- 1Departamento de Biotecnología, Instituto de Agroquímica y Tecnología de Alimentos (IATA), Consejo Superior de Investigaciones Científicas (CSIC), Paterna, Valencia, Spain
- 2Departament de Bioquímica i Biologia Molecular and Estructura de Recerca Interdisciplinar en Biotecnologia i Biomedicina (ERI BIOTECMED), Universitat de València, Burjassot, Valencia, Spain
- 3Instituto de Biología Molecular y Celular de Plantas (IBMCP), Consejo Superior de Investigaciones Científicas (CSIC)—Universidad Politécnica de Valencia (UPV), Valencia, Spain
The present work describes the effects on iron homeostasis when copper transport was deregulated in Arabidopsis thaliana by overexpressing high affinity copper transporters COPT1 and COPT3 (COPTOE). A genome-wide analysis conducted on COPT1OE plants, highlighted that iron homeostasis gene expression was affected under both copper deficiency and excess. Among the altered genes were those encoding the iron uptake machinery and their transcriptional regulators. Subsequently, COPTOE seedlings contained less iron and were more sensitive than controls to iron deficiency. The deregulation of copper (I) uptake hindered the transcriptional activation of the subgroup Ib of basic helix-loop-helix (bHLH-Ib) factors under copper deficiency. Oppositely, copper excess inhibited the expression of the master regulator FIT but activated bHLH-Ib expression in COPTOE plants, in both cases leading to the lack of an adequate iron uptake response. As copper increased in the media, iron (III) was accumulated in roots, and the ratio iron (III)/iron (II) was increased in COPTOE plants. Thus, iron (III) overloading in COPTOE roots inhibited local iron deficiency responses, aimed to metal uptake from soil, leading to a general lower iron content in the COPTOE seedlings. These results emphasized the importance of appropriate spatiotemporal copper uptake for iron homeostasis under non-optimal copper supply. The understanding of the role of copper uptake in iron metabolism could be applied for increasing crops resistance to iron deficiency.
Introduction
Copper (Cu) and iron (Fe) are transition metals with redox properties that form coordination complexes with organic molecules, acting as essential cofactors in numerous proteins, including components of the respiratory and photosynthetic electron transport chains (Puig et al., 2007; Nouet et al., 2011; Yruela, 2013). However, these redox properties also make Cu and Fe potentially toxic since they facilitate the formation of reactive oxygen species (ROS). ROS produce damage at different levels, as they are able to react with proteins, DNA, and lipids in cell membranes, altering their function (Ravet and Pilon, 2013). Metal ion-dependent redox biology constitutes a fundamental theme of aerobic life. Nowadays, both multicopper oxidases (MCO), functioning as metallooxidases, and metalloreductases from the FERRIC REDUCTASE OXIDASE (FRO) family are necessary in redox cycling processes required for metal trafficking in eukaryotic cells (Kosman, 2018). Since evolved in an anaerobic environment, the proto-aerobe organisms developed metalloreductases to supply reduced metals Fe2+ and Cu+ to transporters. Changes in the bioavailability of both metals throughout the evolution of the atmosphere led to a decrease and increase in the Fe and Cu bioavailability, respectively, allowing their substitution as cofactors in different proteins to perform similar functions (Crichton and Pierre, 2001). For instance, in Arabidopsis thaliana, Cu/Zinc (Zn) superoxide dismutase (SOD) is replaced by its Fe counterpart when Cu is scarce (Yamasaki et al., 2007). Metalloprotein substitution contributes to the increase of Fe content under Cu deficiency and vice versa (Waters et al., 2012).
Higher plants have developed sophisticated mechanisms to efficiently acquire and use micronutrients such as Cu and Fe. Cu and specially Fe deficiencies cause losses in agriculture by decreasing the productivity and nutritional value of crops. The deficiency of Fe in agriculture is due to its low bioavailability, especially in alkaline soils (Marschner, 2012). From the two classically described pathways for Fe acquisition in plants, Arabidopsis uses strategy I that is based on the reduction of Fe3+ to Fe2+ by the reductase FRO2 present in the root plasma membrane (Robinson et al., 1999). The ZIP-type divalent cation transporter IRON-REGULATED TRANSPORTER 1 (IRT1) incorporates the Fe2+ into the root cell (Connolly et al., 2002; Varotto et al., 2002; Vert et al., 2002). In the chloroplast, Fe participates in the electron transport chain, chlorophyll biosynthesis, the assembly of Fe/S clusters, and the biosynthesis of heme groups, among other processes (Yruela, 2013). Mitochondria are also high Fe consumer organelles mainly for the electron transport chain and the assembly of Fe/S groups (Nouet et al., 2011). Therefore, due to the possibility of forming ROS, Fe is complexed by the ferritin protein (Reyt et al., 2015). The transcription factor FIT (bHLH29) (FER-like IRON DEFICIENCY INDUCED TRANSCRIPTION FACTOR) is a basic helix-loop-helix (bHLH) that binds to DNA in response of Fe deficiency (Colangelo and Guerinot, 2004). FIT interacts and forms heterodimers with the subgroup Ib of bHLH proteins (bHLH38, bHLH39, bHLH100 and bHLH101), which are required to properly respond to Fe deficiency (Wang et al., 2013). Among the genes activated by FIT are those encoding the reductase FRO2 and the Fe and Cu transporters IRT1 and COPT2, respectively (Colangelo and Guerinot, 2004). FIT activity is regulated at multiple levels by hormones, oxidative stress, and other signals, being considered as a hub modulating the Arabidopsis strategy I response to Fe deficiency (Kobayashi, 2019).
Other regulators of Fe uptake are the IRON MAN/FE-UPTAKE-INDUCING PEPTIDE (IMA/FEP), a group of conserved plant peptides induced under Fe deficiency in vascular tissues (Grillet et al., 2018; Hirayama et al., 2018). Moreover, several phytohormones are involved in modulating Fe deficiency responses in plants. Ethylene, auxin, gibberellin, and salicylic acid function as positive factors of the Fe-deficiency responses, whereas cytokinin, brassinosteroid, abscisic acid, and jasmonic acid act as negative factors of Fe uptake (Kobayashi, 2019). The balance between cellular proliferation and differentiation in the Arabidopsis root has been attributed to the transcription factor UPBEAT1 (UPB1), which regulates this balance through ROS control (Tsukagoshi et al., 2010). Among the upstream regulators is ILR3 (bHLH105), from the subgroup IVc of bHLH transcription factors, which is induced under Fe deficiency and inhibits the expression of genes such as At-NEET, FER1, and FER3 (Tissot et al., 2019). ILR3 and bHLH115 interact with the E3 ubiquitin ligase BRUTUS (BTS), which ubiquitinates them for degradation (Selote et al., 2015). BTS is a protein that participate in Fe sensing, with highly conserved domains including 3 hemerythrin domains, and a protein-protein interaction domain denoted REALLY INTERESTING NEW GENE (RING) (Long et al., 2010; Kobayashi et al., 2013). BTS and its paralogs are negative regulators of Fe assimilatory responses (Hindt et al., 2017).
Cu is found in the form of Cu2+ in the soil, although under metal deficiency it is introduced in the root cells as Cu+ by high affinity Ctr transporters, denoted COPT (COPPER TRANSPORTERS) in plants (Puig, 2014; Peñarrubia et al., 2015). Previous to Cu+ uptake, reductases, from the FRO family participate in the reduction of Cu2+ (Bernal et al., 2012). The Ctr protein family is conserved in eukaryotes and mediates cellular Cu+ acquisition (Nevitt et al., 2012). The recently solved X-ray structure of a Ctr member has confirmed that each monomer contains three transmembrane segments that assemble as homotrimers or heterotrimers and that Cu+ is incorporated through a central pore (Ren et al., 2019). From the six genes identified in Arabidopsis that encode COPT transporters (Sancenón et al., 2003; Garcia-Molina et al., 2013), COPT1 and COPT3 are both induced by Cu deficiency and their encoded proteins are located into plasma membrane and at a compartment of the secretory pathway, respectively (Andrés-Colás et al., 2010; Andrés-Colás et al., 2018). The phenotypes of plants with altered levels of COPT1 and COPT3 correlate with their expression at the pollen grains and suggest a predominant role of COPT1, also expressed at the root tip, in the acquisition of Cu from the soil (Sancenón et al., 2004; Andrés-Colás et al., 2010; Andrés-Colás et al., 2018). Constitutive COPT1 and COPT3 overexpression (COPTOE plants) lead to increased Cu uptake, oxidative stress, and phenotypes related to altered circadian rhythms (Andrés-Colás et al., 2010; Rodrigo-Moreno et al., 2013; Perea-García et al., 2016; Sanz et al., 2019). Furthermore, the Arabidopsis COPT1 transporter has been overexpressed in Oryza sativa plants, leading to increased Fe content in polished grains (Andrés-Bordería et al., 2017). These results underscore the effects of Cu status on Fe traffic and mobilization. Moreover, COPT2 is the Arabidopsis transporter with the highest expression along the root, and plants defective in COPT2 are more resistant to double Cu and Fe deficiency (Perea-García et al., 2013). In fact, the COPT2 promoter contains E-Box elements to which FIT binds (Colangelo and Guerinot, 2004). On the other hand, Cu deficiency involves the regulation of many metabolic processes to allocate the little Cu present to essential proteins. The Zn finger transcription factor SQUAMOSA-PROMOTER BINDING-LIKE PROTEIN 7 (SPL7) binds to GTAC consensus sequences present in the promoters of various genes that are expressed under Cu deficiency, such as COPT2 (Yamasaki et al., 2009; Bernal et al., 2012). The presence of both cis regulatory elements justifies COPT2 induction in the double deficiency of Cu and Fe (Perea-García et al., 2013).
The importance of the regulation of metal homeostasis, at both the cellular and the systemic levels, and its implications in agriculture and human health is evident. However, the molecular mechanisms underlying the interaction between both metals remain poorly understood (Gulec and Collins, 2014). As far as metal homeostasis networks for a specific metal are becoming well understood, the crosstalk between different metals is starting to emerge as a possibility to improve global metal nutritional levels for optimal organismal performing.
Materials and Methods
Plant Growth Conditions and Treatments
Seeds of A. thaliana, ecotype Columbia-0 (Col-0) and of the transgenic lines COPT1OE and COPT3OE were surface-sterilized and stratified for 2 days at 4°C and were germinated in ½ MS medium (Sigma) plates including 1% sucrose (Murashige and Skoog, 1962) (½ MS) or supplemented with 10 μM CuSO4 (½ MS + 10 Cu) for the microarray analysis. Seedlings were grown as previously described (Andrés-Colás et al., 2010) for 7 days with a 12 h neutral photoperiod (65 μmol m−2 of cool-white fluorescent light) at 23°C/16°C temperature cycle.
In order to obtain ½ MS medium with the indicated concentrations of either Cu or Fe, the solution was prepared by adding macronutrients (Sigma) and micronutrients consisted in a mix of 50 μM H3BO3, 36.6 μM MnSO4 H2O, 15 μM ZnSO4 7H2O, 0.57 μM NaMoO4 2H2O, 0.25 mM KI, and 0.05 μM CoCl2 6H2O. Finally, 0.05% MES, 1% sucrose, and 0.8% phytoagar was added, and the pH was adjusted to 5.7–5.8 with diluted KOH. The Cu concentration in the Cu deficiency (0 μM CuSO4) media including commercial phytoagar (Duchefa Biochemie) measured by ICP-MS is <0.008 μM Cu. To study the effects that the different Cu and Fe content have in plants, 50 μM Fe-citrate and 1 μM CuSO4 5H2O were added to the medium for Cu and Fe sufficiency conditions. Moreover, seedlings were grown in Cu deficiency (0 μM CuSO4) and Cu excess (10 μM CuSO4). On the other hand, Fe-sufficient and slight or severe Fe deficiency medium was supplemented with 50, 10 and 0 μM Fe-citrate, respectively. Other metal concentrations and treatments were used for specific experiments as indicated in the Supplementary Figure Legends.
The chlorophyll content in seedlings and leaves was determined by the trichlorometric method (Parsons and Strickland, 1965). Root length was measured using the Image J 1.42q software (http://rsb.info.nih.gov./ij). Values represent the arithmetic mean ± standard deviation (SD) of three biological replicates (n = 3).
For the determination of ferroreductase activity, three seedlings of 7-day-old were collected and weighed. Next, a 1:1 mixture made with 300 μM bathophenantroline disulfonate (BPDS, Sigma) and 100 μM Fe III-EDTA was added to the seedlings and incubated at 30°C with stirring 225 rpm in the dark. After 30 min, the solution was collected and absorbance A535 was measured in a spectrophotometer (Grillet et al., 2014). Values represent the arithmetic mean ± standard deviation (SD) of three biological replicates (n = 3).
For root Fe3+ detection by Perl’s staining, four to five seedlings of 11-day-old were vacuum infiltrated with equal volumes of 4% (v/v) HCl and 4% (w/v) K- ferrocyanide (Perl´s stain solution for 15 min) and incubated at room temperature for 30 min (Stacey et al., 2008). One representative photograph is shown in the figure. This method is based on the Fe3+ dependent conversion of ferrocyanide into insoluble crystals of Prussian blue under acidic conditions. Localization of Fe3+ was observed and analyzed with a (Olympus CX41) microscope equipped with (Leyca MC170HD) camera and (LAS V4.10) software.
