- Xinjiang Key Laboratory of Biological Resources and Genetic Engineering, College of Life Science and Technology, Xinjiang University, Urumqi, China
Variations of photosynthetic structures in different tissues or cells are in coordination with changes in various aspects, e.g. physiology, biochemistry, gene expression, etc. Most C4 plant species undergo developmental enhancement of the photosynthetic system, which may present different modes of changes between anatomy and physiology/biochemistry. In the current study, we investigated a Kranz-type C4 species Salsola ferganica with the progressive development of photosynthetic (PS) structure, performance of PS physiology, induction of PS enzymes, and transcriptional and translational regulation of PS genes, results revealed that S. ferganica presented C3 type anatomy in cotyledons but C4 type in leaves (C3/L4), with the C4 system separation of initial carbon fixation in the palisade mesophyll (M) cells and the following incorporation into triosephosphates and sugars in the bundle sheath (BS) cells, respectively. The BS cells continuously surrounded the vascular bundles and water storage cells in leaf anatomic structure. Compared to the single-cell C4 species Suaeda aralocaspica, S. ferganica exhibited similar developmental enhancement of C4 syndrome temporally and spatially in anatomic structures, enzyme activities, and gene expression, which suggests that completion of differentiation of the photosynthetic system is necessary for a C4 assimilation pathway. Besides, S. ferganica also displayed some different characteristics compared to S. aralocaspica in photosynthetic physiology, e.g. a more flexible δ13C value, much lower phosphoenolpyruvate carboxylase (PEPC) activity, and an insensitive response to stimuli, etc., which were not typical C4 characteristics. We speculate that this may suggest a different status of these two species in the evolutionary process of the photosynthesis pathway. Our findings will contribute to further understanding of the diversity of photosynthesis systems in Kranz-type C4 species and the Salsola genus.
Introduction
Photosynthetic carbon assimilation pathways at least include C3, C4, CAM (Crassulacean acid metabolism), and/or C2 (C3-C4 intermediate) types (Raghavendra and Das, 1993; Khoshravesh et al., 2016), each is associated with distinct features of leaf anatomy, physiology, biochemistry, etc. (Sage and Stata, 2015). Compared to the C3 type, C4 photosynthesis is advantageous when limited carbon acquisition occurs under high temperature, drought, or saline conditions (Sage, 2004). According to documentation, Amaranthaceae (Chenopodiaceae has been classified into this family currently) possesses the most diverse photosynthetic types and the largest number of C4 species in dicotyledonous plants (Pyankov et al., 2000; Pyankov et al., 2001; Kadereit et al., 2003). As one of the largest genera in the family, Salsola has the most abundant photosynthetic types recorded so far (Pyankov et al., 2010; Wen and Zhang, 2011), in which many species are widely distributed and can adapt to extreme desert conditions (Wei et al., 2008; Ma et al., 2016). The classical C4 photosynthetic pathway differentiates a specialized leaf anatomy (Kranz structure), which consists of two distinct photosynthetic cell types (mesophyll [M] cells and bundle sheath [BS] cells) (Hatch, 1987); whereas single-cell (SC) C4 plants can accomplish C4 and C3 cycles within the same chlorenchyma cell by biochemical compartmentation of the related enzymes and separation of dimorphic chloroplasts in distinct positions (Erlinghaeuser et al., 2016). So far, four SC-C4 terrestrial species have been recorded, i.e. Bienertia sinuspersici, Bienertia Cycloptera, Bienertia kavirense, and Suaeda aralocaspica, all belonging to the Amaranthaceae (Chenopodiaceae before), which have different spatial distributions of organelles and photosynthetic enzymes in a single chlorenchyma cell (Lung et al., 2012). Usually, behaviors (distribution, activity, etc.) of photosynthetic enzymes in single cells will be correspondingly changed with the structural adjustment (Voznesenskaya et al., 2001a). These variations of photosynthetic anatomy and enzyme function may promote a deeper understanding of carbon assimilation pathway in plants.
Studies have shown that similar anatomy may express different physiological and biochemical characteristics, e.g. plants with C4 structure may act with C3-like behavior (Edwards et al., 1990; Wen and Zhang, 2015): it has been suggested that these species might be in the process of evolution from a C3 to C4 photosynthetic pathway on both anatomical and biochemical levels (Ku et al., 1983). Four C3-C4 intermediate species, Flaveria anomala, Flaveria liaris, Flaveria pubescens, and Flaveria ramosissima in the Flaveria genus have Kranz-like leaf anatomy, whereas the activities of C4 enzyme phosphoenolpyruvate carboxylase (PEPC), pyruvate orthophosphate dikinase (PPDK), etc. and some photosynthetic physiological characteristics are similar to C3 species (Ku et al., 1983). In the Salsola genus, Salsola arbusculiformis has the intermediate anatomic features of two-to-three layers of M cells and Kranz-like BS cells, while the photosynthetic enzyme activity, immunolocalization, and 14CO2 labeling of initial fixation products are presented as a C4 type (Voznesenskaya et al., 2001b); a C4 anatomic structure in Salsola laricifolia, however, appears with C3 or C3-C4 physiological characteristics (Wen and Zhang, 2015). The inconsistency between photosynthetic biochemical characteristics and anatomic structures in Salsola species cannot include all the cases (Voznesenskaya et al., 2013). All available evidence suggests that the evolutionary process from C3 ancestors to C4 plants occurred many times independently in taxonomically diverse groups (Ku et al., 1983; Soros and Dengler, 2001; Voznesenskaya et al., 2013), which may result in various intermediates matched or not matched in between anatomy and physiological or biochemical characteristics.
All forms of C4 species studied so far have been observed with the gradient differentiation of the photosynthetic structure and biochemistry, which finally results in establishment of a fully functional C4 syndrome (Koteyeva et al., 2014; Koteyeva et al., 2016). In the Kranz-type C4 species representatives, maize and Arundinella hirta in the Poaceae, the latter has not only M and BS cells (normal Kranz cells) but also another type of Kranz cells (named as distinctive [D] cells), which are not associated with vascular bundles (VB); however, all cell types show progressive differentiation and enzyme induction pattern, i.e. PEPC or ribulose-1,5-bisphosphate carboxylase (RUBPC) accumulates in M, BS, or D cells along the base-to-tip maturation gradient in developing leaves (Langdale et al., 1988; Wakayama et al., 2003). Suaeda taxifolia and Suaeda eltonica (Chenopodiaceae), with M and BS cells distributed around the leaf periphery and surrounding VB in the central plane, respectively, both present a longitudinal gradient development of photosynthetic structure and biochemistry in young leaves. In coordination with formation of Kranz anatomy, the chloroplast dimorphism and mitochondrial differentiation are established, and the expression of PPDK, NAD-malic enzyme (NAD-ME), RUBPC, or PEPC is significantly increased in parallel (Koteyeva et al., 2011a). Different types of anatomy between Cleome angustifolia and Cleome gynandra (with single and multiple Kranz units, respectively) suggest the independent evolutionary origins of C4 photosynthesis, but they present similar developmental enhancement of C4 system (Koteyeva et al., 2014). In SC-C4 species S. aralocaspica and B. sinuspersici, a gradual enhancement of development along a longitudinal gradient of the young leaf is accompanied by increasing activity of photosynthetic enzymes (e.g. PEPC and RUBPC) (Voznesenskaya et al., 2003; Lara et al., 2008; Koteyeva et al., 2016). Taken together, the progressive development of C4 anatomy and related biochemistry should be an adaptive strategy for C4 species formed in the long-term evolutionary process.
In the Salsola genus, there are a total of nine anatomic types among the C4 plant species (Wen and Zhang, 2011), which makes this genus a good candidate for the study of photosynthetic related anatomy, physiology, and biochemistry (Voznesenskaya et al., 2013). Salsola ferganica is an annual herbaceous halophyte of Salsola and distributed in desert areas of high temperature, drought, and salinization (Zhao et al., 2002; Ma et al., 2018). So far, reports on photosynthetic structures in S. ferganica were limited (Wen et al., 2010), and the relevant physiology, biochemistry, or gene expression on photosynthesis has not been documented. Our previous work on S. ferganica revealed that the photosynthetic structure and δ13C value significantly varied with developmental progression and environmental changes. Based on our observations and updates on the Salsola genus, and by employing SC-C4 plant species S. aralocaspica as the control, in the present study, we address the following questions: (1) Does the photosynthetic anatomy in S. ferganica become optimized with plant development progression and if so how does it change? (2) How do the physiology and biochemistry processes progress in coordination with photosynthetic structure differentiation? (3) What photosynthetic enzymes and genes act in the process? By addressing these questions, our findings expand our knowledge of the phenomenon of developmental enhancement of the C4 system in S. ferganica and increase our understanding of the physiology and biochemistry of carbon fixation in photosynthetic pathways.
Materials and Methods
Plant Cultivation and Treatments
S. ferganica and S. aralocaspica seeds were sown in pots containing perlite: vermiculite (1:3, v/v) in a greenhouse. After emergence, seedlings were cultivated at 18–37°C (Outdoor: 15–36°C), 5–30% relative humidity, 50–300 (Outdoor: 400–2,000) μmol m-2 s-1 light source, and a 14–16 h light/8–10 h dark photoperiod. Half-strength Hoagland solution (Hoagland and Arnon, 1950) was applied at 2-week intervals. Chenopodium glaucum (Chenopodiaceae) and Nicotiana tabacum were grown under the same cultivation system for assay of PEPC and RUBPC activity.
For paraffin section preparation (for cotyledon or leaf anatomic structure, starch staining, immuno-histochemical localization of photosynthetic enzyme assays), samples were collected as described in Supplementary Figure 1. The image was taken from an indoor plant (the morphology was different from that arising under natural field conditions as shown in Supplementary Figure 2). Three types of leaves from different parts of the plant were collected according to the following criteria: 0.5–0.6 cm leaf on the top part, 1.3–1.5 cm (S. ferganica) or 1.8–1.9 cm (S. aralocaspica) on the upper part, 1.0 cm (S. ferganica) or 2.0 cm (S. aralocaspica) on the lower part, respectively; each of the different sizes of leaves were carefully divided into tip, middle, and bottom segments, which were then fixed in formalin acetic alcohol (FAA) fixation solution. For qRT-PCR or western blotting analysis of photosynthetic gene or enzyme expression, different leaf segments were immediately frozen in liquid nitrogen for RNA or protein extraction.
For NaCl treatment, different concentrations of NaCl solution (100, 300, 500 mmol L-1) prepared with half-strength Hoagland solution were applied to pots from seed sowing time at an interval of 5 days till the experiment was finished: each time the soil matrix was thoroughly saturated with appropriate NaCl solutions. Half strength Hoagland solution was used as the control medium. Four samples of each treatment at 7th d, 15th d, and 30th d after seedling emergence were collected, and ground on ice upon being frozen in liquid nitrogen for immediate assay or stored at −80°C for a short time.
Observation of Micro-Structure of Leaf, Starch Staining or Immuno-Histochemical Localization
The first completely developed leaf from the top of plant was sampled and three leaves for each species were collected, each leaf was cut into three fragments (tip, middle, bottom) (Koteyeva et al., 2016). FAA solution (90 ml 50% ethanol: 5 ml formaldehyde: 5 ml glacial acetic acid) was used to fix plant tissues. In order to immediately and thoroughly fix the materials, the sealed small glass bottle containing FAA solution and tissues was placed under vacuum repeatedly and gently with a syringe to evacuate the air until the tissues fell down to the bottom, the bottles were then placed at 4°C for 24 h. Through a series of ethanol dehydration, xylene clearing, paraffin inclusion, and embedding, the paraffin blocks containing tissues were sliced into 6–12 μm sections with a microtome (Leica RM2126), and were then expanded and deparaffinized. For micro-structure observation, sections were counterstained with 1% safranine and 1% fast green; for starch staining, after 1 h staining with 1% safranine, slides were immersed into 1% I2-KI stock solution (8 g KI + 1 g I2 dissolve in 100 ml distilled water) for 20–30 min, then quickly and gently washed with trichloroethane followed by rinsing in distilled water for 30 s. For immuno-histochemical localization, sections were spread on siliconized slides, and treated with 3% hydrogen peroxide methanol solution for 10 min to remove endogenous peroxidase and then rinsed with PBST (phosphate buffered saline [0.01 M, pH 7.2–7.4] [Cat. No. P1010, Solarbio, China] containing 0.05% Tween-20) for 5 min (three times). Following microwave heating repair at 95°C in citrate buffer for 11 min, the slides were rinsed with PBST as described above. Then slides were blocked with 1% bovine serum album (BSA) for 30–60 min, incubated with 1:10 diluted (with 1% BSA) primary antibodies of RUBPC and PEPC, which were made by Abmart (Abmart Shanghai Co., Ltd., China). The epitopic amino acid sequences for polyclonal primary antibody of PEPC and RUBPC were CEKLSSIDAQLR (N-terminal phosphorylation site of typical plant type of PEPC) and QARNEGRDLAREGN (conserved amino acids of plant RUBPC large subunit), respectively. After incubation at 37°C for 1 h, slides were rinsed with PBST buffer for 6 times/15 min each, then incubated with horseradish peroxidase (HRP) labeled secondary antibody (1:500 diluted with 1% BSA) at 37°C for 1 h. The treated slides were stained with diaminobenzidine (DAB) (Sangon, Shanghai), and rinsed with PBST for 5 times/15 min each (Huang et al., 2015). For all above, sections were then treated in absolute ethanol for 5 min (two changes) and then in xylene for 5 min (two changes) for dehydration. After mounting, slides were sealed with neutral balsam and incubated at 37°C for drying and finally inspected. The images were acquired by an inverted fluorescence microscope (Nikon ECLIPSE Ti-E, Japan), and photographs were taken by using Nis-Elemens software (Japan).
Measurement of MC: BSC or Cd: Cp Area Ratio and Chloroplast Size
Leaves from outdoor plants (4–8 weeks old) were used for measurement. The paraffin sections were prepared before inspection under the microscope (as described above). For area of mesophyll cells (MC) and bundle sheath cells (BSC) in S. ferganica, or Cd and Cp (referring to the distal end and proximal end areas of a single chlorenchyma cell, respectively; these were obtained from the division at the apparently chloroplast-free area) in S. aralocaspica, the tip segment of mature leaf was employed, 5 leaves with 5 views of each, and 6 MCs and BSCs (S. ferganica) or chlorenchyma cell (S. aralocaspica) of each view were measured; for the chloroplast size, 3 leaves with 3 cells of each, and 5 chloroplasts from each cell were measured. Two measurements were conducted under 100×oil immersion lens, and Image J 1.48U software (National Institutes of Health, USA) was used in calculation of cell area and chloroplast size; Microsoft Excel software was used in the data analysis.
Determination of δ Value of 13C Carbon Isotope
Leaves collected from S. ferganica and S. aralocaspica (cultivated in greenhouse or outdoors) were immediately placed at 150°C for 30 min to deactivate enzymes, then transferred to an aerated oven at 70°C overnight, finally the tissue was ground into an homogeneous powder and sent for δ13C value determination (Beijing Ailemengtuo Science and Technology Co. Ltd., Beijing). δ13C value was calculated by reference to the international standard PDB [Pee Dee Belemnite (Bender et al., 1973)].
Assay of PEPC and RUBPC Activity
Enzyme-catalyzed reactions were employed in determination of activity of PEPC and RUBPC. For PEPC, young fresh leaves (0.1 g) from the upper part of plant were homogenized on ice in extraction buffer (1.0 ml) containing 100 mM Tris-H2SO4 (pH 8.2), 7 mM β-mercaptoethanol, 1 mM EDTA, and 5% glycerol. The homogenate was centrifuged at 1,800 g for 15–20 min at 4°C and the supernatant was immediately used. The following steps were performed according to protocols described by Cao’s reaction mixture (1.0 ml) consisted of 70 mM MgSO4·7H2O (143 μl), 70 mM NaHCO3 (143 μl), 14 mM phosphoenolpyruvic acid (286 μl), 5 mM NADH (429 μl) with only modification by addition of 10 U malate dehydrogenase and 20 μl crude enzyme to initiate reactions (Cao et al., 2015). For RUBPC assays, young fresh leaves (0.1 g) from the upper part of the plant were ground on ice in extraction buffer (1.5 ml) containing 40 mM Tris-HCl (pH 7.6), 10 mM MgCl2·6H2O, 0.25 mM EDTA, and 5 mM glutathione, followed by centrifugation as mentioned above, the supernatant was then immediately used for assay. The reaction mixture (1.0 ml) consisted of 0.2 mM NaHCO3 (67 μl), reaction buffer (467 μl) (100 mM Tris-HCl [pH 7.8], 12 mM MgCl2·6H2O, 0.4 mM EDTA), crude enzyme extract (133 μl), 5 mM NADH (67 μl), 50 mM ATP (67 μl), 50 mM DL-dithiothreitol (67 μl), distilled water (33 μl), 160 U·ml-1 phosphoglycerate kinase (33 μl), 160 U·ml-1 phosphoglyceraldehyde dehydrogenase (33 μl), and 25 mM RuBP (33 μl) (Cao et al., 2015). The absorbance of both reaction mixtures was recorded on a UV-3010 spectrophotometer (Shimadzu, Japan) for 3 min at 340 nm. Enzyme activity of PEPC and RUBPC was defined as 0.01 optical density value decrease per minute equaling 1 U (NC-IUB, 1979). Total protein content was determined by measuring the absorbance at 595 nm. For diurnal changes of enzyme activity, the light intensity, temperature, and relative humidity indoor or outdoor were measured simultaneously at 2 h intervals (Table 1).
