- 1Department of Ecology and Evolutionary Biology, University of Colorado, Boulder, CO, United States
- 2Aerospace Engineering Sciences, University of Colorado, Boulder, CO, United States
- 3Space Lab Technologies, LLC, Boulder, CO, United States
Duckweed is a promising food crop with multiple benefits for space applications. Fresh duckweed could deliver synergistically acting essential antioxidant nutrients to a crew – but only if growth conditions provide the plant with the right cues to trigger antioxidant formation. We grew Lemna gibba under continuous growth light ranging from low to very high intensities (photosynthetic photon flux densities = PPFDs) in order to investigate the effect on plant growth, photosynthesis, and level of carotenoid antioxidants that are essential human micronutrients. Lemna gibba achieved remarkably high growth rates under modest growth PPFD by virtue of superior light absorption resulting from minimal self-shading and high chlorophyll levels. Conversely, L. gibba’s growth rate remained high even under very high growth PPFDs. This notable ability of L. gibba to avoid inactivation of photosynthesis and diminished growth under very high growth PPFDs resulted from a combination of downregulation of chlorophyll synthesis and increased biochemical photoprotection that limited a build-up of excessive excitation energy. This biochemical photoprotection included accumulation of zeaxanthin (an essential human micronutrient) and high levels of zeaxanthin-catalyzed thermal energy dissipation of excess excitation. Compared to the light levels needed to saturate L. gibba photosynthesis and growth, higher light levels were thus required for strong induction of the essential antioxidant zeaxanthin. These results indicate a need for design of light protocols that achieve simultaneous optimization of plant yield, nutritional quality, and light-use efficiency to circumvent the fact that the light requirement to saturate plant growth is lower than that for production of high zeaxanthin levels. How this trade-off between light-use efficiency of growth and nutritional quality might be minimized or circumvented to co-optimize all desired features is discussed.
Introduction
Self-sufficient life support systems for long-duration space exploration require reliable autonomous systems that use minimal amounts of expendables. Integration of photosynthetic organisms offers multi-functional regenerative life support in space, including production of food and oxygen, recycling of CO2 and other human waste, and recovery of water and nutrients. A good space crop should have a high growth rate, a high harvest index (be mostly edible), and high nutritional value, while requiring minimal resources such as occupied volume, water, and energy.
Duckweeds (family Lemnaceae) are small, floating aquatic plants that are 100% edible, nutritious, non-toxic, fast growing, and able to purify nutrient-rich wastewater (Leng, 1999; Appenroth et al., 2017, 2018; Chen et al., 2018; Iatrou et al., 2019; Sree et al., 2019). Duckweed grows continuously by propagation and is among the fastest-growing plants with respect to its doubling rate, e.g., doubling its biomass in as little as 1 to 3 days (Ziegler et al., 2015). Duckweed has, therefore, received attention as a potential crew food supplement or wastewater treatment for the human space program (Ward and Wilks, 1963; Wilks, 1964; Woverton and McDonald, 1977; Gale et al., 1989; Bluem and Paris, 2001; Polyakov et al., 2010). Moreover, Yuan and Xu (2017) found that simulated microgravity actually stimulated duckweed growth and called duckweed “one of the most attractive higher plants” for long-duration space life support.
To realize duckweed’s full potential, optimal growing conditions for high yields of nutritious food with the fewest spacecraft resources need to be defined in an environment relevant for space missions. Although duckweed has tremendous potential for both high productivity and nutritional value, duckweed can be expected to be subject to a universal trade-off in plant physiology. Energy-efficient plant biomass production comes at the cost of poor micronutrient quality of plant biomass (especially antioxidant vitamins) and vice versa. This trade-off is caused by the fact that a growth light intensity (photosynthetic photon flux density, PPFD) just enough to support maximal plant growth is not enough to induce high vitamin/antioxidant levels for principal reasons (Polutchko et al., 2015). Only exposure to excess PPFD, defined as more light than needed to saturate growth, prompts plants to accumulate protective antioxidants that prevent damage by excess light. However, such excess PPFD has the potential to negatively impact photosynthesis, and, by the above definition, represents input of more light than needed to maximize growth, which lowers the light-use efficiency of plant productivity. This fundamental link leads to a trade-off between light-use efficiency and antioxidant micronutrient content, the severity of which varies among plant species and growth conditions. Plant species vary in their tolerance of very low or very high growth PPFD and most species do not thrive equally well in deep shade and full sun (Adams et al., 2018). Shade-tolerant species, in particular, can exhibit lasting depressions in the efficiency of primary photochemistry (a phenomenon termed photoinhibition of photosynthesis) when grown under high PPFD (Adams et al., 2013, 2014, 2018). A major component of the response to growth under higher PPFD compared to low light is the increased synthesis of photoprotectors and antioxidants (Grace and Logan, 1996; Logan et al., 1996, 1998a,b).
Antioxidant metabolites can be categorized into a large group of water-soluble metabolites and a small set of water-insoluble, membrane-bound metabolites. We here focus on water-insoluble carotenoids (the xanthophylls zeaxanthin and lutein as well as β-carotene) that protect biological membranes (Demmig-Adams and Adams, 2013; Demmig-Adams et al., 2013; Polutchko et al., 2015). Deficiency in these antioxidants causes production of membrane break-down products that trigger chronic inflammation in humans as root causes of all major chronic diseases and disorders (Demmig-Adams and Adams, 2002, 2013). Ionizing radiation, a major challenge in space, produces oxidants and triggers chronic inflammation throughout the body (Azzam et al., 2012). Foods rich in zeaxanthin, lutein, and β-carotene were shown to protect against damage by ionizing radiation in a population of airline pilots (Yong et al., 2009; see also Asker et al., 2007). Zeaxanthin is the primary antioxidant photoprotector in leaves as well as the human eye and other tissues (Demmig-Adams and Adams, 2002, 2013), yet is much harder to come by in the diet than lutein or β-carotene. Lutein plays a secondary role in the protection of leaves and the human eye, yet is more abundant in plant-based food (Demmig-Adams and Adams, 2002, 2013; Polutchko et al., 2015). Several plant regulatory mechanisms ensure that zeaxanthin is formed in leaves only under excess light and is removed quickly upon return to non-excessive light levels (Demmig-Adams et al., 1996b). β-carotene can also serve as an antioxidant in both plants (Telfer, 2014) and animals (Stahl and Sies, 2012) and is, furthermore, the precursor (pro-vitamin A) in the synthesis of vitamin A that is converted to the light-absorbing (retinal) component of the human eye (Polutchko et al., 2015). Zeaxanthin also has a second role in the direct protection of membrane lipids and acts synergistically with vitamin E in this role (Wrona et al., 2003, 2004; Schneider, 2005).
Unlike most plants, duckweed contains all amino acids essential for humans and has a fat composition with high levels of beneficial essential fatty acids (Appenroth et al., 1982, 2017; Yan et al., 2013). Duckweeds also produce high levels of other essential micronutrients for humans that have to be supplied by the human diet and include the synergistically acting antioxidant carotenoids zeaxanthin, lutein, and β-carotene and the antioxidant vitamins C (ascorbic acid) and E (especially α-tocopherol essential to human health) (Appenroth et al., 2017, 2018). Dietary zeaxanthin, in particular, increases visual acuity, protects the human eye against damage from intense light (prevents cataracts and blindness), and combats inflammation throughout the body (Demmig-Adams and Adams, 2013). A good dietary supply of zeaxanthin is thus critical for astronauts exposed to dangerous levels of damaging radiation. However, uptake of these micronutrients from supplements can be poor in the human gut, particularly for the water-insoluble vitamins A, E, and carotenoids that are best taken up from a food matrix (Tran and Demmig-Adams, 2007; Demmig-Adams and Adams, 2013; Rodriguez-Casado, 2016). Plants produce these antioxidants for their own protection against damaging radiation that contributes to oxidant production. In the case of zeaxanthin, leaves accumulate a zeaxanthin precursor (violaxanthin) and convert the latter to zeaxanthin only under exposure to more light than plants can use for growth (Demmig-Adams and Adams, 2002). Fresh duckweed can deliver a balanced mix of these synergistically acting essential nutrients to a crew – but only if growth conditions provide the plant with the right cues to trigger antioxidant formation. This critical link between plant growth conditions and leaf antioxidant content is the focus of the present study. We grew Lemna gibba over a wide range of growth PPFDs (with continuous light, i.e., 24 h per day), ranging from low (100 μmol m–2 s–1) to very high (700 μmol m–2 s–1), in order to investigate the effect of growth light intensity on growth rate and the production of essential human micronutrient carotenoids.
Materials and Methods
Plant Material and Growth Conditions
Lemna gibba L. 7741 (G3), obtained from Rutgers Duckweed Stock Cooperative1, was used for all experiments. Plants were grown in 150 × 75 mm PYREX Crystallizing Dishes (Corning Inc., Corning, NY, United States) containing 1000 mL of Schenk and Hildebrandt Medium (bioWORLD, Dublin, OH, United States; Schenk and Hildebrandt, 1972) at a concentration of 1.6 g L–1 and a pH of 5.5 (adjusted via 1% [w/v] KOH). To minimize microbial contamination, media were prepared with recently boiled water prior to each transfer of fronds to new dishes. The volume of each dish was monitored every day and adjusted with recently boiled water to compensate for evaporative water loss. Stock cultures were maintained under a constant PPFD of 50 μmol m–2 s–1 supplied via fluorescent (F72T12/CW/HO; Philips, Somerset, NJ, United States) and incandescent (100W, 130V; EiKO, Shawnee, KS, United States) bulbs and an air, water, and frond temperature of 25°C in a Conviron PGR15 growth chamber (Controlled Environments Ltd., Winnipeg, MB, Canada). During cultivation, a subset of approximately 20 fronds from each dish were transferred using sterile inoculation loops (2865, Globe Scientific, Mahwah, NJ, United States) to fresh media in clean dishes at least once per week (typically after 4 days).
Experimental plants were grown under four light intensities, with PPFDs of 100, 200, 500, and 700 μmol m–2 s–1. All plants were grown under continuous light at ambient CO2 concentrations (approximately 400 ppm in Boulder, CO) and a water and frond temperature of 25°C since duckweed growth is enhanced under continuous light versus shorter photoperiods (Yin et al., 2015; Liu et al., 2018). For experiments, plants were transferred to, and cultivated under, each respective final growth PPFD for 7 days (Figure 1). For growth experiments under 100 and 200 μmol photons m–2 s–1, plants were acclimated to these PPFDs for 3 days, followed by 4 days of experimentation with full monitoring of growth rates and other parameters (Figure 1). For experiments under growth PPFDs of 500 and 700 μmol m–2 s–1, plants were transferred to 200 μmol m–2 s–1 for 3 days before transfer to the respective final experimental PPFDs (Figure 1). At the time of each transfer to a new growth PPFD as well as at the start of the 4-day active phase of each experiment subsequent to 3 days of acclimation, frond density was reset to 20 fronds per dish by transferring only 20 fronds to fresh medium in a new dish. Growth experiments were conducted successively in the same growth chamber with three replicate dishes illuminated by three identical custom-built panels of white LEDs (C503C-WAN; CREE Inc., Durham, NC, United States; for details on LED features, see Burch, 2016). Temperature of media was monitored during the 3-day acclimation phase using alcohol-sterilized thermometers, and frond temperature kept constant at 25°C. Under the higher growth PPFDs, air temperature was lowered from 25°C until temperature of the media was maintained at 25°C for 24 h. Resulting adjusted air temperatures were 24 and 20°C for growth PPFDs of 500 and 700 μmol m–2 s–1, respectively. Since floating duckweed has unlimited access to water and its stomates are inactive, i.e., are kept fully open over a wide range of conditions (McLaren and Smith, 1976), any differences in transpiration rate at these different air temperatures would not be expected to impact the parameters assessed in this study. Immediately prior to both acclimation and onset of experimental phases, a subset of approximately 20 fronds from each dish was transferred to fresh, filtered medium in clean dishes. Medium was filtered through Fisherbrand P5 filter paper (Fisher Scientific, Hampton, NH, United States) prior to the acclimation phase and 0.22-μm Millipak-20 filters (Millipore, Billerica, MA, United States) prior to the active test phase.
