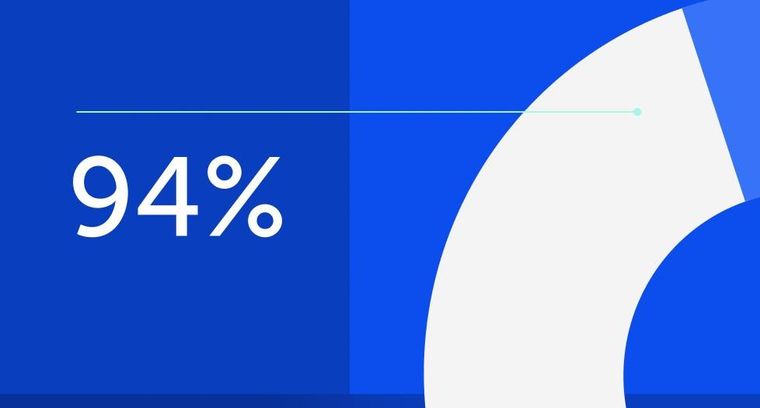
94% of researchers rate our articles as excellent or good
Learn more about the work of our research integrity team to safeguard the quality of each article we publish.
Find out more
ORIGINAL RESEARCH article
Front. Plant Sci., 14 February 2020
Sec. Plant Abiotic Stress
Volume 10 - 2019 | https://doi.org/10.3389/fpls.2019.01752
Abscisic acid-, stress-, and ripening-induced (ASR) genes are involved in responding to abiotic stresses, but their precise roles in enhancing grain yield under stress conditions remain to be determined. We cloned a rice (Oryza sativa) ASR gene, OsASR1, and characterized its function in rice plants. OsASR1 expression was induced by abscisic acid (ABA), salt, and drought treatments. Transgenic rice plants overexpressing OsASR1 displayed improved water regulation under salt and drought stresses, which was associated with osmolyte accumulation, improved modulation of stomatal closure, and reduced transpiration rates. OsASR1-overexpressing plants were hypersensitive to exogenous ABA and accumulated higher endogenous ABA levels under salt and drought stresses, indicating that OsASR1 is a positive regulator of the ABA signaling pathway. The growth of OsASR1-overexpressing plants was superior to that of wild-type (WT) plants under paddy field conditions when irrigation was withheld, likely due to improved modulation of stomatal closure via modified ABA signaling. The transgenic plants had higher grain yields than WT plants for four consecutive generations. We conclude that OsASR1 has a crucial role in ABA-mediated regulation of stomatal closure to conserve water under salt- and drought-stress conditions, and OsASR1 overexpression can enhance salinity and drought tolerance, resulting in improved crop yields.
The continuous use of irrigation systems and increasingly frequent dry periods lead to the simultaneous occurrence of salinity and drought on arable land around the world (Hu and Schmidhalter, 2005). Both salinity and drought are significant plant stressors with major impacts on plant development and grain yield, causing economic losses in agricultural production (Flowers, 2004; Godfray et al., 2010). Much research is focused on improving agricultural production and developing stress-tolerant crops, but current efforts still fail to meet the demands of food security in the face of global population growth, global warming, water shortages, and soil salinization (Hu and Xiong, 2014).
Plants have evolved numerous mechanisms to adapt to salinity and drought, such as stomatal adjustment, osmoregulation, selective uptake, and compartmentation of ions (Blumwald, 2000). These physiological responses are primarily regulated by the plant stress hormone abscisic acid (ABA) (Verma et al., 2016). ABA accumulates rapidly in response to salinity and drought conditions and mediates numerous responses that help plants survive the stresses. ABA regulates guard cells to maintain their water content under osmotic stress and induces the expression of genes encoding proteins involved in cell dehydration resistance, including late embryogenesis abundant (LEA) proteins, water transporters, protein kinases, protein phosphatases, transcription factors, and enzymes involved in osmolyte synthesis (Fujita et al., 2011; Golldack et al., 2014).
ABA-, stress-, and ripening-induced (ASR) proteins were first reported in tomato (Solanum lycopersicum L.) (Iusem et al., 1993). These plant-specific, small, and hydrophilic proteins are induced by ABA during drought stress and ripening. Subsequently, ASR genes have been identified in various monocot (Riccardi et al., 1998; Vaidyanathan et al., 1999), dicot (Van Berkel et al., 1994; Hong et al., 2002), and gymnosperm (Shen et al., 2005) species. In rice, there are six ASR genes located in four different chromosomes. These genes are thought to arise from tandem and whole genome replication based on their location on the chromosome and gene sequence similarities (Philippe et al., 2010). The ASR protein structure consists of a short N-terminal consensus sequence comprising six to seven His residues that might constitute a Zn-binding site and a DNA-binding site containing about 70 amino acids with a highly conserved ABA/WDS domain (Cakir et al., 2003; Henry et al., 2011; Jia et al., 2016) in the C-terminal region.
Recent studies report that some ASR genes are located in the nucleus and encode transcription factors, regulating gene expression during stress responses (Cakir et al., 2003; Hu et al., 2013; Dominguez and Carrari, 2015). ASR proteins, such as the lily pollen ASR and the rice ASR5, have been localized in both nuclei and cytoplasm (Wang et al., 2005; Konrad and Bar-Zvi, 2008; Arenhart et al., 2013). These studies suggest that ASR proteins may affect transcriptional modulation. Although the exact physiological role of ASR proteins is unknown, they are presumed to have functional duality in plants. A previous study suggested that ASR proteins may serve as a downstream component of the signal transduction cascade involved in plant cell responses to various stressors (Maskin et al., 2001). ASR proteins also participate in mediating plant developmental processes, including senescence, fruit ripening, and pollen maturation (Dominguez and Carrari, 2015). Many ASR genes respond to ABA and abiotic stresses, such as drought, salt, cold, and limited light (Hong et al., 2002; Jeanneau et al., 2002; Yang et al., 2005; Liu et al., 2010; Hu et al., 2013; Golan et al., 2014; Wang et al., 2016b). The role of ASR proteins in drought-stress tolerance was first reported in lily through the LLA23 gene, which encodes an ASR protein. Overexpression of lily ASR conferred drought and salt tolerance to Arabidopsis thaliana by increasing the expression of ABA/stress-regulated genes (Yang et al., 2005). Transgenic tobacco plants overexpressing the ASR gene of brome grass (Brachypodium distachyon, BdASR1) or wheat (Triticum aestivum, TaASR1) exhibited improved tolerance to drought stress (Hu et al., 2013; Wang et al., 2016b). Recombinant Escherichia coli cells expressing the ZnAsr1 gene from Ziziphus nummularia displayed enhanced tolerance to drought stress induced by polyethylene glycol (PEG) (Padaria et al., 2016). Transgenic rice plants overexpressing OsASR1 or OsASR3 display enhanced photosynthetic efficiency under cold and drought stress compared to controls (Joo et al., 2013). Although ASR genes were first identified more than 20 years ago and respond to a variety of abiotic stresses, a complete map of their molecular and physiological functions under salinity and drought stress is still lacking. The majority of studies on ASR genes were performed under controlled conditions in laboratories and greenhouses. There are few reports on the role of ASR genes in salinity and drought tolerance or crop yield in natural fields, although research using these approaches is in progress (Qin et al., 2016).
This study builds on our previous work wherein we found that the ASR1 gene of rice confers stress resistance to yeast cells by promoting scavenging of reactive-oxygen species (ROS) and performing chaperone-like activities (Kim et al., 2012). Here, we report on the role of the rice OsASR1 gene in salinity and drought tolerance and crop grain yield in laboratory and natural paddy field conditions using OsASR1-overexpressing rice plants. Our results show that rice OsASR1 is a promising candidate for transgenic breeding of plants with enhanced resistance to both salinity and drought, thereby reducing the requirements for irrigation and improving crop yield.
Total RNA was extracted from Oryza sativa L. japonica (O. sativa) leaves using an RNeasy Plant Mini Kit (Qiagen, Valencia, CA, USA), and then cDNA was synthesized using the SuperScript III First-Strand Synthesis System (Life Technologies). The regions encoding the OsASR1 cDNA (GenBank accession no. AK062319) were amplified by PCR using Ex Taq polymerase (TaKaRa, Tokyo, Japan) with a specific primer set (OsASR1-FC and OsASR1-RC; Supplementary Table S2). The amplified regions were cloned into the pENTR/SD/D-TOPO vector (Invitrogen, Carlsbad, CA, USA), and then the LR recombination reaction was performed with a pGA2897 binary vector modified from pGA1611.
The plasmid harboring the cloned gene under control of the maize ubiquitin promoter was introduced into Agrobacterium strain LBA 4404 and used to transform rice calli produced from scutella of mature seeds of Oryza sativa cv. Ilmi. The method used for Agrobacterium-mediated rice transformation is described below and was based on that of Hiei et al., 1994. A. tumefaciens strain LBA4404 was grown for 3 d on Agrobacterium minimal (AB) medium containing 50 mg·L-1 hygromycin. Cells were collected by centrifugation at 2,560 rpm for 30 min and resuspended in AAM medium at a density of 3–5 × 109 cells·mL-1. The rice calli described above were immersed in this bacterial suspension for 10–20 min and incubated at 25°C in the dark for 3 d. After co-cultivation, the materials were washed four times with 250 mg·L-1 cefotaxime in sterile water and cultured in 2N6-CH medium for 3 weeks. A white callus proliferated in the first selection medium. The callus was cultured on N6-7-CH medium for 10 d and incubated in a regeneration medium (N6S3-CH) at 25°C under continuous illumination (1500 lux). Regenerated plants were eventually transferred to soil in pots and grown in a greenhouse maintained at 30 ± 4°C during the day and 24 ± 2°C during the night with relative humidity of 60–70%.
Approximately 20 independent T0 transgenic rice plants were screened using hygromycin. Among the transformants, 16 independent T1 transgenic plants grown from T1 seeds were selected using hygromycin and PCR genotyping. Four independent, homozygous T2 plants were identified using the same process, which we refer to as TR1, TR2, TR3, and TR4.
