- College of Resources and Environmental Sciences, Hunan Agricultural University, Changsha, China
As an important nitrogen source, nitrate (NO3−) absorbed by plants is carried throughout the plant via short-distance distribution (cytoplasm to vacuole) and long-distance transportation (root to shoot), the two pathways that jointly regulate the content of NO3− in plants. NO3− accumulation within the vacuole depends on the activities of both tonoplast proton pumps and chloride channel (CLC) proteins, and less NO3− is stored in vacuoles when the activities of these proteins are reduced. The ratio of the distribution of NO3− in the cytoplasm and vacuole affects the long-distance transport of NO3−, which is regulated by the proteins NPF7.3 and NPF7.2 that play opposite but complementary roles. NPF7.3 is responsible for loading NO3− from the root cytoplasm into the xylem, whereas NPF7.2 regulates the unloading of NO3− from the xylem, thereby facilitating the long-distance transport of NO3− through the roots to the shoots. Vegetables, valued for their nutrient content, are consumed in large quantities; however, a high content of NO3− can detrimentally affect the quality of these plants. NO3− that is not assimilated and utilized in plant tissues is converted via enzyme-catalyzed reactions to nitrite (NO2−), which is toxic to plants and harmful to human health. In this review, we describe the mechanisms underlying NO3− distribution and transport in plants, a knowledge of which will contribute to breeding leafy vegetables with lower NO3− contents and thus be of considerable significance from the perspectives of environmental protection and food safety.
Introduction
Nitrogen (N) is an essential element that affects the growth, yield, and quality of crops (Peng et al., 2010; Ju et al., 2015). It is required for the synthesis of macromolecular compounds such as proteins and nucleic acids, which play important roles in the metabolism and energy production of organisms (Li and Gong, 2011). Ammonium nitrogen (NH4+-N) and nitrate nitrogen (NO3−-N) are the main forms of nitrogen absorbed and utilized by crops, with the latter being the main nitrogen source for dryland crops (Tang et al., 2013; Zhao et al., 2018). Exogenous concentrations of NO3− are among the most important factors affecting the accumulation of NO3− in plants. Plants actively take up NO3− from the environment through a proton/nitrate-coupled mechanism (Wang et al., 2018). Several NPF/peptide transporter (PTR) family and NO3− transporter 2 (NRT2) members in Arabidopsis are involved in NO3− uptake, including components of the high-affinity transport system [HATS: NRT2.1, NRT2.2 (Filleur et al., 2001), NRT2.4 (Kiba et al., 2012), and NRT2.5 (Lezhneva et al., 2014)] and low-affinity transport system [LATS: NPF4.6 (Huang et al., 1999)]. Furthermore, NPF6.3 is known to function as a dual-affinity NO3− transporter (Liu et al., 1999), the affinity for NO3− of which is determined by external NO3− concentrations (Ho et al., 2009). Previous studies have shown that differences in NO3− accumulation in different lettuce varieties are largely attributable to differences in NO3− uptake (Burns et al., 2011). In a study examining two varieties of Chinese cabbage with high and low NO3− accumulation, respectively, a stronger NO3− absorption capacity and higher expression of NPF and NRT2 genes were detected in the high NO3−-accumulating variety (Zhao et al., 2011). The NO3− absorbed by plants can be directly assimilated into amino acids by the activities of NO3− reductase (NR), nitrite reductase (NiR), glutamine synthetase (GS), and glutamate synthase (GOGAT) in the cell cytosol (Williams et al., 1987). An imbalance in the efficiency of NO3− absorption and reduction in vegetables has been demonstrated to be an important factor in determining NO3− accumulation, and the activity of NR has been shown to be significantly negatively correlated with the NO3− content in Chinese cabbage leaves (Du et al., 2008). Moreover, a proportion of NO3− is secreted into the rhizosphere, mediated by the NO3− transporter NPF2.7 (Wang et al., 2018).
The NO3− that remains unutilized by plants is distributed throughout the plant via short-distance distribution and long-distance transportation (Han et al., 2015; Zhang, 2017). Short-distance distribution of NO3− occurs at the subcellular level and involves the distribution of NO3− between the cytoplasm and vacuoles, a process regulated by members of the chloride channel (CLC) protein family. Initially, it was believed that CLC proteins were involved only in the transport of Cl−, functioning as chloride channels and Cl−/H+ anti-transporters. However, in a study examining the Arabidopsis clca mutant, De Angeli et al. (2006) demonstrated that CLC family proteins could also transport NO3− across the tonoplast by acting as 2NO3−/H+ antiporters. Compared with wild-type plants, the clca mutant was found to accumulate 50% less NO3− in the vacuole, thereby indicating that AtCLCa plays a key role in regulating the vacuolar storage and short-distance distribution of NO3− in plants (De Angeli et al., 2006).
The long-distance transport of NO3− mainly relies on the coordination of the NPF proteins with low affinity. AtNPF7.3 is a pH-dependent NO3− transporter protein located in the cytomembrane and expressed in the pericycle cells near the xylem. It has been found that in mutants lacking AtNPF7.3, the content of NO3− in the xylem sap and shoots is significantly reduced, thereby indicating that AtNPF7.3 plays an important role in promoting the long-distance transport of NO3− from roots to aerial parts (Lin et al., 2008). In contrast, AtNPF7.2 is mainly expressed in parenchyma cells near the root xylem, where it functions in the unloading of NO3− from the xylem to regulate the distribution ratio of NO3− between the roots and shoots (Li et al., 2010). The concentration of NO3− in plants is thus affected by multiple processes, among which there is a close interdependency that facilitates the coordinated regulation of NO3− accumulation in plants.
As an important source of inorganic nitrogen, the NO3− content of food products may originate from soil, water resources, chemical fertilizers, or food additives, consequently affecting the human food chain (Quijano et al., 2017). To date, numerous studies have been conducted on the NO3− content of plants and have revealed high concentrations of NO3− in different vegetables (Bahadoran et al., 2016), particularly leafy vegetables, which are the most important source of NO3− in the human diet, accounting for more than 80% of the NO3− intake (Anjana and Iqbal, 2007; Bondonno et al., 2018; Chetty et al., 2018). However, during the storage and processing of plants, excess NO3− that remains unassimilated in plant tissues can be enzymatically converted to NO2−. The NO3− ingested by humans can also be reduced to NO2− via the activity of gut microorganisms. NO2− is a strong carcinogen and causes the accumulation of methemoglobin, a compound with potentially toxic effects on human health (Salehzadeh et al., 2020). The content of NO3− in vegetables depends on its distribution and utilization and has become established as one of the indices used to assess the quality of vegetables and their processed products. Consequently, measures that can be used to reduce the concentration of NO3− in leafy vegetables, by regulating its distribution and utilization, are of importance with respect to reducing environmental pollution and ensuring a healthy diet.