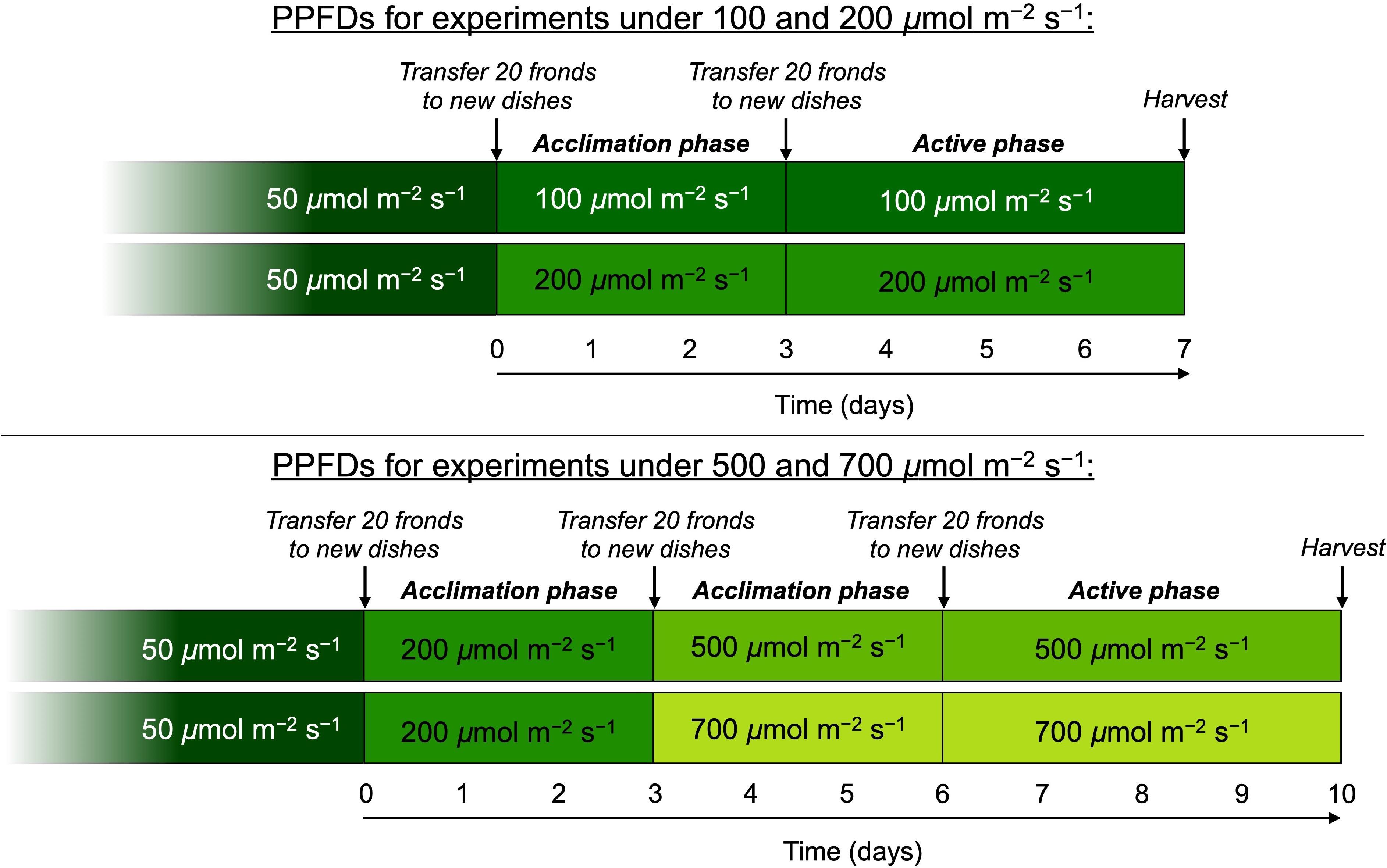
Figure 1. Schematic depicting the protocol for growth of L. gibba under different photosynthetic photon flux densities (PPFDs). All plants were initially cultivated under 50 μmol photons m–2 s–1 and then transferred to either 100 or 200 μmol photons m–2 s–1. Following 3 days of acclimation, growth rate for these two PPFDs was monitored for 4 days after which fronds were sampled for the various features characterized. For the higher growth light intensities, additional fronds were transferred from 200 μmol photons m–2 s–1 to each of the PPFDs where each experienced a 3-day acclimation period followed by 4 days of characterized growth and sampling for the other parameters.
Growth Rate and Photon Flux
Once per day during the 4-day active phase of each experiment, digital photographic images of each dish were taken from directly above and perpendicular to the surface of the media (see images in Figure 2). Frond area was measured using MATLAB Image Processing Toolbox (MathWorks, Natick, MA, United States) by first selecting the pixels containing the water surface inside the dish (i.e., those masking the water surface) and then selecting pixels inside the masked area containing green fronds with a color thresholding algorithm. Frond area was calculated as the percentage of total water surface containing fronds times the known water surface area of the crystallizing dish (145-mm inner diameter).
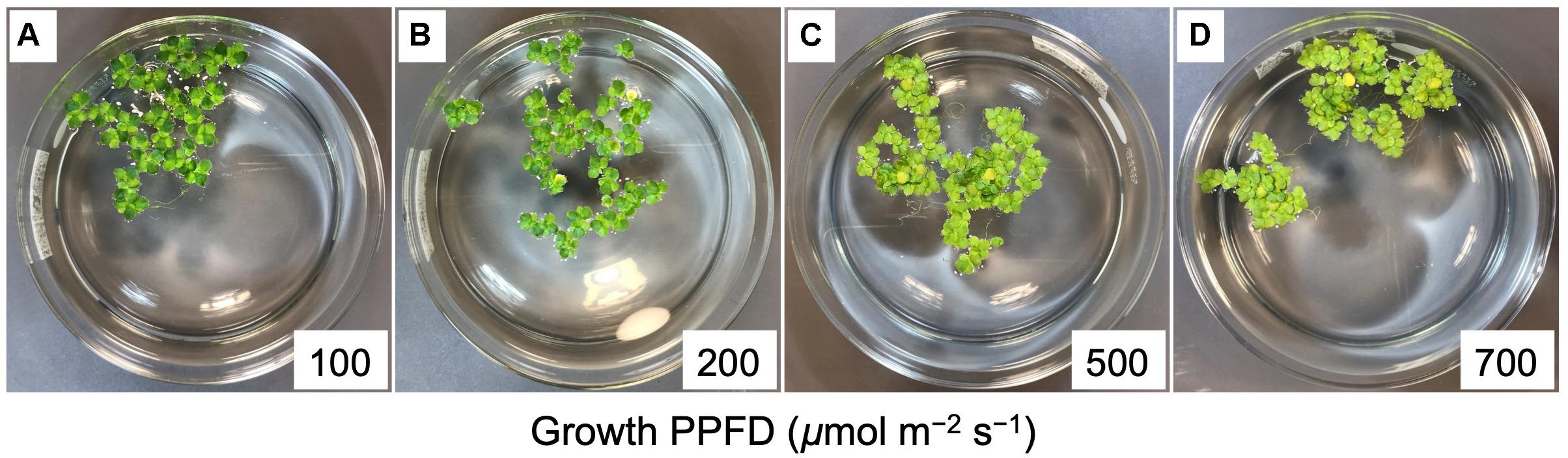
Figure 2. Images of crystallizing dishes with L. gibba fronds following a 4-day growth experiment at each of the four different PPFDs investigated, which correspond to the numbers (100 to 700) in each of the panels A through D. Each dish started with approximately 20 fronds. Mother fronds were slightly darker than daughter fronds. Note the greater number of fronds per colony and greater proliferation of roots at the higher growth PPFDs.
Relative growth rate was calculated as the difference in ln-adjusted frond areas divided by the time elapsed (in days) between the two measurements (see, e.g., Hunt, 1990), as shown in the equation below (where FA4 and FA0 are the frond areas at the end and beginning of the 4-day experiment, respectively, and t4 is the time elapsed between these two measurements):
Relative growth rate (RGR) and initial frond area (FA0) for each dish were then used to construct the following generalized relationship between frond area (in m2) and time elapsed (t):
For each PPFD (in μmol photons m–2 s–1), the rate at which plants within each dish received photons scaled proportionally with their frond area. Thus, the following generalized relationship was used for photon flux for plants within each dish (in mol day–1 with conversion factors of 0.000001 mol μmol–1 and 86,400 s day–1) and time:
Number of photons incident on the plant surface within a dish during the 4-day experiments was estimated using the following equation:
Light-use efficiency (as m2 frond area produced per mol photons received during the experiment) was calculated as:
Frond dry mass was quantified with an A-160 balance (Denver Instruments Company, Denver, CO, United States) from fronds that had been dried at 70°C for 7 days, and frond area of each sample quantified with ImageJ software (Schneider et al., 2012) from images taken before samples were placed in the drying oven.
Photosynthetic Capacity
Photosynthetic capacity was determined as light- and CO2-saturated rate of net photosynthetic oxygen evolution with oxygen electrode systems (Hansatech Instruments Ltd., Norfolk, United Kingdom; see Delieu and Walker, 1981) coupled with circulating water baths set to 25°C as previously described (Dumlao et al., 2012). For plants grown under PPFDs of 100 to 500 μmol m–2 s–1, oxygen evolution was measured from fronds of a known area after 5-min exposures to 500, 1000, and 1500 μmol photons m–2 s–1. Plants grown under 700 μmol photons m–2 s–1 were assayed at PPFDs of 1000, 1500, and 2000 μmol m–2 s–1. Frond areas were quantified from images of samples taken either immediately before or after each measurement using ImageJ.
Chlorophyll Fluorescence
Chlorophyll fluorescence was measured with a PAM-101 chlorophyll fluorometer (Walz, Effeltrich, Germany) to estimate allocation of absorbed light to photosynthesis versus photoprotective thermal energy dissipation as well as excitation energy not removed via either photosynthesis or energy dissipation as previously described (Figure 3; Genty et al., 1989; Demmig-Adams et al., 1996a; Adams and Demmig-Adams, 2004; Logan et al., 2014). Maximal chlorophyll fluorescence yield (with all photosystem II [PSII] centers in the reduced state and unavailable to perform photochemistry) was determined after 5 min of dark incubation (Fm) and after 5 min of exposure to PPFDs corresponding to the respective growth PPFDs (Fm′). Steady-state fluorescence under the respective growth PPFD (F) was determined immediately before measurement of Fm′. The yield of minimal chlorophyll fluorescence (with all PSII centers in the open state ready to perform photochemistry) was determined after 5 min of dark incubation (Fo) and upon brief darkening after steady-state fluorescence had been reached during exposure to actinic light corresponding to the respective growth PPFD (Fo′). Variable fluorescence after dark incubation (Fv) and after exposure to growth PPFD (Fv′) were Fm – Fo and Fm′ – Fo′, respectively. Potential maximal PSII photochemical efficiency after discontinuation of light-dependent thermal energy dissipation activity is given by Fv/Fm of darkened leaves (fronds darkened for 5 min either immediately upon removal from growth PPFD or after 30 min of recovery in low light of 10 μmol m–2 s–1). Maximal photochemical efficiency in the light (in the presence of light-dependent thermal energy dissipation) of those PSII centers that remain open at each growth PPFD is given by Fv′/Fm′. The fraction of PSII centers that are closed (reduced) under each PPFD is given by 1 – qP = (F – Fo′)/(Fm′ – Fo′), where qP stands for photochemical quenching. Photochemical efficiency at the percentage of closed PSII centers under each respective growth PPFD is given by Fv′/Fm′ × qP. The latter efficiency determines the efficiency of photosynthetic electron transport. In leaves that do not exhibit photoinhibitory inactivation of photosynthesis (see section “Results”), the fraction of absorbed photons dissipated by photoprotective energy dissipation under each respective growth PPFD can be assessed as 0.8 – Fv′/Fm′ (see Demmig-Adams et al., 1996a). The fraction of absorbed photons used neither in photochemistry nor in thermal energy dissipation is given by Fv′/Fm′ × (1 – qP) (Demmig-Adams et al., 1996a).
