Corrigendum: The Hsp90 Inhibitor, Monorden, Is a Promising Lead Compound for the Development of Novel Fungicides
- 1Department of Agricultural Chemistry, Institute of Environmentally Friendly Agriculture, College of Agriculture and Life Science, Chonnam National University, Gwangju, South Korea
- 2Department of Agricultural Biotechnology, Seoul National University, Seoul, South Korea
- 3Biological and Genetic Resources Assessment Division, National Institute of Biological Resources, Incheon, South Korea
- 4GPS Screen Team, Drug R&D Institute, Bioneer Corporation, Daejeon, South Korea
- 5Therapeutic & Biotechnology Division, Center for Eco-friendly New Materials, Korea Research Institute of Chemical Technology, Daejeon, South Korea
Endophytic fungi are great resources for the identification of useful natural products such as antimicrobial agents. In this study, we performed the antifungal screening of various plant endophytic fungi against the dollar spot pathogen Sclerotinia homoeocarpa and finally selected Humicola sp. JS-0112 as a potential biocontrol agent. The bioactive compound produced by the strain JS-0112 was identified as monorden known as an inhibitor of heat shock protein 90 (Hsp90). Monorden exhibited strong antagonistic activity against most tested plant pathogenic fungi particularly against tree pathogens and oomycetes with the minimum inhibitory concentration values less than 2.5 μg mL–1. Extensive in planta assays revealed that monorden effectively suppressed the development of several important plant diseases such as rice blast, rice sheath blight, wheat leaf rust, creeping bentgrass dollar spot, and cucumber damping-off. Especially, it showed much stronger disease control efficacy against cucumber damping-off than a synthetic fungicide chlorothalonil. Subsequent molecular genetic analysis of fission yeast and Fusarium graminearum suggested that Hsp90 is a major inhibitory target of monorden, and sequence variation among fungal Hsp90 is a determinant for the dissimilar monorden sensitivity of fungi. This is the first report dealing with the disease control efficacy and antifungal mechanism of monorden against fungal plant diseases and we believe that monorden can be used as a lead molecule for developing novel fungicides with new action mechanism for the control of plant diseases caused by fungi and oomycetes.
Introduction
Plant pathogenic fungi are major causative agents of important plant diseases that threaten the agricultural industry worldwide (Dean et al., 2012). Until now, the control of fungal plant diseases largely depends on synthetic fungicides. However, the long term usage of the chemical fungicides has caused various negative effects such as residual toxicity, environment pollution, phytotoxicity, and the occurrences of drug-resistant pathogenic fungi to commercial fungicides (Brent and Hollomon, 1995; Parnell et al., 2006). Hence, the discovery of new natural metabolites and the development of novel fungicides using the natural metabolites as lead molecules are largely necessary in these days. Ideal lead molecules and fungicides would be highly effective against target pathogenic fungi, have new action mechanisms, and be safer to humans and the environment.
Among antagonistic microorganisms, use of endophytes has been considered as a potential option to substitute to agrochemicals on plant disease management (Ding et al., 2008; Kumar and Kaushik, 2012; Goudjal et al., 2014; De Silva et al., 2019). Endophytic bacteria and fungi are symbiotic microorganisms living inside healthy plant tissues without causing any disease symptoms. Some beneficial endophytes stimulate plant growth by producing plant hormones such as indole-3-acetic acid (Santoyo et al., 2016; Hassan, 2017), improve the tolerance to abiotic stresses (Pandey et al., 2018), or promote the plant immunity against microbial pathogens, insects, and herbivores (Azevedo et al., 2000; Ryan et al., 2008; Li et al., 2015; Bamisile et al., 2018).
Endophytic fungi exhibit the wide diversity that makes them to be great resources for the identification of useful natural products such as antimicrobial agents (Strobel and Daisy, 2003). Several bioactive secondary metabolites that suppress the plant diseases have been known to be produced by fungal endophytes. An oxygenated cyclohexanone derivative ([4S, 5S, 6S]-5,6-epoxy-4-hydroxy-3-ethoxy-5-methyl-cyclohex-2-en-1-one) isolated from an endophytic fungus Amphirosellinia nigrospora showed antifungal and antibacterial activities against various plant pathogens (Nguyen et al., 2019). Several compounds isolated from fungal endophytic Phoma species also exhibited antifungal activities (Wang et al., 2012). These studies demonstrated that endophytic fungi and their metabolites could be prospective resources for the development of novel plant disease control strategies.
The genus Humicola, previously known as an asexual genus in the Chaetomiaceae, was recently redefined to produce both asexual and sexual spores (Wang et al., 2019). Most of Humicola species were commonly found in soil, indoor environment, and composts whereas some of them have been known as endophytes (Radhakrishnan et al., 2015; Wang et al., 2016, 2019). Humicola species have displayed potential on the production of antibiotics that are appropriately used in human medicine and agriculture. Xanthoquinodins showing anticoccidial activity was isolated from soil Humicola sp. FO-888 (Tabata et al., 1993). Humicola fuscoatra KMM 4629 produced fuscoatrol A, 11-epiterpestacin, and β-nitropropionic acid, which showed antimicrobial activity against Staphylococcus aureus and Bacillus subtilis (Smetanina et al., 2004). H. phialophoroides exhibited potential suppression effect on fungal plant diseases (Ko et al., 2011). Fuscoatroside and fuscoatramide produced by H. fuscoatra NRRL 22980 showed antifungal activities against Aspergillus flavus (Joshi et al., 2002). This mycoparasite fungus H. fuscoatra NRRL 22980 also produced monorden, monocillin IV, and cerebrosides (Wicklow et al., 1998).
Monorden was first isolated from Monosporium bonorden as antifungal substance in the early 1950s (Delmotte and Delmotte-Plaquee, 1953). This compound displayed an inhibitory effect on growth and proliferation of fungal pathogens as well as cancer tumor (Sharma et al., 1998; Prodromou et al., 2009; Wicklow et al., 2009). Monorden showed significant biological control of antitumor in animal cells only in in vitro, but not in in vivo (Soga et al., 1999). Even though in vitro antifungal activity of this metabolite against fungal plant pathogens have been previously described well in the previous papers (Wicklow et al., 1998, 2009; Fujita et al., 1999), its disease control has not been reported till now. As for the target site of monorden, it was reported to bind to ATP-binding pocket in N-terminal domain of Hsp90 and then block its activity in cancer cells (Roe et al., 1999). Additionally, Prodromou et al. (2009) reported that replacement of isoleucine instead of leucine in binding site of Hsp90 reduced the affinity of monorden and this chaperone in a yeast model. However, its antifungal mechanism against fungal plant pathogens has not been studied.
Dollar spot is caused by the ascomycete fungus Sclerotinia homoeocarpa and is the most economically important turfgrass disease worldwide (Vargas, 1994). Management of this plant disease have largely been dependent on chemical fungicides such as benzimidazole, dicarboximide, and demethylase inhibitors. However, application of these chemicals is gradually being restricted because of the development of drug-resistant field strains and environmental concerns (Burpee, 1997). Alternative control strategies have been proposed to control dollar spot disease using suppressive composts, antagonistic fungi or bacteria, and antifungal natural products (Lo et al., 1997; Rodriguez and Pfender, 1997; Boulter et al., 2002).
In this study, we performed the massive antifungal screening of endophytic fungi against the dollar spot pathogen S. homoeocarpa and finally selected Humicola sp. JS-0112. Bioactive metabolite was revealed as monorden and this compound showed various antifungal activities against S. homoeocarpa as well as other plant pathogenic fungi and oomycetes. Therefore, this study aims (1) to isolate the fungal endophyte and its antifungal metabolite which could be used for the control of the dollar spot disease, (2) to evaluate the efficacy of the identified antifungal metabolite against other plant pathogenic fungi and oomycetes, and (3) to understand the molecular mechanism underlying the antifungal activity of the compound.
Materials and Methods
Microbial Species and Growth Conditions
Phytopathogenic fungi and oomycetes (Supplementary Table S1) which were used to test antifungal spectrum of the metabolite isolated from Humicola sp. JS-0112 were maintained on potato dextrose agar (PDA, Becton Dickinson, Sparks, MD, United States), except Ophiostoma ulmi which was grown on malt extract agar (MEA, Becton Dickinson) and Phytophthora species which were maintained on V8 agar (V8A) slants at 4°C. Humicola sp. JS-0112 was isolated from the root of Ixeris repens and maintained on PDA slants at 4°C. The fungal strain was inoculated in potato dextrose broth (PDB, Becton Dickinson) for 14 days at 25°C and 150 rpm for production of active compound. All fungal strains were cryogenic stored in glycerol 20% at −80°C. F. graminearum Z-3639 (Bowden and Leslie, 1999) was used as a parent strain for the construction of transgenic strains (Supplementary Table S2). F. graminearum transgenic strains, Δmat2 and HK226 (FgHSP90:PZEAR-FgHSP90), were obtained from previous studies (Lee et al., 2003; Bui et al., 2016).
Isolation and Identification of Endophytic Fungal Strains
Endophytic fungal strains were isolated, sequenced in the ribosomal region for putative identification, and stored for further study according to the procedures described in Nguyen et al. (2019). Each isolate was stored at −80°C as a glycerol stock before use, and deposited at the Wildlife Genetic Resources Bank of National Institute of Biological Resources (Supplementary Table S3). Preliminary identification of the endophytic fungi was based on cultural morphology on PDA and ITS sequences (Nguyen et al., 2019).
For the identification of the fungi showing strong antifungal activities, genomic DNA was extracted from the mycelial segments grown on PDA plates with Qiagen Plant DNA extraction kit (Qiagen, Hilden, Germany). Internal transcribed spacer (ITS) and large subunit of ribosomal DNA (LSU) was amplified with ITS1F (Gardes and Bruns, 1993) and LR5 (Vilgalys and Hester, 1990). Sequencing was conducted using five primers, ITS1F, ITS3, ITS4, LR0R, and LR5 (Vilgalys and Hester, 1990; White et al., 1990; Gardes and Bruns, 1993), to obtain sequences overlapped at least by two primers. Amplified fragments were purified and sequenced at Macrogen, Inc. (Seoul, South Korea). High quality sequences were aligned using Sequencer 5.0 (Ann Arbor, MI, United States) to obtain consensus sequences. BLAST searches were conducted against NCBI nr database and ad hoc BLAST DB. ITS and LSU sequences seen in the BLAST hits and related taxa were retrieved from NCBI. Multiple alignment was conducted with Clustal W, and subsequent phylogenetic analysis was carried out using MEGA version 7 (Tamura and Nei, 1993; Kumar et al., 2018). ITS and LSU sequences from the JS-0112 strain were deposited in NCBI GenBank under the accession number of MN006389 and MN006390, respectively.
In vitro Screening of Antifungal Activities
The antagonistic activity of endophytic fungi isolated from the plant was assessed by 96 well microtiter plate using broth dilution method against S. homoeocarpa, a fungal pathogen of dollar spot of creeping bentgrass (Nguyen et al., 2019). The mycelial fragment suspensions in sterile distilled water (50 mg fresh mycelia per ml), at 1%, were used as inocula for antifungal bioassay using PDB.