Short-Distance (Vacuole to Cytoplasm) NO3− Distribution in Vegetables
The evolutionary success of higher plants is largely attributable to their unique cellular architecture (Kriegel et al., 2015). Vacuoles, as the largest organelles in mature plant cells, account for approximately 90% of the cell volume and are generally utilized for the storage of nutrients (Liao et al., 2002; Shen et al., 2003; Krebs et al., 2010; Liao et al., 2018). Having been absorbed by plants, NO3−, an important plant nutrient, is allocated to the metabolic pool (cytoplasm) or the storage pool (vacuole) (Miller et al., 2007). The concentrations of NO3− in the vacuole and cytoplasm are typically within the ranges of 30 to 50 mol m−3 and 3 to 5 mol m−3, respectively (Martinoia et al., 2000), and the fraction stored in the vacuole cannot be metabolized by plants unless it is initially allocated to the surrounding cytoplasm. The vacuole is accordingly the main storage site of NO3−, and thus the role of this vacuolar fraction in NO3− distribution should not be overlooked in studies on plant NO3− contents (Martinoia et al., 1981; Huang et al., 2012; Han et al., 2015).
In general, the assimilatory power of leaf cell cytoplasm is sufficient with respect to reducing NO3− concentrations. As long as NO3− remains distributed outside the vacuole, it can be rapidly assimilated, and therefore the content of NO3− in plants is largely determined by the ratio of the distribution of NO3− within and outside the vacuole (Shen et al., 2003; Huang et al., 2012; Han et al., 2016). Changes in the relative size of these two NO3− reservoirs are mediated by the 2NO3−/H+ exchange channel CLCa in the tonoplast (Krebs et al., 2010; Liao et al., 2019) and is dependent on the activity of the vacuolar H+-ATPase (V-ATPase) and the vacuolar H+-pyrophosphatase (V-PPase) proton pumps. The combined activity of these vacuolar proton pumps, utilizing MgATP and MgPPi as substrates, respectively, establishes an electrochemical H+ gradient across the tonoplast that drives the transport of NO3− against its electrochemical potential (Martinoia et al., 2007; Lin et al., 2012) (Figure 1A).
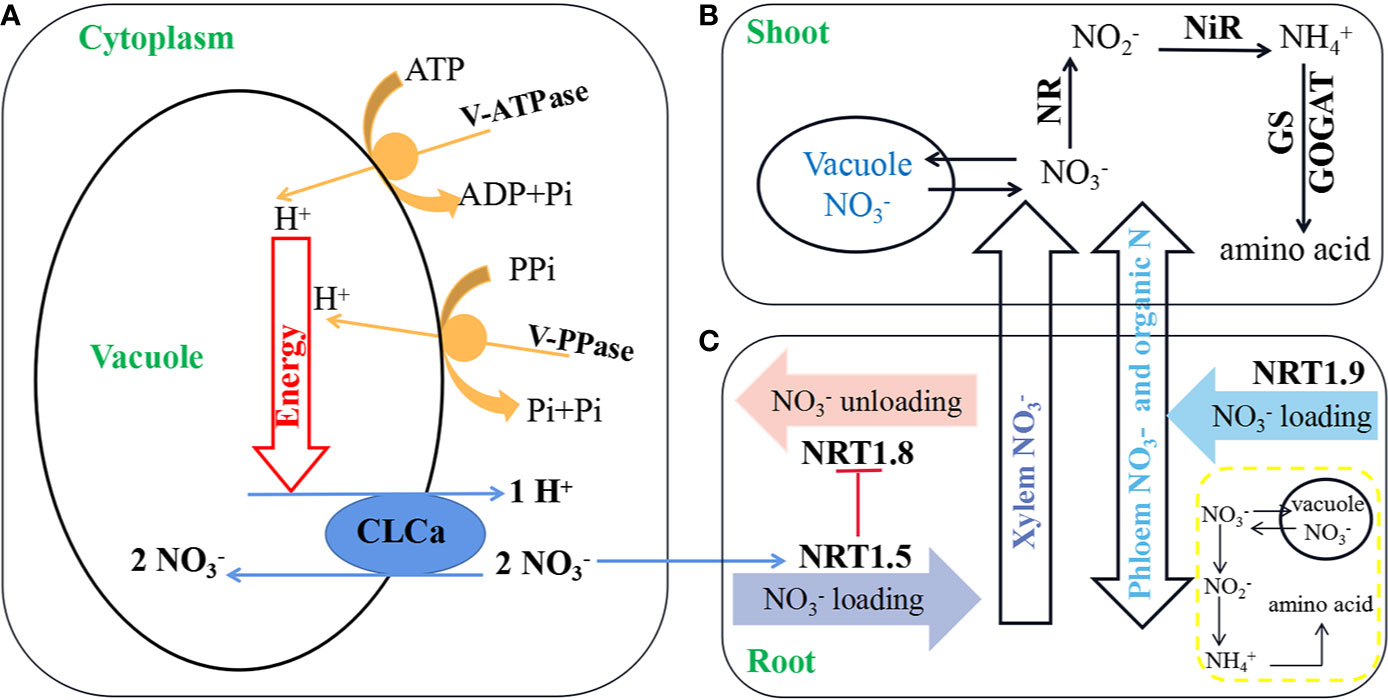
Figure 1 A model of short-distance distribution and long-distance transport of NO3− in plants. Modified from Zhang (2017). (A) Accumulation of NO3− in the vacuole is regulated by chloride channels (CLCa) and relies on an H+ gradient established by V-ATPase and V-PPase. (B) Short-distance distribution of NO3− and reactions catalyzed by NO3− reductase (NR), nitrite reductase (NiR), glutamine synthetase (GS), and glutamate synthase (GOGAT) in shoots. (C) Long-distance transport of NO3− from roots to shoots is mainly regulated by NPF7.3, NPF7.2, and NPF2.9 genes.
These two proton pumps are among the most abundant tonoplast proteins, thereby indicating the considerable amounts of energy expended in vacuolar transport (Carter et al., 2004; Jaquinod et al., 2007). V-ATPases are highly conserved, multi-subunit proton pumps that consist of two sub-complexes, the peripheral V1 and membrane-integral V0 complexes. The peripheral V1 complex comprises eight subunits (VHA-A to -H), which are exposed on the cytoplasmic side of the vacuolar membrane and are responsible for ATP hydrolysis. The membrane-integral V0 complex, which consists of six subunits (VHA-a, -c, -cʹ, cʹʹ, -d, and -e), is integrated within the membrane and functions as a channel for the translocation of protons from the cytoplasm into the lumen of endomembrane compartments and also serves as the binding site for the polymerization and assembly of V1 subunits (Cipriano et al., 2008; Luo, 2012). Compared with tonoplast H+-ATPase, the H+-PPase proton pump, which is widely distributed in plants, a few algae, protozoa, and bacteria, has a simple structure comprising an oligopeptide chain and accounts for approximately 1 to 10% of membrane proteins (Zhao and Liu, 1999; Maeshima, 2000).