Microarrays and Bioinformatics
Seven-day-old seedlings of the WT and the COPT1OE line were grown in the 12 h neutral photoperiod and three biological replicates were obtained for the (½ MS) treatment and four biological replicates were used as 10 μM CuSO4, (½ MS + 10 Cu) samples. Total RNA was isolated using the RNeasy Plant Mini Kit (Qiagen) and aRNA was amplified using the MessageAmp™ II aRNA Amplification kit (Ambion). Long oligonucleotide microarrays were provided by Dr. David Galbraith (University of Arizona, http://www.ag.arizona.edu/microarray/). The hybridization and analysis were performed as described elsewhere (Bueso et al., 2007). The expression values (log2) were obtained using the GenePix Pro 6.0 microarray-analysis software (Molecular Devices, Sunnyvale CA) and normalized with the GenePix Pro 6.0 and Acuity 4.0 software (Molecular Devices, Sunnyvale CA). Differential genes were identified with significance analysis of microarray (SAM) (Tusher et al., 2001) with false discovery rate (FDR) of <6% and 2-fold change (log2 ≤|1|). Biological processes were identified with the Gene Ontology (GO) annotation (Ashburner et al., 2000), performed by the GeneCodis2.0 (http://genecodis.dacya.ucm.es/) (Carmona-Saez et al., 2007; Nogales-Cadenas et al., 2009) program (Table 1). The total differentially regulated genes are shown as Supplementary material (Supplementary Tables SI and SII). The microarray raw data were deposited in the NCBI’s Gene Expression Omnibus (Edgar, 2002) and are accessible through GEO Series accession number GSE143857.
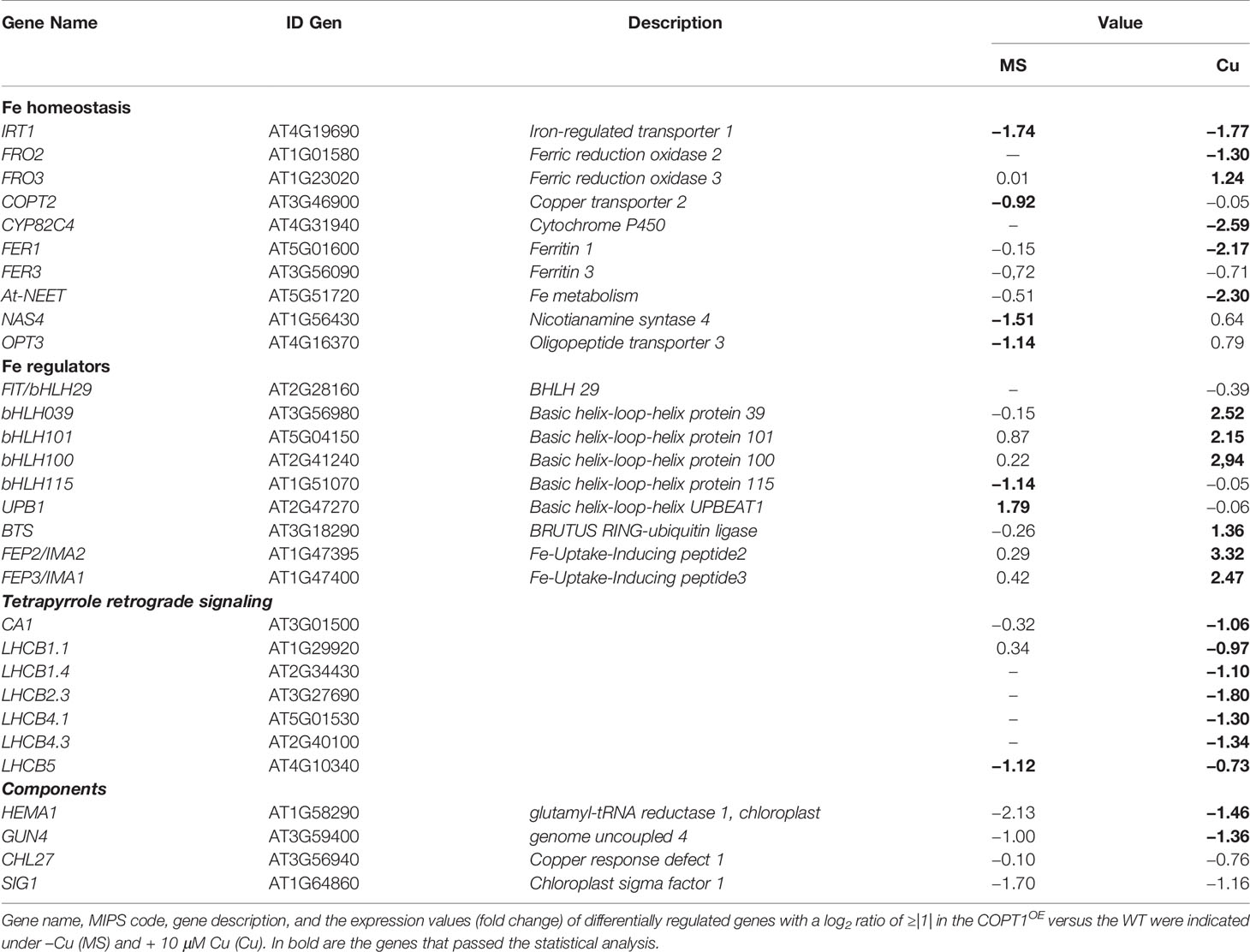
Table 1 Differentially expressed iron- and tetrapyrrole-related genes in COPT1OE vs. WT seedlings in low and high Cu.
Gene Expression by Real-Time Quantitative PCR
Total Arabidopsis RNA was isolated using the RNeasy Plant Mini Kit (Qiagen), was quantified by UV spectrophotometry and its integrity was visually assessed on ethidium bromide-stained agarose gels. After treatment with Dnase I Amp Grade (Invitrogen), cDNA was generated by retro-transcriptase SSII (Invitrogen) as previously described (Andrés-Colás et al., 2006). Real-time quantitative PCR (RT-qPCR) was carried out with SYBR-Green qPCR Super-Mix-UDG with ROX (Invitrogen) with the specific primers detailed in Table SVI in a CFX96 Touch™ Real Time PCR Detection System (BioRad), with one cycle of 95°C for 2 min and 40 cycles consisting in 95°C for 30 s and 60°C for 30 s. Expression values were normalized to UBQ10 and to the WT in deficiency conditions using the 2−ΔΔCt method. Values represent the arithmetic mean ± standard deviation (SD) of three biological replicates (n = 3).
Oxygen Consumption Determination
To study O2 consumption, 14–16 roots of 10-day-old seedlings, grown in different conditions, were used. The roots were cut with a scalpel and resuspended in 1.5 ml of ½ MS liquid medium, as it is described above but without sucrose. The roots were transferred to an airtight chamber and the measurement of O2 consumption for a minimum of 5 min was performed using a Clark type electrode (Oxyview system). The rate of decrease of O2, referenced to fresh weight (F.W.) of the roots (nmol O2/OD600 × F.W.) was taken as an index of respiratory capacity. Values represent the arithmetic mean ± standard deviation (SD) of three biological replicates (n = 3).
Metal and Hormone Determinations
Cu and Fe contents were determined by ICP-MS as described previously (Andrés-Colás et al., 2006; Carrió-Seguí et al., 2015) at the Servei Central de Suport a la Investigació Experimental (SCSIE) of the Universitat de València.
For the determination of the content of ABA, indol-3-acetic acid (IAA), and JA, 8-day-old seedlings were lyophilized, processed and analyzed by UHPLC (ultra-high-pressure liquid chromatography) Q-Exactive (ThermoFisher Scientific) as described previously (da Silva et al., 2017) in the plant hormone quantification service of the Institute of Molecular and Cellular Plant Biology (IBMCP, Valencia). Values represent the arithmetic mean ± standard deviation (SD) of three biological replicates (n = 3).
Statistical Analysis
The statistical analysis of the relative gene expression was performed by the pair wise fixed reallocation randomization test (p-value <0.05) (Pfaffl, 2002). For the remaining parameters, the analysis was carried out using one or two-way ANOVA with the means compared by the Duncan test or a Kruskal–Wallis (p-value <0.05) test for a non-parametric measurements using the InfoStat software, version 2010 (http://www.infostat.com.ar) (Di Rienzo et al., 2011).
Results
A Genome-Wide Expression Analysis Highlights That Iron Homeostasis Is Affected in COPT1OEArabidopsis Plants
To identify at a molecular level the global effects caused by the deregulation of Cu homeostasis in Arabidopsis, we performed a comparative transcriptomic analysis of 7-day-old wild-type (WT) and a previously generated COPT1 overexpressing (COPT1OE) line (Andrés-Colás et al., 2010), grown under Cu deficiency (½ MS) and mild Cu excess (½ MS + 10 Cu) conditions. The induction of COPT1 expression under Cu deficiency in the WT was corroborated by RT-qPCR, as well as its overexpression in COPT1OE seedlings, both under deficiency and excess Cu conditions, in the samples that were used in the hybridizations of the DNA microarrays (data not shown). From the global analysis of gene expression, a total of 583 differentially expressed genes were identified with a log2 ratio of ≥|1| in the COPT1OE versus WT (Supplementary Figure S1 and Tables SI and SII). These were distributed in a total of 482 induced (ratio ≥1) and of 101 repressed genes (ratio ≥−1) in COPT1OE seedlings for the two growth conditions tested (Supplementary Figure S1 and Supplementary Tables SI and SII).
The number of genes induced was greater than that of genes repressed in the two conditions. On the other hand, the mild Cu excess condition showed the greatest number of genes with differential expression compared to Cu deficiency, with 393 genes (312 induced and 81 repressed) compared to 160 genes (142 induced and 18 repressed), respectively. The analysis of the gene ontology (GO) of differentially regulated genes between WT and COPT1OE seedlings indicated that several the Biological Processes categories were overrepresented, including those linked to photosynthesis, photomorphogenesis, transport of metals such as Fe, Zn, manganese, cadmium, and phosphate, oxidative stress and responses to abscisic acid (ABA) and cold stress (Supplementary Table SIII). On the other hand, the Molecular Function of genes differentially regulated in COPT1OE included the chlorophyll and tetrapyrrole binding (Supplementary Table SIV). GO analysis also revealed that the chloroplast was the subcellular compartment most affected by the changes observed in COPT1OE, as 39% of the significantly enriched GO categories of Cellular Compartment are related to chloroplast (chloroplast, chloroplast envelope, thylakoid membrane, chloroplast membrane, stroma, photosystems I and II, and light-harvesting complex) (Supplementary Table SV). The Fe-related genes studied in this work, which expression was affected in COPT1OE plants, are summarized in Table 1.
Fe Assimilation in the COPTOE Seedlings
One of the most relevant result of the transcriptomic analysis in COPT1OE is the altered expression of Fe-related genes (Table 1), such as IRT1 and FRO2, involved in the strategy I of Fe uptake (Table 1). Expression of the Fe transporter IRT1 was reduced in COPT1OE seedling in both Cu deficiency (½ MS) or excess (½ MS + 10 Cu). In contrast, the genes encoding FRO reductases showed a different behavior; expression of FRO2 was induced but that for FRO3 was repressed in COPT1OE seedlings only in Cu excess. In order to further assess the effects of Cu on their expression, seedlings were grown on hand-made ½ MS medium with three different CuSO4 concentrations, including Cu deficiency (0 μM CuSO4), sufficiency (1 μM CuSO4), and mild Cu excess (10 μM CuSO4). Furthermore, in addition to COPT1OE, plants overexpressing the COPT3 transporter (COPT3OE) (Andrés-Colás et al., 2010) were also included to further assess the effect of deregulated Cu+ entrance (Figure 1). IRT1 expression slightly decreased in the WT seedlings as Cu increased in the medium, being significantly lower under Cu sufficiency and excess compared to deficiency conditions (Figure 1A). Similar to the global transcriptomic analysis, IRT1 expression in COPTOE (COPT1OE and COPT3OE lines) was lower than in WT under both Cu deficiency and excess. Since genes encoding reductases FRO2 and FRO3 displayed distinct regulation in COPT1OE seedlings (Table 1), total ferroreductase activity was measured in the roots of 7-day-old COPTOE seedlings (Figure 1B). No changes in ferroreductase activity were observed under Cu deficiency and sufficiency. However, a slightly lower ferroreductase activity was observed under Cu excess in the COPT1OE plants compared to the WT (Figure 1B).
