenase activity. Therefore, our model predicts that increasing the N content per leaf area would enhance the thylakoid and Calvin cycle enzymes, which would change the key physiological processes. This finding has a fundamental application for their N utilization and N uptake in that enzyme activities play a role in the rate of plant growth and photosynthesis. Most enzyme activities showed a higher coefficient of correlation (Figure 1 and Table 1); therefore, we have shown that enzyme dynamics possibly drive the photosynthesis rate and modeling of resource fluxes. The main finding of our research is that our model explains the exponential relationships very significantly. Therefore, our model were tested and fitted compared to linear model. We tested our exponential model where the enzyme activities showed higher significance (P value and R2) and lowest AIC values compared to the linear model (Table 1 and see Supplementary Table 1 in Supplementary Material).
Response of Photosynthetic Rate Dependent on Leaf Nitrogen Content
Some plant species require various N sources to regulate photosynthesis (Evans, 1989; Rotundo and Cipriotti, 2017). This study demonstrates that this variation is a determinant of the amount of leaf [N] under saturated light conditions and exhibits the response of Kp (half-saturation constant) to leaf [N] (Figures 2A–K). C4 species (maize and Sorghum) showed the highest affinity to leaf [N], which contributed to their having the maximum photosynthesis rate (Pmax) (Figures 2A, B). Accordingly, for each plants N allocation to the leaves, an optimum N content exists to maximize its crop biomass production (Sinclair and Horie, 1989). A higher PNUE was observed in maize plants with efficient use of N, to increase their NUE and biomass production (Mu et al., 2016). In this study, it was found that C4 species could enhance their physiological NUE if N storage rates were lowered by enhancing Pmax, which is the PNUE. Thus, C4 species that invest less N greatly enhanced their Pmax, which is in accord with previous studies that C4 species exhibit higher Pmax because of the speed of the Rubisco, which enables them to invest fewer N resources into Rubisco (Young et al., 2016). With the same amount of leaf N content (Evans and von Caemmerer, 2000), maize clearly showed assimilation rates higher than rice. Therefore, maize showed higher N assimilation and higher NUE than the rice (Figure 3). C4 species have a higher ability to utilize CCM to concentrate CO2 around Rubisco and suppress photorespiration and RUBP regeneration (Sage and Zhu, 2011). Previous studies showed that in C4 plants, higher amounts of N investment into the thylakoids could possibly maintain greater NUE (Makino et al., 2003). Thus the present results revealed that every plant species showed substantial differences in leaf N content as a result variance in Pmax. That’s why the differences in plant leaf Pmax among the C4 and C3 species possibly attribute to differences in physiological and biochemical features in their leaves.
C3 plants (Figures 2C–J) have larger P0 than did C4 plants (Figures 2A, B); for this reason, C3 species still require more N to get a higher Pmax. Here, we can evaluate a leaf physiology that both C4 plants invest less N content in leaf production to enhance higher Pmax compared to invest more N required to enhance photosynthesis in C3 plants. Similarly, the previous results have shown a higher N content used in the photosynthetic processes of C3 plants (Evans and Seemann, 1989; Evans, 1989). Unexpectedly, the stored N in the form of P0 was also higher in the C3 species than in the C4 species. C4 species, even at low P0, can maintain a higher Pmax and a higher probability of survival than can C3 species. Hence, nutrient stoichiometry changes due to the availability of N; such variations enable plants to increase their C uptake and enhance the efficiency of using their resources to fix C under both C- and N-limiting conditions for plant growth. As C4 species have a higher importance because of their enzyme activities, and their various resource allocations, as a results, C4 photosynthetic pathways can invest more N into leaf production, than C3 pathways. Our model from the analysis revealed that allocation of N in C4 species is higher into leaf thylakoid and invest less N into Rubisco than in C3 plants.
In our model, photosynthetic rate of C4 species showed greater dependence on N content and light, than the nine C3 species assessed; consequently, these results determined greater NUE in the C4 species than the C3 species. Evolutionary pressures appear to have concentrated the enzymes towards more efficient utilization of CO2 (Jordan and Ogren, 1981). Consequently, the evolution of plants from C3 to C4 is marked by the limitation of photorespiration (Kelly, 2018), which requires a high level of CO2 concentrated around the Rubisco. Previous studies demonstrated that Amaranthus retroflexus showed greater NUE than Chenopodium album (Sage and Pearcy, 1987). In addition, the diversity of the photosynthetic capacity, which is correlated with leaf traits, explained the relative allocations of N to the photosynthetic functions and showed various PNUE among the different crop plants.
Across all C3 plants (Figures 2C–K), rice (Figure 2D) achieved higher leaf N content and a higher Pmax. This study demonstrated that across various C3 species (Figures 2C–K), rice showed the lowest stored P0 after C4 species such as maize and sorghum (Figures 2A, B). Therefore, to get maximum Pmax rates, rice needs a lower amount of stored N in the form of P0. The results of this study revealed that the Pmax rate may be enhanced when more N is supplied. Storage N and various residues of N could be enhanced in the leaves due to the supplies of N (Yasumura and Ishida, 2011; Avice and Etienne, 2014; Wyka et al., 2016). Photosynthesis proteins and young tissues use the stored N for their growth. Under low N conditions, young leaves need a higher supply of N, which leads to decreases in the stored N pool size in mature leaves (Liu et al., 2018). In rice, which showed the highest Pmax, increased carbonic anhydrase activity appears to have a direct relationship with the mesophyll conductance (Makino et al., 1992), which is closely related to the surface area of the chloroplast (Terashima et al., 2005). Our model posits that the amount of N affects leaf thickness, which strongly affects mesophyll conductance and causes variations in the nutrient cycle and plant growth capacity. Thus, higher amounts of N and Rubisco both functioned as stored N proteins and catalytic enzymes, respectively. This was similar to the findings of Warren et al. (2003), according to which, with increasing N concentrations, Rubisco functions as a storage protein in Pinus sylvestris. Such limitations of the leaf N content affect the proteins involved in the Calvin cycle, resulting in the described photosynthetic regulation.