V-ATPase and V-PPase not only serve as key tonoplast proton pump enzymes but also function as determinants of NO3− re-utilization (Shen et al., 2003). From the perspective of reducing NO3− accumulation in plant vacuoles and further improving the nitrogen-utilization efficiency of crops, it is of considerable significance to elucidate the short-distance distribution mechanism of NO3− associated with tonoplast proton pump activity (Han et al., 2014). In a study examining two varieties of rapeseed seedlings with high and low nitrogen-use efficiency, respectively, Han et al. (2015) revealed that V-ATPase and V-PPase activities and NO3− internal flow rate into the vacuole in the highly efficient variety were lower compared with those in the variety with low nitrogen-use efficiency. This indicates that in the former, larger amounts of NO3− are assigned to the cytoplasm for subsequent metabolism, thereby reducing the vacuolar content of NO3− and consequently enhancing the nitrogen-use efficiency of rapeseed (Han et al., 2016). The findings of a further study have indicated that the storage capacity for NO3− is limited in the absence of the AtVHA-a2 and AtVHA-a3 genes, with the vha-a2 vha-a3 double mutant being found to contain 80% less NO3−, whereas the total NO3− reductase activity had increased by 90% (Krebs et al., 2010). Similarly, it has been observed that the vacuoles of atvha-a2, atvha-a3, and atavp1 mutants contain less NO3− (Liao et al., 2019).
Driven by vacuolar proton pumps, NO3− enters the vacuole via chloride channel proteins (Gaxiola et al., 2001; De Angeli et al., 2006; Brüx et al., 2008). Chloride and malic acid channels, two main types of anion channels, are inward rectifying channels located in the tonoplast (Qu and Yu, 2008). CLC proteins facilitate the flux of anions such as Cl− and NO3−, whereas glutamate transport is limited. CLC proteins are activated only in the presence of cytoplasmic Ca2+ (Berechi et al., 1999). This activation is promoted by calmodulin domain protein kinase (CDPK) in the presence of ATP and inhibited by niflumic acid. Unlike general anion channels, CLCs are not inhibited by 4,4′-dinitrostilbene-2,2′-disulfonic acid (White and Broadley, 2001; Yu and Liu, 2004; Qu et al., 2009).
In Arabidopsis, NRT2.7 and CLCa are two transporters that facilitate the transfer of NO3− into the vacuole (Geelen et al., 2000; Chopin et al., 2007). Other transporters with potentially similar functions include CLCc and NPF6.2 (Chiu et al., 2004; Harada et al., 2004). Seven CLC family members have been identified in Arabidopsis thaliana, and De Angeli and colleagues (2006) were the first to demonstrate that AtCLCa is a tonoplast-localized 2NO3−/H+ antiporter involved in the regulation of NO3− sequestration into vacuoles. It has been found that the accumulation of NO3− in the vacuole is reduced by approximately 50% and that the contents of NO3− in both shoots and roots are reduced in atclca mutant plants (Geelen et al., 2000; De Angeli et al., 2006; Monachello et al., 2009). Similar results were obtained in a study by Liao et al. (2019). AtCLCc is also assumed to participate in vacuolar NO3− storage, given that it is tonoplast localized, and plants with a mutation in this proton are characterized by lower NO3− contents (Harada et al., 2004). Among the NPF proteins in Arabidopsis, AtNPF6.2 is specifically expressed in NO3−-rich petioles, thereby indicating that it might participate in the accumulation of NO3− in petiole cell vacuoles. However, the function of AtNPF6.2 has yet to be conclusively established owing to a lack of evidence to indicate whether this protein is located in the vacuolar membrane (Chiu et al., 2004). AtNRT2.7, which is located in the tonoplast and expressed exclusively in seeds, is, to date, the only protein in the NRT family confirmed to participate in the vacuolar storage of NO3−. Within seeds, this protein is responsible for the transport of NO3− from the cytoplasm into the vacuole for storage, thereby contributing to seed nitrogen accumulation (Chopin et al., 2007). Compared with NPF and NRT2 family proteins, CLC proteins are primarily responsible for vacuolar NO3− accumulation during plant growth, and a reduction in their activity enhances the utilization of NO3−, thereby improving the nitrogen-use efficiency of crops (Geelen et al., 2000; De Angeli et al., 2006; Monachello et al., 2009).
With respect to the efflux of NO3− from vacuoles, it has been established that the tonoplast-localized proteins AtCLCb and OsNPF7.2 could transport NO3−, although there is currently no direct evidence to indicate whether these proteins are involved in vacuolar NO3− influx or efflux (von der Fecht-Bartenbach et al., 2010; Hu et al., 2016). AtNPF5.11, AtNPF5.12, and AtNPF5.16, regulate NO3− re-allocation between roots and shoots by mediating NO3− efflux from the vacuole to the cytosol. In triple mutants characterized by disruption of AtNPF5.11, AtNPF5.12, and AtNPF5.16, it has been found that larger amounts of root-fed 15NO3− are translocated to the shoots (He et al., 2017). Moreover, it is speculated that the tonoplast-localized OsNPF7.2 could also be involved in NO3− efflux from the vacuoles, and heterologous expression in Xenopus laevis oocytes has indicated that it mediates NO3− absorption, although, to date, it has yet to be demonstrated whether functional disruption of OsNPF7.2 leads to an accumulation of NO3− within the vacuoles (von der Fecht-Bartenbach et al., 2010; Hu et al., 2016). Furthermore, NO3− supply has a significant effect on NO3− distribution, both in the metabolic pool and the storage pool of leaf blades in three leafy vegetables (rapeseed, Chinese cabbage, and spinach) (Chen et al., 2004).
The 2NO3−/H+ antiporter located in the tonoplast assists in the transfer of NO3− into the vacuole from the cytoplasm (De Angeli et al., 2006; Krebs et al., 2010). From the perspective of nitrogen-use efficiency, the role of vacuolar NO3− in maintaining an osmotic balance within the vacuole is considered wasteful (Shen et al., 2003). However, whether it would be feasible to replace vacuolar NO3− with Cl− to maintain cell osmotic pressure within a certain range, and thereby reduce the level of NO3− accumulated in the vacuole, remains to be ascertained.
Long-Distance (Root to Shoot) No3− Transport in Vegetables
A proportion of the NO3− absorbed by plant roots is stored within vacuoles or assimilated into organic nitrogen through a series of reactions catalyzed by NO3− reductase, nitrite reductase, glutamine synthetase, and glutamate synthase (Williams et al., 1987) (Figure 1C). However, most of the NO3− taken up by roots is transported through cortical tissues into xylem conduits for long-distance transport to different shoot tissues and organs for subsequent assimilation and utilization in plant growth and development (Han et al., 2016; Zhang, 2017) (Figure 1B).