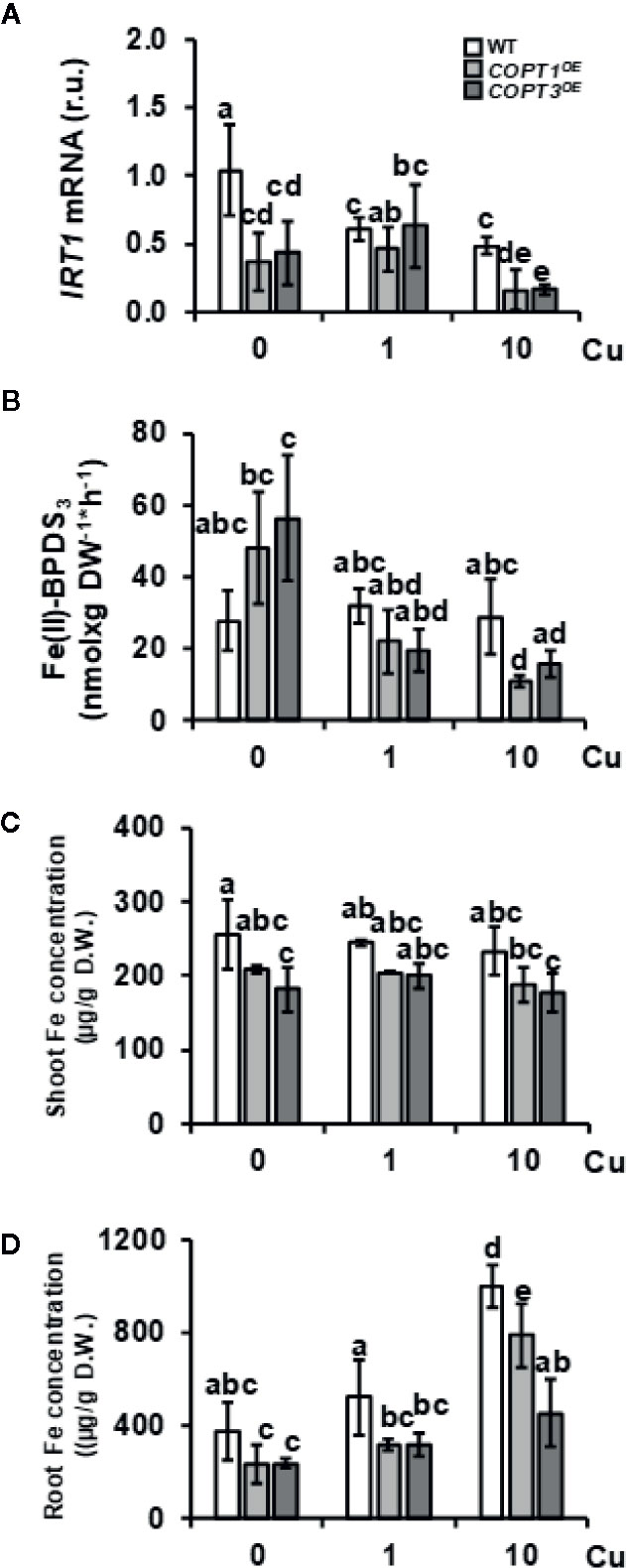
Figure 1 Expression of strategy I components of Fe uptake in COPTOE seedlings. (A) Relative expression of IRT1 gene in 7-day-old WT (white bars), COPT1OE (light grey bars), and COPT3OE (dark grey bars) seedlings grown under Cu deficiency (0 μM CuSO4), sufficiency (1 μM CuSO4) or Cu excess (10 μM CuSO4) was determined by RT-qPCR. Gene expression is represented as relative expression levels (r.u.) in relation to the WT under control conditions. UBQ10 was used as housekeeping gene. Bars correspond to arithmetic means (2−ΔΔCt) ± SD of biological replicates (n = 3). (B) Ferroreductase activity in 7-day-old WT and COPTOE seedlings grown in the same conditions as in (A) measured at A535 nm. Bars correspond to arithmetic means ± SD of biological replicates (n = 3) (C) Shoot and (D) root Fe concentration in 7-day-old WT, COPT1OE and COPT3OE seedlings grown on CuSO4 media specified in (A) and determined as μg/g of dry weight (D.W.). Bars correspond to arithmetic means ± SD of biological replicates (n = 3). Samples with a letter in common are not significantly different (p-value <0.05). D.W., dry weight.
COPT1OE and COPT3OE seedlings were previously shown to incorporate more Cu, both under metal deficiency and excess in the growth medium (Andrés-Colás et al., 2010). Now, the Fe content was determined by ICP-MS in both shoots and roots from 7-day-old WT and COPTOE seedlings grown under the different Cu conditions (Figures 1C, D). We observed that COPTOE seedlings had slightly lower endogenous Fe concentration than the WT both in shoots and roots but only significant under Cu sufficiency and excess. This decrease was more evident in the roots of the COPT3OE seedlings (Figure 1D), which is consistent with their exacerbated reduction in IRT1 expression (Figure 1A).
To corroborate the expression changes of other Fe-related genes involved in Fe storage and metabolism in COPT1OE seedlings, we further investigated the expression of genes included in these processes, such as FER1, FER3, and At-NEET (Table 1 and Figure 2). Previous data have shown that the expression of FER1 is higher among the four genes (FER1, FER2, FER3 and FER4) that encode ferritins (Petit et al., 2001). FER1 and FER3 expression was down-regulated under Cu excess compared to Cu deficiency in WT and this decrease was more exacerbated in COPTOE seedlings (Table 1 and Figures 2A, B). This pattern is also in agreement with the lower Fe content in the COPTOE under Cu excess (Figure 1C). At-NEET encodes a Fe/S protein involved, among other processes, in the Fe metabolism and ROS homeostasis (Nechushtai et al., 2012; Mittler et al., 2019). At-NEET expression was induced under Cu deficiency in the WT and reduced in the COPTOE seedlings under all the Cu conditions studied (Table 1 and Figure 2C). The expression of FER1, FER3, and At-NEET was also analyzed under different Cu and Fe contents in WT seedlings (Supplementary Figure S2). Whereas all of them were induced under Cu deficiency compared to control conditions, only FER1 expression was repressed under Fe deficiency. FER3 and At-NEET were not regulated by Fe levels (Supplementary Figure S2). Altogether, these results indicate that the deregulated Cu+ entrance in COPTOE prevents the induction of genes involved in Fe uptake, leading ultimately to a reduced endogenous Fe content, and suggesting that overexpression of COPT may alter the local response to Fe deficiency in roots.
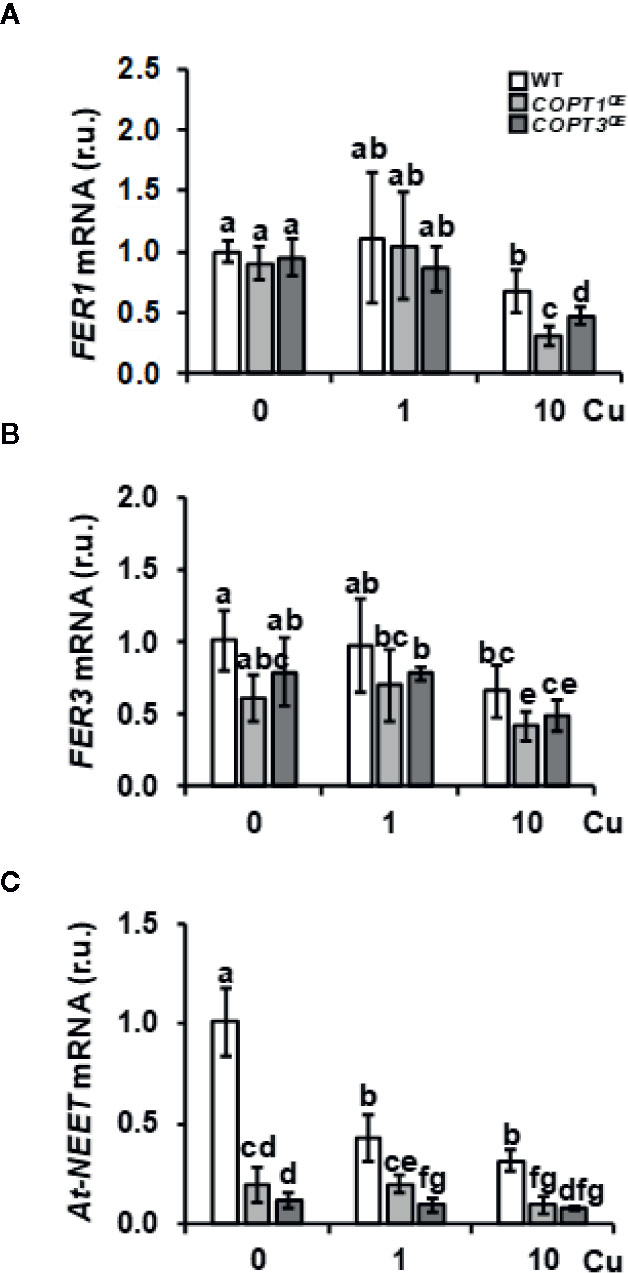
Figure 2 Expression of Fe metabolism genes in COPTOE seedlings. Relative expression of FER1 (A), FER3 (B), and At-NEET (C) genes in WT (white bars), COPT1OE (light grey bars), and COPT3OE (dark grey bars) seedlings grown under Cu deficiency (0 μM CuSO4), sufficiency (1 μM CuSO4) or Cu excess (10 μM CuSO4) was determined by RT-qPCR. Gene expression is represented as relative expression levels (r.u.) in relation to the WT under control conditions. UBQ10 was used as housekeeping gene. Bars correspond to arithmetic means (2−ΔΔCt) ± SD of biological replicates (n = 3). Samples with a letter in common are not significantly different (p-value <0.05).
Expression of Regulators of Fe Homeostasis in the COPTOE Seedlings
Certain Fe deficiency responses are under the control of the Cu-responsive SPL7 transcription factor (Kastoori Ramamurthy et al., 2018). To determine whether the inhibition of IRT1 expression observed in COPTOE could be a SPL7-mediated response, we checked the expression in COPTOE seedlings of SPL7 and SPL7-regulated markers of Cu deficiency responses, such as COPT2 and FSD1 (Supplementary Figure S3), the latter encoding FeSOD (Yamasaki et al., 2009). SPL7 expression remained mostly unaffected in COPTOE. Whereas COPT2 expression was down-regulated in COPTOE seedlings, FSD1 expression was not affected under Cu deficiency (Supplementary Figures S3B, C). Furthermore, other SPL7 targets were not differentially expressed in the COPT1OE seedlings under Cu deficiency (Tables SI and SII), indicating that the SPL7 factor was properly functioning in COPTOE seedlings and it was not differentially affecting Cu deficiency responses in these plants. On the other hand, the expression of CSD2, encoding the chloroplastic Cu/Zn SOD CSD2, was reduced in COPTOE seedlings under Cu sufficiency and excess (Supplementary Figure S3D).
To further address the role of Cu in the lack of Fe-deficiency response, we analyzed the expression of Fe-related bHLH transcription factors and other regulators (Table 1 and Figure 3). A slight increase and a lower expression were observed for FIT under Cu deficiency and excess, respectively, in COPT1OE seedlings compared to the WT (Figure 3A). Consistent with FIT inhibition under Cu excess, the expression of two of its targets, IRT1 and COPT2, was slightly repressed in COPTOE seedlings (Figure 1A and Supplementary Figure S3B), which suggests that high Cu in these plants represses the activity of this master Fe uptake regulator. The expression of the subgroup Ib of Fe-related bHLH (bHLH38, bHLH39, bHLH100 and bHLH101) transcription factors was also analyzed (Figures 3B, C, Supplementary Figures S4A, B). We observed that, in the WT plants, expression of bHLH-Ib factors was in general greater as Cu increased in the growth medium. Remarkably, its expression was highly increased in COPT1OE seedlings under Cu excess conditions (Figure 3 and Supplementary Figure S4). Therefore, the opposite regulation in COPT1OE seedlings under Cu excess was observed for FIT and the rest of regulatory bHLH-Ib factors. Whereas FIT expression was reduced under Cu excess in COPTOE respect to the WT, bHLH-Ib expression was increased under Cu excess (Figure 3 and Supplementary Figure S4).
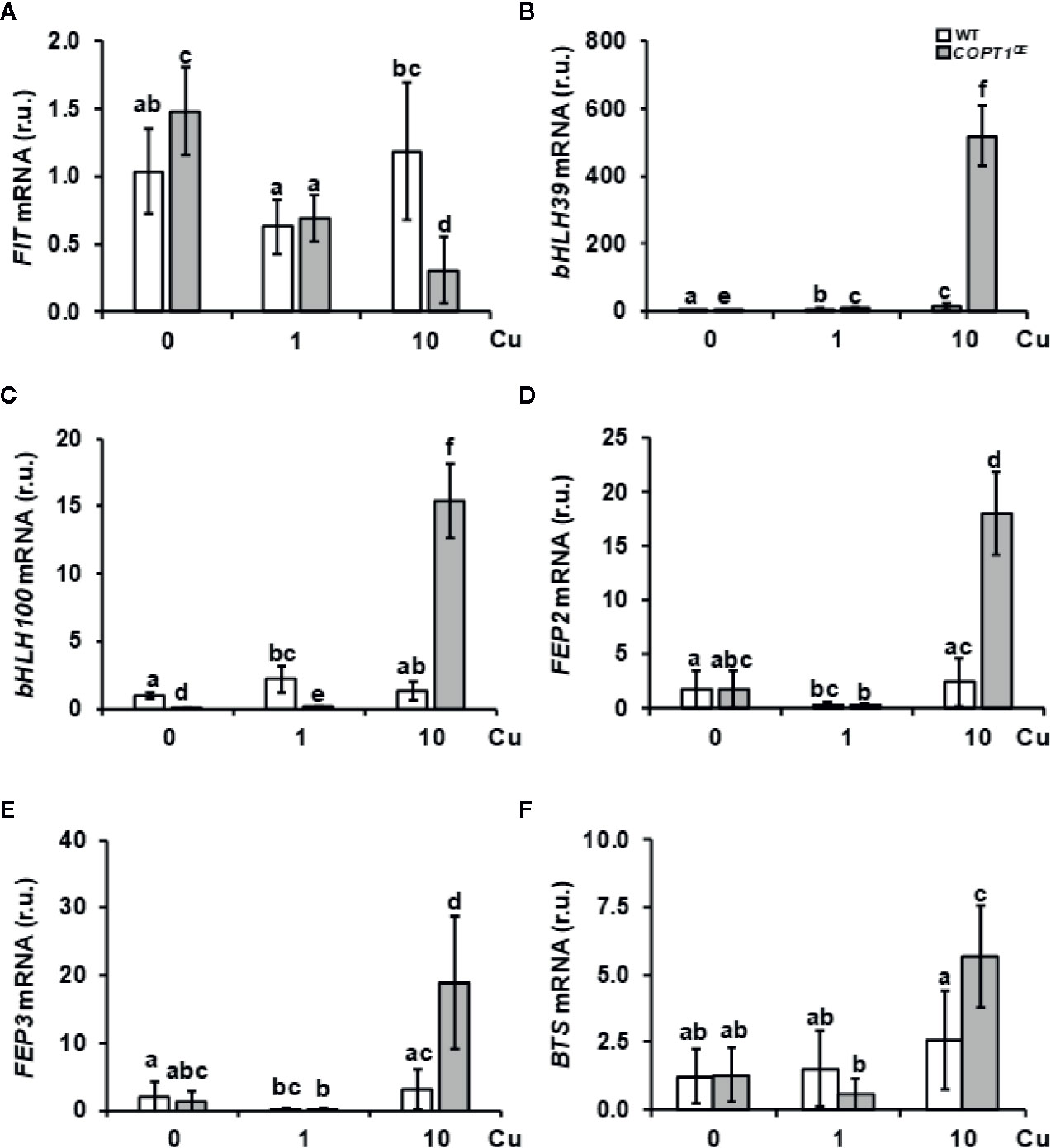
Figure 3 Gene expression of Fe homeostasis regulators in COPT1OE seedlings. The 7-day-old WT (white bars) and COPT1OE plants (grey bars) seedlings grown under Cu deficiency (0 μM CuSO4), sufficiency (1 μM CuSO4) or Cu excess (10 μM CuSO4). Expression of FIT (A), bHLH39 (B), bHLH100 (C), FEP2 (D), FEP3 (E), and BTS (F) was determined by RT-qPCR. Gene expression is represented as relative expression levels (r.u.) in relation to the WT under control conditions. UBQ10 was used as housekeeping gene. Bars correspond to arithmetic means (2−ΔΔCt) ± SD of biological replicates (n = 3). Samples with a letter in common are not significantly different (p-value <0.05).