The PNUE is an important leaf trait that describes adaptive strategies, physiology, and the leaf economics of a species (Onoda et al., 2017) and may indirectly reflect the efficiency of the N utilization (Feng et al., 2008). Most importantly, our results demonstrated that even at low N, C4 species still had higher PNUE, causing higher N allocations, and the upregulation of photosynthesis and increases in C gain. Therefore, C4 species revealed higher C gains than did C3 species. Both the C and N levels control leaf expansion (Lattanzi et al., 2005; Pantin et al., 2011). The higher N content present because of the limited C in the leaves, enhances the capacity of leaf photosynthesis (Liu et al., 2018). Similarly, Rubisco and PEPC activities vary with N nutrition, leaf age, and light intensity during plant growth (Usuda, 1984; Meinzer and Saliendra, 1997). This explains why the photosynthesis rate depends on the N sources, storage N, and species-specific photosynthetic capacity characteristics. At last, the variance response of leaf physiology can be evaluate or judge by the variance in PNUE, leaf N content and N allocation in C3 and C4 plants, explained by our model; which is also explained by other studies (Poorter and Evans, 1998; Westbeek et al., 1999). Therefore, the results reveal the generality of the impact of nitrogen allocation to the interspecific difference in PNUE.
Response of Photosynthetic Rate to Nitrogen Content Under Low and Elevated Pa [CO2]
Our model clearly shows that maize exhibited the highest Pmax under elevated 100 Pa [CO2] (Figure 4B). As shown in Figures 4A, B, however, the leaf N content was affected by the eCO2; however the Pmax rate decreased with eCO2 in rice. On the other hand, anti-rbcS 77 (rbcS antisense rice with 65% wild-type Rubisco) under eCO2 enhanced N content as a results increased Pmax than in rice (Figure 4B). The results revealed that in transgenic rice of anti-rbcS 77, the reduced activity of Rubisco leads to reallocation of N in to leaf thylakoid as a results increased NUE, which is in accord with the earlier study (Makino et al., 1997). Then the increased NUE suggests that anti-rbcS 77 invest more N into photosynthetic apparatus that are involved in Pmax. While on other hand, the result clearly predicts a decrease in the Pmax of rice at elevated Pa, because the CO2 enrichment was correlated with starch accumulation in the plant leaf blades (Nakano et al., 1997), which caused reduction in leaf N content. Thus, the decrease in the photosynthetic capacity could possibly be related to a reduction in the leaf N content. Rice (C3 plant) showed the lowest leaf N content by low [CO2] compared with Spinacia oleraceae and Phaseolus vulgaris (C3 plants); however, the enhanced maximum Pmax was comparable across all C3 plants. Rice maintained higher N utilization in the leaves, when compared with the other C3 plants, under low eCO2 (Figure 4A). This indicates that the reallocation of N can provide a mechanism to enhance the biomass and Pmax rate, as described by Field (1983), thus enhancing the whole-plant C gain. Higher N allocation enhances the PNUE in the photosynthesis process with higher supplies of N (Hikosaka and Hirose, 1998). In general, high photosynthetic rates lead to enhanced growth rates and maintenance rates. Therefore, Farquhar et al. (1980) developed a photosynthetic model for the C3 pathway, a photosynthesis rate limited by Rubisco at low PaCO2 and by electron transport capacity at high PaCO2. The varying Kp responses of the leaf N to CO2 among the different plants, indicated that the photosynthetic responses of the plants to the low and elevated CO2 were not only due to the levels of the CO2 but also the N contents. However, every species has a different photosynthetic capacity to store a high N source and then to utilize it for their maximum performance for new tissue and plant growth. Thus, the reduction in the N content in the leaf is a known indicator of photosynthetic accommodation to eCO2 (Leakey et al., 2009).
N deficiency reduces the size of the chloroplasts (Bondada and Syvertsen, 2005), and thus high levels of stored N may increase the size of the chloroplasts in rice cultivars (Laza et al., 1993); with higher chloroplast sizes being beneficial for higher C gains. In addition, our model showed that the N was influence by high PaCO2 in maize and rice (Figure 4B and Table 2), which directly or indirectly affects the size of the chloroplasts (Bondada and Syvertsen, 2005), because the chloroplast morphology and ultrastructures affect the photosynthesis. Similar results reported that long-term elevated CO2 usually causes a reduction in the photosynthetic capacity, as it is directly related to the decreased levels of Rubisco and other C3 Calvin cycle enzymes (Ghannoum et al., 2000). Although sorghum (C4) uses N more efficiently than most C3 plants, N deficiency suppressed the Ci and photosynthesis (see Supplementary Figure 2 in Supplementary Material). Maranville and Madhavan (2002) reported that N deficiencies also decreased the levels of both Rubisco and PEPC in sorghum leaves. Like some species that have the C3 photosynthetic pathways, Rubisco is likely to represent the single remobilized reserve of protein-N, which generally accounts for 30%–60% of the total soluble protein, 20%–30% of the total leaf N in C3 plants (Makino, 2003), and 5%–9% of the total leaf N in C4 plants (Sage et al., 1987). However, maize still had a high Pmax due to the variance in the regulation of the photosynthetic C4 gene expression (Sheen, 1999).
