- 1State Key Laboratory of Crop Genetics and Germplasm Enhancement, MOA Key Laboratory of Plant Nutrition and Fertilization in Low-Middle Reaches of the Yangtze River, Nanjing Agricultural University, Nanjing, China
- 2CAAS-IRRI Joint Laboratory for Genomics-Assisted Germplasm Enhancement, Agricultural Genomics Institute in Shenzhen, Chinese Academy of Agricultural Sciences, Shenzhen, China
- 3Department of Agronomy, Purdue University, West Lafayette, IN, United States
The NO3- transporter plays an important role in rice nitrogen acquisition and nitrogen-use efficiency. Our previous studies have shown that the high affinity systems for nitrate uptake in rice is mediated by a two-component NRT2/NAR2 transport system. In this study, transgenic plants were successful developed by overexpression of OsNAR2.1 alone, OsNRT2.3a alone and co-overexpression of OsNAR2.1 and OsNRT2.3a. Our field experiments indicated that transgenic lines expressing p35S:OsNAR2.1 or p35S:OsNAR2.1-p35S:OsNRT2.3a constructs exhibited increased grain yields of approximately 14.1% and 24.6% compared with wild-type (cv. Wuyunjing 7, WT) plants, and the agricultural nitrogen use efficiency increased by 15.8% and 28.6%, respectively. Compared with WT, the 15N influx in roots of p35S:OsNAR2.1 and p35S: OsNAR2.1-p35S:OsNRT2.3a lines increased 18.9%‑27.8% in response to 0.2 mM, 2.5 mM 15NO3–, and 1.25 mM 15NH415NO3, while there was no significant difference between p35S:OsNAR2.1 and p35S:OsNAR2.1-p35S:OsNRT2.3a lines; only the 15N distribution ratio of shoot to root for p35S:OsNAR2.1-p35S:OsNRT2.3a lines increased significantly. However, there were no significant differences in nitrogen use efficiency, 15N influx in roots and the yield between the p35S:NRT2.3a transgenic lines and WT. This study indicated that co-overexpression of OsNAR2.1 and OsNRT2.3a could increase rice yield and nitrogen use efficiency.
Introduction
Nitrogen (N) is an essential macronutrient for plant growth and crop productivity, it affects all levels of plant function from metabolism to resource allocation, growth, and development (Crawford, 1995; Scheible et al., 2004). Nitrate nitrogen (NO3–) and ammonium nitrogen (NH4+) are two main inorganic nitrogen sources in plant growth. Conventionally cultivated rice is submerged, and nitrification is inhibited, NH4+ is the main inorganic nitrogen in rhizosphere soil, therefore, rice is generally considered as ammonium-preferring plant (Arth et al., 1998; Kronzucker et al., 1999). However, for its well-developed aerial tissue, rice can transport and secrete oxygen from photosynthesis above ground to the rhizosphere (Aurelio et al., 2003). Oxygen can stimulate the growth and reproduction of nitrifying bacteria in the rhizosphere, so the part of NH4+ in the rhizosphere can be nitrified into NO3– (Li et al., 2008). Therefore, in the actual growth of rice, its root system has been in the mixed nutrition of NH4+ and NO3– (Kirk and Kronzucker, 2005). Aurelio et al. (2003) found that most rice varieties could absorb the same amount of NH4+ and NO3–, and the amount of NO3– absorbed by rice would be much larger than that of NH4+ because of the strong nitrification in rhizosphere. During the late stage of rice growth and development, when it has been going through the process of long-term alternate wetting and drying irrigation or the whole growth stage of upland rice, the uptake of NO3– was also higher than that of NH4+ by rice (Arth et al., 1998; Wang et al., 2004).
In addition to be a nutrient, NO3– also serves as a signaling molecule, which induces changes in plant growth and gene expression (Kirk and Kronzucker, 2005; Liu et al., 2015). For example, NO3– could break seed dormancy (Alboresi et al., 2005), and induced multiple genes for plant growth and development (O’Brien et al., 2016). Furthermore, the development of lateral root, leaf growth and altering flowering time could be regulated by NO3–(Zhang et al., 1999; Rahayu et al., 2005; Castro et al., 2011; Hsu and Tsay, 2013; Naz et al., 2019). NO3– could be sensed by the plant, and the uptake of NO3–, especially in rice, can increase the uptake of NH4+ to a certain extent (Duan et al., 2006; Chen et al., 2017).
Two different NO3– uptake systems in plants, the high-affinity NO3– uptake systems (HATS) and low-affinity NO3– uptake systems (LATS) are regulated by NO3– supply and enable plants to cope with low or high NO3– concentrations in soils (Miller et al., 2007; Xu et al., 2012). As we known, the NRT2 and NPF families contribute to HATS and LATS responding the NO3– uptake and translocation in plants (Miller et al., 2007; Léran et al., 2014). Some high-affinity NO3– transporters belonging to the NRT2 family have been reported to require a partner protein, NAR2, for their function (Tong et al., 2005; Yong et al., 2010; Xu et al., 2012). In rice, two-component NRT2-NAR2 system also exists in the NO3– transport process, and OsNAR2.1 is the essential partner of OsNRT2 nitrate transporters for nitrate uptake over low and high concentration range (Miller et al., 2007; Feng et al., 2011; Liu et al., 2015). OsNRT2.1, OsNRT2.2, and OsNRT2.3a were similarly shown to require OsNAR2.1 for NO3– uptake, and their interaction at the protein level was demonstrated by using a yeast two hybrid assay, an oocyte expression system and western blotting (Feng et al., 2011; Yan et al., 2011; Liu et al., 2014).
Previous studies have shown that pOsNAR2.1:OsNAR2.1 expression or pOsNAR2.1:OsNAR2.1 expression increased the expression of OsNRT2.1 and OsNAR2.1, and OsNRT2.1 or OsNAR2.1 driven by OsNAR2.1 promote can improve nitrate uptake and final grain yield in rice (Chen et al., 2016; Chen et al., 2017). Yan et al. (2011) reported that knockdown of OsNAR2.1 could suppress the expression of OsNRT2.1, OsNRT2.2, and OsNRT2.3a in rice roots, and then reduced nitrate uptake and affected its growth. OsNRT2.1 and OsNRT2.2 are responsible for NO3− uptake from the soil (Katayama et al., 2009; Fan et al., 2017). OsNRT2.3a is a root-to-shoot NO3− transporter (Tang et al., 2012). However, overexpression of OsNRT2.3a alone did not increase nitrogen uptake and transport in rice (Fan et al., 2016). In this study, we focus on co-overexpressed OsNAR2.1 and OsNRT2.3a in rice, overexpression of OsNAR2.1 or OsNRT2.3a alone as control and explored the effect of co-overexpression of OsNAR2.1 and OsNRT2.3a on NO3– uptake, yield and nitrogen use efficiency uptake by rice.
Materials and Methods
Construction of Vectors and Rice Transformation
The open reading frames (ORF) of OsNAR2.1 (AP004023) and OsNRT2.3a (AK109776) were amplified from the full-length cDNA of rice cv. Nipponbare, and the primer sequences were shown in Supplementary Table S1. PrimeSTAR HS DNA Polymerase (TaKaRa Biotechnology Co., Ltd, Dalian, China) was used to amplify the ORF of OsNAR2.1 and OsNRT2.3a, and the two-step PCR parameters were 95 °C for 5 min followed by 98 °C for 10 s, 68 °C for 2 min (30 cycles) and 72 °C for 10 min. Full-length coding sequences of OsNAR2.1 was amplified subcloned into the pSAT4.35SP vector. After PI-PspI digestion of pSAT4.35SP-OsNAR2.1, the fragment was cloned into the PI-PspI site of vector pRCS2-ocs-nptII (Goodin et al., 2007) in the antisense orientation and got OsNAR2.1-pRCS2-ocs-nptII, as p35S:OsNAR2.1, then confirmed by gene sequencing. The ORF of OsNRT2.3a was subcloned into the pSAT6.supP vector. After I-SceI digestion of pSAT6.supP-OsNRT2.3a, the fragment was cloned into the I-SceI site of vector pRCS2-ocs-nptII and got NRT2.3a-pRCS2-ocs-nptII, as p35S:OsNRT2.3a, confirmed by gene sequencing. For the construction of co-overexpression of OsNAR2.1 and OsNRT2.3a (p35S:OsNAR2.1-p35S:OsNRT2.3a), we used I-SceI for digestion of NRT2.3a-pRCS2-ocs-nptII construction, the fragment was cloned into the I-SceI site of construction NAR2.1-pRCS2-ocs-nptII. The vectors were introduced into Agrobacterium tumefaciens strain EHA105 by electroporation and then transformed into rice as described previously (Tang et al., 2012).
qRT-PCR and Southern Blot Analysis
The total RNA extraction of plants and genes expression analysis were performed as described previously (Chen et al., 2016; Chen et al., 2017). The primers for PCR are shown in Table S2.
The Southern blot was used to identify the copy number of inserted T-DNA. The genomic DNA extraction of the T2 plant, DNA digestion and hybridization were following the reported paper (Chen et al., 2017).
Field Experiments for Harvest Yield
We selected the positive T1 transgenic lines by PCR. The sequence of the resistance screening gene (792 bp) on the vector was amplified by forward primer (5’ ATGATTGAACAAGATGGATTGCA 3’) and reverse primer (5’ GAAGAACTCGTCAAGAAGGCGAT 3’). The Southern blot was used to identify the copy number of inserted T-DNA of the T2 plant, we chose the single-copy T2 lines and planted them to screen T3 and T4 generation plants with stable inheritance. T1, T2, and T4 generation plants were grown in plots at the Nanjing Agricultural University from June to October in 2013, 2014, and 2016 in Nanjing, Jiangsu. The rice plants of T3 generation plants were cultivated in plots at the Experimental Site of Zhejiang University June to October 2015 in Changxing, Zhejiang. Soil properties in Nanjing field experiment was described as before (Chen et al., 2016; Chen et al., 2017). Basal applications of 30 kg P/ha as Ca(H2PO4)2 and 60 kg/K ha (KCl) were made to all plots 3 days before transplanting. N fertilizer as urea accounted for 40%, 30% and 40% of the total N fertilizer was applied prior to transplanting, at tillering, just before the heading stage, respectively.
T1‑T2 generation transgenic plants and wild-type (cv. Wuyunjing 7, WT) plants were planted in the plots with 300 kg N/ha. T3 generation transgenic plants and WT plants were planted in 3 plots with 150 kg N/ha and the plots without nitrogen fertilizer as blank control. T4 generation transgenic plants and WT plants were planted in 3 plots with 300 kg N/ha and the plots without nitrogen fertilizer as blank control. T2 generation transgenic plants of cv. Nipponbare and wild-type (cv. Nipponbare, NP) plants were planted in 3 plots with 150 kg N/ha and the plots without nitrogen fertilizer as blank control. The plots size was 2 × 2 m, and the seedlings were planted in a 10 × 10 array. Compared with WT, the flowering period of p35S:OsNAR2.1 lines were 4‑5 days earlier, that of p35S:OsNRT2.3a lines had no significant change, and that of p35S:OsNAR2.1-p35S:OsNRT2.3a lines were 2‑3 days earlier. During rice flowering and mature stages, the samples of T4 generation transgenic plants and WT were collected for further analysis.