Among the most induced Fe-related genes in the COPT1OE line under Cu excess were the Fe peptide regulators FEP2 and FEP3 (Table 1). The expression of these responds to Fe deficiency in a FIT-independent manner (Hirayama et al., 2018). In agreement with low Fe levels in the COPT1OE plants, FEP2 and FEP3 were clearly induced under Cu excess (Figures 3D, E). This result indicated that the FIT-independent signaling pathway for FEP2 and FEP3 induction was not affected by deregulated Cu uptake in COPT1OE under Cu excess.
Taken together these results indicate that strategy I local responses to Fe deficiency cannot take place in COPTOE seedlings under Cu excess despite the increased expression in bHLH-Ib transcription factors and FEP genes. This is probably due to the inhibition of FIT expression (Figure 3A), whereas the opposite occurs under Cu deficiency where, despite slightly enhanced FIT expression in the COPT1OE seedlings, the low bHLH-Ib expression (Figure 3 and Supplementary Figure S4) was precluding assimilatory Fe deficiency responses.
Regarding to upstream regulators of Fe homeostasis that were repressed in COPT1OE (Table 1), we analyzed the expression of the subgroup IVc members of bHLH (bHLH105/IRL3 and bHLH115) transcription factors. IRL3 and bHLH115 were repressed in COPT3OE (Supplementary Figures S4C, D). On the other hand, BTS expression encoding an E3 ubiquitin ligase that participates in Fe sensing (Long et al., 2010; Kobayashi et al., 2013) was enhanced under Cu excess in WT plants and further increased in COPT1OE (Figure 3F). This increased expression in BTS and the reduced Fe uptake response under Cu excess (Figure 1) was according to the BTS role as a negative regulator of Fe assimilatory responses (Hindt et al., 2017).
Plant hormones are involved in regulating the expression of Fe deficiency-responsive genes (Kobayashi, 2019). In order to check if hormone contents were significantly affected in COPT1OE seedlings, abscisic acid (ABA), jasmonic acid (JA), and indol acetic acid (IAA) contents were determined (Supplementary Figure S5). Whereas the ABA levels were not significantly modified with respect to controls, JA and IAA levels decreased in COPT1OE seedlings as Cu increased in the medium (Supplementary Figure S5).
Phenotype of COPTOE Seedlings Under Fe Deficiency
Given the previous data, we studied the phenotype of COPTOE seedlings under Fe deficiency (Figure 4). Thus, 7-day-old WT, COPT1OE, and COPT3OE seedlings were grown in media with Fe sufficiency (50 μM FeSO4) and slight Fe deficiency (10 μM FeSO4) (Figure 4). Whereas WT seedlings increased their root length under slight Fe deficiency conditions, COPTOE presented a similar length of their roots in both media (Figures 4A, B). Root shortening in the COPTOE with respect to WT under slight Fe deficiency was around 50%. No changes were observed in the seedlings fresh weight under sufficiency, while under slight Fe deficiency, the weight was reduced in COPT1OE (Figure 4C). Moreover, the total chlorophyll content did not show significant changes in the WT, while COPTOE seedlings displayed around 50% lower chlorophyll content in Fe deficiency (Figure 4D). Taken together, these data indicate that COPTOE seedlings are more sensitive to slight Fe deficiency conditions than WT.
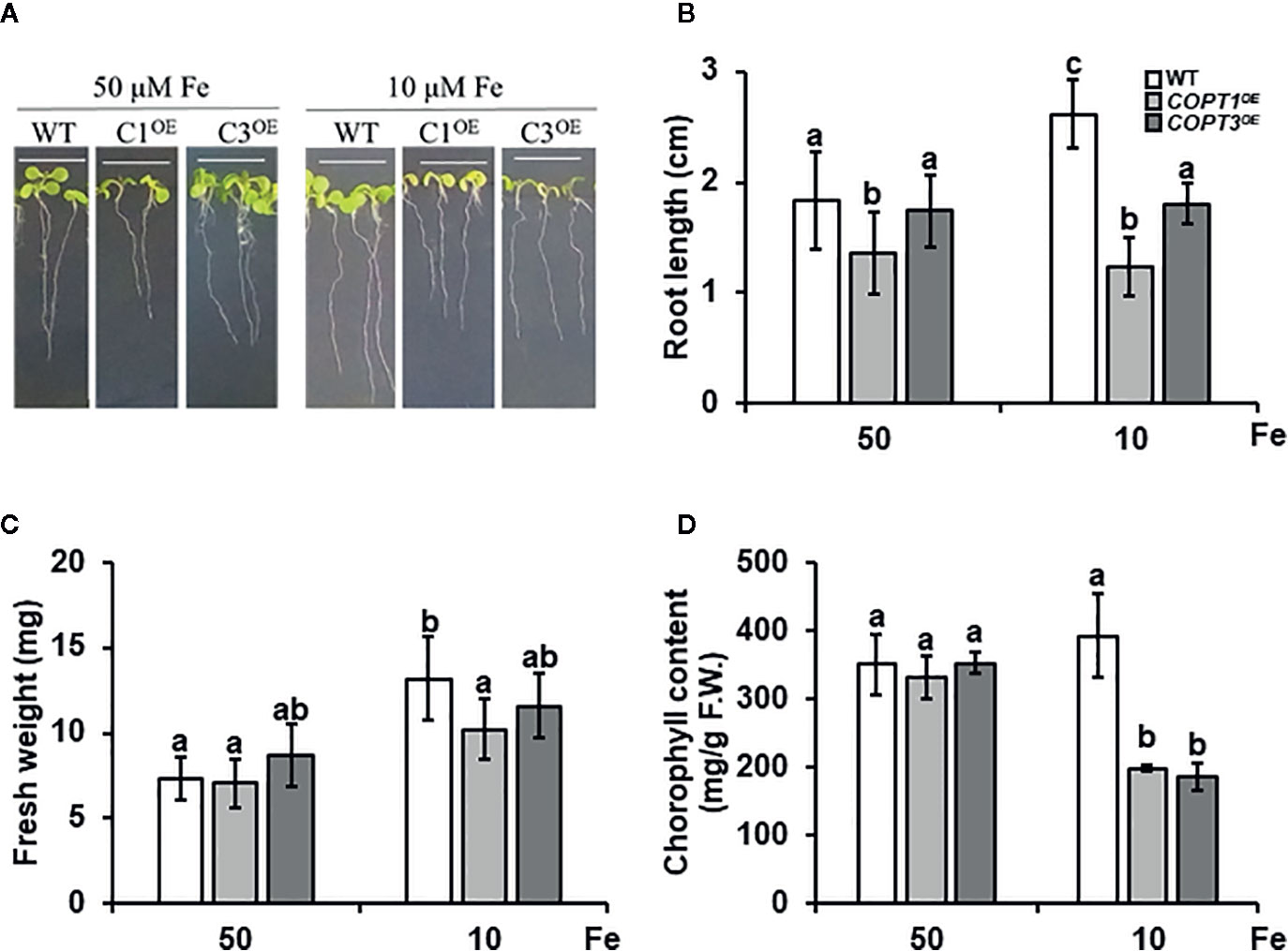
Figure 4 Physiological characterization of COPTOE seedlings under Fe deficiency. (A) Representative photographs of 7-day-old seedlings of WT, COPT1OE and COPT3OE under Fe sufficiency (50 μM FeSO4) or slight Fe deficiency (10 μM FeSO4). White scale bars represent 1 cm. (B) Root length of the of WT (white bars), COPT1OE (light grey bars), and COPT3OE (dark grey bars) under the same growth conditions as in (A). (C) Fresh weight of 5 WT and COPT1OE seedlings grown under the same conditions as in (A). (D) Total chlorophyll content of WT and COPTOE seedlings grown under the same conditions as in (A). Bars correspond to arithmetic means (2−ΔΔCt) ± SD of biological replicates (n = 3). Samples with a letter in common are not significantly different (p-value <0.05).
Then, we analyzed the expression of Fe homeostasis-related genes under Fe sufficiency and deficiency. IRT1 expression increased under Fe deficiency in both WT and COPTOE (Supplementary Figure S6A). On the other hand, an increase in FIT expression was observed under Fe deficiency in the WT plants, while in COPTOE this increase was exacerbated (Supplementary Figure S6B). Furthermore, FEP2 and FEP3 expression was induced in WT plants under Fe deficiency but it was also highly increased in COPT1OE (Supplementary Figures S6C, D). Taken together, these results indicated that COPTOE seedlings properly responded to Fe deficiency under Cu sufficiency and that their defects in Fe homeostasis were restricted to Cu deficiency and excess conditions.
The expression of the Fe homeostasis regulators FIT, bHLH38, bHLH100, and UPB1 was analyzed under different metal deficiency and excess conditions in the WT plants (Supplementary Figure S7). FIT, bHLH38, and bHLH100 where induced under Fe deficiency whereas UPB1 was repressed. Curiously, all of them but FIT were regulated in the same sense under Cu excess and Fe deficiency, either induced (bHLH38 and bHLH100) or repressed (UPB1), suggesting that maybe there are certain similarities or common steps in signal transduction between the responses to Cu excess and Fe deficiency, leading to the induction of these transcriptional factors.
Expression of Genes Involved in Tetrapyrrole Biosynthesis in COPTOE Seedlings
GO analysis of the global transcriptomic analysis revealed that the chloroplast was the subcellular compartment most affected in COPT1OE (Supplementary Table SV). GO categories differentially enriched among genes differentially regulated in COPT1OE seedlings were photosynthesis-associated nuclear genes (PhANG) and genes related to photomorphogenesis, and oxidative stress (Table 1 and Supplementary Tables SIII and SV). Therefore, we decided to assess the alteration of the expression of genes in the tetrapyrrole biosynthesis pathway and the retrograde signaling components (Table 1). The LHCB2.3 expression, a representant among the multiple PhANG, which are down-regulated in the COPT1OE seedlings (Table SII), was confirmed to be repressed (Figure 5A). Moreover, the expression of GUN4, a regulator of the plastidic tetrapyrrole signaling (Davison et al., 2005), was also repressed in most conditions (Figure 5B). In addition, CHL27/CDR1 (Bang et al., 2008) was also down-regulated in COPTOE seedlings (Figure 5C), further confirming the involvement of tetrapyrrole signaling in Cu-dependent Fe deficiency responses. The regulation of a chloroplastic sigma factor SIG1 involved in retrograde signaling (Macadlo et al., 2019) was also down-regulated under Cu deficiency in COPTOE seedlings (Figure 5D). Taken together, these results indicated that the tetrapyrrole signaling pathway was affected under both Cu deficiency and excess, probably as a consequence of the common Fe deficiency conditions faced by COPTOE seedlings.