We used the adapter ligation PCR method to isolate flanking sequences of T-DNA. First, 0.5 µg genomic DNA were digested with 2 U of HpaII restriction enzyme and ligated with 5 U of T4 DNA ligase (Takara, Shiga, Japan) in a 20-µL reaction volume. The reaction mixture, containing T4 DNA ligase buffer and 50 pmol of the adapters, was incubated at 37°C for 1 h. The first PCR was conducted in 20 µL reaction volume containing PCR pre-mix, 0.5 pmol each primer (Ada1 and LB1 or RB), and 1 µL digestion or ligation product. PCR was performed in a PTC-200 thermal cycler (MJ Research, Watertown, MA, USA) and conducted using the primers Ada2 and LB2 or RB2 as follows: first denaturation step at 95°C for 5 min, 20 cycles of 30 s at 94°C and 1 min at 72°C, followed by a final elongation step at 72°C for 10 min. The second PCR was conducted with 5 µL of the first PCR product under the conditions of first denaturation step at 94°C for 5 min, 40 cycles of 30 s at 94°C, 30 s at 60°C, and 1 min at 72°C, followed by a final elongation step at 72°C for 10 min. Amplified products were loaded onto a 1% agarose gel. The PCR products were purified using the HiYield™ Gel/PCR DNA Extraction Kit (Real Biotech Corporation, Taiwan) and sequenced by ABI3730XL (Applied Biosystems, Foster City, CA, USA) using LB2 or RB2 primer (Lim et al., 2016).
Wild-type rice seeds or seeds from the transgenic plants were germinated for 6 d in a growth chamber at 28°C after which the seedlings were transplanted into nursery bed soil (Pungnong, Seoul, South Korea) in plastic pots (10 cm diameter, 9 cm height) at 85% soil field capacity and grown in a greenhouse maintained at 30 ± 4°C during the day and 24 ± 2°C during the night with relative humidity of 60–70% until use. Leaves, roots, and stems were sampled at vegetative and reproductive stages for analysis of OsASR1 expression. The reproductive stage was identified as the first appearance of the inflorescence meristem. Rice embryo samples were isolated from mature seeds.
The stress treatment was conducted in greenhouse conditions maintained at 30 ± 4°C during the day and 24 ± 2°C during the night with relative humidity of 60–70% and average 1200 µmol m−2 s−1 photosynthetic photon flux density (PPFD) in July (15-h light/9-h dark cycles) until use. The PPFD in greenhouse was measured at 12pm. Expression of OsASR1 was analyzed in four-week-old plants exposed to several types of stress. ABA and salt stress were imposed by soaking the plants grown in pots with soil in 100 μM ABA or 200 mM NaCl solution, respectively. Drought stress was imposed by withholding water. Leaves and roots were sampled at 0, 1, 3, 6, 12, or 24 h after imposition of stress.
For salt stress phenotype analysis, four-week-old OsASR1-overexpressing transgenic and WT plants transplanted into soil in pots were soaked in 200 mM NaCl solution for 7 d, and then allowed to recover for 5 d. The number of surviving plants were counted for each line. The drought-stress phenotype was assessed in four-week-old plants treated as follows: plants were not watered for 3 d. After 3 days of exposure to water-free conditions, the soil capacity was 35 ± 5%, and then plants were recovered with daily watering for the next 7 days. The survival percentage was then assessed.
All tests were repeated at least three times.
Total RNA was extracted using TRIzol reagent (Takara) according to the manufacturer's instructions, and reverse transcription reactions were performed with 2 μg total RNA using the SuperScript III First-Strand Synthesis System. Expression of genes after various treatments in rice were analyzed by qRT-PCR. The qRT-PCR analysis was performed using the TB Green Premix Ex Taq II (Tli RNaseH Plus) Kit (TaKaRa) and QuantStudio3 Real-Time PCR system (Applied Biosystems, Foster City, CA, USA). The 20-μl reactions contained 12.5 μl of TB Green Premix Ex Taq II (Tli RNaseH Plus), 0.4 μl of ROX Reference Dye II, 200 ng of cDNA template, 0.4 μl of each primer (10 μM), and 6.26 μl of PCR‐grade water. All samples were analyzed in biological triplicates as follows: initial preheating at 95°C for 30 s, followed by 40 cycles of 95°C for 3 s and 60°C for 30 s. The rice tubulin (Tub) gene was used as a positive control. Fold changes in mRNA were calculated relative to the calibrator. The level of OsASR1 expression in Figure 1 is presented relative to that in WT leaves (which were assigned an expression level of 1) under normal condition. Normalization and relative quantification were conducted using the 2-∆∆Ct method (Livak and Schmittgen, 2001). The primers used for qRT-PCR analysis are listed in the Supplementary Table S2.
Figure 1 Expression analysis of the OsASR1 gene and generation of OsASR1-overexpressing transgenic rice plants. (A) qRT-PCR analysis of expression of OsASR1 levels in different organs of WT plants. OsASR1 expression level in leaves of vegetative growth stage was considered to be 1; described as relative values. (B) Stress-inducible expression of OsASR1 under ABA, NaCl, and drought stress in leaves and roots of WT rice plants. OsASR1 expression difference was calculated relative to the expression level in leaves of untreated WT plants. (C) qRT-PCR analysis of OsASR1 expression in transgenic and WT plants exposed to 200 mM NaCl and drought for 6 h. OsASR1 expression difference was calculated relative to the expression level in WT leaves. Error bars indicate standard error (SE) based on three replicates. TR, OsASR1-overexpressing transgenic rice plants; WT, wild-type rice plants. Asterisks indicate significant differences between treatments determined by Student's t-test (P < 0.05).
To determine RWC under salt and drought-stress conditions, the midsection of the third fully expanded leaf blade of OsASR1-overexpressing transgenic and WT plants was sampled before, during, and after treatment with 200 mM salt and drought stress for 7 and 3 d, respectively. After measuring the fresh weight (FW) of the rice leaves, the leaves were soaked in distilled water for 4 h at room temperature under constant light, and turgid weight (TW) was measured. The leaves were then dried for 24 h at 80°C to determine the total dry weight (DW). RWC was calculated according to the formula RWC = (FW–DW)/(TW–DW)×100 (Gao et al., 2015). The amount of malondialdehyde (MDA) was determined by the thiobarbituric acid (TBA)-based colorimetric method (Hodges et al., 1999). Briefly, 0.1 g leaf sample was homogenized using liquid nitrogen, mixed with a solution containing 20% (w/v) trichloroacetate and 0.65% (w/v) thiobarbituric acid, heated in a water bath at 95°C for 25 min, and centrifuged for 10 min at 12,000 rpm at 4°C. Finally, the MDA concentration of the resulting supernatant was measured at 532 nm based on 600 nm and calculated using a molar extinction coefficient of 1.56 × 105 cm-1 M-1 for MDA.
For analysis of free proline and total soluble sugars, the second leaves were sampled and ground. Then, 0.6 mL of 80% (v/v) hot ethanol was added, and the sample was mixed for 2 min. After centrifugation at 1200 rpm for 5 min, the supernatant was collected. The pellet was re-extracted twice with 0.4 mL of 80% ethanol, then all supernatants were combined. The content of free proline dissolved in ethanol was measured using the ninhydrin method (Wren and Wiggall, 1965). The total soluble sugars dissolved in ethanol were determined using the anthrone method (Irigoyen et al., 1992). Fifty-microliter samples dissolved in ethanol were dried under vacuum, dissolved in 0.1 mL de-ionized water, and proteins were removed by mixing with 0.1 mL 0.3 N Ba(OH)2 and 0.1 mL 5% ZnSO4. After centrifugation at 12000 rpm for 5 min, 0.1 mL of supernatant was reacted with 0.4 mL freshly prepared anthrone reagent (100 mg anthrone in 50 mL 95% H2SO4) at 100°C for 10 min and cooled at 4°C. Finally, the total soluble sugars were determined by measuring absorbance at 620 nm in a spectrophotometer, using glucose as the standard.
Four leaves from each transformant and WT plant were harvested 7 weeks after they had been transplanted in paddy fields. Leaves were cut and soaked in 0.1% nitro blue tetrazolium (NBT) in 10 mM sodium phosphate buffer for detection of superoxide ion (O2-) (Lin et al., 2009). Then, the samples were incubated in a growth chamber for 48 h and the chlorophyll was removed with ethanol (95%). The superoxide anion stains dark blue (Awasthi et al., 2018).
Four-week-old plants were treated with 200 mM NaCl solution for 3 d and the Na+ concentration of leaves was measured. Total leaves from five plants of each transformant and WT plant were homogenized using a mortar and pestle in liquid nitrogen. The ground powder was placed in a 50-mL tube and dried in an oven at 80°C for 72 h. Dried samples (50 mg) were heated for 1 h at 95°C in distilled water, and then filtered through polyimide syringe filters. Na+ and K+ ion concentrations (calculated as NaCl equivalents) were determined using inductively coupled plasma optical emission spectroscopy (PerkinElmer, Shelton, CT, USA).
Leaves of OsASR1-overexpressing transgenic and WT plants exposed in the greenhouse to 200 mM NaCl or drought (5 and 2 d, respectively) or grown in paddy fields without irrigation for 4 d were directly fixed with formaldehyde-acetic acid alcohol (FAA) solution containing 5 mL of ethanol, 0.5 mL of acetic acid, 1 mL of 37% formaldehyde, and 3.5 mL of distilled water. Samples were incubated under vacuum (550 mmHg) and then transferred to a fresh FAA solution at 4°C overnight. Samples were then washed three times with sodium phosphate buffer, dehydrated through an ethanol series at room temperature, and stored in 100% ethanol before use. The stomatal images were obtained with a scanning electron microscope (SEM) (SU 8220, Hitachi, Tokyo, Japan), and the percentages of stomata that were completely open, partially open, and completely closed were calculated (n = 100 stomata). A portable CO2 gas analyzer (LCi, ADC BioScientific Ltd.) was used to test the stomatal conductance, transpiration rate, and net photosynthetic rate in transgenic and WT plants exposed in the greenhouse to 200 mM NaCl or drought for 5 and 2 d, respectively. Net photosynthetic rate, stomatal conductance, and transpiration rate were measured in greenhouse conditions maintained at 30 ± 4°C during the day with relative humidity of 60–70% at 2–3 pm in July. At the time of measurement, the PPFD in the greenhouse was about 843–867 µmol m−2 s−1. Second leaves of four-week-old rice plants from each line were measured.
