- 1Graduate School of Biotechnology and Crop Biotech Institute, Kyung Hee University, Yongin, South Korea
- 2Institute of Plant Biochemistry, Cluster of Excellence on Plant Sciences, Heinrich Heine University, Düsseldorf, Germany
- 3Department of Molecular Biology, Pusan National University, Busan, South Korea
Ribulose-1,5-bisphosphate carboxylase/oxygenase, the key enzyme of photosynthetic carbon fixation, is able to accept both O2 and CO2 as substrates. When it fixes O2, it produces 2-phosphoglycolate, which is detoxified by photorespiration and recycled to the Calvin–Benson–Bassham cycle. To complete photorespiration, metabolite transport across three organelles, chloroplasts, peroxisomes, and mitochondria, is necessary through transmembrane transporters. In rice (Oryza sativa) little is known about photorespiratory transmembrane transporters. Here, we identified the rice plastidic glycolate/glycerate translocator 1 (OsPLGG1), a homolog of Arabidopsis PLGG1. OsPLGG1 mutant lines, osplgg1-1, osplgg1-2, and osplgg1-3, showed a growth retardation phenotype, such as pale green leaf, reduced tiller number, and reduced seed grain weight as well as reduced photosynthetic carbon reduction rate due to low activities of photosystem I and II. The plant growth retardation in osplgg1 mutants was rescued under high CO2 condition. Subcellular localization of OsPLGG1–GFP fusion protein, along with its predicted N-terminal transmembrane domain, confirmed that OsPLGG1 is a chloroplast transmembrane protein. Metabolite analysis indicated significant accumulation of photorespiratory metabolites, especially glycolate and glycerate, which have been shown to be transported by the Arabidopsis PLGG1, and changes for a number of metabolites which are not intermediates of photorespiration in the mutants. These results suggest that OsPLGG1 is the functional plastidic glycolate/glycerate transporter, which is necessary for photorespiration and growth in rice.
Introduction
Ribulose-1,5-bisphosphate carboxylase/oxygenase (Rubisco) fixes the carbon from CO2 to carboxylate ribulose 1,5-bisphosphate (RuBP) during photosynthesis. After the oxygen contents of the atmosphere increased as a consequence of oxygenic photosynthesis by cyanobacteria, algae, and plants, O2 began to compete with CO2 for binding to Rubisco, which has binding affinity to both CO2 and O2. During fixation of O2 the toxic compound 2-phosphoglycolate (2-PG) is produced (Anderson, 1971), which is detoxified to 3-phosphoglycerate (3-PGA) by photorespiration and recycled to the Calvin–Benson–Bassham cycle. Thus, although photorespiration is considered as a wasteful pathway, it comprises the second largest carbon flux followed by photosynthesis in nature (Sharkey, 1988).
The photorespiration pathway is partitioned across multiple organelles, namely chloroplasts, peroxisomes, mitochondria, and the cytosol. In chloroplasts, especially in the stroma, 2-PG phosphatase (PGP) hydrolyzes 2-PG to glycolate, which is exported to peroxisomes across the membranes. Glycolate oxidase (GOX) irreversibly oxidizes glycolate to glyoxylate in peroxisomes, whereas H2O2 as a byproduct is decomposed by catalase (CAT). Then, two enzymes, serine:glyoxylate aminotransferase (SGT) and glutamate:glyoxylate aminotransferase (GGT) independently transaminate glyoxylate to glycine. As the amino donors, SGT more likely uses serine and GGT uses glutamate or alanine with a preference of glutamate over alanine. Glycine then moves to mitochondria where it is converted to serine, CO2, and ammonia through a combined reaction of deamination and decarboxylation by serine hydroxymethyltransferase (SHMT) and glycine decarboxylase (GDC). Serine is transported back to peroxisomes where the amino group is donated to glyoxylate by SGT and hydroxypyruvate is produced. The hydroxypyruvate is then reduced to glycerate by hydroxypyruvate reductase (HPR). Glycerate goes back to chloroplasts and is phosphorylated by glycerate kinase (GLYK) to produce 3-PGA, which is finally recycled to RuBP. In this pathway, one molecule of CO2 is released and three carbons are recycled to the Calvin–Benson–Bassham cycle from two molecules of 2-PG (Ogren, 1984; Bauwe et al., 2010; Eisenhut et al., 2019).
