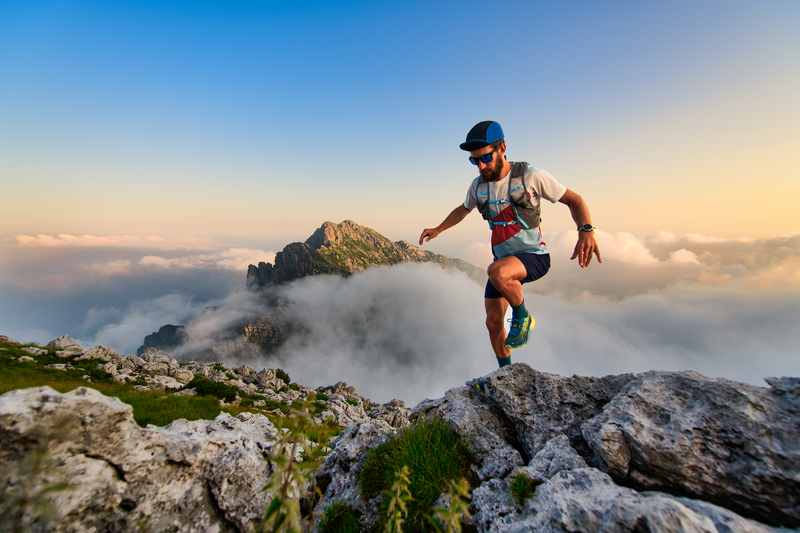
94% of researchers rate our articles as excellent or good
Learn more about the work of our research integrity team to safeguard the quality of each article we publish.
Find out more
ORIGINAL RESEARCH article
Front. Plant Sci. , 24 March 2020
Sec. Plant Biotechnology
Volume 11 - 2020 | https://doi.org/10.3389/fpls.2020.00294
In the process of acquiring mutants mediated by CRISPR/Cas9, plantlets are often regenerated from both mutated and non-mutated cells in a random manner, which increase the odds of chimeric mutated plant. In general, it’s necessary to infect more explants or grow to next generation for the need of generating more biallelic or homozygous mutants. In present study, an efficient way of obtaining biallelic or homozygous mutated lines via fast-growing hairy root system without increasing numbers of infected explants or prolonging sexual propagation generation is reported. The fast growing lateral branches of hair roots are originated deep within the parental root from a small number of founder cells at the periphery, and therefore were employed as a library that classify different editing types in different lateral branches in which the homozygous or biallelic lines were screened. Here, MtPDS was employed in a proof-of-concept experiment to evaluate the efficiency of genome editing with our hairy root system. Homozygous/biallelic mutations were found only 1 of the 20 lines in the 1st generation hairy roots, and 8 lines randomly selected were cultured to obtain their branch roots, homozygous/biallelic mutations were found in 6 of the 8 lines in their branch roots. We also tested the method with MtCOMT gene and got the same result. All of the seedlings regenerated from the homozygous/biallelic hairy root mutation lines of MtPDS displayed albino phenotypes. The entire process from vector design to the recovery of plantlets with homozygous/biallelic mutations took approximately 4.5–6.5 months. The whole process could bring inspiration for efficiently generating homozygous/biallelic mutants through CRISPR/Cas9 system from the hairy root or root system of a chimeric mutated transformants, especially for the rare and endangered plants whose explants sources are very limited or the plants that lack of tissue culture and rapid propagation system.
The clustered regularly interspaced short palindromic repeats (CRISPR) and CRISPR-associated protein 9 (Cas9) system is being harnessed as a powerful tool for gene engineering in crop improvement (Xie et al., 2015; Shah et al., 2018). In the majority of cases, an integration construct comprising CRISPR-Cas9, gRNA expression cassettes and a resistance gene (hph, bar, npt II, etc.) is transferred into plants using Agrobacterium tumefaciens, and the transformants are obtained through a selection process. As long as the resistance gene sequence is integrated into the plant genome and is expressed normally, transformed plants, tissues or calli can survive selection and grow regardless of whether the target sequences are edited by the CRISPR-Cas9 system or not. This results in the recovery of plants containing both mutated and non-mutated cells in a random manner, and increases the odds of recovering chimeric mutated plants (Nishitani et al., 2016; Pan et al., 2016). For example, in a previous study in rice, it was found that a prolonged tissue culture period could increase the chance of inducing de novo mutations in non-mutated cells, but the authors found no evidence that a prolonged tissue culture period could increase the rate of recovery of homozygous or biallelic mutants (Mikami et al., 2015). When introducing the CRISPR/Cas9 construct into Arabidopsis using the floral-dip genetic transformation method, homozygous or biallelic mutations were found in T2 plants but not in T0 or T1 plants (Feng et al., 2014). This is because the gene modifications detected in T1 plants occurred mostly in somatic cells; in other words, the T1 plants were chimeric for mutations in the target gene, and it was necessary to isolate plants with homozygous or biallelic mutations in the next generation (Feng et al., 2014).