The endogenous ABA levels were determined by a Shimadzu Prominence HPLC System (Shimadzu Scientific Instruments, Inc., Columbia, MD, USA) (Changan et al., 2018). Second leaves (2 g) from each line were sampled and put into liquid nitrogen, ground into powder, and extracted into 5 mL 98% (v/v) acetone for 12 h at 4°C. The supernatant was filtered using Whatman No. 1 filter paper. The filtrate was evaporated with evaporator (IKA RV 10 rotary evaporator; IKA®—Werke GmbH & Co., Germany) to remove the acetone. The soluble material deposited on the walls of the glass tube was dissolved in 1% (v/v) acetic acid, and the samples were filtered through a sterilized Millipore filter and injected into the HPLC system. Standard solutions of ABA were directly prepared in 95% (v/v) methanol. Separation was carried out on a Waters Symmetry C18 column (4.6×250 mm, 5 µm) and maintained at 30°C with a gradient elution at the flow rate of 1 mL·min−1. A 1% acetic acid-methanol (40:60, v/v) was the mobile phase. The target component was quantified by the peak areas at the maximum wavelength of 260 nm. The run time was 20 min.
To test the sensitivity to ABA at the germination stage, 30 seeds were placed in Petri dishes containing 0, 2.5, and 5 μM ABA at 16-h light/8-h dark cycles (28°C), and the number of seeds germinated on the fifth day was counted. The seeds of OsASR1-overexpressing transgenic and WT plants germinated on normal 1/2 MS medium were transplanted to 1/2 MS medium containing 0, 2.5, and 5 μM ABA at 16-h light/8-h dark cycles (28°C). The shoot and root lengths of each seedling were measured after 10 d.
To generate drought conditions in paddy fields, the fields were maintained by watering using an irrigation system for 3 d followed by 4 d of withholding water beginning at 4 weeks after transplantation into the field. Once the plants were under drought conditions, we counted the numbers of tillers on rice plants at 4–7 weeks after transplantation into the paddy field to measure stress tolerance in an intermittently irrigated environment.
Four-week-old seedlings of OsASR1-overexpressing transgenic and WT plants were transplanted into a paddy field located at the Gunwi campus of Kyungpook National University each growing season from 2015 (T3) to 2018 (T6). The paddy field was fertilized once a year using commercially available fertilizer, Powerplus (FarmHannong, Seoul, South Korea), based on the fertilization reference value. A completely randomized block design was employed, and each plot contained 30 plants with two rows for each line, 0.2 m between plants, and 0.3 m between plots. When the transgenic plants reached maturity, agronomic traits were calculated for 10 plants per plot. They were harvested and threshed by hand. Filled grains were randomly counted and weighed. The following agronomic traits were scored: total plant weight (g), culm weight (g), root weight (g), number of tillers per plant, number of panicles per plant, total grain yield (g), number of grains per panicle, number of spikelets per panicle, and 1000-grain weight (g).
Temperature and precipitation data for the paddy field located at the Gunwi campus of Kyungpook National University were obtained from the Korea Meteorological Administration (http://www.kma.go.kr/index.jsp).
For the biochemical measurements (RWC, MDA, proline, total soluble sugars, Na+, K+, and ABA) in rice plants, each sample contained three replicates, and each replicate contained five seedlings. For the germination test of in rice seeds on wet filter paper containing 0, 2.5, or 5 μM ABA, each sample contained three replicates, and each replicate contained 30 seeds. In order to measure the tiller number of rice plants at 4–7 weeks after transplantation into the paddy field, we counted the numbers of tillers on the same ten plants per each transgenic and WT plants. Comparisons between individual data points were performed using Student's t-test with P < 0.05 considered as significant. All experiments were performed at least in triplicate, and all results are expressed as the mean ± SE.
Genes and their associated accession numbers from the GenBank database are as follows: Tubulin (AK072502), OsASR1 (AK062319), OsNCED4 (AY838900), OsNCED5 (AY838901), OsRab16C (AK071366), and OsRab16D (AK109096). Genes and their associated accession numbers from the Rice Annotation Project Database are as follows: OsZEP1 (LOC_Os04g37619), OsRab21 (LOC_Os11g26790), OsMOC1 (LOC_Os06g40780), OsDLT (LOC_Os06g03710), OsMPH1 (LOC_Os06g45890), and OsPROG1 (LOC_Os01g03840).
To determine the tissue specificity of endogenous OsASR1 expression in rice, we performed qRT-PCR analysis using mRNA from different rice tissues. OsASR1 was expressed in all tested tissues, including leaf, root, stem, and embryo, regardless of developmental stage (Figure 1A). OsASR1 expression in leaves was higher than in other tissues. To investigate OsASR1 responses to abiotic stress, OsASR1 transcript levels were analyzed in leaf and root tissues before and after exposure to ABA, NaCl, and drought (Figure 1B). The predominant expression of OsASR1 in leaves was confirmed under all tested conditions. OsASR1 transcripts in leaves were most strongly expressed at 3 h after ABA treatment and at 6 h after NaCl- and drought-stress treatment, whereas OsASR1 was expressed continuously in root tissues under drought stress. These results suggest that OsASR1 is responsive to abiotic stresses.
To further analyze the role of OsASR1 in rice plants under salinity and drought conditions, we generated a construct for Agrobacterium-mediated rice transformation (Christensen and Quail, 1996) (Supplementary Figure S1A), which expressed the OsASR1 coding sequence under the control of the constitutive maize ubiquitin promoter (Ubi). Four independent OsASR1-overexpressing transgenic lines were obtained (TR1, TR2, TR3, and TR4) and confirmed by genotyping (Supplementary Figure S1B). Next, to examine whether OsASR1 was expressed under the regulation of the Ubi promoter at the transcriptional level, real-time PCR with OsASR1-F1 and OsASR1-R1 primers (Supplementary Table S2) was performed. We assessed expression in the leaves of four independent 4-week-old transgenic and WT rice plants grown in the greenhouse. OsASR1 expression levels were about 5.9-fold greater in the TR1, TR2, and TR3 transgenic lines and 2-fold greater in the TR4 line than in WT rice plants (Supplementary Figure S2). We selected the three independent transgenic lines TR1, TR2, and TR3 based on their relatively high and similar gene expression levels for subsequent experiments.
A single copy of the Ubi::OsASR1 transgene construct was inserted into the rice genome of the TR1 and TR2 lines (Supplementary Table S1). Two copies of the Ubi::OsASR1 transgene construct were inserted into the rice genome of the TR3 line. T1 to T6 seeds were harvested from each transgenic plant line, and three homozygous T6 lines were used for further analysis of each line.
To evaluate the responses of the transgenic plants to salt and drought stress, the OsASR1 expression levels were analyzed in 4-week-old transgenic plants treated with 200 mM NaCl and drought for 6 h (Figure 1C). The results clearly showed that OsASR1 expression levels were upregulated in transgenic plants compared to wild-type (WT) plants.
To perform a salt and drought tolerance test of OsASR1-overexpressing transgenic plants in soil, 4-week-old transgenic and WT plants at the seedling stage were exposed to 200 mM NaCl and drought for several days. Before stress treatment, no morphological differences were observed between the transgenic and WT plants (Figures 2A and 3A, upper panel, Supplementary Figure S3). Stress-induced phenotypic changes, such as leaf rolling and wilting, occurred earlier in WT plants than in OsASR1-overexpressing plants (Figures 2A and 3A, upper panel, Supplementary Figure S3). Because the phenotype of most of the WT plants was more affected by stress than the OsASR1-overexpressing plants after 3 and 7 d of drought and salt stress, respectively, these periods were used in further experiments (Supplementary Figure S3). The survival rate of transgenic plants was approximately 2-fold higher than that of WT plants (Figures 2B and 3A, lower panel). The enhanced salt and drought tolerance in OsASR1-overexpressing plants prompted us to investigate physiological differences between the transgenic and WT plants. Therefore, we conducted ionomic analyses using leaves of transgenic and WT plants before and after exposure to 200 mM NaCl for 3 d. The Na+ contents and Na+/K+ ratios are known indicators of salt tolerance in plants under salt stress (Gao et al., 2003). Before NaCl treatment, the Na+ contents of both transgenic and WT plants were approximately 10 ppm (Figure 2C), with no significant differences between the values of each line. The Na+ content in WT plants after 3 d of salt stress was more than 1.6-fold higher than that of OsASR1-overexpressing plants (Figure 2C), and the Na+/K+ ratio of WT plants was 2.3-fold higher than that in transgenic plants (Figure 2D). The relative water content (RWC) is an indicator of osmotic stress tolerance. We measured RWC at 7 and 3 d under NaCl and drought stress in whole plants of transgenic and WT lines. OsASR1-overexpressing plants displayed enhanced survival under salt and drought stress compared to WT plants. The RWC of transgenic plants was approximately 1.5- and 2.0-fold higher than that of WT plants under NaCl and drought stress, respectively (Figures 2E and 3B), suggesting that OsASR1 may have a role in preventing water loss under salt- and drought-stress conditions. Malondialdehyde (MDA) is an indicator of membrane damage and was significantly lower in OsASR1-overexpressing plants than in WT plants under the same conditions (Figures 2F and 3C).
Figure 2 Analysis of salt tolerance in OsASR1-overexpressing transgenic plants. (A) Four-week-old seedlings (n = 50) after treatment with 200 mM NaCl for 7 d followed by recovery for 5 d. (B) Survival rates of transgenic and WT plants after recovery for 5 d. (C) Na+ contents and (D) Na+/K+ ratio of transgenic and WT plants. (E) Relative water content (RWC) of transgenic and WT plants after NaCl treatment for 7 d. (F) MDA levels, (G) proline contents, and (H) total soluble sugar contents in transgenic and WT plants. Analyses of Na+, Na+/K+ ratio, MDA, proline, and total sugar were carried out with leaves of rice plants treated with 200 mM NaCl for 3 d. Asterisks indicate significant differences between treatments determined by Student's t-test (P < 0.05).