Dry Weight, Total Nitrogen Measurement, and Calculation of Nitrogen Use Efficiency
We conducted biomass and nitrogen analysis of the T4 generation shoot samples grown under 300 kg N/ha fertilizer condition according to our previous reported method (Chen et al., 2016). Dry matter at anthesis stage (DMA), grain yield (GY), dry matter at maturity stage (DMM), total nitrogen accumulation at anthesis stage (TNAA), grain nitrogen accumulation at maturity stage (GNAM), total nitrogen accumulation at maturity stage (TNAM), harvest index (HI), the contribution of pre-anthesis assimilates to grain yield (CPAGY), dry matter translocation (DMT), dry matter translocation efficiency (DMTE), contribution of pre-anthesis nitrogen to grain nitrogen accumulation (CPNGN), nitrogen translocation (NT), nitrogen translocation efficiency (NTE), nitrogen harvest index (NHI), agronomic nitrogen use efficiency (ANUE), physiological nitrogen use efficiency (PNUE), and nitrogen recovery efficiency (NRE) were calculated according to Chen et al. (2016; 2017). CPAGY (%) = (DMT/GY) × 100%; HI (%) = (GY/DMM) × 100%; DMT (kg/ha) = DMA – (DMM – GY); DMTE (%) = (DMT/DMA) × 100%; CPNGN (%) = (NT/GNAM) × 100%; NT (kg/ha) = TNAA – (TNAM – GNAM); NTE (%) = (NT/TNAA) × 100%; ANUE (kg/kg) = (GY – GY of zero-N plot)/N supply; PNUE (kg/kg) = (GY – GY of zero-N plot)/TNAM; NRE (%) = (TNAM –TNAM of zero-N plot)/N supply.
Determination of Total N Content, Root 15N Influx Rate, and 15N Distribution Ratio
WT and transgenic rice seedlings were grown in IRRI solution containing 1 mM NH4+ for 2 weeks, and then transferred into three different forms of nitrogen treatments including in 0.2 mM NO3–, 2.5 mM NO3–, and 1.25 mM NH4NO3. The full strength IRRI solution used has the following compositions: 1.0 mM MgSO4·7H2O, 1.25 mM NH4NO3, 0.3 mM KH2PO4, 1.0 mM CaCl2, 0.35 mM K2SO4, 0.5 mM Na2SiO3, 20.0 μM Fe-EDTA, 20.0 μM H3BO3, 9.0 μM MnCl2, 0.77 μM ZnSO4, 0.32 μM CuSO4, and 0.39 μM (NH4)6Mo7O24, pH 5.5. The nutrient solution was replaced every 3 days and the pH was adjusted to 5.5 every day. Plants were grown in a growth room with a 14 h light (30°C) (8:00‑22:00)/10 h dark (22°C) (22:00‑8:00) and 60% relative humidity. After 3-week treatment, the biomass and nitrogen concentration of every line under each N treatment were measured.
For root 15N uptake experiment, rice seedlings were grown in 1mM NH4+ for 3 weeks and then under nitrogen starvation condition for 1 week before 15N uptake experiment. The plants were rinsed in 0.1 mM CaSO4 for 1 min, then transferred to a solution containing either 0.2 mM 15NO3–, 2.5 mM 15NO3– or 1.25 mM 15NH415NO3 (atom % 15N: 99%) for 5 min, and finally rinsed again in 0.1 mM CaSO4 for 1 min.
For root 15N distribution ratio experiment, WT and transgenic seedlings were grown in 1 mM NH4+ for 3 weeks, nitrogen starvation was then carried out for 1 week. The plants were rinsed in 0.1 mM CaSO4 for 1 min, then transferred to the nutrient solution containing 0.2 mM 15NO3–, or 1.25 mM 15NH415NO3 for 12 h, and finally rinsed again in 0.1 mM CaSO4 for 1 min. The 15N influx rate and 15N distribution ratio were calculated following the method reported in Chen et al. (2017) and Tang et al. (2012).
Statistical Analysis
For our data statistical analysis, the single factor analysis of variance (ANOVA) and Tukey’s test data analysis was applied. All statistical evaluations were conducted using the IBM SPSS Statistics ver. 20 software. (SPSS Inc., Chicago, IL, United States).
Results
Generation of Transgenic Plants Expressing p35S:OsNAR2.1-p35S:OsNRT2.3a Construction and Field Traits Analysis
In this study, we further introduced the p35S:OsNAR2.1 (Figure S1A), p35S:OsNRT2.3a (Figure S1B) and p35S:OsNAR2.1-p35S:OsNRT2.3a (Figure S1C) expression construction into Wuyunjing7 (WT) which is a high yield rice cultivar used in Jiangsu, China. We generated 18 lines, including 6 p35S:OsNAR2.1 lines (N lines), 6 p35S:OsNRT2.3a lines (A lines), and 6 p35S:OsNAR2.1-p35S:OsNRT2.3a lines (NA lines). Then we conducted field experiments and analyzed the grain yield and biomass of these transgenic lines. T1–T2 generation transgenic plants and WT plants were planted in the plots with 300 kg N/ha in Nanjing, Jiangsu. Compared to WT plants, the yield and biomass for N lines of T1 plants increased by approximately 17.4% and 7.1%, respectively (Figure S2A). However, there was no significant difference between A lines and WT (Figure S2B). The yield and biomass of NA lines of T1 plants increased by approximately 30.5% and 19.5%, respectively (Figure S2C). Based on RNA expression data for the T1 generation (Figures S2D–F) and T2 generation (Figures 1D–F), we selected three N T2 lines named N1, N2, N3 (Figure 1A), three A T2 lines named A1, A2, A3 (Figure 1B), and three NA T2 lines named NA1, NA2, NA3 (Figure 1C) for further analysis. Southern blot analysis showed that N1 and N2 were two independent transgenic lines with one transgenic copy respectively (Figure 1G); A1 and A2 were two independent transgenic lines with one transgenic copy respectively (Figure 1H); NA1 and NA2 were two independent transgenic lines with one transgenic copy respectively (Figure 1I).
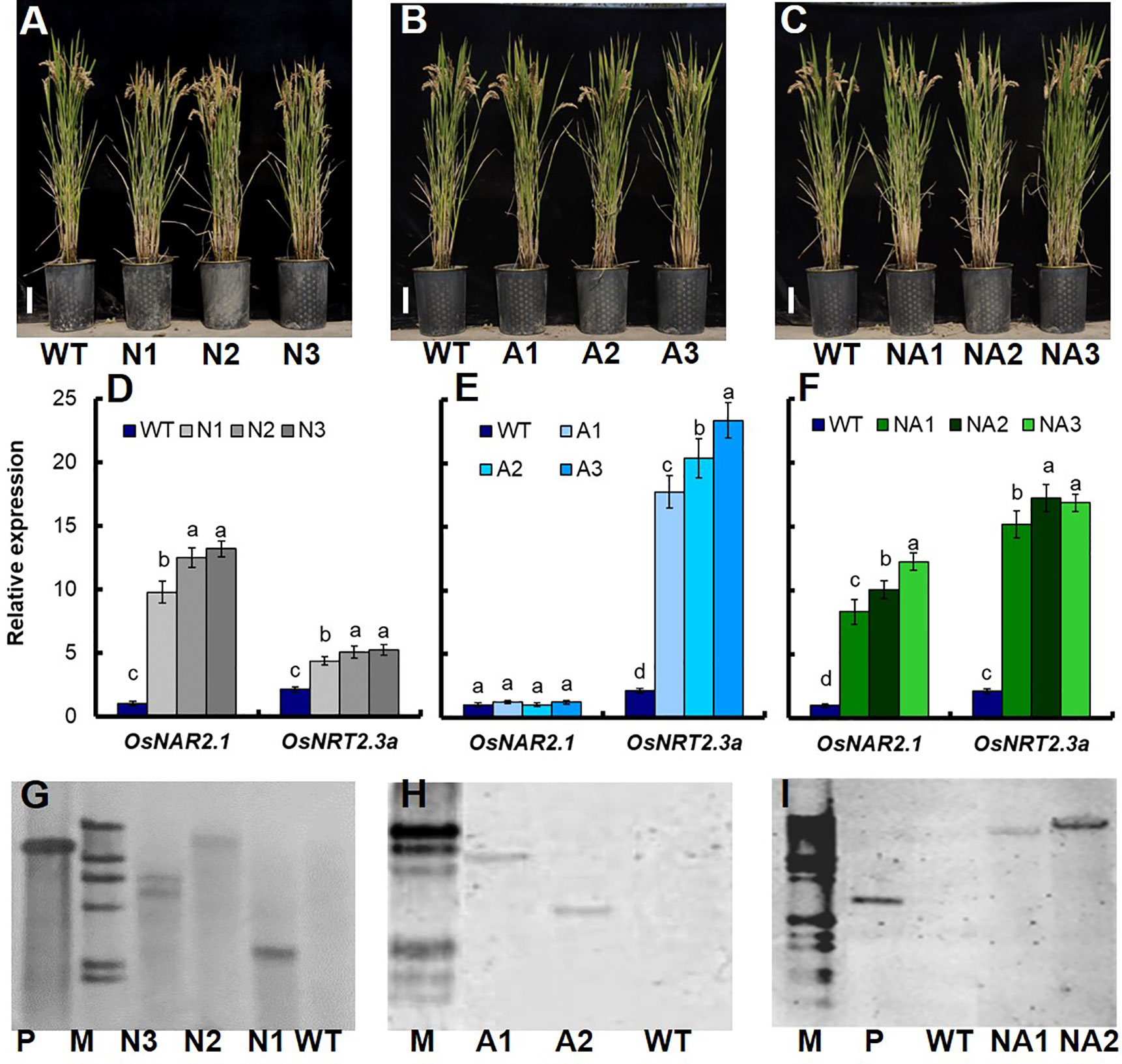
Figure 1 Identification of transgenic lines. Phenotype of (A) p35S:OsNAR2.1 transgenic lines (N1, N2, and N3), (B) p35S:OsNRT2.3a transgenic lines (A1, A2, and A3), and (C) p35S:OsNAR2.1-p35S:OsNRT2.3a transgenic lines (NA1, NA2, and NA3). qRT-PCR analysis the expression of OsNAR2.1 and OsNRT2.3a of (D) p35S:OsNAR2.1 transgenic lines, (E) p35S:OsNRT2.3a transgenic lines, and (F) p35S:OsNAR2.1-p35S:OsNRT2.3a transgenic lines. RNA was extracted from culm. Error bars: SE (n = 3). The different letters indicate a significant difference between the transgenic line and the WT (P < 0.05, one-way ANOVA). Southern blot analysis the copy number of (G) p35S:OsNAR2.1 transgenic line, (H) p35S:OsNRT2.3a transgenic lines, and (I) p35S:OsNAR2.1-p35S:OsNRT2.3a transgenic lines. Genomic DNA isolated from T2 generation transgenic plants was digested with the Hind III and EcoR I restriction enzymes. A G418 gene probe was used for hybridization. P, positive control; M, marker.