Since photorespiration occurs in different organelles, numerous membrane transport steps are necessary for function of the pathway (Reumann and Weber, 2006; Peterhansel et al., 2010). Although the photorespiratory pathway itself and its required enzymes have been well characterized, relatively little is known about the transport proteins that catalyze the metabolic flux between the involved compartments. For instance, in Arabidopsis the plastidic DiT1 and DiT2 were firstly identified as 2-oxoglutarate/malate translocator and glutamate/malate translocator, respectively, which are involved in nitrogen recycling during photorespiration (Somerville and Ogren, 1983; Somerville and Somerville, 1985; Renné et al., 2003; Schneidereit et al., 2006). A mitochondrial transporter A BOUT DE SOUFFLE (BOU) transports glutamate necessary for proper glycine decarboxylase activity (Eisenhut et al., 2013; Porcelli et al., 2018). It was recently proposed that Arabidopsis UCP1 and UCP2, homologs of the mammalian uncoupling protein 1, catalyze aspartate/glutamate exchange across the mitochondrial membrane and contribute to the export of reducing equivalents from the mitochondria in photorespiration (Monné et al., 2018). Earlier publications assumed that the Arabidopsis plastidic glycolate/glycerate transporter 1 (PLGG1) is involved in chloroplast development or functions against cell death due to the visible mutant phenotype of chlorotic leaves in which chloroplasts are destroyed (Yamaguchi et al., 2012; Yang et al., 2012). Later, PLGG1 was demonstrated to move two molecules of glycolate and one molecule of glycerate from/to chloroplasts, respectively (Pick et al., 2013). Thus, the visible symptom in plgg1 mutants is due to the accumulation of toxic concentrations of glycolate and glycerate. In addition, the Arabidopsis bile acid sodium symporter BASS6 was found to export glycolate from chloroplasts. Double mutants of PLGG1 and BASS6 showed an additive growth defect, an increase in glycolate accumulation, and reductions in photosynthetic rates compared with either single mutant (South et al., 2017).
To improve plant growth and biomass production by reducing photorespiration, synthetic pathways which bypass the original photorespiratory pathway have been created in transgenic plants (Kebeish et al., 2007; Maier et al., 2012; Shen et al., 2019; South et al., 2019). For instance, in Arabidopsis introduction of five genes which encode glycolate dehydrogenase, glyoxylate carboligase, and tartonic semialdehyde reductase from Escherichia coli resulted in increased biomass production (Kebeish et al., 2007). In rice, transgenic plants expressing OsGLO3, OsOXO3, and OsCATC encoding glycolate oxidase, oxalate oxidase, and catalase, respectively, that are directed into rice chloroplasts by the rice Rubisco small subunit (rbcS) transit peptide, displayed significant increase in their growth and biomass (Shen et al., 2019). Also, it was shown that applying an artificial pathway which involves targeting of pumpkin malate synthase and chlamydomonas glycolate dehydrogenase to chloroplasts in tobacco plants in which PLGG1 was down-regulated by antisense RNA increased biomass by 40% compared with wild type (WT) (South et al., 2019).
In this study, we identified rice OsPLGG1, an Arabidopsis AtPLGG1 homolog, determined its subcellular localization, and characterized OsPLGG1 mutants morphologically, physiologically, and biochemically. Our results demonstrate that OsPLGG1 functions as a plastidic glycolate/glycerate transporter. It is the first characterized membrane transporter functioning in photorespiration in rice.
Materials and Methods
Plant Materials
Japonica WT [cultivar (cv.) Dongjin] and all the mutant seeds were germinated and grown on 1/2 Murashige and Skoog (MS) media for 10 days, and subsequently transferred to soil. Rice plants were grown either in a greenhouse with a light/dark cycle of 14/10 h (30/20°C) or in an experimental field plot under natural environmental conditions in summer (Eom et al., 2011). To observe the phenotype of mutants under high CO2 condition, WT and osplgg1 mutants were grown with a light/dark cycle of 14/10 h (30/25°C) under either ambient or 0.3% CO2 condition in an environmental chamber.
Bioinformatics Analysis
The Arabidopsis Information Resource (TAIR, https://www.arabidopsis.org/) was used to obtain the genomic and amino acid sequences of AtPLGG1 (At1g32080). To determine the candidate genes in rice, the BLAST tool of NCBI (https://blast.ncbi.nlm.nih.gov/Blast.cgi) was used. To predict cDNAs, all available EST and cDNA data from NCBI as well as MSU Rice Genome Annotation Project version 7 were used.
Molecular Analysis of T-DNA Insertion Mutant Lines
T-DNA mutants for OsPLGG1 and OsPLGG2 were identified from our T-DNA insertional mutant pool (Rice Functional Genomic Express Database; http://signal.salk.edu/cgi-bin/RiceGE). Lines 1A22337, 2D21473, and 3A12105 were chosen for osplgg1-1, osplgg2-1, and osplgg2-2 mutants (cv. Dongjin), respectively (Jeon et al., 2000; Jeong et al., 2002; Jeong et al., 2006). To isolate the homozygous T-DNA mutant lines for OsPLGG1 and OsPLGG2, genomic DNA PCR analysis was performed with primers G1F, G1R, G2F, G2R, T1, T2, and T3 (Table S1). Primer pairs G1F/G1R and G2F/G2R were used to detect the WT copy. Primer pairs T1/G1R, T2/G2R, and T3/G2R were used for T-DNA insertion mutant alleles of osplgg1-1, osplgg2-1, and osplgg2-2, respectively. OsPLGG1 and OsPLGG2 transcript abundances were measured in the respective mutants via quantitative RT-PCR using primer pairs RT1F/RT1R and RT2F/RT2R, respectively (Table S1). Rice Ubiquitin5 (OsUBQ5; LOC_Os01g22490) was used as a loading control with primers UBQ5F/UBQ5R (Table S1).