Figure 3 Analysis of drought tolerance in OsASR1-overexpressing transgenic plants. (A) Four-week-old seedlings (n = 50) after drought exposure for 3 d followed by recovery for 7 d (top). Survival rates of transgenic and WT plants after drought exposure for 3 d and recovery for 7 d (bottom). (B) Relative water content (RWC) after drought treatment for 3 d, (C) MDA content, (D) proline content, and (E) total sugar content in transgenic and WT plants. Analyses of MDA, proline, and total soluble sugars were carried out in rice plants subject to drought for 2 d. Asterisks indicate significant differences between treatments determined by Student's t-test (P < 0.05).
To investigate the relationship between OsASR1 expression and osmolyte synthesis, we measured the contents of free proline and total soluble sugars in OsASR1-overexpressing and WT plants subjected to salt and drought stress. The contents of free proline and water-soluble sugars increased in both OsASR1-overexpressing and WT plants after treatment with salt and drought, although the magnitude of increase significantly differed in the two plant lines (Figures 2G, H and 3D, E). The results show that OsASR1-overexpressing plants accumulate higher contents of osmolytes such as proline and soluble sugars than WT plants, suggesting that OsASR1-overexpressing plants have enhanced tolerance to salt and drought stress compared to WT plants.
Osmotic stress in plants induces water loss primarily through stomata. Therefore, we examined stomatal apertures in OsASR1-overexpressing transgenic plants, which had lower water loss than WT plants under salt and drought stress, using scanning electron microscopy (SEM). Under normal conditions, the percentages of completely closed, partially open, and completely open stomata were essentially the same in OsASR1-overexpressing and WT plants (Figure 4A). By contrast, the percentages of completely closed stomata were higher in OsASR1-overexpressing plants than in WT plants, and the percentages of completely open stomata were lower in OsASR1-overexpressing plants under salt and drought stress. In both transgenic and WT plants, the percentages of completely closed stomata were higher under drought stress than under salt stress. Furthermore, the stomatal conductance transpiration rates, and net photosynthetic rates were significantly reduced in OsASR1-overexpressing plants under salt and drought stress (Figures 4B, C, Supplementary Figure S4). These results suggest that the low percentages of open stomatal apertures and the high percentages of completely closed stomata in OsASR1-overexpressing plants reduced stomatal conductance and transpiration rate, thereby preserving endogenous water content and enhancing salt and drought tolerance by preventing saline absorption in rice.
Figure 4 Stomatal closure, ABA accumulation, and expression of ABA biosynthesis genes and ABA-responsive genes in response to NaCl and drought stress in OsASR1-overexpressing transgenic plants. (A) Scanning electron microscopy images and the percentages of open, closed, and partially open stomatal apertures in leaves of transgenic and WT plants (n = 100 stomata). (B) Stomatal conductance in transgenic and WT plants (n = 5). (C) Transpiration rate in transgenic and WT plants (n = 5). (D) Endogenous ABA contents of transgenic and WT plants (n = 5). qRT-PCR analysis of the expression of (E) ABA biosynthesis genes and (F) ABA-responsive genes in transgenic and WT plants (n = 5) after salt (3 d) and drought (1 d) stress. Stomatal image, stomatal conductance, transpiration rate, and endogenous ABA content analyses were performed with rice leaves subjected to drought and salt stress for 2 and 5 d, respectively. Asterisks indicate significant differences between treatments determined by Student's t-test (P < 0.05).
ABA regulates stomatal movement. Therefore, we measured ABA contents in leaves of OsASR1-overexpressing and WT plants subjected to 200 mM NaCl and drought for 5 and 2 d, respectively. The results showed that ABA levels in transgenic plants were more than 1.25-fold higher than in WT plants (Figure 4D). We hypothesized that OsASR1 had a major regulatory role in the ABA biosynthetic pathway and likely participated in an ABA‐dependent stress‐signaling pathway in response to salt and drought stress.
To test these hypotheses, we analyzed the transcript levels of ABA biosynthetic genes (OsZEP1, OsNCED4, and OsNCED5) and ABA-responsive genes (OsRab21, OsRab16C, and OsRab16D) in OsASR1-overexpressing and WT plants subjected to 200 mM NaCl and drought for 3 and 1 d, respectively. The results showed that the expression levels of all six genes were more strongly upregulated by both salt and drought stresses in OsASR1-overexpressing plants than in WT plants (Figures 4E, F), whereas the expression levels of these genes did not significantly differ in transgenic and WT plants under normal conditions. These combined results indicate that OsASR1 has a significant role in the ABA‐dependent stress‐signaling pathway by upregulating ABA biosynthesis and the expression of ABA-responsive genes under abiotic stress conditions.
ABA-mediated induction of OsASR1 expression was confirmed in previous experiments (Figure 1B). Therefore, we predicted that OsASR1 might be involved in ABA signaling. To confirm this hypothesis, seed germination and growth were examined after treating seeds of OsASR1-overexpressing transgenic plants with exogenous ABA. Transgenic and WT plants displayed similar germination rates in the absence of exogenous ABA (Figure 5A). By contrast, treating seeds with 2.5 and 5 μM ABA significantly reduced germination rates in transgenic plants (66% and 56%, respectively) compared to WT plants (93% and 76%, respectively). These results suggest that OsASR1-overexpressing rice plants were more susceptible to ABA during germination than WT plants.
Figure 5 Abscisic acid (ABA) sensitivity in the germination and growth of seeds from OsASR1-overexpressing transgenic plants. (A) Germination phenotype and percentage of transgenic and WT rice seeds on wet filter paper containing 0, 2.5, or 5 µM ABA 5 d (n = 30). (B) Shoot and root length of transgenic and WT germinated seedlings grown in 1/2 MS medium containing 0, 2.5, or 5 µM ABA for 10 d (n = 10). Asterisks indicate significant differences between treatments determined by Student's t-test (P < 0.05).
Next, we evaluated the sensitivity of seedling growth to exogenous ABA. In the absence of exogenous ABA, there was no significant difference in seedling growth of transgenic and WT plants (Figure 5B). Treatment with 2.5 and 5 μM ABA disrupted the shoot and root growth of transgenic seedlings more strongly than those of WT seedlings. These results showed that OsASR1-overexpression increased the susceptibility to exogenous ABA during seed germination and seedling growth, suggesting that OsASR1 may be a positive regulator of ABA responses.
The preceding results prompted us to investigate the drought tolerance of rice plants in paddy fields. Drought conditions were generated by conducting intermittent irrigation starting at 4 weeks after plants were transplanted into the paddy fields. Phenotypic evaluation of OsASR1-overexpressing and WT plants revealed a significant difference in the vegetative growth of whole plants (Figure 6A). Notably, the number of tillers observed on transgenic plants gradually increased starting at 5 weeks (1 week after the initiation of drought conditions) compared to the number of tillers observed on WT plants (Figure 6B). We analyzed the accumulation of reactive oxygen species (ROS) and MDA produced by drought stress. The leaves of WT plants exhibited a purple-blue coloration after staining with an indicator for superoxide anion (O2–) production, and MDA contents in WT plants were higher than those in transgenic plants (Supplementary Figure S5). Next, we evaluated the stomatal apertures in leaves of OsASR1-overexpressing and WT plants under drought conditions (without irrigation for 4 d). We used the TR1 transgenic line for this experiment because it had a better overall phenotype under salt and drought stress of the two lines that expressed one copy of the transgene (Figures 2 and 3, Supplementary Table S1). The percentage of completely closed stomata was higher in transgenic plants (57%) than in WT plants (38%), and the percentage of completely open stomata was 1.5 times lower in transgenic plants than in WT plants (Figure 6C). At this point, OsASR1-overexpressing plants had greater fresh weight than WT plants (Figure 6E).
Figure 6 Drought tolerance in paddy fields. (A) Phenotypes of OsASR1-overexpressing transgenic and WT rice plants 5 weeks after transplanting into the paddy fields. (B) Number of tillers on OsASR1-overexpressing transgenic and WT rice plants from 4 to 7 weeks after transplanting into the paddy fields. (C) Scanning electron microscopy images and the percentages of open, closed, and partially open stomatal apertures in leaves of transgenic and WT plants (n = 100 stomata). (D) Endogenous ABA contents in leaves of transgenic and WT plants. (E) Phenotypes and fresh weight of transgenic and WT plants. Fresh weight represents the value of one plant per each line. (F) qRT-PCR analysis of genes involved in ABA biosynthesis (OsZEP1, OsNCED4, and OsNCED5) and (G) ABA-responsive genes (OsRab21, OsRab16C, and OsRab16D) from leaves of transgenic and WT plants. Asterisks indicate significant differences between treatments determined by Student's t-test (P < 0.05). Stomatal image, endogenous ABA content, fresh weight, and qRT-PCR analyses were performed with transgenic and WT plant grown in the paddy fields. Plants were irrigated for 4 weeks and then grown for 4 d without irrigation.
To confirm whether these results are associated with ABA signaling, we analyzed the ABA contents and expression levels of ABA biosynthetic and ABA-responsive genes in transgenic and WT plants. The results showed that ABA contents were 1.5-fold higher in transgenic plants than in WT plants under drought conditions in paddy fields (Figure 6D), which was similar to the results obtained in plants subjected to salt and drought stress in the laboratory (Figure 4D). The expression levels of three ABA biosynthetic genes, OsZEP1, OsNCED4, and OsNCED5, were higher in OsASR1-overexpressing plants than in WT plants (Figure 6F). The expression levels of three ABA-responsive genes, OsRab21, OsRab16C, and OsRab16D, also were higher in transgenic plants than in WT plants subjected to drought conditions in paddy fields (Figure 6G). These data further indicated that OsASR1 might have a major role in regulating the expression of ABA biosynthetic and ABA-responsive genes under drought conditions, thereby regulating stomatal aperture and enhancing drought tolerance.