In addition to A. tumefaciens, Agrobacterium rhizogenes, which contains the Ri plasmid and induces hairy roots, is another useful system for generating transgenics. This system has some obvious advantages. First of all, the Ri T-DNA, which induces hairy roots, can be transferred into plant cells along with the Ti T-DNA carrying the target gene. Thus, the CRISPR/Cas9 and gRNA expression cassettes could be transferred into the plant together with the Ri T-DNA via A. rhizogenes transformation (Li et al., 2017; Nakayasu et al., 2018). Secondly, hairy roots have the ability of infinite proliferation, and it takes only a very short time, about 5–10 days, to obtain new lateral branches. More importantly, it is easier to induce hairy roots using A. rhizogenes than to generate transformants using A. tumefaciens. Therefore, an A. rhizogenes-mediated transformation system has been established in many plants (Shi et al., 2014; Mehrotra et al., 2015; Li et al., 2017; Huang et al., 2018; Hudzieczek et al., 2018). The fact that only a small number of founder cells at the periphery of the parental root could be inherited and transferred their genetic materials in new formed lateral branches, but not retain all the calli as the situation in rice (Gibbs and Coates, 2014). This made us realize that the fast-growing branches of hairy roots could be considered a library in which different editing types produced by the CRISPR/Cas9 genome-editing system can be classified in different lateral branches. It is very likely that homozygous and biallelic mutants could be rapidly isolated from the branches of a hairy root line with chimeric mutations in the target gene or from newly created mutations in the process of subculture. In addition, by sub-culturing the hairy roots, more branch roots were gained in which more homozygous and biallelic mutated branches could be efficiently identified, without the need for time-consuming sexual reproduction as that required in transgenic Arabidopsis plants using the floral dip method. In addition, most plant hairy roots can be regenerated into a complete, functional plant that retains the original features of the plant from seed, which allows assessing gene function at a whole plant level (Choi et al., 2004; Crane et al., 2006; Zhou et al., 2007; Jia et al., 2008; Subotic et al., 2009; Sun et al., 2013; Sharafi et al., 2014; Hou et al., 2014; Habibi et al., 2016; Hudzieczek et al., 2018). The advantages of the hairy root system combined with the fact that the CRISPR-Cas9 system is well-established and has been used to efficiently perform gene editing in many plants (Feng et al., 2014; Nishitani et al., 2016; Qi et al., 2016; Wang P. C. et al., 2018) inspired us to engineer a hairy root-based system to efficiently obtain homozygous and biallelic mutants.
To establish this system, we chose to use Medicago truncatula, which has been adopted as a model for the study of genetics and genomics of forage legumes and is particularly useful for the study of root endosymbiotic associations (Horvath et al., 2015; Dhanushkodi et al., 2018), as our research subject. Fertile true transgenic plants have been regenerated from A. rhizogenes-transformed hairy roots of M. truncatula (Crane et al., 2006). In a previous study, it was reported that the CRISPR-Cas9 system could function in M. truncatula when introduced both through A. tumefaciens-mediated leaf disc transformation (Meng et al., 2017) and A. rhizogenes-mediated hairy root induction (Michno et al., 2015). In the present study, we demonstrate a fast and efficient method to obtain homozygous and biallelic mutants based on the M. truncatula hairy root system. We used a tRNA-gRNA expression strategy, in which multiple gRNAs are interspaced with tRNAs; the tRNAs work as a potential transcriptional enhancers for RNA polymerase III, boosting transcription, and can be precisely excised in vivo by endogenous RNases, releasing mature gRNAs (Xie et al., 2015; Cermak et al., 2017). Since it results in a high frequency of mutagenesis (up to 100%) and the tRNA-processing system is ubiquitous, this strategy has been successfully applied to many types of organisms (Qi et al., 2016; Dong et al., 2017; Kirchner et al., 2017; Deaner et al., 2018; Hashimoto et al., 2018; Wang Z. et al., 2018). In present work, a few homozygous/biallelic mutants were detected in the 1st round of hairy root culture produced after transformation, and then a large number of additional homozygous and biallelic mutants were rapidly isolated in branches subcultured from the transgenic hairy roots. Our results indicate that the numerous CRISPR/Cas9-mediated homozygous/biallelic mutants can be obtained in one month after two rounds of hairy root culture. This hairy root selection system can facilitate a highly efficient generation of homozygous/biallelic mutants for genome engineering of forage and other agriculturally important crops.
Agrobacterium rhizogenes strain LBA9402 was employed to induce hairy roots of M. truncatula because this strain only has rifampicin resistance, which facilitates co-transformation with Ti T-DNAs carrying various other resistance genes. In addition, we found that of all the A. rhizogenes strains tested, LBA9402 exhibited the highest hairy root induction rate (Supplementary Table S1). Furthermore, we chose MtPDS as the target gene to examine the efficiency of genome editing in our hairy root system. One target sequence in the second exon of MtPDS containing a MwoI restriction endonuclease cutting site next to the NGG region was selected for genotyping according to Ma et al. (2016; Figure 1A). Moreover, a tRNA-gRNA strategy was used for enhancing gRNA expression as described by Xie et al. (2015; Figure 1B). The CRISPR/Cas9 sequence and the gRNA expression cassette were cloned into a modified pYLCRISPR/Cas9 35S-B vector (Supplementary Figure S1). This vector was transferred into M. truncatula leaf explants together with the Ri T-DNA via LBA9402-mediated transformation. Hairy roots were induced from the leaf explants 10–15 days after inoculation (Figures 1C–E). Transgenic hairy root fragments approximately 2–4 cm length were cut from the leaves and then subcultured on SH solid medium (1st round of culture) (Figure 1F). A fragment sampled from the main root without any lateral roots was used for genomic DNA extraction. Twenty-one transgenic hairy root lines (TLs) were randomly selected, and polymerase chain reaction (PCR) analysis showed that all of these lines contained rolB, which is a marker of hairy roots induced by A. rhizogenes. Of the hairy root lines, 95.5% were co-transformed with the modified binary vector pYLCRISPR/Cas9 35S-B carrying both bar and Cas9, none of them was amplified the virG product which excluded the A. rhizogenes contamination (Figures 1G–J).
Figure 1. Identification of co-transformed hairy root lines of M. truncatula induced by A. rhizogenes strain LBA9402. (A) Schematic of the MtPDS gene and the selected target sequence. The nucleotides in brown font are part of the MwoI recognition site, and the green font indicates the PAM. (B) Schematic illustration of the modified CRISPR/Cas9 construct used in this study. (C) The infected explants were transferred to selection medium containing 1.5 mg/L PPT. (D) Hairy roots began to appear from the leaf explants after inoculation with LBA9402 for about 10–15 days. (E) Approximately 10–15 hairy root lines were induced from the leaf blade. (F) Each line from the first generation of hairy roots was subcultured on a new plate to allow further growth. (G–J) PCR screening of DNA samples from the first generation. Untransformed wild type roots served as a negative control, and LBA9402 harboring the CRISPR/Cas9 plasmid was used as a positive control. The expected sizes of the bar, Cas9, rolB, and virG bands are indicated. TL, transgenic hairy root line.