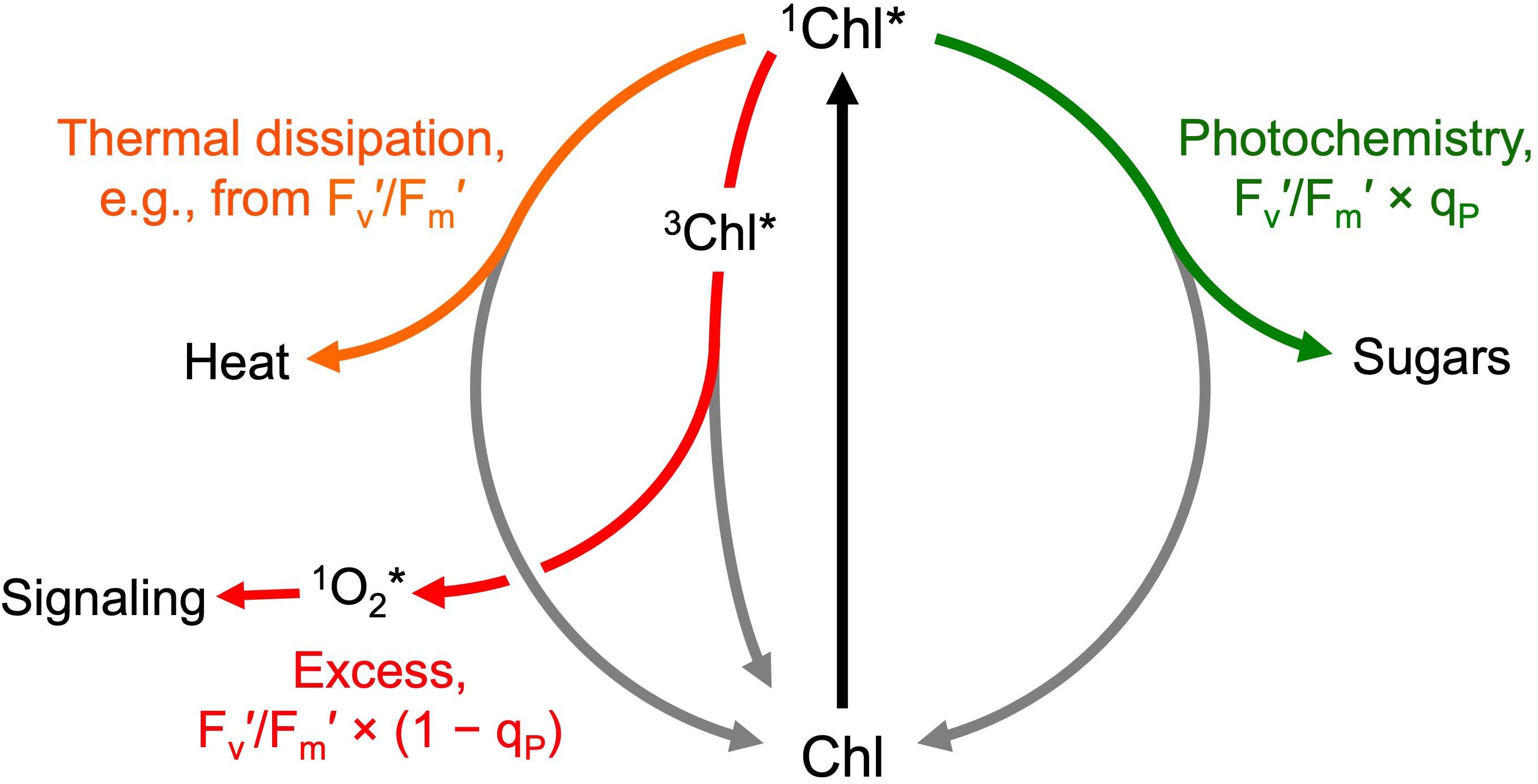
Figure 3. Allocation of absorbed photons (after exciting chlorophyll from the ground state, Chl, to the singlet excited state, 1Chl*) to photochemistry (available for photosynthesis and growth; green arrow), photoprotective thermal energy dissipation (facilitated via zeaxanthin; orange arrow), and the remaining excess excitation energy removed neither via photosynthesis nor thermal energy dissipation (red arrow). The excess excitation energy leaves the system via conversion of singlet excited chlorophyll (1Chl*) to triplet excited chlorophyll (3Chl*) and subsequent transfer of the excitation energy to oxygen resulting in the formation of singlet excited oxygen (1O2*) that can oxidize polyunsaturated membrane lipids to gene regulators (signaling) or, in large amounts, lead to membrane damage. The allocation of photons to these different fates under a given condition can be estimated from chlorophyll fluorescence emission (see text for further explanation of the parameters shown).
Chlorophylls, Carotenoids, and Starch
Chlorophylls a and b, β-carotene, lutein, zeaxanthin, antheraxanthin, violaxanthin, and neoxanthin levels were determined via high-performance liquid chromatography as previously described (Stewart et al., 2015). From each dish under each growth PPFD, two samples of approximately 10 fronds each were harvested for pigment analysis at the same time as samples of fronds were taken for chlorophyll fluorescence measurements, i.e., before and after 30 min of recovery under 10 μmol photons m–2 s–1. After removal of roots, fronds were imaged for area with ImageJ and frozen in liquid nitrogen. Pigment concentrations for each dish were expressed as average concentrations of the two samples harvested before and after 30 min of recovery for those pigments that do not change in concentration over 30 min, i.e., chlorophyll a and b, lutein, β-carotene, the sum of the xanthophyll cycle pigments violaxanthin + antheraxanthin + zeaxanthin (V + A + Z), and total carotenoids (lutein, β-carotene, V, A, Z, and neoxanthin). Zeaxanthin level was calculated separately for samples collected before versus after 30 min of recovery. The reported zeaxanthin levels are from samples collected before the recovery unless otherwise stated.
For qualitative assessment of starch content, fronds were cleared in 70% (v/v) ethanol, stained for 5 min with diluted iodine-potassium iodide solution (Lugol’s solution; Sigma-Aldrich, St. Louis, MO, United States), and immediately mounted and imaged with a high-resolution scanner (Perfection 3200 Photo; Epson America, Inc., Long Beach, CA, United States).
Statistical Analyses
Statistically significant differences among growth PPFDs were determined via analysis of variance (one-way ANOVA) and post hoc Tukey–Kramer test for Honestly Significant Differences. Lines of best fit were obtained using non-linear models. Analyses were conducted with JMP Pro 15.0.0 (SAS Institute Inc., Cary, NC, United States). Sample sizes for each experiment was 3 dishes as also indicated in figure legends.
Results
Growth Rates and Light-Use Efficiency of Growth
Dishes of L. gibba were photographed (Figure 2) once per day over 4 days of growth under PPFDs ranging from 100 to 700 μmol photons m–2 s–1 of continuous light. These images indicate that a similar level of frond growth was maintained over this range of growth PPFDs (Figures 2, 4). Due to these similar trajectories of exponential growth, corresponding growth curves were largely overlapping (Figure 4A). Relative growth rates (difference in ln-adjusted frond areas per unit of time) were consequently also similar (Figure 4B). In other words, L. gibba achieved rather high growth rates at low growth PPFDs and maintained similar growth rates over a wide range of growth PPFDs. Due to the fact that a 7-fold increase in growth PPFD (from 100 to 700 μmol m–2 s–1) did not produce a great deal of additional growth, light-use efficiency of area production was maximal at the lowest growth PPFD and declined precipitously with increasing growth PPFD (Figure 4C). Specifically, a 25% greater relative growth rate at 700 (maximal RGR) versus 100 μmol photons m–2 s–1 came at the cost of a 600% greater input of light.
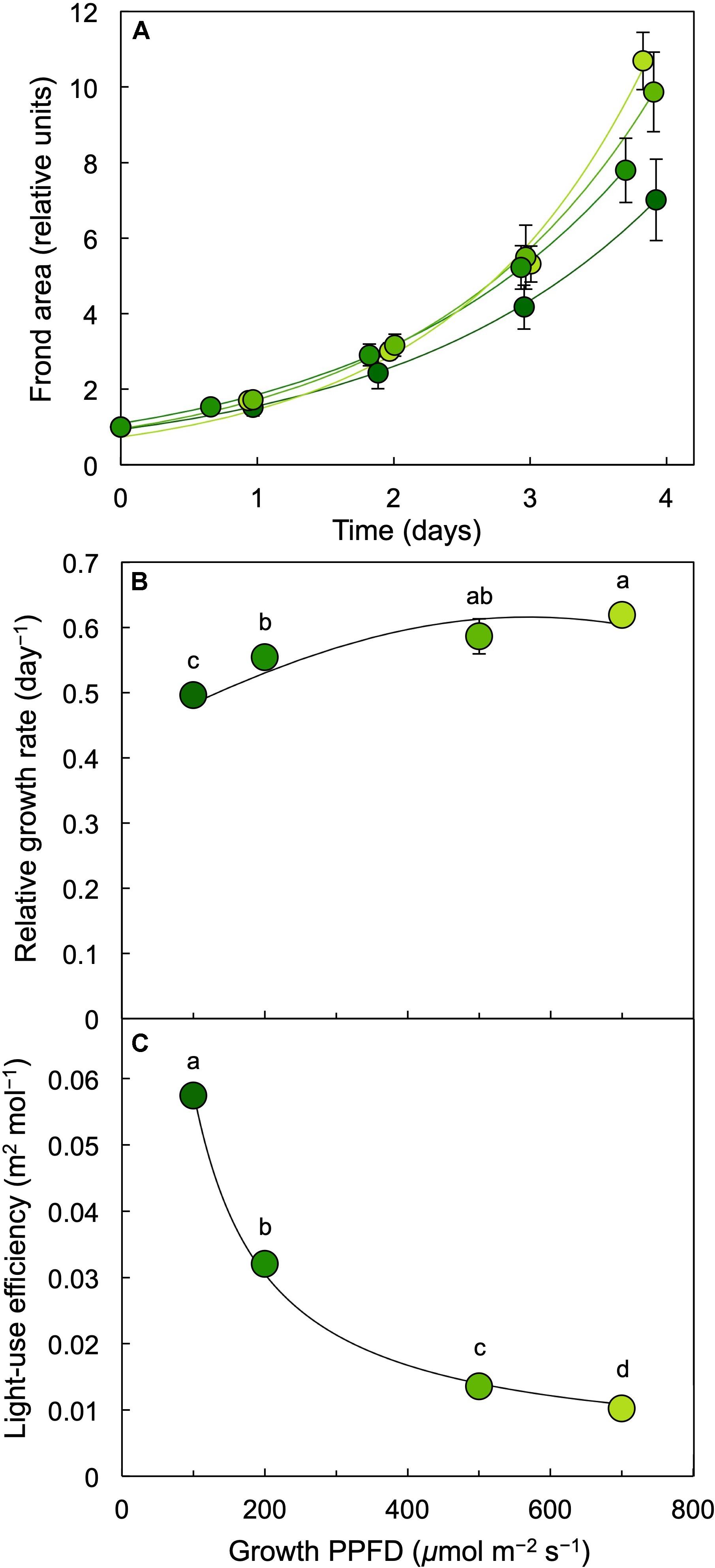
Figure 4. (A) Frond area accumulation (starting values normalized to 1) over a period of 4 days, (B) relative growth rate (difference in ln-adjusted frond areas per time elapsed) as a function of growth PPFD, and (C) light-use efficiency of L. gibba frond area production as a function of growth PPFD. The shades of green to yellow of the symbols correspond to the respective growth PPFDs (see Figure 1 and B,C), ranging from darker green (for 100 μmol m–2 s–1) to light green (for 700 μmol m–2 s–1). Mean values ± standard deviations, n = 3 for all growth PPFDs; different lower-case letters signify statistical differences at P < 0.05 via one-way analysis of variance and post hoc Tukey–Kramer HSD test.
Frond Content of Photosynthetic Pigments
Chlorophyll content on a frond area decreased at growth PPFDs between 200 and 700 μmol m–2 s–1 (Figures 2B–D, 5A). On a frond areas basis, total carotenoids increased with increasing growth PPFD and remained similar up to the highest growth PPFD. This pattern was accounted for by the trends of the constituent carotenoid fractions. While the total pool of the xanthophyll cycle pigments violaxanthin, antheraxanthin, and zeaxanthin (V + A + Z) showed an increasing trend with increasing growth PPFD, the levels of the individual carotenoids lutein and β-carotene declined between 200 and 700 μmol photons m–2 s–1 (Figures 5B,C). The only individual carotenoid that showed a unique, contrasting trend was zeaxanthin that was at negligible levels under the lowest growth PPFD and increased successively with each increase in growth PPFD between 200 and 700 μmol m–2 s–1 (Figure 5A).