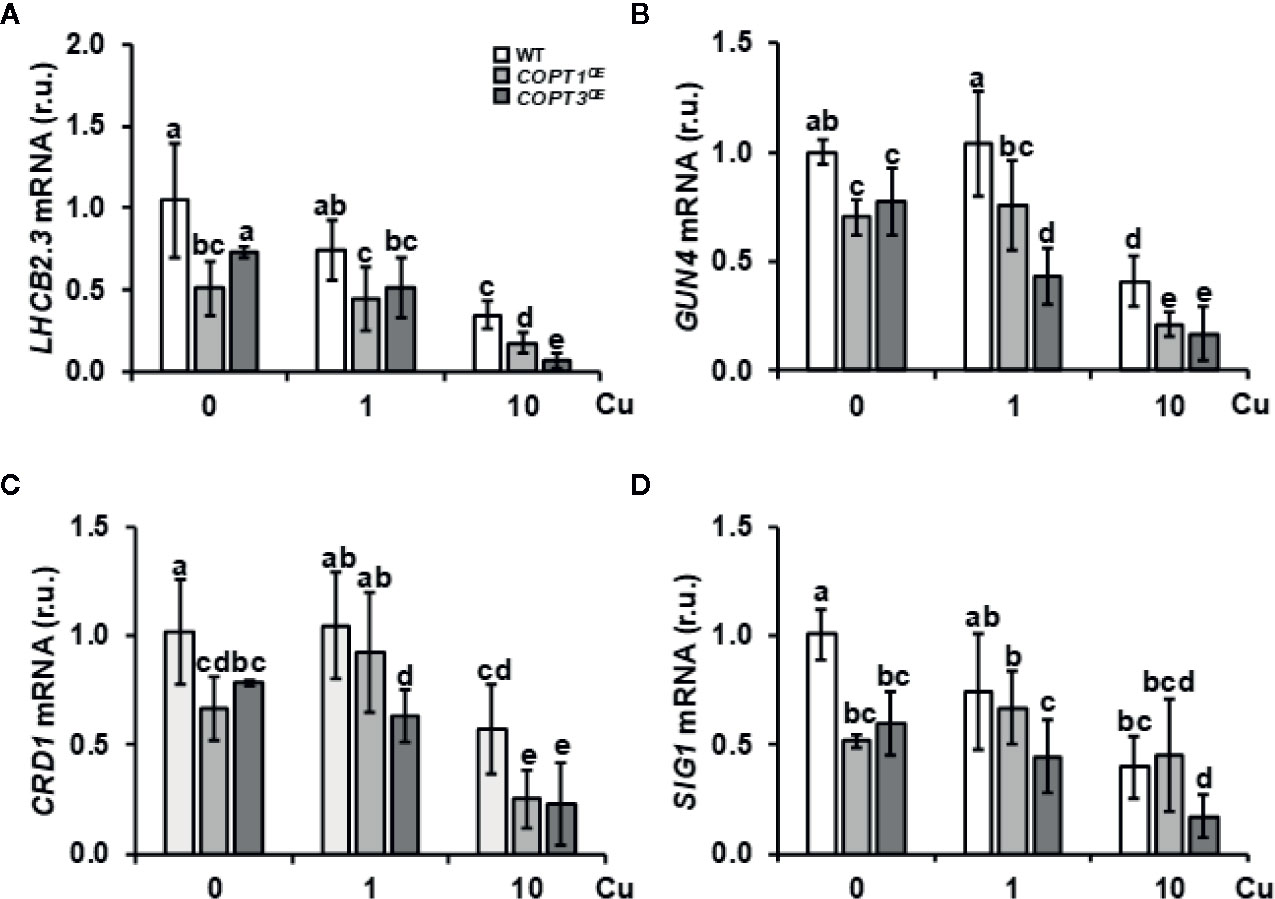
Figure 5 Expression of genes related to tetrapyrrole biosynthesis and retrograde signaling in COPTOE. Expression of the LHCB2.3 (A), GUN4 (B), CDR1 (C), and SIG1A (D) genes in WT (white bars), COPT1OE (light grey bars), and COPT3OE (dark grey bars) seedlings grown under Cu deficiency (0 μM CuSO4), sufficiency (1 μM CuSO4) or Cu excess (10 μM CuSO4) was determined by RT-qPCR. Gene expression is represented as relative expression levels (r.u.) in relation to the WT under control conditions. UBQ10 was used as housekeeping gene. Bars correspond to arithmetic means (2−ΔΔCt) ± SD of biological replicates (n = 3). Samples with a letter in common are not significantly different (p-value <0.05).
Root Respiration and Oxidative Stress in COPTOE Seedlings
Since the respiratory electron transport chain is one of the most metal requiring processes, O2 consumption was measured in 7-day-old roots of WT and COPT1OE seedlings grown in deficiency (0 μM CuSO4), sufficiency (1 μM CuSO4) and excess CuSO4 (10 μM CuSO4) (Figure 6A). The results showed that O2 consumption in WT seedlings did not exhibit significant changes between the three media, while COPT1OE displayed lower O2 consumption when the Cu conditions were not optimal, in both deficiency and in excess, with a decrease of 42 and 46% respectively, compared to WT (Figure 6A). In agreement with a defective functioning of the respiratory electron transport chain, expression of the ALTERNATIVE OXIDASE 1D (AOX-1D), aimed to restore electron flow in a non-phosphorylating bypass (Clifton et al., 2006; Selinski et al., 2018), was increased in COPT1OE (Figure 6B). Moreover, other AOX genes, such as AOX1A (+2.46) and AOX2 (+2.08), were also induced under Cu excess in COPTOE (Supplementary Table SI). On the other hand, expression of the LOW SULPHUR UPREGULATED1 (LSU1) has been shown to prevent chloroplastic ROS production by interacting with FSD2 (Garcia-Molina et al., 2017). LSU1 was also greatly induced under Cu excess (Figure 6C), as well as LSU2 and LSU3 (Supplementary Table SI). Inhibition of FSD1 and CSD2 expression in the COPTOE plants (Supplementary Figure S3) suggested a defective antioxidant capacity by an increase in the radical superoxide (O2.−) and a decrease in hydrogen peroxide (H2O2).
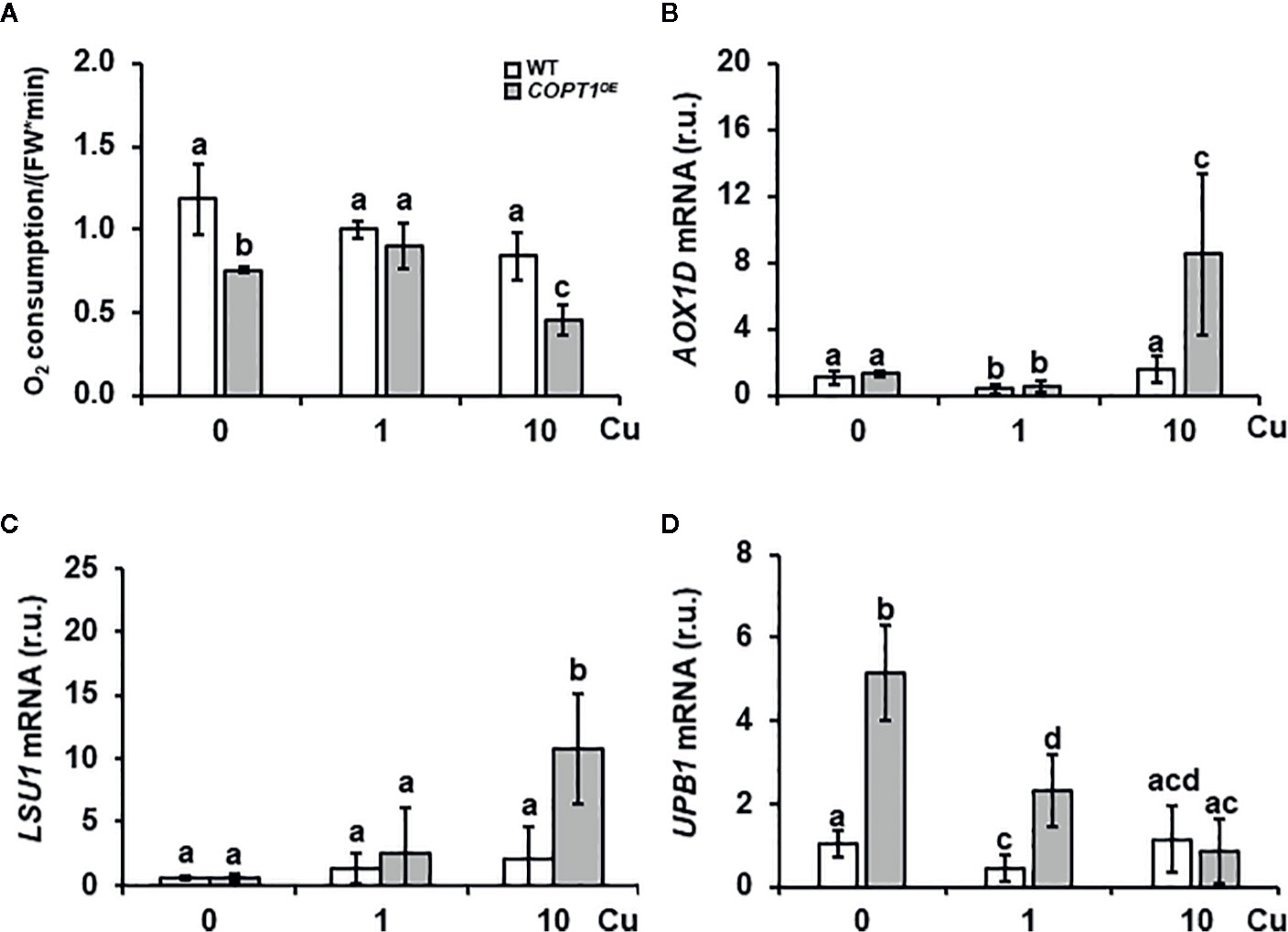
Figure 6 Root O2 consumption and expression of stress-related genes in COPT1OE. (A) O2 consumption in roots of 10-day-old WT and COPT1OE seedlings cultivated with different CuSO4 concentrations. The rate of O2 decrease was referenced to roots F.W. (nmol O2/OD600 * F.W). The values represented are the arithmetic mean ± SD of n = 3 biological replicates. Expression of the AOX-1D (B), LSU1 (C), and UPB1 (D) genes in WT (white bars) and COPT1OE (grey bars) seedlings grown under Cu deficiency (0 μM CuSO4), sufficiency (1 μM CuSO4) or Cu excess (10 μM CuSO4) was determined by RT-qPCR. Gene expression is represented as relative expression levels (r.u.) in relation to the WT under control conditions. UBQ10 was used as housekeeping gene. Bars correspond to arithmetic means (2−ΔΔCt) ± SD of biological replicates (n = 3). Samples with a letter in common are not significantly different (p-value <0.05).
UPB1 spatially regulates ROS distribution in the root transition zone where the balance between O2.− and H2O2 controls the transition between root cell proliferation and differentiation (Tsukagoshi et al., 2010). UPB1 expression was induced under Cu deficiency and sufficiency in COPT1OE seedlings compared to WT (Figure 6D). Accordingly, two of its direct targets, PER39 and PER40, were also up-regulated under Cu deficiency (Supplementary Figures S8A, B). Furthermore, UBP1 expression under different metal stress conditions in the WT indicated that was repressed under Cu excess as well as when Fe is low (Supplementary Figure S7D). These responses indicated clearly different responses of Fe regulators to low and high Cu levels.
Since these results would lead to a further decrease in H2O2, seedlings were grown in Cu-deficient media in the presence of H2O2 and the reductants ascorbic acid (AsH) and 1,4-ditiothreitol (DTT) (Supplementary Figure S9). COPT1OE seedlings showed shorter roots than controls under Cu deficiency. Whereas no clear effects were observed on root length for the H2O2 treatment, reductants such as AsH and DTT, improved the root growth of COPT1OE compared to WT (Supplementary Figure S9) further pointing to increased oxidative stress in the overexpressing line under Cu deficiency.
Additionally, since the Fe content was lower in COPTOE, a Fe excess treatment was also provided with no differential effect compared to controls (Supplementary Figure S9). In order to ascertain the Fe redox state in the roots, 7-day-old seedlings grown in the same media with different Cu content (0, 1, and 10 μM CuSO4) were stained with the Perl´s blue to detect the presence of Fe3+. The intensity of stained roots indicated that Fe3+ increased as Cu rise in the media from 0 to 10 μM Cu in all seedlings´ genotypes. Moreover, COPTOE seedlings were more intensely stained than WT in all the conditions (Figure 7) and this result is reproducible in 11-day-old seedlings (Supplementary Figure S10), indicating that Cu+ uptake through COPT is affecting the redox state of incorporated Fe leading to an increase in the Fe3+/Fe2+ ratio in the COPTOE roots since the lower total Fe concentration (Figure 1D).
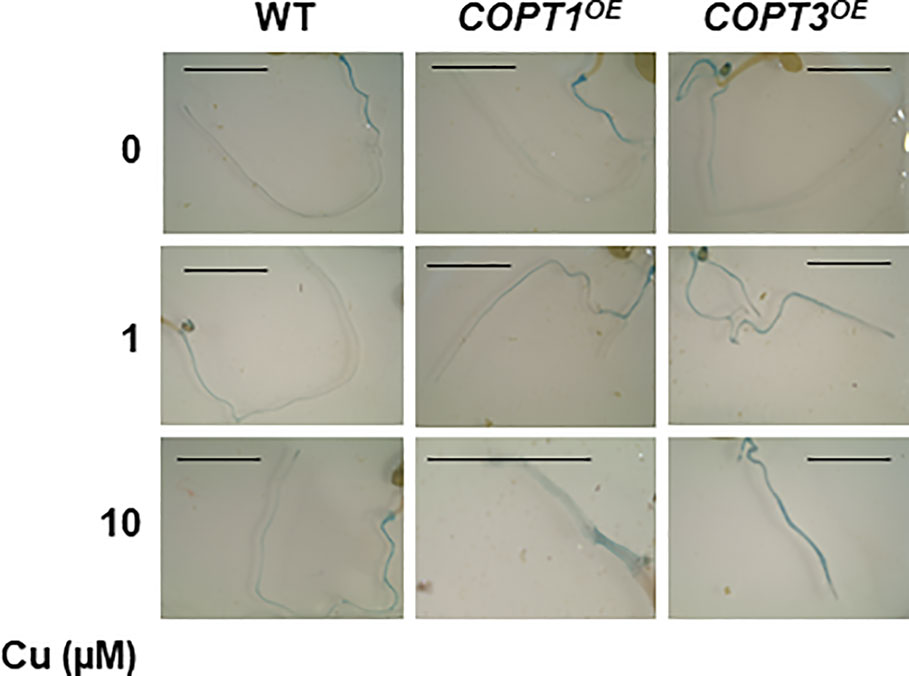
Figure 7 Detection of Fe3+ in COPTOE roots. Seven-day old roots of WT, COPT1OE and COPT3OE seedlings grown under Cu deficiency (0 μM CuSO4), sufficiency (1 μM CuSO4) or Cu excess (10 μM CuSO4) were stained with Perl´s blue to detect Fe3+. Scale bars represent 1 cm.