The region surrounding the targeted sequence in MtPDS was amplified by PCR from 20 co-transformed hairy root lines, and the PCR products were then subjected to MwoI restriction endonuclease analysis. The 1,347 bp PCR product amplified from the wild-type control was completely digested into two fragments of 978 and 369 bp by MwoI. In contrast, the PCR products amplified from all 20 co-transformed hairy root lines included bands at 1,347 bp that were not digested by MwoI, implying that the MwoI cleavage sites were disrupted by genome editing (Figure 2A). There was no digested bands in line 13, which suggesting that the target site of MtPDS in both alleles was edited (Figure 2A). Sequencing further revealed that line 13 was homozygous for a 1 bp insertion (T) (Figure 2B). Moreover, we selected three co-transformed hairy root lines 4, 5, and 11, which had digested bands, for further analysis. The sequence of the undigested PCR product amplified from line 4 showed that this line was a chimera with both the wild-type genotype and various types of mutations, including deletions and insertions (i1, d2, d84, and d57/i4), at the MtPDS target site (Figure 2B). In contrast, line 5 was a heterozygote containing the wild-type allele and a mutant allele with a 1 bp deletion (d1) in the target site (Figure 2B). There was an additional band smaller than the expected 1,347 bp in line 11 (Figure 2B). Unlike the 1,347 bp PCR fragment, the smaller fragment could not be digested by MwoI (Supplementary Figure S2). Sequencing analysis revealed that the short sequence contained a 577 bp deletion, whereas the long sequence contained a 37 bp insertion (d577i37) in the target site (Figure 2B).
Figure 2. Screening for biallelic or homozygous mutated lines in the 1st generation of hairy root lines. (A) PCR/restriction endonuclease assay to identify homozygous/biallelic lines for CRISPR/Cas9 genome edited mutations. Genotyping results for twenty 1st generation hairy root lines are shown. The 1,347 bp band from amplification of MtPDS was cut into two bands 978 and 360 bp in size by the MwoI restriction endonuclease. Lines 1–20, PCR products of co-transformed hairy root lines digested with MwoI. Line 13 was homozygous for a mutation in MtPDS; the 1,347 bp band could not be digested. The PCR products in the other lanes were amplified from chimeric or heterozygous mutated hairy root lines; the 1,347 bp band was partially digested by MwoI. M, 2 kb DNA marker. WT, digested PCR product amplified from the wild-type control. (B) Targeted genome editing on MtPDS gene in M. truncatula hairy roots. The sequence of MtPDS of untransformed hairy root and types of mutations in TL13, TL4, TL5, and the small band of TL11 in the first generation. Red bases indicate the target sequence; Blue bases indicate the PAM. The deletion is indicated by a dashed lines, and the inserted sequences are shown in blue letters, and the replaced sequences are shown in purple letters. d#, number of bases deleted from the target site. i#, number of bases inserted at the target site.
Although the 20 co-transformed hairy root lines from the 1st round of culture were efficiently edited, all of them except line 13 were either heterozygous or chimeric (Figure 2A). Eight co-transformed hairy root lines from the 1st round of culture including line 4, 5, 11, and 13 were subcultured for further investigation. Lateral roots produced from the primary roots in the 1st round of culture were named 2nd generation roots. Similarly, the lateral roots produced by the 2nd generation roots were named the 3rd generation roots, and so on (Figure 3A). Ten 2nd generation hairy roots derived from each 1st generation line were collected for PCR amplification and MwoI restriction endonuclease analysis. At least one homozygous/biallelic root was recovered from the six out of eight lines selected based on the presence of a single indigestible band (Figure 3B). Sequencing data of the PCR products further revealed that, like line 13 in the 1st generation, line 6 was also a homozygous mutant. However, line 6 contained a 3 bp deletion (d3) in the target site of MtPDS (Figure 3C). The homozygous mutation lines were evaluated for five successive generations, it was showed that their PCR products still could not be cut by MwoI (Figures 3C,D). We continued to subcultured lines 6 and 13 and randomly selected their hairy roots to sequence (data not show) analysis, it revealed that the mutation type remained unchanged with continued subculturing (Supplementary Figure S5). In contrast, line 1 was found to be a biallelic mutant with a 50 bp (d50) deletion in one allele and a 5 bp (d5) deletion in the second allele of the target site (Figure 3D). Other types of mutation were found in the biallelic mutation lines: d1/d1 (1 bp deletions at different positions in the two alleles) in line 7–3, d1/d5 in line 12-3, d10/d4 in line 15-1, d1/d9 in line 20-2, and d5/d50 (r1) in line 20-7 (Figure 3D). Surprisingly, homozygous/biallelic mutations were not detected in the second generation roots of lines 4 and 5 (Figure 3B). Therefore, we examined the third and fourth generation roots of these lines. Unfortunately, no homozygous/biallelic mutations were found in the ten lines selected for analysis (Supplementary Figure S3).
Figure 3. Screening of biallelic or homozygous mutation lines in the 2nd generation of transgenic hairy root lines. (A) Schematic description of the different generations of hairy roots harvested. (B) Identification of eight biallelic or homozygous hairy root mutation lines in the 2nd generation of lines 1, 4, 5, 6, 7, 12, 15, and 20. DNA samples from independent lines were analyzed for mutations using the PCR/restriction endonuclease assay. M, 2 kb DNA maker. WT, digested wild-type control; the 1,347 bp band corresponding to MtPDS was cut into two bands, 978 and 360 bp in size, by the MwoI restriction endonuclease. Lanes 1–10, digested PCR products of independent transgenic hairy roots from the 2nd generation of TL1, TL4, TL5, TL6, TL7, TL12, TL15, and TL20 with MwoI. White arrowheads indicate the bands amplified from biallelic or homozygous hairy root mutation lines. (C) PCR/restriction endonuclease assay of five successive generations of line 6 (2nd to 6th) and line 13 (1st to 5th). In all lines of each generation, the 1,347 bp band could not be digested. (D) Types of mutations in the biallelic or homozygous hairy root mutation lines screened from the 2nd generation of hairy roots. Red and green bases indicate the target sequence and PAM region, respectively. Deletions are indicated by dashed lines. d#, number of bases deleted from the target site. r#, number of bases replaced at the target site.
