- 1Department of Plant Biotechnology and Bioinformatics, Ghent University, Ghent, Belgium
- 2VIB Center for Plant Systems Biology, Ghent, Belgium
- 3VIB-VUB Center for Structural Biology, Brussels, Belgium
- 4Brussels Center for Redox Biology, Brussels, Belgium
- 5Structural Biology Brussels, Vrije Universiteit Brussel, Brussels, Belgium
- 6State Key Laboratory of Proteomics, Beijing Proteome Research Center, National Center for Protein Sciences, Beijing Institute of Lifeomics, Beijing, China
In proteins, hydrogen peroxide (H2O2) reacts with redox-sensitive cysteines to form cysteine sulfenic acid, also known as S-sulfenylation. These cysteine oxidation events can steer diverse cellular processes by altering protein interactions, trafficking, conformation, and function. Previously, we had identified S-sulfenylated proteins by using a tagged proteinaceous probe based on the yeast AP-1-like (Yap1) transcription factor that specifically reacts with sulfenic acids and traps them through a mixed disulfide bond. However, the identity of the S-sulfenylated amino acid residues within a protein remained enigmatic. By using the same transgenic YAP1C probe, we present here a technological advancement to identify in situ sulfenylated cysteine sites in Arabidopsis thaliana cells under control condition and oxidative stress. Briefly, the total extract of transgenic YAP1C A. thaliana cells was initially purified on IgG-Sepharose beads, followed by a tryptic digest. Then, the mixed disulfide-linked peptides were further enriched at the peptide level on an anti-YAP1C-derived peptide (C598SEIWDR) antibody. Subsequent mass spectrometry analysis with pLink 2 identified 1,745 YAP1C cross-linked peptides, indicating sulfenylated cysteines in over 1,000 proteins. Approximately 55% of these YAP1C-linked cysteines had previously been reported as redox-sensitive cysteines (S-sulfenylation, S-nitrosylation, and reversibly oxidized cysteines). The presented methodology provides a noninvasive approach to identify sulfenylated cysteines in any species that can be genetically modified.
Introduction
Biotic and abiotic stresses increase the production of reactive oxygen species (ROS) in plants. Hydrogen peroxide (H2O2) is recognized as a secondary messenger and can cause posttranslational modifications on proteins by oxidizing sulfur-containing amino acids, such as methionine and cysteine (Jacques et al., 2015; Waszczak et al., 2015). Cysteine is one of the least abundant amino acids, representing 1.86% of all amino acids in Arabidopsis thaliana (The UniProt Consortium, 2019), but its unique redox sensitivity properties make that it is often found at the active site of proteins (Backus, 2019). The redox sensitivity of Cys residues depends on its pKa and ionization state, both determined by the local structural environment in the protein. Sulfur-hydrogen bonds and neighboring positively charged residues (such as lysine, histidine, or arginine) can lower the pKa value of the thiol group, thereby stabilizing the thiolate form (Roos et al., 2013; Zaffagnini et al., 2019). In addition, also solvent accessibility and redox potential of cysteine will affect its reactivity (McConnell et al., 2019; Poole et al., 2020). Reaction of H2O2 with proteinaceous redox-sensitive cysteine thiols leads to the formation of cysteine sulfenic acid (-SOH), which is generally unstable and frequently an intermediary modification en route to more stable oxidation forms (Roos and Messens, 2011; Gupta and Carroll, 2014). For instance, -SOH can form intra- or intermolecular disulfides or mixed disulfides with another free thiol or glutathione (GSH), making it enzymatically reversible by the action of thioredoxins (TRXs) or glutaredoxins (GRXs), respectively (Roos and Messens, 2011; Akter et al., 2015b). Recently, extracellular H2O2 has been shown to be sensed through disulfide formation of extracellular cysteines in the plasma membrane receptor HYDROGEN PEROXIDE-INDUCED Ca2+ INCREASES 1 (HPCA1), leading to Ca2+ influx in guard cells (Wu et al., 2020). Conversely, besides disulfide formation, -SOH can further oxidize toward sulfinic (-SO2H) and sulfonic acid (-SO3H). Whereas -SO3H is generally considered as an irreversible modification associated with protein degradation (Huang et al., 2018), -SO2H can be reduced via sulfiredoxins (SRXs) (Biteau et al., 2003; Akter et al., 2018). Protein S-sulfenylation can directly regulate protein functions. For instance, in Arabidopsis, H2O2-dependent S-sulfenylation of BRASSINAZOLE-RESISTANT 1 (BZR1) promotes its interaction with transcriptional factors (TFs) and drives gene expression (Tian et al., 2018), whereas, S-sulfenylation of BRASSINOSTEROID INSENSITIVE 2 (BIN2) enhances the formation of phosphorylated BRI1-EMS-SUPPRESSOR 1 (BES1), which cannot transport and bind TFs in nuclei, thereby decreasing brassinosteroid (BR) signaling outputs (Song et al., 2019). Another example is the impact on the levels of the auxin biosynthetic precursor tryptophan by S-sulfenylation of a tryptophan synthetase β subunit 1 (Yuan et al., 2017). Alternatively, sulfenylation of catalytic cysteines can directly inhibit the enzymatic activity (Tanner et al., 2011; Gurrieri et al., 2019). As such, the identification of sulfenylated cysteine sites is a crucial step to advance our understanding of redox-regulated processes.
Over the past decade, indirect and direct approaches have been developed to capture and identify S-sulfenylated proteins (Takanishi et al., 2007; Yang et al., 2014, 2016; Gupta et al., 2017). Initially, carbon nucleophilic SOH-selective probes enabled the in situ detection of S-sulfenylation at the protein level in Arabidopsis and human cells (Leonard et al., 2009; Paulsen et al., 2012; Akter et al., 2015a). Further advancements in affinity-based enrichment strategies allowed the accurate identification of the sulfenylated cysteine residues within the proteins in both human and plant cells (Yang et al., 2014; Yang J. et al., 2015; Akter et al., 2018; Huang et al., 2019). In addition to these chemoproteomics approaches, a genetic construct based on the yeast (Saccharomyces cerevisiae) AP-1-like (Yap1) transcription factor was utilized to detect S-sulfenylated proteins. Yap1 forms mixed disulfides via its redox-active Cys598, located in the C-terminal cysteine-rich domain (cCRD), with the sulfenylated Cys36 of the oxidant receptor protein 1 (Orp1) (Delaunay et al., 2002). A Yap1-cCRD construct, in which Cys620 and Cys629 were mutated to alanine (Ala) and threonine (Thr), respectively, and solely the redox-active Cys598 was retained, was used for the identification of S-sulfenylated proteins in Escherichia coli (Takanishi et al., 2007), yeast (Takanishi and Wood, 2011), and the legume model plant Medicago truncatula (Oger et al., 2012). In Arabidopsis cells, we generated a Yap1-cCRD construct fused to a tandem affinity purification (TAP) tag for improved capture and downstream identification of S-sulfenylated proteins (Waszczak et al., 2014). With this Yap1-cCRD construct, designated YAP1C hereafter, 97 and 132 S-sulfenylated proteins had previously been detected in the Arabidopsis cytosol and chloroplast, respectively (Waszczak et al., 2014; De Smet et al., 2019), but the sulfenylated cysteines remained unknown. Here, we describe how a tailored double affinity purification strategy enables the identification of in situ sulfenylated cysteines in a noninvasive manner.
Materials and Methods
Plant Materials and Growth Conditions
Transgenic cells expressing the YAP1C construct were generated as previously reported (Waszczak et al., 2014). In summary, the Yap1 C-terminal cysteine-rich domain (cCRD) construct, entailing the Saccharomyces cerevisiae Yap1-coding region corresponding to Asn565 to Asn650, was codon-optimized for expression in A. thaliana (L.) Heynh. and synthesized with introduction of the mutations Cys620Ala and Cys629Thr. This genetic construct was fused with an N-terminal TAP tag, containing two IgG-binding domains of protein G and a streptavidin-binding peptide (SBP), separated by the Human Rhinovirus (HRV) 3C protease cleavage site. The YAP1C probe driven by a cauliflower mosaic virus 35S promoter was transformed in Arabidopsis cells. YAP1C expression levels were assessed by western blot analysis (Waszczak et al., 2014). The PSB-D Arabidopsis cell suspension cultures (NASC stock no. CCL84840) were maintained as described in the ABRC Cell Culture Handling Protocol1. For H2O2 treatments, 500 mL of mid-log phase (3 days after culture refreshing, OD600 = 0.9) cells in 1-L glass flasks were treated with 20 mM H2O2 for 30 min before the cells were harvested through a vacuum filtration system (Pall Corporation, Port Washington, NY, United States) and snap-frozen in liquid nitrogen before storage at −70°C.
Protein Extraction
Frozen cell pellets harvested from approximately 1 L of suspension cultures were crushed with fine quartz granules (Merck, Darmstadt, Germany) with a precooled mortar and pestle in ice-cold lysis buffer (25 mM Tris, 15 mM MgCl2, 150 mM NaCl, 15 mM pNO2 PhenylPO4, 60 mM β-glycerophosphate, 0.1% NP-40, 0.1 mM Na3VO4, 1 mM NaF, 1 mM phenylmethylsulfonyl fluoride, 1 μM trans-epoxysuccinyl-L-leucylamido(4-guanidino)butane [E64], 1/50 mL ethylenediaminetetraacetic acid [EDTA]-free Ultra Complete tablet, 5% [v/v] ethylene glycol, 0.1 mg/mL 4-(2-aminoethyl) benzenesulfonyl fluoride hydrochloride [AEBSF], 0.1% benzonase, and 1 μg/mL leupeptin, pH 7.6), supplemented with 10 mM iodoacetamide (IAM) to prevent artificial oxidation of cysteine residues during the extraction procedure. After two rounds of centrifugation (20,000 × g for 20 min; 4°C), the supernatant was collected and protein concentrations were determined with the Bradford Protein Assay (He, 2011).