The endophytic fungal strains were incubated in PDB for 14 days at 25°C and 150 rpm. The fermentation broth was filtered through 4-layer gauze and the filtrate was passed through a 0.2 μm membrane filter to get axenic culture filtrate for bioassay. The mycelia were extracted with acetone and then filtered through filter paper. The acetone filtrate was concentrated to dryness using a rotary vacuum evaporator (N-1110, EYELA Co., Tokyo, Japan) and redissolved using methanol (MeOH) at a concentration of 50 mg mL–1.
Mycelial extracts (500, 250, 125, 62.5, 31.25, and 15.6 mg mL–1) and axenic culture filtrates (20, 10, 5, 2.5, 1.25, 0.63%) were added into each well containing mycelial suspension of each test pathogenic fungus and then diluted in serial 2-fold dilutions. The plates were incubated at 25°C for 4 days. Minimum inhibitory concentration (MIC) values were defined as the lowest concentration of the mycelial extracts or culture filtrates causing complete inhibition of mycelial growth of the tested fungus. The bioassay was repeated two times in triplicate for each sample against S. homoeocarpa at all test concentrations.
Determination of Antifungal Activity by Dual-Culture Assay
To evaluate inhibitory activity of Humicola sp. JS-0112 against mycelial growth of 10 phytopathogenic fungi and oomycetes (Figure 1), dual culture assay was conducted as previously reported with minor modifications (Hamzah et al., 2018). Fungal disks of JS-0112 and pathogen (5 mm) were co-disposed at 5-cm distance on the PDA petri dish for most of the test fungi except Phytophthora species, which used V8 agar medium. The antifungal activity of JS-0112 was estimated by the inhibition of fungal growth of pathogen in comparison to a solely cultivated fungal agar disk. The fungal growth of pathogenic fungi was observed after 5–15 days after incubation at 25°C. The experiments were repeated twice in triplicate.
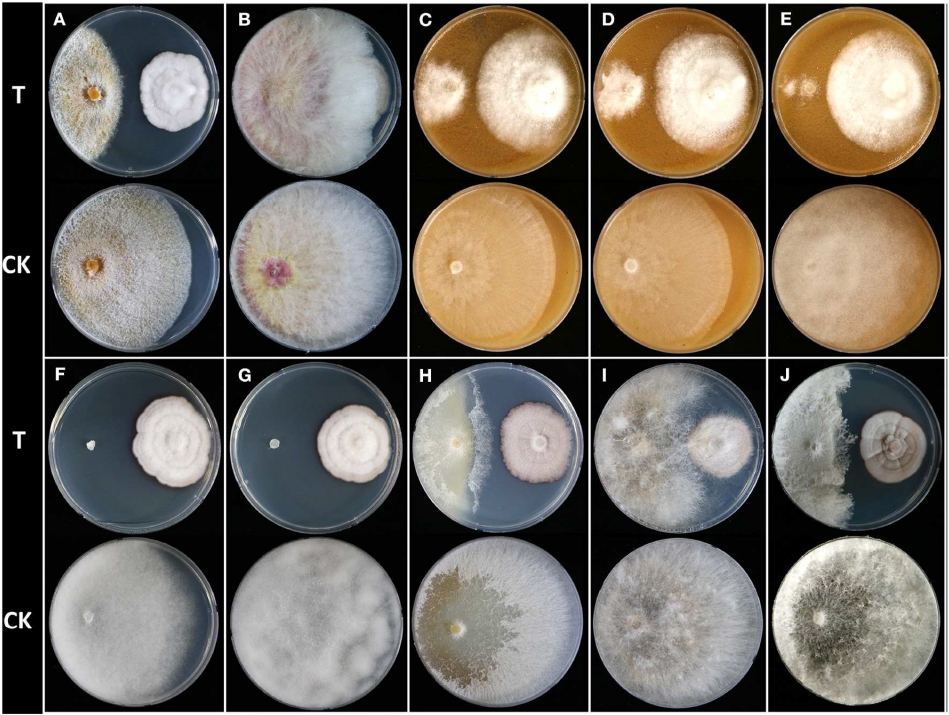
Figure 1. Dual culture assay of Humicola sp. JS-0112 against 10 phytopathogenic fungi and oomycetes. (A) Cryphonectria parasitica, (B) Fusarium graminearum, (C) Phytophthora cambivora, (D) Phytophthora cactorum, (E) Phytophthora cinnamomi, (F) Pythium graminicola, (G) Pythium ultimum, (H) Raffaelea quercus-mongolicae, (I) Rhizoctonia solani, (J) Sclerotinia homoeocarpa; T, co-cultivation of JS-0112 with phytopathogenic fungi; CK, pathogenic fungal strains cultivated alone served as controls.
Isolation of the Antifungal Metabolite
Six L of PDB cultures of JS-0112 were filtered through 4-layer gauze to separate culture filtrate and mycelia. The mycelia was extracted with acetone twice (1 L × 2), and then concentrated to dryness. The acetone extract was redissolved in 500 ml of distilled water and then partitioned with equal volumes of ethyl acetate (EtOAc) twice. The culture filtrate was also partitioned with EtOAc twice. The two EtOAc layers were combined and then concentrated to dryness using a rotary vacuum evaporator (N-1110, EYELA Co). The crude extract (14 g) was loaded onto silica gel (700 g, 70–230 mesh, 5 cm i.d × 60 cm; Merck, Darmstadt, Germany), which was eluted with chloroform:MeOH (99:1, v/v). Through thin layer chromatography analysis and in vitro bioassay using 96 well microtiter broth dilution method against S. homoeocarpa, the fractions showing antifungal activity were pooled and then further separated using a Sephedex LH-20 column (20 g, 2 cm i.d × 40 cm; Sigma-Aldrich Co., St. Louis, MO, United States) eluting progressively with 4:1 dichloromethane:hexane (120 mL) and 3:2 dichloromethane:acetone (220 mL), yielding the purified compound 1 (510 mg).
Structural Elucidation of the Purified Compound
EI-MS and nuclear magnetic resonance spectroscopy (NMR) analyses were performed to determine the chemical structure of compound 1. EI-MS was recorded on a JEOL JMS-700 high resolution mass spectrometer at 70 eV. 1H-NMR and 13C-NMR data of compound 1 were recorded using Bruker Avance III HD 500 MHz instrument (Bruker Biospin GmbH, Rheinstetten, Germany) in chloroform-d (Cambridge Isotope Laboratories, Inc., Andover, MA, United States) and tetramethylsilane (TMS) was used as an internal standard in the NMR analysis.
In vitro Antifungal Activity of Monorden
The antifungal activity of monorden was evaluated by a serial broth dilution method as described above. Phytophathogenic fungi and oomycetes listed in Supporting Information Table S1 were used in this study. These pathogens were grown on PDB except Ophiostoma ulmi that was incubated on malt extract broth (MEB; MB Cell, South Korea). Monorden was dissolved in acetone at a concentration of 10 mg mL–1 as a stock solution, which was used to determine the MIC value against mycelia growth in 96 well-plate. Monorden was treated in a range of 0.078 – 100 μg mL–1. The final concentration of acetone was 1% v/v, and 1% acetone was used as an untreated control. All plates were incubated for 4–5 days at 25°C. MIC values were measured, and the experiment was repeated three times in triplicate against each fungal pathogen.
Disease Control Efficacy of Monorden
To evaluate the disease control efficacy of monorden isolated from Humicola sp. JS-0112 against 9 plant diseases, the chemical was dissolved in acetone. The in vivo bioassays against 7 plant diseases including rice blast (RCB), rice sheath blight (RSB), tomato gray mold (TGM), tomato late blight (TLB), wheat leaf rust (WLR), barley powdery mildew (BPM), and red pepper anthracnose (RPA) were conducted as previously reported (Kim et al., 2001, 2004; Cho et al., 2006). Monorden was applied at 125, 250, and 500 μg mL–1 at 1 day before pathogen inoculation. Tween 20 solution (250 μg mL–1) containing 5% acetone was used as an untreated control and synthetic fungicides were used as positive controls (Table 1). The plant pots were randomly arranged with 3 replications per treatment and all experiments were repeated twice. Disease severity was measured 5 days after inoculation (DAI) for RCB, 8 DAI for RSB, 3 DAI for TGM, 4 DAI for TLB and RPA, 7 DAI for WLR and BPM. The mean values of the six estimates for each treatment were converted into control value.
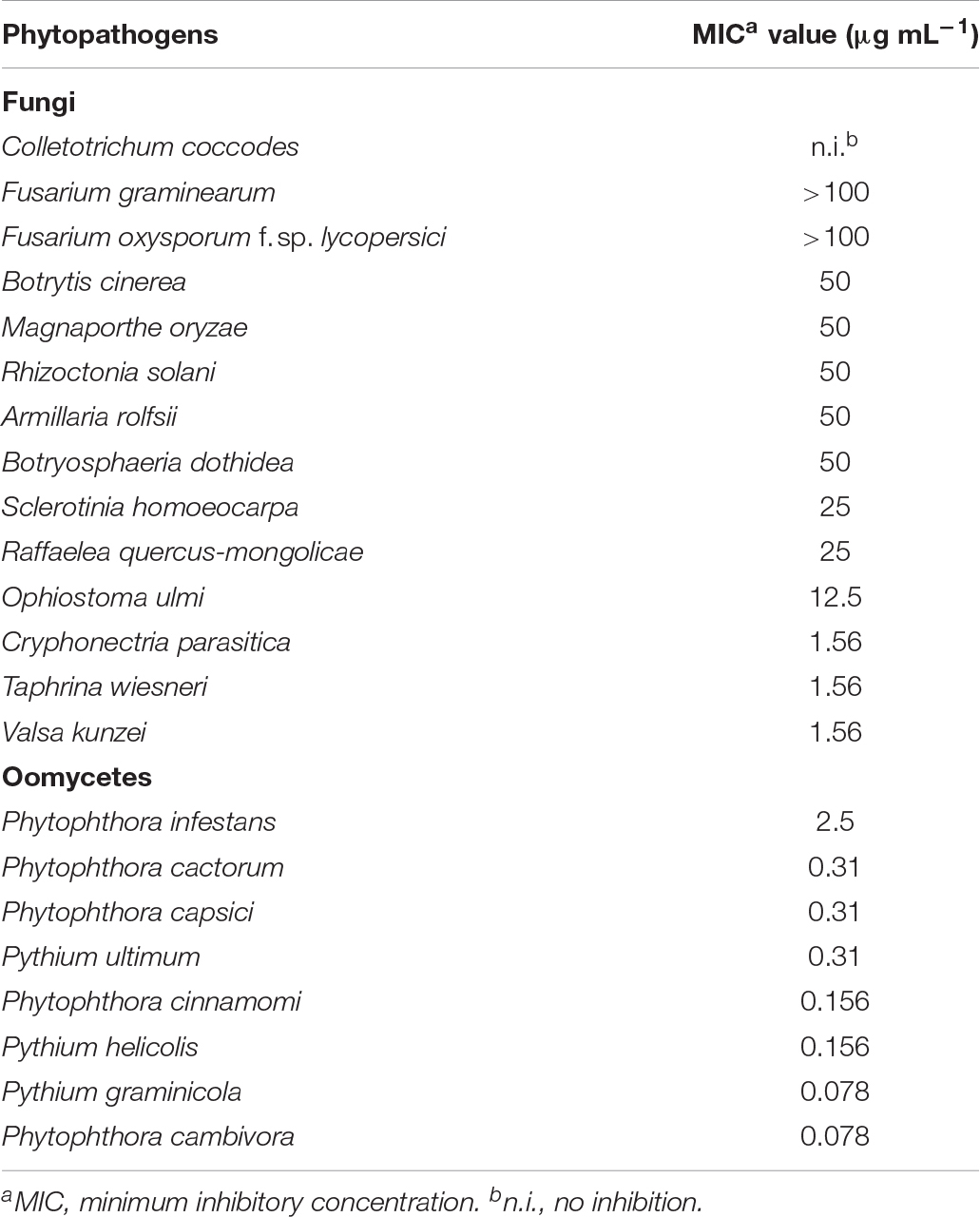
Table 1. In vitro antifungal activity of monorden against mycelial growth of several phytopathogenic fungi.