In order to test the efficiency and stability of our experiment, we also designed a target site of another gene MtCOMT containing a BslI restriction endonuclease cutting sites at target site (Supplementary Figure S4A) and a tRNA-gRNA expression pattern was also used. Fifteen co-transformed hairy root lines of 1st generation were selected for BslI digestion analysis (Supplementary Figure S4B). A total of 447 bp PCR product including the target site amplified from wide type MtCOMT was totally cut into two bands of 255 and 192 bp by BslI restriction endonucleases. One PCR product of the selected lines was found indigestible (Supplementary Figure S4B). Sequencing analysis indicated it was a homozygous mutant with 4-bp deletions at target sites of the two MtCOMT alleles (Supplementary Figure S4C). Four co-transformed lines, TL 1, TL 6, TL 9, and TL 11 were selected for 2nd round selection. Ten 2nd generation hairy roots derived from each 1st generation line were collected for PCR amplification and BslI restriction endonuclease analysis. The homozygous/biallelic lines were found in three lines with the indigestible band as a symbol (Supplementary Figure S4D). Sequence results showed the mutation types of these homozygous/biallelic lines were as follows: d6/d3 in TL 1-8, d3 inTL6-4, d3/d117 in TL6-5, d4/d64 in TL11-2 (Supplementary Figure S4E). No homozygous/biallelic mutations were found in the 10 lines of TL9, sequence analysis showed that it was a chimeric mutant with at least three mutation types (Supplementary Figure S4E).
To obtain genome edited plantlets, calli were induced from the hairy roots with homozygous and biallelic mutations by culturing them on SH3a medium. Calli were then subcultured on MSBK medium for shoot regeneration as described by Jiang et al. (2019). We found that all regenerated plantlets derived from the homozygous/biallelic hairy root mutation lines displayed albino phenotypes as anticipated (Figure 4). The entire processes from target site design to recovery of regenerants took 4.5–6.5 months.
Figure 4. Regeneration of biallelic and homozygous mutated hairy root lines. (A) A represented hairy root line (TL13) with homogenous editing on MtPDS were cut into 1–2 cm lengths and transferred into callus induction medium. (B) Calli induced from hairy root fragments. (C) Close-up image of hairy root calli. (D) Plantlets with biallelic or homozygous mutations regenerated from hairy root calli. (E) Close-up image of plantlets with biallelic or homozygous mutations regenerated from hairy root calli. (F) Schematic of the process for obtaining biallelic and homozygous hairy root lines and the time required for each step. The entire process from vector design to the recovery of plantlets took approximately 4.5–6.5 months.
The expression levels of Cas9 in nine selected hairy root lines from the 1st round of culture were determined. We found great differences in Cas9 expression levels among the nine hairy root lines (Figure 5). Moreover, Cas9 expression level was not correlated with the efficiency of target gene editing in the co-transformed hairy roots. For example, a relatively low level of Cas9 expression was observed in the homozygous mutant (line 13) and the heterozygous and chimeric mutants (lines 1, 4, 6, and 12). In addition, a relatively high level of Cas9 expression was observed in some heterozygous and chimeric mutation lines (Figure 5).
Figure 5. Expression levels of Cas9 in co-transformed hairy root lines. Quantitative real-time PCR analysis of Cas9 expression levels in 1st generation hairy roots of nine lines. Root samples 10–15 days after inoculation were collected from untransformed wild type roots and co-transformed hairy root lines 1, 4, 5, 6, 7, 12, 13, 15, and 20. M. truncatula Actin (Medtr3g095530.2) was used as the reference for normalization. Value are mean ± SD (n = 3). WT, untransformed wild type roots.
Alfalfa (Medicago sativa) is the main source of vegetable protein in meat and milk production systems worldwide and is well known as “the queen of forage crops” because of its high protein content and high yield productivity (Stritzler et al., 2018). Moreover, this legume is also an ideal model for studying the interaction between rhizobia and plant roots (Crane et al., 2006). However, alfalfa has an autotetraploid genome, which limits the ability to identify gene functions (Meng et al., 2017). M. truncatula is a close relative of alfalfa and has several characteristics that make it a good model system, including a diploid genome that is easy to modify, a short life cycle, and a high level of natural diversity (Meng et al., 2017). Studies of M. truncatula could provide theoretical support for genetically modifying alfalfa. The new technology CRISPR/Cas9 is a powerful tool for generating targeted gene mutations and was previously applied successfully in M. truncatula (Meng et al., 2017). However, the rate of homozygous/biallelic mutant recovery was low, only 10% (Meng et al., 2017). In addition, the aseptic seedlings obtained by tissue culture process in previous study were difficult to develop roots, which reduced the recovery of homozygous/biallelic mutants as well (Crane et al., 2006).
Hairy roots of M. truncatula can be regenerated into complete plants that are easy to root (Crane et al., 2006). Therefore, in this paper, we used hairy roots as a system to implement CRISPR/Cas9 genome editing technology. Each hairy root line could be considered as being derived from an independent editing event. Hairy roots and their branches develop from only a small number of cells (Gibbs and Coates, 2014). That is to say, although a hairy root line is chimeric or heterozygous for a mutation, as long as a small number cells, which have the chance to form a branch, are homozygous/biallelic for the mutation, a homozygous/biallelic hairy root line can be isolated via culturing the branch roots. Since hairy roots are fast-growing, homozygous/biallelic lines can be efficiently isolated from the branches in a short period of time.
Previous studies have indicated that the recovery rate of homozygous/biallelic mutations in MtPDS generated by the CRISPR-Cas9 system is approximate 10%, which is higher than that observed in our hairy root lines in the 1st round of culture (5%) (Meng et al., 2017). The different editing efficiencies could be the result of differences in GC content of the target sequences [55% in Meng et al. (2017)’s study vs 45% in our study] and/or secondary of structure between the target and gRNA (Ren et al., 2014; Liu et al., 2016). Although the homozygous/biallelic mutant recovery rate in our system was lower in the 1st round of culture, when we selected homozygous/biallelic branch roots using a restriction enzyme digestion screening method, 75% of the hairy root lines (6 out of 8 lines) were found homozygous/biallelic in the second generation. Thus, the recovery rate dramatically increased from 5 to 75%, and this process only took 10–15 days. This result suggests that the lateral roots can potentially serve as a library containing abundant types of mutations for rapidly screening homozygous/biallelic mutants. Only the homozygous/biallelic branch roots were regenerated into complete plants in the subsequent culture, which avoided the unnecessary regeneration of plants without the desired target gene mutations.