For the T3 transgenic plants were planted in the plots with 150 kg N/ha in Changxing, Zhejiang. With WT as control, the grain yield of N (N1 and N2) lines was increased by 14.5%, and agronomic nitrogen use efficiency increased by 15.1% (Figure S3). The grain yield and agronomic nitrogen use efficiency of NA (NA1 and NA2) lines increased by 27.1% and 31.4% compared with WT (Figure S3). There was no significant difference in grain yield and agronomic nitrogen use efficiency between A lines and WT (Figure S3).
The data of the field experiment for T4 generation which planted in the plots with 300 kg N/ha in Nanjing, Jiangsu Province were analyzed in detail. Compared with WT, the total tiller number per plant and seed setting rate increased by 26.2% and 16.3% respectively, while the panicle length, grain weight and grain number per panicle was reduced by 4.9%, 11.4%, and 11.6% respectively, and the grain yield increased by 14.1% (Table 1). For the NA lines, there was no significant difference in seed setting rate and tiller number between and N lines, but the grain yield increased by 24.6% (Table 1) compared to the control. However, there was no significant difference between all agronomic traits of A lines and WT (Table 1).
Dry Matter Accumulation and N Analysis in Transgenic Lines
Anthesis stage is a particular time from vegetative growth to reproductive growth of rice, and dry matter and nitrogen accumulation during anthesis stage have positive influence on the formation of final yield of rice (Good et al., 2004). We sampled shoot tissues at the anthesis stage and at the mature stage separately to determine the biomass and the total N content. Analysis using WT as control, we found that at the anthesis stage, the biomass of panicles, leaves and culms in N lines increased by 18.7%, 18.3%, and 20.0% respectively; the biomass of panicles, leaves and culms in A lines had no significant difference; the biomass of panicles, leaves and culms in NA lines increased by 21.1%, 19.3%, and 26.6% (Figure 2B). At the mature stage, the biomass of panicles in N lines increased by about 14.0%, but the biomass of leaves and culms for N was not significant. There was no significant difference between the biomass of panicles, leaves and culms between A lines and WT. For the NA lines, the biomass of panicles, leaves and culms was increased by 26.4%, 15.5%, and 12.8% respectively compared with WT (Figure 2C).
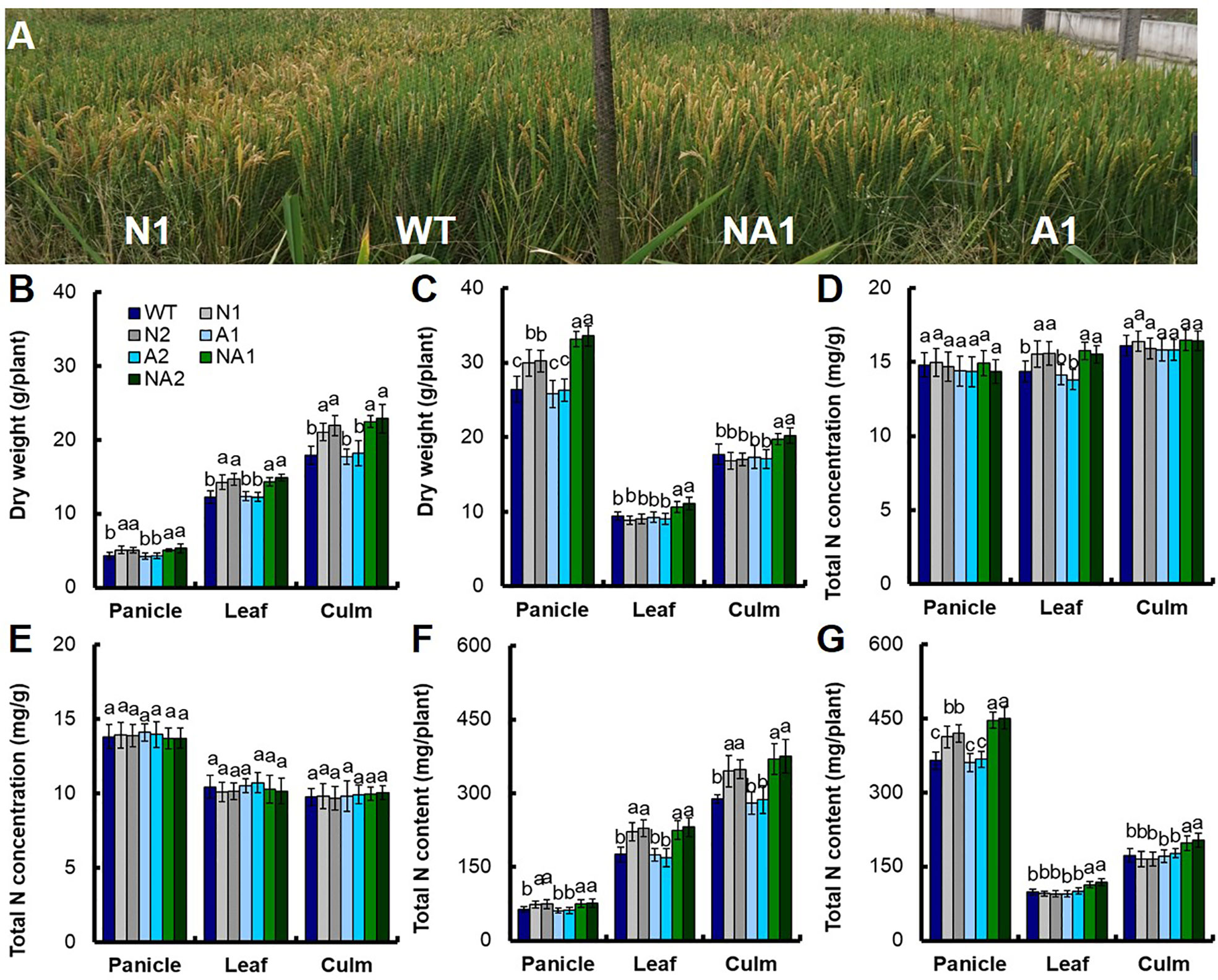
Figure 2 Biomass and nitrogen content in different parts of transgenic lines at the anthesis stage and maturity stage. (A) Photograph of wild-type (cv. Wuyunjing 7, WT) and T4 generation transgenic lines in the field experiment at Nanjing. Biomass in various parts of WT and T4 generation transgenic plants at (B) the anthesis stage and (C) maturity stage. Nitrogen concentration in different parts of transgenic lines and WT at the (D) anthesis stage and (E) maturity stage. Nitrogen content in different parts of transgenic lines and WT at the (F) anthesis stage, and (G) maturity stage. Error bars: SE (n = 3). The different letters indicate a significant difference between the transgenic line and the WT (P < 0.05, one-way ANOVA).
At anthesis stage, there was no significant difference of the total nitrogen concentration in leaves between N lines and NA lines, but increased by about 8.8% compared with WT. For the total nitrogen concentration in panicles and culms, there was no significant difference between N lines, NA lines and WT. There was no significant difference in total nitrogen concentration between different parts of A lines and WT (Figure 2D). At maturity stage, there was no significant difference in total nitrogen concentration between N, A and NA lines and WT (Figure 2E).
The variation of biomass and total nitrogen concentration eventually led to the difference of total nitrogen content per plant. At anthesis stage, there was no significant difference in total nitrogen concentration in panicles, leaves and culms between N and NA lines, but increased by about 18.3%, 29.2%, and 24.7% compared to WT (Figure 2F). At maturity stage, the total nitrogen content in panicles of N lines increased by about 14.2%, there was no significant difference in the total nitrogen content in leaves and culms between N lines and WT. The total nitrogen content in panicles, leaves and culms of NA lines increased by about 23.0%, 17.7%, and 15.7% respectively, however, there was no significant difference in total nitrogen content between A lines and WT in different plant parts (Figure 2G).
Dry matter and N translocation in rice plants were investigated by determining dry matter at anthesis stage (DMA), dry matter at maturity (DMM), grain yield (GY), total N accumulation at anthesis stage (TNAA), total N accumulation at maturity stage (TNAM) and grain nitrogen content at maturity stage (GNAM) respectively. Compared with WT, the DMA, DMM, GY, TNAA, TNAM, and GNAM of N lines increased by 19.0%, 6.2%, 12.1%, 22.5%, 6.8%, and 14.9% respectively, NA lines increased by about 23.3%, 18.6%, 22.6%, 28.3%, 19.5%, and 21.9%, respectively. There were no significant differences in DMA, DMM, GY, TNAA, TNAM, and GNAM between A lines and WT (Table 2).
Nitrogen Use Efficiency Estimation of the Transgenic Lines
We also detected dry matter translocation (DMT), DMT efficiency (DMTE), the contribution of pre-anthesis assimilates to grain yield (CPAGY), nitrogen translocation (NT), NT efficiency (NTE), and the contribution of pre-anthesis N to grain N accumulation (CPNGN), based on a method described by Chen et al. (2016). Compared with WT, DMT, DMTE, CPAGY, NT, NTE, and CPNGN of N lines increased by 106.5%, 73.5%, 81.1%, 50.6%, 22.9%, and 31.1%, respectively, NA lines increased by 59.1%, 28.9%, 29.7%, 40.8%, 9.8%, and 15.7%, respectively. There were no significant differences in DMT, DMTE, CPAY, NT, NTE, and CPNGN between A lines and WT (Table 3).