A phenotypic analysis of whole OsASR1-overexpressing transgenic and WT plants revealed a major difference in plant growth during the vegetative stage in paddy fields (Figure 6A). To evaluate whether this significant physiological difference persists through the reproductive stage, we evaluated the yield components of transgenic plants subjected to drought conditions in paddy fields during four consecutive growing seasons (2015–2018) that had different climatic conditions (Supplementary Figure S6). Three independent homozygous lines of the OsASR1-overexpressing and WT plants were transplanted into paddy fields and grown to maturity. Transgenic plants produced more tillers than WT plants (Figure 6B), which contributed to higher accumulated biomass in transgenic plants than in WT plants under drought conditions in paddy fields (Figure 7A and Table 1). The total plant weight (g), culm weight (g), root weight (g), number of tillers per plant, and number of panicles per plant during the four growing seasons in OsASR1-overexpressing plants were on average 11.4, 18.5, 18.0, 16.8, and 15.8% greater, respectively, than in WT plants (Table 1). The higher numbers of panicles and spikelets in OsASR1-overexpressing transgenic plants contributed to higher grain yields than those in WT plants (Figure 7B and Table 1). These results prompted us to analyze the expression patterns of several genes (OsMOC1, OsDLT, OsMPH1, and OsPROG1) involved in the regulation of tillering, which in turn determines panicle number, a key component of grain yield (Li et al., 2003; Bolle, 2004; Jin et al., 2008; Tong and Chu, 2009; Zhang et al., 2017). The expression levels of these genes were measured in leaf tissue sampled 6 weeks after transplanting, which is when the number of tillers increased dramatically. Gene expression in transgenic plants was upregulated at least 4.7-fold in transgenic plants compared to WT plants at this stage (Figure 7C). These combined results suggest that OsASR1 overexpression significantly improves grain yield by increasing drought tolerance in rice and increasing the expression of productivity-related genes at the reproductive stage.
Figure 7 Grain yields from consecutive generations of transgenic plants in paddy fields under drought conditions. (A) The phenotype of transgenic plants at the reproductive stage in 2015 (T3), 2016 (T4), 2017 (T5), and 2018 (T6). (B) Panicle phenotype of transgenic plants at the reproductive stage. (C) qRT-PCR analysis of grain yield-related genes from transgenic and WT plants grown in paddy fields for 6 weeks. Asterisks indicate significant differences between treatments determined by Student's t-test (P < 0.05).
Table 1 Agronomic traits of OsASR1-overexpressing transgenic plants grown in paddy fields under drought conditions in 2015–2018.
Increases in agricultural salinity and drought have reduced crop growth and crop yields worldwide. It is crucial for future food security to use biotechnology for the development of new crop varieties that can sustain agricultural productivity under these abiotic stresses. Genes that are responsive to saline and drought conditions have been identified through genetic and transcriptomic analysis of plants that are well-adapted to and even thrive in saline soils or drought (Lenka et al., 2011; Ding et al., 2013). For example, SNAC1, OsLEA3-1, and OsMIOX were identified by comparative transcriptome analysis of a drought-resistant upland rice and a drought-sensitive lowland rice, and they have been shown to be involved in drought tolerance (Hu et al., 2006; Xiao et al., 2007; Duan et al., 2012). Several OsASR genes were strongly induced by drought stress in an upland, drought-resistance variety but not in a lowland, drought-sensitive variety (Li et al., 2017). Among them, OsASR5 overexpression contributed to enhanced drought resistance in lowland rice variety, Nipponbare (O. sativa L. ssp. japonica). In addition, transcriptome sequencing of salt-tolerant halophytes has resulted in identification of candidate genes involved in salinity tolerance (Chen et al., 2016; Mishra and Tanna, 2017). For example, overexpression of an ASR gene from the halophyte Salicornia brachiata enhanced salt and drought endurance in the salt-sensitive glycophytes Arachis hypogea and Nicotiana tabacum (Jha et al., 2012; Tiwari et al., 2015). From these studies, it is reasonable to hypothesize that OsASR1 is a salt and drought stress-responsive gene in rice. To test this, we constructed transgenic lines of the lowland rice variety, Ilmi that overexpressed OsASR1.
In our previous study, we founded that the OsASR1 protein accumulated in response to salt stress in Ilmi rice leaves (Kim et al., 2012). This study further confirmed that endogenous OsASR1 in rice was strongly upregulated in response to ABA, salt, and drought (Figure 1B), similar to that observed for ASR expression patterns in other plants (Yang et al., 2005; Hu et al., 2013). Many researchers have suggested that the genes induced in response to abiotic stresses confer abiotic stress tolerance (Yanez et al., 2009; Wang et al., 2016a). The endogenous OsASR1 expression patterns suggested that it might have a positive role in abiotic stress responses to salt and drought. We generated OsASR1-overexpressing transgenic plants to investigate OsASR1 function under salt or drought-stress conditions in the greenhouse and under drought conditions in paddy fields (Supplementary Figure S1).
Salt and drought stresses ultimately induce a state of water deficiency in plants by affecting regulation of stomatal aperture (Munns and Tester, 2008; Lim et al., 2015). Stomatal apertures can regulate plant water status by controlling water loss due to transpiration (Hetherington and Woodward, 2003; Kuromori et al., 2018). Subjecting tomato MYB transcription factor mutants (ars1) to salt stress conditions resulted in high Na+ accumulation and water loss due to high stomatal conductance and transpiration rate caused by loss of stomatal function (Campos et al., 2016). Other studies on salt-tolerant species of Arabidopsis (Wu et al., 2012) and tomato (Koenig et al., 2013) and drought-tolerant rice Azucena (Price et al., 2002) also demonstrated that control of stomatal aperture size and movement has a crucial role in salt or drought stress tolerance and mitigating water loss under salt and drought stress. We observed that, in greenhouse-grown plants subjected to salt or drought stress, a much greater percentage of the stomata of OsASR1-overexpressing plants were completely closed than in the WT plants (Figure 4A). As a result, the OsASR1-overexpressing plants had lower Na+ contents, Na+/K+ ratios, and water loss than WT plants (Figures 2C–E and 3B) due to significantly reduced stomatal conductance and transpiration rates under salt and drought-stress conditions (Figures 4B, C).
Stomatal aperture is primarily regulated by ABA (Desikan et al., 2004). Drought conditions increase the synthesis and accumulation of ABA in guard cells, causing stomata to close to conserve water (Lim et al., 2015). Seven genes that respond to drought and regulate stomatal aperture in rice have been identified, and five of them (SNAC1, OsSDIR1, hrf1, SQS, and OsCPK9) are sensitive to ABA (Hu et al., 2006; Huang et al., 2009; Gao et al., 2011; Zhang et al., 2011; Manavalan et al., 2012; You et al., 2013; Wei et al., 2014). These genes represent elements of a complex ABA regulatory network that is not completely understood (Schroeder et al., 2001; Gampala et al., 2002). In this study, we demonstrated that OsASR1 expression is rapidly induced by exogenous ABA (Figure 1B), and seed germination and growth are hypersensitive to exogenous ABA in OsASR1-overexpressing plants (Figure 5). These knowledge and results suggest that OsASR1 may be involved in the ABA signaling pathway. Furthermore, the endogenous ABA contents of OsASR1-overexpressing plants under salt and drought-stress conditions were much higher than WT plants by enhancing the expression of ABA biosynthesis genes, OsZEP1, OsNCED4, and OsNCED5 (Figures 4D, E). Further support for this contention is that in Vicia faba, overexpression of AtNCED3 and AAO3, genes involved in ABA biosynthesis, dramatically increased ABA levels in guard cells, apparently causing the observed stomatal closure (Melhorn et al., 2008). Also, drought-stressed transgenic Arabidopsis plants overexpressing AtZEP had reduced stomatal apertures and presumably less water loss than the control (Park et al., 2008). ABA also activates several stress-responsive genes involved in the biosynthesis of osmolytes and LEA-like proteins, which enhance osmotic stress tolerance in plants and mitigate stress-induced damage (Cutler et al., 2010; Fujita et al., 2011). Overexpression of the ABA biosynthesis gene enhanced drought or salt resistance by elevating ABA and osmolytes in transgenic petunia overexpressing tomato NCED1 (Estrada-Melo et al., 2015) and transgenic tobacco overexpressing alfalfa ZEP (Zhang et al., 2016). Based on these studies, it seems reasonable that OsASR1-overexpressing plants with high endogenous ABA levels would also increase the levels of osmolytes, such as proline and soluble sugars (Figures 2G, H and 3D, E). Consequently, we analyzed the expression of three LEA family genes: RAB21, RAB16C, and RAB16D, which may be responsive to ABA (Roychoudhury et al., 2007). Also, relevant here is the possible importance of Rab proteins in enhancing plant tolerance to abiotic stress (Mazel et al., 2004; Roychoudhury et al., 2007; El-Esawi and Alayafi, 2019). Overexpression of AtRabG3e in plants resulted in activation of several mechanisms that protect against osmotic stresses, such as reduced generation of ROS (Mazel et al., 2004). The OsRab7 gene enhanced drought in transgenic rice by modulating osmolytes, antioxidants, and abiotic stress-responsive genes expression (Mohamed et al., 2001). In this study, our results showed that the expression of RAB21, RAB16C, and RAB16D genes was significantly higher in OsASR1-overexpressing plants than in WT plants under salt and drought stress (Figure 4F). From this study with drought- and salt-stressed transgenic plants, we conclude that OsASR1 resulted in enhanced ABA accumulation, which led to induced stomatal closure, osmolyte accumulation, and enhanced expression of LEA genes in the ABA-dependent pathway.
Crops in the field are routinely exposed to combinations of environmental stresses (Anjum et al., 2011), and these variable conditions are often not reproduced in controlled studies of stress responses. Notably, transgenic plants with improved tolerance stress in controlled conditions do not always exhibit this tolerance in the field (Mittler, 2006). Therefore, it is important to study the stress response of rice plants under natural paddy field conditions. To do this, we compared the growth of transgenic rice plants that were drought resistance in the greenhouse (Figure 4) to WT plants in the field, where we imposed drought stress with intermittent irrigation. The results in Figure 6 confirm that OsASR1-transgenic plants had drought tolerance not only in greenhouse conditions but also in paddy field conditions. We propose that the better growth of the transgenic plants in the fields was, as in the greenhouse, due to increased endogenous ABA, which induced stomatal closure and upregulated LEA genes.