Our findings also support and show that Rubisco activity is closely related to the low affinity of CO2 (Sage, 2002); thus, a lower level of Rubisco is enough to enhance the Pmax in maize plants. Interestingly, the published data predicted that even maize exhibited a low affinity for Pmax rates, but higher N levels showed that more allocations of N had occurred, and sometimes, Rubisco acted as a storage protein over a long period to enhance the growth and biomass production for a long leaf lifespan, under elevated PaCO2. Besides, N content is enhanced in the maize leaves with eCO2 and suggests that elevated CO2 increased leaf N metabolism and amino acid biosynthesis. Similar results are also reported in root N metabolism (Cohen et al., 2019a). In rice species, leaf [N] reduced from low to elevated CO2 (Figures 4A, B), suggesting that higher investments of the N in Rubisco caused less N to be invested in the PNUE and storage proteins. Consequently, a high amount of leaf N is directed to the biosynthesis of the photosynthetic machinery. From these observations, elevated CO2 influences the limitations in the photosynthetic capacity to decrease N allocations to proteins and Rubisco that are involved in electron transport (Liberloo et al., 2005); hence, the reduction in N allocation possibly serves to enhance available and mobile N for new foliage growth. Hence, results support the reallocation of N among crop plants, which could provide an adaptation mechanism in response to climatic changes, and rising atmCO2 concentrations may improve the physiological process model.
Rubisco expression was adversely affected by elevated CO2 in tomato plants (Cohen et al., 2019a), which suggests that the reduced uptake rate of N occurred due to the atmCO2 concentrations in the leaves of C3 plants, and thus, due to the substantial rise in PNUE. The low leaf N concentration under the eCO2 in rice (C3 plant; Figure 4B), suggests a lower allocation of N to photosynthetic apparatus which is important determinant of PNUE. Thus, our model showed that eCO2 affect the N concentration in rice as a results reduced the Pmax. Thus, a higher NUE could increase the allocation of N to the photosynthetic process due to the higher N availability (Poorter and Evans, 1998). The response of crops to eCO2 (Long et al., 2006) revealed that crop plants under elevated CO2 decreased the activity of Rubisco for the regeneration of RUBP. Since in a C4 plant, a huge utilization of the N occurs in the thylakoid, the CO2 concentrating process maintains greater NUE for photosynthesis (Makino et al., 2003). In this case, the results support that under the various levels of PaCO2, the positive relationships found between the physiology and N content, may be useful in various responses of the photosynthesis in crop plants. Therefore, the reduction in the amount of Rubisco would be an advantage to enhance NUE. Therefore, the high CO2 emissions worldwide and global warming, have intense impacts on important crop plants, their production, and their dynamic metabolic balances, particularly at the leaf level.
Conclusions
The different exponential responses of the key enzyme activities to the photosynthesis rates clearly showed leaf trait variations and the developmental stages of the leaves for wheat and rice. In the young leaves of wheat and rice, the enzyme activities exponentially increased with the photosynthetic rate. Our findings suggest that enzyme activities and photosynthesis rate showed higher exponential correlation than linear correlation. In the young leaves of maize, PCK, NADP-ME, and Rubisco were positively correlated with the photosynthetic rates. In sorghum, it was suggested that both the PEPC and Rubisco increased with the increased leaf N content. All enzymes exhibited higher exponential relations with photosynthesis compared to linear relations. C4 plants (sorghum and maize) exhibited higher affinities to light and N sources than C3 plants; therefore, we identified that C4 plant species had a higher PNUE and higher C gain. Regarding leaf economy, the stored N source provided half of the N required for the maximum photosynthetic capacity used for leaf expansion and plant growth. Our findings suggest that the Kp affinity of various species is a key indicator for various species, which affects resource allocation. Finally, the elevated CO2 had a negative effect on the N concentrations of C3 plant leaves and needs to be investigated further in more crop plants.
Data Availability Statement
All datasets generated for this study are included in the article/Supplementary Material.
Author Contributions
AK and GW designed the research. AK and LL collected the data. AK, LH, and LL analyzed the data. KX and HH designed Figures 1, 2 in Adobe Illustrator CC. AK, KX, and GW wrote the manuscript.
Funding
This work was supported by the National Natural Science Fund of China (31330010) and Natural Science Foundation of Zhejiang Province (LZ13C030002).
Conflict of Interest
The authors declare that the research was conducted in the absence of any commercial or financial relationships that could be construed as a potential conflict of interest.
Acknowledgments
We thank GW and ZW for their helpful comments during the revision of the article. We further thank KX, HH, and LL for their insightful comments on the discussion.
Supplementary Material
The Supplementary Material for this article can be found online at: https://www.frontiersin.org/articles/10.3389/fpls.2020.533341/full#supplementary-material
References
Aguiar-González, B., Packard, T. T., Berdalet, E., Roy, S., Gómez, M. (2012). Respiration predicted from an enzyme kinetic model and the metabolic theory of ecology in two species of marine bacteria. J. Exp. Mar. Bio. Ecol. 412, 1–12. doi: 10.1016/j.jembe.2011.09.018
Ainsworth, E. A., Long, S. P. (2005). What have we learned from 15 years of free-air CO2 enrichment (FACE)? A meta-analytic review of the responses of photosynthesis, canopy properties and plant production to rising CO2. New Phytol. 165, 351–372. doi: 10.1111/j.1469-8137.2004.01224.x
Akaike, H. (1973). “Information theory and an extension of the maximum likelihood principle,” in Second International Symposium on Information Theory. (Budapest: Akademiai Kiado), 267–281.