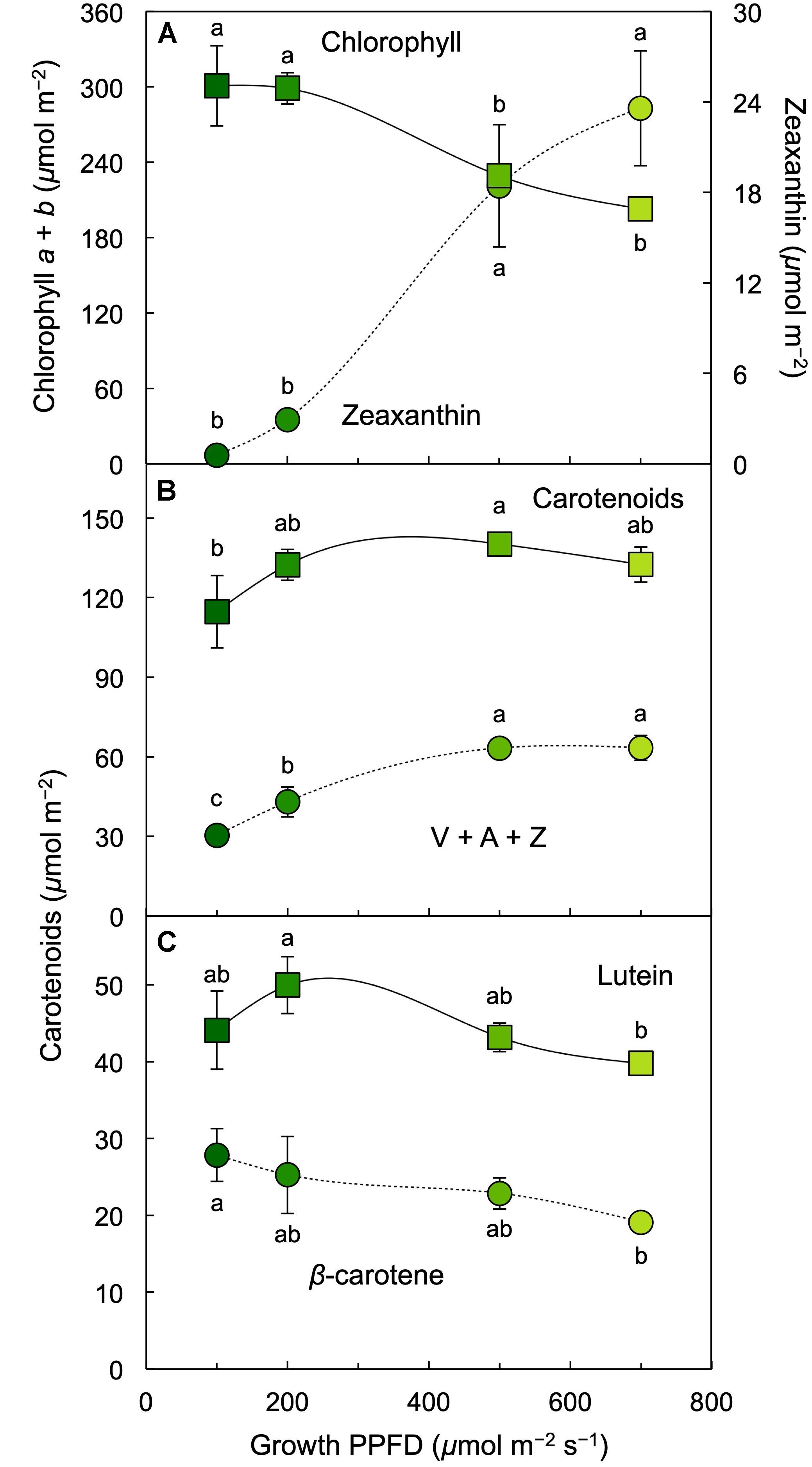
Figure 5. Levels of (A) chlorophyll a + b (squares) and zeaxanthin (circles), (B) carotenoids (sum of V, A, Z, lutein, β-carotene, and neoxanthin; squares) and the xanthophyll cycle pool (V + A + Z; circles), and (C) lutein (squares) and β-carotene (circles) on an L. gibba frond area basis as a function of growth PPFD. A, antheraxanthin; V, violaxanthin; Z, zeaxanthin. Mean values ± standard deviations, n = 3 for all growth PPFDs; different lower-case letters signify statistical differences at P < 0.05 via one-way analysis of variance and post hoc Tukey–Kramer HSD test.
The ratio of chlorophyll a (bound to all chlorophyll antennae) to chlorophyll b (bound only to the outer chlorophyll antennae that maximize light absorption under limited light levels) remained constant between 100 and 700 μmol photons m–2 s–1 (Figure 6A) whereas chlorophyll a + b levels declined (Figure 5A). All carotenoids increased relative to chlorophyll with increasing growth PPFD (Figures 6B,C). The increase of the total carotenoid pool was paralleled by an increase in the xanthophyll cycle pool (violaxanthin, antheraxanthin, and zeaxanthin; Figure 6B) and in lutein on a chlorophyll basis (Figure 6C). β-carotene relative to chlorophyll exhibited only a modest increase with increasing growth PPFD (Figure 6C). The carotenoid that exhibited the strongest increase with growth PPFD on a chlorophyll basis was, again, zeaxanthin (Figure 6A).
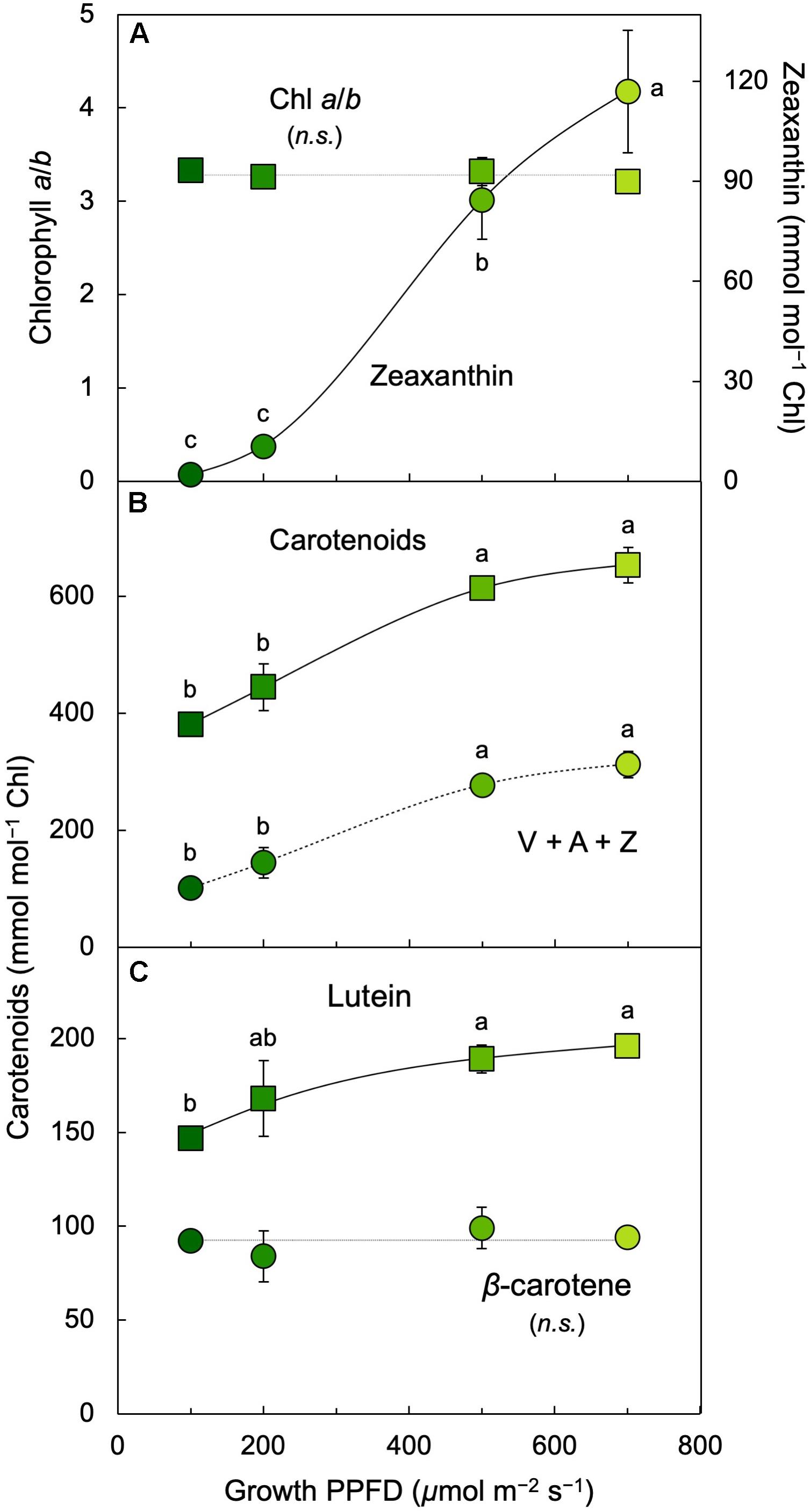
Figure 6. (A) Chlorophyll a/b ratio (squares) and zeaxanthin per chlorophyll (Chl; circles), (B) total carotenoids (sum of V, A, Z, lutein, β-carotene, and neoxanthin; squares) and xanthophyll cycle pool (V + A + Z; circles) per chlorophyll, and (C) lutein (squares) and β-carotene (circles) per chlorophyll in L. gibba as a function of growth PPFD. A, antheraxanthin; V, violaxanthin; Z, zeaxanthin. Mean values ± standard deviations, n = 3 for all growth PPFDs; different lower-case letters signify statistical differences at P < 0.05 via one-way analysis of variance and post hoc Tukey–Kramer HSD test (n.s., not significantly different).
Photosynthetic Capacity, Light-Use Efficiency, Energy Dissipation, and Excess Excitation
Light-and CO2-saturated photosynthetic capacity on a frond area basis was not significantly different between 100 and 700 μmol photons m–2 s–1 (Figure 7A) and neither was dry mass per leaf area (see legend of Figure 7). To probe whether the absence of a PPFD-dependent increase in photosynthetic capacity may be associated with starch accumulation, an iodine starch test was performed. This test indicated modest starch accumulation in fronds grown under 100 μmol photons m–2 s–1 (Figure 7B) and strong starch accumulation in fronds grown under PPFDs between 200 and 700 μmol m–2 s–1 (Figures 7C–E).
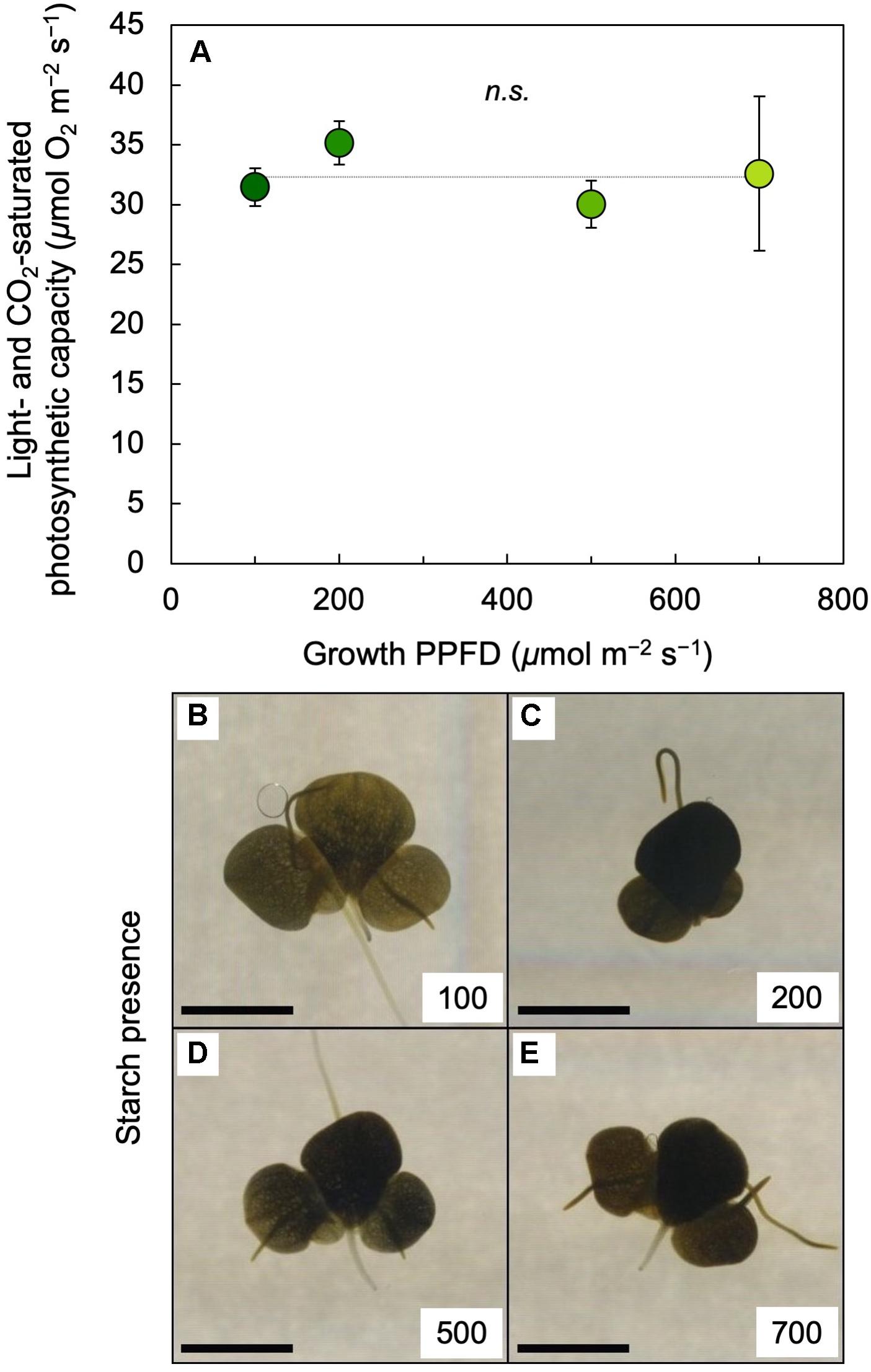
Figure 7. (A) Light- and CO2-saturated rate of oxygen evolution determined at 25°C and (B through E) iodine stain (as a qualitative test for starch presence) of L. gibba fronds as a function of growth PPFD. The numbers (100 to 700) in each of the panels B through E correspond to the PPFD (in μmol photons m–2 s–1) under which fronds were grown; the scale bars represent 5 mm. In A, mean values ± standard deviations, n = 3 for all growth PPFDs; n.s., not significantly different at P < 0.05 via one-way analysis of variance. Mean values ± standard deviations for frond dry mass per area (g m–2) were 26.4 ± 2.2, 37.4 ± 5.5, 37.9 ± 5.3, and 37.8 ± 3.8 for growth PPFDs of 100, 200, 500, and 700 μmol m–2 s–1, respectively (n = 3 for all growth PPFDs).