The disease control efficacy of monorden was also tested against creeping bentgrass dollar spot (CDS). S. homoeocarpa was incubated in wheat-rice bran medium (wheat bran 200 mL, rice bran 100 mL, distilled water 100 mL in a 1-liter Erlenmeyer flask) for 7 days at 25°C. The culture was macerated for 10 s after adding 400 mL sterile distilled water containing 200 μg mL–1 streptomycin sulfate. The 3-week-old creeping bentgrass seedlings in vinyl pots (Φ 7 cm) were inoculated by adding 3.5 mL of the mycelial fragment suspension of S. homoeocarpa to soil. One hour after inoculation, these pots were treated with 10 mL of monorden solutions (250, 125, and 62.5 μg mL–1) by soil drench. A synthetic fungicide, Horikuo (tebuconazole 25%; Farmhannong Co., Ltd., Seoul, South Korea), at 2000-fold dilution was used as a positive control and Tween-20 (250 μg mL–1) solution containing 0.1% acetone was used as untreated control. The plants were grown at 25°C and relative humidity 95% for 7 days with 16 h light per day and then disease severity was assessed. Disease severity was determined as the percentage of infected aerial part. The experiment was carried out twice in triplicates and the mean values of the six estimates for each treatment were converted into control value.
The effect of monorden on the development of cucumber damping-off (CDO) caused by Pythium ultimum was examined by the previously established method with slight modifications (Li et al., 2007). In this study, the in vivo assay was done using 24-well microtiter plates (SPL Life Sciences Co., Ltd., Gyeonggi-do, South Korea). One cucumber seed was sown into each well containing approximately 2 g of sterile sand, and then agar plug of P. ultimum (Φ 5 mm) was inoculated onto seed, followed by covering with 1 g of sterile sand. Monorden was dissolved in acetone and then diluted with Tween 20 solution (250 μg mL–1) at concentrations of 100, 75, 50, 25, and 12.5 μg mL–1. An equal aliquot of 1 mL of each solution was treated into each well. A synthetic fungicide, chlorothalonil (Sigma-Aldrich Co) dissolved in acetone, was used as s positive control and treated at the same concentrations as monorden. Tween 20 solution containing 1% acetone was used as untreated control. The microtiter plates were incubated in a growth chamber for 7 days at 25°C with light of 16 h per day, and then post-emergence damping-off were observed. The experiment was conducted two times with three replications, each consisting of 10 seedlings. The mean values of the six estimates for each treatment were converted into control value.
Drug Target Screening of Monorden in Schizosaccharomyces pombe Heterozygous Deletion Mutant Library
Drug target screening was performed using S. pombe heterozygous deletion mutants from Bioneer (South Korea). S. pombe heterozygous deletion mutant library was constructed as described before (Kim et al., 2010). Here, target screening of monorden was carried out using a Bioneer’s-unique drug-target screening system (GPScreen) at the GI50 of the agent on 94 genes of heat-shock protein-related functional group subset in S. pombe genome, using the principle of drug-induced haploinsufficiency (DIH) that measures growth inhibition by the compound on S. pombe heterozygous deletion mutants. The determination of GI50 of monorden in wild type S. pombe cells (SP286; h+/h+, ade6M210/ade6-M216, ura4-D18/ura4-D18, leu1-32/leu1-32) and the drug target screening were done with the same method as described (Lee et al., 2012). The cells were treated with the GI50 (4 μM) of monodern or with dimethylsulfoxide (DMSO)-only control after incubation at 30°C for 21 h. Fitness value which represents the growth-inhibitory potency of compound at GI50 dose in each deletion mutant was calculated by dividing cell mass (A600 nm) of DMSO control with the one of drug treated. Time-course assay was performed to confirm the cell growth inhibition in another way; after the compound treatment, cultures were incubated at 30 °C, and the cell mass (A600 nm) was recorded every 2 h using a microplate reader (M200Pro; Tecan, Maennedorf, Switzerland). Both assays were performed with three biological replicates.
Construction of F. graminearum Transgenic Strains
To generate the F. graminearum transgenic strain expressing HSP90 of Cryphonectria parasitica, the geneticin resistance gene cassette (GEN)-PEF1α was amplified from the pSKGEN vector (Lee et al., 2011) using the pSKGEN-A and pSKGEN-C primers (Supplementary Table S4), and the HSP90 open reading frame with 3′ region was amplified from genomic DNA of C. parasitica KACC40323 using Cp-A and Cp-C primers. The resulting two fragments were fused and the final construct was amplified using the nest primers pSKGEN-B and Cp-B. Final construct GEN-PEF1α -CpHSP90 was transformed into the F. graminearum wild type strain Z-3639 resulting in the PEF1α -CpHSP90 strain, of which CpHSP90 is under the control of strong promoter PEF1α (Supplementary Table S2) as previously described (Son et al., 2011). Then heterothallic Δmat2 strain was outcrossed with PEF1α -CpHSP90 strain to generate the Δmat2; PEF1α -CpHSP90 strain. Finally, the FgHSP90:PZEAR-FgHSP90; PEF1α -CpHSP90 strain highly expressing CpHSP90 but repressing FgHSP90 was selected from an outcross Δmat2; PEF1α -CpHSP90 × PEF1α -CpHSP90. The PCR primers (Supplementary Table S4) used in this study were synthesized by an oligonucleotide synthesis facility (Bionics, Seoul, South Korea).
For outcross, mycelia of heterothallic female strains (Δmat2 or Δmat2; PEF1α -CpHSP90) grown on carrot agar plates for 5 days were fertilized with 1 mL of a conidial suspension from male strains. After sexual induction, all cultures were incubated under near-UV light (wavelength: 365 nm, Sankyo Denki Co., Ltd., Tokyo, Japan) at 25°C. Presence of PZEAR-FgHSP90 of the progeny from an outcross Δmat2; PEF1α -CpHSP90 × PEF1α -CpHSP90 was confirmed using a PCR assay with H3 and ZEAR-F3 primers (Supplementary Table S4).
Statistical Analysis
Data on disease control efficacy of monorden against RCB, RSB, TGM, TLB, WLR, BPM, RPA, CDS, and CDO were analyzed separately using the statistical software SPSS ver. 23.0 (SPSS Inc., Chicago, IL, United States). All the experimental data are expressed as means ± standard deviation (SD) of replicates and investigated by one-way ANOVA using Duncan’s test. p < 0.05 was considered as statistically significant. The inhibition concentration (IC50) derived from monorden and chlorothalonil dose-response curves were determined using non-linear regression of GraphPad Prism 7 (Graph Pad Software Inc., San Diego, CA, United States).
Results
Antifungal Screening of Endophytic Fungi and Identification of JS-0112
We isolated 219 endophytic fungi from leaf, stem, or root tissues of 29 plant taxa in South Korea, and preliminary identification was carried out based on colony morphology on PDA and ITS sequence analysis (Supplementary Table S3). To screen potential biocontrol agents for the management of dollar spot caused by S. homoeocarpa, MIC values of both culture filtrates and mycelial extracts of 219 fungi against S. homoeocarpa were investigated. Among them, only JS-0112 and JS-0169 strains showed inhibitory effects against mycelial growth of S. homoeocarpa (Supplementary Table S3). Whereas the culture filtrate of JS-0169 displayed a weak in vitro antifungal activity with MIC value over 20%, both culture filtrate and mycelial extract of JS-0112 strongly inhibited the mycelial growth of S. homoeocarpa with MIC values of 1.25% and 125 μg mL–1, respectively. Antifungal activity of JS-0112 strain against S. homoeocarpa was also observed from the dual culture assay (Figure 1).
The JS-0112 strain was identified as Humicola sp. that is closely related to H. pulvericola through phylogenetic analysis using concatenated ITS and LSU sequences (Figure 2A). Colonies on PDA after 7 days at 25°C were mouse gray to pale olivaceous; reverse pink to dull orange at the center and white at the margins (Figure 2B). JS-0112 produced pigmented, thick-walled and single-celled conidia on very short conidiophore along the hyphae, which is one of characteristics of genus Humicola (Wang et al., 2016, 2019; Figure 2C).
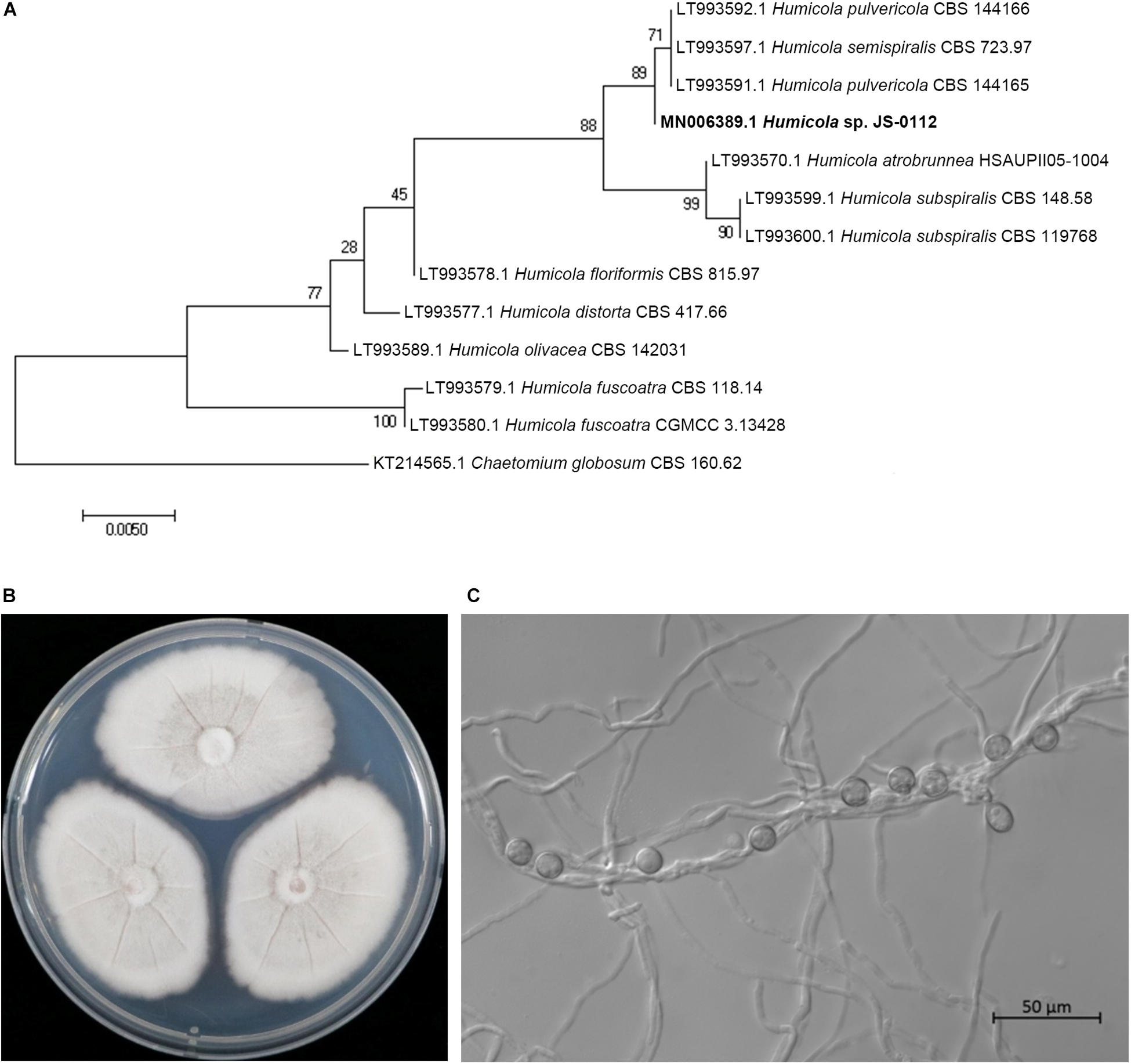
Figure 2. Morphological features and phylogenetic tree of JS-0112. (A) Phylogenetic tree reconstructed by Maximum Likelihood method with concatenated ITS and LSU sequences of JS-0112 and related taxa using MEGA version 7. GenBank accession number of each ITS-LSU sequences from each isolate was shown in front. ITS and LSU sequences of Chaetomium globosum strain CBS 160.62 were used as the outgroup. Bootstrap values with 1,000 replicates were illustrated on the node. (B) Colony morphology grown on PDA for 7 days. (C) Conidia produced on very short conidiophore along the hyphae. Bar indicates 50 μm.