Avice, J. C., Etienne, P. (2014). Leaf senescence and nitrogen remobilization efficiency in oilseed rape (Brassica napus L.). J. Exp. Bot. 65, 3813–3824. doi: 10.1093/jxb/eru177
Baer, G. R., Schrader, L. E. (1985). Relationships between CO2 exchange rates and activities of pyruvate, Pi dikinase and ribulose bisphosphate carboxylase, chlorophyll concentration, and cell volume in maize leaves. Plant Physiol. 77, 612–616. doi: 10.1104/pp.77.3.612
Baudouin-Cornu, P., Surdin-Kerjan, Y., Marlière, P., Thomas, D. (2001). Molecular evolution of protein atomic composition. Science 293, 297–300. doi: 10.1126/science.1061052
Benson, A. A. (2002). Paving the path. Annu. Rev. Plant Biol. 53, 1–25. doi: 10.1146/annurev.arplant.53.091201.142547
Bloom, A. J., Burger, M., Kimball, B. A., Pinter, P. J. (2014). Nitrate assimilation is inhibited by elevated CO2 in field-grown wheat. Nat. Clim. Change 4, 477. doi: 10.1038/nclimate2183
Bondada, B. R., Syvertsen, J. P. (2005). Concurrent changes in net CO2 assimilation and chloroplast ultrastructure in nitrogen deficient citrus leaves. Environ. Exp. Bot. 54, 41–48. doi: 10.1016/j.envexpbot.2004.05.005
Busch, F. A., Sage, R. F., Farquhar, G. D. (2018). Plants increase CO2 uptake by assimilating nitrogen via the photorespiratory pathway. Nat. Plants 4, 46–54. doi: 10.1038/s41477-017-0065-x
Calvin, M. (1989). Forty years of photosynthesis and related activities. Photosynth. Res. 21, 3–16. doi: 10.1007/bf00047170
Carr, G. M., Duthie, H. C., Taylor, W. D. (1997). Models of aquatic plant productivity: a review of the factors that influence growth. Aquat. Bot. 59, 195–215. doi: 10.1016/S0304-3770(97)00071-5
Cohen, I., Halpern, M., Yermiyahu, U., Bar-Tal, A., Gendler, T., Rachmilevitch, S. (2019a). CO2 and nitrogen interaction alters root anatomy, morphology, nitrogen partitioning and photosynthetic acclimation of tomato plants. Planta 250, 1423–1432. doi: 10.1007/s00425-019-03232-0
Cohen, I., Rapaport, T., Chalifa-Caspi, V., Rachmilevitch, S. (2019b). Synergistic effects of abiotic stresses in plants: a case study of nitrogen limitation and saturating light intensity in. Arabidopsis thaliana. Physiol. Plant 165, 755–767. doi: 10.1111/ppl.12765
Cook, M. G., Evans, L. T. (1983). Nutrient responses of seedlings of wild and cultivated Oryza species. Field Crops Res. 6, 205–218. doi: 10.1016/0378-4290(83)90061-8
Crafts-Brandner, S. J., Hölzer, R., Feller, U. (1998). Influence of nitrogen deficiency on senescence and the amounts of RNA and proteins in wheat leaves. Physiol. Plant 102, 192–200. doi: 10.1034/j.1399-3054.1998.1020206.x
Dellero, Y., Lamothe-Sibold, M., Jossier, M., Hodges, M. (2015). Arabidopsis thaliana ggt1 photorespiratory mutants maintain leaf carbon/nitrogen balance by reducing Rubisco content and plant growth. Plant J. 83, 1005–1018. doi: 10.1111/tpj.12945
Evans, J., Seemann, J. R. (1989). The allocation of protein nitrogen in the photosynthetic apparatus: Costs, consequences, and control. Plant Biol. 8, 183–205.
Evans, J., von Caemmerer, S. (2000). Would C4 rice produce more biomass than C3 rice? Stud. Plant Sci. 7, 53–71. doi: 10.1016/S0928-3420(00)80006-3
Evans, J. R. (1983). Nitrogen and photosynthesis in the flag leaf of wheat (Triticum aestivum L.). Plant Physio. 72, 297–302. doi: 10.1104/pp.72.2.297
Evans, J. R. (1989). Photosynthesis and nitrogen relationships in leaves of C3 plants. Oecologia 78, 9–19. doi: 10.1007/BF00377192
Farquhar, G. D., von Caemmerer, S. V., Berry, J. A. (1980). A biochemical model of photosynthetic CO2 assimilation in leaves of C3 species. Planta 149, 78–90. doi: 10.1007/BF00386231
Feng, Y. L., Fu, G. L., Zheng, Y. L. (2008). Specific leaf area relates to the differences in leaf construction cost, photosynthesis, nitrogen allocation, and use efficiencies between invasive and noninvasive alien congeners. Planta 228, 383–390. doi: 10.1007/s00425-008-0732-2
Field, C. (1983). Allocating leaf nitrogen for the maximization of carbon gain: leaf age as a control on the allocation program. Oecologia 56, 341–347. doi: 10.1007/BF00379710
Ghannoum, O., Caemmerer, S. V., Ziska, L. H., Conroy, J. P. (2000). The growth response of C4 plants to rising atmospheric CO2 partial pressure: a reassessment. Plant Cell Environ. 23, 931–942. doi: 10.1046/j.1365-3040.2000.00609.x
Ghannoum, O., Evans, J. R., Chow, W. S., Andrews, T. J., Conroy, J. P., von Caemmerer, S. (2005). Faster Rubisco is the key to superior nitrogen-use efficiency in NADP-malic enzyme relative to NAD-malic enzyme C4 grasses. Plant Physiol. 137, 638–650. doi: 10.1104/pp.104.054759
Glazier, D. S. (2018). Resource supply and demand both affect metabolic scaling: a response to Harrison. Trends Ecol. Evol. 33, 237–238. doi: 10.1016/j.tree.2018.01.006
Halpern, M., Bar-Tal, A., Lugassi, N., Egbaria, A., Granot, D., Yermiyahu, U. (2019). The role of nitrogen in photosynthetic acclimation to elevated [CO2] in tomatoes. Plant Soil 434, 397–411. doi: 10.1007/s11104-018-3857-5