Anti-C598SEIWDR Antibody Production and Its Coupling on Magnetic Beads
The C598SEIWDR peptide was synthetized (purity >85%) and conjugated to Keyhole Limpet Hemocyanin (KLH) as a carrier (GenScript, Nanjing, China) and 0.2 mg of the C598SEIWDR-KLH conjugate, together with Freund’s incomplete adjuvant, were injected subcutaneously into four New Zealand rabbits at 14, 28, and 42 days. Seven days after the second and third immunization, approximately 20 and 40 mL (60 mL) of serum, respectively, were obtained from each animal. Three sera were retained for further purification, based on their high specificity against the “C598SEIWDR” peptide (high ELISA titer, >1: 512,000), with a “C598SEIWDR” peptide-coupled affinity iodoacetyl resin. Subsequently, the anti-C598SEIWDR antibodies were coupled on BcMagTM Epoxy-Activated Magnetic Beads (Bioclone Inc., San Diego, CA, United States) (Hamperl et al., 2014). Five mg of antibody was diluted to 3 mg/mL with coupling buffer (0.1 M sodium phosphate, pH 7.4) and incubated with 15 mg equilibrated Epoxy-Activated beads for 18 h at 30°C with gentle rotation (1000 rpm). After the beads had been washed twice with 100 mM glycine-HCl (pH 2.5) and then once with 10 mM Tris–HCl (pH 8.8), they were inactivated by 0.1 M trimethylamine and washed four times with phosphate-buffered saline (PBS) buffer (pH 7.4) and then twice with the PBS buffer (pH 7.4) containing 0.5% (w/v) Triton X-100. Finally, the antibody-coupled beads were suspended in 1 mL PBS buffer (pH 7.4), containing 0.02% (w/v) sodium azide and stored at 4°C until use.
Affinity Purification
The initial purification at the protein level on IgG-Sepharose 6 Fast Flow beads (GE Healthcare, Chicago, IL, United States) was performed as described with some modifications (Van Leene et al., 2007). Briefly, 150 mg of protein extract was first incubated with 300 μL of IgG-Sepharose beads, preequilibrated with 3 x 1 mL washing buffer (10 mM Tris–HCl, pH 7.6, 150 mM NaCl, 1 μM E64, 0.5 mM EDTA-free Ultra Complete tablet, 0.1 mg/mL AEBSF, and 1 μg/mL leupeptin) for 2 h at 4°C with gentle rotation. IgG-Sepharose beads were transferred to a 1-mL Mobicol column (MoBiTec GmbH, Göttingen, Germany) and washed with 5 × 1 mL washing buffer and 2 x 1 mL digestion buffer (50 mM Tris–HCl, pH 8.0). Then, the IgG-enriched proteins were digested on the beads with mass spectrometry-grade Trypsin/Lys-C Mix (Promega, Madison, WI, United States) at a 1:50 (enzyme/substrate) ratio for 18 h at 37°C. Additional trypsin at a 1:100 (enzyme/substrate) ratio was added for an extra 4 h at 37°C. The peptides were collected by gentle rotation (1000 rpm for 1 min at 4°C), and the beads were eluted twice with digestion buffer. All three fractions were pooled. One-sixth of the tryptic digestion was used for protein-level identification with liquid chromatography-tandem mass spectrometry (LC-MS/MS) analysis. The remainder of the tryptic digestion was incubated with 200 μL magnetic beads coupled to anti-C598SEIWDR antibodies for 2 h at 4°C with gentle rotation (1000 rpm). The tube was placed in the magnetic separator until the beads were captured on the magnet side, where after, the clear supernatant was removed. Collected beads were washed three times with cold washing buffer (10 mM Tris, 150 mM NaCl, pH 7.6). The enriched peptides were eluted by incubation with 400 μL of 0.2 M glycine buffer (pH 2.5) for 10 min with rotation (1000 rpm). The supernatant was collected on the separator and supplemented with 100 μL 1 M Tris–HCl buffer (pH 9.0) for neutralization. After desalting with OMIX C18 pipette tips (Agilent, Santa Clara, CA, United States), the peptide samples were eluted with 100 μL 75% (v/v) acetonitrile containing 0.1% (v/v) formic acid and dried by vacuum centrifugation. The dried peptide samples were subjected to LC-MS/MS.
LC-MS/MS
For the LC-MS/MS analyses, a Q Exactive Plus instrument was used (Thermo Fisher Scientific, Waltham, MA, United States) operated with an Easy-nLC1000 system (Thermo Fisher Scientific). Samples were reconstituted in 0.1% (v/v) formic acid, followed by centrifugation (16,000 × g for 10 min). The supernatants were pressure-loaded onto a 2-cm microcapillary precolumn packed with C18 (3 μm, 120 Å; SunChrom, Friedrichsdorf, Germany). The precolumn was connected to a 12-cm 150-μm-inner diameter microcapillary analytical column packed with C18 (1.9 μm, 120 Å; Dr. Maisch GmbH, Ammerbuch-Entringen, Germany) and equipped with a home-made electrospray emitter tip. The spray voltage was set to 2.0 kV and the heated capillary temperature to 320°C. The LC gradient A consisted of 0 min, 8% B; 14 min, 13% B; 51 min, 25% B; 68 min, 38% B; 69–75 min, 95% B [A, water; B, 80% (v/v) acetonitrile] at a flow rate of 600 nL/min. Higher-energy collisional dissociation (HCD) MS/MS spectra were recorded in the data-dependent mode with a Top20 method. The first MS spectra were measured with a resolution of 70,000, an AGC target of 3e6, a maximum injection time of 20 ms, and a mass range from m/z 300 to 1,400. The HCD MS/MS spectra were acquired with a resolution of 17,500, an AGC target of 1e6, a maximum injection time of 60 ms, a m/z 1.6 isolation window, and normalized collision energy of 30. The m/z of the peptide precursors that triggered MS/MS scans were dynamically excluded from further MS/MS scans for 18 s.
MS/MS Data Processing
RAW files were examined with pLink 2 algorithm version 2.3.5 (Chen et al., 2019) and converted to MGF files by the MSFileReader (Thermo Fisher Scientific). Spectra were searched against representative Araport11 proteins (27,655 entries, v1.10.4, release on 06/2016), supplemented with the YAP1C protein sequence. Notably, the last seven amino acids “RDWLESC” of AT1G74260, of which the reverse sequence is an isobaric “CSELWDR” peptide, were omitted, because they resulted in high-scoring decoy matches, reflecting the enriched YAP1C-derived “C598SEIWDR.” A precursor tolerance of 20 ppm and a fragment mass tolerance of 20 ppm (HCD spectra) were specified. A specific tryptic search was used with a maximum of two allowed missed cleavages. Variable modifications included methionine oxidation, cysteine carbamidomethylation, and protein N-terminal acetylation. No fixed modifications were set. Results were filtered at a false discovery rate (FDR) threshold of 1% at the spectrum, peptide, and protein levels.
Bioinformatics
Cross-Linked Peptide-to-Protein Assignment
Cross-linked peptides were extracted from pLink 2 cross-linked (CL) peptide reports (≤1% FDR). Due to trypsin miscleavages, redundant CL peptides were identified for approximately 10% of the YAP1C-CL protein sites. To remove this redundancy, CL peptides matching an identical protein site were grouped and the peptide matching the least proteins was chosen as representative, resulting in 1,748 YAP1C-CL protein sites (Supplementary Dataset S1).
Enrichment Analyses
The 570 proteins that uniquely matched CL peptides were analyzed for gene set enrichment in the Gene Ontology (GO) and the Kyoto Encyclopedia of Genes and Genomes (KEGG) pathways by means of the DAVID tool (Huang et al., 2009). In addition, the overrepresentation of Cys-SOHs was determined in protein domains (PROSITE profiles) (Sigrist et al., 2013) as described previously (Huang et al., 2019).
C598SEIWDR Cross-Link Peptide Fingerprint Scanning
Peak list (MGF) files generated by pLink 2 (Chen et al., 2019) were parsed by an in-house script to detect the occurrence of the 10 characteristic fragment ions. Per MS/MS spectrum, the numbers of peaks with a matching m/z value (≤0.01 Da) were counted, irrespective of their intensity. MS/MS spectra containing the full fingerprint or a high number of characteristic fragment ions hint at the fragmentation of C598SEIWDR CL peptides, although a CL search algorithm remains necessary to uncover their identity.
Data Availability
Thermo RAW files and pLink 2 result tables are available on the PRIDE repository with the identifier PXD016723. The 1,747 sulfenylated cysteine residues identified were submitted to the Plant PTM Viewer (Willems et al., 2019).