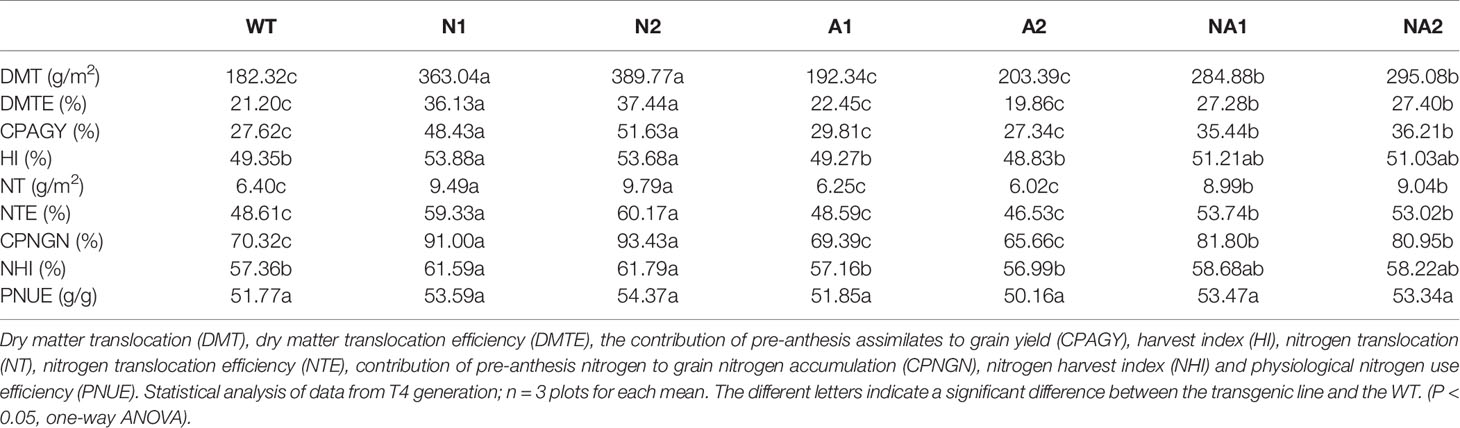
Table 3 Dry matter transport efficiency, N transport efficiency, and N-use efficiency of transgenic lines.
Based on a method described by Chen et al. (2016), we calculated the physiological nitrogen use efficiency (PNUE), nitrogen harvest index (NHI), harvest index (HI), agronomic nitrogen use efficiency (ANUE) and nitrogen recovery efficiency (NRE). Compared with WT, the PNUE of N lines did not change significantly, NHI and HI increased by about 7.6% and 9.0% respectively; the PNUE, NHI, and HI of A and NA lines had no significant difference compared with WT (Table 3). But the ANUE and NRE of N lines increased by 15.8% and 11.1% respectively; the NA lines increased by 28.6% and 21.2% respectively, while the ANUE and NRE of A lines did not change compared with WT (Figures 3A, B).
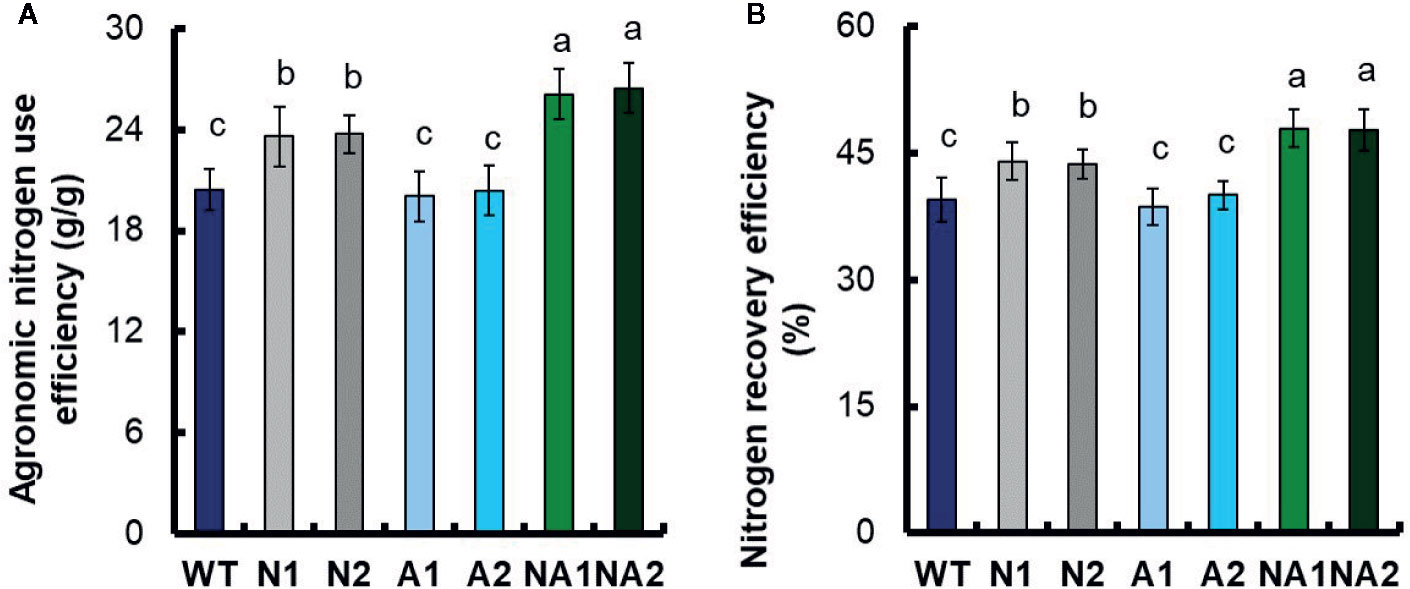
Figure 3 Comparison of nitrogen use efficiency between the WT and transgenic lines. (A) Agronomic nitrogen use efficiency (ANUE), (B) nitrogen recovery efficiency (NRE). Error bars: SE (n = 3). The different letters indicate a significant difference between the transgenic line and the WT (P < 0.05, one-way ANOVA).
The Expression of OsNAR2.1 and OsNRT2s in Transgenic Lines in Different Growth Stages of WT and Transgenic Lines
Chen et al. (2016, 2017) reported that the expression of OsNAR2.1 and OsNRT2.1 in culms significantly affected rice yield and nitrogen use efficiency. In this study, we analyzed the expression of OsNAR2.1 and OsNRT2s in culms of WT and transgenic lines at 30 days (the vegetative growth period), 60 days (the anthesis stage), and 75 days (the grain filling stage) separately. During these three periods, the expressions of OsNAR2.1 and OsNRT2.3a of N (N1, N2, and N3) or NA (N1, NA2, and N3) lines were significantly higher compared with WT. But there was no significant difference of the expressions of OsNRT2.1and OsNRT2.2 between N and NA lines (Figure 4). Compared with WT, the expression of OsNAR2.1 increased by about 14 times in N lines, while OsNRT2.3a increased by about 51% in those lines. The expression of OsNAR2.1 and OsNRT2.3a in NA lines increased about 12-fold and 10-fold respectively (Figure 4). Compared with WT, the expression of OsNRT2.3a in A (A1, A2, and A3) lines increased about 10 times, while the expression of OsNAR2.1, OsNRT2.1, and OsNRT2.2 did not change significantly (Figure 4).
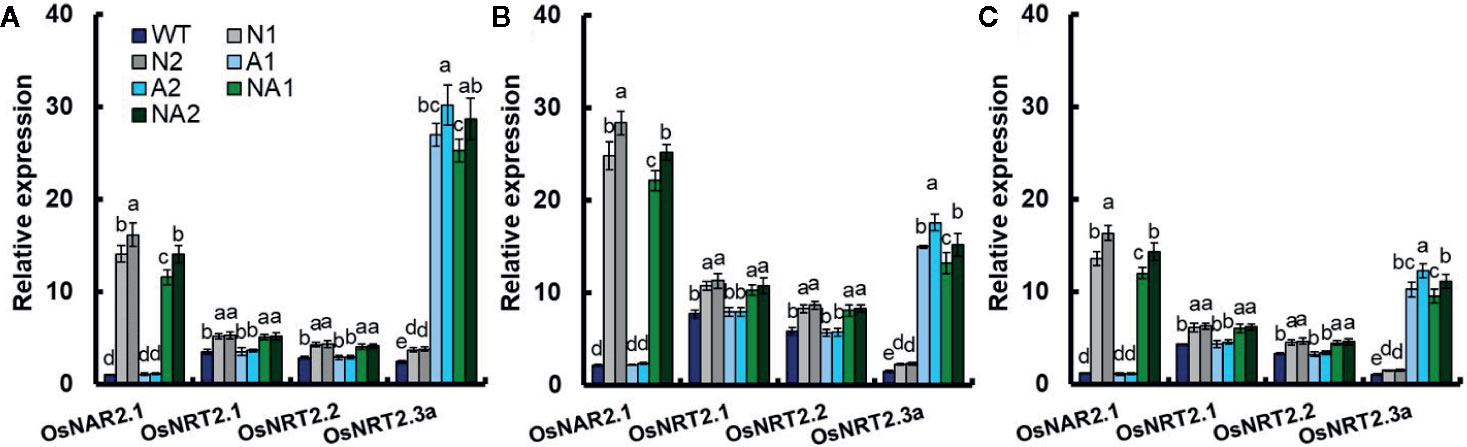
Figure 4 Expression of OsNAR2.1 and OsNAR2s in transgenic lines and WT during the experimental growth period. Samples were collected (A) 30 days (the vegetative growth period), (B) 60 days (the anthesis stage) and (C) 75 days (the grain filling stage) after seedlings were transplanted to the field. RNA was extracted from culms. Error bars: SE (n = 3). The different letters indicate a significant difference between the transgenic line and the WT (P < 0.05, one-way ANOVA).
Agronomic Nitrogen Use Efficiency Estimation for Co-Overexpression of OsNAR2.1 and OsNRT2.3a in the cv. Nipponbare Rice
We also introduced the p35S:OsNAR2.1 (Figure S1A), p35S:OsNRT2.3a (Figure S1B), and p35S:OsNAR2.1-p35S:OsNRT2.3a (Figure S1C) expression construct into Nipponbare cultivar. We generated 6 lines, including 2 p35S:OsNAR2.1 lines (n lines), 2 p35S:OsNRT2.3a lines (a lines), and 2 p35S:OsNAR2.1-p35S:OsNRT2.3a lines (na lines) (Figure 5C). Southern blot analysis showed that n1 and n2 were two independent transgenic lines with one transgenic copy respectively (Figure 5B); a1 and a2 were two independent transgenic lines with one transgenic copy respectively (Figure 5C); na1 and na2 were two independent transgenic lines with one transgenic copy respectively (Figure 5D). Based on Southern blot analysis and gene expression analysis (Figures 5E–G) of T2 plants, they are independent lines. Compared with NP (Nipponbare), the grain yields of n lines and na lines increased by 16.0% and 37.3% (Figure 5H), and the agronomic nitrogen use efficiency increased by 14.9% and 35.2% (Figure 5I), respectively.