Hikosaka, K., Hirose, T. (1998). Leaf and canopy photosynthesis of C3 plants at elevated CO2 in relation to optimal partitioning of nitrogen among photosynthetic components: theoretical prediction. Ecol. Model. 106, 247–259. doi: 10.1016/S0304-3800(97)00198-1
Hohmann-Marriott, M. F., Blankenship, R. E. (2011). Evolution of photosynthesis. Annu. Rev. Plant Biol. 62, 515–548. doi: 10.1146/annurev-arplant-042110-103811
Hu, Y. K., Pan, X., Liu, G. F., Li, W. B., Dai, W. H., Tang, S. L., et al. (2015). Novel evidence for within-species leaf economics spectrum at multiple spatial scales. Front. Plant Sci. 6:901. doi: 10.3389/fpls.2015.00901
Huber, S. C., Sugiyama, T., Alberte, R. S. (1989). Photosyntheticdeterminantsof growth in maizeplants: effects of nitrogen nutrition on growth, carbon fixation and photochemical feature. Plant Cell Physiol. 30, 1063–1072. doi: 10.1093/oxfordjournals.pcp.a077846
Imai, K., Suzuki, Y., Makino, A., Mae, T. (2005). Effects of nitrogen nutrition on the relationships between the levels of rbcS and rbcL mRNAs and the amount of ribulose 1·5-bisphosphate carboxylase/oxygenase synthesized in the eighth leaves of rice from emergence through senescence. Plant Cell Environ. 28, 1589–1600. doi: 10.1111/j.1365-3040.2005.01438.x
Imai, K., Suzuki, Y., Mae, T., Makino, A. (2008). Changes in the synthesis of rubisco in rice leaves in relation to senescence and N influx. Ann. Bot. 101, 135–144. doi: 10.1093/aob/mcm270
Ishimaru, K., Kobayashi, N., Ono, K., Yano, M., Ohsugi, R. (2001). Are contents of Rubisco, soluble protein and nitrogen in flag leaves of rice controlled by the same genetics? J. Exp. Bot. 52, 1827–1833. doi: 10.1093/jexbot/52.362.1827
Ishizuka, M., Makino, A., Suzuki, Y., Mae, T. (2004). Amount of Ribulose-1,5-bisphosphate carboxylase/oxygenase (Rubisco) Protein and levels of mRNAs of rbcS and rbcL in the leaves at different positions in transgenic rice plants with decreased content of Rubisco. Soil Sci Plant Nutr. 50, 233–239. doi: 10.1080/00380768.2004.10408472
Jordan, D. B., Ogren, W. L. (1981). Species variation in the specificity of ribulose biphosphate carboxylase/oxygenase. Nature 291, 513–515. doi: 10.1038/291513a0
Kanai, R., Edwards, G. E. (1999). “The biochemistry of C4 photosynthesis,” in C4 Plant Biololgy UK. Eds. Sage, R. F., Monson, R. K. (London: Academic Press), 49–87.
Kelly, S. (2018). The amount of nitrogen used for photosynthesis modulates molecular evolution in plants. Mol. Biol. Evol. 35, 1616–1625. doi: 10.1093/molbev/msy043
Lattanzi, F. A., Schnyder, H., Thornton, B. (2005). The sources of carbon and nitrogen supplying leaf growth. Assessment of the role of stores with compartmental models. Plant Physiol. 137, 383–395. doi: 10.1104/pp.104.051375
Laza, R. C., Bergan, B., Vergara, B. S. (1993). Cultivar differences in growth and chloroplast ultrastructure in rice as affected by nitrogen. J. Exp. Bot. 44, 1643–1648. doi: 10.1093/jxb/44.11.1643
Leakey, A. D., Ainsworth, E. A., Bernacchi, C. J., Rogers, A., Long, S. P., Ort, D. R. (2009). Elevated CO2 effects on plant carbon, nitrogen, and water relations: six important lessons from FACE. J. Exp. Bot. 60, 2859–2876. doi: 10.1093/jxb/erp096
LeBauer, D. S., Treseder, K. K. (2008). Nitrogen limitation of net primary productivity in terrestrial ecosystems is globally distributed. Ecology 89, 371–379. doi: 10.1890/06-2057.1
Liberloo, M., Dillen, S. Y., Calfapietra, C., Marinari, S., Luo, Z. B., De Angelis, B., et al. (2005). Elevated CO2 concnetration, fertilization and their interaction: growth stimulation in a short-rotation poplar coppice (EUROFACE). Tree Physiol. 25, 179–189. doi: 10.1093/treephys/25.2.179
Liu, T., Ren, T., White, P. J., Cong, R., Lu, J. (2018). Storage nitrogen co-ordinates leaf expansion and photosynthetic capacity in winter oilseed rape. J. Exp. Bot. 69, 2995–3007. doi: 10.1093/jxb/ery134
Long, S. P., Zhu, X. G., Naidu, S. L., Ort, D. R. (2006). Can improvement in photosynthesis increase crop yields? Plant Cell Environ. 29, 315–330. doi: 10.1111/j.1365-3040.2005.01493.x
Mae, T., Kai, N., Makino, A., Ohira, K. (1984). Relation between ribulose bisphosphate carboxylase content and chloroplast number in naturally senescing primary leaves of wheat. Plant Cell Physiol. 25, 333–336. doi: 10.1093/oxfordjournals.pcp.a076718
Makino, Y., Ueno, O. (2018). Structural and physiological responses of the C4 grass Sorghum bicolor to nitrogen limitation. Plant Prod. Sci. 21, 39–50. doi: 10.1080/1343943X.2018.1432290
Makino, A., Mae, T., Ohira, K. (1984). Relation between nitrogen and ribulose-1,5-bisphosphate carboxylase in rice leaves from emergence through senescence. Plant Cell Physiol. 25, 429–437. doi: 10.1093/oxfordjournals.pcp.a076730