Results and Discussion
Identification of YAP1C-Resolved S-Sulfenylated Sites Through a Protein- and Peptide-Level Purification Strategy
Previously, we had identified S-sulfenylated Arabidopsis proteins in vivo by means of a transgenically expressed YAP1C probe fused to an affinity purification tag (Waszczak et al., 2014; De Smet et al., 2019). Crucial steps in this strategy were the nucleophilic attack and the subsequent formation of a mixed disulfide bond by Cys598 of YAP1C and the sulfenylated Cys (Cys-SOH) residue in oxidized target proteins (Delaunay et al., 2002; Takanishi et al., 2007). In these approaches, the mixed disulfide YAP1C complexes were purified in a sequential affinity purification strategy, firstly by IgG-Sepharose beads trapping the IgG-binding domain of protein G (ProtG) and, subsequently, after cleavage with protease, by Streptavidin-Sepharose beads targeting the streptavidin-binding peptides (SBP). As such, approximately 230 in vivo S-sulfenylated protein targets had been detected by MS (Waszczak et al., 2014; De Smet et al., 2019). However, information on the identity of the S-sulfenylated sites remained unknown in the proteins harboring more than one cysteine. An average of 7.85 Cys residues per Arabidopsis protein (309,122 Cys residues in 39,364 proteins, The UniProt Consortium, 2019) implied that for most S-sulfenylation proteins downstream validation experiments are required, including mutational approaches and/or in vitro protein studies. We reasoned that, at least under non-reducing conditions, trypsin cleavage would result in disulfide-bound peptides between the nucleophilic YAP1C Cys598 and the sulfenylated cysteine in the target proteins. Hence, these mixed peptides entail the necessary information on the sulfenylated cysteines. Theoretically, trypsin cleavage around the disulfide bond generates a mixed peptide involving the 7-amino-acid peptide from the YAP1C probe “C598SEIWDR” that contains the redox-active Cys598, and the sulfenylated cysteine-containing peptide from the YAP1C-bound protein (Figure 1). Typically, CL peptides represent solely a minor fraction in complex peptide mixtures that are dominated by tryptic linear peptides. Therefore, enrichment procedures are performed in chemical cross-link proteomics (Barysz and Malmström, 2018). Similarly, we devised an enrichment strategy for the C598SEIWDR CL peptides by generating polyclonal antibodies directed toward the C598SEIWDR peptide (see Materials and Methods). Afterward, we tested four workflows for the detection of C598SEIWDR CL peptides by pLink 2 (Chen et al., 2019; Figure 1). Proteins were extracted from YAP1C cells treated with 20 mM H2O2 for 30 min. Note that for all tested workflows, equal fractions of proteins were used for LC-MS/MS (0.5 mg). Firstly, a trypsin-digested proteome was submitted for MS (shotgun proteomics), resulting in the identification of 5,917 linear (regular) peptides that provided a general proteome reference (Figure 1, sample A). However, no C598SEIWDR CL peptides were found, highlighting the need for YAP1C enrichment strategies. Hence, we subjected the digested proteome to an anti-C598SEIWDR enrichment (Figure 1, sample B). However, such direct peptide-level enrichment didn’t result in C598SEIWDR CL peptide identifications. To reduce the peptide complexity prior to the anti-C598SEIWDR enrichment, the proteome was first enriched on the IgG-Sepharose beads and, second, after an on-beads trypsin digestion, the eluted sample was subjected to an anti-C598SEIWDR affinity purification and analyzed by MS (Figure 1, sample C). This double enrichment strategy proved highly successful, because 475 C598SEIWDR CL peptides were identified (≤1% FDR; Supplementary Dataset S1). Lastly, we tested the CL peptide identifications of the eluted sample only after IgG-Sepharose enrichment (Figure 1, sample D). After protein-level enrichment, solely one single CL peptide, “C#SEIWDR-VIEYC#K” (with C#, indicating a CL cysteine), was identified that matched the S PHASE KINASE-ASSOCIATED PROTEIN (SPK)-like proteins. Hence, a dedicated cross-link enrichment step is required after the IgG-Sepharose enrichment for large-scale identification of YAP1C CL peptides. Moreover, the 6,721 identified linear peptides provide complementary information on the YAP1C protein interactors. Taken together, analysis of YAP1C protein interactors, after IgG-Sepharose enrichment, followed by the identification of YAP1C CL sites, after an additional anti-C598SEIWDR enrichment step, enables the proteome-wide detection of sulfenylated cysteines.
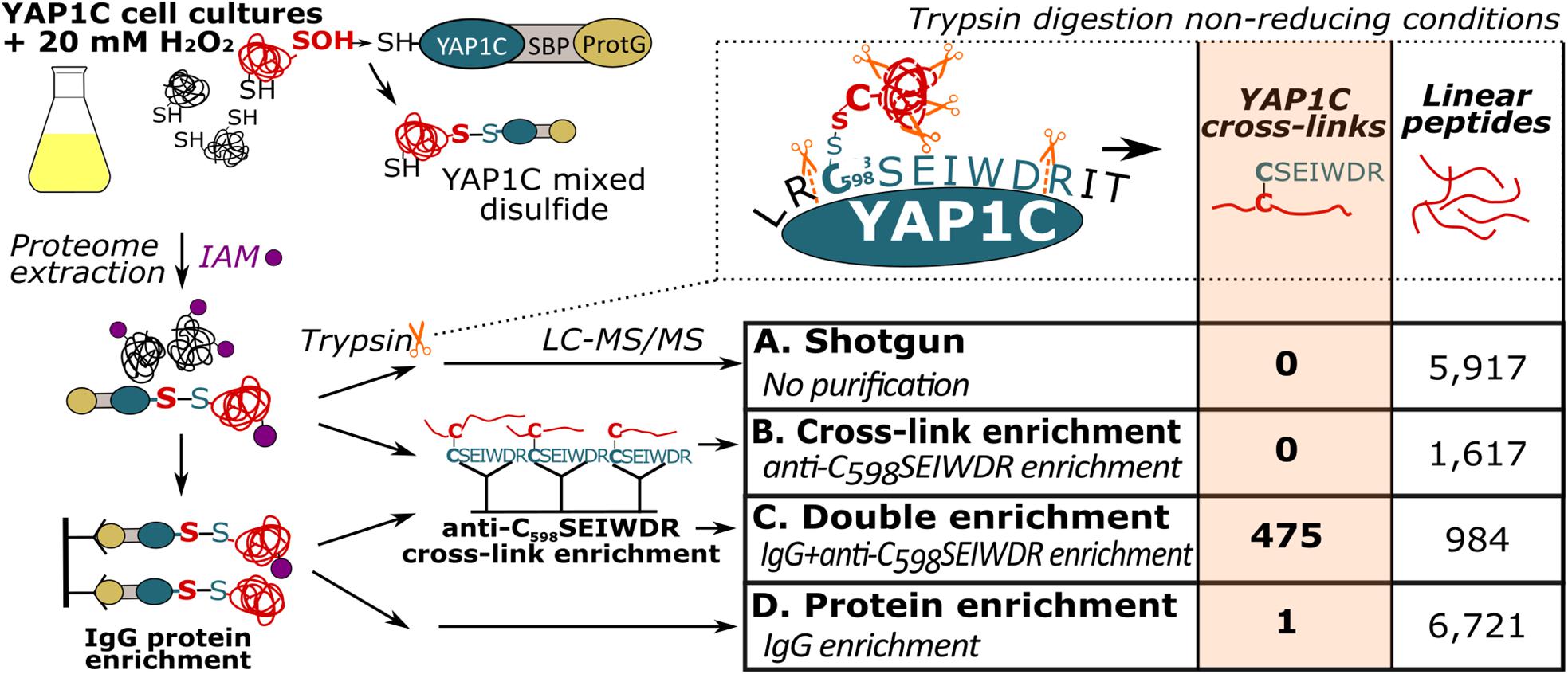
Figure 1. Enrichment strategies for YAP1C cross-linked (CL) peptide identification. Trypsin digestion under non-reducing conditions results in YAP1C C598SEIWDR CL peptides containing the redox-active Cys598. Next to a non-enriched proteomic shotgun (sample A), CL peptide enrichment was tested with anti-C598SEIWDR polyclonal antibodies (sample B). In addition, YAP1C-interacting protein complexes were purified on IgG-Sepharose beads, followed by an on-bead trypsin digestion (sample D). The obtained peptides were further enriched for C598SEIWDR CL peptides on beads coupled to the anti-C598SEIWDR antibody (sample C). IAM, iodoacetamide, indicated by purple dots; LC-MS/MS, liquid chromatography-tandem mass spectrometry; ProtG, two IgG-binding domains of protein G; SBP, streptavidin-binding peptide.
Identification With YAP1C of Sulfenylated Cysteines Under H2O2 Stress
To identify sulfenylated cysteines in untreated and treated (20 mM H2O2 for 30 min) YAP1C cells (three replicates per condition), we analyzed YAP1C protein complexes after IgG-Sepharose enrichment and their YAP1C CL sites after an additional anti-C598SEIWDR enrichment step. In total, 1,930 C598SEIWDR CL peptides were detected (7,040 peptide-to-spectrum matches (PSMs); Supplementary Dataset S2). Due to trypsin missed cleavages, some CL peptides specified the same sulfenylated cysteine. For instance, “C#ATITPDEGR-C#SEIWDR” and “C#ATITPDEGRVTEFGLK-C#SEIWDR,” both indicate S-sulfenylation of Cys75 in CYTOSOLIC NADP+-DEPENDENT ISOCITRATE DEHYDROGENASE. Removal of this redundancy (see Materials and Methods) resulted in a total of 1,747 non-redundant C598SEIWDR CL peptides (Supplementary Dataset S2). Of these 7,040 PSMs, 25 (0.36%) are indicative of inter-YAP1C cross-links between the redox-active Cys598, suggesting that a minor artifactual self-trapping of YAP1C is possible. In favor of high-confident identifications, we retained 1,132 out of the 1,747 C598SEIWDR CL peptides with at least two PSMs across the six samples (Supplementary Dataset S2). We identified more C598SEIWDR CL peptides in untreated samples (1,082 CL peptides) than in the H2O2-treated cells (759 CL peptides). The YAP1C probe is continuously overexpressed and trapping of S-sulfenylated cysteines starts before the addition of 20 mM H2O2. This oxidative stress treatment might result in the overoxidation of certain cysteines, leading to the formation of sulfinic and sulfonic acids, which cannot be trapped by YAP1C. Nevertheless, the majority of the C598SEIWDR CL peptides [709 out of 1,132 (63%)] were identified under both conditions and 50 CL peptides were exclusively identified after H2O2 stress (Figure 2A). From the 1,132 C598SEIWDR CL peptides, 307 could not be attributed unambiguously to a unique Arabidopsis protein, meaning that the YAP1C CL Arabidopsis peptides are present in at least two different proteins. The remaining 825 CL peptides matched uniquely to 570 different proteins, implying that some Arabidopsis proteins contain multiple S-sulfenylated sites. For 94% of the 570 YAP1C CL proteins, at least one peptide was identified after IgG-Sepharose enrichment, thus complementarily confirmed as a YAP1C-interacting protein. From the 307 CL peptides that match multiple Arabidopsis proteins, protein-level evidence was exclusively available for solely one of the possible matching proteins, for instance, the CL peptide “KLKEC#EK-C#SEIWDR” represents a C598SEIWDR CL to either Cys124 in PROTEIN PHOSPHATASE5 (PP5, AT2G42810) or Cys487 in PROTON PUMP INTERACTOR2 (PPI2, AT3G15340) (Supplementary Dataset S2). Prior to the anti-C598SEIWDR purification step, 23 linear peptides (194 PSMs) were identified for PP5, whereas none for PPI2. As such, besides the complementary evidence of the YAP1C-interacting proteins, the MS analysis of the IgG-Sepharose-enriched samples are helpful for protein identification in case of ambiguous peptide-to-protein matching.