Quantitative RT-PCR (qPCR)
Total RNA was isolated from S. ferganica or S. aralocaspica seedlings using a Plant RNA Kit (R6827-02, Omega, US). Reverse transcription was performed with 1 µg of total RNA in 20 µl by using M-MLV (TaKaRa, Dalian, China) according to the manufacturer’s instruction. qPCR primers are shown in Table 2. For primer design, the coding regions of PEPC1, PEPC2, PPDK, RBCL (large subunit of RUBPC) from different species reported in Chenopodiaceae were acquired, and the degenerate primers were designed to get short DNA sequences of each gene from S. ferganica or S. aralocaspica, respectively; then the accurate qPCR primers were designed based on the acquired short sequences of each gene. For PEPC1 and PEPC2 primers, it was necessary to first find out the most conserved region of PEPC1 or PEPC2 among different species reported in Chenopodiaceae, then to select the most different sequences between PEPC1 and PEPC2 from these conserved regions, which could only amplify one of these two genes in S. ferganica or S. aralocaspica. β-ACTIN from S. ferganica or β-TUBULIN from S. aralocaspica was used for an internal reference gene. The above amplification was performed at the following conditions: 95°C 2 min followed by 40 cycles of 95°C 5 s, 60°C 30 s. qPCR was performed with SuperReal PreMix Plus (SYBR Green) Kit (Cat. FP205-02; Tiangen, China) and ABI 7500 Real time PCR system (Applied Biosystem, USA). Relative quantification of specific mRNA level was calculated using the cycle threshold (Ct) 2−ΔΔCt method (Shi and Chiang, 2005), where ΔΔCT = ΔCT target sample - ΔCT control sample, ΔCT target sample= CT test gene - CT reference gene. Three samples (biological replicates) of each treatment were duplicated (technical replicates) in each qPCR experiment. The final value of relative quantification was described as fold change of gene expression in target sample compared to the control of the bottom segment of leaf in each test gene.
Western Blotting Analysis of Photosynthetic Enzymes
Leaf samples (approximately 0.2 g) of different developmental stages, i.e. young leaf (YL, 0.2–0.3 cm), intermediate leaf (IL, 0.5–0.6 cm), mature leaf (1.2–1.5 cm), from different positions (plant upper leaf [PUL], plant middle leaf [PML], plant lower leaf [PLL]), and leaf segments (approximately 0.1 g; intermediate leaf divided into three parts towards the bottom, middle, and tip), were used for extraction of the soluble protein according to the method described by Koteyeva et al. (2011b). The supernatant (protein solution) was mixed with loading buffer [250 mM Tris-HCl, pH 6.8, 10% (w/v) SDS, 50% (v/v) glycerol, 5% (v/v) β -mercaptoethanol, and 0.5% (w/v) bromphenol blue] in 4:1 (v:v) and boiled for 10 min, then used for SDS-PAGE after centrifuged at 10,000 g for 10 min at 4°C. Protein concentration was determined with a Bradford protein assay kit (Solarbio, Beijing). Protein samples (10 μg of each) were resolved by 12% (w/v) SDS-PAGE, and transferred to a polyvinylidene fluoride membrane for immunoblotting analysis of the photosynthetic enzymes. All the primary antibodies used in the present study were raised against the predicted optimal epitopic antigens of the conserved amino acid sequences of PEPC, PPDK, NAD-ME, RBCL from S. aralocaspica, the amino acid residues of the epitopic antigens of these antibodies and the working dilution were as follows: anti-SaPEPC (EKLSSIDAQLR) IgG (1:500), anti-SaNAD-ME (NGRTGHVNQGNNMY) IgG (1:200), anti-SaPPDK (KLATEKGRAAKPSL) IgG (1:200), and anti-SaRBCL (QARNEGRDLAREGN [large subunit of RUBPC]) IgG (1:500), the epitopic antigens of all these enzymes from S. aralocaspica could be used in western blotting detection of S. ferganica based on sequence analysis. The secondary antibody—goat anti-rabbit IgG (conjugated horseradish peroxidase) (1:2000)—was used for detection. Bound antibodies were visualized by enhanced chemiluminescent (Biosharp, Beijing), and images were acquired by luminescent image analyzer (FUJIFILM LAS-4000, Japan). The bands were quantified by Image J 1.41 (Wayne Rasband National Institutes of Health, USA) and calculated as relative intensity (RI, %). For RI of each enzyme in YL, IL, PUL, PML, and PLL, the 'PUL' was used while for bottom, middle, and tip of leaf, the ‘Tip’ was used as reference in calculations.
Statistical Analysis
Data were analyzed and graphs were prepared by Graphad Prism 5.0 for Windows (GraphPad Software, San Diego, CA). One-way or two-way ANOVA was used to compare more than two variables. Significant differences were analyzed by a multiple comparison Tukey test at 0.05, 0.01, or 0.001 significance level.
Results
Plant Development and Morphological Characteristics of S. ferganica
S. ferganica is an annual halophyte distributed in harsh natural habitats. Its seed with winged perianth can germinate on the soil surface in the presence of high salinity. Early seedlings of S. ferganica presented with two smooth flat lanceolate cotyledons (Supplementary Figure 2A), while the true leaf was succulent and appeared as a long clavate shape, more interestingly, which was covered with white, thick, long, and soft trichomes (Supplementary Figure 2B), and these remained on the plant from an early seedling to an early adult stage (Supplementary Figure 2C). With further plant development, the trichomes became thinner and shorter on leaves until almost invisible (Supplementary Figures 2D, E). We found that the dynamics of trichome growth and decline on plants were corresponding to the development rhythms of S. ferganica, i.e. trichomes were thick and long at an early stage while shorter and thinner at a later stage, which suggests that trichomes must be an adaptive structure to protect seedling survival.
Cotyledon and Leaf Anatomic Structures of S. ferganica and S. aralocaspica
Besides the different appearance between the cotyledons and the true leaf form in S. ferganica, the anatomic structure was also different (Figures 1A–F). In the case of the cotyledon, M cells had no significant differentiation in their micro-structure (Figures 1A–C); however, M cells of the true leaf were specialized and organized into a layer of elongated, palisade-like cells around a ring of BS cells (Kranz structure) inside (Figures 1D–F); in contrast, the appearance and anatomic structures of the cotyledon (Figures 1I–K) and leaf (Figures 1L–N) of S. aralocaspica were similar, in which the chlorenchyma cells were organized as a layer of elongated, palisade-like cells. Analysis of immuno-histochemical localization showed that, in S. ferganica, PEPC was distributed throughout the M cells, while RUBPC presented in BS cells (Figures 1G, H); in S. aralocaspica, PEPC was observed in all of chlorenchyma cells while RUBPC was only found at the proximal end (Figures 1O, P).
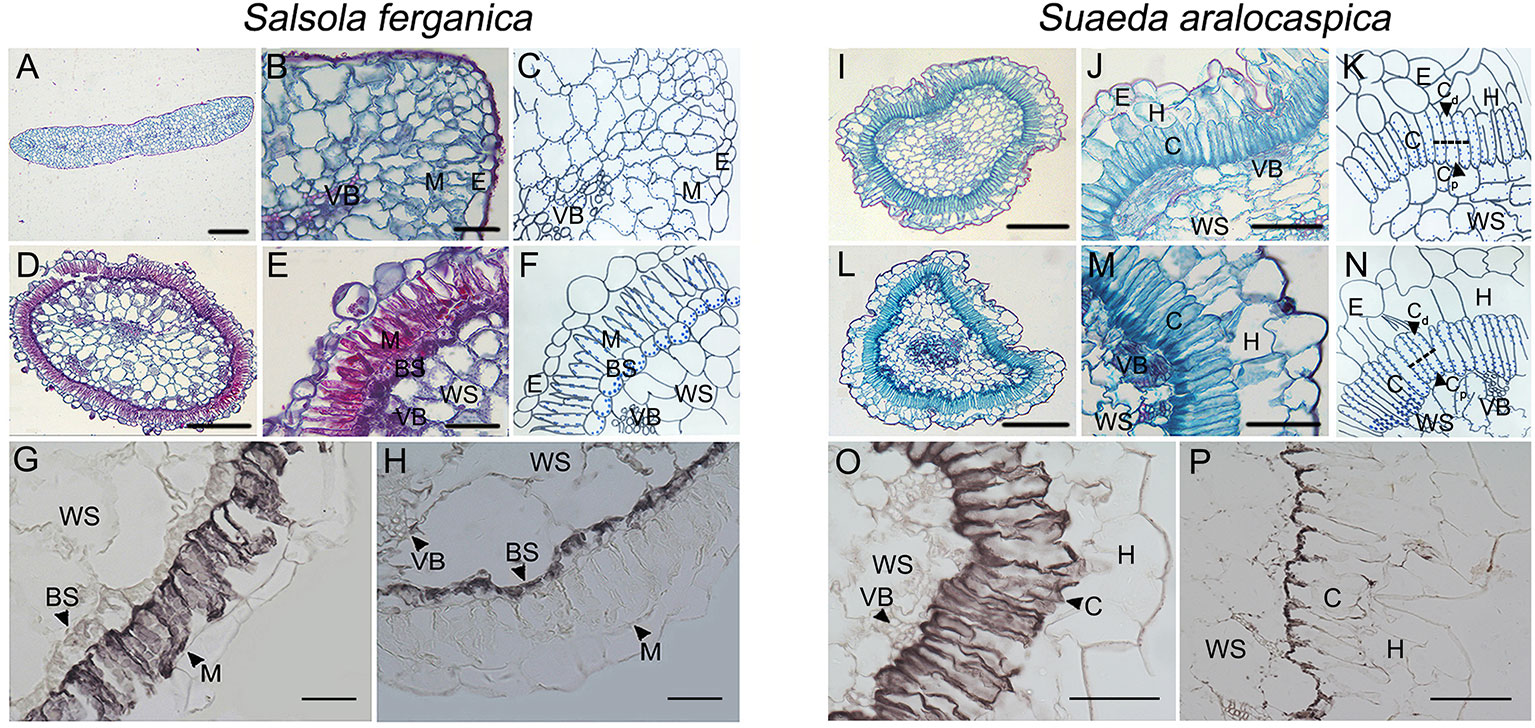
Figure 1 Anatomic structures of cotyledon and leaf and immunohistochemical localization of PEPC and RUBPC of leaf in S. ferganica and S. aralocaspica. (A–H) S. ferganica; (I–P) S. aralocaspica; (A, D, I, L) Images of whole leaf cross section; (B, E, J, M) Enlarged images of part leaf cross section; (A, B, I, J) Cotyledon; (D, E, L, M) Leaf; (C, F, K, N) The schematic drawing corresponding to B, E, J, M; (G, O) PEPC; (H, P) RUBPC. BS, bundle sheath; C, chlorenchyma; M, mesophyll; VB, vascular bundle; WS, water storage. The images of anatomic structure and immunohistochemical localization of PEPC and RUBPC were all acquired from mature leaves of outdoor plant. Scale bar in A, D, I, L is 200 μm; B, E, G, H, J, M, O, P is 50 μm.
Starch Staining in Developing Cotyledon and Leaf in S. ferganica and S. aralocaspica
Starch staining in cotyledon at 0 d, 4th d, and 8th d after germination was visualized in both plant species by paraffin sections under the microscope (no true leaf emerged at this moment in either S. ferganica or S. aralocaspica) (Figure 2). Starch granules in the cotyledon of S. ferganica (C3 structure) at 0 d were mainly accumulated in the interior M cells (Figures 2A, E), but were distributed in most of the M cells on the 4th d as the cotyledon developed and the starch staining was stronger in cells near the lower surface than the upper surface (Figures 2B, F). At the 8th d, the starch distribution pattern in cotyledons was similar to that of the 4thd (Figures 2C, G). In the mature true leaf of S. ferganica (C4 structure), starch granules were mainly distributed in the interior bottom of BS cells, and some in the periplasm of water storage (WS) cells (Figures 2D, H).
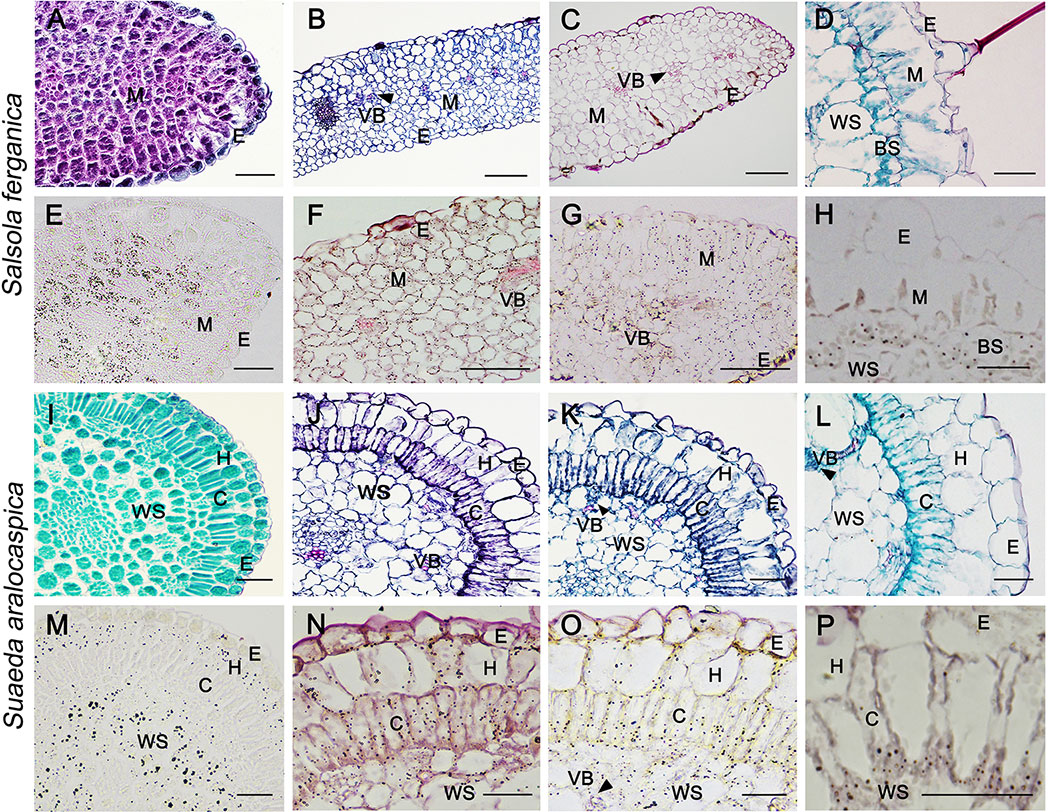
Figure 2 General anatomy (upper panel) and starch localization (lower panel) in developing cotyledon and true leaf of S. ferganica and S. aralocaspica. (A, E, I, M) Cotyledon, 0 d; (B, F, J, N) Cotyledon, 4th d; (C, G, K, O) Cotyledon, 8th d; (D, H, L, P) Mature leaf. BS, bundle sheath; C, chlorenchyma; E, epidermis; H, hypodermis; M, mesophyll; VB, vascular bundle; WS, water storage. Scale bar is 50 μm.
In S. aralocaspica, starch granules in the cotyledon (C4 structure) at 0 d were mainly distributed in outer the layer of hypodermal cells and inner WS cells (Figures 2I, M). With cell structure differentiation, the single layer of chlorenchyma cells was elongated in the radial direction and formed a palisade-like cell layer with cytoplasm polarized towards the proximal and distal ends. At the 4th d, starch granules were distributed in the peripheral cytosol of chlorenchyma, hypodermal and WS cells (Figures 2J, N). At the 8th d, palisade-like chlorenchyma cells became longer, and starch granules were accumulated at the proximal end of chlorenchyma cells (Figures 2K, O). In the mature true leaf, starch granules were mainly distributed at the proximal end of apparently elongated chlorenchyma cells (Figures 2L, P).