In our study, we found that Cas9 expression levels have no correlationship with the recovery of homozygous/biallelic mutants. For example, homozygous mutant line 13 did not have higher Cas9 RNA levels than other heterozygous mutant or chimeric lines. Cas9 expression levels in first generation hairy roots of lines 1 and 6 were low, and biallelic mutations were detected in the second generation. In contrast, no homozygous or biallelic mutations were detected in the second generation hairy roots of line 5, in which Cas9 expression levels were relatively high (Figures 3, 5). One explanation for this might be that the RNA level is not the only factor that affects the activity of Cas9. Other possible causes, such as the abundance of Cas9 protein and the cell micro-environment, which were not investigated in the present study, would also influence Cas9 editing efficiency.
Another paradoxical phenomenon in our study is there were no biallelic or homozygous mutations were found in lines 4 and 5, even in its 3rd and 4th generation hairy roots lines (Figure 3B and Supplementary Figure S3). We ascribed this to the hairy root formation model and the sampling probability. The biallelic or homozygous lines could be detected only if two conditions were satisfied. Firstly, because hairy root can transfer only part but not all of its genetic materials to its branch roots, not like the situation in calli transformation method. Then, biallelic or homozygous lines can be identified only if cells carrying the mutation develop into a branch. In addition, the biallelic or homozygous branches were found only if they were selected for subculturing or subsequent analysis. Therefore, the possibility that biallelic or homozygous lines would have been detected by selecting more than 10 hairy roots from lines 4 and 5 cannot be ruled out. Moreover, we randomly sequenced the hairy root lines of line 5 of 3rd and 4th generations, there was still only one mutation type of 1 bp deletion at target site, no more new mutation type was detected (Supplementary Figure S3). This was consistent with the results in Arabidopsis, in which more than half (53%) of the mutations detected in T2 plants were not found at T1, but most T3 plants did not have new mutation (Feng et al., 2014). Likewise, in M. truncatula, it was found that mutations in T1 plant caused by CRISPR/Cas9 were transmitted through the germline rather than created de novo in T1 (Cermak et al., 2017). Therefore, it was suggested that taking more independent hairy roots of the 2nd generation was more effective way to obtain biallelic or homozygous lines than subculturing more generations.
Taken together, we have reported an efficient method for obtaining biallelic or homozygous mutation lines using the fast-growing hairy root system. Because the plants regenerated from M. truncatula hairy roots are fertile, the mutated plants obtained by this strategy could allow assessment of gene function at the whole plant level. This strategy could be applied to other plants whose hairy roots can be regenerated into a normal plant, thus providing a different path for studying gene functions in non-model plants without a mature tissue culture regeneration system.
We replaced the fragment surrounded by BsaI restriction sites and the CCDB gene in pYLCRISPR/Cas9 P35s-B (GenBank accession number: AI133729.1) (Ma et al., 2015) with sequences containing the AtU3b promoter and the sgRNA which were cloned from pYLsgRNA-AtU3b (GenBank accession number: KR029097.1), and there were also two new BsaI restriction sites (Supplementary Figure S1A,B) between the promoter and sgRNA sequences. We named the new plasmid pYLCRISPR/Cas9 P35s-B-AtU3b and its annotated DNA sequences were in Supplementary Table S3. Then we used the simplified two-step method for vector construction with tRNA-gRNA cassettes described by Ma et al. (2016) and Xie et al. (2015) (Supplementary Figure S1C). The specific steps were as follows. Fragments were amplified from pGTR which contained the tRNA-sgRNA sequences and were synthesized by Sangon Biotech (Supplementary Figure S1D) (the primers used were listed in Supplementary Table S2), then the pYLCRISPR/Cas9 35s-B-AtU3b vector and amplified fragments were mixed for BsaI enzyme digestion and T4 ligation (Ma et al., 2016; Xie et al., 2015). The vector containing the target sequence and the tRNA-gRNA expression cassette was transferred into A. rhizogenes strain LBA9402 for subsequent experiments.
Sequences with 5′-N20NGG within either strand of the MtPDS (Medtr3g084830) were selected as candidate targets. According to Ma et al. (2016), sequences that having GC content lower than 40% were removed. And then we eliminate the candidate targets having four or more consecutive T nucleotides to avoid early transcriptional termination of AtU3b. Then, for convenient identification of the homozygous or biallelic mutation line, we detected the restriction enzyme cutting sites of the rest candidate sequences. The target sequence, TGGTCACAAGCCTATATTGC(TGG), having a MwoI cutting sites close to the NGG, were selected as our target sequence.
Healthy leaves and petioles of aseptic seedlings of M. truncatula genotype R108 (Hoffmann et al., 1997) were employed for A. rhizogenes strain LBA9402 infection as describe by Crane et al. (2006). After 2 days of cocultivation in SH medium containing 0.196 mg l–1 acetosyringone (MDBio, Taiwan, China), the infected explants were transferred to selection medium, SH medium supplemented with 10 g l–1 sucrose, 1.5 mg l–1 phosphinothricin (PPT) (PhytoTechnology Laboratories) and 400 mg l–1 timentin (MDBio, Taiwan, China). Hairy roots were subcultured every 10–15 days onto fresh selection medium.
The T0 generation hairy roots were cut from leaves and subcultured on new SH medium. The branches of the T0 generation hairy roots were named 1st generation hairy roots. Similarly, branches of 1st generation hairy roots were named 2nd generation hairy roots, and so on. Hairy roots of different generations were collected. The bar, Cas9, and rolB genes were amplified using T5 direct PCR kit (plant) (Tsingke biotech, Beijing, China) with the primers listed in Supplementary Table S2. PCR was performed in a thermal cycler with the following program: 95°C for 5 min, 30 cycles of 95°C for 30 s, 55 for 30 s, and 72°C for 1.5 min, and 72°C for 10 min.