NO3− is primarily transported upward by the xylem and downward by the phloem (Dechorgnat et al., 2011). The long-distance transport and distribution of NO3− between roots and shoots in Arabidopsis are mainly co-regulated by NPF7.3 and NPF7.2, two members of the PTR family, the expression of which is strongly induced by NO3− (Lin et al., 2008; Li et al., 2010; Chen et al., 2012; Léran et al., 2014; Zhang Z. H. et al., 2014) (Figure 1C). AtNPF7.3 is predominantly expressed in the columnar sheath cells surrounding the protoxylem in the root and is responsible for the loading of NO3− from the root cytoplasm into the xylem for transport to the shoots (Lin et al., 2008). AtNPF7.2 is expressed in xylem parenchyma cells and affects NO3− transport from roots to shoots by regulating NO3− unloading from the xylem (Li et al., 2010). Thus, although playing opposing roles in the loading and unloading of NO3− into and out of the xylem, AtNPF7.3 and AtNPF7.2, respectively, cooperatively contribute to the long-distance transport of NO3− from the roots to the shoots. Furthermore, OsNPF2.2 has been shown to be involved in unloading NO3− from the xylem, thereby facilitating root to shoot NO3− transport and plant development. osnpf2.2 mutant plants have been found to maintain high levels of NO3− in the roots and have a low shoot:root NO3− ratio (Li et al., 2015). Moreover, it has been observed that AtNPF2.3 plays an important role in root to shoot NO3− translocation in plants subjected to saline conditions (Taochy et al., 2015).
Currently, AtNPF2.9 is the only gene that has been confirmed to play a role in the loading of NO3− into root phloem and negatively regulates the root to shoot transport of NO3− (Fan et al., 2009; Wang and Tsay, 2011). It has also been established that AtNPF5.11, AtNPF5.12, and AtNPF5.16 play roles in the uptake of NO3− from the vacuole to the cytoplasm in Arabidopsis to regulate the shoot:root ratio, and in a triple mutant for these genes, larger amounts of NO3− were found to be translocated to the shoots (He et al., 2017). In Arabidopsis, AtNPF2.9, AtNPF7.2, and AtNPF7.3 are the main NO3− transporters contributing to the regulation of the long-distance transport and redistribution of NO3− from roots to shoots (Figure 1C). In this regard, it is worth noting that the concentration of NO3− transported in the xylem is typically several tens to hundreds of times higher than that carried in the phloem, which accordingly explains why studies on NO3− distribution in shoots and roots have focused primarily on NO3− transport in the xylem rather than in the phloem (Lin et al., 2008; Li et al., 2010; Wang and Tsay, 2011). To date, research on the relationships between NPF genes and NO3− long-distance transport in crop plants has mainly been conducted on wheat, rice, and rapeseed. However, only the responses of the NPF7.3 and NPF7.2 genes to nitrogen stress have been studied in wheat (Xuan et al., 2014), whereas the physiological characteristics of nitrogen have been analyzed in rice lines overexpressing OsNPF7.3 (Ma, 2011). In our studies, we have analyzed the response of NPF7.3 and NPF7.2 to NO3− deficiency and constructed a co-expression network to identify key genes involved in NO3− transport from root to shoot in rapeseed seedlings (Hua et al., 2018; Liang et al., 2019). Evidence that has accumulated to date essentially confirms that NPF7.3 and NPF7.2 regulate NO3− transport and distribution in a range of crop plants, in which they play roles similar to those observed in A. thaliana. We thus believe that the relationships between NO3− long-distance transport and NPF genes described in Arabidopsis would also be applicable to cultivated crops or vegetables.
Xylem flow is known to be strongly influenced by transpiration, which in turn has a considerable effect on the long-distance transport of NO3−. NO3− loaded into the xylem is continuously transported to the above-ground parts of plants, mediated through the influence of the transpirational pull, thereby promoting the transport of NO3− from the roots to shoots (Li et al., 2010; Chen et al., 2012). Consistently, it has been demonstrated that the inhibition of transpiration reduces the transport of NO3− to the shoots in pea plants, thus resulting in an increase in the accumulation of NO− in the roots and a reduction in contents in the edible parts of plants (Hernandez et al., 1997). The long-distance transport of NO3− not only determines the distribution and assimilation in different tissues, but also represents an important physiological mechanism whereby plants respond to environmental change (Zhang G. B. et al., 2014). Chen et al. (2012) and Li et al. (2010) have shown that transpiration regulates the transport of nutrient elements from roots to shoots under normal conditions, whereas NO3− accumulation in roots, which is also mediated by AtNPF7.3 and AtNPF7.2, might be the dominant regulatory factor in NO3− distribution in plants exposed to stress. For example, under conditions of cadmium stress, wild-type Arabidopsis plants have been observed to maintain a higher root to shoot NO3− ratio than atnpf7.2 mutant plants, indicating that this ratio is actively regulated by NPF7.2 and other transporters, rather than passively by transpiration (Li et al., 2010). Therefore, by inhibiting the expression of the NPF7.3 gene and enhancing that of NPF7.2 in plants, it might be possible to control the long-distance transport of NO3− from roots to shoots, which would represent a useful approach for reducing the content of NO3− in leafy vegetables.
Conclusions and Future Developments
In plants, NO3− accumulation depends on its absorption and metabolism. Plants actively take up NO3− from the environment through a proton/nitrate-coupled mechanism (Wang et al., 2018). The NO3− absorbed by plants can be directly assimilated into organic nitrogen, secreted into the rhizosphere, accumulated in the vacuole, or transported from roots to shoots (Zhang, 2017). The concentrations of NO3− in plants are thus affected by multiple processes, among which there is a close interdependency that facilitates the coordinated regulation of NO3− accumulation in plants.
Vacuolar NO3− accumulation and release play important roles in regulating the concentration and re-allocation of NO3−. The influx of vacuolar NO3− is mainly affected by the tonoplast-localized proteins, such as AtCLCa, AtCLCc, AtNPF6.2, and AtNRT2.7, whereas AtCLCb and OsNPF7.2 are believed to play transporting roles, although there is currently no direct evidence to indicate whether these proteins are involved in vacuolar NO3− influx or efflux (von der Fecht-Bartenbach et al., 2010; Hu et al., 2016). Furthermore, AtNPF5.11, AtNPF5.12, and AtNPF5.16 regulate NO3− re-allocation between roots and shoots by mediating NO3− efflux from the vacuole to the cytosol (He et al., 2017). However, the main factors regulating the short-distance of NO3− are the tonoplast CLC proteins and proton pump transport systems (Chiu et al., 2004; Chopin et al., 2007). The NO3− transport system is present in the vacuolar membrane in all plant tissues and represents the main process whereby short-distance NO3− distribution is regulated (De Angeli et al., 2006; Krebs et al., 2010). By inhibiting the activity of both tonoplast proton pumps and the CLC proteins, a larger proportion of plant NO3− could be re-distributed in the cytoplasm, wherein it can subsequently be metabolized, thereby contributing to a reduction in the NO3− content of vegetables.