Characteristics of MC: BSC and Cd: Cp Ratio and δ13C Value in S. ferganica and S. aralocaspica
Results of cell area ratio showed that it was 1.42 (MC : BSC) for S. ferganica, and 1.67 for S. aralocaspica (Cd : Cp of single chlorenchyma cell). At the same time, two different sizes of chloroplasts were observed in both species, i.e. in the inner BS cell of S. ferganica or proximal end of chlorenchyma cells of S. aralocaspica oval chloroplasts were observed, but in peripheral M cells or the distal end, long thin oval chloroplasts were present (Figure 3; Table 3). Analysis of the δ13C value indicated that it changed significantly under different conditions in S. ferganica, i.e. −16.15‰ in outdoor adult plant in summer; −20.41‰ or −21.73‰ in greenhouse seedlings in winter or greenhouse adult plant in summer. Compared to SC-C4 S. aralocaspica with values of −14.87‰ in greenhouse seedlings in winter, and the C3 species C. album (−34.15‰) or N. tabacum (−33.10‰) in greenhouse adult plant in summer, the above results suggest that the δ13C value of S. ferganica is flexible and can adjust with the development progression of the plant and when environmental conditions change (Table 4).
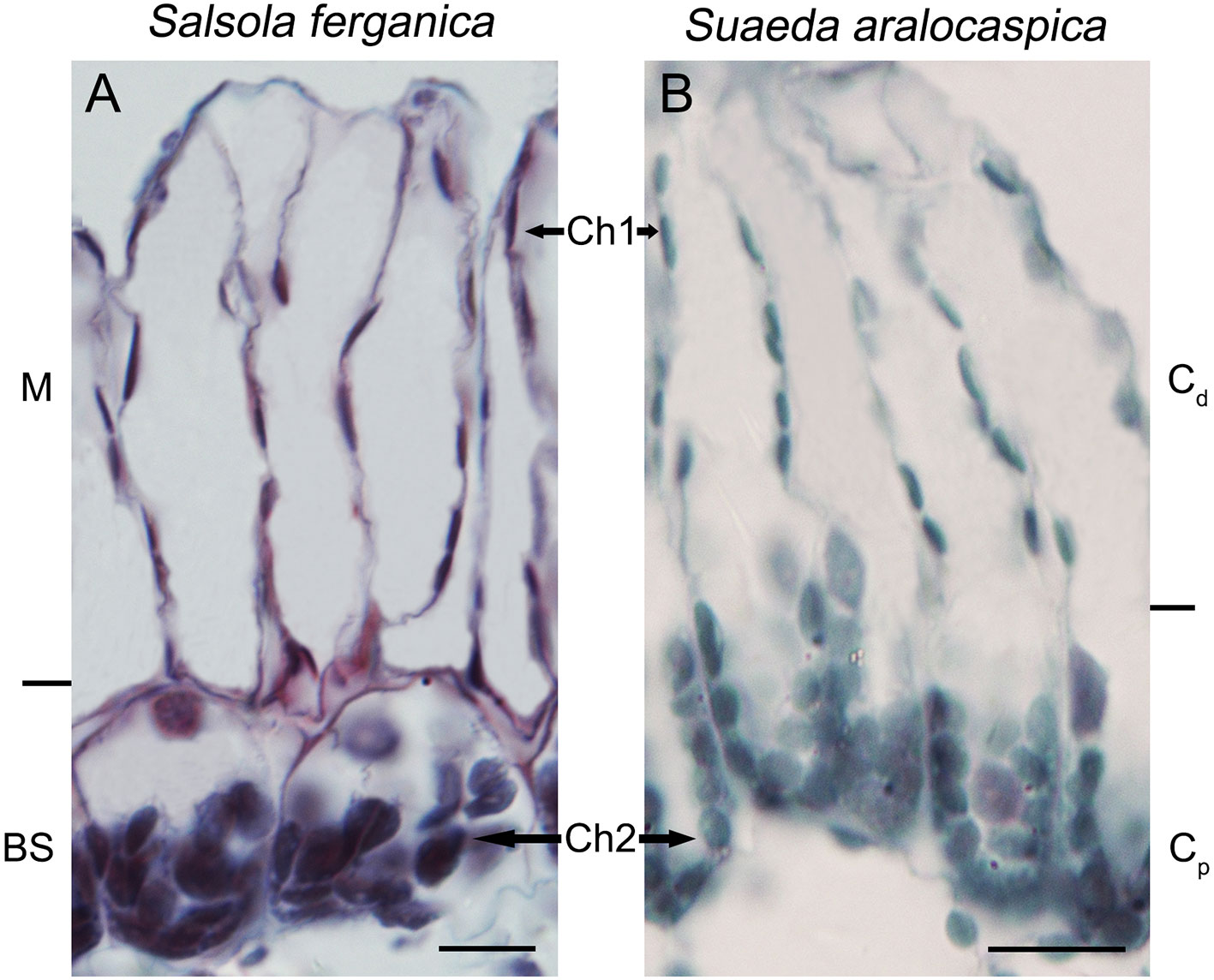
Figure 3 The shapes and sizes of dimorphic chloroplasts in S. ferganica and S. aralocaspica. (A) S. ferganica; (B) S. aralocaspica. Samples were collected from indoor plant. BS, bundle sheath; Ch1, chloroplast in MC (S. ferganica) or Cd (S. aralocaspica); Ch2, chloroplast in BSC (S. ferganica) or Cp (S. aralocaspica); Cd, distal end of chlorenchyma cell; Cp, proximal end of chlorenchyma cell; M, mesophyll. Scale bar is 10 μm.

Table 3 Properties of anatomic structures of mesophyll cells (MC), bundle sheath cells (BSC), chlorenchyma cells (CC), and dimorphic chloroplasts in S. ferganica and S. aralocaspica.
Characteristics of PEPC and RUBPC Activity in S. ferganica and S. aralocaspica
Diurnal Changes
To understand PEPC and RUBPC activity in response to the alteration of light intensity during a whole day, we measured the enzyme activity from 8:00 am (08:00) in the morning until 10:00 pm (22:00) in the evening at 2 h intervals (Figures 4A–F). The overall PEPC activity (PA) of S. ferganica was much lower than that of S. aralocaspica (Figures 4A, B), especially that of outdoor plants; while RUBPC activity (RA) exhibited the opposite trend between the two species (Figures 4D, E), with higher RA in indoor plants compared to activity in outdoor plants. Between the two enzymes, RA was higher than that of PA in S. ferganica, especially from indoor plants (Figures 4A, D); while in S. aralocaspica, the two enzymes showed the opposite trend (Figures 4B, E). The highest PA was observed in outdoor grown S. aralocaspica (Figures 4A, B), while the highest RA appeared in S. ferganica that had been grown indoors (Figures 4D, E). Our results indicate a tendency of PA in S. ferganica and S. aralocaspica to present as a “double peak” pattern; for indoor plants, two higher PA values presented at 12:00 pm (noon) and 6:00 pm (18:00) in the early evening (Figures 4A, B); for outdoor plants, these were at 10:00 am or 12:00 pm in the morning and at noon, and at 8:00 pm (20:00) in the evening. As a C3 plant control, C. glaucum presented a different pattern on PA and RA in response to diurnal changes, which were at a lower activity level and appeared to be insensitive to light intensity (Figures 4C, F). Our results suggest that the differences in activity of two photosynthetic enzymes exist between S. ferganica and S. aralocaspica, in which the former appeared as non-typical C4 plant characteristics compared to that of S. aralocaspica.
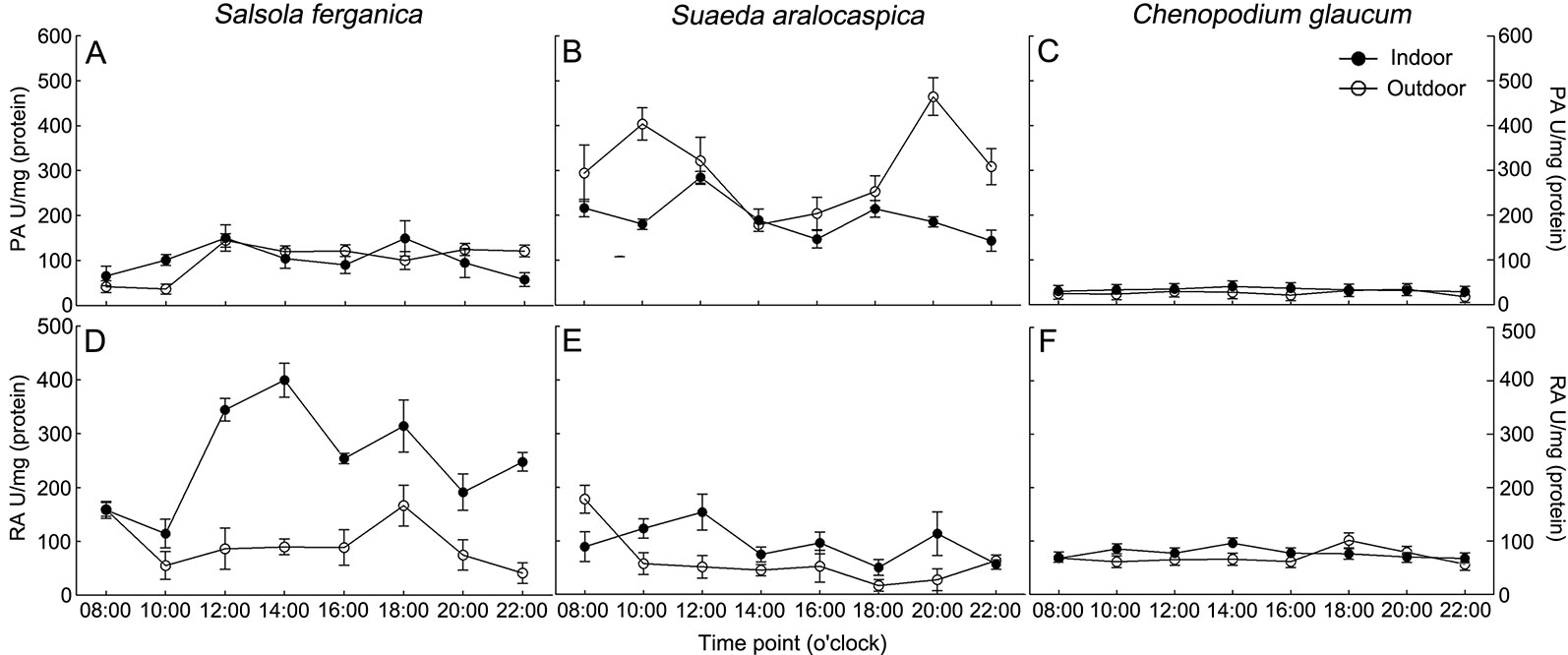
Figure 4 Diurnal changes of PEPC and RUBPC activity in S. ferganica, S. aralocaspica and C. glaucum. (A, D) S. ferganica; (B, E) S. aralocaspica; (C, F) C. glaucum; (A–C) PEPC activity; (D–F) RUBPC activity. Indoor, in greenhouse; Outdoor, out of greenhouse. PA, PEPC activity; RA, RUBPC activity.
Developmental Changes and Salt Responses
PA and RA under NaCl treatment or different growth periods showed that, for S. ferganica, PA was increased at 15 d and 30 d compared to that of 7 d plants, while there was no large change between 15 d and 30 d plants (Figure 5A). Moreover, at early developmental time (7 d or 15 d), PA was increased with rising NaCl concentration; whereas RA was increased significantly at 30 d compared to that of 7 d or 15 d; however, there was no large change of PA or RA between 7 d and 15 d plants, or among different NaCl concentrations (Figure 5B). For S. aralocaspica, PA was increased with seedling development at lower or medium NaCl concentrations between 7 d and 15 d (or 30 d) after emergence (Figure 5D); RA was increased at 15 d while reduced at 30 d but there was no significant change among different NaCl concentrations (Figure 5E). For the C3 species control plants: C. glaucum (halophyte) or N. tabacum (glycophyte) presented a much lower PA level than that of S. ferganica or S. aralocaspica, there was almost no large change among different developmental periods or NaCl concentrations (Figures 5G, J); RA of C. glaucum was at a similar level and pattern to RA in S. aralocaspica, and declined with increasing NaCl concentration (Figure 5H); while RA of N. tabacum was the highest among the four species, and was increased with seedling development, although reduced with increasing NaCl concentration (Figure 5K). The above changes of PA and RA resulted in an increase of PEPC : RUBPC ratio (P:R) from 7 d to 15 d but this decreased at 30 d plants in S. ferganica: the highest value observed was about 10 (500 mM NaCl at 15 d) and the lowest was around 3 (control at 7 d and 500 mM NaCl at 30 d) (Figure 5C). For S. aralocaspica, the P:R ratio was increased from 7 d to 30 d except for the higher NaCl concentrations at 15 d or 30 d (Figure 5F), the highest value was about 40 (control at 30 d) and the lowest was around 5 (500 mM NaCl at 15 d); for C. glaucum, P:R was increased with increasing NaCl concentration and most of the values were lower than 1.0 (Figure 5I); for N. tabacum, P:R was reduced with seedling development and in general lower than 0.5 (Figure 5L). Results of PA and P:R suggest that the photosynthetic physiology of S. ferganica presents a difference compared to that of S. aralocaspica, which exhibited as non-typical C4 characteristics.
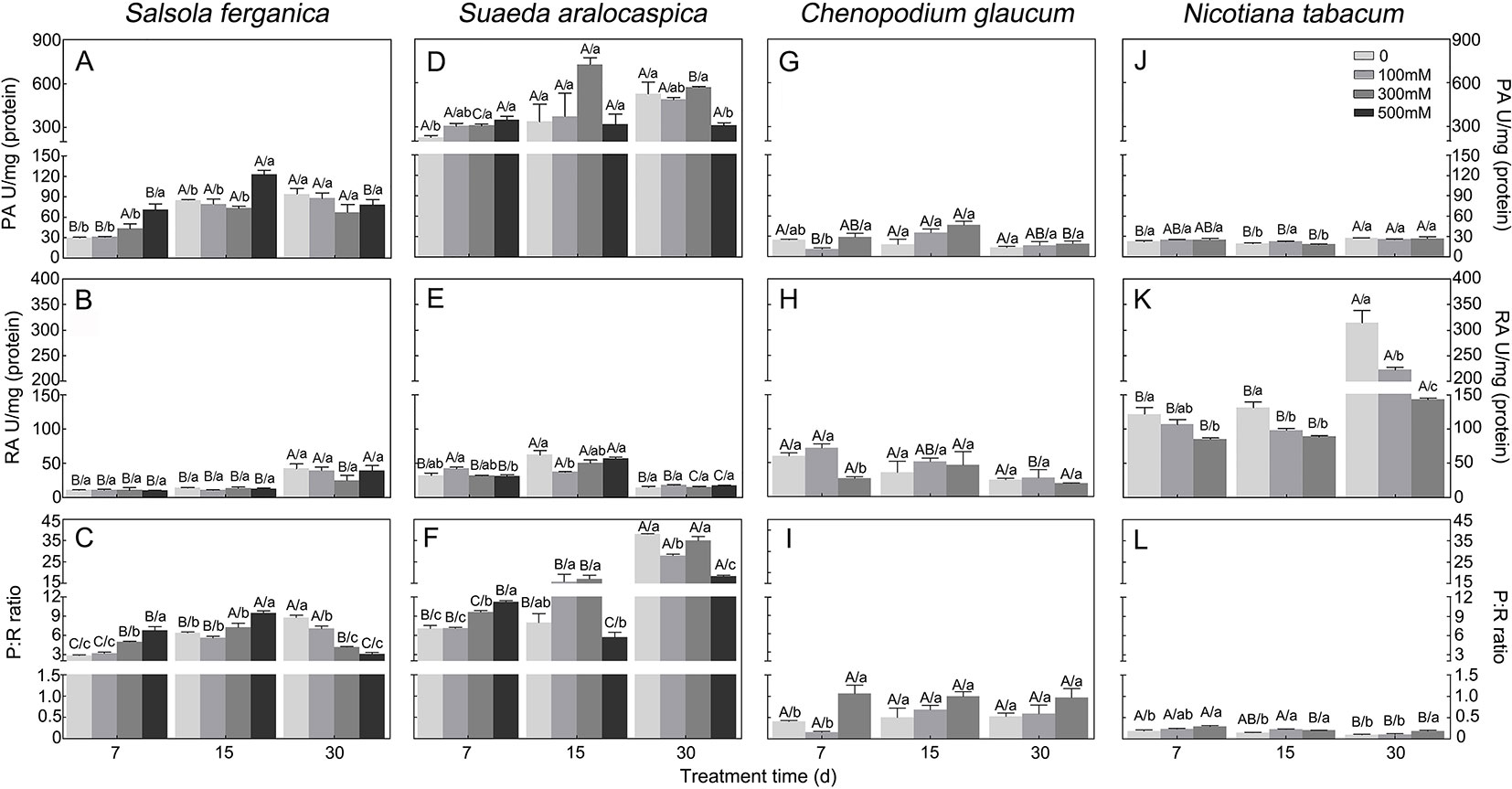
Figure 5 Changes of PEPC and RUBPC activity and its ratio in S. ferganica, S. aralocaspica, C. glaucum, and N. tabacum under NaCl treatment at different developmental stages. (A–C) S. ferganica; (D–F) S. aralocaspica; (G–I) C. glaucum; (J–L) N. tabacum; (A, D, G, J) PEPC activity; (B, E, H, K) RUBPC activity; (C, F, I, L) Ratio of PEPC: RUBPC. PA, PEPC activity; RA, RUBPC activity; P: R, PEPC activity: RUBPC activity; 7, 15, 30: Days of seedling after emergence. Different lowercase letters above columns indicate significant differences (P < 0.05 or 0.01) among different NaCl concentrations at the same developmental stage; different uppercase letters above columns indicate significant difference (P < 0.05 or 0.01) among different developmental stages at the same NaCl concentration. Values are means ± SE of four replicates.