Polymerase chain reaction amplification of a 1,347 bp region surrounding the target sequence was performed on selected co-transformed DNA samples using the primers listed in Supplementary Table S2. PCR was performed using T5 direct PCR kit (plant) (Tsingke biotech, Beijing, China) in a thermal cycler with the following program: 95°C for 5 min, 30 cycles of 95°C for 30 s, 55°C for 30 s, 72°C for 1.5 min, and 72°C for 10 min. The PCR products were then run on a 1% agarose gel, and the target bands were extracted using the Omega Gel Extraction Kit (Omega Bio-tek, GA, United States). Target gel extraction products (∼1,000 ng) were digested with 5 u MwoI restriction enzyme at 60°C for 40 min. Digested DNA was then run on a 1% agarose gel in 1 × TBE for 20 min at 180 V, then imaged on a UV gel imager (Bio-Rad Laboratories, Shanghai, China). Gel bands that appeared to be undigested were then extracted using the Omega Gel Extraction Kit (Omega Bio-tek, GA, United States). And cloned into the pMD19-T easy vector (TaKaRa, Toyoto, Japan). Five to twenty clones for each line were sequenced.
The homozygous and biallelic hairy root lines were cut into 1–2 cm lengths and transferred onto SH3a medium [N6 major salts, SH minor salts and SH vitamins, supplemented with 4.0 mg l–1 2,4-D, 0.5 mg l–1 BAP, 3% (w/v) sucrose, 200 mg l–1 timentin (MDBio, Taiwan, China), and solidified with 0.75% (w/v) agar (Sangon Biotech, Shanghai, China)]. Induced calli were transferred onto MSBK medium [MS basal medium supplemented with 0.5 mg l–1 BAP, 1 mg l–1 kinetin, 3% (w/v) sucrose, 200 mg l–1 timentin (MDBio, Taiwan, China) and solidified with 0.75% (w/v) agar] for bud differentiation as described by Jiang et al. (2019).
Total RNA was extracted from individual hairy roots of M. truncatula using Invitrogen Trizol Reagent (Invitrogen, CA, United States) according to manufacturer’s instructions. qRT-PCR was conducted on LightCycler 480 Detection System (Roche, Penzberg, Germany) using SYBR Premix Ex Taq (TaKaRa, Toyoto, Japan) as described by Hu et al. (2010). The primer sets used are listed in Supplementary Table S2. An Actin gene (Medtr3g095530.2) was used as an internal control to calibrate variation in cDNA concentration (Niu et al., 2015).
Samples collected from three technique replicates of each TL. The mean values were used for statistical analysis. Data from each trait were subjected to one-way analysis of variance (ANOVA). Standard derivations (SD) were provided in all figures and tables as appropriate.
All datasets generated for this study are included in the article/Supplementary Material.
HLZ and YC contributed equally to this work. HLZ, YC, CZ, DS, and CF designed the research. HLZ, YC, HZ, YX, WL, RZ, CS, and JL performed the experiments. ZS, SG, and ZH analyzed the data. YC and CF wrote the manuscript.
The work was supported by the Major Program of Shandong Province Natural Science Foundation (No. ZR2018ZB0213), Shandong Technology Innovation Center of Synthetic Biology (sdsynbio-2018-PY-07), the Ministry of Science and Technology of China (No. 2015CB943500), the National Natural Science Foundation of China (No. 31802124), and the assisted project by Heilong Jiang Postdoctoral Funds for scientific research initiation.
The authors declare that the research was conducted in the absence of any commercial or financial relationships that could be construed as a potential conflict of interest.
We thank Prof. Yaoguang Liu for providing the pYLCRISPR/Cas9 35S-B and pYLsgRNA-AtU3b plasmids.
The Supplementary Material for this article can be found online at: https://www.frontiersin.org/articles/10.3389/fpls.2020.00294/full#supplementary-material
Cermak, T., Curtin, S. J., Gil-Humanes, J., Cegan, R., Kono, T. J. Y., Konecna, E., et al. (2017). A multipurpose toolkit to enable advanced genome engineering in plants. Plant Cell 29, 1196–1217. doi: 10.1105/tpc.16.00922
Choi, P. S., Kim, Y. D., Choi, K. M., Chung, H. J., Choi, D. W., and Liu, J. R. (2004). Plant regeneration from hairy-root cultures transformed by infection with Agrobacterium rhizogenes in Catharanthus roseus. Plant Cell Rep. 22, 828–831. doi: 10.1007/s00299-004-0765-763
Crane, C., Wright, E., Dixon, R. A., and Wang, Z. Y. (2006). Transgenic Medicago truncatula plants obtained from Agrobacterium tumefaciens-transformed roots and Agrobacterium rhizogenes-transformed hairy roots. Planta 223, 1344–1354. doi: 10.1007/s00425-006-0268-262
Deaner, M., Holzman, A., and Alper, H. S. (2018). Modular ligation extension of guide RNA operons (LEGO) for multiplexed dCas9 regulation of metabolic pathways in Saccharomyces cerevisiae. Biotechnol. J. 13:e1700582. doi: 10.1002/biot.201700582
Dhanushkodi, R., Matthew, C., McManus, M. T., and Dijkwel, P. P. (2018). Drought-induced senescence of Medicago truncatula nodules involves serpin and ferritin to control proteolytic activity and iron levels. New Phytol. 220, 196–208. doi: 10.1111/nph.15298
Dong, F., Xie, K., Chen, Y., Yang, Y., and Mao, Y. (2017). Polycistronic tRNA and CRISPR guide-RNA enables highly efficient multiplexed genome engineering in human cells. Biochem. Biophys. Res. Commun. 482, 889–895. doi: 10.1016/j.bbrc.2016.11.129
Feng, Z., Mao, Y., Xu, N., Zhang, B., Wei, P., Yang, D. L., et al. (2014). Multigeneration analysis reveals the inheritance, specificity, and patterns of CRISPR/Cas-induced gene modifications in Arabidopsis. Proc. Natl. Acad. Sci. U.S.A. 111, 4632–4637. doi: 10.1073/pnas.1400822111
Gibbs, D. J., and Coates, J. C. (2014). AtMYB93 is an endodermis-specific transcriptional regulator of lateral root development in Arabidopsis. Plant Signal. Behav. 9:e970406. doi: 10.4161/15592316.2014.970406
Habibi, P., de Sa, M. F., da Silva, A. L., Makhzoum, A., da Luz Costa, J., Borghetti, I. A., et al. (2016). Efficient genetic transformation and regeneration system from hairy root of Origanum vulgare. Physiol. Mol. Biol. Plants 22, 271–277. doi: 10.1007/s12298-016-0354-352
Hashimoto, R., Ueta, R., Abe, C., Osakabe, Y., and Osakabe, K. (2018). Efficient multiplex genome editing induces precise, and self-ligated type mutations in tomato plants. Front. Plant Sci. 9:916. doi: 10.3389/fpls.2018.00916
Hoffmann, B., Trinh, T. H., Leung, J., Kondorosi, A., and Kondorosi, E. (1997). A new Medicago truncatula line with superior in vitro regeneration, transformation, and symbiotic properties isolated through cell culture selection. Mol. Plant. Microbe. Int. 10, 307–315. doi: 10.1094/Mpmi.1997.10.3.307
Horvath, B., Domonkos, A., Kereszt, A., Szucs, A., Abraham, E., Ayaydin, F., et al. (2015). Loss of the nodule-specific cysteine rich peptide, NCR169, abolishes symbiotic nitrogen fixation in the Medicago truncatula dnf7 mutant. Proc. Natl. Acad. Sci. U.S.A. 112, 15232–15237. doi: 10.1073/pnas.1500777112
Hou, L., Shi, H., Yu, W., Tsang, P. K., and Chow, C. F. (2014). Induction of polyploid in hairy roots of Nicotiana tabacum and its plant regeneration. Chin. J. Biotechnol. 30, 581–594.