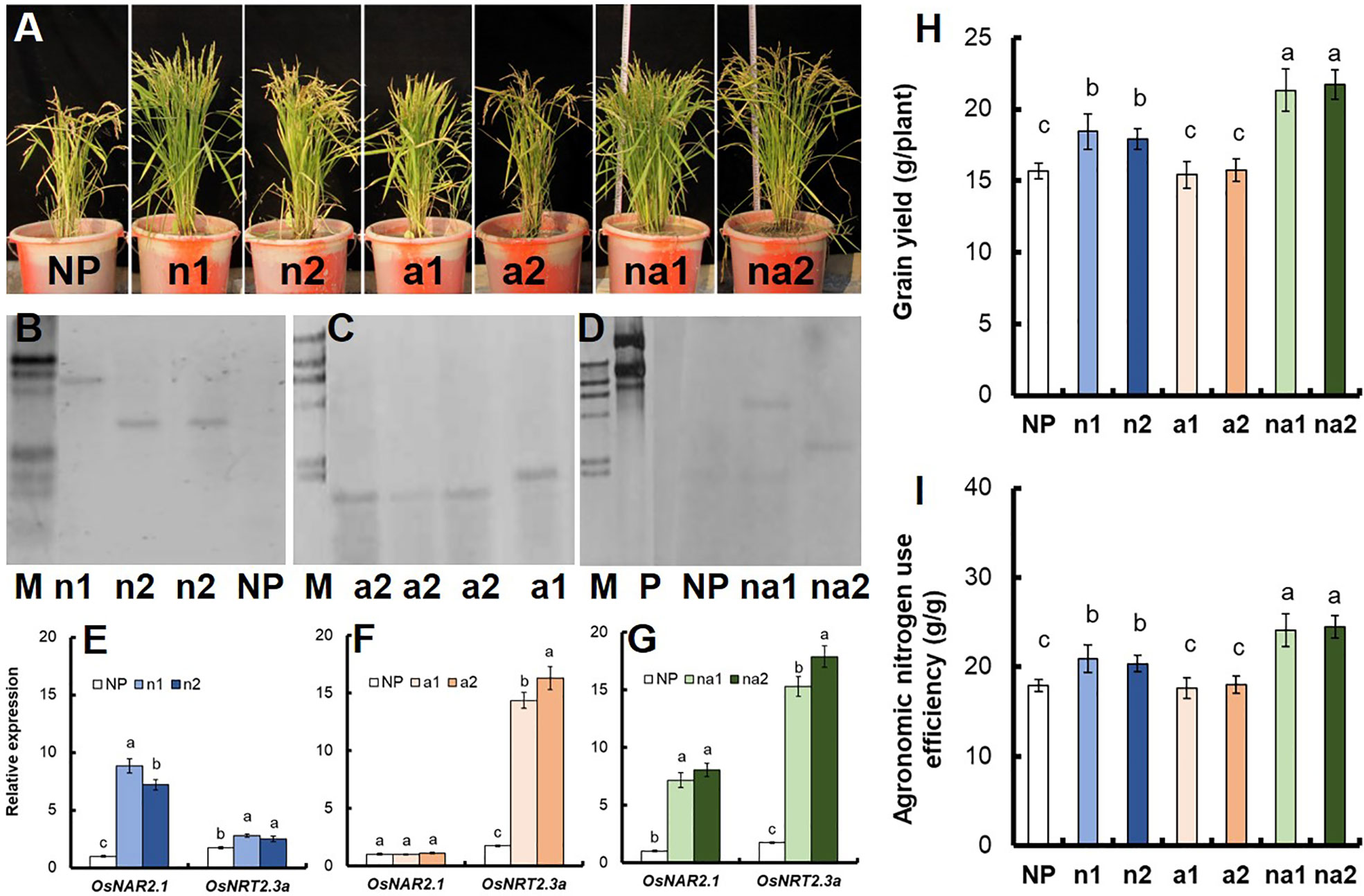
Figure 5 Grain yield and agronomic nitrogen use efficiency of Nipponbare transgenic lines. (A) Phenotype of wild-type (cv. Nipponbare, NP), p35S:OsNAR2.1 transgenic lines (n1 and n2), p35S:OsNRT2.3a transgenic lines (a1 and a2), and p35S:OsNAR2.1-p35S:OsNRT2.3a transgenic lines (na1, na2). Southern blot analysis the copy number of (B) p35S:OsNAR2.1 transgenic lines, (C) p35S:OsNRT2.3a transgenic lines, and (D) p35S:OsNAR2.1-p35S:OsNRT2.3a transgenic lines. Genomic DNA isolated from T2 generation transgenic plants was digested with the Hind III and EcoR I restriction enzymes. A G418 gene probe was used for hybridization. P, positive control; M, marker. qRT-PCR analysis the expression of OsNAR2.1 and OsNRT2.3a of (E) p35S:OsNAR2.1 transgenic lines, (F) p35S:OsNRT2.3a transgenic lines, and (G) p35S:OsNAR2.1-p35S:OsNRT2.3a transgenic lines. RNA was extracted from culm. Comparison of (H) grain yield and (I) agronomic nitrogen use efficiency between the NP and transgenic lines. Error bars: SE (n = 3). The different letters indicate a significant difference between the transgenic line and the WT (P < 0.05, one-way ANOVA).
The Plant Seedling Growth and Total Nitrogen Content Evaluation in Transgenic Lines
We further analyzed the growth and nitrogen uptake of transgenic lines at seedling stage. WT and transgenic rice seedlings were grown in IRRI solution containing 1 mM NH4+ for 2 weeks and then transferred into 0.2 mM NO3–, 2.5 mM NO3–, or 1.25 mM NH4NO3 for 3 additional weeks (Figure S4). Compared with WT, under 0.2 mM NO3– treatment, the biomass of root and shoot in N lines increased 151.2% and 102.7% respectively, it increased 204.0% and 150.7% respectively in NA lines (Figure 6A). Under 2.5 mM NO3– treatment, the biomass of root and shoot in N lines increased 136.8% and 142.8% respectively, it increased 170.1% and 185.7% respectively in NA lines (Figure 6B). Under 1.25 mM NH4NO3 treatment, the biomass of root shoot in N lines increased 58.9% and 56.2% respectively, it increased 85.5% and 102.1% respectively in NA lines (Figure 6C).
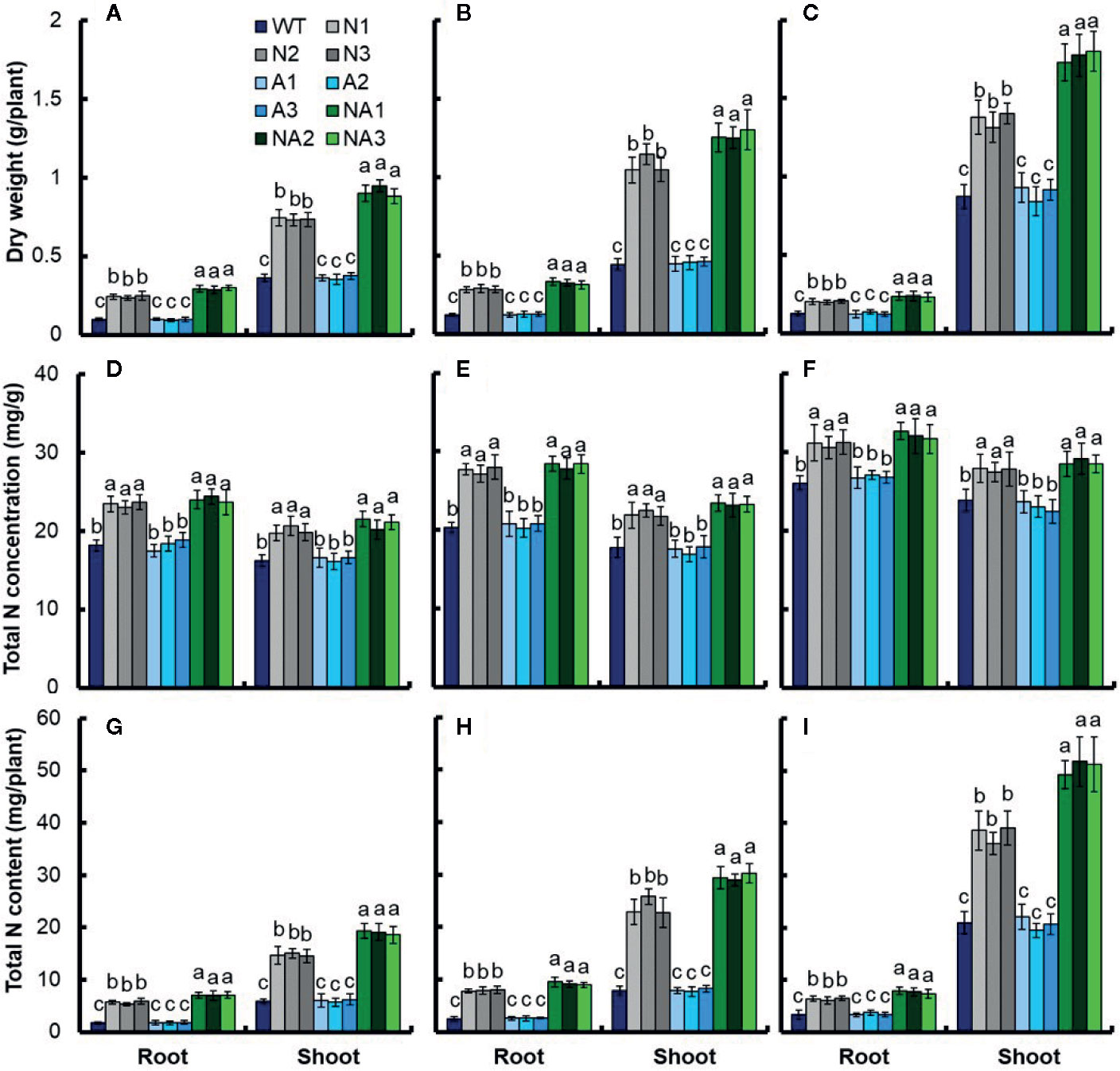
Figure 6 Total nitrogen content of transgenic plants at different nitrogen supply levels. WT and transgenic rice seedlings in the solution containing 1 mM NH4+ of IRRI for 2 weeks, and in different forms of nitrogen for 3 additional weeks. Dry weight of seedlings treated with (A) 0.2 mM NO3–, (B) 2.5 mM NO3–, and (C) 1.25 mM NH4NO3; Total nitrogen concentration of seedlings treated with (D) 0.2 mM NO3–, (E) 2.5 mM NO3–, and (F) 1.25 mM NH4NO3; Total N content of seedlings grown with (G) 0.2 mM NO3–, (H) 2.5 mM NO3– and (I) 1.25 mM NH4NO3. Error bars: SE (n = 4). The different letters indicate a significant difference between the transgenic line and the WT (P < 0.05, one-way ANOVA).
Moreover, we measured the total nitrogen concentration, and found that there was no significant difference in total nitrogen concentration between N lines and NA lines under different treatments. Compared with WT, the total nitrogen concentration in root and shoot of N lines or NA lines increased by 30.5% and 26.6% respectively under 0.2 mM NO3– treatment (Figure 6D), increased by 37.4% and 27.4% respectively under 2.5 mM NO3– treatment (Figure 6E), increased by 21.1% and 18.0% respectively under 1.25 mM NH4NO3 treatment (Figure 6F).
The total nitrogen content per plant was also calculated. After 0.2 mM NO3– treatment, the total nitrogen content of the root and shoot in N lines increased 223.5% and 151.2% respectively, it increased 302.0% and 224.0% respectively in NA lines (Figure 6G). After 2.5 mM NO3– treatment, the total nitrogen content increased 221.7% and 201.2% respectively in N lines, it increased 275.0% and 273.9% respectively NA lines (Figure 6H); under 1.25 mM NH4NO3 treatment, the total nitrogen content increased 89.1% and 81.3% respectively in N lines, it increased 128.7% and 142.7% respectively in NA lines (Figure 6I).