The photochemical efficiency of those PSII centers that remained open at each growth PPFD (Fv′/Fm′) declined with increasing growth PPFD (Figure 8A), which indicates increasing removal of excess excitation energy by photoprotective thermal energy dissipation (see Figure 3). The actual fraction of PSII centers that are closed (reduced) under each growth PPFD, 1 – qP, increased with increasing growth PPFD up to 500 μmol photons m–2 s–1, but did not increase further under 700 μmol photons m–2 s–1 (Figure 8B). Photochemical efficiency at the actual percentage of closed PSII centers under each respective growth PPFD (Fv′/Fm′ × qP) decreased strongly with increasing growth PPFD (Figure 8B). Potential maximal PSII photochemical efficiency (Fv/Fm) of fronds darkened for 5 min immediately upon removal from growth PPFD (− Recovery) exhibited a moderate decline as a function of growth PPFD, but rebounded quickly over 30 min of recovery (+ Recovery) in low light (Figure 8C). The resulting high dark Fv/Fm levels in L. gibba after this brief recovery period indicate an absence of photoinhibitory inactivation of photochemistry, which is consistent with L. gibba’s continuously high growth rates (Figures 2, 4). Figure 9 shows that dark Fv/Fm increased in proportion to removal of zeaxanthin (expressed as zeaxanthin level relative to the total xanthophyll cycle pool), indicating that the residual minor depression of dark Fv/Fm after 30 min of recovery is due to some sustained thermal energy dissipation. Since this residual minor depression in dark Fv/Fm precludes quantification of energy dissipation from the degree of quenching of maximal fluorescence in light versus darkness (Adams and Demmig-Adams, 2004; Adams et al., 2013, 2014; Logan et al., 2014), alternative approaches (see Figure 3 and section “Materials and Methods”) were used to estimate energy dissipation activity (Figure 10).
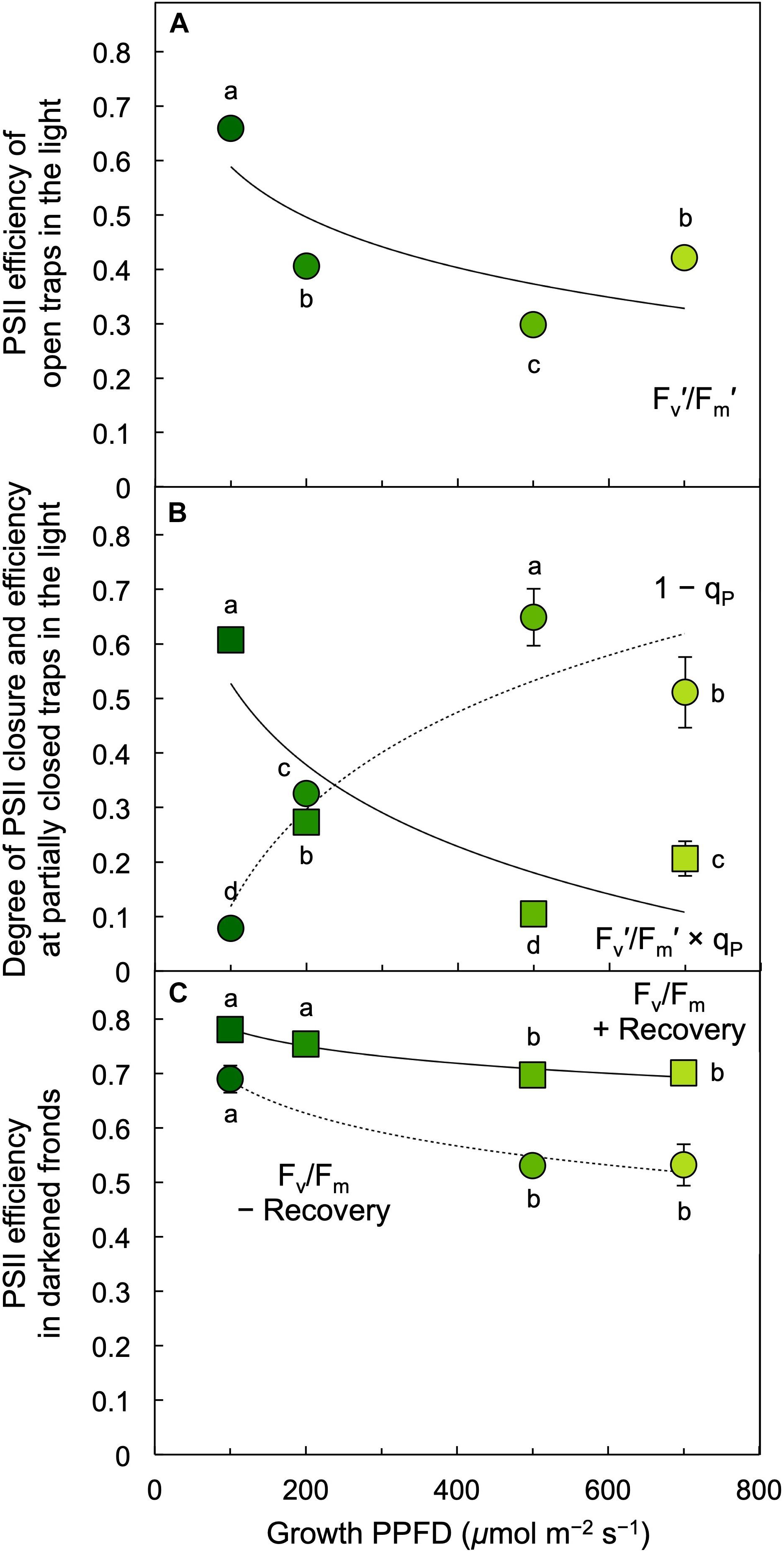
Figure 8. (A) Efficiency of open photosystem II (PSII) centers (traps) during exposure to each respective growth PPFD, (B) fraction of PSII reaction centers (traps) that are closed, 1 – qP = (F – Fo′)/(Fm′ – Fo′) (circles), and PSII efficiency at the degree of center closure (Fv′/Fm′ × qP; squares) under each respective growth PPFD, and (C) the efficiency of PSII in the dark (Fv/Fm) in L. gibba fronds grown under each respective PPFD. Dark Fv/Fm was determined immediately upon removal of fronds from growth light conditions (– Recovery) and again after 30 min in low light (+ Recovery). Fm′, maximal fluorescence under actinic light; Fv′, variable fluorescence under actinic light (Fm′ – minimal fluorescence Fo′); PSII, photosystem II; qP, photochemical quenching. Mean values ± standard deviations, n = 3 for all growth PPFDs; different lower-case letters signify statistical differences at P < 0.05 via one-way analysis of variance and post hoc Tukey–Kramer HSD test.
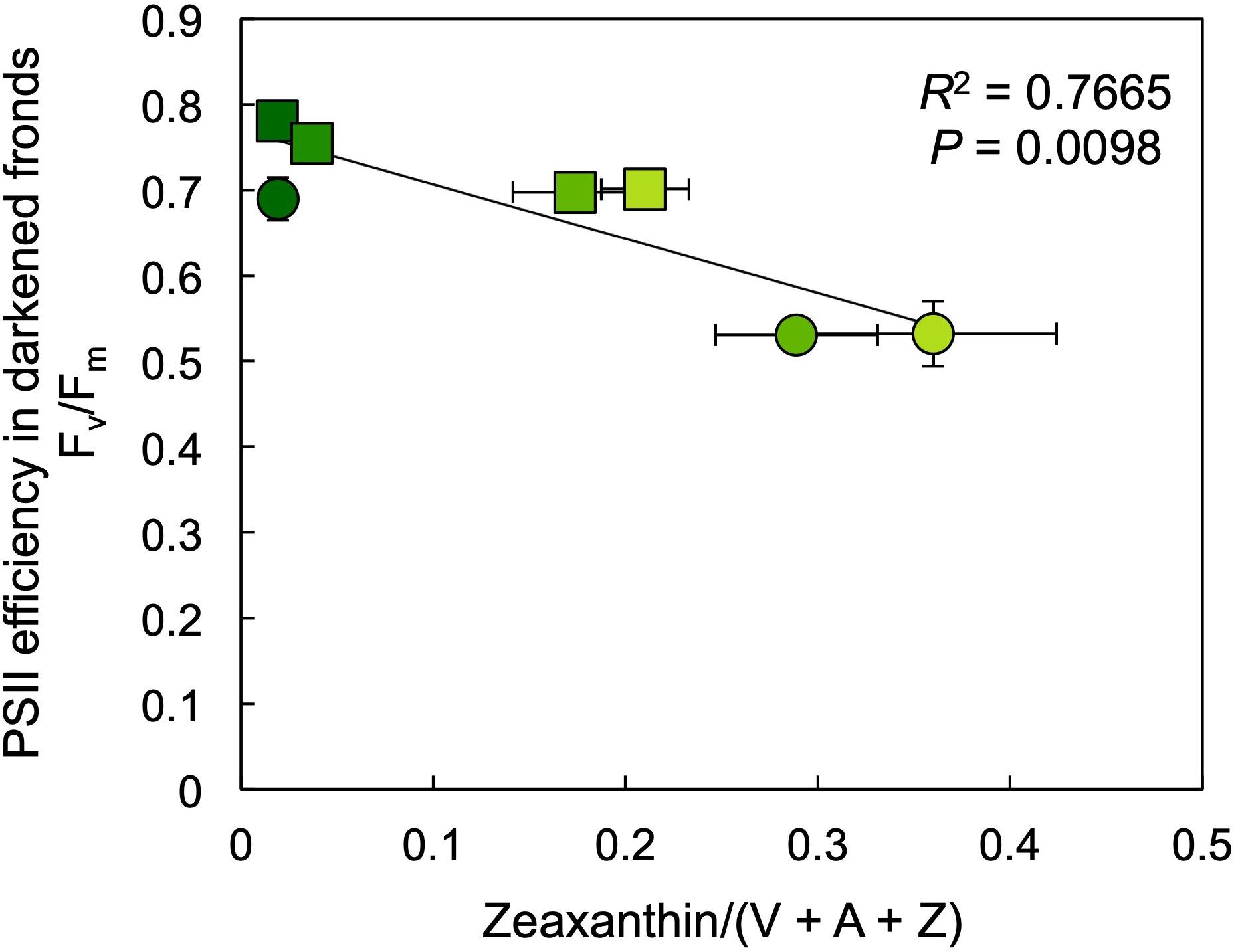
Figure 9. Relationship between the efficiency of open photosystem II (PSII) centers, Fv/Fm, and zeaxanthin content (as a fraction of the total xanthophyll cycle pool, violaxanthin [V], antheraxanthin [A], and zeaxanthin [Z]) in samples collected either immediately (circles) or after a recovery period of 30 min under a low PPFD of 10 μmol m–2 s–1 (squares) subsequent to removal from growth PPFDs. Fm, maximal fluorescence in the dark; Fv, variable fluorescence in the dark (Fm – minimal fluorescence in the dark, Fo). Mean values ± standard deviations, n = 3; analyzed statistically via linear regression.