Developmental Changes of Amount and Localization of PEPC and RUBPC in Leaf of S. ferganica and S. aralocaspica
In both S. ferganica and S. aralocaspica, structural differentiation of BS and M cells (in S. ferganica) or chlorenchyma cells (in S. aralocaspica) of young leaves (0.5–0.6 cm long) was inspected using transverse (TS) and longitudinal (LS) sections under the microscope (Figures 6 and 7). In situ immuno-histochemical localization (ISIHL) was analyzed based on results of paraffin sections.
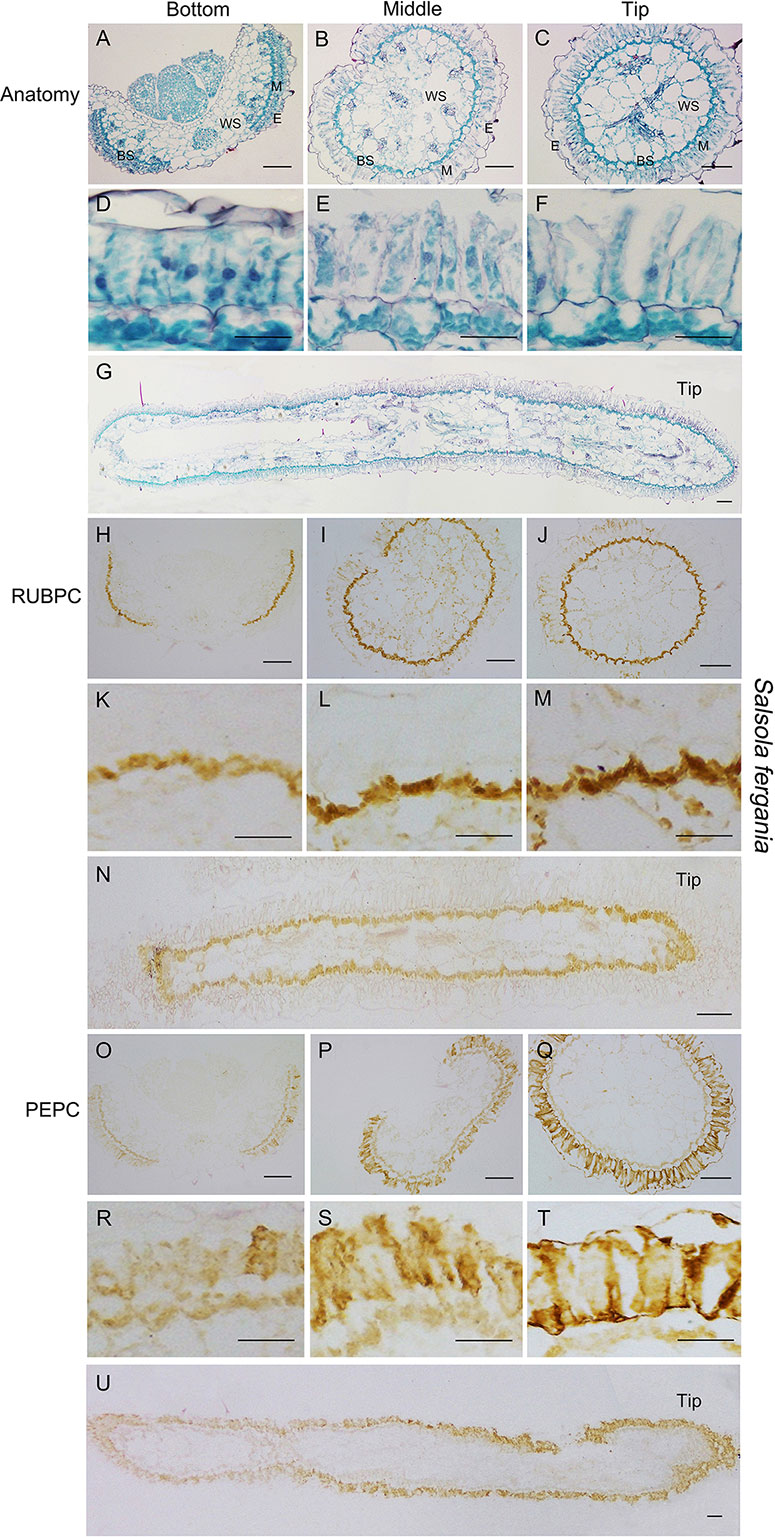
Figure 6 Anatomic structure of leaf and in situ immuno-histochemical localization (ISIHL) of RUBPC and PEPC of S. ferganica with transverse and longitudinal sections of young leaves (0.5–0.6 cm), showing a progressive development with gradual structure differentiation. (A–G) Anatomic structure of young leaf; (H–U) ISIHL results; (A–C) Transverse sections from the bottom to tip of leaf; (D–F) Detailed views corresponding to A–C; (G) Longitudinal sections of leaf; (H–J) ISIHL of RUBPC (RBCL) at the bottom, middle, and tip of leaf; (K–M) Detailed views corresponding to H–J; (N) ISIHL of RUBPC (RBCL) of longitudinal direction of leaf; (O–Q) ISIHL of PEPC at bottom, middle, and tip of leaf; (R–T) Detailed views corresponding to O–Q; (U) ISIHL of PEPC of longitudinal direction of leaf. For LS structure of leaf, the tip is on the right. BS, bundle sheath; E, epidermis; H, hypodermis; M, mesophyll; WS, water storage. Scale bar in A–C, G–J, N–Q, U is 100 µm; in D–F, K-M, R–T is 50 µm.
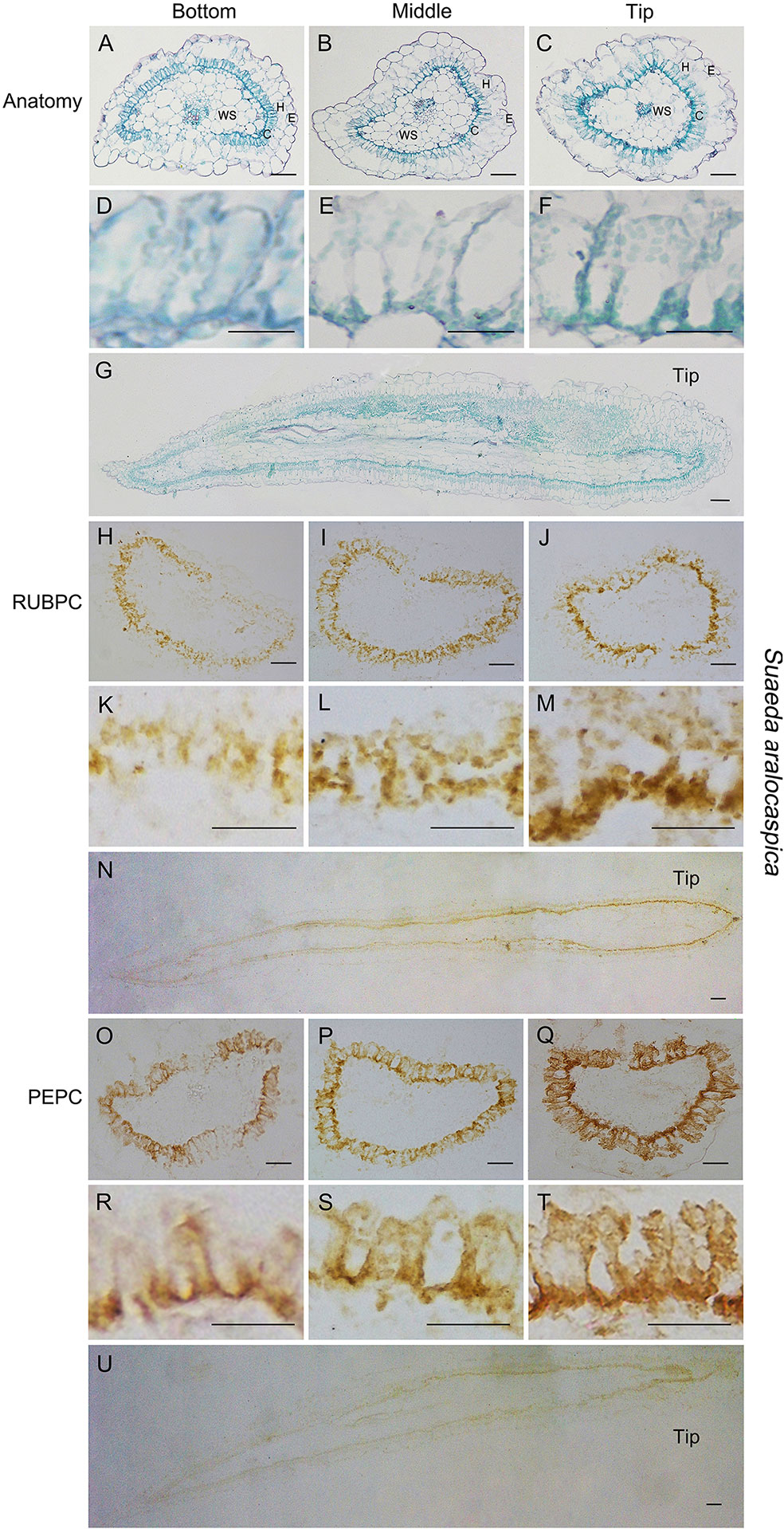
Figure 7 Anatomic structure of leaf and in situ immune-histochemical localization (ISIHL) of RUBPC and PEPC in S. aralocaspica with transverse and longitudinal sections of young leaves (0.5–0.6 cm), showing a progressive development with gradual structure differentiation. (A–G) Anatomic structure of young leaf; (H–U) ISIHL results; (A–C) Transverse sections from the bottom to tip of leaf; (D–F) Detailed views corresponding to A–C; (G) Longitudinal sections of leaf; (H–J) ISIHL of RUBPC (RBCL) at the bottom, middle, and tip of leaf; (K–M) Detailed views corresponding to H–J; (N) ISIHL of RUBPC (RBCL) of longitudinal direction of leaf; (O–Q) ISIHL of PEPC at the bottom, middle, and tip of leaf; (R–T) Detailed views corresponding to O–Q; (U) ISIHL of PEPC of longitudinal direction of leaf. For LS structure of leaf, the tip is on the right. C, chlorenchyma; E, epidermis; H, hypodermis; WS, water storage. Scale bar in A–C, G–J, N–Q, U is 100 µm; in K–M, R–T is 50 µm; D–F is 25 µm.
In S. ferganica, TS results showed that cells at the bottom of the leaf were less developed, BS cells presented as a discontinuous structure and were compact oblate in appearance, M cells were tightly arranged around the BS with thick cytosol and striking nucleus; while at the tip of the leaf, a continuous layer of BS cells was observed (Figures 6A–F). In addition, the cell volume became larger: M cells were enlarged and elongated, their cytosol became thinner, and chloroplasts became distributed along the radial cell periplasm. Compared to cells at the bottom, an interspace was introduced among M cells and between M cells and epidermal cells. LS results visualized a clear differentiation in BS and M cells from the bottom to the tip of the leaf, which presented a gradual developmental variation in structural specialization accompanying cell expansion (Figure 6G). Structure observations showed a higher differentiation in the tip cells while there was less among the bottom cells of the leaf. ISIHL analysis revealed that RUBPC was mainly distributed in BS cells and PEPC was widely distributed in the M cells in S. ferganica (Figures 6H–U). With photosynthetic tissue differentiated from the bottom to the tip of the leaf, the amount of RUBPC and PEPC protein was increased. Based on the analyses of TS and LS anatomic structure and enzyme localization we found that the expression of two enzymes was enhanced from the bottom to the tip of the leaf in S. ferganica.
In S. aralocaspica, TS and LS results showed that chlorenchyma cells near the leaf base were smaller and shorter, while cells at the tip were apparently developmentally advanced and similar to mature chlorenchyma cells, in which the chloroplasts were distributed to the proximal end or distal part, an apparent “chloroplast-free region” was also present in the middle part of chlorenchyma cells (Figures 7A–G). ISIHL analysis showed that the amount of RUBPC and PEPC protein increased with the leaf maturation, and from the bottom to the tip cells of the leaf (Figures 7H–U). The distribution of RUBPC was apparently concentrated to the proximal end in the tip cells compared to an irregular distribution in the bottom cells of young leaves (Figures 7H–N); PEPC was enhanced around the whole periplasm of chlorenchyma cells (Figures 7O–U).
Apart from the longitudinal gradient development of young leave, photosynthetic structural and biochemical enhancement was also observed with plant development progression. In S. ferganica, clear differentiation in BS and M cells was present from early seedling (0.2 cm leaf) to mature seedling (1.0 cm leaf) (Figures 8A–J), both types of cells were expanded, the BS cells were arranged from discontinuous to continuous, and M cells were elongated. These changes presented a similar pattern with the differentiation in the same leaf from the bottom to the tip (Figure 6). ISIHL analysis showed that RUBPC was mainly distributed in BS cells while PEPC was in the whole periplasm of M cells (Figures 8K–T). With the maturation of the leaf, the expression of RUBPC and PEPC was increased.