The vacuolar–cytoplasmic distribution of NO3− affects not only the distribution and utilization of NO3− (Gaxiola et al., 2001; Brüx et al., 2008; Krebs et al., 2010), but also affects the expression and function of genes induced by NO3− (Glass et al., 2002; Huang et al., 2013). NPF2.9, NPF7.3, and NPF7.2, which are responsible for NO3− loading in the phloem, loading in the xylem, and unloading from the xylem, respectively, are the main regulators of long-distance NO3− transport. However, the phloem is primarily involved in the transport of organic nitrogen, whereas the concentration of inorganic nitrogen carried in the phloem is typically in the order of tens to hundreds of times smaller than that in the xylem (Lin et al., 2008; Fan et al., 2009; Li et al., 2010; Wang and Tsay, 2011). Therefore, NPF7.3 and NPF7.2 are the main regulators of the long-distance transport of NO3−. Enhancing the activity of the NPF7.2 protein in conjunction with a reduction in the activity of the NPF7.3 protein would contribute to favorably regulating the long-distance transport of NO3−, thus reducing the transport of NO3− from roots to shoots, and thereby the contents of NO3− in leafy vegetables.
Research on mechanisms underlying the short- and long-distance translocation of NO3− needs to be further expanded to address the following questions (Li et al., 2010; Schroeder et al., 2013). (1) In addition to regulating osmotic functions, how does the storage of NO3− in vacuoles affect the expression and function of its inducible genes? (2) What is the difference between the contribution of chloride channels (CLC) and proton pumps with respect to the distribution of NO3− in vacuoles? (3) Given that the transport of NO3− from cytoplasm to vacuoles is primarily regulated by CLCa, how does this process affect the further assimilation of NO3−? On the basis of the findings of previous studies, the vacuolar NO3− distribution system, which plays an important role in the short-distance NO3− distribution, and the vascular bundle long-distance transportation system, which is important in long-distance NO3− transportation, are identified as primary targets to further studies on mechanisms underlying the short-distance distribution and long-distance transport of NO3−. Gaining a better understanding of these mechanisms will contribute to facilitating a more effective control of NO3− contents in plants and provide important guidance for the breeding and cultivation of leafy vegetables with low NO3− concentrations.
Author Contributions
GL organized and wrote the original manuscript. GL and ZZ discussed and revised the manuscript and approved the final version.
Funding
This work was financially supported in part by the National Key R&D Program of China (2017YFD0200100 and 2017YFD0200103), the Hunan Provincial Recruitment Program of Foreign Experts, the National Oilseed Rape Production Technology System of China, “2011 Plan” supported by The Chinese Ministry of Education, and the Double FirstClass Construction Project of Hunan Agricultural University (kxk201801005).
Conflict of Interest
The authors declare that the research was conducted in the absence of any commercial or financial relationships that could be construed as a potential conflict of interest.
References
Anjana, S. U., Iqbal, M. (2007). Nitrate accumulation in plants, factors affecting the process, and human health implications. Agron. Sustain Dev. 27, 45–57. doi: 10.1051/agro:2006021
Bahadoran, Z., Mirmiran, P., Jeddi, S., Azizi, F., Ghasemi, A., Hadaegh, F. (2016). Nitrate and nitrite content of vegetables, fruits, grains, legumes, dairy products, meats and processed meats. J. Food Compos. Anal. 51, 93–105. doi: 10.1016/j.jfca.2016.06.006
Berechi, G., Varga, Z., Van Iren, F., Van Duijn, B. (1999). Anion channels in characorallina tonoplast membrane: calcium dependence and rectification. J. Membr. Biol. 172, 159–168. doi: 10.1007/s002329900593
Bondonno, C. P., Blekkenhorst, L. C., Liu, A. H., Bondonno, N. P., Ward, N. C., Croft, K. D., et al. (2018). Vegetable-derived bioactive nitrate and cardiovascular health. Mol. Asp. Med. 61, 83–91. doi: 10.1016/j.mam.2017.08.001
Brüx, A., Liu, T. Y., Krebs, M., Stierhof, Y. D., Lohmann, J. U., Miersch, O., et al. (2008). Reduced V–ATPPase activity in the trans–Golgi network causes oxylipin–dependent hypocotyl growth inhibition in Arabidopsis. Plant Cell 20, 1088–1100. doi: 10.1105/tpc.108.058362
Burns, I. G., Zhang, K., Turner, M. K., Meacham, M., Al-Redhiman, K., Lynn, J., et al. (2011). Screening for genotype and environment effects on nitrate accumulation in 24 species of young lettuce. J. Sci. Food Agric. 91, 553–562. doi: 10.1002/jsfa.4220
Carter, C., Pan, S., Zouhar, J., Avila, E. L., Girke, T., Raikhel, N. V. (2004). The vegetative vacuole proteome of Arabidopsis thaliana reveals predicted and unexpected proteins. Plant Cell 16, 3285–3303. doi: 10.1105/tpc.104.027078
Chen, B. M., Wang, Z. H., Li, S. X., Wang, G. X., Song, H. X., Wang, X. N. (2004). Effects of nitrate supply on plant growth, nitrate accumulation, metabolic nitrate concentration and nitrate reductase activity in three leafy vegetables. Plant Sci. 167, 635–643. doi: 10.1016/j.plantsci.2004.05.015
Chen, C. Z., Lv, X. F., Li, J. Y., Yi, H. Y., Gong, J. M. (2012). Arabidopsis NRT1.5 is another essential component in the regulation of nitrate reallocation and stress tolerance. Plant Physiol. 159, 1582–1590. doi: 10.1104/pp.112.199257
Chetty, A. A., Prasad, S., Pinho, O. C., de Morais, C. M. (2018). Estimated dietary intake of nitrate and nitrite from meat consumed in Fiji. Food Chem. 278, 630–635. doi: 10.1016/j.foodchem.2018.11.081
Chiu, C. C., Lin, C. S., Hsia, A. P., Su, R. C., Lin, H. L., Tsay, Y. F. (2004). Mutation of a nitrate transporter, AtNRT1.4, results in a reduced petiole nitrate content and altered leaf development. Plant Cell Physiol. 45, 1139–1148. doi: 10.1093/pcp/pch143
Chopin, F., Orsel, M., Dorbe, M. F., Chardon, F., Truong, H. N., Miller, A. J., et al. (2007). The Arabidopsis ATNRT2.7 nitrate transporter controls nitrate content in seeds. Plant Cell 19, 1590–1602. doi: 10.1105/tpc.107.050542
Cipriano, D. J., Wang, Y., Bond, S., Hinton, A., Jefferies, K. C., Qi, J., et al. (2008). Structure and regulation of the vacuolar ATPase. Biochim. Biophys. Acta 1777, 599–604. doi: 10.1016/j.bbabio.2008.03.013
De Angeli, A., Monachello, D., Ephritikhine, G., Frachisse, J. M., Thomine, S., Gambale, F., et al. (2006). The nitrate/proton antiporter AtCLCa mediates nitrate accumulation in plant vacuoles. Nature 442, 939–942. doi: 10.1038/nature05013