These results suggested that the reduction in Fe content observed in COPTOE could be due to the increased Fe3+/Fe2+ ratio when Cu+ entrance was enhanced and deregulated in COPTOE seedlings. Thus, a model for the Cu excess effects on Fe homeostasis in COPTOE seedlings has been proposed (Figure 8). The deregulated Cu+ entrance in COPTOE plants under high Cu activated the expression of the bHLH-Ib factors but not FIT. This effect redounded in Fe deficiency symptoms in COPTOE probably as a consequence of the lack of Fe assimilatory response. Cu excess increased the Fe3+/Fe2+ ratio in COPTOE roots, probably leading to signaling pathways that could further inhibit Fe uptake.
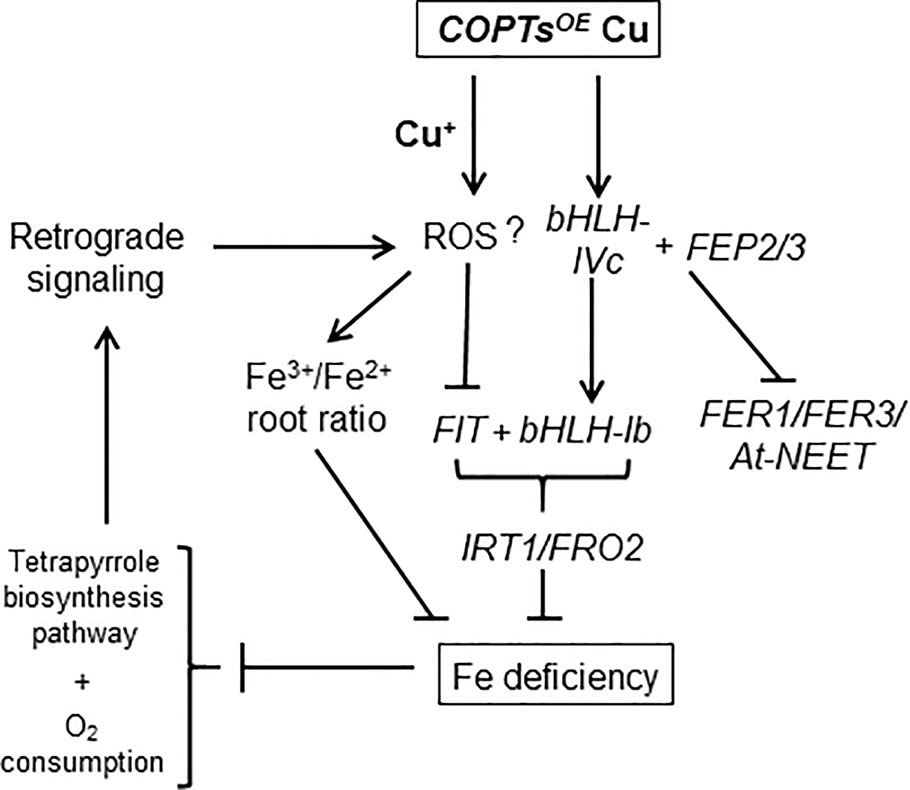
Figure 8 Genetic model of the Cu effects on Fe homeostasis in COPTOE. Deregulated Cu+ entrance by constitutive COPT overexpression under high Cu increased the Fe3+/Fe2+ ratio in roots. COPTOE displayed Fe deficiency symptoms probably as a consequence of the lack of Fe assimilatory response (IRT1/FRO2), since under high Cu conditions COPTOE plants activated the expression of the bHLH-Ib factors but not FIT. Cu excess increased ROS that could activate FEP2/FEP3 expression, which up-regulate bHLH-Ib factors. BTS affected bHLH-IVc stability and expression of its target genes (FER1, FER3 and At-NEET). Fe deficiency affected tetrapyrrole biosynthesis pathway and O2 consumption that could further increase ROS production and inhibit Fe uptake through retrograde signaling.
Discussion
In this report, we studied the effects of deregulated Cu+ entrance in COPTOE seedlings, which overexpress COPT Cu transporters (Andrés-Colás et al., 2010), on global gene expression when seedlings were grown in Cu concentrations within the physiological range that plants encounter in their natural environment. Our results indicate that Fe transport and homeostasis were altered in COPTOE. Despite containing low Fe, COPTOE was not able to orchestrate an optimal local Fe-uptake response at their roots under both Cu deficiency and excess (Figure 1). The causes for the Cu interference with the Fe-uptake response seem different in both conditions. Under Cu deficiency, COPTOE induced FIT, but the expression of subgroup Ib of bHLH remained low. On the contrary, under Cu excess, FIT was repressed, despite high FEPs and bHLH-Ib expression (Figure 3). Based on the necessity of expressing both FIT and subgroup Ib of bHLH transcription factors for Fe-uptake responses (Yuan et al., 2008; Wang et al., 2013), COPTOE seedlings were unable to properly respond to Fe deficiency under non-optimal Cu supply.
Fe and Cu homeostasis interact at different levels in plants (Puig et al., 2007; Bernal et al., 2012; Perea-García et al., 2013; Kastoori Ramamurthy et al., 2018). For instance, high Cu concentrations in the media lower leaf Fe content (Waters et al., 2012; Waters and Armbrust, 2013). In our experimental conditions, a slight decrease in IRT1 expression was observed in the WT seedlings under Cu excess, which was further exacerbated in COPTOE. On the other hand, higher Cu content was observed under Fe deficiency in WT (Kastoori Ramamurthy et al., 2018). Among the reasons for increased Cu content under Fe deficiency are the increased uptake of both the unspecific Cu2+ entrance through ZIP transporters and the specific Cu+ entrance through COPT2 (Colangelo and Guerinot, 2004; Perea-García et al., 2013). Nevertheless, the reason for Cu+ requirement under Fe deficiency remains unsolved.
Although recent data suggested that Fe deficiency responses are under the control of SPL7 (Kastoori Ramamurthy et al., 2018), Cu-dependent SPL7-mediated responses did not seem to be the cause of IRT1 down-regulation in COPTOE since the expression of other SPL7 target genes was not significantly affected in these plants. On the other hand, different hormones are involved in modulating Fe deficiency responses in plants (Kobayashi, 2019). JA has been shown to inhibit the expression of bHLH-Ib and promote FIT degradation, resulting in reduced expression of the Fe uptake genes IRT1 and FRO2 and increased sensitivity to Fe deficiency (Cui et al., 2018). Since JA is a negative regulator of Fe deficiency responses and JA content was reduced in COPT1OE, lower levels of JA could not justify the decrease in Fe uptake under our experimental conditions. However, auxins can enhance FIT and FRO2 expression (Chen et al., 2010), and, in this sense, we cannot discard that IAA decreased levels in COPT1OE seedlings under Cu excess could at least partially account for the low FIT expression in COPT1OE plants.
The oxidative stress produced by metal deficiencies and excess could also account for the Fe and Cu crosstalk (Ravet and Pilon, 2013). Indeed, Cu phytotoxicity has been attributed to increased H2O2 production (Cuypers et al., 2010) and the deficiency of Cu affects the functioning of the respiratory and photosynthetic electron transport chains, which increases the production of the O2.− (Abdel-Ghany et al., 2005; Yamasaki et al., 2009). Therefore, the majority type of ROS could be different under conditions of metal deficiency or excess. Increased oxidative stress leading to lipid peroxidation has been previously reported to occur in COPTOE seedlings (Andrés-Colás et al., 2010). According to the slightly increased Cu content in COPTOE seedlings (Andrés-Colás et al., 2010), they should have increased CSD2 levels under Cu excess, instead of the observed lower levels. This result suggests a difficulty of the COPTOE chloroplasts in detoxifying O2.− under Cu excess, since they cannot completely activate neither FSD1 nor CSD2 compared to the WT, maybe redounding in increased oxidative stress. The induction of several members of the LSU family (LSU1, LSU2 and LSU3) under Cu excess in the COPTOE plants is in agreement with their induction under sulfur deficiency and by Fe deficiency and Cu excess (Garcia-Molina et al., 2017). LSU1 interacts with chloroplastic FSD2 and stimulates its enzymatic activity in vivo and in vitro conforming a hub in coordinating plant responses to a wide spectrum of abiotic stress conditions (Garcia-Molina et al., 2017). Moreover, COPT1OE seedlings showed higher Cu-induced K+ efflux and net Ca2+ influx at the root tip level compared to the WT (Rodrigo-Moreno et al., 2013), although no significant changes of the membrane potential were detected upon Cu addition (Sanz et al., 2019).
Although increased O2 consumption has been also reported in sugar beet and cucumber roots (Lopez-Millan et al., 2000; Vigani et al., 2009), the ability to consume O2 decreased in COPT1OE roots under both Cu deficiency and excess (Figure 6A). High Cu in COPT1OE triggers a response that includes the expression of genes related to mitochondrial stress (Arnholdt-Schmitt et al., 2006; Selinski et al., 2018), such as AOX1A, AOX1D and AOX2. Results shown here support the respiratory shield hypothesis, where the mitochondrial Fe proteins are necessary to maintain high O2 consumption in the respiratory electron transport chain contributing to the microaerobic environment necessary to maintain a Fe2+ pool. The problem due to lack of respiratory shield could be aggravated in conditions of Fe deficiency and high and temporarily deregulated Cu+ entry. In this sense, the defect of Fe/S and heme proteins will lead to defective respiratory shield in strains with high metabolism (López-Torrejón et al., 2016; Wofford et al., 2019), aggravated in COPT1OE seedlings by deregulated Cu+ uptake. In any case, our observations support that optimal Cu supply is required for normal O2 consumption in COPT1OE plants.
Cu deficiency affects Fe homeostasis specifically causing a defect in root-to-shoot translocation, which has been attributed to a decrease in Cu-dependent ferroxidase activity (Bernal et al., 2012; Waters and Armbrust, 2013). Assuming that a certain Fe3+/Fe2+ and Cu2+/Cu+ intracellular ratios are necessary for uptake/mobilization processes, Cu+ uptake could affect the Fe3+/Fe2+ ratio at different levels. Firstly, due to its lower standard reduction potential, Cu+ could directly reduce Fe3+ to Fe2+. However, this is not the case since increased Fe3+ was observed in COPTOE seedlings (Figure 7). Moreover, highly reactive, cytosolic free Cu+ is almost absent in the cytosol (Rae et al., 1999). Instead, increased oxidative stress provoked by deregulated Cu+ entrance (Rodrigo-Moreno et al., 2013) could influence intracellular general redox status, leading to Fe oxidation. On the other hand, Cu deficiency would facilitate Fe2+ incorporation through the enhanced expression of FRO metalloreductases and inhibit Cu-dependent ferroxidase activity (Bernal et al., 2012; Waters et al., 2012; Waters and Armbrust, 2013). Since the Fe3+/Fe2+ ratio depends on the relative ferroxidase versus ferroreductase activities, an increased Fe3+/Fe2+ ratio would be expected in the presence of excess Cu. The other way around, the Fe3+/Fe2+ ratio should be decreased under Cu deficiency. Under our experimental conditions, we were not able to detect a significant change in ferroreductase activity and just a slight decrease is observed in COPTOE seedlings under Cu excess (Figure 1B), questioning this activity being the only cause of the Fe deficiency. Finally, Cu uptake increased Fe3+ in the roots, probably by means of the oxidative conditions created. As a consequence, decreased Fe mobilization in roots could lead to Fe deficiency in leaves. In this sense, Cu excess and deregulated Cu+ uptake in COPTOE seedlings increased the Fe3+/Fe2+ ratio leading to Fe deficiency effects, being it at least one of the problems faced by Cu+ toxicity (Figure 8). Accordingly, this suggestion could explain previous results where plants grown without Fe were more susceptible to Cu toxicity (Waters and Armbrust, 2013).
In order to address how Cu influenced the signaling pathways involved in the Fe deficiency response, the expression of BTS and several other genes encoding RING E3 ubiquitin ligases were induced in the COPT1OE seedlings. This result points to the putative Fe-sensing BTS (Selote et al., 2015), and maybe other RING E3 ubiquitin ligases, as candidates in upstream sensing of the lack of Fe mobilization in COPT1OE plants under mild Cu excess. On the other hand, our results indicated that tetrapyrrole signaling was affected under both Cu deficiency and excess, probably as a consequence of the common Fe deficiency conditions faced by COPTOE seedlings (Supplementary Tables SIII and SIV). Most of the genes involved in tetrapyrrole metabolism showed synchronized and light-dependent expression patterns (Matsumoto et al., 2004). Multiple PhANG were down-regulated in COPT1OE under both Cu deficiency and excess in addition to several regulators. Curiously, CHL27/CRD1 (COPPER RESPONSE DEFECT1) was first characterized in a screening for Cu conditional phenotypes in Chlamydomonas where Cu scarcity in plastocyanin is counteracted by a heme-containing cytochrome (Moseley et al., 2000), although this substitution has not been shown to occur in Arabidopsis. Moreover, the GUN4 porphyrin-binding protein enhances Mg-chelatase activity (Davison et al., 2005) and its repression under Cu excess could play a role in the control of substrate flow into the heme or chlorophyll branch (Tanaka and Tanaka, 2007). On the other hand, nuclear-encoded sigma factor, such as SIG1, could be involved in the integration of light and circadian signals that regulate chloroplast transcription (Belbin et al., 2017). These results underscore the importance of tightly regulated Cu homeostasis at the spatial–temporal level, in order to orchestrate an optimal Fe distribution and to avoid oxidative damage to highly sensitive Fe-dependent processes, such as Fe/S cluster assembly and tetrapyrrole biosynthesis. It is tempting to speculate that Fe and other metals, which affect at the cellular redox state and either participate or interfere with sensitive processes, have to be subjected to differential diurnal variation in the expression patterns of their homeostatic components (Peñarrubia et al., 2015; Zhang and Krämer, 2018). In this sense, the induced expression of UPB1 in COPTOE plants under Cu deficiency could emphasize the requirement of spatially appropriate Cu uptake at the root tip, where normally COPT1 is expressed (Sancenón et al., 2003), to avoid oxidative interference along the root with Fe translocation to the aerial part (Tsukagoshi et al., 2010).