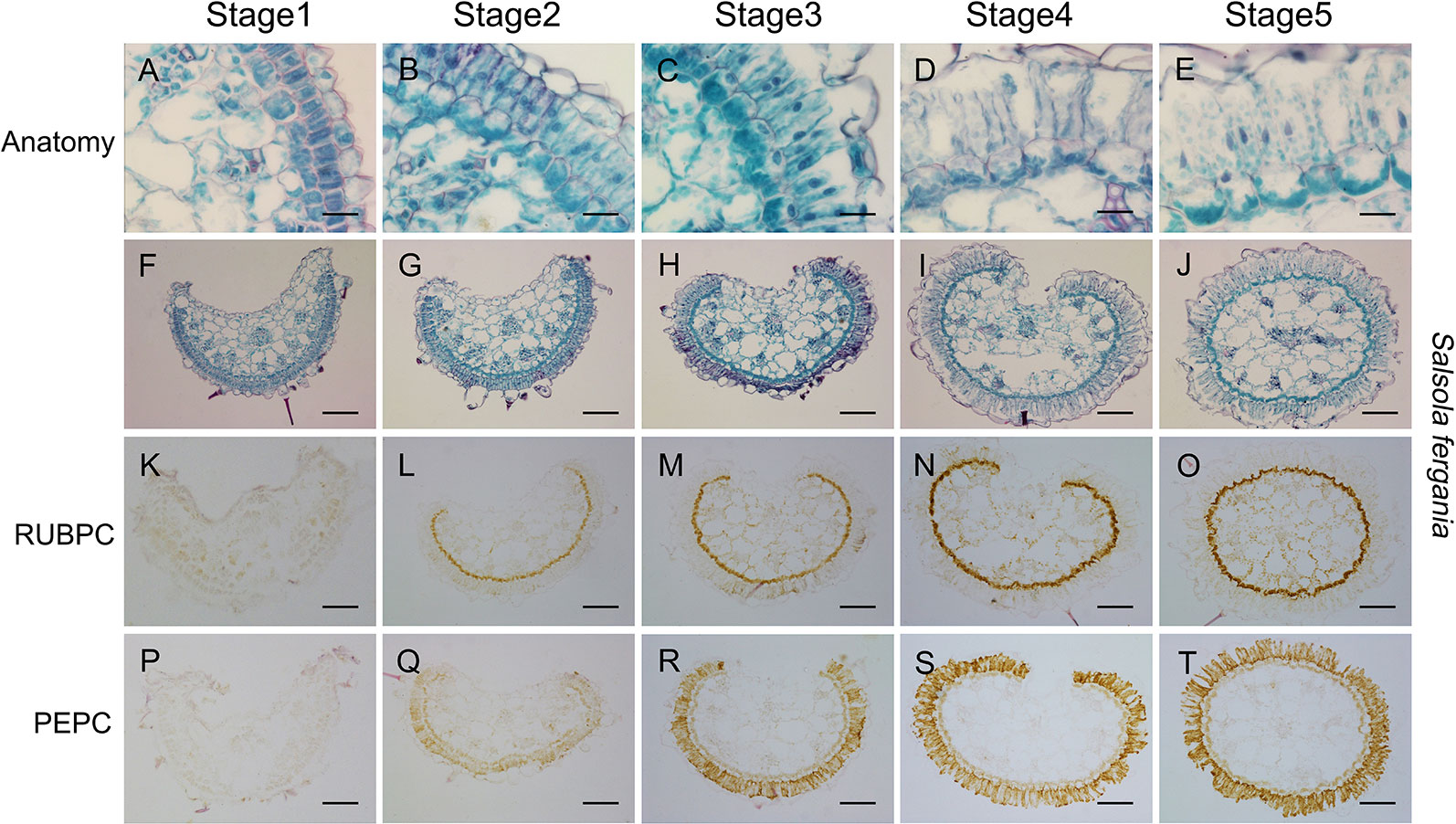
Figure 8 Anatomic structure of leaf and in situ immuno-histochemical localization (ISIHL) of RUBPC and PEPC of S. ferganica with transverse sections in the middle part of leaves at different developmental stages, showing a developmental gradient of structure differentiation. (A–J) Anatomic structure; (K–T) ISIHL results; (A–E) Middle part of transverse sections of leaf at different developmental stages (0.2 cm, 0.5 cm, 1.0 cm of leaf); (F–J) Detailed views of bundle sheath and mesophyll cells corresponding to images in A–E; (K–O) ISIHL of RUBPC (RBCL) corresponding to images in A–E; (P–T) ISIHL of PEPC corresponding to images in A–E. BS, bundle sheath; E, epidermis; H, hypodermis; M, mesophyll; VB, vascular bundle; WS, water storage. Scale bar in A–E is 25 µm; in F–T is 100 µm.
Besides the above findings, we also found that photosynthetic structures in sepals also presented a differentiation gradient similar to that observed for C4 type Kranz-anatomy (Figures 9A–I), although the flower would produce an embryo with C3-type cotyledons. With flower development, both BS cells and M cells in sepals were expanded and extended, chloroplasts were differentiated and re-located around cells (Figures 9G–I).
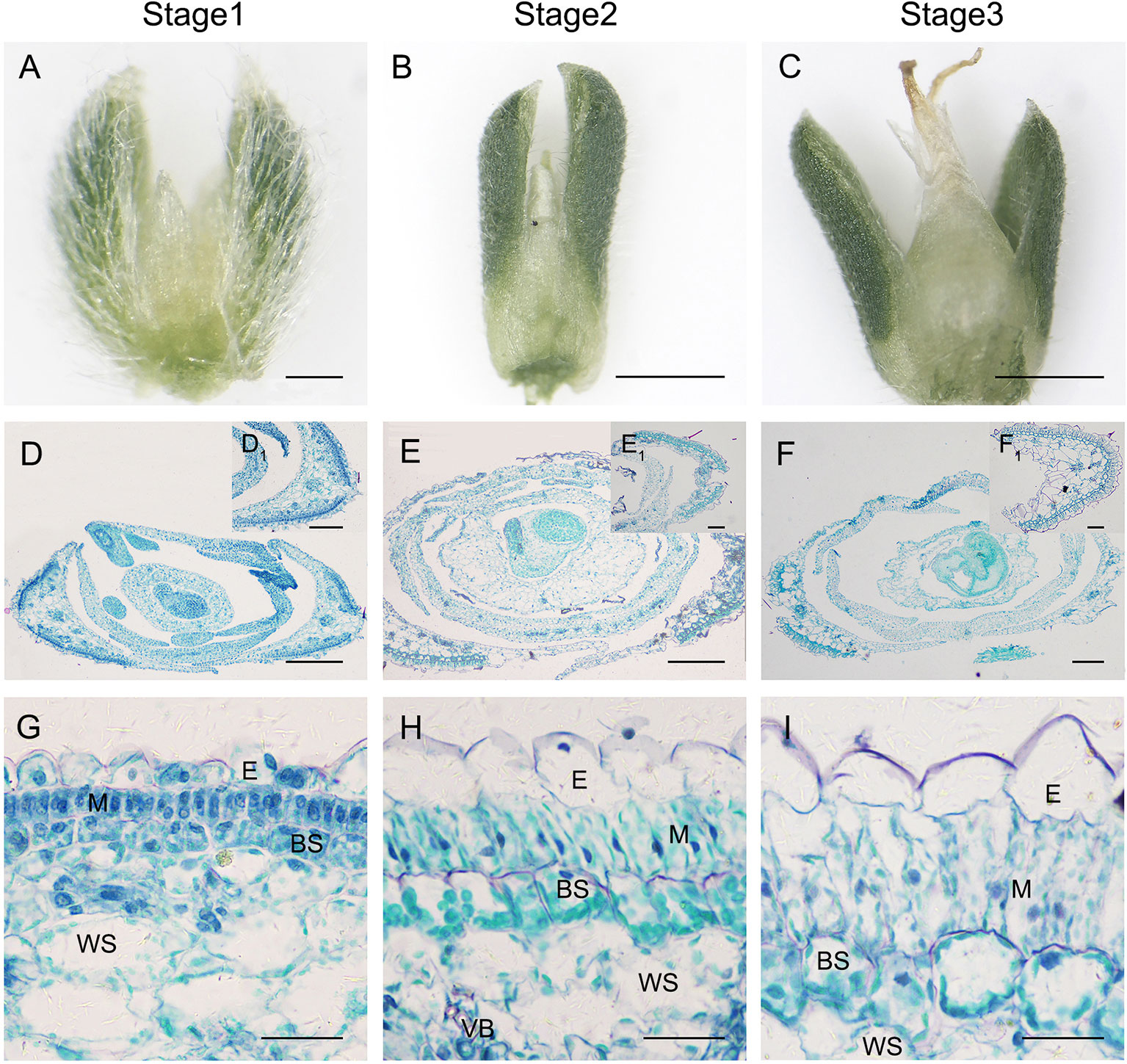
Figure 9 Anatomic structure of sepal in S. ferganica with transverse sections in the middle part of the flower. (A–C) Developing flowers, showing sepals on both sides; (D–F) Transverse sections of developing flowers; (D1–F1) Sepal section insets in the upper right position of D–F; (G–I) Detailed views of anatomic structure of sepal. Stage 1, Stage 2, Stage 3: different developmental times of flower. BS, bundle sheath; E, epidermis; H, hypodermis; M, mesophyll; VB, vascular bundle; WS, water storage. The images were acquired from the middle part of the flowers of indoor plant. Scale bar in A, (D1–F1) is 100 µm; in B, C is 500 µm; in D–F is 200 µm; in G-I is 50 µm.
Transcriptional Expression Profiles of Photosynthetic Genes Along Longitudinal Leaf Direction in S. ferganica and S. aralocaspica
To investigate expression patterns of photosynthesis-related genes in leaf development in S. ferganica and S. aralocaspica, qPCR analyses of PEPC1, PEPC2, PPDK, and RBCL (large subunit gene of RUBPC) in leaves located in different positions of the plant or along the longitudinal direction of the leaf were conducted. Results showed that, for whole plants, the highest expression level was found in the top part (Figures 10A, B), the next was that of the upper part (Figures 10C, D), the lowest was in the lower part of leaves (Figures 10E, F); for along the longitudinal direction of a leaf, four genes were remarkably up-regulated from the bottom to the tip, especially in young leaves (0.5–0.6 cm) (Figures 10A, B). We also distinguished a transcriptional expression profile between PEPC1 and PEPC2, which we had also identified in our previous work. These two genes presented a similar expression pattern, in which PEPC1 was more active than PEPC2 in different parts of the plant or at different longitudinal positions of the leaf.
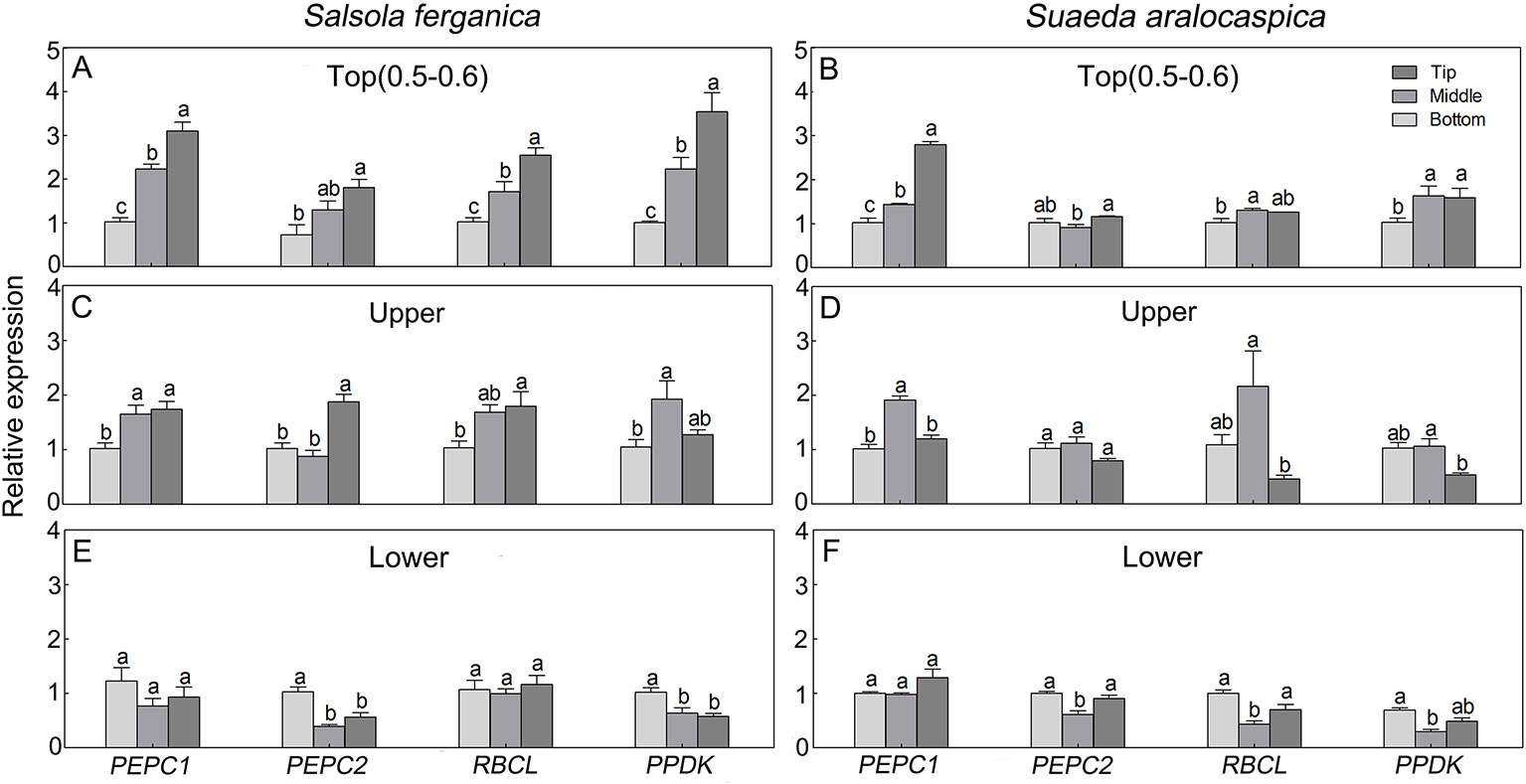
Figure 10 Transcriptional expression patterns of key photosynthetic genes in leaf of different positions of plant or along longitudinal direction in S. ferganica and S. aralocaspica. (A, C, E) S. ferganica; (B, D, F) S. aralocaspica; (A, B) 0.5–0.6 cm young leaf in top of plant; (C, D) Upper part leaf of plant (1.3–1.5 cm leaf in B; 1.8–1.9 cm in E); (E, F) Lower part leaf of plant (1 cm leaf in C; 2 cm in F). Bottom, Middle, Tip: Different segments along longitudinal leaf gradient. All samples were collected from indoor plants. Relative expression level is represented as the sample transcripts subtract the reference transcripts. Different lowercase letters above columns indicate significant differences (P < 0.05 or 0.01) of the same gene between positions in one leaf. Values are means ± SE of three biological and two technical replicates (total of six replicates).
Translational Expression Patterns of Photosynthetic Enzymes Along Longitudinal Leaf Direction in S. ferganica
Translational accumulation of the representative C4 enzymes [RBCL (large subunit of RUBPC), NAD-ME, PPDK, PEPC] was analyzed by western blotting during leaf development in S. ferganica (Figure 11). All four proteins increased gradually as development progressed from the bottom to the tip of a single leaf, and with the highest level found in PUL or PML in mature leaves (Figures 11A–C). Among these, RBCL appeared to be the most abundant, this would be the expected given level of RBCL typically found in leaves (Koteyeva et al., 2011a; Koteyeva et al., 2014; Koteyeva et al., 2016), which increased along the gradient from the lower (PLL) to the middle (PML) or the upper (PUL) leaves of the plant. PEPC, NAD-ME, and PPDK were also remarkably accumulated as leaf development progressed and along the longitudinal direction of a single leaf. The bottom segment of young leaves presented with considerably lower levels of these proteins, except for RBCL. In total, the accumulation of the soluble protein of each enzyme showed initially a lower level in young leaves or the lower leaves of plants or at the bottom of a single leaf, and at higher levels in mature leaves or the upper leaves of plants or the tip of a single leaf.