Identification of Monorden and Its Control Efficacy for Creeping Bentgrass Dollar Spot (CDS)
The antifungal metabolite, compound 1, was purified from Humicola sp. JS-0112. Electron ionization mass spectrometry (EI-MS; 70 eV) of this compound appeared molecular ion peak at m/z 364, which inferred that the molecular formula is C18H17ClO6. 1H- and 13C-NMR data of monorden are presented in Supplementary Table S5. Compound 1 was identified as monorden by comparing with mass spectrometry (MS) and nuclear magnetic resonance spectroscopy (NMR) data reported by Wicklow et al. (1998), Yamamoto et al. (2003b), and Wicklow et al. (2009).
Then the disease control efficacy of monorden was tested against CDS. The results showed that the purified monorden alone was highly effective in reducing the development of CDS caused by S. homoeocarpa. Its control values were about 88, 75, and 53% at 250, 125, and 62.5 μg mL–1, respectively (Figures 3A,B). In particular, the CDS development was similarly suppressed by monorden 250 μg mL–1 compared to the synthetic chemical fungicide Horikuo (tebuconazole 25%).
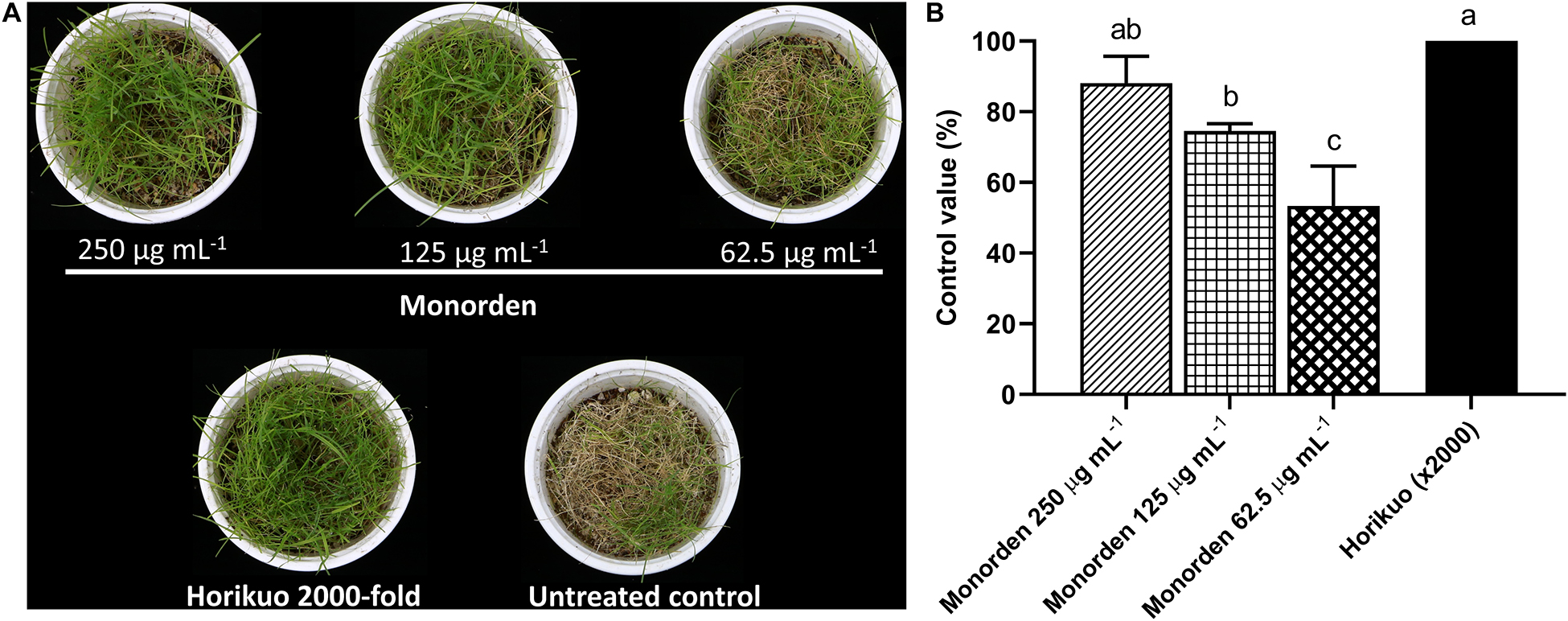
Figure 3. Disease control efficacy of monorden against dollar spot of creeping bentgrass caused by Sclerotinia homoeocarpa. (A) Symptoms of dollar spot on creeping bentgrass 7 days after inoculation. (B) Control values of monorden against creeping bentgrass dollar spot 7 days after inoculation. Disease severity was determined as the percentage of infected aerial part. Each value represents the means ± standard deviation of two runs with three replicates per run. Different lowercase letters show values that are significantly different (p < 0.05) level by Duncan’s test.
In vitro Antifungal Activity of JS-0112 and Monorden Against Various Fungal Pathogens
To explore the potential for use of JS-0112 or purified monorden in a broader range of fungal disease controls, we first performed the dual culture assay between JS-0112 and various plant pathogenic fungi and oomycetes (Supplementary Table S1 and Figure 1). Overall, Humicola sp. JS-0112 inhibited the mycelial growth of all tested plant pathogenic fungi and oomycetes (Figure 1). JS-0112 moderately retarded the radial growth of Rhizoctonia solani, and growth of F. graminearum was slightly delayed in dual culture assay with the JS-0112. Mycelial growth of C. parasitica and R. quercus-mongolicae as well as S. homoeocarpa was markedly inhibited when they were co-cultured with JS-0112. In particular, Phytophthora species were strongly sensitive to JS-0112, while mycelial growth of Pythium species was completely inhibited. The result suggests that antifungal activity of monorden is highly variable depending on fungal species.
We then investigated in vitro antifungal activity of monorden isolated from Humicola sp. JS-0112 against plant pathogenic fungi and oomycetes. Monorden inhibited the mycelial growth of all of the tested fungi and oomycetes with various MIC values except Colletotrichum coccodes (Table 1). Among the tested fungal species, tree pathogens (C. parasitica, Taphrina wiesneri, and Valsa kunzei) tend to be highly sensitive to monorden with MIC values of 1.56 μg mL–1, whereas Fusarium species (F. graminearum and F. oxysporum f. sp. lycopersici) showed moderate resistances toward monorden (Table 1). Intriguingly, most oomycetes were highly sensitive to monorden with MIC values of 0.078 – 2.5 μg mL–1; MIC values of most tested oomycetes were below than 0.39 μg mL–1 except Phytophthora infestans (2.5 μg mL–1). Puccinia recondita and Blumeria graminis which are causal agents for WLR and BPM, respectively, could not be used for in vitro experiment because they are obligate parasites.
Disease Control Efficacy of Monorden Against Several Plant Diseases
We tested disease control efficacy of monorden isolated from Humicola sp. JS-0112 culture against seven plant diseases caused by fungi or oomycetes such RCB, RSB, TGM, TLB, WLR, BPM, and RPA (Table 2). Chemical fungicides widely used for each plant disease control were used for positive controls. Our virulence assays showed that monorden effectively suppressed the development of RCB caused by Magnaporthe oryzae, RSB caused by R. solani and WLR caused by Puccinia recondita in a dose-dependent manner. RCB was the most effectively controlled by monorden with control values of 90.0, 78.8, and 25.0% at 500, 250, and 125 μg mL–1, respectively. However, monorden was virtually inactive in reducing the development of TGM, TLB, BPM, and RPA (Table 2).
Monorden very effectively reduced the development of CDO caused by P. ultimum. Monorden even showed higher control efficacy against CDO than a commercial fungicide, chlorothalonil (Figure 4). The calculated IC50 (the concentration that controls 50% of the disease severity) values were 18.44 μg mL–1 for monorden and 40.01 μg mL–1 for chlorothalonil.
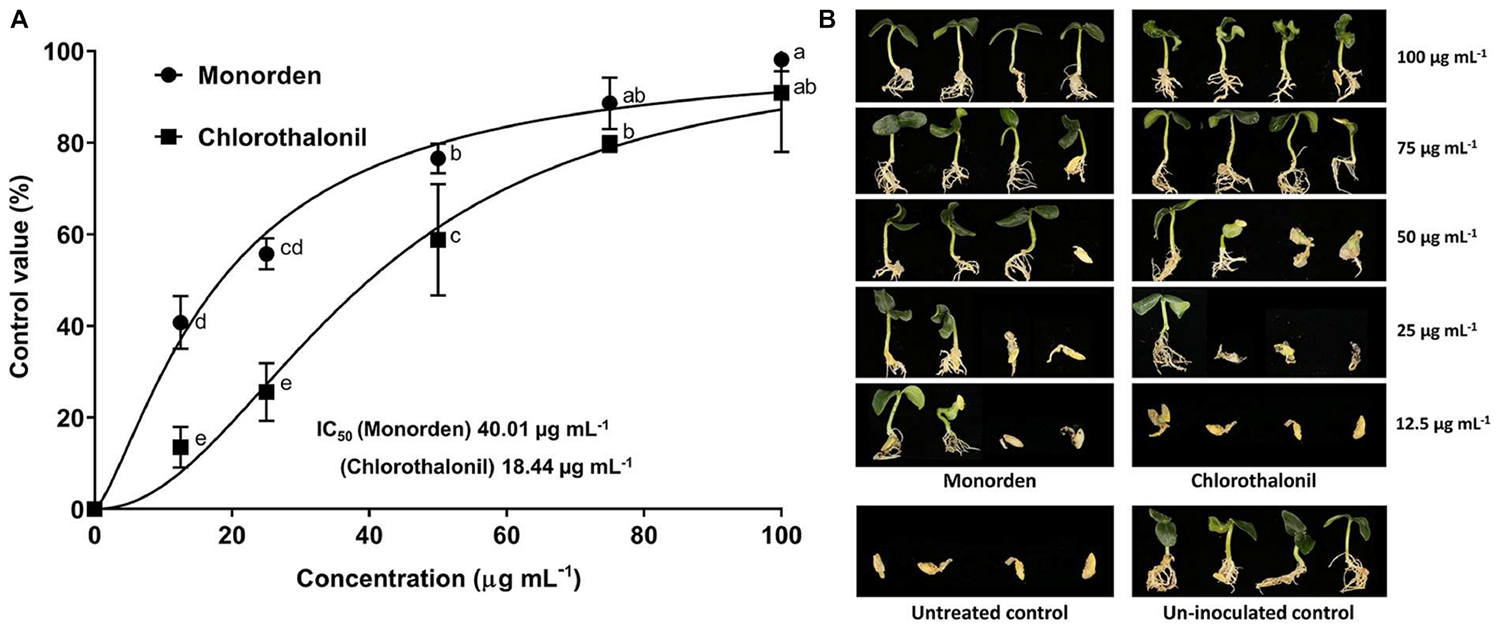
Figure 4. Disease control efficacy of monorden against cucumber damping-off (CDO) caused by Pythium ultimum. (A) Dose-response curve and IC50 values in the control of CDO. Each value represents the means ± standard deviation of two runs with three replicates per run. Each replicate consisted of ten seedlings. Data from two runs were plotted using Graph Pad Prism Version 7 (Graph Pad Software Inc., San Diego, CA, United States). Different small letters indicate significant different values (Duncan’s test, p < 0.05). (B) Post emergence damping-off of cucumber 7 days after inoculation.