Taken together, these results indicated that high Cu renders increased Fe3+ in the root. Despite low Fe in the shoot, the presence of high Fe3+/Fe2+ ratio in the root prevented local responses to Fe deficiency (Figure 8). The understanding of Cu influence on Fe mobilization and redistribution in the plant could help to ameliorate field treatments to maximize crop production under Fe deficiency through optimizing Cu homeostasis.
Data Availability Statement
The datasets generated for this study can be found in the: The microarray raw data were deposited in the NCBI’s Gene Expression Omnibus and are accessible through GEO Series accession number GSE143857.
Author Contributions
LP and SP conceived the idea and wrote the manuscript. MP-A and FV-S performed the COPT1OE global analysis. AP-G and AA-B performed the physiological and molecular experiments in mutant plants. All authors contributed to the article and approved the submitted version.
Funding
This work was supported by grant BIO2017-87828-C2-1-P from the Spanish Ministry of Economy and Competitiveness, and by FEDER funds from the European Union.
Conflict of Interest
The authors declare that the research was conducted in the absence of any commercial or financial relationships that could be construed as a potential conflict of interest.
Acknowledgments
We acknowledge the SCSIE (Universitat de València) for the ICPMS service, and the Plant Hormone Quantification Service of the (IBMCP) (CSIC-UPV, Valencia) for hormone level quantification. This work was supported by grant BIO2017-87828-C2-1-P from the Spanish Ministry of Economy and Competitiveness, and by FEDER funds from the European Union.
Supplementary Material
The Supplementary Material for this article can be found online at: https://www.frontiersin.org/articles/10.3389/fpls.2020.01106/full#supplementary-material
References
Abdel-Ghany, S. E., Burkhead, J. L., Gogolin, K. A., Andres-Colas, N., Bodecker, J. R., Puig, S., et al. (2005). AtCCS is a functional homolog of the yeast copper chaperone Ccs1/Lys7. FEBS Lett. 579, 2307–2312. doi: 10.1016/j.febslet.2005.03.025
Andrés-Bordería, A., Andrés, F., Garcia-Molina, A., Perea-García, A., Domingo, C., Puig, S., et al. (2017). Copper and ectopic expression of the Arabidopsis transport protein COPT1 alter iron homeostasis in rice (Oryza sativa L.). Plant Mol. Biol. 95, 17–32. doi: 10.1007/s11103-017-0622-8
Andrés-Colás, N., Sancenón, V., Rodríguez-Navarro, S., Mayo, S., Thiele, D. J., Ecker, J. R., et al. (2006). The Arabidopsis heavy metal P-type ATPase HMA5 interacts with metallochaperones and functions in copper detoxification of roots. Plant J. 45, 225–236. doi: 10.1111/j.1365-313X.2005.02601.x
Andrés-Colás, N., Perea-García, A., Puig, S., Peñarrubia, L. (2010). Deregulated copper transport affects Arabidopsis development especially in the absence of environmental cycles. Plant Physiol. 153, 170–184. doi: 10.1104/pp.110.153676
Andrés-Colás, N., Carrió-Seguí, A., Abdel-Ghany, S. E., Pilon, M., Peñarrubia, L. (2018). Expression of the intracellular COPT3-mediated Cu transport is temporally regulated by the TCP16 transcription factor. Front. Plant Sci. 9:910. doi: 10.3389/fpls.2018.00910
Arnholdt-Schmitt, B., Costa, J. H., de Melo, D. F. (2006). AOX - a functional marker for efficient cell reprogramming under stress? Trends Plant Sci. 11, 281–287. doi: 10.1016/j.tplants.2006.05.001
Ashburner, M., Ball, C. A., Blake, J. A., Botstein, D., Butler, H., Cherry, J. M., et al. (2000). Gene Ontology: tool for the unification of biology. Nat. Genet. 25, 25–29. doi: 10.1038/75556
Bang, W. Y., Jeong, I. S., Kim, D. W., Im, C. H., Ji, C., Hwang, S. M., et al. (2008). Role of Arabidopsis CHL27 protein for photosynthesis, chloroplast development and gene expression profiling. Plant Cell Physiol. 49, 1350–1363. doi: 10.1093/pcp/pcn111
Belbin, F. E., Noordally, Z. B., Wetherill, S. J., Atkins, K. A., Franklin, K. A., Dodd, A. N. (2017). Integration of light and circadian signals that regulate chloroplast transcription by a nuclear-encoded sigma factor. New Phytol. 213, 727–738. doi: 10.1111/nph.14176
Bernal, M., Casero, D., Singh, V., Wilson, G. T., Grande, A., Yang, H., et al. (2012). Transcriptome sequencing identifies SPL7-regulated copper acquisition genes FRO4/FRO5 and the copper dependence of iron homeostasis in Arabidopsis. Plant Cell 24, 738–761. doi: 10.1105/tpc.111.090431
Bueso, E., Alejandro, S., Carbonell, P., Perez-Amador, M. A., Fayos, J., Bellés, J. M., et al. (2007). The lithium tolerance of the Arabidopsis cat2 mutant reveals a cross-talk between oxidative stress and ethylene. Plant J. 52, 1052–1065. doi: 10.1111/j.1365-313X.2007.03305.x
Carmona-Saez, P., Chagoyen, M., Tirado, F., Carazo, J. M., Pascual-Montano, A. (2007). GENECODIS: a web-based tool for finding significant concurrent annotations in gene lists. Genome Biol. 8, R3. doi: 10.1186/gb-2007-8-1-r3
Carrió-Seguí, A., Garcia-Molina, A., Sanz, A., Peñarrubia, L. (2015). Defective copper transport in the copt5 mutant affects cadmium tolerance. Plant Cell Physiol. 56, 442–454. doi: 10.1093/pcp/pcu180
Chen, W. W., Yang, J. L., Qin, C., Jin, C. W., Mo, J. H., Ye, T., et al. (2010). Nitric oxide acts downstream of auxin to trigger root ferric-chelate reductase activity in response to iron deficiency in arabidopsis. Plant Physiol. 154, 810–819. doi: 10.1104/pp.110.161109
Clifton, R., Harvey Millar, A., Whelan, J. (2006). Alternative oxidases in Arabidopsis: A comparative analysis of differential expression in the gene family provides new insights into function of non-phosphorylating bypasses. Biochim. Biophys. Acta 1757, 730–741. doi: 10.1016/j.bbabio.2006.03.009
Colangelo, E. P., Guerinot, M. L. (2004). The essential basic helix-loop-helix protein FIT1 is required for the iron deficiency response. Plant Cell 16, 3400–3412. doi: 10.1105/tpc.104.024315
Connolly, E. L., Fett, J. P., Guerinot, M. L. (2002). Expression of the IRT1 metal transporter is controlled by metals at the levels of transcript and protein accumulation. Plant Cell 14, 1347–1357. doi: 10.1105/tpc.001263
Crichton, R. R., Pierre, J. L. (2001). Old iron, young copper: from Mars to Venus. Biometals 14, 99–112. doi: 10.1023/a:1016710810701
Cui, Y., Chen, C. L., Cui, M., Zhou, W. J., Wu, H. L., Ling, H. (2018). Four IVa bHLH transcription factors are novel interactors of FIT and mediate JA inhibition of iron uptake in Arabidopsis. Mol. Plant 11, 1166–1183. doi: 10.1016/j.molp.2018.06.005
Cuypers, A., Karen, S., Jos, R., Kelly, O., Els, K., Tony, R., et al. (2010). The cellular redox state as a modulator in cadmium and copper responses in Arabidopsis thaliana seedlings. J. Plant Physiol. 168, 309–316. doi: 10.1016/j.jplph.2010.07.010
da Silva, E. M., Silva, G. F. F. E., Bidoia, D. B., da Silva Azevedo, ,. M., de Jesus, F. A., Pino, L. E., et al. (2017). microRNA159-targeted SlGAMYB transcription factors are required for fruit set in tomato. Plant J. 92, 95–109. doi: 10.1111/tpj.13637
Davison, P. A., Schubert, H. L., Reid, J. D., Iorg, C. D., Heroux, A., Hill, C. P., et al. (2005). Structural and biochemical characterization of Gun4 suggests a mechanism for its role in chlorophyll biosynthesis. Biochemistry 44, 7603–7612. doi: 10.1021/bi050240x
Di Rienzo, J. A., Casanoves, F., Balzarini, M. G., Gonzalez, L., Tablada, M., Robledo, C. W. (2011). InfoStat.
Edgar, R. (2002). Gene Expression Omnibus: NCBI gene expression and hybridization array data repository. Nucleic Acids Res. 30, 207–210. doi: 10.1093/nar/30.1.207
Garcia-Molina, A., Andrés-Colás, N., Perea-García, A., Neumann, U., Dodani, S. C., Huijser, P., et al. (2013). The Arabidopsis COPT6 Transport Protein Functions In Copper Distribution Under Copper-Deficient Conditions. Plant Cell Physiol. 0, 1–13. doi: 10.1093/pcp/pct088
Garcia-Molina, A., Altmann, M., Alkofer, A., Epple, P. M., Dangl, J. L., Falter-Braun, P. (2017). LSU network hubs integrate abiotic and biotic stress responses via interaction with the superoxide dismutase FSD2. J. Exp. Bot. 68, 1185–1197. doi: 10.1093/jxb/erw498
Grillet, L., Ouerdane, L., Flis, P., Hoang, M. T. T., Isaure, M. P., Lobinski, R., et al. (2014). Ascorbate efflux as a new strategy for iron reduction and transport in plants. J. Biol. Chem. 289, 2515–2525. doi: 10.1074/jbc.M113.514828
Grillet, L., Lan, P., Li, W., Mokkapati, G., Schmidt, W. (2018). IRON MAN is a ubiquitous family of peptides that control iron transport in plants. Nat. Plants. 4, 953–963. doi: 10.1038/s41477-018-0266-y
Gulec, S., Collins, J. F. (2014). Molecular mediators governing iron-copper interactions. Annu. Rev. Nutr. 34, 95–116. doi: 10.1146/annurev-nutr-071812-161215
Hindt, M. N., Akmakjian, G. Z., Pivarski, K. L., Punshon, T., Baxter, I., Salt, D. E., et al. (2017). BRUTUS and its paralogs, BTS LIKE1 and BTS LIKE2, encode important negative regulators of the iron deficiency response in Arabidopsis thaliana. Metallomics 9, 876–890. doi: 10.1039/c7mt00152e
Hirayama, T., Lei, G. J., Yamaji, N., Nakagawa, N., Ma, J. F. (2018). The putative peptide gene FEP1 regulates iron deficiency response in Arabidopsis. Plant Cell Physiol. 59, 1739–1752. doi: 10.1093/pcp/pcy145
Kastoori Ramamurthy, R., Xiang, Q., Hsieh, E. J., Liu, K., Zhang, C., Waters, B. M. (2018). New aspects of iron-copper crosstalk uncovered by transcriptomic characterization of Col-0 and the copper uptake mutant: Spl7 in Arabidopsis thaliana. Metallomics 10, 1824–1840. doi: 10.1039/c8mt00287h
Kobayashi, T., Nagasaka, S., Senoura, T., Itai, R. N., Nakanishi, H., Nishizawa, N. K. (2013). Iron-binding haemerythrin RING ubiquitin ligases regulate plant iron responses and accumulation. Nat. Commun. 4, 2792–2804. doi: 10.1038/ncomms3792
Kobayashi, T. (2019). Understanding the complexity of iron sensing and signaling cascades in plants. Plant Cell Physiol. 60, 1440–1446. doi: 10.1093/pcp/pcz038
Kosman, D. J. (2018). The teleos of metallo-reduction and metallo-oxidation in eukaryotic iron and copper trafficking. Metallomics 10, 370–377. doi: 10.1039/c8mt00015h
Long, T. A., Tsukagoshi, H., Busch, W., Lahner, B., Salt, D. E., Benfey, P. N. (2010). The bHLH transcription factor POPEYE regulates response to iron deficiency in arabidopsis roots. Plant Cell 22, 2219–2236. doi: 10.1105/tpc.110.074096
Lopez-Millan, A. F., Morales, F., Andaluz, S., Gogorcena, Y., Abadia, A., De Las Rivas, J., et al. (2000). Responses of sugar beet roots to iron deficiency. Changes in carbon assimilation and oxygen use? Plant Physiol. 124, 885–897. doi: 10.1104/pp.124.2.885
López-Torrejón, G., Jiménez-Vicente, E., Buesa, J. M., Hernandez, J. A., Verma, H. K., Rubio, L. M. (2016). Expression of a functional oxygen-labile nitrogenase component in the mitochondrial matrix of aerobically grown yeast. Nat. Commun. 7, 1–6. doi: 10.1038/ncomms11426
Macadlo, L. A., Ibrahim, I. M., Puthiyaveetil, S. (2019). Sigma factor 1 in chloroplast gene transcription and photosynthetic light acclimation. J. Exp. Bot. 71, 1029–1038. doi: 10.1093/jxb/erz464
Marschner, P. (2012). Marschner's Mineral Nutrition of Higher Plants Third Edition (Amsterdam: Elsevier).