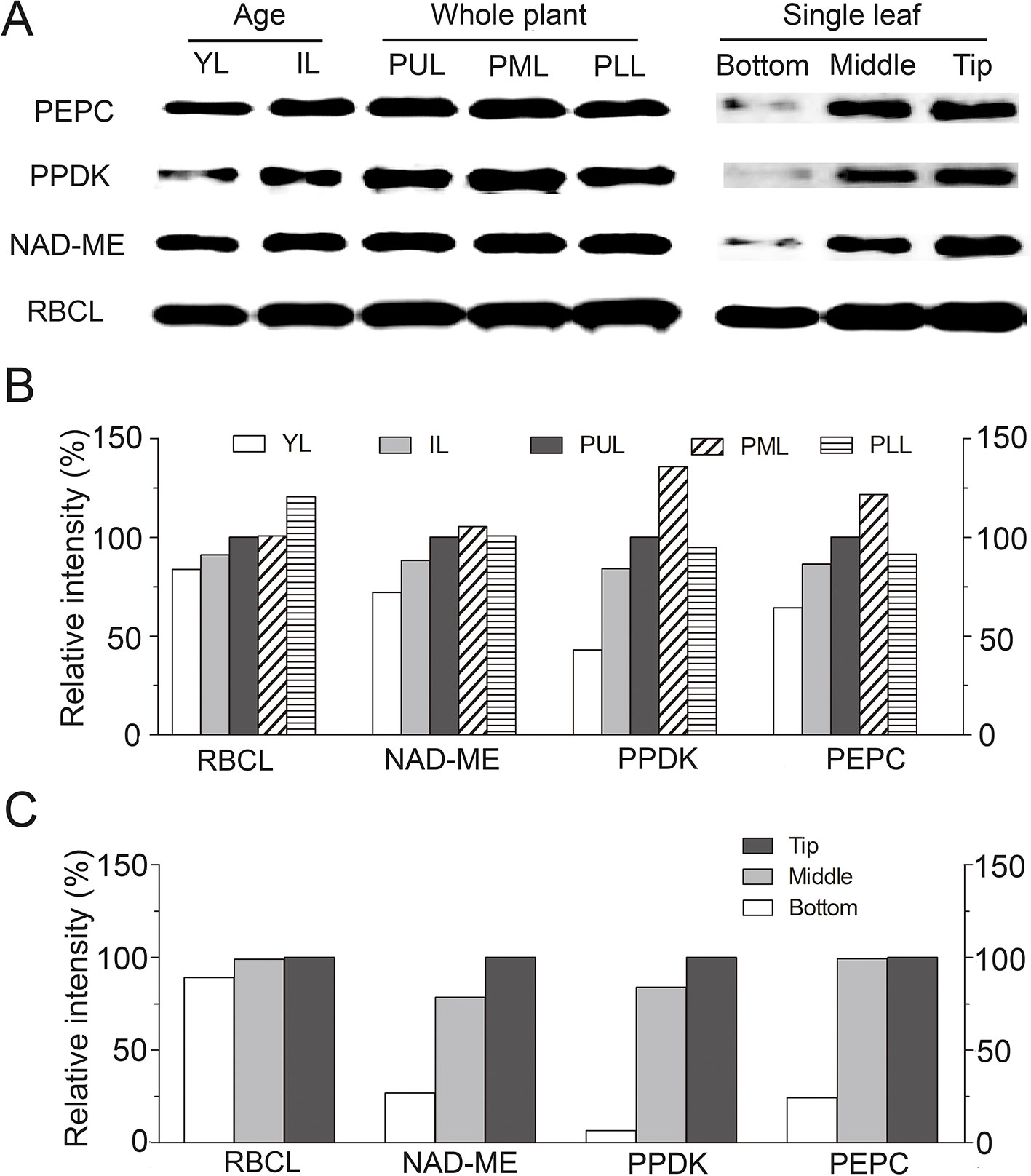
Figure 11 Translational expression patterns of key photosynthetic enzymes in leaf of different positions of plant or along longitudinal direction in S. ferganica. (A) Western blotting showing the detection of four proteins; (B, C) Quantitative expression of western blotting data by ImageJ. YL, young leaf (0.2–0.3 cm); IL, intermediate leaf (0.5–0.6 cm); PUL, PML, PLL: the upper, middle, lower layer of the plant; Bottom, Middle, Tip: Different segments, along longitudinal leaf gradient. Relative intensity (RI) is represented as the sample intensity divides the reference intensity. For RI of each enzyme in YL, IL, PUL, PML, and PLL, the ‘PUL’ was used while for bottom, middle, and tip of leaf, the 'Tip' was used as reference in calculation. All samples were collected from indoor plants. Total soluble proteins were extracted from different ages of leaves or different parts of single leaf. Protein loading amount in each lane was 10 μg. Preparation and dilution of first and secondary antibodies were described in Materials and Methods.
Discussion
Salsola is a large genus in Amaranthaceae, in which most species belong to halophytes or xerophytes and are distributed in extremely harsh environments (Akhani et al., 1997). A variety of structural and physiological adaptation mechanisms have been formed in these species in long-term evolutionary processes, among which the diversity of photosynthetic assimilation pathways is one of the typical characteristics in response to stresses (Pyankov et al., 2010). S. ferganica is one of the halophytes in Salsola, which possesses an enhanced ability for adaptation to adverse environments (Huang, 2005). So far, documentation suggests that S. ferganica has C4 Kranz-type structure and its δ13C value is in the range of C4 plants (Wen and Zhang, 2011). However, we found that large changes existed in leaf anatomic structures at different developmental stages and in some photosynthetic physiological behaviors in this species. Based on the previous findings, in the present study, we tried to explore the possible mechanisms of enhancement in photosynthetic structures and corresponding enzyme activities and gene expression at transcriptional and translational levels with plant development, as well as the behaviors of photosynthetic physiology in response to varying conditions in S. ferganica. Our results revealed that cotyledons had typical C3 structures in S. ferganica; while in leaves the photosynthetic structures, chloroplast dimorphism, PEPC:RUBPC ratio, starch staining, PEPC and RUBPC localization all presented as C4-Kranz Salsoloid type; moreover, with leaf or plant development, the anatomic structures and corresponding biochemistry of C4 syndrome were enhanced, i.e. in coordination with the changes of photosynthetic structures, the enzyme activity and gene expression (in transcriptional and translational levels) were increased from the lower to upper part of a plant and from the bottom to the tip of single young leaves. Besides, we also found that PEPC and RUBPC behaviors in S. ferganica were different from the typical C4 species S. aralocaspica. Whether our results indicate S. ferganica as being in a unique status during an evolutionary process for a photosynthetic pathway or not remains unknown; however, our findings should contribute to better understanding of diversity of C4 photosynthetic pathways in developmental and physiological aspects.
The prominent characteristics in determination of photosynthetic types are anatomic structures, which are usually associated with biochemical features (Ku et al., 1983). It has been documented that at least four major types of anatomic structures of C4 assimilation tissues exist in Chenopodiaceae, i.e. Atriplicoid, Kochioid, Salsoloid, and Suaedoid (Freitag and Stichler, 2000). In the present study, we visualized the significant difference in photosynthetic structure between cotyledon (C3 type) and true leaf (C4 type) in S. ferganica, the latter presented as continuous layers of BS and M cells surrounding WS cells and vascular tissues in well-developed leaf, which belongs to Salsoloid C4 type based on reported classification (Freitag and Stichler, 2000). In Salsola, most species have Salsoloid anatomy with typical Kranz BS cells and C4 assimilation pathway, e.g. Salsola arbuscula, Salsola chivensis, etc. (Freitag and Stichler, 2000; Voznesenskaya et al., 2001b). It has been proposed that Salsoloid anatomy evolved from Sympegmoid type (Akhani et al., 1997), the latter presents two-to-three layers of M cells, instead of Kranz cells, a discontinuous layer of BS cells is arranged adjacent to the peripheral bundles, e.g. Salsola webbii (C3), S. arbusculiformis (C3-C4) in Salsola, and C3 species in genus Sympegma (Voznesenskaya et al., 2001b). Besides anatomic structures, the ratio between MC and BSC area is also important for classification of C4 photosynthetic type (Bongard-Pierce et al., 1996). In Salsola, MC : BSC ratio in different assimilation types probably ranges around 9–15 (C3), 5–10 (C3-C4), or 2–5 (C4) (Voznesenskaya et al., 2013). In the present study, S. ferganica showed a ratio of 1.42, which fell into C4 category. C4 plants with Salsoloid-type Kranz anatomy, e.g. Caroxylon orientale and Xylosalsola richteri, present ratios of 4.5 and 1.9, respectively (Voznesenskaya et al., 2013). Generally, C4 structure is coupled with dimorphic chloroplast partitioning. Chloroplasts in BS cells are usually different in size, structure, function, etc., from those in M cells. In the classical C4 plant Zea mays, chloroplasts in BS cells are deficient in grana compared to M cells (Prakitchai et al., 2016); whereas in different Kranz-type species of Chenopodiaceae, MC chloroplasts have a reduced grana size and granal index (the length of all appressed thylakoid membranes as a percentage to total length of all thylakoid membranes in chloroplasts) compared to BSC (Gamalei and Voznesenskaya, 1986; Ueno, 2005). In SC-C4 species B. sinuspersici, dimorphic chloroplasts are biochemically located in the peripheral cytoplasm (PC, for C4 cycle) or central compartment (CC, for C3 cycle) around the nucleus: chloroplasts in PC are bigger than that of CC ones (Sascha et al., 2011). In the closely related SC-C4 species B. cycloptera, the granal index of CC chloroplasts is much higher than that in PC chloroplasts, which may suggest reduced Photosystem II activity in PC chloroplasts (Voznesenskaya et al., 2002). In the present study, we found that, in S. ferganica, larger, oval chloroplasts were distributed in BS cells, while smaller, long thin oval chloroplasts were located in M cells; in the SC-C4 plant S. aralocaspica, dimorphic chloroplasts were distributed in opposite ends of elongated chlorenchyma cells, larger ones were in the proximal ends and smaller ones in the distal ends in our experiment. However, the details of internal structure of dimorphic chloroplasts in the above two species need further experiments to clarify the extent of specialization between the types. Starch is presumably used as carbon and energy sources in leaf development. Previous studies showed that starch could be accumulated in all cell types of immature maize (classical C4 species) leaf tissue, but upon maturation, accumulation stops in mesophyll cells (Weise et al., 2011). In the present study, in the C3 type of cotyledon in S. ferganica, starch granules were randomly distributed in the mesophyll cells, while in mature C4 leaves starch was mainly distributed at the bottom of the BS cells; in S. aralocaspica, starch granules were mainly distributed in the periplasm of WS and hypodermal cells in incipient leaves, while in mature leaves, starch became accumulated at the proximal ends of chlorenchyma cells. Among different C4 species in Chenopodiaceae, only Rubisco-containing chloroplasts tend to accumulate starch (Voznesenskaya et al., 1999; Voznesenskaya et al., 2003; Voznesenskaya et al., 2005), which suggests that the presence of starch is closely associated to re-location of photosynthetic enzymes (Lara et al., 2008). Based on above data, we further analyzed and confirmed that RUBPC and PEPC of S. ferganica were localized in BS cells and M cells, respectively, while those of S. aralocaspica were distributed at the proximal end (RUBPC) and the whole chlorenchyma cell (PEPC), which was consistent with the previous report (Voznesenskaya et al., 2013). Taken together, the characteristics in anatomy, ratio of MC: BSC, dimorphic chloroplast differentiation, starch staining, and localization of photosynthetic enzymes suggest that S. ferganica belongs to a Salsoloid C4 photosynthetic type.
On the basis of anatomy, physiological behaviors, e.g. activities of photosynthetic enzymes, are also important in determination of carbon assimilation status for C4 species (Voznesenskaya et al., 2001b). In general, the activity of PEPC is about 50 times higher in C4 plants, and it is about 3 times higher in C3-C4 intermediate plants, than that of a typical C3 type (Voznesenskaya et al., 2001b). In the present study, PEPC activity (PA) in S. ferganica was lower than that of S. aralocaspica, especially in outdoor plants. In maize, PA in leaves presents the highest value at noon while 6 times or 3 times lower in the morning or in the afternoon (Peng et al., 1998). Other reports on maize indicate that under optimal conditions PA is higher and no diurnal change is observed; whereas under suboptimal conditions it is reduced by over 90% and presents a “unimodal” trend in response to light intensity variation (Kalt-Torres et al., 1987). In the present study, a similar expression pattern of PA diurnal variation was found in these two species exhibiting a “bimodal” curve, the lowest value was observed at 14:00 (2:00 pm) in the afternoon; however, the fluctuation of the PA values in S. ferganica was much smaller compared to that of S. aralocaspica. We also found that PA of C. glaucum and N. tabacum (C3 species) were insensitive to light intensity in the present study. It has been reported that in C3 plants PA has no significant change over a whole day; however, strong light and high temperature at noon enhance PA in C4 species like maize (Peng et al., 1998). Light regulation of PA has also been reported in other C4 species. Compared to maize, Salsola soda displayed a more substantial effect on PA in response to light diurnal fluctuation (Karabourniotis et al., 1985). Our results on PA for the two C4 desert plant species seemed to present a “midday depression of photosynthesis,” which may be in response to the strong light intensity and high temperature in the afternoon (Table 1). Other photosynthetic enzymes also present different trends between C3 and C4 plants, the amount of RUBPC in C3 is 3–6 times higher than in C4 species (Ku et al., 1979). In rice, the increase of RUBPC activity manifests as a “bimodal” pattern, in which the midday depression is in between two increases at 10:00 (maximum) and after 14:00 (slight rise) for the stomatal closing under high temperature (Weng et al., 1999). In the present study, RA in N. tabacum (C3) and S. ferganica (C4) was significantly higher than that of S. aralocaspica, especially that of indoor plants. High temperature can significantly decrease RA (Markus et al., 2006). It may be the effect of acquisition of oxygenase activity of RUBPC under rising temperature (Laing et al., 1974). Our results suggest that in S. ferganica PEPC and RUBPC behave as non-typical C4 species compared to that of SC-C4 species of S. aralocaspica.
In the present study, PA and the ratio of PEPC : RUBPC (P:R) in S. ferganica were lower (3–5 times lower in activity; 2–5 times lower in ratio) than that of S. aralocaspica, while they were remarkable higher than that of C. glaucum and N. tabacum (C3). The P:R ratio of C4 plants is usually larger while C3 species is less than 1, and C3-C4 intermediate in Salsola is generally less than 1 but higher than C3 species (Crespo et al., 1979). Our data showed that the P:R ratio in S. ferganica and S. aralocaspica was higher than 1, while that of the latter was much higher than the former. In addition, the performance of key photosynthetic enzymes between these two C4 species was apparently different. It is known that PA increase accompanying RA decrease with stress enhancement is a typical response in C4 species (Fontaine et al., 2003; Dizengremel et al., 2009). The increased PA can potentially improve carbon metabolism during a period of reduced stomatal conductance (Cushman and Borland, 2002; Carmosilva et al., 2008). Our results suggest that PA and RA in S. ferganica does not match with the typical C4 performance. Apart from enzyme activity, in the present study, another photosynthetic physiology index -δ13C in S. ferganica was also apparently affected by environmental variations, the value of outdoor (−16.15‰) or greenhouse (−21.73‰) plants presented much greater difference (C. album [C3]: −34.15‰, N. tabacum [C3]: −33.10‰ in greenhouse in our test), which was much higher than the previous reported value of −12.754‰ in S. ferganica (Wen and Zhang, 2011). The δ13C value is vulnerable to external environmental conditions such as water moisture, temperature, drought, etc. (Chen et al., 2002; Pan et al., 2016). Usually a difference of 4–7‰ is observed between indoor grown and outdoor grown plants (Voznesenskaya et al., 2013), which might explain our difference (5.58‰) between outdoor and indoor for S. ferganica plants, however, the value of the typical C4 plant S. aralocaspica was −14.87‰ in greenhouse which was much smaller than that of S. ferganica in greenhouse (−21.73‰) or outdoor (−16.15‰). Taken together, our results suggest that the key photosynthetic physiology in S. ferganica behaves with characteristics of a non-typical C4 species compared to SC-C4 type S. aralocaspica. Whether these differences arise because S. ferganica is at different evolutionary position in its development of its C4 photosynthetic pathway compared with S. aralocaspica remains to be a question for further exploration.
To complete C4 photosynthesis at high efficiency, a progressive development is usually coupled with leaf differentiation relating to photosynthetic structure and biochemistry in many C4 plant species (Koteyeva et al., 2016). Studies on a typical Kranz-anatomy species, S. taxifolia, revealed that a basipetally developmental mode of C4 structure and biochemistry is visualized by analysis of longitudinal leaf sections (Koteyeva et al., 2011a). In the present study, the anatomic structure and ISIHL analyses indicate that Kranz-anatomy C4 species S. ferganica presented a progressive development mode for both photosynthetic structure and biochemistry along the longitudinal gradient of young leaf, moreover, such gradient changes also applied to different developmental stages in leaves of lower, middle and upper parts of plants in coordination with the differentiation of BS and M cells, dimorphic chloroplasts, and enhancement of the photosynthetic enzymes’ expression and distribution. Our data suggest that, despite the independent origins and distinct photosynthetic structures, leaves of Kranz-type C4 species S. ferganica and SC-C4 species S. aralocaspica experienced similar base-to-tip transitions to form a C4 type in both structure and biochemistry. Such a phenomenon has been found in many different C4 species studied so far, including Kranz type, Kranz-like type, or SC-C4 species (Koteyeva et al., 2016). The representatives of Kranz-type C4 species from Poaceae have been reported to have M and BS cells that are differentiated along longitudinal gradients of leaf veins, accompanying with accumulation of enzymes or mRNAs for the C4 pathway (Langdale et al., 1988). In the C4 grass A. hirta (Poaceae), however, PEPC and RUBPC accumulation along the base-to-tip developmental gradient of leaves is not associated with veins (Wakayama et al., 2003). Different types of Kranz anatomy of C4 species in Chenopodiaceae (e.g. S. taxifolia, S. eltonica) and Cleomaceae (e.g. C. angustifolia) are differentiated basipetally and enhanced both in structure and biochemistry (Koteyeva et al., 2011a; Koteyeva et al., 2014). Besides developmental enhancement, in other C4 species, e.g. S. aralocaspica, at early developmental stage (i.e. seed germination), light can induce the transition of identical structure of plastids in the incipient chlorenchyma of cotyledons to form dimorphic chloroplasts, synthesize C4 enzymes, and generate structural and biochemical compartmentation, which ultimately leads to SC-C4 syndrome (Voznesenskaya et al., 2003). Such developmental or inducible enhancement of photosynthetic structure and biochemistry suggests that complete structural differentiation, in coordination with other C4 developmental processes, is essential for full C4 functions (Koteyeva et al., 2011a), which should have important biological and ecological significance in evolutionary processes and be a smart strategy in adaptation to harsh habitats.