We further analyzed the expression of OsNAR2s and OsNRT2s in transgenic lines treated with 0.2 mM NO3– and 1.25 mM NH4NO3. The expression patterns of the transgenic lines were similar under the treatment of 0.2 mM NO3– and 1.25 mM NH4NO3. In the N lines, the expression of OsNAR2.1 increased by about 12.4 times compared with WT, and the expression of OsNRT2.1, OsNRT2.2, and OsNRT2.3a increased by 55%-98% (Figures S5A, D). In the A lines, the expression of OsNRT2.3a in increased about 11 times, but the expression of OsNAR2.1, OsNRT2.2, and OsNRT2.2 did not change significantly (Figures S5B, E). However, for the NA lines, the expression of OsNAR2.1 increased by 13.8 times, the expression of OsNRT2.3a increased by 10.1 times, and the expression of OsNRT2.1 and OsNRT2.2 increased by 53%-120% (Figures S5C, F). The expression of OsNAR2.2, OsNRT2.3b and OsNRT2.4 did not change significantly in N, A, and NA lines (Figure S6).
15N Influx Rates and 15N Distribution Ratio Influx Determination
We analyzed short-term 15N uptake in same-size seedlings of the transgenic lines and WT which exposed to 0.2 mM 15NO3–, 2.5 mM 15NO3–, or 1.25 mM 15NH415NO3 for 5 min. There was no significant difference of 15N influx rate between N lines and A lines. Compared with WT, the influx rate of 15NO3– increased 27.6% and 20.1% in response to 0.2 mM 15NO3– and 2.5 mM 15NO3–, respectively; the influx rate of 15NH415NO3 increased 20.6% responded to 1.25 mM 15NH415NO3 (Figures 7A–C). The influx rate of 15N did not change compared with that of WT in the A lines (Figures 7A–C).
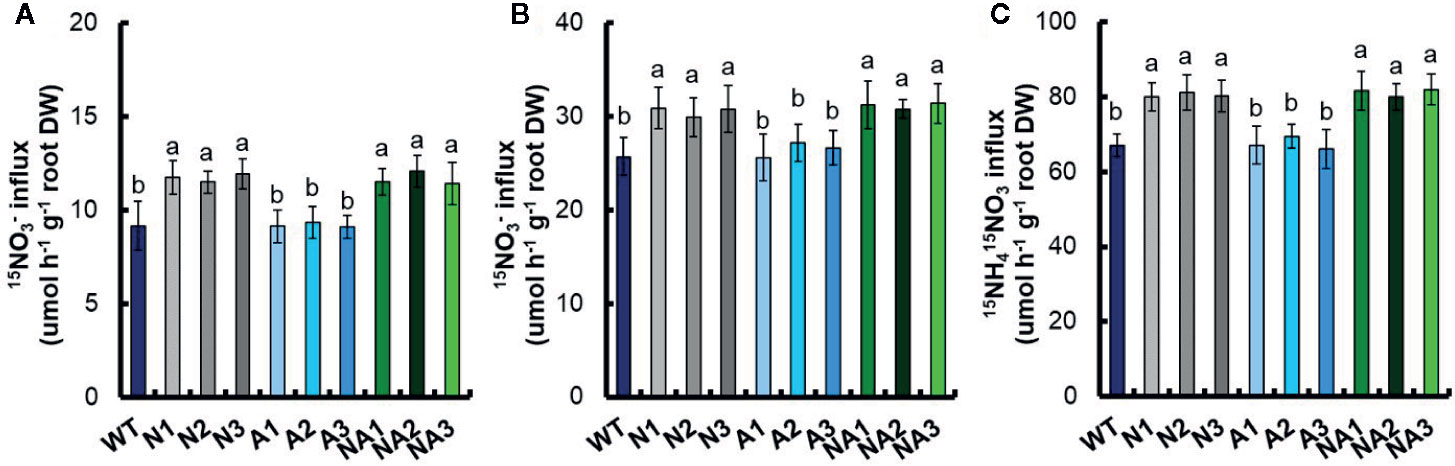
Figure 7 15N influx rates of transgenic lines. WT and transgenic seedlings were grown in 1 mM NH4+ for 3 weeks and nitrogen starved for 1 week. 15N influx rates were then measured at (A) 0.2 mM 15NO3–, (B) 2.5 mM 15NO3–, and (C) 1.25 mM 15NH415NO3 during 5 min. DW, dry weight. Error bars: SE (n = 4). The different letters indicate a significant difference between the transgenic line and the WT (P < 0.05, one-way ANOVA).
We also detected 15N distribution ratio experiment in root. WT and transgenic seedlings were grown in 1 mM NH4+ for 3 weeks and treated in nitrogen starvation condition for 1 week. 15N concentration experiment was conducted under the nutrient solution containing 0.2 mM 15NO3– or 1.25 mM 15NH415NO3 for 12 h. Under 0.2mM 15NO3– treatment, there was no significant difference of the 15N concentration in roots between N and NA lines, but the 15N concentration increased by 19.7% compared with WT. 15N concentration in roots of N and NA lines increased by 25.5% and 48.1%, respectively compared with WT (Figure 8A). Under 1.25 mM 15NH415NO3 treatment, there was no significant difference in 15N concentration in roots between N and NA lines, but it increased by 20.0% compared with WT. 15N concentration in roots of N and NA lines increased by 21.2% and 33.2%, respectively (Figure 8B). Under the treatment of 0.2 mM 15NO3– and 1.25 mM 15NH415NO3, there was no significant difference in 15N concentration in roots and shoots between A lines and WT (Figures 8A, B). Finally, compared with WT, the 15N of shoot to root ratio in the NA lines increased by 25.9% and 20.7% respectively under 0.2 mM 15NO3– and 1.25 mM 15NH415NO3 treatments (Figures 8C, D). There was no significant difference of the 15N in shoot to root ratio between WT, N lines and A lines under 0.2 mM 15NO3– or 1.25 mM 15NH415NO3 (Figures 8C, D).
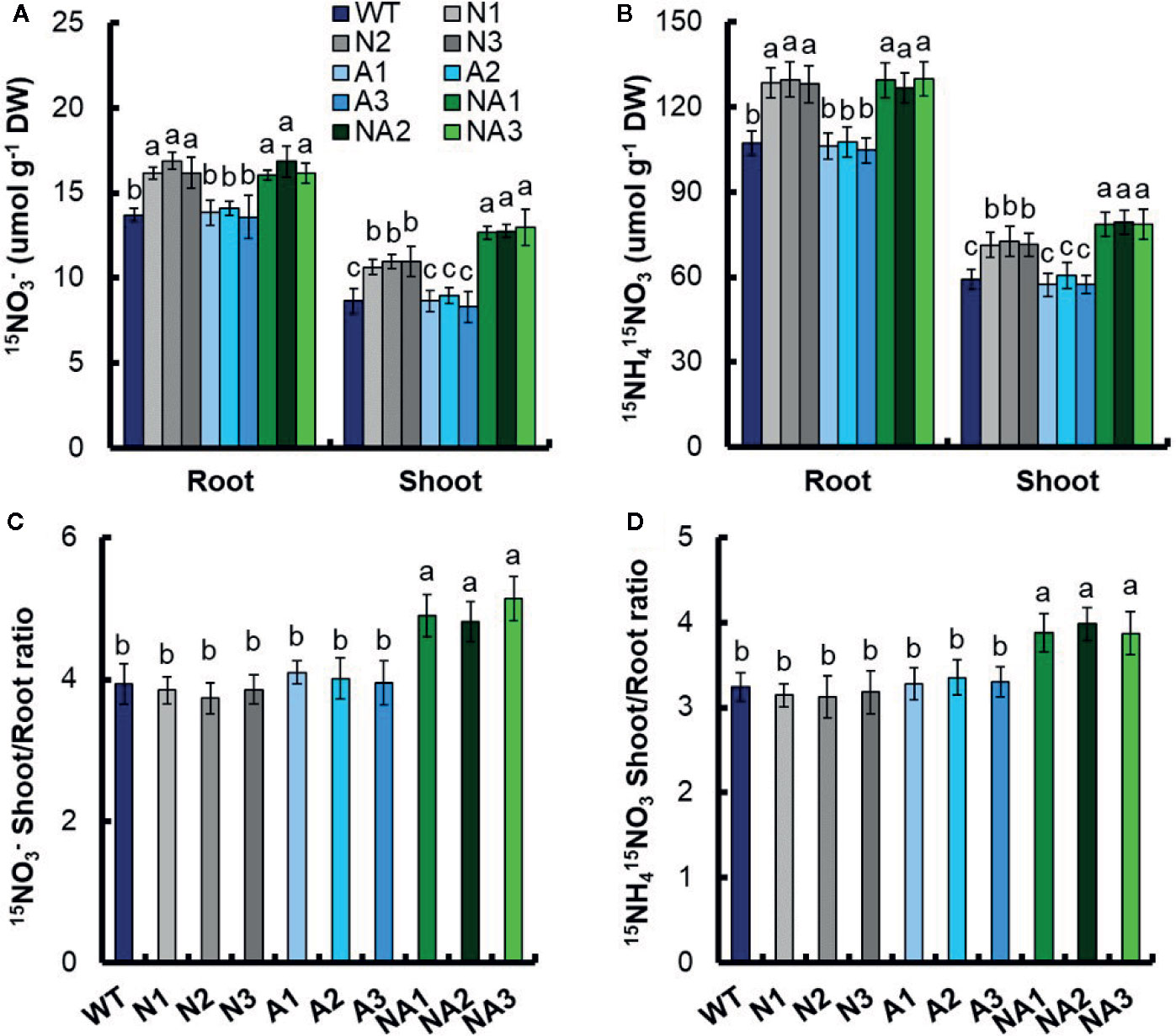
Figure 8 15N concentration and 15N distribution ratio in WT and transgenic lines. WT and transgenic seedlings were grown in 1 mM NH4+ for 3 weeks and nitrogen starved for 1 week. 15N concentration in the roots and shoots of the WT and transgenic lines under the nutrient solution containing (A) 0.2 mM 15NO3– or (B) 1.25 mM 15NH415NO3 for 12 h. The shoot-to-root ratio of the total 15N content at (C) 0.2 mM NH4+ or (D) 1.25 mM 15NH415NO3. DW, dry weight. Error bars: SE (n = 4). The different letters indicate a significant difference between the transgenic line and the WT (P < 0.05, one-way ANOVA).