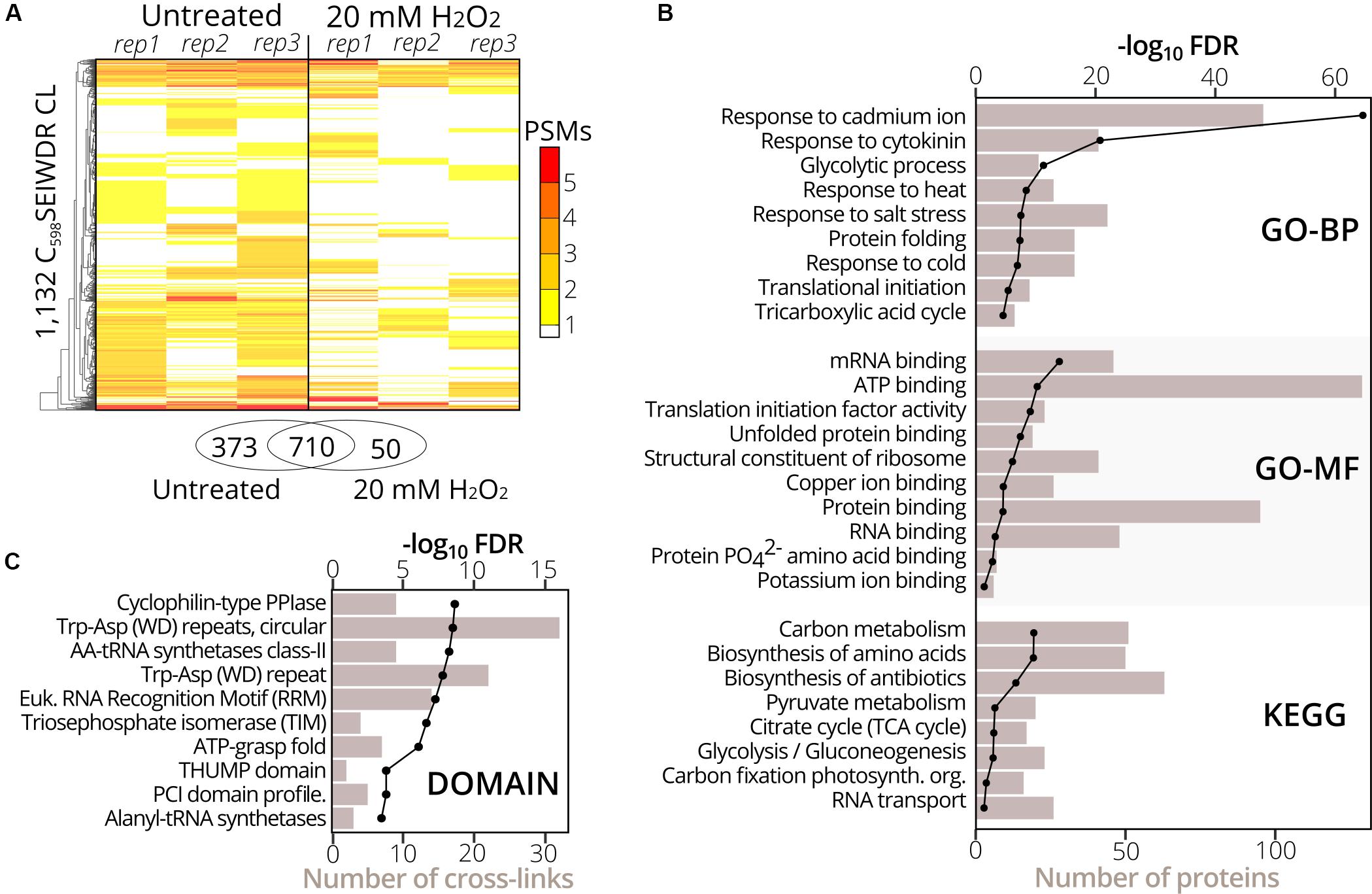
Figure 2. Gene set and protein domain enrichment of YAP1C C598SEIWDR CL proteins and sites. (A) Heatmap of the 1,132 C598SEIWDR CL peptides (FDR ≤ 1%; ≥2 PSMs) showing the number of PSMs per replicate in cells untreated (left) and treated with H2O2 for 30 min (right). The overlap of C598SEIWDR CL peptides between conditions is shown in a Venn diagram. (B) Gene set enrichment of the 570 proteins matched uniquely by the C598SEIWDR CL peptides with the DAVID tool (Huang et al., 2009). The assessed gene sets were KEGG pathways, gene ontology (GO) terms for biological process (GO-BP), molecular function (GO-MF), and cellular component (GO-CC, not plotted). All results are presented in Supplementary Dataset S3A. (C) Enrichment of 1,132 sulfenylated cysteines in PROSITE profiles (see section “Materials and Methods”). Enrichment was plotted as a black line (-log10 FDR, top x-axis) and the number of CL sites as a bar chart (bottom x-axis). All results are reported in Supplementary Dataset S3B.
To functionally categorize the identified S-sulfenylated proteins, we carried out a gene set enrichment analysis on the 570 proteins for which 825 sulfenylated cysteines had unambiguously been identified by C598SEIWDR CL peptides. In accordance with the cytosolic localization of the YAP1C probe, the strongest overrepresented GO term was the cellular component “cytosol” (FDR 2.3e–202; Supplementary Dataset S3A). In addition, proteins of several stress-related adaptive processes (Cd+, salt, heat, and cold), metabolism, and RNA processes were strongly enriched (Figure 2B), corresponding with our previous reports that demonstrated that enzymes involved in core carbon metabolic pathways, such as glycolysis, amino acid metabolism, and carbon fixation, were prone to S-sulfenylation (Huang et al., 2018, 2019). Also, the GO-MF term “mRNA-binding” and the KEGG pathway “RNA transport” were overrepresented (FDR 3.6e–16 and 0.04, respectively) (Figure 2B). Sulfenylated cysteines were overrepresented in various protein domains (Figure 2C). For instance, in the Arabidopsis proteome, the amino acyl-transfer RNA (AA-tRNA) synthetase domain is present in seven proteins and contains 96 cysteines in total, of which nine found within the AA-tRNA synthetase domain were detected as sulfenylated and were overrepresented (FDR 5.9e–9; Supplementary Dataset S3B). In addition, the RNA-binding RNA-recognition motif (RRM) was overrepresented (FDR 5.5e–8) that had previously been reported as a S-sulfenylation hotspot (Huang et al., 2019). Taken together, functional enrichment of S-sulfenylated proteins and cysteines identified by C598SEIWDR CL peptides match previous observations by other tools.
YAP1C Cross-Linked Peptides Are Associated With Characteristic Disulfide Ions
In a next phase, we used the 7,000 PSMs of C598SEIWDR CL peptides to characterize in detail their general properties and MS/MS fragmentation patterns. Firstly, we compared the properties of C598SEIWDR CL peptides to the linear peptides identified prior to the anti-C598SEIWDR purification. As CL peptides are the combination of two peptides with charged N-termini and tryptic C-termini ending on Arg/Lys, CL peptides typically have both a higher mass and precursor charge than the linear peptides. In accordance, the peptide mass of C598SEIWDR (907 Da) approximates the median peptide mass difference of identified C598SEIWDR CL peptides with linear peptides (Figure 3A, 892 Da). In addition, precursors of the C598SEIWDR CL peptides are more positively charged than the linear peptides, with approximately 93% of the PSMs charged ≥3+ (Figure 3B). As such, both peptide mass and charge are in line with typical CL peptide properties. Importantly, the CL peptide identification is more challenging than that of linear peptides, because the fragmentation of CL peptides results in intermixed fragment ions derived from both peptides. Noteworthy, the CL peptide search with pLink 2 was not biased toward C598SEIWDR CL and 27 intra-protein and 20 inter-protein (non-YAP1C) CL peptides were identified (102 PSMs, FDR ≤ 1%) (Supplementary Dataset S4). Hence, despite the search for CL peptides between or within 26,000 Arabidopsis proteins, the YAP1C C598SEIWDR CL peptides are by far preponderant (7,040 C598SEIWDR CL PSMs versus 102 non-YAP1C PSMs), and emphasize the effectiveness and necessity of the anti-C598SEIWDR purification. Moreover, Cys598 of YAP1C was also CL to Arabidopsis peptides via the trypsin-missed cleaved peptides “EGSLLRC#SEIWDR” and “C#SEIWDRITTHPK.” For instance, the peptide “HMIEDDC#TDNGIPLPNVTSK” of the E3 ubiquitin ligase SKP-like protein 1B (SKP1B; AT5G42190) was CL to both “C#SEIWDR” (Figure 3C) and “C#SEIWDRITTHPK” (Supplementary Dataset S2). This cytosolic protein had been identified previously to be S-sulfenylated by the original protein-level YAP1C-TAP strategy (Waszczak et al., 2014).