A common feature of developmental enhancement of C4 syndrome is the differentiation of BS and M cells to form specialized functioning C4 photosynthetic tissues (Wakayama et al., 2003), therefore, the leaves located at different positions of the shoot may present distinct structure and biochemistry. For studying the progressive development of the C4 system, the leaf age (or size) and the distance to the leaf base are important (Koteyeva et al., 2011a). Leaf size less than 0.5–0.7 cm is suitable for observation of photosynthetic enhancement, while in fully expanded leaf (2–3 cm in S. taxifolia; 1.5–2 cm in S. aralocaspica) such phenomenon is not apparently present or has nearly disappeared (Koteyeva et al., 2011a; Koteyeva et al., 2016). In the present study, in S. ferganica, cell differentiation in distinct lengths (or ages) of leaves (e.g. 0.2 cm, 0.5 cm, 1.0 cm) and different positions on the shoot was diverse, 0.2 or 0.5 cm of leaf presented an enhanced development pattern along the longitudinal gradient; the differentiation degree in 0.2 cm leaf was relatively lower, in which the M cells were arranged neatly and compactly, and the BS cells were smaller, however, with the size increasing, the leaf around 1 cm was fully differentiated, both M and BS cells were significantly expanded, and BS cells were arranged from discontinuous to continuous, M cells were significantly elongated. So the leaf age (or size) is an important indicator for determination of developmental progression (Lara et al., 2008; Koteyeva et al., 2011a).
It has been suggested that biochemical compartmentation (e.g. PEPC accumulation in M cells) may serve as a developmental signal for structural differentiation (Dengler et al., 1995): such changes on photosynthetic enzymes should be regulated by relevant gene expression (Sheen, 1999). In classical C4 species (Kranz-anatomy), e.g. maize, corresponding to the progressive development of leaf structure, the majority of the photosynthetic genes are up-regulated in the tip of the leaf, which may be related to chloroplast differentiation or photosynthetic strategy (Cahoon et al., 2008). In SC-C4 species of B. sinuspersici, with the progressive transition from C3 mode to specialized functions of a C4 system, various photosynthetic genes are up-regulated significantly corresponding to the developmental enhancement in structure and biochemistry from the bottom to tip of the leaf (Lara et al., 2008). In the present study, we found that the transcripts of RUBPC, PEPC, and PPDK in S. ferganica or S. aralocaspica were accumulated from the lower to upper part of plant or the bottom to tip section of a young leaf, which was well-matched with the progressive enhancement of photosynthetic structure and biochemistry. In addition, we distinguished the expression pattern between PEPC1 and PEPC2 in S. ferganica or S. aralocaspica (Cheng et al., 2016), compared to the increase of PEPC1, PEPC2 altered in a limited range with the developmental enhancement of the C4 system. PEPC1 and PEPC2 encode two isoenzymes functioning in the phosphoenolpyruvate shuttle (O’Leary et al., 2011). It has been reported that PEPC1 regulates the carbon flux and lipid accumulation in the cell (Deng et al., 2014), while PEPC2 negatively affects intracellular lipid accumulation in Chlamydomonas reinhardtii (Deng et al., 2011). Previous work in S. aralocaspica revealed that two types of PEPC exhibited different expression patterns in response to various stresses (Cheng et al., 2016). In tomato (Solanum lycopersicum) fruit development, both PEPC1 and PEPC2 are regulated; however, under salt stress, the latter is up-regulated while the former gives no response (Yin et al., 2010). PEPC (1/2) from endosperm of castor seed is involved in fatty acid synthesis and the following malate production in leucoplasts (Blonde, 2003). So far, few studies have specialized on analyses between PEPC1 and PEPC2 and their impact on gradient development of photosynthetic structure. In maize, the tip-base ratio of PEPC1 transcripts in leaf is 68 times (Cahoon et al., 2008). In SC-C4 species of B. sinuspersici, a similar expression trend is observed (Lara et al., 2008). PEPC1 and PEPC2 perform different functions; however, which type contributes more in CO2 fixation still needs further evidence to clarify this point. Taken together, our data support the positive correlation between photosynthetic gene expression pattern and structural and biochemical enhancement of C4 syndrome in S. ferganica and S. aralocaspica.
The completion of the biochemical enhancement of C4 photosynthesis has to rely on the translational expression of relevant genes. A basipetal developmental progression of protein accumulation has been revealed in SC-C4 species of B. sinuspersici (Lara et al., 2008; Koteyeva et al., 2016), B. cycloptera (Voznesenskaya et al., 2005), and S. aralocaspica (Voznesenskaya et al., 2003; Koteyeva et al., 2016), in which Rubisco (rbcL) was accumulated earlier than other C4 enzymes in very young leaves, followed by a great increase of both PEPC and RUBPC activity in mature leaves (Koteyeva et al., 2014). In the present study, a gradient development in cellular differentiation and biochemical enhancement (e.g. rising expression of C4 photosynthetic enzymes) from the bottom to the tip of young leaves was also found in S. ferganica (Kranz anatomy). Analysis of the expression of proteins associated with C4 photosynthesis in S. ferganica showed that the related enzymes were large accumulated with the leaf progressive development both in different layers of the plant and along the leaf longitudinal gradient, in which a substantial RUBPC was present at the bottom of intermediate leaf (IL, 0.5–0.6 cm) during early development, meanwhile, a lag in the accumulation of C4 enzymes also existed, especially the PPDK, which might be a rate-limiting step for the developing C4 syndrome at an early stage (Lara et al., 2008; Koteyeva et al., 2011a; Koteyeva et al., 2014; Koteyeva et al., 2016). With the progression of structural differentiation and gene/protein expression, leaves located on different parts of the plant are driven to a more advanced developmental stage and proceed to a final mature C4 syndrome (Lara et al., 2008; Koteyeva et al., 2011a; Koteyeva et al., 2016).
Conclusions
In the present study, we revealed the progressive development and enhancement of photosynthetic structure and biochemistry for C4 syndrome in a Kranz-anatomy species S. ferganica. Compared to SC-C4 species S. aralocaspica in our experiment, S. ferganica behaved as a non-typical C4 species in photosynthetic physiology, e.g. flexible δ13C value, lower PEPC activity, insensitive response to light intensity, etc. It is well-known that C4 plants are evolved for adaptation to harsh habitats (Su et al., 2012). The C4 assimilation pathway is a complicated system which has evolved across a long process and accumulated a variety of natural variations in anatomy and biochemistry related to the ancestral C3 forms (Sage et al., 2012; Heckmann et al., 2013; Covshoff et al., 2014), it means that different C4 types with different photosynthetic anatomy and physiology may survive diverse disasters in evolutionary processes. In the present study, on photosynthetic physiological characteristics, S. aralocaspica was stable while S. ferganica was more flexible in response to heterogeneous habitats: it has been suggested that the biochemical compartmentation in SC-C4 species is more advanced organization mode (Sage, 2002; Koteyeva et al., 2016). All these changes and differences among C4 species may represent different evolutionary steps for carbon assimilation pathways. Our findings revealed that both C4 species in this study shared similar developmental enhancement in their C4 system differentiation, and the completion of this system is essential for optimal practice of C4 photosynthesis (Voznesenskaya et al., 2003). Our results should contribute to further understanding of the C4 photosynthetic pathway in response to environmental variation. However, what the differences in photosynthetic physiology mean to C4 system evolution between these two C4 species needs more experimental evidence to elucidate.
Data Availability Statement
All datasets generated for this study are included in the article/Supplementary Material.
Author Contributions
HL, YL, and TM designed the experiments and methodology. YL and HL wrote the manuscript. YL, TM, and JZ conducted the experiments and collected the data. YL, TM, JZ, and YM analyzed the data. All authors contributed critically to the manuscript and gave final approval for publication.
Funding
National Natural Science Foundation of China (31960037, 31660068); Open Funding of Key Laboratory of Xinjiang Uygur Autonomous Region (2016D03015).
Conflict of Interest
The authors declare that the research was conducted in the absence of any commercial or financial relationships that could be construed as a potential conflict of interest.
Acknowledgments
The authors thank the reviewers and all of the editors for their helpful comments and suggestions on this paper.
Supplementary Material
The Supplementary Material for this article can be found online at: https://www.frontiersin.org/articles/10.3389/fpls.2020.00152/full#supplementary-material
Supplementary Figure 1 | Schematic diagram of location and position of cotyledon and true leaf of S. ferganica and S. aralocaspica. (A) S. ferganica; (B) S. aralocaspica. 0.5–0.6: 0.5–0.6 cm leaf; Upper: 1.3–1.5 cm leaf in A, 1.8–1.9 cm in B; Lower: 1.0 cm leaf in A, 2.0 cm in B. The image was taken from indoor plant. Scale bar is 1 cm.
Supplementary Figure 2 | The developmental stage and morphology of S. ferganica in natural habitats. (A) Early seedling with two cotyledons only; (B) Seedling developed with small leaves covered with white, thick, long, and soft trichomes; (C) Plant with many branches and leaves covered with white, thick, long, and soft trichomes; (D) Plant initiates blossom covered with much thinner and shorter trichomes on leaf; (E) Plant at full blossom stage.
References
Akhani, H., Trimborn, P., Ziegler, H. (1997). Photosynthetic pathways in Chenopodiaceae from Africa, Asia and Europe with their ecological, phytogeographical and taxonomical importance. Plant Syst. Evol. 206, 187–221. doi: 10.1007/bf00987948
Bender, M. M., Rouhani, I., Vines, H. M., Black, C. C. (1973). 13C/12C ratio changes in Crassulaceae acid metabolism. Plant Physiol. 52, 427–430. doi: 10.1104/pp.52.5.427
Blonde, J. D. (2003). Structural and kinetic properties of high and low molecular mass phosphoenolpyruvate carboxylase isoforms from the endosperm of developing castor oilseeds. J. Biol. Chem. 278, 11867–11873. doi: 10.1074/jbc.M211269200
Bongard-Pierce, D. K., Evans, M. M. S., Poethig, R. S. (1996). Heteroblastic features of leaf anatomy in maize and their genetic regulation. Int. J. Plant Sci. 157, 331–340. doi: 10.1086/297353
Cahoon, A. B., Takacs, E. M., Sharpe, R. M., Stern, D. B. (2008). Nuclear, chloroplast, and mitochondrial transcript abundance along a maize leaf developmental gradient. Plant Mol. Biol. 66, 33–46. doi: 10.1007/s11103-007-9250-z
Cao, J., Lv, X. Y., Chen, L., Xing, J. J., Lan, H. Y. (2015). Effects of salinity on the growth, physiology and relevant gene expression of an annual halophyte grown from heteromorphic seeds. Aob Plants 7, plv112. doi: 10.1093/aobpla/plv112
Carmosilva, A. E., Silva, A. B. D., Keys, A. J., Parry, M. A. J., Arrabaca, M. C. (2008). The activities of PEP carboxylase and the C4 acid decarboxylases are little changed by drought stress in three C4 grasses of different subtypes. Photosynth. Res. 97, 223–233. doi: 10.1007/s11120-008-9329-7
Chen, T., Fen, H. Y., Xu, S. J., Qiang, W. Y., An, L. Z. (2002). Stable carbon isotope composition of desert plant leaves and water-use efficiency. J. Desert Res. 22, 288–291. doi: 10.1088/1009-1963/11/5/313
Cheng, G., Wang, L., Lan, H. Y. (2016). Cloning of PEPC-1 from a C4 halophyte Suaeda aralocaspica without Kranz anatomy and its recombinant enzymatic activity in responses to abiotic stresses. Enzyme Microb. Tech. 83, 57–67. doi: 10.1016/j.enzmictec.2015.11.006
Covshoff, S., Burgess, S. J., Kneřová, J., Kümpers, B. M. C. (2014). Getting the most out of natural variation in C4 photosynthesis. Photosynth. Res. 119, 157–167. doi: 10.1007/s11120-013-9872-8
Crespo, H. M., Frean, M., Cresswell, C. F., Tew, J. (1979). The occurrence of both C3 and C4 photosynthetic characteristics in a single Zea mays plant. Planta 147, 257–263. doi: 10.2307/23374366
Cushman, J. C., Borland, A. M. (2002). Induction of crassulacean acid metabolism by water limitation. Plant Cell Environ. 25, 295–310. doi: 10.1007/0-306-48155-3_17
Deng, X. D., Li, Y. J., Fei, X. W. (2011). The mRNA abundance of pepc2 gene is negatively correlated with oil content in Chlamydomonas reinhardtii. Biomass Bioenerg. 35, 1811–1817. doi: 10.1016/j.biombioe.2011.01.005
Deng, X., Cai, J., Li, Y., Fei, X. W. (2014). Expression and knockdown of the PEPC1 gene affect carbon flux in the biosynthesis of triacylglycerols by the green alga Chlamydomonas reinhardtii. Biotechnol. Lett. 36, 2199–2208. doi: 10.1007/s10529-014-1593-3
Dengler, N. G., Dengler, R. E., Donnelly, P. M., Filosa, M. F. (1995). Expression of the C4 pattern of photosynthetic enzyme accumulation during leaf development in Atriplex rosea (Chenopodiaceae). Am. J. Bot. 82, 318–327. doi: 10.2307/2445577
Dizengremel, P., Thiec, D. L., Hasenfratz-Sauder, M. P., Vaultier, M. N., Bagard, M., Jolivet, Y. (2009). Metabolic-dependent changes in plant cell redox power after ozone exposure. Plant Biol. 11, 35–42. doi: 10.1111/j.1438-8677.2009.00261.x
Edwards, G. E., Sheta, E., Moore, B. D., Dai, Z. Y., Franceschi, V. R., Cheng, S. H., et al. (1990). Photosynthetic characteristics of cassava (Manihot esculenta Crantz), a C3 species with chlorenchymatous bundle sheath cells. Plant Cell Physiol. 31, 1199–1206. doi: 10.1094/Phyto-80-1469
Erlinghaeuser, M., Hagenau, L., Wimmer, D., Offermann, S. (2016). Development, subcellular positioning and selective protein accumulation in the dimorphic chloroplasts of single-cell C4 species. Curr. Opin. Plant Biol. 31, 76–82. doi: 10.1016/j.pbi.2016.03.017
Fontaine, V., Cabane, M., Dizengremel, P. (2003). Regulation of phosphoenolpyruvate carboxylase in Pinus halepensis needles submitted to ozone and water stress. Physiol. Plantarum. 117, 445–452. doi: 10.1034/j.1399-3054.2003.00052.x
Freitag, H., Stichler, W. (2000). A remarkable new leaf type with unusual photosynthetic tissue in a central Asiatic genus of Chenopodiaceae. Plant Biol. 2, 154–160. doi: 10.1055/s-2000-9462