We observed drought tolerance and improved productivity in the transgenic plants in the paddy fields over four consecutive growing seasons (Figure 7A and Table 1). The increased grain yield was primarily caused by increases in the number of tillers, panicles, and spikelets per panicle (Figures 6B, 7B and Table 1). There are many studies on genes directly involved in determining grain yield by tillering and panicle branching (Liang et al., 2013). MOC1 and DLT are members of the plant-specific GRAS family proteins, which function in diverse aspects of plant development and responses to abiotic stress (Bolle, 2004). Studies with mutants have demonstrated that these genes promote the production of rice tillers and panicle branches (Li et al., 2003; Tong and Chu, 2009; Tong et al., 2009). MYB and zinc-finger genes also influence both plant development and ABA-mediated abiotic stress tolerance (Feller et al., 2011; Baek et al., 2015; Wang et al., 2015). Overexpression of OsMPH1 (MYB-like transcription factor) and OsPROG1 (zinc-finger transcription factor) leads to increases in grain yield through regulation of plant height and tillers in rice (Jin et al., 2008; Zhang et al., 2017). Our results showed that these genes related to grain yield (OsMOC1, OsDLT, OsMPH1, and OsPROG1) were significantly induced in OsASR1-overexpressing plants (Figure 7C). These combined results suggest that OsASR1-overexpressing plants increased grain yield by improving drought resistance due to efficient stomatal closure and upregulating grain yield regulated-genes.
Based on our knowledge, we tried to summarize a model to explain the role of OsASR1 in improving salt and drought stress tolerance and enhancing grain yield through modulation of stomatal closure in plant (Figure 8). In conclusion, we demonstrated that OsASR1 is a positive regulator of ABA accumulation by enhancing the expression of ABA biosynthesis genes. Increased ABA accumulation in OsASR1-overexpressing transgenic rice plants leads to enhancing water retention and tolerance to salt and drought stress by modulating osmolytes accumulation, the stomatal closure, and LEA genes expression to avoid water loss. OsASR1 can improve grain yield in rice grown in paddy fields under drought conditions due to improved drought tolerance and upregulation of grain yield-related genes, which indicates that OsASR1 has a crucial role in drought tolerance and crop improvement. Therefore, this study suggests that OsASR1 may be an effective transgene for genetic engineering approaches to develop crops that are tolerant to salt or drought stress and have enhanced productivity.
Figure 8 A proposed model describing the function of OsASR1 in enhancing salt and drought stress tolerance and improving grain yield through modulation of stomatal closure.
All datasets for this study are included in the article/Supplementary Material.
S-IP, Y-SK, and H-SY conceived the research and wrote the article. S-IP performed most of the experiments and analyzed data, J-JK assisted in phenotypic analyses, S-YS constructed the plasmid vector, and H-SY supervised the research.
This work was supported by grants from the Next-Generation BioGreen 21 Program (No. PJ01366701), Rural Development Administration, Republic of Korea, and the Basic Science Research Program through the National Research Foundation of Korea (NRF) funded by the Ministry of Education (2018R1D1A3B07049385), Republic of Korea.
The authors declare that the research was conducted in the absence of any commercial or financial relationships that could be construed as a potential conflict of interest.
The Supplementary Material for this article can be found online at: https://www.frontiersin.org/articles/10.3389/fpls.2019.01752/full#supplementary-material
Anjum, S. A., Xie, X. Y., Wang, L. C., Saleem, M. F., Man, C., Lei, W. (2011). Morphological, physiological and biochemical responses of plants to drought stress. Afr. J. Agr. Res. 6, 2026–2032. doi: 10.5897/AJAR10.027
Arenhart, R. A., Lima, J. C., Pedron, M., Carvalho, F. E., Silveira, J. A., Rosa, S. B., et al. (2013). Involvement of ASR genes in aluminium tolerance mechanisms in rice. Plant Cell Environ. 36, 52–67. doi: 10.1111/j.1365-3040.2012.02553.x
Awasthi, J. P., Saha, B., Chowardhara, B., Devi, S. S., Borgohain, P., Panda, S. K. (2018). Qualitative analysis of lipid peroxidation in plants under multiple stress through Schiff's reagent: a histochemical approach. Bio Protoc. 8, e2807. doi: 10.21769/BioProtoc.2807
Baek, D., Cha, J. Y., Kang, S., Park, B., Lee, H. J., Hong, H., et al. (2015). The Arabidopsis a zinc finger domain protein ARS1 is essential for seed germination and ROS homeostasis in response to ABA and oxidative stress. Front. Plant Sci. 6, 963. doi: 10.3389/fpls.2015.00963
Blumwald, E. (2000). Sodium transport and salt tolerance in plants. Curr. Opin. Cell Biol. 12, 431–434. doi: 10.1016/S0955-0674(00)00112-5
Bolle, C. (2004). The role of GRAS proteins in plant signal transduction and development. Planta 218, 683–692. doi: 10.1007/s00425-004-1203-z
Cakir, B., Agasse, A., Gaillard, C., Saumonneau, A., Delrot, S., Atanassova, R. (2003). A grape ASR protein involved in sugar and abscisic acid signaling. Plant Cell 15, 2165–2180. doi: 10.1105/tpc.013854
Campos, J. F., Cara, B., Perez-Martin, F., Pineda, B., Egea, I., Flores, F. B., et al. (2016). The tomato mutant ars1 (altered response to salt stress 1) identifies an R1-type MYB transcription factor involved in stomatal closure under salt acclimation. Plant Biotechnol. J. 14, 1345–1356. doi: 10.1111/pbi.12498
Changan, S. S., Ali, K., Kumar, V., Garg, N. K., Tyagi, A. (2018). Abscisic acid biosynthesis under water stress: anomalous behavior of the 9-cis-epoxycarotenoid dioxygenase1 (NCED1) gene in rice. Biol. Plantarum 62, 663–670. doi: 10.1007/s10535-018-0807-2
Chen, Y., Chen, C., Tan, Z., Liu, J., Zhuang, L., Yang, Z., et al. (2016). Functional identification and characterization of genes cloned from halophyte seashore paspalum conferring salinity and cadmium tolerance. Front. Plant Sci. 7, 102. doi: 10.3389/fpls.2016.00102
Christensen, A. H., Quail, P. H. (1996). Ubiquitin promoter-based vectors for high-level expression of selectable and/or screenable marker genes in monocotyledonous plants. Transgenic Res. 5, 213–218. doi: 10.1007/BF01969712
Cutler, S. R., Rodriguez, P. L., Finkelstein, R. R., Abrams, S. R. (2010). Abscisic acid: emergence of a core signaling network. Annu. Rev. Plant Biol. 61, 651–679. doi: 10.1146/annurev-arplant-042809-112122
Desikan, R., Cheung, M. K., Bright, J., Henson, D., Hancock, J. T., Neill, S. J. (2004). ABA, hydrogen peroxide and nitric oxide signalling in stomatal guard cells. J. Exp. Bot. 55, 205–212. doi: 10.1093/jxb/erh033
Ding, X. P., Li, X. K., Xiong, L. Z. (2013). Insight into differential responses of upland and paddy rice to drought stress by comparative expression profiling analysis. Int. J. Mol. Sci. 14, 5214–5238. doi: 10.3390/ijms14035214
Dominguez, P. G., Carrari, F. (2015). ASR1 transcription factor and its role in metabolism. Plant Signal Behav. 10, e992751. doi: 10.4161/15592324.2014.992751
Duan, J. Z., Zhang, M. H., Zhang, H. L., Xiong, H. Y., Liu, P. L., Ali, J., et al. (2012). OsMIOX, a myo-inositol oxygenase gene, improves drought tolerance through scavenging of reactive oxygen species in rice (Oryza sativa L.). Plant Sci. 196, 143–151. doi: 10.1016/j.plantsci.2012.08.003
El-Esawi, M. A., Alayafi, A. A. (2019). Overexpression of rice Rab7 gene improves drought and heat tolerance and increases grain yield in rice (Oryza sativa L.). Genes (Basel) 10, 56. doi: 10.3390/genes10010056
Estrada-Melo, A. C., Ma, C., Reid, M. S., Jiang, C. Z. (2015). Overexpression of an ABA biosynthesis gene using a stress-inducible promoter enhances drought resistance in petunia. Hortic. Res. 2, 15013. doi: 10.1038/hortres.2015.13
Feller, A., Machemer, K., Braun, E. L., Grotewold, E. (2011). Evolutionary and comparative analysis of MYB and bHLH plant transcription factors. Plant J. 66, 94–116. doi: 10.1111/j.1365-313X.2010.04459.x
Flowers, T. J. (2004). Improving crop salt tolerance. J. Exp. Bot. 55, 307–319. doi: 10.1093/jxb/erh003
Fujita, Y., Fujita, M., Shinozaki, K., Yamaguchi-Shinozaki, K. (2011). ABA-mediated transcriptional regulation in response to osmotic stress in plants. J. Plant Res. 124, 509–525. doi: 10.1007/s10265-011-0412-3
Gampala, S. S., Finkelstein, R. R., Sun, S. S., Rock, C. D. (2002). ABI5 interacts with abscisic acid signaling effectors in rice protoplasts. J. Biol. Chem. 277, 1689–1694. doi: 10.1074/jbc.M109980200
Gao, T., Wu, Y., Zhang, Y., Liu, L., Ning, Y., Wang, D., et al. (2011). OsSDIR1 overexpression greatly improves drought tolerance in transgenic rice. Plant Mol. Biol. 76, 145–156. doi: 10.1007/s11103-011-9775-z
Gao, X., Ren, Z., Zhao, Y., Zhang, H. (2003). Overexpression of SOD2 increases salt tolerance of Arabidopsis. Plant Physiol. 133, 1873–1881. doi: 10.1104/pp.103.026062
Gao, Y., Jiang, W., Dai, Y., Xiao, N., Zhang, C., Li, H., et al. (2015). A maize phytochrome-interacting factor 3 improves drought and salt stress tolerance in rice. Plant Mol. Biol. 87, 413–428. doi: 10.1007/s11103-015-0288-z
Godfray, H. C., Beddington, J. R., Crute, I. R., Haddad, L., Lawrence, D., Muir, J. F., et al. (2010). Food security: the challenge of feeding 9 billion people. Science 327, 812–818. doi: 10.1126/science.1185383
Golan, I., Dominguez, P. G., Konrad, Z., Shkolnik-Inbar, D., Carrari, F., Bar-Zvi, D. (2014). Tomato Abscisic Acid Stress Ripening (ASR) gene family revisited. PloS One 9, e107117. doi: 10.1371/journal.pone.0107117