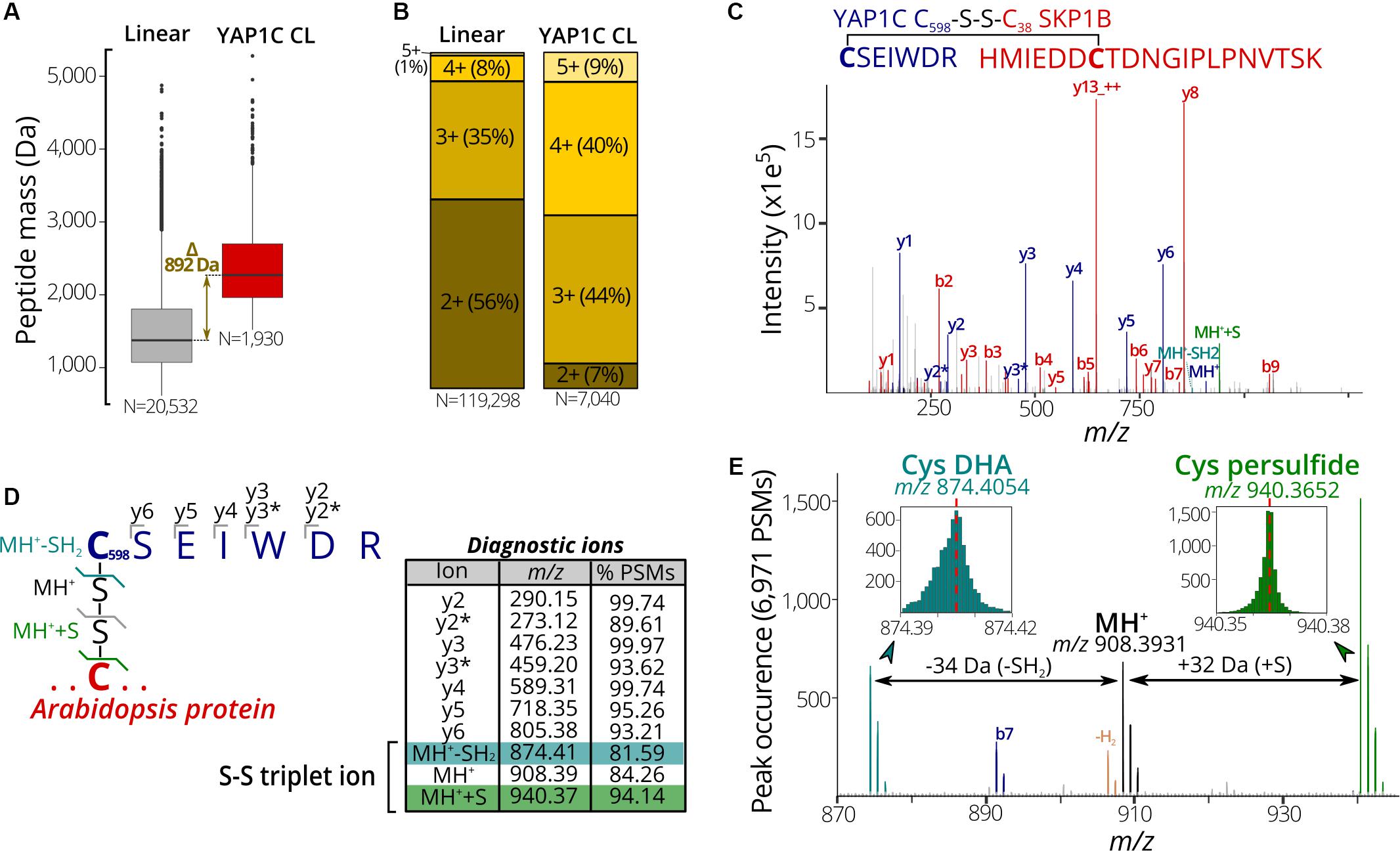
Figure 3. Characteristic properties and fragmentation ions of C598SEIWDR CL peptides. (A) Mass distribution for non-redundant linear (left) and C598SEIWDR CL peptides (right). A 892-Da difference is indicated between the median peptide masses of both distributions. (B) Peptide precursor charge distribution for PSMs to linear and C598SEIWDR CL peptides. (C) Annotated spectrum of C#SEIWDR CL (blue fragment ions) to HMIEDDC#TDNGIPLPNVTSK (red fragment ions). (D) Parsing of 6,971 PSMs of the YAP1C cross-links (FDR ≤ 1%) containing at least five C598SEIWDR b, y, or precursor ions (within m/z 0.01) for diagnostic ions. To this end, the occurrence of a peak was counted at a m/z 0.001 interval (irrespective of its intensity) to identify consistent fragment ions. Ten characteristic C598SEIWDR CL peptide ions were displayed in the peptide fragmentation scheme and table, with y2* and y3* indicating an ammonia neutral loss (-NH3). A triplet ion resulting from disulfide fragmentation patterns is highlighted. (E) Occurrence of triplet ion peaks characteristic of disulfide cleavage in 6,971 PSMs of C598SEIWDR CL peptides (FDR ≤ 1%, ≥5 fragment ions). The expected C598SEIWDR precursor mass is indicated in black (m/z 908.3931) and is flanked by Cys dehydroalanine (DHA, blue; m/z 874.4054) and Cys persulfide (green; m/z 970.3652). Brown peaks correspond to the MH+ precursor minus two hydrogens corresponding to an intact disulfide bond. MH+, C598SEIWDR single-charged peptide precursor; MH++S, Cys persulfide precursor; MH+-SH2, Cys DHA precursor.
Next, we aimed to identify characteristic fragment ions of C598SEIWDR CL peptides. Such characteristic ions could help in the assessment of the PSM quality or future identification of C598SEIWDR CL peptides. To detect consistent fragment ions associated with C598SEIWDR CL peptide fragmentation, we counted the occurrence of MS/MS m/z peaks in 6,971 PSMs (98.8% of 7,040 total C598SEIWDR CL PSMs), containing at least five b, y, or precursor ions of C598SEIWDR. The C598SEIWDR fragment y ions are well represented, occurring in 93% to 99% of the cases (Figure 3D), as well as y2 and y3 ions with neutral loss of NH3 (Figure 3D, y2∗ and y3∗, respectively). Interestingly, besides the C598SEIWDR precursor ion (MH+, m/z 908.39), neighboring masses corresponding to the precursor ion with cysteine persulfide formation (blue; +S, m/z 940.37) and a cysteine-to-dehydroalanine conversion (green; -SH2, m/z 874.41) are consistently present in C598SEIWDR CL PSMs (Figures 3D,E). This distinctive pattern of triplet ions is characteristic for inter-protein disulfides (Janecki and Nemeth, 2011) and used, for instance, by dedicated disulfide CL identification algorithms, such as DBond (Na et al., 2015) and MS2DB+ (Murad et al., 2011). Together with the C598SEIWDR y fragment ions, these precursor triplet ions form a distinctive C598SEIWDR fragment ion fingerprint. For example, 4,220 PSMs (59.9%) of the identified C598SEIWDR CL PSMs contain all 10 of these characteristic ions, whereas 6,503 (92.4%) and 5,847 PSMs (83.1%) had eight and nine out of 10 ions, respectively. As such, these characteristic ions can help in PSM quality assessment of C598SEIWDR CL peptides, with, for instance, all characteristic ions present in the “HMIEDDC#TDNGIPLPNVTSK-C#SEIWDR” PSM (Figure 3C). In addition, we used the characteristic fragment ions (Figure 3D) as a fingerprint to scan potential C598SEIWDR CL peptides in the raw proteomics data obtained after IgG-Sepharose and/or anti-C598SEIWDR enrichment strategies (Figure 1). After IgG-Sepharose enrichment, 33 MS/MS spectra contained the full C598SEIWDR CL fingerprint (Supplementary Dataset S5), indicating that more than a single C598SEIWDR CL might be fragmented, but not identified in the pLink 2 search (FDR ≤ 1%; Supplementary Dataset S1). Missing identifications can arise due to numerous reasons, such as the Arabidopsis peptide CL to C598SEIWDR being shorter than six amino acids (default pLink 2 search settings), noisy spectra with low-abundant fragment ions, or too stringent FDR scoring. In line with the high number of C598SEIWDR CL peptides identified by pLink 2 (475 peptides; Supplementary Dataset S1), 1,502 MS/MS spectra contained the full C598SEIWDR CL fingerprint after the additional anti-C598SEIWDR enrichment step (Supplementary Dataset S5). In contrast, no spectra with a full C598SEIWDR CL fingerprint were found in the proteome shotgun analysis or after direct anti-C598SEIWDR enrichment (Figure 1, samples A and B, respectively), indicating a high specificity for the fingerprint toward C598SEIWDR CL peptides. As such, the proposed 10 characteristic ions provide a useful and distinctive fingerprint for C598SEIWDR CL peptides for quality assessment of individual spectral matches or raw proteomics data.