Drug-Induced Haploinsufficiency (DIH) Assay in the Fission Yeast S. pombe
Monorden has been known as an inhibitor of Hsp90, which is a chaperone protein embarking in protein folding and degradation (Sharma et al., 1998). In order to fully apprehend the mode of action of monorden in fungi, we exploited drug-induced haploinsufficiency (DIH) assay with the fission yeast S. pombe heterozygous deletion mutant library (Kim et al., 2010) and a high-throughput genome-wide drug target identification system (Lee et al., 2012). For this study, deletion mutants of 94 genes related to posttranslational modification, protein turnover, chaperones among S. pombe genome-wide deletion mutant library (4,845 genes) were used to screen the drug target of monorden (Supplementary Table S6).
Monorden showed potent anti-proliferative activity against wild-type S. pombe cells for 21 h (GI50 = 4 μM; Supplementary Figure S1). DIH by monorden in various S. pombe heterozygous deletion mutants revealed that fitness scores of five gene deletion mutants (hsp90, pss1, cct1, cct6, and cct7) were over 3.0 among the mutants that were inhibited by monorden at GI50 (Figure 5). For target validation, the wild-type strain and five deletion mutants were cultured and treated with either DMSO-only or monorden (4 μM). As the results, the cell growth of hsp90, pss1, and cct1 mutants was markedly inhibited by monorden compared to wild type (WT) of S. pombe cells (Figures 5B,C, and Supplementary Figure S2). The results may demonstrate that Pss1, Cct1, Cct6, and Cct7 could be putative targets of monorden. However, in silico protein-protein network analysis using STRING (Szklarczyk et al., 2018) showed that putative targets of monorden identified in this study (Pss1, Cct1, Cct6, and Cct7) interact with Hsp90, demonstrating that they are not directly targeted by monorden (Supplementary Figure S3).
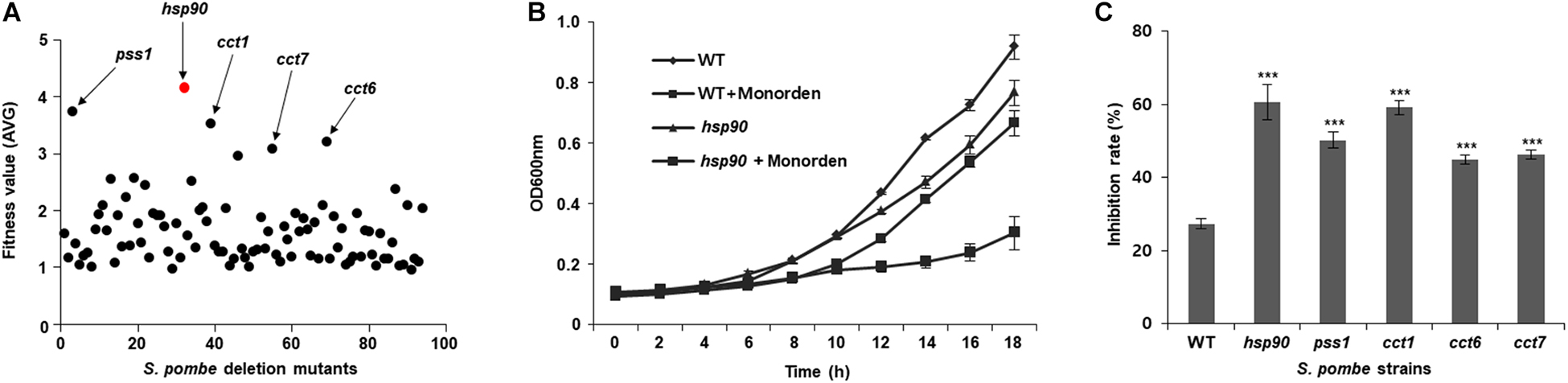
Figure 5. Identification of potential targets of monorden using the drug target screening system of a S. pombe mutant library. (A) Monorden was treated to culture of S. pombe genome-wide deletion library at the GI50 (4 μM) for 21 h at 30 °C and the fitness values of each mutant were plotted as the mean of three biological replicates (Supplementary Table S6). (B) Time-course assay was performed to confirm the cell growth inhibition of S. pombe mutants; after the compound treatment, cultures were incubated at 30 °C, and the cell mass (A600 nm) was recorded every 2 h. Data was presented as the mean and standard error of three biological replicates. (C) Inhibition rate of each S. pombe strains at 18 h after the treatment in (B) and Supplementary Figure S2 was calculated. ***p < 0.001.
Relationship Between Hsp90 Sequence Variation and Monorden Sensitivity
The result of the DIH assay demonstrates that fungal Hsp90 orthologs are major target for monorden, although some other chaperones are possibly inhibited by this compound. Supposing that structural differences among Hsp90 orthologs contribute to the dissimilar sensitivity against monorden in fungi and oomycetes, we first compared Hsp90 ortholog sequences. Phylogenetic and alignment analyses showed that Hsp90 orthologs of oomycetes are more variable and have distinct C-terminal regions compared to those of fungi (Supplementary Figures S4, S5). In particular, many Fusarium specific sequence regions were observed among fungal Hsp90 proteins, suggesting that structural alteration caused by these sequence variations is important for the resistant nature of Fusarium species against monorden (Supplementary Figure S6). All of the analyzed Hsp90 orthologs except that of JS-0112 strain do not have L34I mutation (Red asterisk in Supplementary Figure S6) which is responsible for the monorden resistance in monorden-producing fungus H. fuscoatra (Prodromou et al., 2009).
To gather insights into the relationship between global Hsp90 sequence variation and monorden sensitivity, we applied the molecular genetics approach with two Hsp90 orthologs of F. graminearum and C. parasitica which showed the lowest and highest sensitivities toward monorden among tested fungi (Table 1). We hypothesized that the F. graminearum transgenic strain expressing HSP90 of C. parasitica (CpHSP90) without its native HSP90 (FgHSP90) would be more sensitive toward monorden than the wild-type strain. Since HSP90 is essential gene in F. graminearum, we used HSP90 knock-down mutant HK226 (FgHSP90:PZEAR-FgHSP90) for this study (Bui et al., 2016). PZEAR is a zearalenone inducible promoter and therefore expression of FgHSP90 remains very low without supplementation of zearalenone (Lee et al., 2010). The FgHSP90:PZEAR-FgHSP90; PEF1α -CpHSP90 strain similarly grew on PDA compared to the wild-type strain, suggesting that CpHSP90 is the functional ortholog of FgHSP90 (Figure 6).
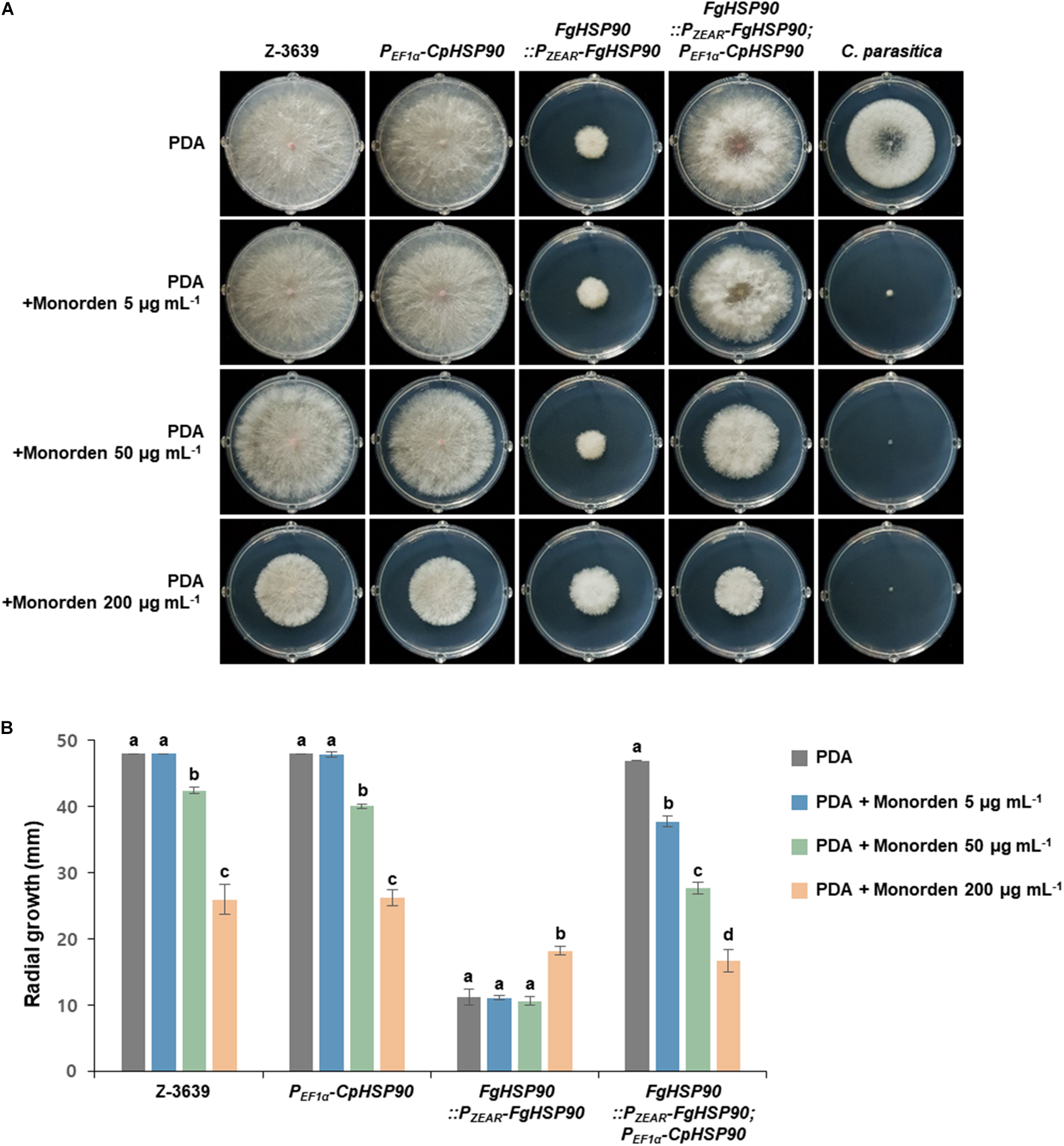
Figure 6. Mycelial growth of F. graminearum strains on PDA and PDA supplemented with monorden. (A) Colony morphology and (B) Radial growth of fungal strains. F. graminearum Z-3639, Cryphonectria parasitica KACC40323, and transgenic F. graminearum strains carrying PEF1α -CpHSP90 and/or PZEAR-FgHSP90 were inoculated on PDA and PDA supplemented with different concentrations of monorden (5, 50, and 200 μg mL–1). Data were obtained 4 days after inoculation. Three replicate plates of each medium per isolate were used and radial growth was measured three times for each plate. For each strain, same letters mean there is no significant difference among culture conditions, different letters represent statistically significant differences at p < 0.05.