Golldack, D., Li, C., Mohan, H., Probst, N. (2014). Tolerance to drought and salt stress in plants: unraveling the signaling networks. Front. Plant Sci. 5, 151. doi: 10.3389/fpls.2014.00151
Henry, I. M., Carpentier, S. C., Pampurova, S., Van Hoylandt, A., Panis, B., Swennen, R., et al. (2011). Structure and regulation of the Asr gene family in banana. Planta 234, 785–798. doi: 10.1007/s00425-011-1421-0
Hetherington, A. M., Woodward, F. I. (2003). The role of stomata in sensing and driving environmental change. Nature 424, 901–908. doi: 10.1038/nature01843
Hiei, Y., Ohta, S., Komari, T., Kumashiro, T. (1994). Efficient transformation of rice (Oryza sativa L.) mediated by Agrobacterium and sequence analysis of the boundaries of the T-DNA. Plant J. 6, 271–282. doi: 10.1046/j.1365-313X.1994.6020271.x
Hodges, D. M., Delong, J. M., Forney, C. F., Prange, R. K. (1999). Improving the thiobarbituric acid-reactive-substances assay for estimating lipid peroxidation in plant tissues containing anthocyanin and other interfering compounds. Planta 207, 604–611. doi: 10.1007/s004250050524
Hong, S. H., Kim, I. J., Yang, D. C., Chung, W. I. (2002). Characterization of an abscisic acid responsive gene homologue from Cucumis melo. J. Exp. Bot. 53, 2271–2272. doi: 10.1093/jxb/erf075
Hu, H., Xiong, L. (2014). Genetic engineering and breeding of drought-resistant crops. Annu. Rev. Plant Biol. 65, 715–741. doi: 10.1146/annurev-arplant-050213-040000
Hu, H., Dai, M., Yao, J., Xiao, B., Li, X., Zhang, Q., et al. (2006). Overexpressing a NAM, ATAF, and CUC (NAC) transcription factor enhances drought resistance and salt tolerance in rice. Proc. Natl. Acad. Sci. U.S.A. 103, 12987–12992. doi: 10.1073/pnas.0604882103
Hu, W., Huang, C., Deng, X., Zhou, S., Chen, L., Li, Y., et al. (2013). TaASR1, a transcription factor gene in wheat, confers drought stress tolerance in transgenic tobacco. Plant Cell Environ. 36, 1449–1464. doi: 10.1111/pce.12074
Hu, Y., Schmidhalter, U. (2005). Drought and salinity: a comparison of their effects on mineral nutrition of plants. J. Plant Nutr. Soil Sci. 168, 541–549. doi: 10.1002/jpln.200420516
Huang, X. Y., Chao, D. Y., Gao, J. P., Zhu, M. Z., Shi, M., Lin, H. X. (2009). A previously unknown zinc finger protein, DST, regulates drought and salt tolerance in rice via stomatal aperture control. Genes Dev. 23, 1805–1817. doi: 10.1101/gad.1812409
Irigoyen, J. J., Einerich, D. W., Sánchez-Díaz, M. (1992). Water stress induced changes in concentrations of proline and total soluble sugars in nodulated alfalfa (Medicago sativd) plants. Physiol. Plant 84, 55–60. doi: 10.1111/j.1399-3054.1992.tb08764.x
Iusem, N. D., Bartholomew, D. M., Hitz, W. D., Scolnik, P. A. (1993). Tomato (Lycopersicon esculentum) transcript induced by water deficit and ripening. Plant Physiol. 102, 1353–1354. doi: 10.1104/pp.102.4.1353
Jeanneau, M., Gerentes, D., Foueillassar, X., Zivy, M., Vidal, J., Toppan, A., et al. (2002). Improvement of drought tolerance in maize: towards the functional validation of the Zm-Asr1 gene and increase of water use efficiency by over-expressing C4-PEPC. Biochimie 84, 1127–1135. doi: 10.1016/S0300-9084(02)00024-X
Jha, B., Lal, S., Tiwari, V., Yadav, S. K., Agarwal, P. K. (2012). The SbASR-1 gene cloned from an extreme halophyte Salicornia brachiata enhances salt tolerance in transgenic tobacco. Mar. Biotechnol. (N. Y.) 14, 782–792. doi: 10.1007/s10126-012-9442-7
Jia, H., Jiu, S., Zhang, C., Wang, C., Tariq, P., Liu, Z., et al. (2016). Abscisic acid and sucrose regulate tomato and strawberry fruit ripening through the abscisic acid-stress-ripening transcription factor. Plant Biotechnol. J. 14, 2045–2065. doi: 10.1111/pbi.12563
Jin, J., Huang, W., Gao, J. P., Yang, J., Shi, M., Zhu, M. Z., et al. (2008). Genetic control of rice plant architecture under domestication. Nat. Genet. 40, 1365–1369. doi: 10.1038/ng.247
Joo, J., Lee, Y. H., Kim, Y. K., Nahm, B. H., Song, S. I. (2013). Abiotic stress responsive rice ASR1 and ASR3 exhibit different tissue-dependent sugar and hormone-sensitivities. Mol. Cells 35, 421–435. doi: 10.1007/s10059-013-0036-7
Kim, I. S., Kim, Y. S., Yoon, H. S. (2012). Rice ASR1 protein with reactive oxygen species scavenging and chaperone-like activities enhances acquired tolerance to abiotic stresses in Saccharomyces cerevisiae. Mol. Cells 33, 285–293. doi: 10.1007/s10059-012-2253-x
Koenig, D., Jimenez-Gomez, J. M., Kimura, S., Fulop, D., Chitwood, D. H., Headland, L. R., et al. (2013). Comparative transcriptomics reveals patterns of selection in domesticated and wild tomato. Proc. Natl. Acad. Sci. U.S.A. 110, E2655–E2662. doi: 10.1073/pnas.1309606110
Konrad, Z., Bar-Zvi, D. (2008). Synergism between the chaperone-like activity of the stress regulated ASR1 protein and the osmolyte glycine-betaine. Planta 227, 1213–1219. doi: 10.1007/s00425-008-0693-5
Kuromori, T., Seo, M., Shinozaki, K. (2018). ABA transport and plant water stress responses. Trends Plant Sci. 23, 513–522. doi: 10.1016/j.tplants.2018.04.001
Lenka, S. K., Katiyar, A., Chinnusamy, V., Bansal, K. C. (2011). Comparative analysis of drought-responsive transcriptome in Indica rice genotypes with contrasting drought tolerance. Plant Biotechnol. J. 9, 315–327. doi: 10.1111/j.1467-7652.2010.00560.x
Li, J., Li, Y., Yin, Z., Jiang, J., Zhang, M., Guo, X., et al. (2017). OsASR5 enhances drought tolerance through a stomatal closure pathway associated with ABA and H2O2 signalling in rice. Plant Biotechnol. J. 15, 183–196. doi: 10.1111/pbi.12601
Li, X., Qian, Q., Fu, Z., Wang, Y., Xiong, G., Zeng, D., et al. (2003). Control of tillering in rice. Nature 422, 618–621. doi: 10.1038/nature01518
Liang, W. H., Shang, F., Lin, Q. T., Lou, C., Zhang, J. (2013). Tillering and panicle branching genes in rice. Gene 537, 1–5. doi: 10.1016/j.gene.2013.11.058
Lim, C. W., Baek, W., Jung, J., Kim, J. H., Lee, S. C. (2015). Function of ABA in stomatal defense against biotic and drought stresses. Int. J. Mol. Sci. 16, 15251–15270. doi: 10.3390/ijms160715251
Lim, H., Hwang, H. J., Kim, A. R., Cho, M. H., Ji, H., Kim, C. K., et al. (2016). A simple, rapid and systematic method for the developed GM rice analysis. Plant Biotechnol. Rep. 10, 25–33. doi: 10.1007/s11816-015-0384-1
Lin, Z. F., Liu, N., Lin, G. Z., Peng, C. L. (2009). In situ localisation of superoxide generated in leaves of Alocasia macrorrhiza (L.) Shott under various stresses. J. Plant Biol. 52, 340–347. doi: 10.1007/s12374-009-9044-8
Liu, H. Y., Dai, J. R., Feng, D. R., Liu, B., Wang, H. B., Wang, J. F. (2010). Characterization of a novel plantain Asr gene, MpAsr, that is regulated in response to infection of Fusarium oxysporum f. sp. cubense and abiotic stresses. J. Integr. Plant Biol. 52, 315–323. doi: 10.1111/j.1744-7909.2010.00912.x
Livak, K. J., Schmittgen, T. D. (2001). Analysis of relative gene expression data using real-time quantitative PCR and the 2-∆∆Ct. Methods 25, 402–408. doi: 10.1006/meth.2001.1262
Manavalan, L. P., Chen, X., Clarke, J., Salmeron, J., Nguyen, H. T. (2012). RNAi-mediated disruption of squalene synthase improves drought tolerance and yield in rice. J. Exp. Bot. 63, 163–175. doi: 10.1093/jxb/err258
Maskin, L., Gubesblat, G. E., Moreno, J. E., Carrari, F. O., Frankel, N., Sambade, A., et al. (2001). Differential expression of the members of the Asr gene family in tomato (Lycopersicon esculentum). Plant Sci. 161, 739–746. doi: 10.1016/S0168-9452(01)00464-2
Mazel, A., Leshem, Y., Tiwari, B. S., Levine, A. (2004). Induction of salt and osmotic stress tolerance by overexpression of an intracellular vesicle trafficking protein AtRab7 (AtRabG3e). Plant Physiol. 134, 118–128. doi: 10.1104/pp.103.025379
Melhorn, V., Matsumi, K., Koiwai, H., Ikegami, K., Okamoto, M., Nambara, E., et al. (2008). Transient expression of AtNCED3 and AAO3 genes in guard cells causes stomatal closure in Vicia faba. J. Plant Res. 121, 125–131. doi: 10.1007/s10265-007-0127-7
Mishra, A., Tanna, B. (2017). Halophytes: potential resources for salt stress tolerance genes and promoters. Front. Plant Sci. 8, 829. doi: 10.3389/fpls.2017.00829
Mittler, R. (2006). Abiotic stress, the field environment and stress combination. Trends Plant Sci. 11, 15–19. doi: 10.1016/j.tplants.2005.11.002
Mohamed, R., Meilan, R., Ostry, M. E., Michler, C. H., Strauss, S. H. (2001). Bacterio-opsin gene overexpression fails to elevate fungal disease resistance in transgenic poplar (Populus). Can. J. For. Res. 31, 268–275. doi: 10.1139/cjfr-31-2-268
Munns, R., Tester, M. (2008). Mechanisms of salinity tolerance. Annu. Rev. Plant Biol. 59, 651–681. doi: 10.1146/annurev.arplant.59.032607.092911
Padaria, J. C., Yadav, R., Tarafdar, A., Lone, S. A., Kumar, K., Sivalingam, P. N. (2016). Molecular cloning and characterization of drought stress responsive abscisic acid-stress-ripening (Asr 1) gene from wild jujube, Ziziphus nummularia (Burm.f.) Wight & Arn. Mol. Biol. Rep. 43, 849–859. doi: 10.1007/s11033-016-4013-z
Park, H. Y., Seok, H. Y., Park, B. K., Kim, S. H., Goh, C. H., Lee, B. H., et al. (2008). Overexpression of Arabidopsis ZEP enhances tolerance to osmotic stress. Biochem. Biophys. Res. Commun. 375, 80–85. doi: 10.1016/j.bbrc.2008.07.128
Philippe, R., Courtois, B., Mcnally, K. L., Mournet, P., El-Malki, R., Le Paslier, M. C., et al. (2010). Structure, allelic diversity and selection of Asr genes, candidate for drought tolerance, in Oryza sativa L. and wild relatives. Theor. Appl. Genet. 121, 769–787.