YAP1C Cross-Linked Cysteines Report Protein Redox-Sensitive Cysteine Sites
We assessed whether the identified C598SEIWDR CL peptides (Supplementary Dataset S2) are in agreement with related redox studies. First, in 67 out of the 97 S-sulfenylated proteins (69%) identified previously as YAP1C interactors (Waszczak et al., 2014), 102 S-sulfenylated sites were found, including, for instance, Cys20 of DEHYDROASCORBATE REDUCTASE 2 (DHAR2) (Figure 4A) that had been shown to be S-glutathionylated via an S-sulfenylation intermediary by 5,5-dimethyl-1,3-cyclohexadione (dimedone) labeling and MS identification of recombinantly produced DHAR2 (Waszczak et al., 2014; Bodra et al., 2017). This laborious approach to identify the trapped S-sulfenylation sites can be avoided thanks to the identification of C598SEIWDR CL peptides, as shown here for DHAR2. In AtDHAR2, the sulfenylation of the active site cysteine is part of the ping-pong mechanistic cycle, which we described previously (Bodra et al., 2017). During the reduction of dehydroascorbate (DHA) to ascorbate (AsA), the active site thiol gets sulfenylated and becomes more electrophilic for the next step of the catalytic mechanism, being the formation of a mixed-disulfide with glutathione. Also, here, the micro-environment of this specific cysteine determines its reactivity with both DHA and GSH. Besides Cys20 of DHAR2, Cys6 located at the protein N-terminus was identified as YAP1C CL site as well (Supplementary Dataset S2 and Figure 4B; Bodra et al., 2017). Furthermore, we highlighted the residues (96 out of 213, 45%) covered by 8 linear peptides (52 PSMs, Supplementary Dataset S2) identified after YAP1C protein-level enrichment and giving strong complementary evidence. Taken together, this procedure will help hypothetically formulate the mode-of-action of potential redox switches and fast-forward downstream experiments.
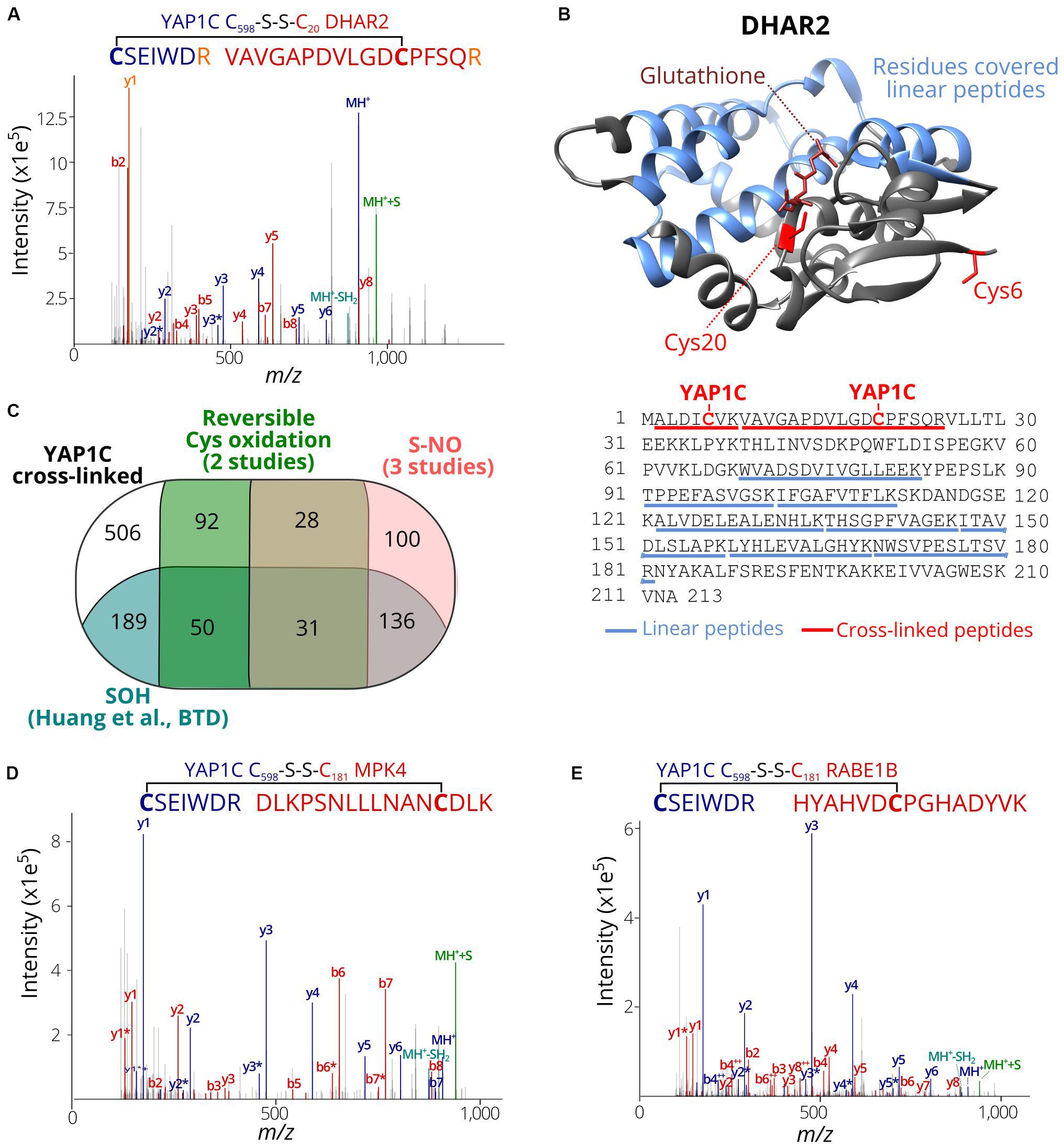
Figure 4. S-sulfenylated sites identified by C598SEIWDR CL peptides corresponding to previously identified redox-sensitive cysteines. (A) Annotated MS/MS spectrum of C#SEIWDR (blue) linked to VAVGAPDVLGDC#PFSQR (red, Cys20 of DHAR2). The y1 fragment ion (corresponding to the C-terminal Arg) (orange) is shared by both peptides. (B) DHAR2 structure (PDB ID: 5LOL) (Bodra et al., 2017) is shown. YAP1C disulfide CL Cys6 and Cys20 are highlighted in red sticks, glutathione in the active site in brown, and the 96 residues covered by linear peptides in blue. The structure was generated using Chimera version 1.12 (Pettersen et al., 2004). (C) Venn diagram displaying the overlap of 1,132 S-sulfenylated sites (≥ 2PSMs) identified in this study (black) with previously reported Arabidopsis S-sulfenylated sites (blue) (Huang et al., 2019), S-nitrosylated sites (pink) (Fares et al., 2011; Puyaubert et al., 2014; Hu et al., 2015) and reversibly oxidized cysteine sites (green) (Liu et al., 2014, 2015). (D) Annotated MS/MS spectrum of C#SEIWDR (blue) linked to DLKPSNLLLNANC#DLK (red; Cys181 of MPK 4). (E) Annotated MS/MS spectrum of C#SEIWDR (blue) linked to VAVGAPDVLGDCPFSQR (red). For all annotated MS/MS spectra, the C598SEIWDR fragment ions are colored in dark blue. Persulfide and cysteine-to-dehydroalanine fragment ions are in green and light blue, respectively. Fragment ions of Arabidopsis peptides linked with sulfenylated cysteines are in red.
Then, the S-sulfenylated cysteines determined by C598SEIWDR CL peptides were compared to redox-sensitive Arabidopsis cysteines reported previously. Recently, we have identified more than 1,500 sulfenylated Cys residues in Arabidopsis cells by means of a chemoselective benzothiazindioxide (BTD)-based carbon nucleophile (Huang et al., 2019). Comparison of the 1,132 YAP1C CL peptides (Supplementary Dataset S2; ≥2 PSMs) revealed 406 sites (36%) that overlapped with BTD-targeted Cys (Figure 4C). For instance, the C598SEIWDR CL peptides “C#SEIWDR-DLKPSNLLLNANC#DLK” (Figure 4D) matched the Cys181 of MITOGEN-ACTIVATED PROTEIN KINASE 4 (MPK4) that had been experimentally verified (Huang et al., 2019). Also, other site-specific reversibly oxidized cysteine studies (Liu et al., 2014, 2015) and S-nitrosylation studies (Fares et al., 2011; Puyaubert et al., 2014; Hu et al., 2015) were compared (Willems et al., 2019). In total, 295 S-nitrosylation and 201 reversible previously reported cysteine oxidation sites overlapped with the YAP1C CL sites (Figure 4C). Taken together, 626 out of the 1,132 sulfenylated cysteines identified here by C598SEIWDR CL peptides (55.3%) had already been reported as redox sensitive in independent studies, of which some had been confirmed biochemically. For instance, “C#SEIWDR-LRGLIAEKNC#APIMVR” matched Cys32 of ascorbate peroxidase 1 (APX1), an important ROS-scavenging enzyme, that has been identified previously as S-sulfenylated (Huang et al., 2019) and S-nitrosylated (Fares et al., 2011; Liu et al., 2014). Nitrosylation of Cys32 had been confirmed to increase its H2O2-scavenging enzymatic activity (Yang H. et al., 2015). Moreover, another C598SEIWDR CL Cys49 of APX1 was also found as S-nitrosylated (Hu et al., 2015) and S-sulfenylated (Huang et al., 2019). In addition, “C#SEIWDR-HYAHVDC#PGHADYVK” matched Cys149 of the chloroplastic elongation factor Tu (EF-Tu) RAB GTPASE HOMOLOG E1B (RABE1B) (Figure 4E), a site identified previously as S-sulfenylated (Huang et al., 2019) and S-nitrosylated (Hu et al., 2015). Interestingly, sulfenylation of the corresponding cysteine site (Cys82; Supplementary Figure S1) in the EF-Tu ortholog of Cyanobacterium synechocystis, a popular model organism for studies of photosynthesis as chloroplast ancestor, inactivates EF-Tu in a reversible manner (Puyaubert et al., 2014; Yutthanasirikul et al., 2016). Taken together with the approximately 3.0-fold increased S-sulfenylation under H2O2 treatment (Huang et al., 2019), the plastidial EF-Tu protein probably exhibits a conserved redox sensitivity in Arabidopsis. All in all, the high agreement of the S-sulfenylated sites identified by C598SEIWDR CL peptides with other studies of redox-sensitive cysteines demonstrates that the YAP1C-resolved cysteines are in general highly susceptible to oxidative redox modifications. Identification of these redox-sensitive sites will greatly facilitate the possible formulation and our understanding of the redox signaling processes.