Discussion
Increasing NO3– uptake is an effective way to improve yield and nitrogen use efficiency in rice. Fang et al. (2013) found that overexpression of nitrate transporter OsPTR9 could promote lateral root formation, increase grain yield of rice. Overexpression of OsNRT1.1B, a low affinity NO3– transporter protein gene, increased rice nitrogen use efficiency by about 30% (Hu et al., 2015). Overexpression of high affinity nitrate transporter gene OsNRT2.3b increased the buffer capacity of cell pH and significantly increased grain yield and nitrogen use efficiency of rice (Fan et al., 2016). Overexpression of nitrate transporter gene OsNRT1.1A in rice greatly improved nitrogen utilization and grain yield, and maturation time was also significantly shortened (Wang et al., 2018). Gao et al. (2019) reported that overexpression of OsNR2 increased the activity of nitrate reductase and the uptake of nitrate by rice, thus increasing the yield and nitrogen use efficiency of rice. Overexpression of OsNAR2.1 or OsNRT2.1 by the native promoter of OsNAR2.1 can improve grain yield and nitrogen use efficiency of rice (Chen et al., 2016; Chen et al., 2017; Luo et al., 2018; Chen et al., 2019). OsNAC42 as a transcription factor enhances nitrate uptake in rice by regulating nitrate transporter gene OsNPF6.1, thereby enhancing the regulation of rice nitrogen use efficiency (Tang et al., 2019). In this study, we investigated the effect of co-overexpression of OsNAR2.1 and OsNRT2.3a on NO3– uptake, yield and nitrogen use efficiency uptake by rice.
OsNAR2.1 act as a partner protein with OsNRT2s for transport of NO3– in rice (Yan et al., 2011; Tang et al., 2012; Liu et al., 2014). Chen et al. (2017) found that pOsNAR2.1:OsNAR2.1 increased the expression of OsNAR2.1 by 3‑5 times in rice, and in this study the expression of OsNAR2.1 in p35S:OsNAR2.1 increased by more than 10 times (Figure 1D, Figure 4, Figures S6A, D). This because different promoters lead to different expression patterns of OsNAR2.1, which leads to inconsistent growth phenotypes of transgenic rice. Compared with WT, the DMA, DMM, GY, TNAA, TNAM, and GNAM of p35S:OsNAR2.1 lines increased by 19.0%, 6.2%, 12.1%, 22.5%, 6.8%, and 14.9% respectively (Table 2), of pOsNAR2.1:OsNAR2.1 lines increased by 25%, 25%, 24%, 33%, 34%, and 35% (Chen et al., 2017). This result also accords with our previous conclusion that controlling the expression ratio of OsNAR2.1 and OsNRT2.1 in rice in an appropriate proportion can improve more grain yield and nitrogen use efficiency in rice (Chen et al., 2016; Chen et al., 2017).
Compared with WT, the dry matter and total nitrogen accumulation of NA lines increased significantly at anthesis and maturity (Figure 2, Table 2), the DMTE, NTE, CPAGY, and CPNGN increased by 28.9%, 9.8%, 29.7%, and 15.7% respectively (Table 3). During rice filling, 70%‑90% of nitrogen was transported from vegetative organs to panicles (Yoneyama et al., 2016). The accumulation of nitrogen and biomass in early stage has a great influence on rice yield. Compared with WT, the GY, ANUE and NRE of NA lines increased by 22.6%, 28.6% and 21.2% respectively (Table 3, Figure 3). Compared with WT, the GY, ANUE, and NRE of N lines increased by 12.1%, 15.8%, and 11.1% respectively (Table 3, Figure 3), which was significantly lower than that of NA lines. Similarly, co-overexpression of OsNAR2.1 and OsNRT2.3a increased agronomic nitrogen use efficiency of cv. Nipponbare rice. Compared with Nipponbare rice, the grain yields of p35S:OsNAR2.1 and p35S:NAR2.1-p35S:NRT2.3a lines increased by 16.0% and 37.3% (Figure 5H), and the agronomic nitrogen use efficiency increased by 14.9% and 35.2% (Figure 5I), respectively. This means the co-overexpression of OsNAR2.1 and OsNRT2.3a transgenic plants have functions in different rice varieties and it can provide a physiological basis for rice breeding.
Kronzucker et al. (2000) used 13N to show that the presence of NO3– promotes NH4+ uptake, accumulation, and metabolism in rice. Duan et al. (2006) found that increasing NO3– uptake promotes dry weight and NO3– accumulation and assimilation of NH4+ and NO3– by ‘Nanguang’, which is an N-efficient rice cultivar, during the entire growth period. Li et al. (2006) showed that supplying NH4+ and NO3– enhances OsAMT1;3, OsAMT1;2, and OsAMT1;1 expression compared with supplying only NH4+ or NO3–, thereby enhancing NH4+ uptake by rice. The influx rates of 15NH4+ and 15NO3– in pOsNAR2.1:OsNAR2.1 transgenic lines increased by 21 and 22% in 1.25 mM 15NH4NO3 and 1.25 mM NH415NO3, respectively (Chen et al., 2017). Further studies showed that when 0.2 mM NO3–, 2.5 mM NO3– or 1.25 mM NH4NO3 were provided as the sole nitrogen source, the dry weight and total nitrogen content of NA lines were higher than that of N lines (Figure 6). In the 5-min 15N absorption experiment, we found that there was no significant difference in the influx rate of 15N between N and NA strains at 0.2 mM 15NO3–, 2.5 mM 15NO3–, or 1.25 mM 15NH415NO3 (Figures 7A–C). Compared with N lines, the 15N shoot to root ratio of NA lines increased by 25.9% and 20.7% respectively under 0.2 mM 15NO3– and 1.25 mM 15NH415NO3 treatments for 12 h (Figures 8C, D). OsNRT2.1 and OsNRT2.2 are responsible for NO3– uptake at the roots, while OsNRT2.3a is responsible for NO3– transport from roots to shoots (Feng et al., 2011; Yan et al., 2011; Tang et al., 2012; Chen et al., 2016). Overexpression of OsNAR2.1 can increase the NO3– and NH4NO3 uptake from roots (Figures 8A, B), but it cannot increase the transport ratio from roots to shoots (Figures 8C, D), this because more OsNRT2.3a needs to be expressed in order to increase the roots-to-shoots transport ratio. In this study, we also found that overexpression of OsNRT2.3a alone did not change rice growth. There was no significant difference in NO3– uptake, agronomic traits, biomass and nitrogen accumulation between A lines and WT (Tables 1 and 2, Figure 2, Figure S3). The reason may be that the expression of OsNRT2.3a alone did not affect the expression of OsNAR2.1 (Figures 1 and 4, Figure S5).
In conclusion, co-overexpression of OsNAR2.1 and OsNRT2.3a could increase the uptake as well as the transport rate of NO3– and NH4NO3 from roots to shoots, eventually leading to increasing the total nitrogen content and biomass of rice seedlings under 0.2 mM NO3–, 2.5 mM NO3–, and 1.25 mM NH4NO3 conditions. Field experiments also showed that co-overexpression of OsNAR2.1 and OsNRT2.3a could increase rice biomass and total nitrogen accumulation, as well as improve rice dry matter transport efficiency and nitrogen transport efficiency, inducing improving rice yield, agronomic nitrogen use efficiency and nitrogen recovery efficiency in rice. Through co-overexpression of OsNAR2.1 and OsNRT2.3a have the same advantages in different rice varieties, it may also function in different crops. This approach provides an effective way to improve grain yield and nitrogen use efficiency in plant.
Data Availability Statement
The raw data supporting the conclusions of this article will be made available by the authors, without undue reservation.
Author Contributions
Conceived and designed the experiments: JC, XL, XiaoroF, and GX. Performed the experiments: JC, XL, SL, XiaoruF, LZ, and MS. Analyzed the data: JC, XL, and XiaoroF. Wrote and revised the paper: JC, XL, XiaoroF, and GX.
Funding
This work is supported by the Transgenic Project (Grant 2016ZX08001003-008), the Fundamental Research Funds for the Central Universities (KYZ202006), Innovative Research Team Development Plan of Ministry of Education of China (IRT_17R56; KYT201802), Jiangsu Science and Technology Development Program (Grant BE2019375-1), and Dapeng District Industry Development Special Funds (KY20180218).
Conflict of Interest
The authors declare that the research was conducted in the absence of any commercial or financial relationships that could be construed as a potential conflict of interest.