We compared mycelial growth of F. graminearum strains on PDA with or without supplementation of monorden (Figure 6). The FgHSP90 knock-down mutant (FgHSP90:PZEAR-FgHSP90) showed reduced sensitivity to excess monorden (200 μg mL–1), whereas the F. graminearum wild-type strain were reduced in growth in a dose-dependent manner and showed about 50% growth reduction on PDA with 200 μg mL–1 monorden treatment. This result indicates that FgHsp90 is the primary inhibitory target of monorden although F. graminearum is highly resistant to monorden compared to other fungal species. When CpHSP90 was expressed in FgHSP90 knock-down mutant, the transgenic strain (FgHSP90:PZEAR-FgHSP90; PEF1α -CpHSP90) was significantly reduced in growth even under 5 μg mL–1 monorden as C. parasitica (Figure 6). This is the clear evidence demonstrating that CpHsp90 is much more susceptible to monorden than FgHsp90 in the F. graminearum cell system.
Discussion
The polyketide monorden was first isolated and identified in 1953 from a culture of M. bonorden (Delmotte and Delmotte-Plaquee, 1953) and named in 1964 (McCapra et al., 1964). Mirrington et al. (1964) also isolated this compound from the culture filtrate of Nectria radicicola and gave the name “Radicicol.” Monorden has been known to be produced by Pochonia species (Stadler et al., 2003), Penicillium luteo-aurantium (Nozawa and Nakajima, 1979), Pochonia chlamydosporia var. catenulata (Hellwig et al., 2003), Chaetomium chiversii (Turbyville et al., 2006), Colletotrichum graminicola (Wicklow et al., 2009), and several Humicola species (Wicklow et al., 1998; Fujita et al., 1999). Moreover, biological activities of monorden have already been described in previous reports (Wicklow et al., 1998; Fujita et al., 1999; Winssinger and Barluenga, 2007). This chemical is known to act as a selective Hsp90 inhibitor, and exhibits antifungal and antitumor properties (Ayer et al., 1980; Sharma et al., 1998; Wicklow et al., 2009). In particular, monorden has been shown to exert antimicrobial activity against the oomycete Pythium debaryanum with an MIC value of 10 μg mL–1 (lowest concentration evaluated) and the fungal pathogen R. solani (MIC = 100 μg mL–1) (Ayer et al., 1980). However, to the best of our knowledge, in planta assays of monorden activity in the context of fungal plant diseases have not been performed.
In this study, we found that endophytic fungus Humicola sp. JS-0112 produced monorden. In addition, extensive in vitro and in planta assays showed that monorden significantly suppressed mycelial growth and/or virulence of several economically important plant pathogenic fungi. Most fungal pathogens were highly sensitive to monorden while Fusarium species (F. graminearum and F. oxysporum f. sp. lycopersici) and C. coccodes showed moderate resistance to this compound (Table 1). In agreement with the results of our in vitro assays, monorden successfully reduced the development of RCB (M. oryzae), RSB (R. solani), WLR (P. recondita), and CDS (S. homoeocarpa) in a dose-dependent manner (Table 1). Although B. cinerea showed similar levels of sensitivity toward monorden when compared with M. oryzae and R. solani (MIC = 50 μg mL–1), and the mycelial growth of P. infestans was highly sensitive (MIC = 2.5 μg mL–1), monorden failed to reduce TGM (B. cinerea) and TLB (P. infestans) severity in infected plants. This means that the disease control efficacy of monorden depends not only on drug sensitivity but also on other factors including variable host physiologies or pathosystems. Interestingly, tree pathogenic fungi (Botryosphaeria dothidea, C. parasitica, O. ulmi, R. quercus-mongolicae, T. wiesneri, and V. kunzei) tend to be more sensitive to monorden than pathogens of crop or vegetable plants. Because the control of tree diseases is very tricky and largely dependent on chemical fungicides, further in planta studies of these tree pathogenic fungi could increase the likelihood of developing an industrial application for monorden in the forestry industry.
Together with plant pathogenic fungi, some oomycete-derived plant diseases have long been a source of concern in the agricultural industry (Kamoun et al., 2015). Although oomycetes are superficially similar to filamentous fungi, they are more closely related to algae and plants than fungi (Van de Peer and De Wachter, 1997). Therefore, fungi and oomycetes generally have quite different physiology including variations in sensitivity to synthetic fungicides and natural metabolites (Latijnhouwers et al., 2003). As the management of oomycete diseases primarily depends on chemical fungicides, the development of novel natural products with strong antagonism toward oomycetes remains an industry priority.
Several natural products demonstrating strong antagonistic effects on oomycetes have been reported. Chaetomin (MIC = 2.5 μg mL–1) and 4-phenyl-3-butenoic acid (MIC = 0.5 μg mL–1) have potent antimicrobial activity against P. ultimum (Di Petro et al., 1992; Lee et al., 2005). Xanthyletin (MIC = 5 μg mL–1) and 4-formylsyringol (MIC = 10 μg mL–1) were revealed to possess strong antagonistic effects against Pythium insidiosum (Suthiwong et al., 2017). Our comprehensive in vitro and in planta assays reveal that monorden is also a strong antagonistic agent of various oomycetes. Mycelial growth of oomycete species, except for P. infestans (MIC = 2.5 μg mL–1), was severely inhibited following treatment with monorden with MIC values of less than 0.31 μg mL–1 (Table 1). Moreover, the disease control efficacy of monorden against CDO—caused by P. ultimum—was much higher than that of the widely used chemical fungicide chlorothalonil (Figure 4). These data indicate that, to date, monorden and its derivatives could potentially be the most effective fungi-derived antimicrobial agents for the control of plant diseases caused by oomycetes.
Fungi and oomycetes show variable degrees of sensitivity toward monorden depending on the species. Fusarium species, in particular, possess efficient molecular mechanisms allowing for moderate monorden resistance while other tree pathogenic fungi and oomycetes were rendered inviable even at very low concentrations of monorden. The results of the DIH assays and heterologous expression experiments suggested that Hsp90 orthologs are the major inhibitory target for monorden in fungi even in the monorden-resistant fungus F. graminearum. Sequence discrepancies in the Hsp90 orthologs from different fungi seem to be the major determinants of the degree of susceptibility to monorden. Although Hsp90 orthologs of Fusarium species did not carry the L34I mutation (Prodromou et al., 2009), there are dozens of Fusarium-specific sequences. Moreover, oomycetes have distinct and dissimilar N- and C-terminal sequences compared to other fungal species (Supplementary Figures S5, S6). Since both N-terminal (IRP036890, HSP90-like ATPase) and C-terminal (IRP037196, HSP90, C-terminal domain) regions are important for the proper function of Hsp90 proteins, we suspect that structural changes derived from sequence variation might be important in determining the resistance profile of fungi and oomycetes toward monorden (Allan et al., 2006). Further in-depth molecular genetic analysis will focus on the characterization of the links between sequence variations in Hsp90 and monorden resistance in fungi and oomycetes.
Several metabolites have been synthesized from monorden in order to improve its stability and antitumor activity. Cycloproparadicicol, synthesized from monorden and cyclopropane, has been shown to have excellent antitumor activity when compared to monorden (Yamamoto et al., 2003a). Novel halohydrin and oxime derivatives of monorden were synthesized and some of these have been shown to have improved antitumor activities compared with their parental compound (Agatsuma et al., 2002). Likewise, we demonstrated that monorden could be used as a lead compound to develop effective fungicides for the treatment of plant pathogenic fungi and oomycetes. A number of fungicides for the control of fungal plant diseases have been developed and they can be divided into four groups based on their mode of action: sterol synthesis inhibitors, mitochondrial electron transport inhibitors, multi-site enzyme inhibitors, nucleic acid and protein synthesis inhibitors (Tillman et al., 1973; Berg et al., 1986; Bartlett et al., 2002). To the best of our knowledge, there is no commercial fungicide with Hsp90 inhibition as the mechanism of action.
Conclusion
Conclusively, we proved that monorden itself is a strong antimicrobial agent for the effective control of several important plant diseases caused by fungi and oomycetes. Because structural changes of Hsp90 protein mainly affect monorden susceptibility, the approach of target-based drug discovery using monorden as a lead compound will be possible to develop novel fungicides for the control of plant diseases caused by fungi and oomycetes.
Data Availability Statement
The datasets generated for this study can be found in the MN006389 and MN006390.
Author Contributions
J-CK, HS, and HN designed and conceived the experiments. SC, HY, C-HB, and JY isolated the endophytic fungi and identified the strains. HN isolated and identified monorden. HN, AP, NY, and GC performed in vitro and in vivo antifungal bioassays. J-HL conducted the drug-induced haploinsufficiency (DIH) screening. SC and HS examined the relationship between Hsp90 sequence variation of different fungi and monorden sensitivity by using Fusarium graminearum transgenic strain expressing HSP90 of C. parasitica. HN, SC, SK, J-HL, AP, NY, HY, C-HB, and JY analyzed the data. All authors contributed to interpretation of the results, read, and approved the final manuscript. HN, SC, HS, and J-CK wrote the manuscript with inputs from all authors.
Funding
This work was supported by a grant from the National Institute of Biological Resources (NIBR), funded by the Ministry of Environment (MOE) of the South Korea (NIBR20201820).
Conflict of Interest
The authors declare that the research was conducted in the absence of any commercial or financial relationships that could be construed as a potential conflict of interest.