Price, A. H., Cairns, J. E., Horton, P., Jones, H. G., Griffiths, H. (2002). Linking drought-resistance mechanisms to drought avoidance in upland rice using a QTL approach: progress and new opportunities to integrate stomatal and mesophyll responses. J. Exp. Bot. 53, 989–1004.
Qin, Y., Druzhinina, I. S., Pan, X., Yuan, Z. (2016). Microbially mediated plant salt tolerance and microbiome-based solutions for saline agriculture. Biotechnol. Adv. 34, 1245–1259.
Riccardi, F., Gazeau, P., De Vienne, D., Zivy, M. (1998). Protein changes in response to progressive water deficit in maize. Quantitative variation and polypeptide identification. Plant Physiol. 117, 1253–1263.
Roychoudhury, A., Roy, C., Sengupta, D. N. (2007). Transgenic tobacco plants overexpressing the heterologous lea gene Rab16A from rice during high salt and water deficit display enhanced tolerance to salinity stress. Plant Cell Rep. 26, 1839–1859.
Schroeder, J. I., Allen, G. J., Hugouvieux, V., Kwak, J. M., Waner, D. (2001). Guard cell signal transduction. Annu. Rev. Plant Physiol. Plant Mol. Biol. 52, 627–658.
Shen, G., Pang, Y., Wu, W., Deng, Z., Liu, X., Lin, J., et al. (2005). Molecular cloning, characterization and expression of a novel Asr gene from Ginkgo biloba. Plant Physiol. Biochem. 43, 836–843.
Tiwari, V., Chaturvedi, A. K., Mishra, A., Jha, B. (2015). Introgression of the SbASR-1 gene cloned from a halophyte Salicornia brachiate enhances salinity and drought endurance in transgenic groundnut (Arachis hypogaea)and acts as a transcription factor. PloS One 10, e0131567.
Tong, H., Chu, C. (2009). Roles of DLT in fine modulation on brassinosteroid response in rice. Plant Signal Behav. 4, 438–439.
Tong, H., Jin, Y., Liu, W., Li, F., Fang, J., Yin, Y., et al. (2009). Dwarf and Low-Tillering, a new member of the GRAS family, plays positive roles in brassinosteroid signaling in rice. Plant J. 58, 803–816.
Vaidyanathan, R., Kuruvilla, S., Thomas, G. (1999). Characterization and expression pattern of an abscisic acid and osmotic stress responsive gene from rice. Plant Sci. 140, 21–30.
Van Berkel, J., Salamini, F., Gebhardt, C. (1994). Transcripts accumulating during cold storage of potato (Solanum tuberosum L.) tubers are sequence related to stress-responsive genes. Plant Physiol. 104, 445–452.
Verma, V., Ravindran, P., Kumar, P. P. (2016). Plant hormone-mediated regulation of stress responses. BMC Plant Biol. 16, 86.
Wang, G., Zhang, S., Ma, X., Wang, Y., Kong, F., Meng, Q. (2016a). A stress-associated NAC transcription factor (SlNAC35) from tomato plays a positive role in biotic and abiotic stresses. Physiol. Plant 158, 45–64.
Wang, H. J., Hsu, C. M., Jauh, G. Y., Wang, C. S. (2005). A lily pollen ASR protein localizes to both cytoplasm and nuclei requiring a nuclear localization signal. Physiol. Plant 123, 314–320.
Wang, L., Hu, W., Feng, J., Yang, X., Huang, Q., Xiao, J., et al. (2016b). Identification of the ASR gene family from Brachypodium distachyon and functional characterization of BdASR1 in response to drought stress. Plant Cell Rep. 35, 1221–1234.
Wang, W., Liu, B., Xu, M., Jamil, M., Wang, G. (2015). ABA-induced CCCH tandem zinc finger protein OsC3H47 decreases ABA sensitivity and promotes drought tolerance in Oryza sativa. Biochem. Biophys. Res. Commun. 464, 33–37.
Wei, S., Hu, W., Deng, X., Zhang, Y., Liu, X., Zhao, X., et al. (2014). A rice calcium-dependent protein kinase OsCPK9 positively regulates drought stress tolerance and spikelet fertility. BMC Plant Biol. 14, 133.
Wren, J. J., Wiggall, P. H. (1965). An improved colorimetric method for the determination of proline in the presence of other ninhydrin-positive compounds. Biochem. J. 94, 216–220.
Wu, H. J., Zhang, Z. H., Wang, J. Y., Oh, D. H., Dassanayake, M., Liu, B. H., et al. (2012). Insights into salt tolerance from the genome of Thellungiella salsuginea. Proc. Natl. Acad. Sci. U.S.A. 109, 12219–12224.
Xiao, B., Huang, Y., Tang, N., Xiong, L. (2007). Over-expression of a LEA gene in rice improves drought resistance under the field conditions. Theor. Appl. Genet. 115, 35–46.
Yanez, M., Caceres, S., Orellana, S., Bastias, A., Verdugo, I., Ruiz-Lara, S., et al. (2009). An abiotic stress-responsive bZIP transcription factor from wild and cultivated tomatoes regulates stress-related genes. Plant Cell Rep. 28, 1497–1507.
Yang, C. Y., Chen, Y. C., Jauh, G. Y., Wang, C. S. (2005). A Lily ASR protein involves abscisic acid signaling and confers drought and salt resistance in Arabidopsis. Plant Physiol. 139, 836–846.
You, J., Zong, W., Li, X., Ning, J., Hu, H., Li, X., et al. (2013). The SNAC1-targeted gene OsSRO1c modulates stomatal closure and oxidative stress tolerance by regulating hydrogen peroxide in rice. J. Exp. Bot. 64, 569–583. doi: 10.1093/jxb/ers349
Zhang, L., Xiao, S., Li, W., Feng, W., Li, J., Wu, Z., et al. (2011). Overexpression of a Harpin-encoding gene hrf1 in rice enhances drought tolerance. J. Exp. Bot. 62, 4229–4238. doi: 10.1093/jxb/err131
Zhang, Y., Yu, C., Lin, J., Liu, J., Liu, B., Wang, J., et al. (2017). OsMPH1 regulates plant height and improves grain yield in rice. PloS One 12, e0180825. doi: 10.1371/journal.pone.0180825
Keywords: abscisic acid, abscisic acid-, stress-, and ripening-induced genes, grain yield, salt and drought stress tolerance, stomatal closure, transgenic rice plant
Citation: Park S-I, Kim J-J, Shin S-Y, Kim Y-S and Yoon H-S (2020) ASR Enhances Environmental Stress Tolerance and Improves Grain Yield by Modulating Stomatal Closure in Rice. Front. Plant Sci. 10:1752. doi: 10.3389/fpls.2019.01752
Received: 25 July 2019; Accepted: 13 December 2019;
Published: 14 February 2020.
Edited by:
Sonia Negrao, University College Dublin, IrelandReviewed by:
Vivekanand Tiwari, Agricultural Research Organization (ARO), IsraelCopyright © 2020 Park, Kim, Shin, Kim and Yoon. This is an open-access article distributed under the terms of the Creative Commons Attribution License (CC BY). The use, distribution or reproduction in other forums is permitted, provided the original author(s) and the copyright owner(s) are credited and that the original publication in this journal is cited, in accordance with accepted academic practice. No use, distribution or reproduction is permitted which does not comply with these terms.
*Correspondence: Young-Saeng Kim, a3lzbGhoMTIyOEBoYW5tYWlsLm5ldA==; Ho-Sung Yoon, aHN5QGtudS5hYy5rcg==
Disclaimer: All claims expressed in this article are solely those of the authors and do not necessarily represent those of their affiliated organizations, or those of the publisher, the editors and the reviewers. Any product that may be evaluated in this article or claim that may be made by its manufacturer is not guaranteed or endorsed by the publisher.
Research integrity at Frontiers
Learn more about the work of our research integrity team to safeguard the quality of each article we publish.