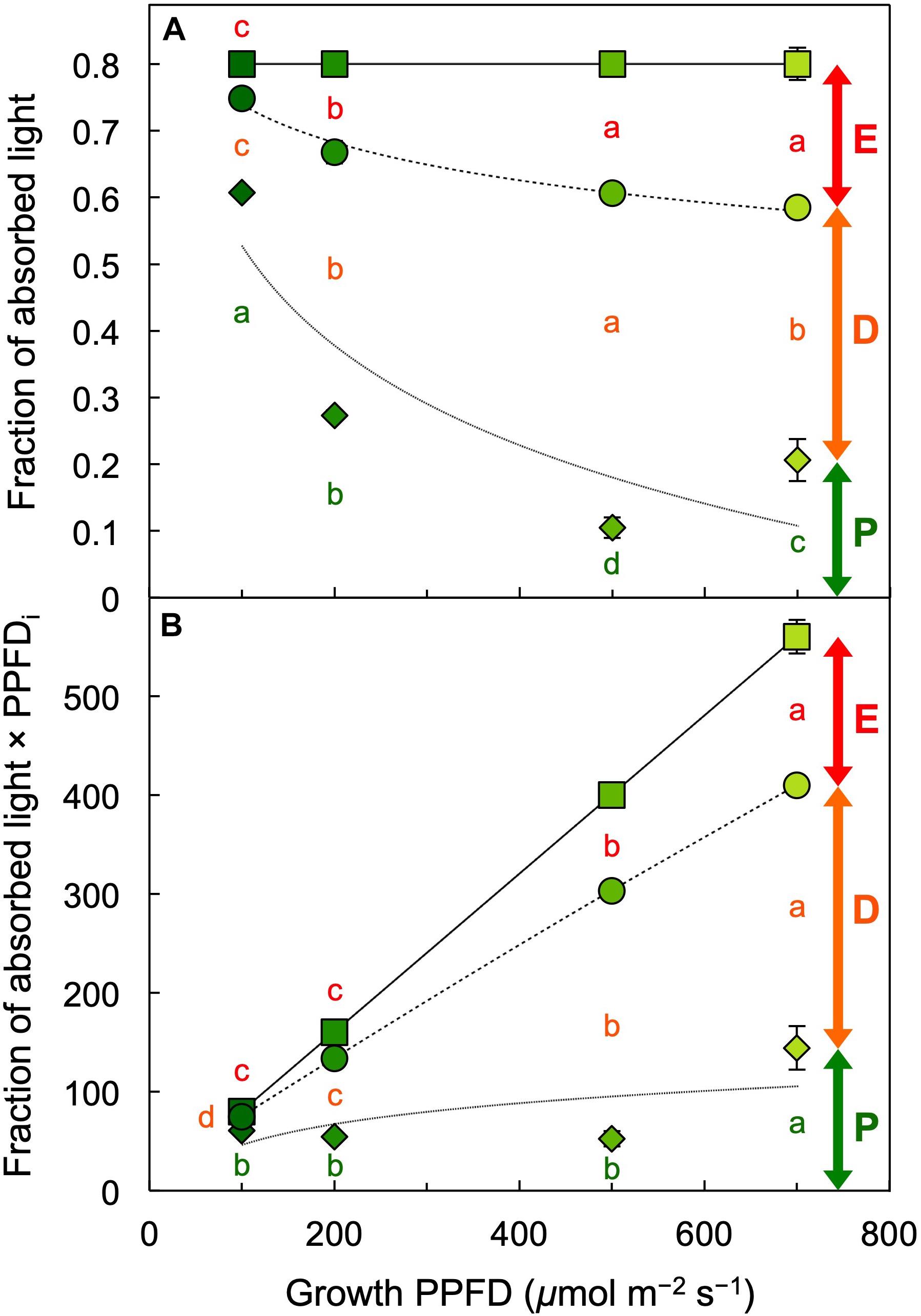
Figure 10. (A) Fraction of absorbed light utilized in photochemistry (P, green span labeled for 700 μmol photons m–2 s–1), thermally dissipated (D, orange span labeled for 700 μmol photons m–2 s–1), and in excess (E, red span labeled for 700 μmol photons m–2 s–1) and (B) the product of the latter fractions and photosynthetic photon flux density incident (PPFDi) upon L. gibba fronds as a function of growth PPFD. See the section “Materials and Methods” and Demmig-Adams et al. (1996a) for calculations. Mean values ± standard deviations, n = 3 for all growth PPFDs; different lower-case letters signify statistical differences at P < 0.05 via one-way analysis of variance and post hoc Tukey–Kramer HSD test.
Figure 10 provides a comprehensive accounting of absorbed photons and their pathways in the photochemical system of L. gibba as a function of growth PPFD. The percentage of absorbed photons utilized in photochemistry (P) was highest in fronds grown under 100 μmol photons m–2 s–1 and declined with increasing growth PPFD (P in Figure 10A), which is consistent with the pronounced decline in light-use efficiency of frond area production as a function of growth PPFD (Figure 4C). In the absence of photoinhibitory inactivation of photosynthesis in these plants, the fraction of absorbed photons dissipated in photoprotective thermal energy dissipation (D) under each respective growth PPFD can be assessed as 0.8 – Fv′/Fm′, which increased strongly and then plateaued with increasing growth PPFD (D in Figure 10A). The fraction of absorbed photons used neither in photochemistry nor dissipated thermally (E for Excess), Fv′/Fm′ × (1 – qP), increased only modestly and then also plateaued (E in Figure 10A).
In contrast to the fraction of photons, the actual number of photons entering the three possible pathways continues to increase with increasing absorption of light under increasing growth PPFD. Multiplication of each fraction of absorbed photons × the total number of photons absorbed at each growth PPFD would provide numbers of photons entering each pathway (P, D, and E). The total number of absorbed photons is, however, not available since absorptance measurements in these fronds could not separate photons into those absorbed by chlorophyll versus those absorbed by the yellow (carotenoid) pigments that do not transfer photons to photochemical reaction centers. To illustrate the concept of increasing rates of P, D, and E with increasing growth PPFD, Figure 10B shows the product of incident PPFD (PPFDi) × the fractions of absorbed photons going into pathways P, D, and E from Figure 10A. The declining chlorophyll a + b levels with increasing growth PPFD (Figures 2, 5A) presumably cause photon absorption to deviate increasingly from incident PPFD, and true rates of P, D, and E will thus be proportionally lower. Nevertheless, the estimated rate of thermal energy dissipation shown in Figure 10B provides the expected match for the level of the dissipater zeaxanthin on a chlorophyll basis (Figure 6A), with both parameters exhibiting continued increases with increasing growth PPFD. This result is also consistent with the close correlation between zeaxanthin level and dark Fv/Fm (Figure 9) subsequent to removal from growth PPFD.
Discussion
This study addressed the impact of growth light intensity on plant growth and photosynthesis as well as on the concentrations of photosynthetic pigments with nutritional value for the human consumer. We focused on select vitamins and other essential antioxidant metabolites with well-documented health benefits that are (i) diet-derived nutrients for the human consumer, (ii) act synergistically with each other in both plants and animals, and (iii) are upregulated in plants in response to environmental cues (Grace and Logan, 1996; Logan et al., 1996, 1998a,b; Demmig-Adams and Adams, 2002; Adams et al., 2016). Humans require a number of antioxidant metabolites that cannot be synthesized in the human body and must be supplied by the diet, preferably via consumption of whole foods. Future studies should address the effect of growth light intensity on additional antioxidants with relevance to human nutrition, such as vitamins C and E, and phenolics.
Maintenance of Similar Growth Rates Over an Extreme Range of Light Environments
The results reported here demonstrate that L. gibba is able to achieve a remarkably high growth rate and light-use efficiency of plant growth under low growth light intensity. Lemna gibba achieves this high growth rate by superior light absorption – due to a combination of apparent minimal self-shading in the arrangement of its leaves and high chlorophyll levels under low light. The low levels of self-shading in the thin L. gibba leaves represent a natural adaptation that favors high light-use efficiency and is an alternative to current efforts to engineer crops with truncated chlorophyll antennae by way of reduced levels of outer chlorophyll antennae Lhcb (Ort et al., 2015; Kirst et al., 2017, 2018; Song et al., 2017).
Conversely, L. gibba’s growth rate also remained remarkably high even under a growth PPFD that supplied a total amount of photons per day similar to that received on the brightest and longest day of the year on Earth. One can consider this maintenance of high growth rates as evidence for a high degree of plant phenotypic plasticity, or robustness, of L. gibba with respect to extreme variation in the environment. It should be noted that L. gibba maintained these high growth rates under very high growth PPFDs despite the minimal self-shading in its relatively thin leaves. Self-shading in thick, high-light-grown leaves, as well as the overlapping nature of leaves in the canopy, of other plant species offers some structural photoprotection against excess light. The ability of L. gibba to avoid photoinhibition of photosynthesis under high growth PPFDs despite possessing thin leaves is thus a remarkable quality, which is apparently based on a combination of strong downregulation of chlorophyll synthesis and strongly increased biochemical photoprotection that kept excess excitation energy from building up beyond a modest level. This pronounced biochemical photoprotection included high levels of thermal dissipation of excitation energy, accumulation and retention of zeaxanthin in the amount of up to a third of the xanthophyll cycle pool at the highest growth PPFD for a period of 30 min in low PPFD, and accumulation of lutein. García-Plazaola et al. (2002) likewise found that L. minor strongly upregulated the pool of xanthophyll cycle pigments when grown under higher versus low PPFD and synthesized additional zeaxanthin when transferred to an even higher PPFD for several hours. The ability to sustain high area growth over a wide range of light environments is presumably advantageous for a floating plant like duckweed that occurs both in open ponds and areas around the edge of ponds that may be shaded by emergent macrophytes (e.g., cattails, sedges), overhanging terrestrial vegetation (e.g., willows, cottonwoods), or man-made structures (docks, bridges, etc.). Rapid coverage, and shading, of a pond by duckweed presumably serves to discourage the growth of algae that compete for nutrients.
High Phenotypic Plasticity With Respect to Highly Excessive Light
Lutein is abundant in all leaves even under low growth PPFDs because it is not a competitor with photochemistry; lutein detoxifies a triplet excited state of chlorophyll not used for photochemistry (Dall’Osto et al., 2006). In contrast, zeaxanthin is a direct competitor for photochemistry and de-excites the same singlet excited state of chlorophyll used for photochemistry (Demmig-Adams and Adams, 1992; Park et al., 2018, 2019). Several regulatory mechanisms ensure that zeaxanthin is formed in leaves only under excess light and is typically removed quickly upon return to non-excessive light levels (Demmig-Adams et al., 1996b).
The declining chlorophyll content and increase in the proportion of individual carotenoids relative to chlorophyll at high growth PPFD were consistent with some downregulation of the outer chlorophyll light-harvesting complex that maximizes light absorption in low light (Lhcb; the major Chl [a+b]- and lutein-binding light-harvesting complex) and with the role of lutein in Chl triplet de-excitation in Lhcb (Dall’Osto et al., 2006) as well as some downregulation of photosystem-core antenna complexes (that bind β-carotene). The strong accumulation of zeaxanthin despite the decline in chlorophyll level is consistent with localization of important zeaxanthin-binding sites with roles in thermal energy dissipation (Park et al., 2018, 2019) in linker proteins between photosystems and their outer Lhcb complexes. Under the highest growth PPFDs, additional zeaxanthin may be located in the lipid fraction of the photosynthetic membrane, where it can contribute to direct protection of membrane lipids (Havaux and García-Plazaola, 2014) in synergistic interaction with vitamin E (Wrona et al., 2003, 2004; Schneider, 2005). The findings of the present study thus confirm that zeaxanthin production in L. gibba requires very high growth PPFDs, while production of both lutein and β-carotene on a frond area basis declined somewhat at the highest growth PPFDs used here. β-carotene can serve as an antioxidant in both plants (Telfer, 2014) and animals (Stahl and Sies, 2012) and is, furthermore, the precursor (pro-vitamin A) in the synthesis of vitamin A, the precursor of the light-absorbing (retinal) component of vision purple in the human eye (Polutchko et al., 2015).
Coupling of chlorophyll downregulation and upregulation of zeaxanthin-associated photoprotection by L. gibba under excess levels of light combines two mechanisms with different features. The downregulation of chlorophyll content under high growth PPFDs cannot be reversed on short time scales of minutes to hours. However, rapidly reversible, thermal dissipation of excess excitation offers a high degree of flexibility with simultaneous strong photoprotection and a quick resumption of high light-use efficiency upon return to limiting light. The combination of these two biochemical adjustments – modulation of light-harvesting capacity/chlorophyll content and modulation of carotenoid levels and energy dissipation (assessed from Fv′/Fm′) – was apparently potent enough to (i) effectively limit build-up of excess excitation as well as prevent PSII centers from closing entirely even at the highest growth PPFDs and (ii) preserve the ability to quickly return to high PSII efficiency upon transfer to low light.
The concomitant changes in zeaxanthin content (and xanthophyll cycle conversion state to zeaxanthin) with changes in PSII efficiency are consistent with the well-documented role of zeaxanthin in thermal dissipation of excess absorbed light (Demmig-Adams and Adams, 1996, 2006; Demmig-Adams et al., 2012, 2014; Park et al., 2018, 2019). Theory predicts that the relationship between the absolute zeaxanthin level and the fraction of absorbed photons dissipated by zeaxanthin as thermal energy is curvilinear since the fraction of absorbed photons dissipated thermally is constrained to a maximum of 80% of absorbed photons. In contrast, zeaxanthin level and the rate constant of thermal dissipation both increase linearly with the absolute amount of photons dissipated thermally (Demmig-Adams et al., 1989a, b). A linear relationship between the rate constant of thermal energy dissipation and zeaxanthin level on a dry mass basis was empirically observed in leaves of several plant species by Demmig-Adams et al. (1989a, b).
Source-Sink Balance in Duckweed
Duckweed’s storage capacity for photosynthetically produced sugars is presumably limited by the virtual absence of non-green parts that can serve as major sinks for sugars in other plant species (Adams et al., 2014, 2018). This absence of non-green parts is what makes duckweed near-100% edible. Furthermore, this scenario may be the reason for duckweed’s prolific growth of new fronds as the species’ sole or main sink for sugars and the associated fast area growth and doubling times of duckweed species (Sree et al., 2015; Ziegler et al., 2015). The finding that maximal photosynthetic capacity did not increase with increasing growth PPFD between 100 and 700 μmol photons m–2 s–1 is consistent with sink limitation and starch accumulation as factors counteracting photosynthetic upregulation as part of a feedback loop that regulates photosynthesis by the demand for carbohydrate from the rest of the plant (Adams et al., 2013, 2014, 2018; Demmig-Adams et al., 2017). However, it is noteworthy that, despite the accumulation of starch, there was neither downregulation of photosynthetic capacity (see also McLaren and Smith, 1976) nor any decrease in area growth rate with increasing growth PPFD up to the highest growth PPFD. As stated above, high area growth rates are presumably ecologically advantageous for a floating plant like duckweed that benefits from an ability to rapidly cover large areas of water.