Makino, A., Mae, T., Ohira, K. (1988). Differences between wheat and rice in the enzymic properties of ribulose-1, 5-bisphosphate carboxylase/oxygenase and the relationship to photosynthetic gas exchange. Planta 174, 30–38. doi: 10.1007/BF00394870
Makino, A., Sakashita, H., Hidema, J., Mae, T., Ojima, K., Osmond, B. (1992). Distinctive responses of ribulose-1, 5-bisphosphate carboxylase and carbonic anhydrase in wheat leaves to nitrogen nutrition and their possible relationships to CO2-transfer resistance. Plant Physiol. 100, 1737–1743. doi: 10.1104/pp.100.4.1737
Makino, A., Shimada, T., Takumi, S., Kaneko, K., Matsuoka, M., Shimamoto, M., et al. (1997). Does decrease in ribulose-1,5-bisphosphate carboxylase by antisense rbcS lead to a higher N-use efficiency of photosynthesis under saturation CO2 and light in rice plants? Plant Physiol. 114, 483–491. doi: 10.1104/pp.114.2.483
Makino, A., Sakuma, H., Sudo, E., Mae, T. (2003). Differences between maize and rice in N-use efficiency for photosynthesis and protein allocation. Plant Cell Physiol. 44, 952–956. doi: 10.1093/pcp/pcg113
Makino, A. (2003). Rubisco and nitrogen relationships in rice: leaf photosynthesis and plant growth. Soil Sci. Plant Nutr. 49, 319–327. doi: 10.1080/00380768.2003.10410016
Maranville, J. W., Madhavan, S. (2002). Physiological adaptation for nitrogen use efficiency in sorghum. Plant Soil 245, 25–34. doi: 10.1007/978-94-017-1570-6_10
Meinzer, F. C., Saliendra, N. Z. (1997). Spatial patterns of carbon isotope discrimination and allocation of photosynthetic activity in sugarcane leaves. Funct. Plant Biol. 24, 769–775. doi: 10.1071/PP97021
Michaelis, L., Menten, M. L. (1913). The kinetics of the inversion effect. Biochem. Z 49, 333–369. doi: 10.1021/bi2012844
Miyagawa, Y., Tamoi, M., Shigeoka, S. (2001). Overexpression of a cynobacterial fructose-1,6-bisphosphatase in tobacco enhances photosynythesis and growth. Nat. Biotechnol. 19, 965–969. doi: 10.1038/nbt1001-965
Mu, X., Chen, Q., Chen, F., Yuan, L., Mi, G. (2016). Within-leaf nitrogen allocation in adaptation to low nitrogen supply in maize during grain-filling stage. Front. Plant Sci. 7, 699. doi: 10.3389/fpls.2016.00699
Nagaraj, R., Sharpley, M. S., Chi, F., Braas, D., Zhou, Y., Kim, R., et al. (2017). Nuclear localization of mitochondrial TCA cycle enzymes as a critical step in mammalian zygotic genome activation. Cell 168, 210–223. doi: 10.1016/j.cell.2016.12.026
Nakano, H., Makino, A., Mae, T. (1995). Effects of panicle removal on the photosynthetic characteristics of the flag leaf of rice plants during the ripening stage. Plant Cell Physiol. 36, 653–659. doi: 10.1093/oxfordjournals.pcp.a078806
Nakano, H., Makino, A., Mae, T. (1997). The effect of elevated partial pressures of CO2 on the relationship between photosynthetic capacity and N content in rice leaves. Plant Physiol. 115, 191–198. doi: 10.1104/pp.115.1.191
Nie, G., Long, S. P., Garcia, R., Kimball, B., Lamorte, R., Pinter, P., et al. (1995). Effects of free-air CO2 enrichment on the development of the photosynthetic apparatus in wheat, as indicated by changes in leaf proteins. Plant Cell Environ. 18, 855–864. doi: 10.1111/j.1365-3040.1995.tb00594.x
Niinemets, Ü., Keenan, T. F., Hallik, L. (2015). A worldwide analysis of within-canopy variations in leaf structural, chemical and physiological traits across plant functional types. New Phytol. 205, 973–993. doi: 10.1111/nph.13096
Nose, A., Uehara, M., Kawamitsu, Y. (1994). Variations in leaf gas exchange traits of saccharum including feral sugarcane, Saccharum spontaneum L. Jpn. J. Crop Sci. 63, 489–495. doi: 10.1626/jcs.63.489
Onoda, Y., Schieving, F., Anten, N. P. (2008). Effects of light and nutrient availability on leaf mechanical properties of Plantago major: a conceptual approach. Ann. Bot. 101, 727–736. doi: 10.1093/aob/mcn013
Onoda, Y., Wright, I. J., Evans, J. R., Hikosaka, K., Kitajima, K., Niinemets, Ü., et al. (2017). Physiological and structural tradeoffs underlying the leaf economics spectrum. New Phytol. 214, 1447–1463. doi: 10.1111/nph.14496
Osada, N., Onoda, Y., Hikosaka, K. (2010). Effects of atmospheric CO2 concentration, irradiance, and soil nitrogen availability on leaf photosynthetic traits of Polygonum sachalinense around natural CO2 springs in northern Japan. Oecologia 164, 41–52. doi: 10.1007/s00442-010-1635-z
Osnas, J. L., Katabuchi, M., Kitajima, K., Wright, S. J., Reich, P. B., Van Bael, S. A., et al. (2018). Divergent drivers of leaf trait variation within species, among species, and among functional groups. Proc. Natl. Acad. Sci. U.S. A. 115, 5480–5485. doi: 10.1073/pnas.1803989115
Pantin, F., Simonneau, T., Rolland, G., Dauzat, M., Muller, B. (2011). Control of leaf expansion: a developmental switch from metabolics to hydraulics. Plant Physiol. 156, 803–815. doi: 10.1104/pp.111.176289