Conclusion
Here, we report an innovative approach for in situ identification of S-sulfenylation sites by means of the transgenic probe YAP1C. By IgG purification at the protein level, followed by anti-C598SEIWDR purification at the peptide level, large-scale capture and identification of YAP1C CL peptides are possible, thereby uncovering in vivo S-sulfenylated protein sites. Importantly, this method can detect sulfenylated cysteines in a noninvasive manner and might easily be adapted to detect sulfenylated cysteines in specific organelles (De Smet et al., 2019). The proposed genetically based methodology holds great promise for in planta mining of S-sulfenylated sites, in which rigid plant tissues limit the penetration and use of chemoselective probes. All in all, thanks to this noninvasive approach based on the YAP1C probe, the site-specific identification of protein S-sulfenylation was successfully shown for Arabidopsis cells under H2O2 stress. Importantly, this method can be implemented for any species that can be genetically modified.
Data Availability Statement
The datasets generated for this study can be found in the PRIDE repository with the identifier–PXD016723, https://www.ebi.ac.uk/pride/profile/reviewer06458, Username: cmV2aWV3ZXIwNjQ1OEBlYmkuYWMudWs=, Password: sFGF4Srq, For data in Plant PTM Viewer, the linking is https://www.psb.ugent.be/webtools/ptm-viewer/experiment.php?id=280&key=731ECC4C.
Author Contributions
BW, PW, JH, JM, and FV conceived the research and wrote the manuscript. BW, CT, and JY conducted the experiments. BW and PW analyzed the data. All authors contributed to the article and approved the submitted version.
Funding
This work was supported by the Research Foundation-Flanders, Fonds de la Recherche Scientifique (Excellence of Science project no. 30829584 to Claire Remacle, Kate Carroll, Didier Vertommen, JM and FV), the Research Foundation-Flanders (grants G0D7914N to JM and FV, 1508316N to JM, G003809N to Kris Gevaert and FV, G055416N and G06916N to FV), the China Scholarship Council (CSC no. 201606690036 to BW), the National Natural Science Foundation of China (no. 21922702 to JY), and the Ghent University Special Research Fund (BOF Grant 01J11311 to FV). JH is indebted to the Research Foundation-Flanders for a postdoctoral fellowship (no. 1227020N).
Conflict of Interest
The authors declare that the research was conducted in the absence of any commercial or financial relationships that could be construed as a potential conflict of interest.
Acknowledgments
The authors thank Dr. Didier Vertommen and Dr. Barbara De Smet for helpful discussions, Dr. Lieven Sterck for submitting data to the Plant PTM Viewer, and Dr. Martine De Cock for excellent help in preparing the manuscript.
Supplementary Material
The Supplementary Material for this article can be found online at: https://www.frontiersin.org/articles/10.3389/fpls.2020.00777/full#supplementary-material
Footnotes
References
Akter, S., Fu, L., Jung, Y., Lo Conte, M., Lawson, J. R., Lowther, W. T., et al. (2018). Chemical proteomics reveals new targets of cysteine sulfinic acid reductase. Nat. Chem. Biol. 14, 995–1004. doi: 10.1038/s41589-018-0116-2
Akter, S., Huang, J., Bodra, N., De Smet, B., Wahni, K., Rombaut, D., et al. (2015a). DYn-2 based identification of Arabidopsis sulfenomes. Mol. Cell. Proteomics 14, 1183–1200. doi: 10.1074/mcp.M114.046896
Akter, S., Huang, J., Waszczak, C., Jacques, S., Gevaert, K., Van Breusegem, F., et al. (2015b). Cysteines under ROS attack in plants: a proteomics view. J. Exp. Bot. 66, 2935–2944. doi: 10.1093/jxb/erv044
Backus, K. M. (2019). Applications of reactive cysteine profiling. Curr. Top. Microbiol. Immunol. 420, 375–417. doi: 10.1007/82.2018.120
Barysz, H. M., and Malmström, J. (2018). Development of large-scale cross-linking mass spectrometry. Mol. Cell. Proteomics 17, 1055–1066. doi: 10.1074/mcp.R116.061663
Biteau, B., Labarre, J., and Toledano, M. B. (2003). ATP-dependent reduction of cysteine-sulphinic acid by S. cerevisiae sulphiredoxin. Nature 425, 980–984. doi: 10.1038/nature02075
Bodra, N., Young, D., Astolfi Rosado, L., Pallo, A., Wahni, K., De Proft, F., et al. (2017). Arabidopsis thaliana dehydroascorbate reductase 2: conformational flexibility during catalysis. Sci. Rep. 7:42494. doi: 10.1038/srep42494
Chen, Z.-L., Meng, J.-M., Cao, Y., Yin, J.-L., Fang, R.-Q., Fan, S.-B., et al. (2019). A high-speed search engine pLink 2 with systematic evaluation for proteome-scale identification of cross-linked peptides. Nat. Commun. 10:3404. doi: 10.1038/s41467-019-11337-z
De Smet, B., Willems, P., Fernandez-Fernandez, A. D., Alseekh, S., Fernie, A. R., Messens, J., et al. (2019). In vivo detection of protein cysteine sulfenylation in plastids. Plant J. 97, 765–778. doi: 10.1111/tpj.14146
Delaunay, A., Pflieger, D., Barrault, M.-B., Vinh, J., and Toledano, M. B. (2002). A thiol peroxidase is an H2O2 receptor and redox-transducer in gene activation. Cell 111, 471–481. doi: 10.1016/s0092-8674(02)01048-6
Fares, A., Rossignol, M., and Peltier, J.-B. (2011). Proteomics investigation of endogenous S-nitrosylation in Arabidopsis. Biochem. Biophys. Res. Commun. 416, 331–336. doi: 10.1016/j.bbrc.2011.11.036
Gupta, V., and Carroll, K. S. (2014). Sulfenic acid chemistry, detection and cellular lifetime. Biochim. Biophys. Acta 1840, 847–875. doi: 10.1016/j.bbagen.2013.05.040
Gupta, V., Yang, J., Liebler, D. C., and Carroll, K. S. (2017). Diverse redoxome reactivity profiles of carbon nucleophiles. J. Am. Chem. Soc. 139, 5588–5595. doi: 10.1021/jacs.7b01791
Gurrieri, L., Distefano, L., Pirone, C., Horrer, D., Seung, D., Zaffagnini, M., et al. (2019). The thioredoxin-regulated α-amylase 3 of Arabidopsis thaliana is a target of glutathionylation. Front. Plant Sci. 10:993. doi: 10.3389/fpls.2019.00993
Hamperl, S., Brown, C. R., Perez-Fernandez, J., Huber, K., Wittner, M., Babl, V., et al. (2014). “Purification of specific chromatin domains from single-copy gene loci in Saccharomyces cerevisiae,” in Functional Analysis of DNA and Chromatin (Methods in Molecular Biology, 1094), eds J. C. Stockert, J. Espada, and A. Blázquez-Castro (Totowa, NJ: Humana Press), 329–341. doi: 10.1007/978-1-62703-706-8_26
Hu, J., Huang, X., Chen, L., Sun, X., Lu, C., Zhang, L., et al. (2015). Site-specific nitrosoproteomic identification of endogenously S-nitrosylated proteins in Arabidopsis. Plant Physiol. 167, 1731–1746. doi: 10.1104/pp.15.00026
Huang, D. W., Sherman, B. T., and Lempicki, R. A. (2009). Systematic and integrative analysis of large gene lists using DAVID bioinformatics resources. Nat. Protoc. 4, 44–57. doi: 10.1038/nprot.2008.211
Huang, J., Willems, P., Van Breusegem, F., and Messens, J. (2018). Pathways crossing mammalian and plant sulfenomic landscapes. Free Radic. Biol. Med. 122, 193–201. doi: 10.1016/j.freeradbiomed.2018.02.012
Huang, J., Willems, P., Wei, B., Tian, C., Ferreira, R. B., Bodra, N., et al. (2019). Mining for protein S-sulfenylation in Arabidopsis uncovers redox-sensitive sites. Proc. Natl. Acad. Sci. U.S.A. 116, 21256–21261. doi: 10.1073/pnas.1906768116
Jacques, S., Ghesquière, B., De Bock, P.-J., Demol, H., Wahni, K., Willems, P., et al. (2015). Protein methionine sulfoxide dynamics in Arabidopsis thaliana under oxidative stress. Mol. Cell. Proteomics 14, 1217–1229. doi: 10.1074/mcp.M114.043729
Janecki, D. J., and Nemeth, J. F. (2011). Application of MALDI TOF/TOF mass spectrometry and collision-induced dissociation for the identification of disulfide-bonded peptides. J. Mass Spectrom. 46, 677–688. doi: 10.1002/jms.1938
Leonard, S. E., Reddie, K. G., and Carroll, K. S. (2009). Mining the thiol proteome for sulfenic acid modifications reveals new targets for oxidation in cells. ACS Chem. Biol. 4, 783–799. doi: 10.1021/cb900105q
Liu, P., Zhang, H., Wang, H., and Xia, Y. (2014). Identification of redox-sensitive cysteines in the Arabidopsis proteome using OxiTRAQ, a quantitative redox proteomics method. Proteomics 14, 750–762. doi: 10.1002/pmic.201300307