The Next Step in Duckweed Agriculture for Space Exploration
Compared to the light levels needed for remarkably high rates of L. gibba photosynthesis and growth, much higher light levels are required for strong induction of the essential antioxidant zeaxanthin. A similar relationship has been demonstrated in many other plant species for this relationship between light level needed to saturate photosynthesis and induction of strong zeaxanthin formation (see, e.g., Demmig-Adams et al., 1989a) as well as induction of strong accumulation of the antioxidant vitamins C and E and the enzymatic antioxidant glutathione (Grace and Logan, 1996; Logan et al., 1996). Excessively high growth light intensity (see Seginer et al., 2006 for lettuce) or other environmental stressors, on the other hand, can drive up excitation pressure to a level that causes growth reductions and photoinhibition (see, e.g., Adams et al., 2013) and even antioxidant destruction by light stress (see Havaux and García-Plazaola, 2014). Therefore, the challenge in designing light protocols for simultaneous optimization of plant yield, nutritional quality, and resource-use efficiency is that light-use efficiency of plant growth is maximal under low light input, while production of zeaxanthin and other essential antioxidants requires high light. In applications where the cost of light-energy input is not an issue, plants could be grown under high PPFD. However, if light input is an issue, the input of 600% more light to attain a 25% greater relative growth rate (as seen at 700 versus 100 μmol photons m–2 s–1) may not be justifiable.
Insight from plant ecophysiology can offer solutions for co-optimization of plant yield, energy-use efficiency, and nutritional quality. We discovered (Adams et al., 1999) that plants growing in the shaded understorey of a forest periodically punctuated by shafts of full sunlight (sunflecks penetrating the canopy) produce and continuously retain considerable amounts of zeaxanthin. Based on this finding, we used a combination of low background light intensity and a few high-light pulses in climate-controlled growth chambers to simultaneously keep energy-use efficiency high and yet produce and retain zeaxanthin under low background light. We obtained proof-of-concept for the validity of this approach with the model species Arabidopsis thaliana (Cohu et al., 2014). The latter study offered the conclusion that “growth light environment … can be exploited to simultaneously optimize nutritional quality … as well as biomass production of leafy greens suitable as bioregenerative systems for long-duration manned spaceflight missions.” It may thus be possible to minimize, or circumvent altogether, the trade-off between light-use efficiency of growth and nutritional quality with this novel growth protocol to co-optimize all desired features.
Data Availability Statement
The data presented in this manuscript are available from the corresponding author upon reasonable request.
Author Contributions
BD-A, CE, and WA wrote the grant on which this study is based. JS carried out the experiments, biochemical assays, and statistical analyses with contributions from CE, BD-A, ML-P, and WA. BD-A wrote the manuscript with contributions from WA, JS, and CE.
Funding
This work was supported by the Translational Research Institute for Space Health through Cooperative Agreement NNX16AO69A and by the University of Colorado.
Conflict of Interest
CE has financial interest in Space Lab Technologies, LLC, a company that may be affected by this research. A Memorandum of Understanding approved by the University of Colorado manages potential conflicts arising from this relationship.
The remaining authors declare that the research was conducted in the absence of any commercial or financial relationships that could be construed as a potential conflict of interest.
Acknowledgments
We thank Dr. Adam Escobar for valuable discussion and feedback, Mr. Paul Fourounjian for valuable contributions, and Ms. Naiara Doherty Garcia and Ms. Gabrielle Glime for assistance with data collection.
Footnotes
References
Adams, M. S., Adams, R. B., Wessman, C. A., and Demmig-Adams, B. (2016). Nutritional cues tie living organisms to their environment and its sustainability. Front. Nutr. 3:28. doi: 10.3389/fnut.2016.00028
Adams, W. W. III, and Demmig-Adams, B. (2004). “Chlorophyll fluorescence as a tool to monitor plant response to the environment,” in Chlorophyll a Fluorescence: A Signature of Photosynthesis. Advances in Photosynthesis, Vol. 19, eds G. C. Papageorgiou, and Govindjee (Dordrecht: Springer), 583–604. doi: 10.1007/978-1-4020-3218-9_22
Adams, W. W. III, Demmig-Adams, B., Logan, B. A., Barker, D. H., and Osmond, C. B. (1999). Rapid changes in xanthophyll cycle-dependent energy dissipation and photosystem II efficiency in two vines, Stephania japonica and Smilax australis, growing in the understory of an open Eucalyptus forest. Plant Cell Environ. 22, 125–136. doi: 10.1046/j.1365-3040.1999.00369.x
Adams, W. W. III, Muller, O., Cohu, C. M., and Demmig-Adams, B. (2013). May photoinhibition be a consequence, rather than a cause, of limited plant productivity? Photosynth. Res. 117, 31–44. doi: 10.1007/s11120-013-9849-7
Adams, W. W. III, Muller, O., Cohu, C. M., Demmig-Adams, B. (2014). “Photosystem II efficiency and non-photochemical quenching in the context of source-sink balance,” in Non-Photochemical Quenching and Energy Dissipation in Plants, Algae and Cyanobacteria. Advances in Photosynthesis and Respiration, Vol. 40, eds B. Demmig-Adams, G. Garab, and W. W. Adams III, and Govindjee (Dordrecht: Springer), 503–529. doi: 10.1007/978-94-017-9032-1_23
Adams, W. W. III, Stewart, J. J., and Demmig-Adams, B. (2018). “Photosynthetic modulation in response to plant activity and environment,” in The Leaf: A Platform for Performing Photosynthesis. Advances in Photosynthesis and Respiration, Vol. 44, eds W. W. Adams and III I. Terashima (Cham: Springer), 493–563. doi: 10.1007/978-3-319-93594-2_18
Appenroth, K.-J., Augsten, H., Liebermann, B., and Feist, H. (1982). Effects of light quality on amino acid composition of proteins in Wolffia arrhiza (L.) Wimm. using a specially modified Bradford method. Biochem. Physiol. Pflanz. 177, 251–258. doi: 10.1016/S0015-3796(82)80008-5
Appenroth, K.-J., Sree, K. S., Bog, M., Ecker, J., Seeliger, C., Böhm, V., et al. (2018). Nutritional value of the duckweed species of the genus Wolffia (Lemnaceae) as human food. Front. Chem. 6:483. doi: 10.3389/fchem.2018.00483
Appenroth, K.-J., Sree, S., Vöhm, V., Hammann, S., Vetter, W., Leiterer, M., et al. (2017). Nutritional value of duckweeds (Lemnaceae) as human food. Food Chem. 217, 266–273. doi: 10.1016/j.foodchem.2016.08.116
Asker, D., Beppu, T., and Ueda, K. (2007). Unique diversity of carotenoid-producing bacteria isolated from Misasa, a radioactive site in Japan. Appl. Microbiol. Biotechnol. 77, 383–392. doi: 10.1007/s00253-007-1157-8
Azzam, E. I., Jay-Gerin, J.-P., and Pain, D. (2012). Ionizing radiation-induced metabolic oxidative stress and prolonged cell injury. Cancer Lett. 327, 48–60. doi: 10.1016/j.canlet.2011.12.012
Bluem, V., and Paris, F. (2001). Aquatic modules for bioregenerative life support systems based on the C.E.B.A.S. biotechnology. Acta Astronaut. 48, 5–12. doi: 10.1016/S0094-5743(01)00025-X
Burch, T. A. (2016). The Compatible Solute Glycerol as a Photosynthetic Sink and Energy Carrier in Freshwater and Marine Chlamydomonas. Ph.D. dissertation, University of Colorado, Boulder, CO.
Chen, G., Fang, Y., Huang, J., Zhao, Y., Li, Q., Lai, F., et al. (2018). Duckweed systems for eutrophic water purification through converting wastewater nutrients to high starch biomass: comparative evaluation of three different genera (Spirodela polyrhiza, Lemna minor and Landoltia punctata) in monoculture or polyculture. RSC Adv. 8, 17927–17937. doi: 10.1039/c8ra0156a
Cohu, C. M., Lombardi, E., Adams, W. W. III, and Demmig-Adams, B. (2014). Increased nutritional quality of plants for long-duration spaceflight missions through choice of plant variety and manipulation of growth conditions. Acta Astronaut. 94, 799–806. doi: 10.1016/j.actaastro.2013.10.009
Dall’Osto, L., Lico, C., Alric, J., Giuliano, G., Havaux, M., and Bassi, R. (2006). Lutein is needed for efficient chlorophyll triplet quenching in the major LHCII antenna complex of higher plant and effective photoprotection in vivo under strong light. BMC Plant Biol. 6:32. doi: 10.1186/1471-2229-6-32
Delieu, T., and Walker, D. A. (1981). Polarographic measurement of photosynthetic oxygen evolution by leaf discs. New Phytol. 89, 165–178. doi: 10.1111/j.1469-8137.1981.tb07480
Demmig-Adams, B., and Adams, R. B. (2013). Eye nutrition in context: Mechanisms, implementation, and future directions. Nutrients 5, 2483–2501. doi: 10.3390/nu5072483
Demmig-Adams, B., and Adams, W. W. III (1992). Photoprotection and other responses of plants to high light stress. Annu. Rev. Plant Physiol. Plant Mol. Biol. 43, 599–626. doi: 10.1146/annurev.arplant.43.1.599
Demmig-Adams, B., and Adams, W. W. III (1996). The role of xanthophyll cycle carotenoids in the protection of photosynthesis. Trends Plant Sci. 1, 21–26. doi: 10.1016/S1360-1385(96)80019-7
Demmig-Adams, B., and Adams, W. W. III (2002). Antioxidants in photosynthesis and human nutrition. Science 298, 2149–2153. doi: 10.1126/science.1078002
Demmig-Adams, B., and Adams, W. W. III (2006). Photoprotection in an ecological context: the remarkable complexity of thermal energy dissipation. New Phytol. 172, 11–21. doi: 10.1111/j.1469-8137.2006.01835.x
Demmig-Adams, B., Cohu, C. M., Amiard, V., van Zadelhoff, G., Veldink, G. A., Muller, O., et al. (2013). Emerging trade-offs – Impact of photoprotectants (PsbS, xanthophylls, and vitamin E) on oxylipins as regulators of development and defense. New Phytol. 197, 720–729. doi: 10.1111/nph.12100
Demmig-Adams, B., Cohu, C. M., Muller, O., and Adams, W. W. III (2012). Modulation of photosynthetic energy conversion efficiency in nature: from seconds to seasons. Photosynth. Res. 113, 75–88. doi: 10.1007/s11120-012-9761-6
Demmig-Adams, B., Adams, W. W. III, Barker, D. H., Logan, B. A., Bowling, D. L., and Verhoeven, A. S. (1996a). Using chlorophyll fluorescence to assess the fraction of absorbed light allocated to thermal dissipation of excess excitation. Physiol. Plant. 98, 253–264. doi: 10.1034/j.1399-3054.1996.980206.x
Demmig-Adams, B., Gilmore, A., and Adams, W. W. III (1996b). Carotenoids 3: in vivo functions of carotenoids in higher plants. FASEB J. 10, 403–412. doi: 10.1096/fasebj.10.4.8647339
Demmig-Adams, B., Koh, S. C., Cohu, C. M., Muller, O., Stewart, J. J., and Adams, W. W. III (2014). “Non-photochemical quenching in contrasting plant species and environments,” in Non-Photochemical Quenching and Energy Dissipation in Plants, Algae and Cyanobacteria. Advances in Photosynthesis and Respiration, Vol. 40, eds B. Demmig-Adams, G. Garab, and W. W. Adams III (Dordrecht: Springer), 531–552. doi: 10.1007/978-94-017-9032-1_24
Demmig-Adams, B., Stewart, J. J., and Adams, W. W. III (2017). Environmental regulation of maximal photosynthetic capacity. Curr. Opin. Plant Biol. 37, 34–41. doi: 10.1016/j.pbi.2017.03.008
Demmig-Adams, B., Winter, K., Krüger, A., and Czygan, F.-C. (1989a). Light response of CO2 assimilation, dissipation of excess excitation energy, and zeaxanthin content of sun and shade leaves. Plant Physiol. 90, 881–886. doi: 10.1104/pp.90.3.881
Demmig-Adams, B., Winter, K., Krüger, A., and Czygan, F.-C. (1989b). Zeaxanthin and the induction and relaxation kinetics of the dissipation of excess excitation energy in leaves in 2% O2, 0% CO2. Plant Physiol. 90, 887–893. doi: 10.1104/pp.90.3.887
Dumlao, M. R., Darehshouri, A., Cohu, C. M., Muller, O., Mathias, J., and Adams, W. W. III, et al. (2012). Low temperature acclimation of photosynthetic capacity and leaf morphology in the context of phloem loading type. Photosynth. Res. 113, 181–189. doi: 10.1007/s11120-012-9762-5
Gale, J., Smernoff, D. T., Macler, B. A., and MacElroy, R. D. (1989). Carbon balance and productivity of Lemna gibba, a candidate plant for CELSS. Adv. Space Res. 9, 43–52. doi: 10.1016/0273-1177(89)90027-6
García-Plazaola, J. I., Hernández, A., Artetxe, U., and Becerril, J. M. (2002). Regulation of the xanthophyll cycle pool size in duckweed (Lemna minor) plants. Physiol. Plant. 116, 121–126. doi: 10.1034/j.1399-3054.2002.1160115.x
Genty, B., Briantais, J.-M., and Baker, N. R. (1989). The relationship between the quantum yield of photosynthetic electron transport and quenching of chlorophyll fluorescence. Biochim. Biophys. Acta 990, 87–92. doi: 10.1016/S0304-4165(89)80016-9
Grace, S. C., and Logan, B. A. (1996). Acclimation of foliar antioxidant systems to growth irradiance in three broad-leaved evergreen species. Plant Physiol. 112, 1631–1640. doi: 10.1104/pp.112.4.1631
Havaux, M., García-Plazaola, J. I. (2014). “Beyond non-photochemical quenching: the overlapping antioxidant function of zeaxanthin and tocopherols,” in Non-Photochemical Quenching and Energy Dissipation in Plants, Algae and Cyanobacteria. Advances in Photosynthesis and Respiration, Vol. 40, eds B. Demmig-Adams, G. Garab, and W. W. Adams III, and Govindjee (Dordrecht: Springer), 583–603. doi: 10.1007/978-94-017-9032-1_26
Hunt, R. (ed.). (1990). “Relative growth rates,” in Basic Growth Analysis: Plant Growth Analysis for Beginners, (Dordrecht: Springer), 25–34. doi: 10.1007/978-94-010-9117-6_3
Iatrou, E. I., Kora, E., and Stasinakis, A. S. (2019). Investigation of biomass production, crude protein and starch content in laboratory wastewater treatment systems planted with Lemna minor and Lemna gibba. Environ. Technol. 40, 2649–2656. doi: 10.1080/09593330.2018.1448002
Kirst, H., Gabilly, S. T., Niyogi, K. K., Lemaux, P. G., and Melis, A. (2017). Photosynthetic antenna engineering to improve crop yields. Planta 245, 1009–1020. doi: 10.1007/s00425-017-2659-y
Kirst, H., Shen, Y., Vamvaka, E., Betterle, N., Xy, D., Warek, U., et al. (2018). Downregulation of the CpSRP43 gene expression confers a truncated light-harvesting antenna (TLA) and enhances biomass and leaf-to-stem ratio in Nicotiana tabacum canopies. Planta 248, 139–154. doi: 10.1007/s00425-018-2889-7
Leng, R. A. (1999). Duckweed: A Tiny Aquatic Plant with Enormous Potential for Agriculture and Environment. Rome: FAO.