Gamalei, Y. V., Voznesenskaya, E. V. (1986). Structural-biochemical types of C4 plants. Soviet. Plant Physiol. 33, 616–630. doi: 10.1016/0034-6667(86)90064-3
Hatch, M. D. (1987). C4 photosynthesis: a unique blend of modified biochemistry, anatomy and ultrastructure. BBA 895, 81–106. doi: 10.1016/S0304-4173(87)80009-5
Heckmann, D., Schulze, S., Denton, A., Gowik, U., Westhoff, P., Weber, A. P. M., et al. (2013). Predicting C4 photosynthesis evolution: modular, individually adaptive steps on a Mount Fuji fitness landscape. Cell 153, 1579–1588. doi: 10.1016/j.cell.2013.04.058
Hoagland, D. R., Arnon, D. S. (1950). The water culture method for growing plants without soil. Cal. Agric. Exp. Stn. Circ. 347, 1–32. doi: 10.1016/S0140-6736(00)73482-9
Huang, D. H., Wang, C., Yuan, J. W., Cao, J., Lan, H. Y. (2015). Differentiation of the seed coat and composition of the mucilage of Lepidium perfoliatum L.: a desert annual with typical myxospermy. Acta Bioch. Bioph. Sin. 47, 775–787. doi: 10.1093/abbs/gmv078
Huang, J. H. (2005). Geographical Distribution of Salsola L. in China, Arid Land. Geography 28, 325–329. doi: 10.1080/02533839.2005.9671036
Kadereit, G., Borsch, T., Weising, K., Freitag, H. (2003). Phylogeny of Amaranthaceae and Chenopodiaceae and the evolution of C4 photosynthesis. Int. J. Plant Sci. 164, 959–986. doi: 10.1086/378649
Kalt-Torres, W., Kerr, P. S., Usuda, H., Huber, S. C. (1987). Diurnal changes in maize leaf photosynthesis I. carbon exchange rate, assimilate export rate, and enzyme activities. Plant Physiol. 83, 283–288. doi: 10.2307/4270412
Karabourniotis, G., Manetas, Y., Gavalas, N. A. (1985). Detecting photoactivation of phosphoenolpyruvate carboxylase in C4 plants: an effect of pH. Plant Physiol. 77, 300–302. doi: 10.1104/pp.77.2.300
Khoshravesh, R., Stinson, C. R., Stata, M., Busch, F. A., Sage, R. F., Ludwig, M., et al. (2016). C3-C4 intermediacy in grasses: organelle enrichment and distribution, glycine decarboxylase expression, and the rise of C2 photosynthesis. J. Exp. Bot. 67, 3065–3078. doi: 10.1093/jxb/erw150
Koteyeva, N. K., Voznesenskaya, E. V., Berry, J. O., Chuong, S. D. X., Franceschi., V. R., Edwardset, G. E. (2011a). Development of structural and biochemical characteristics of C4 photosynthesis in two types of Kranz anatomy in genus Suaeda (family Chenopodiaceae). J. Exp. Bot. 62, 3197–3212. doi: 10.1093/jxb/err021
Koteyeva, N. K., Voznesenskaya, E. V., Roalson, E. H., Edwards, G. E. (2011b). Diversity in forms of C4 in the genus Cleome (Cleomaceae). Ann. Bot-London. 107, 269–283. doi: 10.1093/aob/mcq239
Koteyeva, N. K., Voznesenskaya, E. V., Cousins, A. B., Edwards, G. E. (2014). Differentiation of C4 photosynthesis along a leaf developmental gradient in two Cleome species having different forms of Kranz anatomy. J. Exp. Bot. 65, 3525–3541. doi: 10.1093/jxb/eru042
Koteyeva, N. K., Voznesenskaya, E. V., Berry, J. O., Cousins, A. B., Edwards, G. E. (2016). The unique structural and biochemical development of single cell C4 photosynthesis along longitudinal leaf gradients in Bienertia sinuspersici and Suaeda aralocaspica (Chenopodiaceae). J. Exp. Bot. 67, 2587–2601. doi: 10.1093/jxb/erw082
Ku, M. S. B., Schmitt, M. R., Edwards, G. E. (1979). Quantitative determination of RuBP carboxylas-oxygenase protein in leaves of several C3 and C4 plants. J. Exp. Bot. 30, 89–98. doi: 10.1093/jxb/30.1.89
Ku, M. S. B., Monson, R. K., Littlejohn, R. O., Nakamoto, H., Fisher, D. B., Edwards, G. E. (1983). Photosynthetic characteristics of C3-C4 intermediate flaveria species I. leaf anatomy, photosynthetic responses to O2 and CO2, and activities of key enzymes in the C3 and C4 pathways. Plant Physiol. 71, 944–948. doi: 10.1104/pp.71.4.944
Laing, W. A., Ogren, W. L., Hageman, R. H. (1974). Regulation of soybean net photosynthetic CO2 fixation by the interaction of CO2, O2, and ribulose 1,5-diphosphate carboxylase. Plant Physiol. 54, 678–685. doi: 10.1104/pp.54.5.678
Langdale, J. A., Rothermel, B. A., Nelson, T. (1988). Cellular pattern of photosynthetic gene expression in developing maize leaves. Gene. Dev. 2, 106–115. doi: 10.1101/gad.2.1.106
Lara, M. V., Offermann, S., Smith, M., Okita, T. W., Andreo, C. S., Edward, G. E. (2008). Leaf development in the single-cell C4 system in Bienertia sinuspersici: expression of genes and peptide levels for C4 metabolism in relation to chlorenchyma structure under different light conditions. Plant Physiol. 148, 593–610. doi: 10.1104/pp.108.124008
Lung, S. C., Yanagisawa, M., Chuong, S. D. X. (2012). Recent progress in the single-cell C4 photosynthesis in terrestrial plants. Front. Biol. 7, 539–547. doi: 10.1007/s11515-012-9248-z
Ma, Y. L., Zhang, J. H., Li, X. R., Zhang, S. Y., Lan, H. Y. (2016). Effects of environmental stress on seed germination and seedling growth of Salsola ferganica (Chenopodiaceae). Acta Ecol. Sinica. 36, 456–463. doi: 10.1016/j.chnaes.2016.09.008
Ma, Y. L., Wang, J., Zhang, J. H., Zhang, S. Y., Liu, Y. X., Lan, H. Y. (2018). Seed heteromorphism and effects of light and abiotic stress on germination of a typical annual halophyte Salsola ferganica in cold desert. Front. Plant Sci. 8, 2257. doi: 10.3389/fpls.2017.02257
Markus, V., Lurie, S., Bravdo, B., Stevens, M. A., Rudich, J. (2006). High temperature effects on RuBP carboxylase and carbonic anhydrase activity in two tomato cultivars. Physiol. Plantarum. 53, 407–412. doi: 10.1111/j.1399-3054.1981.tb02723.x
Nomenclature committee of the international union of biochemistry (NC-IUB) (1979). Units of enzyme activity. Eur. J. Biochem. 97, 319–320. doi: 10.1111/j.1432-1033.1979.tb13116.x
O’Leary, B., Park, J., Plaxton, W. C. (2011). The remarkable diversity of plant PEPC (phosphoenolpyruvate carboxylase): recent insights into the physiological functions and post-translational controls of non-photosynthetic PEPCs. Biochem. J. 436, 15–34. doi: 10.1042/BJ20110078
Pan, J., Li, R., Hu, X. W. (2016). Effect of water conditions on carbon isotope composition photosynthesis and branch growth of Reaumuria soongorica. Acta Bot. Boreali-Occidentalia Sin. 6, 1190–1198. doi: 10.7606/j.issn.1000-4025.2016.06.1190
Peng, C. L., Lin, Z. F., Lin, G. Z. (1998). Diurnal changes of photooxidation response in leaves of C3 and C4 plants. J. Trop. Subtropical Bot. 6, 233–238. doi: 10.3969/j.issn.1005-3395.1998.3.010
Prakitchai, C., Alice, B., Wollman, F. A. (2016). Dynamics of chloroplast translation during chloroplast differentiation in maize. PloS Genet. 12, 1–28. doi: 10.1371/journal.pgen.1006106
Pyankov, V. I., Voznesenskaya, E. V., Kuzmin, A. N., Ku, M. S. B., Ganko, E., Franceschi, V. R., et al. (2000). Occurrence of C3 and C4 photosynthesis in cotyledons and leaves of Salsola species (Chenopodiaceae). Photosynth. Res. 63, 69–84. doi: 10.1023/a:1006377708156
Pyankov, V. I., Ziegler, H., Kuz’min, A., Edwards, G. E. (2001). Origin and evolution of C4 photosynthesis in the tribe Salsoleae (Chenopodiaceae) based on anatomical and biochemical types in leaves and cotyledons. Plant Syst. Evol. 230, 43–74. doi: 10.1007/s006060170004
Pyankov, V., Black, C., Stichler, W., Ziegler, H. (2010). Photosynthesis in Salsola species (Chenopodiaceae) from southern africa relative to their C4 syndrome origin and their african-asian arid zone migration pathways. Plant Biol. 4, 62–69. doi: 10.1055/s-2002-20437
Raghavendra, A. S., Das, V. S. R. (1993). “C4 photosynthesis and C3-C4 intermediacy: adaptive strategies for semiarid tropics,” in Photoreactions to Plant Productivity. eds. Abrol, P., Mohanty, V. S. R. Govindjee (Dordrecht, Netherlands: Springer) 317–338 doi: 10.1007/978-94-011-2708-0_12
Sage, R. F., Stata, M. (2015). Photosynthetic diversity meets biodiversity: the C4 plant example. J. Plant Physiol. 172, 104–119. doi: 10.1016/j.jplph.2014.07.024
Sage, R. F., Li, M., Monson, R. K. (1999). “The taxonomic distribution of C4 photosynthesis-C4 plant biology-16,” in C4 Plant Biol. eds. Sage, R. F., Monson, R. K. (San Diego, CA, USA: Academic Press) 551–584. doi: 10.1016/B978-012614440-6/50017-3
Sage, R. F., Sage, T. L., Kocacinar, F. (2012). Photorespiration and the evolution of C4 photosynthesis. Annu. Rev. Plant Bio. 63, 19–47. doi: 10.1146/annurev-arplant-042811-105511
Sage, R. F. (2002). C4 photosynthesis in terrestrial plants does not require Kranz anatomy. Trends Plant Sci. 7, 283–285. doi: 10.1016/s1360-1385(02)02293-8
Sage, R. F. (2004). The evolution of C4 photosynthesis. New Phytol. 161, 341–370. doi: 10.1111/j.1469-8137.2004.00974.x
Sascha, O., Thomas, W. O., Gerald, E. E. (2011). Resolving the compartmentation and function of C4 photosynthesis in the single-cell C4 species Bienertia sinuspersici. Plant Physiol. 155, 1612–1628. doi: 10.1104/pp.110.170381
Sheen, J. (1999). C4 gene expression. Annu. Rev. Plant Biol. 50, 187–217. doi: 10.1146/annurev.arplant.50.1.187
Shi, R., Chiang, V. L. (2005). Facile means for quantifying microRNA expression by real-time PCR. Biotechniques 39, 519–525. doi: 10.2144/000112010
Soros, C. L., Dengler, N. G. (2001). Ontogenetic derivation and cell differentiation in photosynthetic tissues of C3 and C4 Cyperaceae. Am. J. Bot. 88, 992–1005. doi: 10.2307/2657080
Su, P. X., Yan, Q. D., Xie, T. T., Zhou, Z. J., Gao, S. (2012). Associated growth of C3, and C4, desert plants helps the C3, species at the cost of the C4 species. Acta Physiol. Plant 34, 2057–2068. doi: 10.1007/s11738-012-1003-1
Ueno, O. (2005). Variation in the activity of some enzymes of photorespiratory metabolism in C4 grasses. Ann. Bot-London. 96, 863–869. doi: 10.1093/aob/mci238
Voznesenskaya, E. V., Franceschi, V. R., Pyankov, V. I., Edwards, G. E. (1999). Anatomy, chloroplast structure and compartmentation of enzymes relative to photosynthetic mechanisms in leaves and cotyledons of species in the tribe Salsoleae (Chenopodiaceae). J. Exp. Bot. 50, 1779–1795. doi: 10.1093/jxb/50.341.1779
Voznesenskaya, E. V., Franceschi, V. R., Kiirats, O., Freitag, H., Edwards, G. E. (2001a). Kranz anatomy is not essential for terrestrial C4 plant photosynthesis. Nature 414, 543–546. doi: 10.1038/35107073
Voznesenskaya, E. V., Artyusheva, E. G., Franceschi, V. R., Pyankov, V. I., Kiirats, O. (2001b). Salsola arbusculiformis, a C3-C4 intermediate in Salsoleae (Chenopodiaceae). Ann. Bot-London. 88, 337–348. doi: 10.1006/anbo.2001.1457
Voznesenskaya, E. V., Franceschi, V. R., Kiirats, O., Artyusheva, E. G., Freitag, H., Edwards, G. E. (2002). Proof of C4 photosynthesis without Kranz anatomy in Bienertia cycloptera (Chenopodiaceae). Plant J. 31, 649–662. doi: 10.1046/j.1365-313X.2002.01385.x
Voznesenskaya, E. V., Edwards, G. E., Kiirats, O., Artyusheva, E. G., Franceschi, V. R. (2003). Development of biochemical specialization and organelle partitioning in the single celled C4 system in leaves of Borszczowia aralocaspica (Chenopodiaceae). Am. J. Bot. 90, 1669–1680. doi: 10.3732/ajb.90.12.1669
Voznesenskaya, E. V., Koteyeva, N. K., Choung, S. D., Akhani, H., Edwards, G. E., Franceschi, V. (2005). Differentiation of cellular and biochemical features of the single-cell C4 syndrome during leaf development in Bienertia cycloptera (Chenopodiaceae). Am. J. Bot. 92, 1784–1795. doi: 10.2307/4125508
Voznesenskaya, E. V., Koteyeva, N. K., Akhani, H., Roalson, E. H., Edwards, G. E. (2013). Structural and physiological analyses in Salsoleae (Chenopodiaceae) indicate multiple transitions among C3, intermediate, and C4 photosynthesis. J. Exp. Bot. 64, 3583–3604. doi: 10.1093/jxb/ert191
Wakayama, M., Ueno, O., Ohnishi, J. (2003). Photosynthetic enzyme accumulation during leaf development of Arundinella hirta, a C4 grass having Kranz cells not associated with vascular tissues. Plant Cell Physiol. 44, 1330–1340. doi: 10.1093/pcp/pcg159
Wei, Y., Dong, M., Huang, Z. Y., Tan, D. Y. (2008). Factors influencing seed germination of Salsola affinis (Chenopodiaceae), a dominant annual halophyte inhabiting the deserts of Xinjiang, China. Flora 203, 134–140. doi: 10.1016/j.flora.2007.02.003
Weise, S. E., Van Wijk, K. J., Sharkey, T. D. (2011). The role of transitory starch in C3, CAM, and C4 metabolism and opportunities for engineering leaf starch accumulation. J. Exp. Bot. 62, 3109–3118. doi: 10.1093/jxb/err035
Wen, Z. B., Zhang, M. L. (2011). Anatomical types of leaves and assimilating shoots and carbon 13C/12C isotope fractionation in Chinese representatives of Salsoleae s.l. (Chenopodiaceae). Flora 206, 720–730. doi: 10.1016/j.flora.2010.11.015
Wen, Z. B., Zhang, M. L. (2015). Salsola laricifolia, another C3-C4 intermediate species in tribe Salsoleae s.l. (Chenopodiaceae). Photosynth. Res. 123, 33–43. doi: 10.1007/s11120-014-0037-1
Wen, Z. B., Zhang, M. L., Zhu, G. L., Sanderson, S. C. (2010). Phylogeny of Salsoleae s.l. (Chenopodiaceae) based on DNA sequence data from ITS, psbB-psbH, andrbcL, with emphasis on taxa of northwestern China. Plant Syst. Evol. 288, 25–42. doi: 10.1007/s00606-010-0310-5
Weng, X. Y., Jiang, D. A., Lu, Q. (1999). Influence of enzymes and relatives factors on diurnal variation of photosynthetic rate in rice. J. Biomath. 14, 495–500. doi: CNKI:SUN:SWSX.0.1999-04-019
Yin, Y. G., Tominaga, T., Iijima, Y., Aoki, K., Shibata, D., Ashihara, H., et al. (2010). Metabolic alterations in organic acids and γ-aminobutyric acid in developing tomato (Solanum lycopersicum L.) fruits. Plant Cell Physiol. 51, 1300–1314. doi: 10.1093/pcp/pcq090
Keywords: C4 photosynthetic system, developmental enhancement, Kranz-type anatomy, Salsola ferganica, Suaeda aralocaspica
Citation: Liu Y, Maimaitijiang T, Zhang J, Ma Y and Lan H (2020) The Developmental Enhancement of a C4 System With Non-Typical C4 Physiological Characteristics in Salsola ferganica (Kranz Anatomy), an Annual Desert Halophyte. Front. Plant Sci. 11:152. doi: 10.3389/fpls.2020.00152
Received: 30 July 2019; Accepted: 31 January 2020;
Published: 06 March 2020.
Edited by:
Julian Eaton-Rye, University of Otago, New ZealandReviewed by:
Hongchang Cui, Florida State University, United StatesSusanne Hoffmann-Benning, Michigan State University, United States
Copyright © 2020 Liu, Maimaitijiang, Zhang, Ma and Lan. This is an open-access article distributed under the terms of the Creative Commons Attribution License (CC BY). The use, distribution or reproduction in other forums is permitted, provided the original author(s) and the copyright owner(s) are credited and that the original publication in this journal is cited, in accordance with accepted academic practice. No use, distribution or reproduction is permitted which does not comply with these terms.
*Correspondence: Haiyan Lan, bGFuaGFpeWFuQHhqdS5lZHUuY24=
†These authors have contributed equally to this work