Hu, R. B., Qi, G., Kong, Y. Z., Kong, D. J., Gao, Q., and Zhou, G. K. (2010). Comprehensive analysis of NAC domain transcription factor gene family in Populus trichocarpa. BMC Plant Biol. 10:145. doi: 10.1186/1471-2229-10-145
Huang, P., Xia, L., Liu, W., Jiang, R., Liu, X., Tang, Q., et al. (2018). Hairy root induction and benzylisoquinoline alkaloid production in Macleaya cordata. Sci. Rep. 8:11986. doi: 10.1038/s41598-018-30560-30560
Hudzieczek, V., Cegan, R., Cermak, T., Bacovska, N., Machalkova, Z., Dolezal, K., et al. (2018). Agrobacterium rhizogenes-mediated transformation of a dioecious plant model Silene latifolia. New Biotechnol. 48, 20–28. doi: 10.1016/j.nbt.2018.04.001
Jia, H., Zhao, B., Wang, X., and Wang, Y. (2008). Agrobacterium rhizogenes-mediated transformation and regeneration of the Apocynum venetum. Chin. J. Biotechnol. 24, 1723–1728. doi: 10.1016/s1872-2075(08)60071-0
Jiang, Q., Fu, C., and Wang, Z. Y. (2019). A unified agrobacterium-mediated transformation protocol for alfalfa (Medicago sativa L.) and Medicago truncatula. Methods Mol. Biol. 1864, 153–163. doi: 10.1007/978-1-4939-8778-8_11
Kirchner, T. W., Niehaus, M., Debener, T., Schenk, M. K., and Herde, M. (2017). Efficient generation of mutations mediated by CRISPR/Cas9 in the hairy root transformation system of Brassica carinata. PloS One 12:e0185429. doi: 10.1371/journal.pone.0185429
Li, B., Cui, G., Shen, G., Zhan, Z., Huang, L., Chen, J., et al. (2017). Targeted mutagenesis in the medicinal plant Salvia miltiorrhiza. Sci. Rep. 7:43320.
Liu, X. X., Homma, A., Sayadi, J., Yang, S., Ohashi, J., and Takumi, T. (2016). Sequence features associated with the cleavage efficiency of CRISPR/Cas9 system. Sci. Rep. 6:19675. doi: 10.1038/srep19675
Ma, X., Zhang, Q., Zhu, Q., Liu, W., Chen, Y., Qiu, R., et al. (2015). A robust CRISPR/Cas9 system for convenient, high-efficiency multiplex genome editing in monocot and dicot plants. Mol. Plant 8, 1274–1284. doi: 10.1016/j.molp.2015.04.007
Ma, X., Zhu, Q., Chen, Y., and Liu, Y. G. (2016). CRISPR/Cas9 platforms for genome editing in plants: developments and applications. Mol. Plant 9, 961–974. doi: 10.1016/j.molp.2016.04.009
Mehrotra, S., Goel, M. K., Srivastava, V., and Rahman, L. U. (2015). Hairy root biotechnology of Rauwolfia serpentina: a potent approach for the production of pharmaceutically important terpenoid indole alkaloids. Biotechnol. Lett. 37, 253–263. doi: 10.1007/s10529-014-1695-y
Meng, Y. Y., Hou, Y. L., Wang, H., Ji, R. H., Liu, B., Wen, J. Q., et al. (2017). Targeted mutagenesis by CRISPR/Cas9 system in the model legume Medicago truncatula. Plant Cell Rep. 36, 371–374. doi: 10.1007/s00299-016-2069-9
Michno, J. M., Wang, X. B., Liu, J. Q., Curtin, S. J., Kono, T. J. Y., and Stupar, R. M. (2015). CRISPR/Cas mutagenesis of soybean and Medicago truncatula using a new web-tool and a modified Cas9 enzyme. GM Crops Food 6, 243–252. doi: 10.1080/21645698.2015.1106063
Mikami, M., Toki, S., and Endo, M. (2015). Parameters affecting frequency of CRISPR/Cas9 mediated targeted mutagenesis in rice. Plant Cell Rep. 34, 1807–1815. doi: 10.1007/s00299-015-1826-1825
Nakayasu, M., Akiyama, R., Lee, H. J., Osakabe, K., Osakabe, Y., Watanabe, B., et al. (2018). Generation of alpha-solanine-free hairy roots of potato by CRISPR/Cas9 mediated genome editing of the St16DOX gene. Plant Physiol. Biochem. 131, 70–77. doi: 10.1016/j.plaphy.2018.04.026
Nishitani, C., Hirai, N., Komori, S., Wada, M., Okada, K., Osakabe, K., et al. (2016). Efficient genome editing in apple using a CRISPR/Cas9 system. Sci. Rep. 6:31481. doi: 10.1038/srep31481
Niu, L. F., Lin, H., Zhang, F., Watira, T. W., Li, G. F., Tang, Y. H., et al. (2015). LOOSE FLOWER, a WUSCHEL-like Homeobox gene, is required for lateral fusion of floral organs in Medicago truncatula. Plant J. 81, 480–492. doi: 10.1111/tpj.12743
Pan, C. T., Ye, L., Qin, L., Liu, X., He, Y. J., Wang, J., et al. (2016). CRISPR/Cas9-mediated efficient and heritable targeted mutagenesis in tomato plants in the first and later generations. Sci. Rep. 6:24765. doi: 10.1038/srep24765
Qi, W. W., Zhu, T., Tian, Z. R., Li, C. B., Zhang, W., and Song, R. T. (2016). High-efficiency CRISPR/Cas9 multiplex gene editing using the glycine tRNA-processing system-based strategy in maize. BMC Biotechnol. 16:58. doi: 10.1186/s12896-016-0289-282