Matsumoto, F., Obayashi, T., Sasaki-Sekimoto, Y., Ohta, H., Takamiya, K., Tatsuru, , M. (2004). Gene expression profiling of the tetrapyrrole metabolic pathway in Arabidopsis with a mini-array system. Plant Physiol. 135, 2379–2391. doi: 10.1104/pp.104.042408
Mittler, R., Darash-Yahana, M., Sohn, Y. S., Bai, F., Song, L., Cabantchik, I. Z., et al. (2019). NEET Proteins: A new link between iron metabolism, reactive oxygen species, and cancer. Antioxid. Redox Signal. 30, 1083–1095. doi: 10.1089/ars.2018.7502
Moseley, J., Quinn, J., Eriksson, M., Merchant, S. (2000). The Crd1 gene encodes a putative di-iron enzyme required for photosystem I accumulation in copper deficiency and hypoxia in Chlamydomonas reinhardtii. EMBO J. 19, 2139–2151. doi: 10.1093/emboj/19.10.2139
Murashige, T., Skoog, F. (1962). A Revised Medium for Rapid Growth and Bio Assays with Tobacco Tissue Cultures. Physiol. Plant 15, 473–497. doi: 10.1111/j.1399-3054.1962.tb08052.x
Nechushtai, R., Conlan, A. R., Harir, Y., Song, L., Yogev, O., Eisenberg-Domovich, Y., et al. (2012). Characterization of Arabidopsis NEET reveals an ancient role for NEET proteins in iron metabolism. Plant Cell 24, 2139–2154. doi: 10.1105/tpc.112.097634
Nevitt, T., Öhrvik, H., Thiele, D. J. (2012). Charting the travels of copper in eukaryotes from yeast to mammals. Biochim. Biophys. Acta 1823, 1580–1593. doi: 10.1016/j.bbamcr.2012.02.011
Nogales-Cadenas, R., Carmona-Saez, P., Vazquez, M., Vicente, C., Yang, X., Tirado, F., et al. (2009). GeneCodis: Interpreting gene lists through enrichment analysis and integration of diverse biological information. Nucleic Acids Res. 37, 317–322. doi: 10.1093/nar/gkp416
Nouet, C., Motte, P., Hanikenne, M. (2011). Chloroplastic and mitochondrial metal homeostasis. Trends Plant Sci. 16, 395–404. doi: 10.1016/j.tplants.2011.03.005
Parsons, T., Strickland, J. (1965). Discussion of spectrophotometric determination of marine-plant pigments, with revised equations for ascertaining chlorophylls and carotenoids. Deep Sea Res. Oceanogr. Abstr. 12, 619. doi: 10.1016/0011-7471(65)90662-5
Peñarrubia, L., Romero, P., Carrió-seguí, A., Andrés-bordería, A., Moreno, J., Sanz, A. (2015). Plant Traffic and Transport Temporal aspects of copper homeostasis and its crosstalk with hormones. Front. Plant Sci. 6, 255–273. doi: 10.3389/fpls.2015.00255
Perea-García, A., Garcia-Molina, A., Andrés-Colás, N., Vera-Sirera, F., Pérez-Amador, M. A., Puig, S., et al. (2013). Arabidopsis copper transport protein COPT2 participates in the cross talk between iron deficiency responses and low-phosphate signaling. Plant Physiol. 162, 180–194. doi: 10.1104/pp.112.212407
Perea-García, A., Andrés-Bordería, A., Mayo de Andrés, S., Sanz, A., Davis, A. M., Davis, S. J., et al. (2016). Modulation of copper deficiency responses by diurnal and circadian rhythms in Arabidopsis thaliana. J. Exp. Bot. 67, 391–403. doi: 10.1093/jxb/erv474
Petit, J. M., Briat, J. F., Lobréaux, S. (2001). Structure and differential expression of the four members of the Arabidopsis thaliana ferritin gene family. Biochem. J. 359, 575–582. doi: 10.1042/0264-6021:3590575
Pfaffl, M. W. (2002). Relative expression software tool (REST(C)) for group-wise comparison and statistical analysis of relative expression results in real-time PCR. Nucleic Acids Res. 30:e36. doi: 10.1093/nar/30.9.e36
Puig, S., Andrés-Colás, N., García-Molina, A., Peñarrubia, L. (2007). Copper and iron homeostasis in Arabidopsis: Responses to metal deficiencies, interactions and biotechnological applications. Plant Cell Environ. 30, 271–290. doi: 10.1111/j.1365-3040.2007.01642.x
Puig, S. (2014). Function and regulation of the plant COPT family of high-affinity copper transport proteins. Adv. Bot. 2014, 1–9. doi: 10.1155/2014/476917
Rae, T. D., Schmidt, P. J., Pufahl, R. A., Culotta, V. C., O’Halloran, T. V. (1999). Undetectable intracellular free copper: The requirement of a copper chaperone for superoxide dismutase. Sci. (80-. ). 284, 805–808. doi: 10.1126/science.284.5415.805
Ravet, K., Pilon, M. (2013). Copper and iron homeostasis in plants: The challenges of oxidative stress. Antioxid. Redox Signal. 19, 919–932. doi: 10.1089/ars.2012.5084
Ren, F., Logeman, B. L., Zhang, X., Liu, Y., Thiele, D. J., Yuan, P. (2019). X-ray structures of the high-affi nity copper transporter Ctr1. Nat. Commun. 10, 1386–1395. doi: 10.1038/s41467-019-09376-7
Reyt, G., Boudouf, S., Boucherez, J., Gaymard, F., Briat, J. F. (2015). Iron- and ferritin-dependent reactive oxygen species distribution: Impact on arabidopsis root system architecture. Mol. Plant 8, 439–453. doi: 10.1016/j.molp.2014.11.014
Robinson, N. J., Procter, C. M., Connolly, E. L., Guerinot, M. (1999). A ferric-chelate reductase for iron uptake from soils. Nature 397, 694–697. doi: 10.1038/17800
Rodrigo-Moreno, A., Andrés-Colás, N., Poschenrieder, C., Gunsé, B., Peñarrubia, L., Shabala, S. (2013). Calcium- and potassium-permeable plasma membrane transporters are activated by copper in Arabidopsis root tips: Linking copper transport with cytosolic hydroxyl radical production. Plant Cell Environ. 36, 844–855. doi: 10.1111/pce.12020
Sancenón, V., Puig, S., Mira, H., Thiele, D. J., Peñarrubia, L. (2003). Identification of a copper transporter family in Arabidopsis thaliana. Plant Mol. Biol. 51, 577–587. doi: 10.1023/A:1022345507112
Sancenón, V., Puig, S., Mateu-Andrés, I., Dorcey, E., Thiele, D. J., Peñarrubia, L. (2004). The Arabidopsis Copper Transporter COPT1 Functions in Root Elongation and Pollen Development. J. Biol. Chem. 279, 15348–15355. doi: 10.1074/jbc.M313321200
Sanz, A., Pike, S., Khan, M. A., Carrió-Seguí, À., Mendoza-Cózatl, D. G., Peñarrubia, L., et al. (2019). Copper uptake mechanism of Arabidopsis thaliana high-affinity COPT transporters. Protoplasma 256, 161–170. doi: 10.1007/s00709-018-1286-1
Selinski, J., Scheibe, R., Day, D. A., Whelan, J. (2018). Alternative Oxidase Is Positive for Plant Performance. Trends Plant Sci. 23, 588–597. doi: 10.1016/j.tplants.2018.03.012
Selote, D., Samira, R., Matthiadis, A., Gillikin, J. W., Long, T. A. (2015). Iron-binding E3 ligase mediates iron response in plants by targeting basic helix-loop-helix transcription factors. Plant Physiol. 167, 273–286. doi: 10.1104/pp.114.250837
Stacey, M. G., Patel, A., McClain, W. E., Mathieu, M., Remley, M., Rogers, E. E., et al. (2008). The arabidopsis AtOPT3 protein functions in metal homeostasis and movement of iron to developing seeds. Plant Physiol. 146, 589–601. doi: 10.1104/pp.107.108183
Tanaka, R., Tanaka, A. (2007). Tetrapyrrole biosynthesis in higher plants. Annu. Rev. Plant Biol. 58, 321–346. doi: 10.1146/annurev.arplant.57.032905.105448
Tissot, N., Robe, K., Gao, F., Grant-Grant, S., Boucherez, J., Bellegarde, F., et al. (2019). Transcriptional integration of the responses to iron availability in Arabidopsis by the bHLH factor ILR3. New Phytol. 223, 1433–1446. doi: 10.1111/nph.15753
Tsukagoshi, H., Busch, W., Benfey, P. N. (2010). Transcriptional regulation of ROS controls transition from proliferation to differentiation in the root. Cell 143, 606–616. doi: 10.1016/j.cell.2010.10.020
Tusher, V., Tibshirani, R., Chu, G. (2001). Significance analysis of microarrays applied to the ionizing radiation response. Proc. Natl. Acad. Sci. 98, 5116–5121. doi: 10.1073/pnas.091062498
Varotto, C., Maiwald, D., Pesaresi, P., Jahns, P., Salamini, F., Leister, D. (2002). The metal ion transporter IRT1 is necessary for iron homeostasis and efficient photosynthesis in Arabidopsis thaliana. Plant J. 31, 589–599. doi: 10.1046/j.1365-313x.2002.01381.x
Vert, G., Grotz, N., Dédaldéchamp, F., Gaymard, F., Guerinot, L., Briat, J., et al. (2002). IRT1, an Arabidopsis transporter essential for iron uptake from the soil and for plant growth. Plant Cell 14, 1223–1233. doi: 10.1105/tpc.001388
Vigani, G., Maffi, D., Zocchi, G. (2009). Iron availability affects the function of mitochondria in cucumber roots. New Phytol. 182, 127–136. doi: 10.1111/j.1469-8137.2008.02747.x
Wang, N., Cui, Y., Liu, Y., Fan, H., Du, J., Huang, Z., et al. (2013). Requirement and functional redundancy of Ib subgroup bHLH proteins for iron deficiency responses and uptake in arabidopsis thaliana. Mol. Plant 6, 503–513. doi: 10.1093/mp/sss089
Waters, B. M., Armbrust, L. C. (2013). Optimal copper supply is required for normal plant iron deficiency responses. Plant Signal. Behav. 8, 1–5. doi: 10.4161/psb.26611
Waters, B. M., McInturf, S. A., Stein, R. J. (2012). Rosette iron deficiency transcript and microRNA profiling reveals links between copper and iron homeostasis in Arabidopsis thaliana. J. Exp. Bot. 63, 5903–5918. doi: 10.1093/jxb/ers239
Wofford, J. D., Bolaji, N., Dziuba, N., Wayne Outten, F., Lindahl, P. A. (2019). Evidence that a respiratory shield in Escherichia coli protects a low-molecular-mass FeII pool from O2-dependent oxidation. J. Biol. Chem. 294, 50–62. doi: 10.1074/jbc.RA118.005233
Yamasaki, H., Abdel-Ghany, S. E., Cohu, C. M., Kobayashi, Y., Shikanai, T., Pilon, M. (2007). Regulation of copper homeostasis by micro-RNA in Arabidopsis. J. Biol. Chem. 282, 16369–16378. doi: 10.1074/jbc.M700138200
Yamasaki, H., Hayashi, M., Fukazawa, M., Kobayashi, Y., Shikanai, T. (2009). SQUAMOSA promoter binding protein-like7 is a central regulator for copper homeostasis in Arabidopsis. Plant Cell 21, 347–361. doi: 10.1105/tpc.108.060137
Yruela, I. (2013). Transition metals in plant photosynthesis. Metallomics 5, 1090–1109. doi: 10.1039/c3mt00086a
Yuan, Y., Wu, H., Wang, N., Li, J., Zhao, W., Du, J., et al. (2008). FIT interacts with AtbHLH38 and AtbHLH39 in regulating iron uptake gene expression for iron homeostasis in Arabidopsis. Cell Res. 18, 385–397. doi: 10.1038/cr.2008.26
Keywords: Arabidopsis thaliana, copper uptake, high affinity copper importer 1, iron homeostasis, metal interactions, metal mobilization
Citation: Perea-García A, Andrés-Bordería A, Vera-Sirera F, Pérez-Amador MA, Puig S and Peñarrubia L (2020) Deregulated High Affinity Copper Transport Alters Iron Homeostasis in Arabidopsis. Front. Plant Sci. 11:1106. doi: 10.3389/fpls.2020.01106
Received: 24 January 2020; Accepted: 06 July 2020;
Published: 23 July 2020.
Edited by:
Louis Grillet, Academia Sinica, TaiwanReviewed by:
Takashi Hirayama, Okayama University, JapanJeeyon Jeong, Amherst College, United States
Ya-Fen Lin, National Taiwan University, Taiwan
Copyright © 2020 Perea-García, Andrés-Bordería, Vera-Sirera, Pérez-Amador, Puig and Peñarrubia. This is an open-access article distributed under the terms of the Creative Commons Attribution License (CC BY). The use, distribution or reproduction in other forums is permitted, provided the original author(s) and the copyright owner(s) are credited and that the original publication in this journal is cited, in accordance with accepted academic practice. No use, distribution or reproduction is permitted which does not comply with these terms.
*Correspondence: Lola Peñarrubia, cGVuYXJydWJAdXYuZXM=
†Present address: Amparo Andrés-Bordería, Departamento de Fisiología, Facultad de Medicina, Universitat de València, Valencia, Spain