Liu, P., Zhang, H., Yu, B., Xiong, L., and Xia, Y. (2015). Proteomic identification of early salicylate- and flg22-responsive redox-sensitive proteins in Arabidopsis. Sci. Rep. 5:8625. doi: 10.1038/srep08625
McConnell, E. W., Berg, P., Westlake, T. J., Wilson, K. M., Popescu, G. V., Hicks, L. M., et al. (2019). Proteome-wide analysis of cysteine reactivity during effector-triggered immunity. Plant Physiol. 179, 1248–1264. doi: 10.1104/pp.18.01194
Murad, W., Singh, R., and Yen, T.-Y. (2011). An efficient algorithmic approach for mass spectrometry-based disulfide connectivity determination using multi-ion analysis. BMC Bioinformatics 12(Suppl. 1):S12. doi: 10.1186/1471-2105-12-S1-S12
Na, S., Paek, E., Choi, J.-S., Kim, D., Lee, S. J., and Kwon, J. (2015). Characterization of disulfide bonds by planned digestion and tandem mass spectrometry. Mol. Biosyst. 11, 1156–1164. doi: 10.1039/c4mb00688g
Oger, E., Marino, D., Guigonis, J.-M., Pauly, N., and Puppo, A. (2012). Sulfenylated proteins in the Medicago truncatula-Sinorhizobium meliloti symbiosis. J. Proteomics 75, 4102–4113. doi: 10.1016/j.jprot.2012.05.024
Paulsen, C. E., Truong, T. H., Garcia, F. J., Homann, A., Gupta, V., Leonard, S. E., et al. (2012). Peroxide-dependent sulfenylation of the EGFR catalytic site enhances kinase activity. Nat. Chem. Biol. 8, 57–64. doi: 10.1038/nchembio.736
Pettersen, E. F., Goddard, T. D., Huang, C. C., Couch, G. S., Greenblatt, D. M., Meng, E. C., et al. (2004). UCSF chimera-a visualization system for exploratory research and analysis. J. Comput. Chem. 25, 1605–1612. doi: 10.1002/jcc.20084
Poole, L. B., Furdui, C. M., and King, S. B. (2020). Introduction to approaches and tools for the evaluation of protein cysteine oxidation. Essays Biochem. 64, 1–17. doi: 10.1042/ebc20190050
Puyaubert, J., Fares, A., Rézé, N., Peltier, J.-B., and Baudouin, E. (2014). Identification of endogenously S-nitrosylated proteins in Arabidopsis plantlets: effect of cold stress on cysteine nitrosylation level. Plant Sci. 21, 150–156. doi: 10.1016/j.plantsci.2013.10.014
Roos, G., Foloppe, N., and Messens, J. (2013). Understanding the pKa of redox cysteines: the key role of hydrogen bonding. Antioxid. Redox Signal. 18, 94–127. doi: 10.1089/ars.2012.4521
Roos, G., and Messens, J. (2011). Protein sulfenic acid formation: from cellular damage to redox regulation. Free Radic. Biol. Med. 51, 314–326. doi: 10.1016/j.freeradbiomed.2011.04.031
Sigrist, C. J. A., de Castro, E., Cerutti, L., Cuche, B. A., Hulo, N., Bridge, A., et al. (2013). New and continuing developments at PROSITE. Nucleic Acids Res. 41, D344–D347. doi: 10.1093/nar/gks1067
Song, S., Wang, H., Sun, M., Tang, J., Zheng, B., Wang, X., et al. (2019). Reactive oxygen species-mediated BIN2 activity revealed by single-molecule analysis. New Phytol. 223, 692–704. doi: 10.1111/nph.15669
Takanishi, C. L., Ma, L.-H., and Wood, M. J. (2007). A genetically encoded probe for cysteine sulfenic acid protein modification in vivo. Biochemistry 46, 14725–14732. doi: 10.1021/bi701625s
Takanishi, C. L., and Wood, M. J. (2011). A genetically encoded probe for the identification of proteins that form sulfenic acid in response to H2O2 in Saccharomyces cerevisiae. J. Proteome Res. 10, 2715–2724. doi: 10.1021/pr1009542
Tanner, J. J., Parsons, Z. D., Cummings, A. H., Zhou, H., and Gates, K. S. (2011). Redox regulation of protein tyrosine phosphatases: structural and chemical aspects. Antioxid. Redox Signal. 15, 77–97. doi: 10.1089/ars.2010.3611
The UniProt Consortium (2019). UniProt: a worldwide hub of protein knowledge. Nucleic Acids Res. 47, D506–D515. doi: 10.1093/nar/gky1049
Tian, Y., Fan, M., Qin, Z., Lv, H., Wang, M., Zhang, Z., et al. (2018). Hydrogen peroxide positively regulates brassinosteroid signaling through oxidation of the BRASSINAZOLE-RESISTANT1 transcription factor. Nat. Commun. 9:1063. doi: 10.1038/s41467-018-03463-x
Van Leene, J., Stals, H., Eeckhout, D., Persiau, G., Van De Slijke, E., Van Isterdael, G., et al. (2007). A tandem affinity purification-based technology platform to study the cell cycle interactome in Arabidopsis thaliana. Mol. Cell. Proteomics 6, 1226–1238. doi: 10.1074/mcp.M700078-MCP200
Waszczak, C., Akter, S., Eeckhout, D., Persiau, G., Wahni, K., Bodra, N., et al. (2014). Sulfenome mining in Arabidopsis thaliana. Proc. Natl. Acad. Sci. U.S.A. 111, 11545–11550. doi: 10.1073/pnas.1411607111
Waszczak, C., Akter, S., Jacques, S., Huang, J., Messens, J., and Van Breusegem, F. (2015). Oxidative post-translational modifications of cysteine residues in plant signal transduction. J. Exp. Bot. 66, 2923–2934. doi: 10.1093/jxb/erv084
Willems, P., Horne, A., Van Parys, T., Goormachtig, S., De Smet, I., Botzki, A., et al. (2019). The plant PTM Viewer, a central resource for exploring plant protein modifications. Plant J. 99, 752–762. doi: 10.1111/tpj.14345
Wu, F., Chi, Y., Jiang, Z., Xu, Y., Xie, L., Huang, F., et al. (2020). Hydrogen peroxide sensor HPCA1 is an LRR receptor kinase in Arabidopsis. Nature 578, 577–581. doi: 10.1038/s41586-020-2032-3
Yang, H., Mu, J., Chen, L., Feng, J., Hu, J., Li, L., et al. (2015). S-nitrosylation positively regulates ascorbate peroxidase activity during plant stress responses. Plant Physiol. 167, 1604–1615. doi: 10.1104/pp.114.255216
Yang, J., Carroll, K. S., and Liebler, D. C. (2016). The expanding landscape of the thiol redox proteome. Mol. Cell. Proteomics 15, 1–11. doi: 10.1074/mcp.O115.056051
Yang, J., Gupta, V., Carroll, K. S., and Liebler, D. C. (2014). Site-specific mapping and quantification of protein S-sulphenylation in cells. Nat. Commun. 5, 4776. doi: 10.1038/ncomms5776
Yang, J., Gupta, V., Tallman, K. A., Porter, N. A., Carroll, K. S., and Liebler, D. C. (2015). Global, in situ, site-specific analysis of protein S-sulfenylation. Nat. Protoc. 10, 1022–1037. doi: 10.1038/nprot.2015.062
Yuan, H. M., Liu, W. C., and Lu, Y. T. (2017). Catalase2 coordinates SA-mediated repression of both auxin accumulation and JA biosynthesis in plant defenses. Cell Host Microbe 21, 143–155. doi: 10.1016/j.chom.2017.01.007
Yutthanasirikul, R., Nagano, T., Jimbo, H., Hihara, Y., Kanamori, T., Ueda, T., et al. (2016). Oxidation of a cysteine residue in elongation factor EF-Tu reversibly inhibits translation in the cyanobacterium Synechocystis sp. PCC 6803. J. Biol. Chem. 291, 5860–5870. doi: 10.1074/jbc.M115.706424
Keywords: S-sulfenylation (-SOH), YAP1C, hydrogen peroxide, cross-linked peptide identification, affinity purification, disulfide, Arabidopsis thaliana
Citation: Wei B, Willems P, Huang J, Tian C, Yang J, Messens J and Van Breusegem F (2020) Identification of Sulfenylated Cysteines in Arabidopsis thaliana Proteins Using a Disulfide-Linked Peptide Reporter. Front. Plant Sci. 11:777. doi: 10.3389/fpls.2020.00777
Received: 17 March 2020; Accepted: 15 May 2020;
Published: 02 July 2020.
Edited by:
Christian Lindermayr, Helmholtz Zentrum München, GermanyReviewed by:
Mirko Zaffagnini, University of Bologna, ItalyThomas Leustek, Rutgers, The State University of New Jersey, United States
Copyright © 2020 Wei, Willems, Huang, Tian, Yang, Messens and Van Breusegem. This is an open-access article distributed under the terms of the Creative Commons Attribution License (CC BY). The use, distribution or reproduction in other forums is permitted, provided the original author(s) and the copyright owner(s) are credited and that the original publication in this journal is cited, in accordance with accepted academic practice. No use, distribution or reproduction is permitted which does not comply with these terms.
*Correspondence: Joris Messens, Sm9yaXMubWVzc2Vuc0B2dWIuYmU=; Frank Van Breusegem, RnJhbmsudmFuYnJldXNlZ2VtQHBzYi52aWItdWdlbnQuYmU=; ZnJicmVAcHNiLnZpYi11Z2VudC5iZQ==
†These authors have contributed equally to this work