Supplementary Material
The Supplementary Material for this article can be found online at: https://www.frontiersin.org/articles/10.3389/fpls.2020.00371/full#supplementary-material
References
Agatsuma, T., Ogawa, H., Akasaka, K., Asai, A., Yamashita, Y., Mizukami, T., et al. (2002). Halohydrin and oxime derivatives of radicicol: synthesis and antitumor activities. Bioorg. Med. Chem. 10, 3445–3454. doi: 10.1016/S0968-0896(02)00260-2
Allan, R. K., Mok, D., Ward, B. K., and Ratajczak, T. (2006). Modulation of chaperone function and cochaperone interaction by novobiocin in the C-terminal domain of Hsp90: evidence that coumarin antibiotics disrupt Hsp90 dimeriation. J. Biol. Chem. 281, 7161–7171. doi: 10.1074/jbc.M512406200
Ayer, W. A., Lee, S. P., Tsuneda, A., and Hiratsuka, Y. (1980). The isolation, identification, and bioassay of the antifungal metabolites produced by Monocillium nordinii. Can. J. Microbiol. 26, 766–773. doi: 10.1139/m80-133
Azevedo, J. L., Maccheroni, W. Jr., Pereira, J. O., and De Araújo, W. L. (2000). Endophytic microorganisms: a review on insect control and recent advances on tropical plants. Electron. J. Biotechnol. 3, 40–65. doi: 10.2225/vol3-issue1-fulltext-4
Bamisile, B. S., Dash, C. K., Akutse, K. S., Keppanan, R., and Wang, L. (2018). Fungal endophytes: beyond herbivore management. Front. Microbiol. 9:544. doi: 10.3389/fmicb.2018.00544
Bartlett, D. W., Clough, J. M., Godwin, J. R., Hall, A. A., and Hamer, M. (2002). The strobilurin fungicides. Pest Manag. Sci. 58, 649–662. doi: 10.1002/ps.520
Berg, D., Büchel, K. H., Plempel, M., and Zywietz, A. (1986). Action mechanisms of cell-division-arresting benzimidazoles and of sterol biosynthesis-inhibiting imidazoles, 1, 2, 4-triazoles, and pyrimidines. Mycoses 29, 221–229. doi: 10.1111/j.1439-0507.1986.tb03958.x
Boulter, J. I., Boland, G. J., and Trevors, J. T. (2002). Evaluation of composts for suppression of dollar spot (Sclerotinia homoeocarpa) of turfgrass. Plant Dis. 86, 405–410. doi: 10.1094/PDIS.2002.86.4.405
Bowden, R. L., and Leslie, J. F. (1999). Sexual recombination in Gibberella zeae. Phytopathology 89, 182–188. doi: 10.1094/PHYTO.1999.89.2.182
Brent, K. J., and Hollomon, D. W. (1995). Fungicide Resistance in Crop Pathogens: How Can it be Managed?. Belgium: Fungicide Resistance Action Committee.
Bui, D.-C., Lee, Y., Lim, J. Y., Fu, M., and Kim, J.-C. (2016). Heat shock protein 90 is required for sexual and asexual development, virulence, and heat shock response in Fusarium graminearum. Sci. Rep. 6:28154. doi: 10.1038/srep28154
Burpee, L. L. (1997). Control of dollar spot of creeping bentgrass caused by an isolate of Sclerotinia homoeocarpa resistant to benzimidazole and demethylation-inhibitor fungicides. Plant Dis. 81, 1259–1263. doi: 10.1094/PDIS.1997.81.11.1259
Cho, J.-Y., Choi, G.-J., Lee, S.-W., Jang, K.-S., and Lim, H.-K. (2006). Antifungal activity against Colletotrichum spp. of curcuminoids isolated from Curcuma longa L. rhizomes. J. Microbiol. Biotechnol. 16, 280–285.
De Silva, N. I., Brooks, S., Lumyong, S., and Hyde, K. D. (2019). Use of endophytes as biocontrol agents. Fungal Biol. Rev. 33, 133–148. doi: 10.1016/j.fbr.2018.10.001
Dean, R., Van Kan, J. A. L., Pretorius, Z. A., Hammond-Kosack, K. E., Di Pietro, A., and Spanu, P. D. (2012). The top 10 fungal pathogens in molecular plant pathology. Mol. Plant Pathol. 13, 414–430. doi: 10.1111/j.1364-3703.2011.00783.x
Delmotte, P., and Delmotte-Plaquee, J. (1953). A new antifungal substance of fungal origin. Nature 171:344. doi: 10.1038/171344a0
Di Petro, A., Gut-Rella, M., Pachlatko, J. P., and Schwinn, F. J. (1992). Role of antibiotics produced by Chaetomium globosum in biocontrol of Pythium ultimum, a causal agent of damping-off. Phytopathology 82, 131–135.
Ding, G., Liu, S., Guo, L., Zhou, Y., and Che, Y. (2008). Antifungal metabolites from the plant endophytic fungus Pestalotiopsis foedan. J. Nat. Prod. 71, 615–618. doi: 10.1021/np070590f
Fujita, K.-I., Irie, M., Ping, X., and Taniguchi, M. (1999). Antifungal activity of radicicol against Mucor flavus IFO 9560. J. Biosci. Bioeng. 88, 380–386. doi: 10.1016/S1389-1723(99)80214-X
Gardes, M., and Bruns, T. D. (1993). ITS primers with enhanced specificity for basidiomycetes−application to the identification of mycorrhizae and rusts. Mol. Ecol. 2, 113–118. doi: 10.1111/j.1365-294x.1993.tb00005.x
Goudjal, Y., Toumatia, O., Yekkour, A., Sabaou, N., Mathieu, F., Zitouni, A., et al. (2014). Biocontrol of Rhizoctonia solani damping-off and promotion of tomato plant growth by endophytic actinomycetes isolated from native plants of Algerian Sahara. Microbiol. Res. 169, 59–65. doi: 10.1016/j.micres.2013.06.014
Hamzah, T. N. T., Lee, S. Y., Hidayat, A., Terhem, R., Faridah-Hanum, I., Mohamed, R., et al. (2018). Diversity and characterization of endophytic fungi isolated from the tropical mangrove species, Rhizophora mucronata, and identification of potential antagonists against the soil-borne fungus, Fusarium solani. Front. Microbiol. 9:1707. doi: 10.3389/fmicb.2018.01707
Hassan, S. E.-D. (2017). Plant growth-promoting activities for bacterial and fungal endophytes isolated from medicinal plant of Teucrium polium L. J. Adv. Res. 8, 687–695. doi: 10.1016/j.jare.2017.09.001
Hellwig, V., Mayer-Bartschmid, A., Müller, H., Greif, G., Kleymann, G., Zitzmann, W., et al. (2003). Pochonins A-F, new antiviral and antiparasitic resorcylic acid lactones from Pochonia chlamydosporia var. catenulata. J. Nat. Prod. 66, 829–837. doi: 10.1021/np020556v
Joshi, B. K., Gloer, J. B., and Wicklow, D. T. (2002). Bioactive natural products from a sclerotium-colonizing isolate of Humicola fuscoatra. J. Nat. Prod. 65, 1734–1737. doi: 10.1021/np020295p
Kamoun, S., Furzer, O., Jones, J. D. G., Judelson, H. S., Ali, G. S., Dalio, R. J., et al. (2015). The top 10 oomycete pathogens in molecular plant pathology. Mol. Plant Pathol. 16, 413–434. doi: 10.1111/mpp.12190
Kim, D.-U., Hayles, J., Kim, D., Wood, V., Park, H.-O., Won, M., et al. (2010). Analysis of a genome-wide set of gene deletions in the fission yeast Schizosaccharomyces pombe. Nat. Biotechnol. 28:617. doi: 10.1038/nbt.1628
Kim, J.-C., Choi, G. J., Lee, S.-W., Kim, J.-S., Chung, K. Y., Cho, K. Y., et al. (2004). Screening extracts of Achyranthes japonica and Rumex crispus for activity against various plant pathogenic fungi and control of powdery mildew. Pest Manag. Sci. 60, 803–808. doi: 10.1002/ps.811
Kim, J.-C., Choi, G. J., Park, J.-H., Kim, H. T., and Cho, K. Y. (2001). Activity against plant pathogenic fungi of phomalactone isolated from Nigrospora sphaerica. Pest Manag. Sci. 57, 554–559. doi: 10.1002/ps.318
Ko, W.-H., Yang, C.-H., Lin, M.-J., Chen, C.-Y., and Tsou, Y.-J. (2011). Humicola phialophoroides sp. nov. from soil with potential for biological control of plant diseases. Bot. Stud. 52, 197–202.
Kumar, S., and Kaushik, N. (2012). Metabolites of endophytic fungi as novel source of biofungicide: a review. Phytochem. Rev. 11, 507–522. doi: 10.1007/s11101-013-9271-y
Kumar, S., Stecher, G., Li, M., Knyaz, C., and Tamura, K. (2018). MEGA X: molecular evolutionary genetics analysis across computing platforms. Mol. Biol. Evol. 35, 1547–1549. doi: 10.1093/molbev/msy096
Latijnhouwers, M., De Wit, P. J. G. M., and Govers, F. (2003). Oomycetes and fungi: similar weaponry to attack plants. Trends Microbiol. 11, 462–469. doi: 10.1016/j.tim.2003.08.002
Lee, J., Lee, T., Lee, Y.-W., Yun, S.-H., and Turgeon, B. G. (2003). Shifting fungal reproductive mode by manipulation of mating type genes: obligatory heterothallism of Gibberella zeae. Mol. Microbiol. 50, 145–152. doi: 10.1046/j.1365-2958.2003.03694.x
Lee, J., Son, H., Lee, S., Park, A. R., and Lee, Y.-W. (2010). Development of a conditional gene expression system using a zearalenone-inducible promoter for the ascomycete fungus Gibberella zeae. Appl. Environ. Microbiol. 76, 3089–3096. doi: 10.1128/AEM.02999-09
Lee, J.-H., Yeon, J.-H., Kim, H., Roh, W., Chae, J., Park, H. O., et al. (2012). The natural anticancer agent plumbagin induces potent cytotoxicity in MCF-7 human breast cancer cells by inhibiting a PI-5 kinase for ROS generation. PLoS One 7:e45023. doi: 10.1371/journal.pone.0045023
Lee, J. Y., Lee, J. Y., Moon, S. S., and Hwang, B. K. (2005). Isolation and antifungal activity of 4-phenyl-3-butenoic acid from Streptomyces koyangensis strain VK-A60. J. Agric. Food Chem. 53, 7696–7700. doi: 10.1021/jf050957r
Lee, S., Son, H., Lee, J., Min, K., Choi, G. J., Kim, J. C., et al. (2011). Functional analyses of two acetyl coenzyme A synthetases in the ascomycete Gibberella zeae. Eukaryot. Cell 10:1043. doi: 10.1128/EC.05071-11
Li, B., Ravnskov, S., Xie, G., and Larsen, J. (2007). Biocontrol of Pythium damping-off in cucumber by arbuscular mycorrhiza-associated bacteria from the genus Paenibacillus. BioControl 52, 863–875. doi: 10.1007/s10526-007-9076-2
Li, H., Soares, M. A., Torres, M. S., Bergen, M., and White, J. F. Jr. (2015). Endophytic bacterium, Bacillus amyloliquefaciens, enhances ornamental hosta resistance to diseases and insect pests. J. Plant Interact. 10, 224–229. doi: 10.1080/17429145.2015.1056261
Lo, C. T., Nelson, E. B., and Harman, G. E. (1997). Improved biocontrol efficacy of Trichoderma harzianum 1295-22 for foliar phases of turf diseases by use of spray applications. Plant Dis. 81, 1132–1138. doi: 10.1094/PDIS.1997.81.10.1132
McCapra, F., Scott, A. I., Delmotte, P., Delmotte-Plaquee, J., and Bhacca, N. S. (1964). The constitution of monorden, an antibiotic with tranquilising action. Tetrahedron Lett. 5, 869–875. doi: 10.1016/S0040-4039(00)90398-8
Mirrington, R. N., Ritchie, E., Shoppee, C. W., Taylor, W. C., and Sternhell, S. (1964). The constitution of radicicol. Tetrahedron Lett. 5, 365–370. doi: 10.1016/0040-4039(64)80029-0
Nguyen, H. T., Kim, S., Yu, N. H., Park, A. R., Yoon, H., Bae, C. H., et al. (2019). Antimicrobial activities of an oxygenated cyclohexanone derivative isolated from Amphirosellinia nigrospora JS-1675 against various plant pathogenic bacteria and fungi. J. Appl. Microbiol. 126, 894–904. doi: 10.1111/jam.14138
Nozawa, K., and Nakajima, S. (1979). Isolation of radicicol from Penicillium luteo-aurantium, and meleagrin, a new metabolite from Penicillium meleagrinum. J. Nat. Prod. 42, 374–377. doi: 10.1021/np50004a004
Pandey, P. K., Singh, S., Singh, M. C., Singh, A. K., and Yadav, S. K. (2018). “Diversity, ecology, and conservation of fungal and bacterial endophytes,” in Microbial Resource Conservation, eds S. K. Sharma and A. Varma (New York, NY: Springer), 393–430.