Supplementary Material
The Supplementary Material for this article can be found online at: https://www.frontiersin.org/articles/10.3389/fpls.2020.01245/full#supplementary-material
References
Alboresi, A., Gestin, C., Leydecker, M. T., Bedu, M., Meyer, C., Truong, H. N. (2005). Nitrate, a signal relieving seed dormancy in Arabidopsis. Plant Cell Environ. 28, 500–512. doi: 10.1111/j.1365-3040.2005.01292.x
Arth, I., Frenzel, P., Conrad, R. (1998). Denitrification coupled to nitrification in the rhizosphere of rice. Soil Biol. Biochem. 30, 509–515. doi: 10.1016/S0038-0717(97)00143-0
Aurelio, M., Briones, J., Satoshi, O., Yoshiaki, U., Niels-Birger, R., Wolfgang, R., et al. (2003). Ammonia-oxidizing bacteria on root biofilms and their possible contribution to N use efficiency of different rice cultivars. Plant Soil 250, 335–348. doi: 10.1023/A:1022897621223
Castro, M. I., Loef, I., Bartetzko, L., Searle, I., Coupland, G., Stitt, M., et al. (2011). Nitrate regulates floral induction in Arabidopsis, acting independently of light, gibberellin and autonomous pathways. Planta 233, 539–552. doi: 10.1007/s00425-010-1316-5
Chen, J., Zhang, Y., Tan, Y., Zhang, M., Zhu, L., Xu, G., et al. (2016). Agronomic nitrogen-use efficiency of rice can be increased by driving OsNRT2.1 expression with the OsNAR2.1 promoter. Plant Biotechnol. J. 14, 1705–1715. doi: 10.1111/pbi.12531
Chen, J., Fan, X., Qian, K., Zhang, Y., Song, M., Liu, Y., et al. (2017). pOsNAR2.1:OsNAR2.1 expression enhances nitrogen uptake efficiency and grain yield in transgenic rice plants. Plant Biotechnol. J. 15, 1273–1283. doi: 10.1111/pbi.12714
Chen, J., Qi, T., Hu, Z., Fan, X., Zhu, L., Iqbal, M. F., et al. (2019). OsNAR2.1 positively regulates drought tolerance and grain yield under drought stress conditions in rice. Front. Plant Sci. 10:197:197. doi: 10.3389/fpls.2019.00197
Crawford, N. M. (1995). Nitrate: nutrient and signal for plant growth. Plant Cell. 7, 859–868. doi: 10.1105/tpc.7.7.859
Duan, Y. H., Zhang, Y. L., Shen, Q. R., Wang, S. W. (2006). Nitrate effect on rice growth and nitrogen absorption and assimilation at different growth stages. Pedosphere 16, 707–717. doi: 10.1016/S1002-0160(06)60106-9
Fan, X., Tang, Z., Tan, Y., Zhang, Y., Luo, B., Yang, M., et al. (2016). Over expression of a pH sensitive nitrate transporter in rice increases crop yields. Proc. Natl. Acad. Sci. U. S. A. 113, 7118–7123. doi: 10.1073/pnas.1525184113
Fan, X., Naz, M., Fan, X., Xuan, W., Miller, A. J., Xu, G. (2017). Plant nitrate transporters: from gene function to application. J. Exp. Bot. 68, 2463–2475. doi: 10.1093/jxb/erx011
Fang, Z., Xia, K., Yang, X., Grotemeyer, M. S., Meier, S., Rentsch, D., et al. (2013). Altered expression of the PTR/NRT1 homologue OsPTR9 affects nitrogen utilization efficiency, growth and grain yield in rice. Plant Biotechnol. J. 11, 446–458. doi: 10.1111/pbi.12031
Feng, H., Yan, M., Fan, X., Li, B., Shen, Q., Miller, A. J., et al. (2011). Spatial expression and regulation of rice high-affinity nitrate transporters by nitrogen and carbon status. J. Exp. Bot. 62, 2319–2332. doi: 10.1093/jxb/erq403
Gao, Z., Wang, Y., Chen, G., Zhang, A., Yang, S., Shang, L., et al. (2019). The indica nitrate reductase gene OsNR2 allele enhances rice yield potential and nitrogen use efficiency. Nat. Commun. 10, 5207. doi: 10.1038/s41467-019-13110-8
Good, A. G., Shrawat, A. K., Muench, D. G. (2004). Can less yield more? Is reducing nutrient input into the environment compatible with maintaining crop production? Trends Plant Sci. 12, 597–605. doi: 10.1016/j.tplants.2004.10.008
Goodin, M. M., Chakrabarty, R., Banerjee, R., Yelton, S., DeBolt, S. (2007). New gateways to discovery. Plant Physiol. 145, 1100–1109. doi: 10.1104/pp.107.106641
Hsu, P. K., Tsay, Y. F. (2013). Two phloem nitrate transporters, NRT1.11 and NRT1.12, are important for redistributing xylem-borne nitrate to enhance plant growth. Plant Physiol. 163, 844–856. doi: 10.1104/pp.113.226563
Hu, B., Wang, W., Ou, S., Tang, J., Li, H., Che, R., et al. (2015). Variation in NRT1.1B contributes to nitrate-use divergence between rice subspecies. Nat. Genet. 47, 834–838. doi: 10.1038/ng.3337
Katayama, H., Mori, M., Kawamura, Y., Tanaka, T., Mori, M., Hasegawa, H. (2009). Production and characterization of transgenic rice plants carrying a high-affinity nitrate transporter gene (OsNRT2.1). Breed. Sci. 59, 237–243. doi: 10.1270/jsbbs.59.237
Kirk, G. J. D., Kronzucker, H. J. (2005). The potential for nitrification and nitrate uptake in the rhizosphere of wetland plants: a modelling study. Ann. Bot. 96, 639–646. doi: 10.1093/aob/mci216
Kronzucker, H. J., Glass, A. D. M., Siddiqi, M. Y. (1999). Inhibition of Nitrate uptake by Ammonium in barley analysis of component fluxes. Plant Physiol. 120, 283–291. doi: 10.1104/pp.120.1.283
Kronzucker, H. J., Glass, A. D. M., Siddiqi, M. Y., Kirk, G. J. D. (2000). Comparative kinetic analysis of ammonium and nitrate acquisition by tropical lowland rice: implications for rice cultivation and yield potential. New Phytol. 145, 471–476. doi: 10.1046/j.1469-8137.2000.00606.x
Léran, S., Varala, K., Boyer, J. C., Chiurazzi, M., Crawford, N., Daniel-Vedele, F., et al. (2014). A unified nomenclature of NITRATE TRANSPORTER 1/PEPTIDE TRANSPORTER family members in plants. Trends Plant Sci. 19, 5–9. doi: 10.1016/j.tplants.2013.08.008
Li, B., Xin, W., Sun, S., Shen, Q., Xu, G. (2006). Physiological and molecular responses of nitrogen-starved rice plants to re-supply of different nitrogen sources. Plant Soil 287, 145–159. doi: 10.1007/s11104-006-9051-1
Li, Y. L., Fan, X. R., Shen, Q. R. (2008). The relationship between rhizosphere nitrification and nitrogen-use efficiency in rice plants. Plant Cell Environ. 31, 73–85. doi: 10.1111/j.1365-3040.2007.01737.x
Liu, X., Huang, D., Tao, J., Miller, A. J., Fan, X., Xu, G. (2014). Identification and functional assay of the interaction motifs in the partner protein OsNAR2.1 of the two-component system for high-affinity nitrate transport. New Phytol. 204, 74–80. doi: 10.1111/nph.12986
Liu, X. Q., Feng, H. M., Huang, D. M., Fan, X. R., Xu, G. H. (2015). Two short sequences in the promoter of OsNAR2.1 are necessary for fully activating the nitrate induced gene expression in rice. Sci. Rep. 5:11950. doi: 10.1038/srep11950
Luo, B., Chen, J., Zhu, L., Liu, S., Li, B., Lu, H., et al. (2018). Overexpression of a high-affinity Nitrate transporter OsNRT2.1 increases yield and manganese accumulation in rice under alternating wet and dry condition. Front. Plant Sci. 9, 1192. doi: 10.3389/fpls.2018.01192
Miller, A. J., Fan, X., Orsel, M., Smith, S. J., Wells, D. M. (2007). Nitrate transport and signalling. J. Exp. Bot. 58, 2297–2306. doi: 10.1093/jxb/erm06
Naz, M., Luo, B., Guo, X., Li, B., Chen, J., Fan, X. (2019). Overexpression of nitrate transporter OsNRT2.1 enhances nitrate-dependent root elongation. Genes 10, 290. doi: 10.3390/genes10040290
O’Brien, J. A., Vega, A., Bouguyon, E., Krouk, G., Gojon, A., Coruzzi, G., et al. (2016). Nitrate transport, sensing, and responses in plants. Mol. Plant 9, 837–856. doi: 10.1016/j.molp.2016.05.004
Rahayu, Y. S., Walch-Liu, P., Neumann, G., Römheld, V., von, Wiren, N., Bangerth, F. (2005). Root-derived cytokinins as long-distance signals for NO3–induced stimulation of leaf growth. J. Exp. Bot. 56, 1143–1152. doi: 10.1093/jxb/eri107
Scheible, W. R., Morcuende, R., Czechowski, T., Fritz, C., Osuna, D., Palacios-Rojas, N., et al. (2004). Genome-wide reprogramming of primary and secondary metabolism, protein synthesis, cellular growth processes, and the regulatory infrastructure of Arabidopsis in response to nitrogen. Plant Physiol. 136, 2483–2499. doi: 10.1104/pp.104.047019
Tang, Z., Fan, X., Li, Q., Feng, H., Miller, A. J., Shen, Q., et al. (2012). Knockdown of a rice stelar nitrate transporter alters long-distance translocation but not root influx. Plant Physiol. 160, 2052–2063. doi: 10.1104/pp.112.204461
Tang, W., Ye, J., Yao, X., Zhao, P., Xuan, W., Tian, Y., et al. (2019). Genome-wide associated study identifies NAC42-activated nitrate transporter conferring high nitrogen use efficiency in rice. Nat. Commun. 10, 5279. doi: 10.1038/s41467-019-13187-1
Tong, Y., Zhou, J. J., Li, Z., Miller, A. J. (2005). A two-component high-affinity nitrate uptake system in barley. Plant J. 41, 442–450. doi: 10.1111/j.1365-313X.2004.02310.x
Wang, X., Zhang, W., Huang, Y., Li, S. (2004). Modeling and simulation of point-non-point source effluent trading in Taihu Lake area: perspective of non-point sources control in China. Sci. Total Environ. 325, 39–50. doi: 10.1016/j.scitotenv.2004.01.001
Wang, W., Hu, B., Yuan, D., Liu, Y., Che, R., Hu, Y., et al. (2018). Expression of the nitrate transporter gene OsNRT1.1A/OsNPF6.3 confers high yield and early maturation in rice. Plant Cell. 30, 638–651. doi: 10.1105/tpc.17.00809
Xu, G., Fan, X., Miller, A. J. (2012). Plant nitrogen assimilation and use efficiency. Annu. Rev. Plant Biol. 63, 153–182. doi: 10.1146/annurev-arplant-042811-105532
Yan, M., Fan, X., Feng, H., Miller, A. J., Sheng, Q., Xu, G. (2011). Rice OsNAR2.1 interacts with OsNRT2.1, OsNRT2.2 and OsNRT2.3a nitrate transporters to provide uptake over high and low concentration ranges. Plant Cell Environ. 34, 1360–1372. doi: 10.1111/j.1365-3040.2011.02335.x
Yoneyama, T., Tanno, F., Tatsumi, J., Mae, T. (2016). Whole-plant dynamic system of nitrogen use for vegetative growth and grain filling in rice plants (Oryza sativa L.) as revealed through the production of 350 grains from a germinated seed over 150 days: a review and synthesis. Front. Plant Sci. 7, 1151. doi: 10.3389/fpls.2016.01151
Yong, Z., Kotur, Z., Glass, A. D. (2010). Characterization of an intact two-component high-affinity nitrate transporter from Arabidopsis roots. Plant J. 63, 739–748. doi: 10.1111/j.1365-313X.2010.04278.x
Keywords: Oryza sativa, OsNAR2.1, OsNRT2.3a, co-overexpression, agronomic nitrogen use efficiency, nitrogen recovery efficiency
Citation: Chen J, Liu X, Liu S, Fan X, Zhao L, Song M, Fan X and Xu G (2020) Co-Overexpression of OsNAR2.1 and OsNRT2.3a Increased Agronomic Nitrogen Use Efficiency in Transgenic Rice Plants. Front. Plant Sci. 11:1245. doi: 10.3389/fpls.2020.01245
Received: 30 May 2020; Accepted: 29 July 2020;
Published: 12 August 2020.
Edited by:
Nandula Raghuram, Guru Gobind Singh Indraprastha University, IndiaReviewed by:
Leandro Azevedo Santos, Universidade Federal Rural do Rio de Janeiro, BrazilMuhammad Zafar Iqbal, Sichuan Agricultural University, China
Copyright © 2020 Chen, Liu, Liu, Fan, Zhao, Song, Fan and Xu. This is an open-access article distributed under the terms of the Creative Commons Attribution License (CC BY). The use, distribution or reproduction in other forums is permitted, provided the original author(s) and the copyright owner(s) are credited and that the original publication in this journal is cited, in accordance with accepted academic practice. No use, distribution or reproduction is permitted which does not comply with these terms.
*Correspondence: Xiaorong Fan, eGlhb3JvbmdmYW5AbmphdS5lZHUuY24=
†These authors have contributed equally to this work