Dechorgnat, J., Nguyen, C. T., Armengaud, P., Jossier, M., Diatloff, E., Filleur, S., et al. (2011). From the soil to the seeds: the long journey of nitrate in plants. J. Exp. Bot. 62, 1349–1359. doi: 10.1093/jxb/erq409
Du, S. T., Li, L. L., Zhang, Y. S., Lin, X. Y. (2008). Nitrate accumulation discrepancies and variety selection in different Chinese cabbage (Brassica chinensis L.) genotypes. J. Plant Nutr. 14, 969–975. doi: 10.11674/zwyf.2008.0524
Fan, S. C., Lin, C. S., Hsu, P. K., Lin, S. H., Tasy, Y. F. (2009). The Arabidopsis nitrate transporter NRT1.7, expressed in phloem, is responsible for source–to–sink remobilization of nitrate. Plant Cell 21, 2750–2761. doi: 10.1105/tpc.109.067603
Filleur, S., Dorbe, M. F., Cerezo, M., Orsel, M., Granier, F., Gojon, A., et al. (2001). An Arabidopsis T-DNA mutant affected in NRT2 genes is impaired in nitrate uptake. FEBS Lett. 489, 220–224. doi: 10.1016/S0014-5793(01)02096-8
Gaxiola, R. A., Li, J., Undurraga, S., Dang, L. M., Allen, G. J., Alper, S. L., et al. (2001). Drought and salt tolerant plants result from over expression of the AVP1 H+–pump. Proc. Natl. Acad. Sci. U. S. A. 98, 11444–11449. doi: 10.1073/pnas.191389398
Geelen, D., Lurin, C., Bouchez, D., Frachisse, J. M., Lelièvre, F., Courtial, B., et al. (2000). Disruption of putative anion channel gene AtCLC–a in Arabidopsis suggests a role in the regulation of nitrate content. Plant J. 21, 259–267. doi: 10.1046/j.1365-313x.2000.00680.x
Glass, A. D., Britto, D. T., Kaiser, B. N., Kinghorn, J. R., Kronzucker, H. J., Kumar, A., et al. (2002). The regulation of nitrate and ammonium transport systems in plants. J. Exp. Bot. 53, 855–864. doi: 10.1093/jexbot/53.370.855
Han, Y. L., Liu, Q., Gu, J. D., Gong, J. M., Guan, C. Y., Lepo, J. E., et al. (2014). V–ATPase and V–PPase at the Tonoplast Affect NO3– Content in Brassica napus by Controlling Distribution of NO3– Between the Cytoplasm and Vacuole. J. Plant Growth Regul. 34, 22–34. doi: 10.1007/s00344-014-9439-8
Han, Y. L., Liao, Q., Yu, Y., Song, H. X., Liu, Q., Rong, X. M., et al. (2015). Nitrate reutilization mechanisms in the tonoplast of two Brassica napus genotypes with different nitrogen use efficiency. Acta Physiol. Planturum 37, 42. doi: 10.1007/s11738-014-1744-0
Han, Y. L., Song, H. X., Liao, Q., Yu, Y., Jian, S. F., Lepo, J. E., et al. (2016). Nitrogen use efficiency is mediated by vacuolar nitrate sequestration capacity in roots of Brassica napus. Plant Physiol. 170, 1684–1698. doi: 10.1104/pp.15.01377
Harada, H., Kuromori, T., Hirayama, T., Shinozaki, K., Leigh, R. A. (2004). Quantitative trait loci analysis of nitrate storage in Arabidopsis leading to an investigation of the contribution of the anion channel gene, AtCLC-c, to variation in nitrate levels. J. Exp. Bot. 55, 2005–2014. doi: 10.1093/jxb/erh224
He, Y. N., Peng, J. S., Cai, Y., Liu, D. F., Guan, Y., Yi, H. Y., et al. (2017). Tonoplast-localized Nitrate Uptake Transporters Involved in Vacuolar Nitrate Efflux and Reallocation in Arabidopsis. Sci. Rep. 7, 6417. doi: 10.1038/s41598-017-06744-5
Hernandez, L. E., Garate, A., CarpenaRuiz, R. (1997). Effects of cadmium on the uptake, distribution and assimilation of nitrate in Pisum sativum. Plant Soil 189, 97–106. doi: 10.1023/A:1004252816355
Ho, C. H., Lin, S. H., Hu, H. C., Tsay, Y. F. (2009). CHL1 functions as a nitrate sensor in plants. Cell 138, 1184–1194. doi: 10.1016/j.cell.2009.07.004
Hu, R., Qiu, D., Chen, Y., Miller, A. J., Fan, X., Pan, X., et al. (2016). Knock-down of a tonoplast localized low-affinity nitrate transporter OsNPF7.2 affects rice growth under high nitrate supply. Front. Plant Sci. 7, 1529. doi: 10.3389/fpls.2016.01529
Hua, Y. P., Zhou, T., Liao, Q., Song, H. X., Guan, C. Y., Zhang, Z. H. (2018). Genomics-Assisted Identification and Characterization of the Genetic Variants Underlying Differential Nitrogen Use Efficiencies in Allotetraploid Rapeseed Genotypes. G3 8, 2757–2771. doi: 10.1534/g3.118.200481
Huang, N. C., Liu, K. H., Lo, H. J., Tsay, Y. F. (1999). Cloning and functional characterization of an Arabidopsis nitrate transporter gene that encodes a constitutive component of low-affinity uptake. Plant Cell 11, 1381–1392. doi: 10.1105/tpc.11.8.1381
Huang, H. T., Zhang, Z. H., Song, H. X. (2012). Nitrate nitrogen reutilization based on proton pump of vacuole membrane. Chin. J. Eco. 31, 731–737. doi: 10.13292/j.1000-4890.2012.0142
Huang, H. T., Rong, X. M., Song, H. X., Liu, Q., Liao, Q., Luo, J. P., et al. (2013). Effects of nitrate reductase (NR) inhibitor on NR activity in oilseed rape and its relation to nitrate content. Acta Agro. Sin. 39, 1668–1673. doi: 10.3724/SP.J.1006.2013.01668
Jaquinod, M., Villiers, F., Kieffer-Jaquinod, S., Hugouvieux, V., Bruley, C., Garin, J., et al. (2007). A proteomics dissection of Arabidopsis thaliana vacuoles isolated from cell culture. Mol. Cell Proteomics 6, 394–412. doi: 10.1074/mcp.M600250-MCP200
Ju, C. X., Buresh, R. J., Wang, Z. Q., Zhang, H., Liu, L. J., Yang, J. C., et al. (2015). Root and shoot traits for rice varieties with higher grain yield and higher nitrogen use efficiency at lower nitrogen rates application. Field Crops Res. 175, 47–55. doi: 10.1016/j.fcr.2015.02.007
Kiba, T., Feria-Bourrellier, A. B., Lafouge, F., Lezhneva, L., Boutet-Mercey, S., Orsel, M., et al. (2012). The Arabidopsis nitrate transporter NRT2.4 plays a double role in roots and shoots of nitrogen-starved plants. Plant Cell 24, 245–258. doi: 10.1105/tpc.111.092221
Krebs, M., Beyhl, D., Görlich, E., Al-Rasheid, K. A., Marten, I., Stierhof, Y. D., et al. (2010). Arabidopsis V-ATPase activity at the tonoplast is required for efficient nutrient storage but not for sodium accumulation. Proc. Natl. Acad. Sci. U. S. A. 107, 3251–3256. doi: 10.1073/pnas.0913035107
Kriegel, A., Andrés, Z., Medzihradszky, A., Krüger, F., Scholl, S., Delang, S., et al. (2015). Job sharing in the endomembrane system: vacuolar acidification requires the combined activity of V-ATPase and V-PPase. Plant Cell 27, 3383–3396. doi: 10.1105/tpc.15.00733
Léran, S., Varala, K., Boyer, J. C., Chiurazzi, M., Crawpord, N., Daniel-Vedele, F., et al. (2014). A unified nomenclature of nitrate transporter 1/peptide transporter family members in plants. Trends Plant Sci. 19, 5–9. doi: 10.1016/j.tplants.2013.08.008
Lezhneva, L., Kiba, T., Feria-Bourrellier, A. B., Lafouge, F., Boutet-Mercey, S., Zoufan, P., et al. (2014). The Arabidopsis nitrate transporter NRT2.5 plays a role in nitrate acquisition and remobilization in nitrogen-starved plant. Plant J. 80, 230–241. doi: 10.1111/tpj.12626
Li, J. Y., Gong, J. M. (2011). Nitrate signal sensing and transduction in higher plants. Plant Physiol. J. 47, 111–118.