Paponov, I. A., Engels, C. (2003). Effect of nitrogen supply on leaf traits related to photosynthesis during grain filling in two maize genotypes with different N efficiency. J. Plant Nutr. Soil Sci. 166, 756–763. doi: 10.1002/jpln.200320339
Poorter, H., Evans, J. R. (1998). Photosynthetic nitrogen-use efficiency of species that differ inherently in specific leaf area. Oecologia 116, 26–37. doi: 10.1007/s004420050560
Posada, D., Buckley, T. R. (2004). Model selection and model averaging in phylogenetics: Advantages of Akaike information criterion and Bayesian approaches over likelihood ratio tests. Syst. Biol. 53, 793–808. doi: 10.1080/10635150490522304
Prasad, P. V., Vu, J. C., Boote, K. J., Allen, L. H. (2009). Enhancement in leaf photosynthesis and upregulation of Rubisco in the C4 sorghum plant at elevated growth carbon dioxide and temperature occur at early stages of leaf ontogeny. Funct. Plant Biol. 36, 761–769. doi: 10.1071/FP09043
P’yankov, V., Ivanov, L., Lambers, H. (2001). Plant construction cost in the boreal species differing in their ecological strategies. Russ. J. Plant Physiol. 48, 67–73. doi: 10.1023/A:1009002715572
Rotundo, J. L., Cipriotti, P. A. (2017). Biological limits on nitrogen use for plant photosynthesis: a quantitative revision comparing cultivated and wild species. New Phytol. 214, 120–131. doi: 10.1111/nph.14363
Sage, R. F., Pearcy, R. W. (1987). The nitrogen use efficiency of C3 and C4 plants. II. Leaf nitrogen effects on the gas exchange characteristics of Chenopodium album (L.) and Amaranthus retroflexus (L.). Plant Physiol. 84, 959–963. doi: 10.1104/pp.84.3.959
Sage, R. F., Zhu, X. G. (2011). Exploiting the engine of C4 photosynthesis. J. Exp. Bot. 62, 2989–3000. doi: 10.1093/jxb/err179
Sage, R. F., Pearcy, R. W., Seemann, J. R. (1987). The nitrogen use efficiency of C3 and C4 plants. III. Leaf nitrogen effects on the activity of carboxylating C3 an C4 enzymes in Chenopodium Album (L.) and Amaranthus retroflexus (L.). Plant Physiol. 85, 355–359. doi: 10.1104/pp.85.2.355
Sage, R. F. (2002). Variation in the k cat of Rubisco in C3 and C4 plants and some implications for photosynthetic performance at high and low temperature. J. Exp. Bot. 53, 609–620. doi: 10.1093/jexbot/53.369.609
Sakai, H., Hasegawa, T., Kobayashi, K. (2006). Enhancement of rice canopy carbon gain by elevated CO2 is sensitive to growth stage and leaf nitrogen concentration. New Phytol. 170, 321–332. doi: 10.1111/j.1469-8137.2006.01688.x
Sardans, J., Peñuelas, J. (2015). Potassium: a neglected nutrient in global change. Glob. Ecol. Biogeogr. 24, 261–275. doi: 10.1111/geb.12259
Sesták, Z. (2012). Photosynthesis during leaf development Vol. 11 (Springer Science & Business Media), 396. doi: 10.1007/978-94-009-5530-1
Sheen, J. (1999). C4 gene expression. Annu. Rev. Plant Biol. 50, 187–217. doi: 10.1146/annurev.arplant.50.1.187
Sinclair, T. R., Horie, T. (1989). Leaf nitrogen, photosynthesis, and crop radiation use efficiency. Rev. Crop Sci. 29, 90–98. doi: 10.2135/cropsci1989.0011183X002900010023x
Sudo, E., Makino, A., Mae, T. (2003). Differences between rice and wheat ribulose-1,5-bisphosphate regeneration capacity per unit of leaf-N content. Plant Cell Environ. 26, 255–263. doi: 10.1046/j.1365-3040.2003.00955.x
Sugiyama, T., Mizuno, M., Hayashi, M. (1984). Partitioning of nitrogen among ribulose-1, 5-bisphosphate carboxylase/oxygenase, phosphoenolpyruvate carboxylase, and pyruvate orthophosphate dikinase as related to biomass productivity in maize seedlings. Plant Physiol. 75, 665–669. doi: 10.1104/pp.75.3.665
Suzuki, Y., Nakamoto, H., Ku, M. S. B., Edwards, G. E. (1987). Influence of leaf age on photosynthesis, enzyme activity, and metabolite levels in wheat. Plant Physiol. 84, 1244–1248. doi: 10.1104/pp.84.4.1244
Suzuki, I., Cretin, C., Omata, T., Sugiyama, T. (1994). Transcriptional and posttranscriptional regulation of nitrogen-responding expression of phosphoenolpyruvate carboxylase gene in maize. Plant Physiol. 105, 1223–1229. doi: 10.1104/pp.105.4.1223
Suzuki, Y., Makino, A., Mae, T. (2001). Changes in the turnover of Rubisco and levels of mRNAs of rbcL and rbcS in leaves of emergence to senescence. Plant Cell Environ. 24, 1353–1360. doi: 10.1046/j.0016-8025.2001.00789.x
Terashima, I., Hanba, Y. T., Tazoe, Y., Vyas, P., Yano, S. (2005). Irradiance and phenotype: comparative eco-development of sun and shade leaves in relation to photosynthetic CO2 diffusion. J. Exp. Bot. 57, 343–354. doi: 10.1093/jxb/erj014
Uribelarrea, M., Crafts-Brandner, S. J., Below, F. E. (2009). Physiological N response of field-grown maize hybrids (Zea mays L.) with divergent yield potential and grain protein concentration. Plant Soil 316, 151. doi: 10.1007/s11104-008-9767-1
Usuda, H., Ku, M. S. B., Edwards, G. E. (1984). Rates of photosynthesisrelative to activity of photosynthetic enzymes, chlorophyll and soluble protein content among ten C4 species. Aust. J. Plant Physiol. 11, 509–517. doi: 10.1071/PP9840509