Liu, Y., Wang, X., Fang, Y., Huang, M., Chen, X., Zhang, Y., et al. (2018). The effects of photoperiod and nutrition on duckweed (Landoltia punctata) growth and starch accumulation. Ind. Crops Prod. 115, 243–249. doi: 10.1016/j.indcrop.2018.02.033
Logan, B. A., Barker, D. H., Demmig-Adams, B., and Adams, W. W. III (1996). Acclimation of leaf carotenoid composition and ascorbate levels to gradients in the light environment within an Australian rainforest. Plant Cell Environ. 19, 1083–1090. doi: 10.1111/j.1365-3040.1996.tb00215.x
Logan, B. A., Demmig-Adams, B., and Adams, W. W. III (1998a). Antioxidants and xanthophyll cycle-dependent energy dissipation in Cucurbita pepo L. and Vinca major L. upon a sudden increase in growth PPFD in the field. J. Exp. Bot. 49, 1881–1888. doi: 10.1093/jxb/49.328.1881
Logan, B. A., Demmig-Adams, B., Adams, W. W. III, and Grace, S. C. (1998b). Antioxidants and xanthophyll cycle-dependent energy dissipation in Cucurbita pepo L. and Vinca major L. acclimated to four growth PPFDs in the field. J. Exp. Bot. 49, 1869–1879. doi: 10.1093/jxb/49.328.1869
Logan, B. A., Demmig-Adams, B., Adams, W. W. III, Bilger, W. (2014). “Context, quantification, and measurement guide for non-photochemical quenching of chlorophyll fluorescence,” in Non-Photochemical Quenching and Energy Dissipation in Plants, Algae and Cyanobacteria. Advances in Photosynthesis and Respiration, Vol. 40, eds B. Demmig-Adams, G. Garab, and W. W. Adams III, and Govindjee (Dordrecht: Springer), 187–201. doi: 10.1007/978-94-017-9032-1_7
McLaren, J. S., and Smith, H. (1976). Effect of abscisic acid on growth, photosynthetic rate and carbohydrate metabolism in Lemna minor L. New Phytol. 76, 11–20. doi: 10.1111/j.1469-8137.1976.tb01433.x
Ort, D. R., Merchant, S. S., Alric, J., Barkan, A., Blankenship, R. E., Bock, R., et al. (2015). Redesigning photosynthesis to sustainably meet global food and bioenergy demand. Proc. Natl. Acad. Sci. U.S.A. 112, 8529–8536. doi: 10.1073/pnas.1424031112
Park, S., Fischer, A. L., Steen, C. J., Iwai, M., Morris, J. M., Walla, P. J., et al. (2018). Chlorophyll-carotenoid excitation energy transfer in high-light-exposed thylakoid membranes investigated by snapshot transient absorption spectroscopy. J. Am. Chem. Soc. 140, 11965–11973. doi: 10.1021/jacs.8b04844
Park, S., Steen, C. J., Lyska, D., Fischer, A. L., Endelman, B., Iwai, M., et al. (2019). Chlorophyll-carotenoid excitation energy transfer and charge transfer in Nannochloropsis oceanica for the regulation of photosynthesis. Proc. Natl. Acad. Sci. U.S.A. 116, 3385–3390. doi: 10.1073/pnas.1819011116
Polutchko, S. K., Stewart, J. J., and Demmig-Adams, B. (2015). “Integrative view of the nutrition of the eye,” in Nutraceuticals and Functional Foods in Human Health and Disease Prevention, eds D. Bagchi, H. G. Preuss, and A. Swaroop (Boca Raton, FL: CRC Press), 407–417. doi: 10.1201/b19308-34
Polyakov, Y. S., Musaev, I., and Polyakov, S. V. (2010). Closed bioregenerative life support systems: applicability to hot deserts. Adv. Space Res. 46, 775–786. doi: 10.1016/j.asr.2010.05.004
Rodriguez-Casado, A. (2016). The health potential of fruits and vegetables phytochemicals: notable examples. Crit. Rev. Food Sci. Nutr. 56, 1095–1107. doi: 10.1080/10408398.2012.755149
Schenk, R. U., and Hildebrandt, A. C. (1972). Medium and techniques for induction and growth of monocotyledonous and dicotyledonous plant cell cultures. Can. J. Bot. 50, 199–204. doi: 10.1139/b72-026
Schneider, C. (2005). Chemistry and biology of vitamin E. Mol. Nutr. Food Res. 49, 7–30. doi: 10.1002/mnfr.200400049
Schneider, C. A., Rasband, W. S., and Eliceiri, K. W. (2012). NIH image to ImageJ: 25 years image analysis. Nat. Methods 9, 671–675. doi: 10.1038/nmeth.2089
Seginer, I., Albright, L. D., and Ioslovich, I. (2006). Improved strategy for a constant daily light integral in greenhouses. Biosyst. Eng. 93, 69–80. doi: 10.1016/j.biosystemseng.2005.09.007
Song, Q., Wang, Y., Qu, M., Ort, D. R., and Zhu, X.-G. (2017). The impact of modifying photosystem antenna size on canopy photosynthetic efficiency – Development of a new canopy photosynthesis model scaling from metabolism to canopy level processes. Plant Cell Environ. 40, 2946–2957. doi: 10.1111/pce.13401
Sree, K. S., Dahse, H.-M., Chandran, J. N., Schneider, B., Jahreis, G., and Appenroth, K. J. (2019). Duckweed for human nutrition: no cytotoxic and no anti-proliferative effects on human cell lines. Plant Food Hum. Nutr. 74, 223–224. doi: 10.1007/s11130-019-00725-x
Sree, K. S., Sudakaran, S., and Appenroth, K. J. (2015). How fast can angiosperms grow? Species and clonal diversity of growth rates in the genus Wolffia (Lemnaceae). Acta Physiol. Plant. 37:204. doi: 10.1007/s11738-015-1951-3
Stahl, W., and Sies, H. (2012). β-Carotene and other carotenoids in protection from sunlight. Am. J. Clin. Nutr. 96, 1179S–1184S. doi: 10.3945/ajcn.112.034819
Stewart, J. J., Adams, W. W. III, Cohu, C. M., Polutchko, S. K., Lombardi, E. M., and Demmig-Adams, B. (2015). Differences in light-harvesting, acclimation to growth light environment, and leaf structural development between Swedish and Italian ecotypes of Arabidopsis thaliana. Planta 242, 1277–1290. doi: 10.1007/s00425-015-2368-3
Telfer, A. (2014). Singlet oxygen production by PSII under light stress: mechanism, detection and the protective role of β-carotene. Plant Cell Physiol. 55, 1216–1223. doi: 10.1093/pcp/pcu040
Tran, E., and Demmig-Adams, B. (2007). Vitamins and minerals: powerful medicine or potent toxins? Nutr. Food Sci. 37, 50–60. doi: 10.1108/00346650710726959
Ward, C. H., and Wilks, S. S. (1963). Use of algae and other plants in the development of life support systems. Am. Biol. Teach. 25, 512–521. doi: 10.2307/4440442
Wilks, S. S. (1964). “Plant systems as long-term flight nourishment sources,” in Proceedings of the Conference on Nutrition in Space and Related Waste Problems (Washington, DC: NASA), 305–310.
Woverton, B. C., and McDonald, R. (1977). Compiled Data on the Vascular Aquatic Plant Program, 1975-1977. Technical Report No. NASA-TM-79511 Washington, DC: NASA.
Wrona, M., Korytowksi, W., Różanowska, M., Sarna, T., and Truscott, T. G. (2003). Cooperation of antioxidants in protection against photosensitized oxidation. Free Radic. Biol. Med. 35, 1319–1329. doi: 10.1016/j.freeradbiomed.2003.07.005
Wrona, M., Różanowska, M., and Sarna, T. (2004). Zeaxanthin in combination with ascorbic acid or α-tocopherol protects APRE-19 cells against photosensitized peroxidation of lipids. Free Radic. Biol. Med. 36, 1094–1101. doi: 10.1016/j.freeradbiomed.2004.02.005
Yan, Y., Candreva, J., Shi, H., Ernst, E., Martienssen, R., Schwender, J., et al. (2013). Survey of the total fatty acid and triacylglycerol composition and content of 30 duckweed species and cloning of a Δ6-desaturase responsible for the production of γ-linolenic and stearidonic acids in Lemna gibba. BMC Plant Biol 13, 201. doi: 10.1186/1471-2229-13-201
Yin, Y., Yu, C., Yu, L., Zhao, J., Sun, C., Ma, Y., et al. (2015). The influence of light intensity and photoperiod on duckweed biomass and starch accumulation for bioethanol production. Bioresource Technol. 187, 84–90. doi: 10.1016/j.biortech.2015.03.097
Yong, L. C., Petersen, M. R., Sigurdson, A. J., Sampson, L. A., and Ward, E. M. (2009). High dietary antioxidant intakes are associated with decreased chromosome translocation frequency in airline pilots. Am. J. Clin. Nutr. 90, 1402–1410. doi: 10.3945/ajcn.2009.28207
Yuan, J., and Xu, K. (2017). Effects of simulated microgravity on the performance of the duckweeds Lemna aequinoctialis and Wolffia globosa. Aquat. Bot. 137, 65–67. doi: 10.1016/j.aquabot.2016.11.010
Keywords: co-optimization, duckweed, energy dissipation, human nutrition, photoprotection, photosynthesis, spaceflight, zeaxanthin
Citation: Stewart JJ, Adams WW III, Escobar CM, López-Pozo M and Demmig-Adams B (2020) Growth and Essential Carotenoid Micronutrients in Lemna gibba as a Function of Growth Light Intensity. Front. Plant Sci. 11:480. doi: 10.3389/fpls.2020.00480
Received: 07 January 2020; Accepted: 31 March 2020;
Published: 07 May 2020.
Edited by:
Thomas Graham, University of Guelph, CanadaReviewed by:
Raquel Esteban, University of the Basque Country, SpainAlexei E. Solovchenko, Lomonosov Moscow State University, Russia
Copyright © 2020 Stewart, Adams, Escobar, López-Pozo and Demmig-Adams. This is an open-access article distributed under the terms of the Creative Commons Attribution License (CC BY). The use, distribution or reproduction in other forums is permitted, provided the original author(s) and the copyright owner(s) are credited and that the original publication in this journal is cited, in accordance with accepted academic practice. No use, distribution or reproduction is permitted which does not comply with these terms.
*Correspondence: Barbara Demmig-Adams, YmFyYmFyYS5kZW1taWctYWRhbXNAY29sb3JhZG8uZWR1