Parnell, S., Van Den Bosch, F., and Gilligan, C. A. (2006). Large-scale fungicide spray heterogeneity and the regional spread of resistant pathogen strains. Phytopathology 96, 549–555. doi: 10.1094/PHYTO-96-0549
Prodromou, C., Nuttall, J. M., Millson, S. H., Roe, S. M., Sim, T.-S., Tan, D., et al. (2009). Structural basis of the radicicol resistance displayed by a fungal Hsp90. ACS Chem. Biol. 4, 289–297. doi: 10.1021/cb9000316
Radhakrishnan, R., Khan, A. L., Kang, S. M., and Lee, I.-J. (2015). A comparative study of phosphate solubilization and the host plant growth promotion ability of Fusarium verticillioides RK01 and Humicola sp. KNU01 under salt stress. Ann. Microbiol. 65, 585–593. doi: 10.1007/s13213-014-0894-z
Rodriguez, F., and Pfender, W. F. (1997). Antibiosis and antagonism of Sclerotinia homoeocarpa and Drechslera poae by Pseudomonas fluorescens Pf-5 in vitro and in planta. Phytopathology 87, 614–621. doi: 10.1094/PHYTO.1997.87.6.614
Roe, S. M., Prodromou, C., O’brien, R., Ladbury, J. E., Piper, P. W., Pearl, L. H., et al. (1999). Structural basis for inhibition of the Hsp90 molecular chaperone by the antitumor antibiotics radicicol and geldanamycin. J. Med. Chem. 42, 260–266. doi: 10.1021/jm980403y
Ryan, R. P., Germaine, K., Franks, A., Ryan, D. J., and Dowling, D. N. (2008). Bacterial endophytes: recent developments and applications. FEMS Microbiol. Lett. 278, 1–9. doi: 10.1111/j.1574-6968.2007.00918.x
Santoyo, G., Moreno-Hagelsieb, G., Orozco-Mosqueda, C., and Glick, B. R. (2016). Plant growth-promoting bacterial endophytes. Microbiol. Res. 183, 92–99. doi: 10.1016/j.micres.2015.11.008
Sharma, S. V., Agatsuma, T., and Nakano, H. (1998). Targeting of the protein chaperone, HSP90, by the transformation suppressing agent, radicicol. Oncogene 16, 2639. doi: 10.1038/sj.onc.1201790
Smetanina, O. F., Kuznetsova, T. A., Gerasimenko, A. V., Kalinovsky, A. I, Pivkin, M. V., Dmitrenok, P. C., et al. (2004). Metabolites of the marine fungus Humicola fuscoatra KMM 4629. Russ. Chem. Bull. 53, 2643–2646. doi: 10.1007/s11172-005-0167-x
Soga, S., Neckers, L. M., Schulte, T. W., Shiotsu, Y., Akasaka, K., Narumi, H., et al. (1999). KF25706, a novel oxime derivative of radicicol, exhibits in vivo antitumor activity via selective depletion of Hsp90 binding signaling molecules. Cancer Res. 59:2931.
Son, H., Lee, J., Park, A. R., and Lee, Y.-W. (2011). ATP citrate lyase is required for normal sexual and asexual development in Gibberella zeae. Fungal Genet. Biol. 48, 408–417. doi: 10.1016/j.fgb.2011.01.002
Stadler, M., Tichy, H.-V., Katsiou, E., and Hellwig, V. (2003). Chemotaxonomy of Pochonia and other conidial fungi with Verticillium-like anamorphs. Mycol. Prog. 2, 95–122. doi: 10.1007/s11557-006-0048-1
Strobel, G., and Daisy, B. (2003). Bioprospecting for microbial endophytes and their natural products. Microbiol. Mol. Biol. Rev. 67, 491–502. doi: 10.1128/MMBR.67.4.491-502.2003
Suthiwong, J., Thongsri, Y., and Yenjai, C. (2017). A new furanocoumarin from the fruits of Scaevola taccada and antifungal activity against Pythium insidiosum. Nat. Prod. Res. 31, 453–459. doi: 10.1080/14786419.2016.1188100
Szklarczyk, D., Gable, A. L., Lyon, D., Junge, A., Wyder, S., Huerta-Cepas, J., et al. (2018). STRING v11: protein–protein association networks with increased coverage, supporting functional discovery in genome-wide experimental datasets. Nucleic Acids Res. 47, D607–D613. doi: 10.1093/nar/gky1131
Tabata, N., Suzumura, Y., Tomoda, H., Masuma, R., Haneda, K., Kishi, M., et al. (1993). Xanthoquinodins, new anticoccidial agents produced by Humicola sp. J. Antibiot. 46, 749–755. doi: 10.7164/antibiotics.46.749
Tamura, K., and Nei, M. (1993). Estimation of the number of nucleotide substitutions in the control region of mitochondrial DNA in humans and chimpanzees. Mol. Biol. Evol. 10, 512–526. doi: 10.1093/oxfordjournals.molbev.a040023
Tillman, R. W., Siegel, M. R., and Long, J. W. (1973). Mechanism of action and fate of the fungicide chlorothalonil (2,4,5,6-tetrachloroisophthalonitrile) in biological systems: I. Reactions with cells and subcellular components of Saccharomyces pastorianus. Pest. Biochem. Physiol. 3, 160–167. doi: 10.1016/0048-3575(73)90100-4
Turbyville, T. J., Wijeratne, E. M. K., Liu, M. X., Burns, A. M., Seliga, C. J., Luevano, L. A., et al. (2006). Search for Hsp90 inhibitors with potential anticancer activity: isolation and SAR studies of radicicol and monocillin I from two plant-associated fungi of the Sonoran desert. J. Nat. Prod. 69, 178–184. doi: 10.1021/np058095b
Van de Peer, Y., and De Wachter, R. (1997). Evolutionary relationships among the eukaryotic crown taxa taking into account site-to-site rate variation in 18S rRNA. J. Mol. Evol. 45, 619–630. doi: 10.1007/PL00006266
Vilgalys, R., and Hester, M. (1990). Rapid genetic identification and mapping of enzymatically amplified ribosomal DNA from several Cryptococcus species. J. Bacteriol. 172:4238. doi: 10.1128/jb.172.8.4238-4246.1990
Wang, L.-W., Xu, B.-G., Wang, J.-Y., Su, Z.-Z., Lin, F.-C., Zhang, C. L., et al. (2012). Bioactive metabolites from Phoma species, an endophytic fungus from the Chinese medicinal plant Arisaema erubescens. Appl. Microbiol. Biotechnol. 93, 1231–1239. doi: 10.1007/s00253-011-3472-3
Wang, X. W., Houbraken, J., Groenewald, J. Z., Meijer, M., Andersen, B., Nielsen, K. F., et al. (2016). Diversity and taxonomy of Chaetomium and chaetomium-like fungi from indoor environments. Stud. Mycol. 84, 145–224. doi: 10.1016/j.simyco.2016.11.005
Wang, X. W., Yang, F. Y., Meijer, M., Kraak, B., and Sun, B. D. (2019). Redefining Humicola sensu stricto and related genera in the Chaetomiaceae. Stud. Mycol. 93, 65–153. doi: 10.1016/j.simyco.2018.07.001
White, T. J., Bruns, T., Lee, S., and Taylor, W. J. (1990). “Amplification and direct sequencing of fungal ribosomal RNA genes for phylogenetics,” in PCR Protocols: A Guide to Methods and Applications, eds M. A. Innis, D. H. Gelfand, J. J. Sninsky, and T. J. White (San Diego, CA: Academic Press), 315–322.
Wicklow, D. T., Jordan, A. M., and Gloer, J. B. (2009). Antifungal metabolites (monorden, monocillins I. II, III) from Colletotrichum graminicola, a systemic vascular pathogen of maize. Mycol. Res. 113, 1433–1442. doi: 10.1016/j.mycres.2009.10.001
Wicklow, D. T., Joshi, B. K., Gamble, W. R., Gloer, J. B., and Dowd, P. F. (1998). Antifungal metabolites (monorden, monocillin IV, and cerebrosides) from Humicola fuscoatra Traaen NRRL 22980, a mycoparasite of Aspergillus flavus sclerotia. Appl. Environ. Microbiol. 64, 4482–4484.
Winssinger, N., and Barluenga, S. (2007). Chemistry and biology of resorcylic acid lactones. Chem. Commun. 1, 22–36. doi: 10.1039/B610344H
Yamamoto, K., Garbaccio, R. M., Stachel, S. J., Solit, D. B., Chiosis, G., Rosen, N., et al. (2003a). Total synthesis as a resource in the discovery of potentially valuable antitumor agents: cycloproparadicicol. Angew. Chem. Int. Edit. 42, 1280–1284. doi: 10.1002/anie.200390329
Keywords: antifungal activity, disease control efficacy, Hsp90, mode of action, monorden
Citation: Nguyen HTT, Choi S, Kim S, Lee J-H, Park AR, Yu NH, Yoon H, Bae C-H, Yeo JH, Choi GJ, Son H and Kim J-C (2020) The Hsp90 Inhibitor, Monorden, Is a Promising Lead Compound for the Development of Novel Fungicides. Front. Plant Sci. 11:371. doi: 10.3389/fpls.2020.00371
Received: 11 October 2019; Accepted: 16 March 2020;
Published: 02 April 2020.
Edited by:
Keiko Yoshioka, University of Toronto, CanadaReviewed by:
Mark Findlay Belmonte, University of Manitoba, CanadaCarla Caruso, University of Tuscia, Italy
Copyright © 2020 Nguyen, Choi, Kim, Lee, Park, Yu, Yoon, Bae, Yeo, Choi, Son and Kim. This is an open-access article distributed under the terms of the Creative Commons Attribution License (CC BY). The use, distribution or reproduction in other forums is permitted, provided the original author(s) and the copyright owner(s) are credited and that the original publication in this journal is cited, in accordance with accepted academic practice. No use, distribution or reproduction is permitted which does not comply with these terms.
*Correspondence: Hokyoung Son, hogongi7@snu.ac.kr; Jin-Cheol Kim, kjinc@chonnam.ac.kr; kjinc@jnu.ac.kr