Li, J. Y., Fu, Y. L., Pike, S. M., Bao, J., Tian, W., Zhang, Y., et al. (2010). The Arabidopsis nitrate transporter NRT1.8 functions in nitrate removal from the xylem sap and mediates cadmium tolerance. Plant Cell 22, 1633–1646. doi: 10.1105/tpc.110.075242
Li, Y., Ouyang, J., Wang, Y. Y., Hu, R., Xia, K., Duan, J., et al. (2015). Disruption of the rice nitrate transporter OsNPF2.2 hinders root-to-shoot nitrate transport and vascular development. Sci. Rep. 5, 9635. doi: 10.1038/srep09635
Liang, G. H., Hua, Y. P., Zhou, T., Liao, Q., Song, H. X., Zhang, Z. H. (2019). Bioinformatics Analysis and Response to Nitrate-Cadmium Stress of NRT1.5 and NRT1.8 Family Genes in Brassica napus. Acta Agro. Sin. 45, 365–380. doi: 10.3724/SP.J.1006.2019.84099
Liao, X. R., Chen, T., Liu, X. L. (2002). The formation and function of plant vacuole. Chin. J. Cell Biol. 24, 95–101. doi: 10.3969/j.issn.1674-7666.2002.02.010
Liao, Q., Zhou, T., Yao, J. Y., Han, Q. F., Song, H. X., Guan, C. Y., et al. (2018). Genome-scale characterization of the vacuole nitrate transporter Chloride Channel (CLC) genes and their transcriptional responses to diverse nutrient stresses in allotetraploid rapeseed. PLoS One. 13, e0208648. doi: 10.1371/journal.pone.0208648
Liao, Q., Jian, S. F., Song, H. X., Guan, C. Y., Lepo, J. E., Ismail, A. M., et al. (2019). Balance between nitrogen use efficiency and cadmium tolerance in Brassica napus and Arabidopsis thaliana. Plant Sci. 284, 57–66. doi: 10.1016/j.plantsci.2019.04.003
Lin, S. H., Kuo, H. F., Canivenc, G., Lin, C. S., Lepetit, M., Hsu, P. K., et al. (2008). Mutation of the Arabidopsis NRT1.5 nitrate transporter causes defective root-to-shoot nitrate transport. Plant Cell 20, 2514–2528. doi: 10.1105/tpc.108.060244
Lin, S. M., Tsai, J. Y., Hsiao, C. D., Huang, Y. T., Chiu, C. L., Liu, M. H., et al. (2012). Crystal structure of a membrane-embedded H+-translocating pyrophosphatase. Nature 484, 399–403. doi: 10.1038/nature10963
Liu, K. H., Huang, C. Y., Tsay, Y. F. (1999). CHL1 is a dual-affinity nitrate transporter of Arabidopsis involved in multiple phases of nitrate uptake. Plant Cell 11, 865–874. doi: 10.1105/tpc.11.5.865
Luo, Y. S. (2012). Research progress of H+-ATPase activities in plant plasma membrane and vacuolar membrane under salt stress. J. Anhui Agric. Sci. 40, 1263–1265, 1280. doi: 10.13989/j.cnki.0517-6611.2012.03.001
Ma, C. (2011). Characteristics of over–expression for nitrate transporter genes OsNRT1.2 and OsNRT1.5 in rice. Nanjing: Master Dissertation Nanjing Agric. Univ.