Ren, X., Yang, Z., Xu, J., Sun, J., Mao, D., Hu, Y., et al. (2014). Enhanced specificity and efficiency of the CRISPR/Cas9 system with optimized sgRNA parameters in Drosophila. Cell Rep. 9, 1151–1162. doi: 10.1016/j.celrep.2014.09.044
Shah, T., Andleeb, T., Lateef, S., and Noor, M. A. (2018). Genome editing in plants: advancing crop transformation and overview of tools. Plant Physiol. Biochem. 131, 12–21. doi: 10.1016/j.plaphy.2018.05.009
Sharafi, A., Sohi, H. H., Azadi, P., and Sharafi, A. A. (2014). Hairy root induction and plant regeneration of medicinal plant Dracocephalum kotschyi. Physiol. Mol. Biol. Plants 20, 257–262. doi: 10.1007/s12298-013-0217-z
Shi, H., Yu, W., Zhang, G., Tsang, P. E., and Chow, C. F. (2014). Induction of polyploid hairy roots and its plant regeneration in Pogostemon cablin. Chin. J,. Biotechnol. 30, 1235–1246.
Stritzler, M., Elba, P., Berini, C., Gomez, C., Ayub, N., and Soto, G. (2018). High-quality forage production under salinity by using a salt-tolerant AtNXH1-expressing transgenic alfalfa combined with a natural stress-resistant nitrogen-fixing bacterium. J. Biotechnol. 27, 42–45. doi: 10.1016/j.jbiotec.2018.04.013
Subotic, A., Jevremovic, S., Grubisic, D., and Jankovic, T. (2009). Spontaneous plant regeneration and production of secondary metabolites from hairy root cultures of Centaurium erythraea Rafn. Methods Mol. Biol. 547, 205–215. doi: 10.1007/978-1-60327-287-2_17
Sun, J., Xu, J. S., Zhao, L. Z., Wei, J. H., Yang, H. Y., and Sui, C. (2013). Induction of hairy roots and plantlet regeneration of Bupleurum chinense DC. Acta Pharm. Sin. 48, 1491–1497.
Wang, P. C., Zhang, J., Sun, L., Ma, Y. Z., Xu, J., Liang, S. J., et al. (2018). High efficient multisites genome editing in allotetraploid cotton (Gossypium hirsutum) using CRISPR/Cas9 system. Plant Biotechnol. J. 16, 137–150. doi: 10.1111/pbi.12755
Wang, Z., Wang, S., Li, D., Zhang, Q., Li, L., Zhong, C., et al. (2018). Optimized paired-sgRNA/Cas9 cloning and expression cassette triggers high-efficiency multiplex genome editing in kiwifruit. Plant Biotechnol. J. 16, 1424–1433. doi: 10.1111/pbi.12884
Xie, K. B., Minkenberg, B., and Yang, Y. N. (2015). Boosting CRISPR/Cas9 multiplex editing capability with the endogenous tRNA-processing system. Proc. Natl. Acad. Sci. U.S.A. 112, 3570–3575. doi: 10.1073/pnas.1420294112
Keywords: CRISPR/Cas9, genome editing, hairy root, homozygous/biallelic mutant, Medicago truncatula
Citation: Zhang H, Cao Y, Zhang H, Xu Y, Zhou C, Liu W, Zhu R, Shang C, Li J, Shen Z, Guo S, Hu Z, Fu C and Sun D (2020) Efficient Generation of CRISPR/Cas9-Mediated Homozygous/Biallelic Medicago truncatula Mutants Using a Hairy Root System. Front. Plant Sci. 11:294. doi: 10.3389/fpls.2020.00294
Received: 27 May 2019; Accepted: 27 February 2020;
Published: 24 March 2020.
Edited by:
Paulo Arruda, University of Campinas, BrazilReviewed by:
Colby Starker, University of Minnesota, Twin Cities, United StatesCopyright © 2020 Zhang, Cao, Zhang, Xu, Zhou, Liu, Zhu, Shang, Li, Shen, Guo, Hu, Fu and Sun. This is an open-access article distributed under the terms of the Creative Commons Attribution License (CC BY). The use, distribution or reproduction in other forums is permitted, provided the original author(s) and the copyright owner(s) are credited and that the original publication in this journal is cited, in accordance with accepted academic practice. No use, distribution or reproduction is permitted which does not comply with these terms.
*Correspondence: Chunxiang Fu, ZnVjeEBxaWJlYnQuYWMuY24=; Dequan Sun, c3VuZGVxdWFuMDQ1MUAxNjMuY29t
†These authors have contributed equally to this work
Disclaimer: All claims expressed in this article are solely those of the authors and do not necessarily represent those of their affiliated organizations, or those of the publisher, the editors and the reviewers. Any product that may be evaluated in this article or claim that may be made by its manufacturer is not guaranteed or endorsed by the publisher.
Research integrity at Frontiers
Learn more about the work of our research integrity team to safeguard the quality of each article we publish.