Usuda, H. (1984). Variations in the photosynthesis rate and activity of photosynthetic enzymes in maize leaf tissue of different ages. Plant Cell Physiol. 25, 1297–1301. doi: 10.1093/oxfordjournals.pcp.a076838
Vogan, P. J., Sage, R. F. (2011). Water-use efficiency and nitrogen-use efficiency of C3-C4 intermediate speciesof Flaveria juss. (Asteracea). Plant Cell Environ. 34, 1415–1430. doi: 10.1111/j.1365-3040.2011.02340.x
Von Caemmerer, S., Millgate, A., Farquhar, G. D., Furbank, R. T. (1997). Reduction of ribulose-1, 5-bisphosphate carboxylase/oxygenase by antisense RNA in the C4 plant Flaveria bidentis leads to reduced assimilation rates and increased carbon isotope discrimination. Plant Physiol. 113, 469–477. doi: 10.1104/pp.113.2.469
Von Caemmerer, S. (2000). Biochemical models of leaf photosynthesis (Csiro publishing). doi: 10.1071/9780643103405
Warren, C. R., Dreyer, E., Adams, M. A. (2003). Photosynthesis-Rubisco relationships in foliage of Pinus sylvestris in response to nitrogen supply and the proposed role of Rubisco and amino acids as nitrogen stores. Trees 17, 359–366. doi: 10.1007/s00468-003-0246-2
Westbeek, M. H., Pons, T. L., Cambridge, M. L., Atkin, O. K. (1999). Analysis of differences in photosynthetic nitrogen use efficiency of alpine and lowland Poa species. Oecologia 120, 19–26. doi: 10.1007/s004420050828
Wong, S. C., Cowan, I. R., Farquhar, G. D. (1985). Leaf conductance in relation to rate of CO2 assimilation. I. Influence of nitrogen nutrition, phosphorus nutrition, photon flux density, and ambient partial pressure of CO2 during ontogeny. Plant Physiol. 78, 821–825. doi: 10.1104/pp.78.4.821
Wyka, T. P., Żytkowiak, R., Oleksyn, J. (2016). Seasonal dynamics of nitrogen level and gas exchange in different cohorts of Scots pine needles: a conflict between nitrogen mobilization and photosynthesis? Eur. J. For. Res. 135, 483–493. doi: 10.1007/s10342-016-0947-x
Xu, G., Fan, X., Miller, A. J. (2012). Plant nitrogen assimilation and use efficiency. Annu. Rev. Plant Biol. 63, 153–182. doi: 10.1146/annurev-arplant-042811-105532
Yabiku, T., Ueno, O. (2017). Variations in physiological, biochemical, and structural traits of photosynthesis and resource use efficiency in maize and teosintes (NADP-ME-type C4). Plant Prod. Sci. 20, 448–458. doi: 10.1080/1343943x.2017.1398050
Yamaoka, C., Suzuki, Y., Makino, A. (2016). Differential expression of genes of the Calvin-Benson cycle and its related genes during leaf development in rice. Plant Cell Physiol. 57, 115–124. doi: 10.1093/pcp/pcv183
Yamori, W., Takahashi, S., Makino, A., Price, G. D., Badger, M. R., von Caemmerer, S. (2011). The roles of ATP synthase and the cytochrome b6/f complexes in limiting chloroplast electron transport and determining photosynthetic capacity. Plant Physiol. 155, 956–962. doi: 10.1104/pp.110.168435
Yasumura, Y., Ishida, A. (2011). Temporal variation in leaf nitrogen partitioning of a broad-leaved evergreen tree. Quercus Myrsinaefolia J. Plant Res. 124, 115–123. doi: 10.1007/s10265-010-0358-x
Ye, Z. P., Robakowski, P., Suggett, D. J. (2013). A mechanistic model for the light response of photosynthetic electron transport rate based on light harvesting properties of photosynthetic pigment molecules. Planta 237, 837–847. doi: 10.1007/s00425-012-1790-z
Young, J. N., Heureux, A. M. C., Sharwood, R. E., Rickaby, R. E. M., Morel, F. M. M., Whitney, S. M. (2016). Large variation in the Rubisco kinetics of diatoms reveals diversity among their carbon-concentrating mechanisms. J. Exp. Bot. 67, 3445–3456. doi: 10.1093/jxb/erw163
Keywords: photosynthetic rate, leaf nitrogen content, crop plants, storage nitrogen, nitrogen absorption, CO2 concentrations, photosynthetic nitrogen use efficiency, nitrogen use efficiency
Citation: Khan A, Wang Z, Xu K, Li L, He L, Hu H and Wang G (2020) Validation of an Enzyme-Driven Model Explaining Photosynthetic Rate Responses to Limited Nitrogen in Crop Plants. Front. Plant Sci. 11:533341. doi: 10.3389/fpls.2020.533341
Received: 07 February 2020; Accepted: 09 September 2020;
Published: 25 September 2020.
Edited by:
Robert Peter Walker, University of Perugia, ItalyReviewed by:
Robert Edward Sharwood, Australian National University, AustraliaXinguang Zhu, Center of Excellence for Molecular Plant Sciences and State Key Laboratory of Plant Molecular Genetics (CAS), China
Copyright © 2020 Khan, Wang, Xu, Li, He, Hu and Wang. This is an open-access article distributed under the terms of the Creative Commons Attribution License (CC BY). The use, distribution or reproduction in other forums is permitted, provided the original author(s) and the copyright owner(s) are credited and that the original publication in this journal is cited, in accordance with accepted academic practice. No use, distribution or reproduction is permitted which does not comply with these terms.
*Correspondence: Genxuan Wang, d2FuZ2d4QHpqdS5lZHUuY24=