Maeshima, M. (2000). Vacuolar H+-pyrophosphatase. Biochim. Biophys. Acta 1465, 37–51. doi: 10.1016/S0005-2736(00)00130-9
Martinoia, E., Heck, U., Wiemken, A. (1981). Vacuoles as storage compartments for nitrate in barley leaves. Nature 289, 292–294. doi: 10.1038/289292a0
Martinoia, E., Massonneau, A., Frangne, N. (2000). Transport processes of solutes across the vacuolar membrane of higher plants. Plant Cell Physiol. 41, 1175–1186. doi: 10.1093/pcp/pcd059
Martinoia, E., Maeshima, M., Neuhaus, H. E. (2007). Vacuolar transporters and their essential role in plant metabolism. J. Exp. Bot. 58, 83–102. doi: 10.1093/jxb/erl183
Miller, A. J., Fan, X., Orsel, M., Smith, S. J., Well, D. M. (2007). Nitrate transport and signaling. J. Exp. Bot. 58, 2297–2306. doi: 10.1093/jxb/erm066
Monachello, D., Allot, M., Oliva, S., Krapp, A., Daniel-Vedele, F., Barbier-Brygoo, H., et al. (2009). Two anion transporters AtClCa and AtClCe fulfil interconnecting but not redundant roles in nitrate assimilation pathways. New Phytol. 183, 88–94. doi: 10.1111/j.1469-8137.2009.02837.x
Peng, S., Buresh, R. J., Huang, J., Huang, J., Zhong, X., Zou, Y., et al. (2010). Improving nitrogen fertilization in rice by site-specific N management. Agro. Sustain. Dev. 30, 649–656. doi: 10.1051/agro/2010002
Qu, Y. N., Yu, B. J. (2008). Effects of chloride channel blockers on ion contents and other physiological indexes of glycine soja and glycinemax seedlings under NaCl stress. J. Nanjing Agric. Univ. 31, 17–21. doi: 10.3321/j.issn:1000-2030.2008.02.004
Qu, Y. N., Zhou, Q., Yu, B. J. (2009). Effects of Zn2+ and nuflumic acid on photosynthesis in Glycine soja and Glycine max seedlings under NaCl stress. Environ. Exp. Bot. 65, 304–309. doi: 10.1016/j.envexpbot.2008.11.005
Quijano, L., Yusà, V., Font, G., McAllister, C., Torres, C., Pardo, O. (2017). Risk assessment and monitoring programme of nitrates through vegetables in the region of Valencia (Spain). Food Chem. Toxicol. 100, 42–49. doi: 10.1016/j.fct.2016.12.010
Salehzadeh, H., Maleki, A., Rezaee, R., Shahmoradi, B., Ponnet, K. (2020). The nitrate content of fresh and cooked vegetables and their health-related risks. PLoS One 15, e0227551. doi: 10.1371/journal.pone.0227551
Schroeder, J. L., Delhaize, E., Frommer, W. B., Guerinot, M. L., Harrison, M. J., Herrera-Estrella, L., et al. (2013). Using membrane transporters to improve crops for sustainable food production. Nature 497, 60–66. doi: 10.1038/nature11909
Shen, Q. R., Tang, L., Xu, Y. C. (2003). A review on the behavior of nitrate in vacuoles of plants. Acta Pedol. Sin. 40, 465–470. doi: 10.3321/j.issn:0564-3929.2003.03.022
Tang, Y., Sun, X., Hu, C., Tan, Q., Zhao, X. (2013). Genotypic differences in nitrate uptake, translocation and assimilation of two Chinese cabbage cultivars [Brassica campestris L. ssp. Chinesnsis (L.)]. Plant Physiol. Biochem. 70, 14–20. doi: 10.1016/j.plaphy.2013.04.027
Taochy, C., Gaillard, I., Ipotesi, E., Oomen, R., Leonhardt, N., Zimmermann, S., et al. (2015). The Arabidopsis root stele transporter NPF2.3 contributes to nitrate translocation to shoots under salt stress. Plant J. 83, 466–479. doi: 10.1111/tpj.12901
von der Fecht-Bartenbach, J., Bogner, M., Dynowski, M., Ludewig, U. (2010). CLC-b-mediated NO3-/H+ exchange across the tonoplast of Arabidopsis vacuoles. Plant Cell Physiol. 51, 960–968. doi: 10.1093/pcp/pcq062
Wang, Y. Y., Tsay, Y. F. (2011). Arabidopsis nitrate transporter NRT1.9 is important in phloem nitrate transport. Plant Cell 23, 1945–1957. doi: 10.1105/tpc.111.083618
Wang, Y. Y., Cheng, Y. H., Chen, K. E., Tsay, Y. F. (2018). Nitrate Transport, Signaling, and Use Efficiency. Annu. Rev. Plant Biol. 69, 85–122. doi: 10.1146/annurev-arplant-042817-040056
White, P. J., Broadley, M. R. (2001). Chloride in soils and its uptake and movement within the plant: a review. Ann. Bot. 88, 967–988. doi: 10.1006/anbo.2001.1540
Williams, K., Percival, F., Merino, J., Mooney, H. A. (1987). Estimation of tissue construction cost from heat of combustion and organic nitrogen content. Plant Cell Environ. 10, 725–734. doi: 10.1111/1365-3040.ep11604754
Xuan, H. M., Wang, Y. H., Wei, L. T. (2014). Transcription analysis of the genes encoding nitrate transporter NRT1 and NRT2 families in response to nitrogen starvation in wheat seedling leaves. J. Triticeae Crops 34, 1019–1028. doi: 10.7606/j.issn.1009-1041.2014.08.01
Yu, B. J., Liu, Y. L. (2004). Chlorine, Chloride channel and chloride tolerance in plants. Chin. Bull. Bot. 21, 402–410. doi: 10.3969/j.issn.1674-3466.2004.04.003
Zhang, G. B., Yi, H. Y., Gong, J. M. (2014). The Arabidopsis ethylene/jasmonic acid-NRT signaling module coordinates nitrate reallocation and the trade-off between growth and environmental adaption. Plant Cell 26, 3984–3998. doi: 10.1105/tpc.114.129296
Zhang, Z. H., Song, H. X., Liu, Q. (2014). Distribution Characters of Absorption Nitrogen in Oilseed Rape (Brassica napus L.) at Different Growth Stages. J. Plant Nutr. 37, 1648–1660. doi: 10.1080/01904167.2014.888747
Zhang, Z. H. (2017). The relationship between nitrate transport and utilization in crop and nitrogen utilization efficiency. J. Plant Nutr. 23, 217–223. doi: 10.11674/zwyf.15357
Zhao, L. H., Liu, Y. L. (1999). Tonoplast H+-pyrophosphatase and its response to stresses. Plant Physiol. J. 35, 441–445. doi: 10.13592/j.cnki.ppj.1999.06.001
Zhao, S. P., Zhang, R. L., Xu, M. F., Zheng, J. C. (2011). Influence of Different NH4+/NO3- Ratios on Nitrate Accumulation in Different Genotypes of Chinese Cabbage. Soils 43, 32–38. doi: 10.13758/j.cnki.tr.2011.01.024
Keywords: nitrate, proton pumps, chloride channel protein, NPF7.3, NPF7.2, vegetable, health
Citation: Liang G and Zhang Z (2020) Reducing the Nitrate Content in Vegetables Through Joint Regulation of Short-Distance Distribution and Long-Distance Transport. Front. Plant Sci. 11:1079. doi: 10.3389/fpls.2020.01079
Received: 30 April 2020; Accepted: 30 June 2020;
Published: 16 July 2020.
Edited by:
Pietro Santamaria, University of Bari Aldo Moro, ItalyReviewed by:
Xiaorong Fan, Nanjing Agricultural University, ChinaGrażyna Kłobus, University of Wrocław, Poland
Leandro Azevedo Santos, Universidade Federal Rural do Rio de Janeiro, Brazil
Copyright © 2020 Liang and Zhang. This is an open-access article distributed under the terms of the Creative Commons Attribution License (CC BY). The use, distribution or reproduction in other forums is permitted, provided the original author(s) and the copyright owner(s) are credited and that the original publication in this journal is cited, in accordance with accepted academic practice. No use, distribution or reproduction is permitted which does not comply with these terms.
*Correspondence: Zhenhua Zhang, emh6aDE0NjhAMTYzLmNvbQ==