Expression of GFP Fusion Protein in Maize Protoplast
To determine subcellular localization of OsPLGG1, the OsPLGG1 full-length cDNA without stop codon was amplified with primer pairs FLCF/FLCR (Table S1). The resulting cDNA was fused in-frame to the Green Fluorescence Protein (GFP) gene by inserting it between the CaMV35S promoter and GFP of a binary vector pH7FWG2 (Karimi et al., 2005). The constructed vector was transformed into maize protoplasts (Cho et al., 2009). GFP was visualized by excitation with the 488 nm line of the argon laser and a capturing emission at 522 nm by laser-scanning confocal microscopy (LSM 510 META; Carl Zeiss, Jena, Germany).
Creation of CRISPR/Cas9 Mutant Lines
To determine an effective protospacer adjacent motif (PAM) and avoid any off-target for CRISPR-Cas9, the CRIPRdirect program (https://crispr.dbcls.jp/; Naito et al., 2015) and Centroidfold (http://rtools.cbrc.jp/centroidfold/) program were used. Two guide RNAs (5′-CCTCTTCTACGTCCCTTCCC-3′ and 5′-ACTGTACTGGGATACATGGT-3′) for osplgg1-2 and osplgg1-3, respectively, were cloned to the pOs-sgRNA vector (Miao et al., 2013). Each of the resulting vectors was cloned into a destination vector, pH-Ubi-cas9-7, using the Gateway™ system (Miao et al., 2013) and then introduced into WT (cv. Dongjin) using Agrobacterium-mediated transformation (Jeon et al., 2000). The mutations of target sites were detected by sequencing of the amplified PCR products with primer pairs MF/MR (Table S1).
Analysis of Photosynthetic Rate
Net photosynthetic carbon reduction activity was measured on the uppermost fully expanded leaves of 1-month-old rice plants grown in the paddy field using a portable gas-exchange system (Li-6400; Li-Cor, Lincoln, NE) at 1200 µmol m−2 s−1 photon flux density, leaf temperature of 25℃, and CO2 concentration of 350 µmol mol−1. Four biological replicates with three technical replicates each were tested for this analysis. Student's t-test was performed to show statistically significant differences.
Analysis of Chlorophyll Fluorescence
In vivo chlorophyll fluorescence kinetics were measured using dark-adapted (overnight) leaves of 1-month-old plants grown in the paddy field using an IMAGING-PAM M-Series chlorophyll fluorometer (Walz, Effeltrich, Germany). Fo delineating the minimum fluorescence was determined after turning on the measuring light, and Fm delineating the maximum fluorescence was determined during illumination with an 0.8 s saturating pulse using dark-adapted leaves. Fm′ delineating the maximum fluorescence was measured using leaves under actinic light illumination, and F delineating the fluorescence level was measured just before the determination of Fm′. The photochemical efficiency of photosystem (PS) II or Fv/Fm = (Fm − Fo)/Fm; the effective PS II quantum yield Y(II) = (Fm′ − F)/Fm′; the quantum yield of non-regulated energy dissipation, Y(NO) = F/Fm; and the quantum yield of regulated energy dissipation, Y(NPQ) = (F/Fm′) − (F/Fm); these equations were used during analyzing (Klughammer and Schreiber, 2008a). For high light stress, leaf segments were illuminated either in 900 µmol m−2 s−1 for 20 min or 1,800 µmol m−2 s−1 for 3 h. Fv/Fm was measured after dark-adaptation for 15 min.
Analysis of P700 Absorption
P700 was determined as described by Klughammer and Schreiber (2008b) with slight modifications using a pulse amplitude modulated fluorometer (PAM101/102/103, Walz, Effeltrich, Germany). Quantum yield of photochemical energy conversion of PS I, Y(I) = (Pm′ − P)/(Pm − Po); quantum yield of non-photochemical energy dissipation due to donor-side limitation of PS I, Y(ND) = (P − Po)/(Pm − Po); and quantum yield of non-photochemical energy dissipation due to acceptor-side limitation of PS I, Y(NA) = (Pm − Pm′)/(Pm − Po); these equations were used during analyzing. In these equations, Po is the zero P700 signal level, P is the intermediate P700 signal after illumination of actinic light (120 μmol photons m−2 s−1) for 5 min, Pm′ is the maximal P700 signal measured during actinic light illumination with an 0.8 s saturating pulse, and Pm is the maximal P700 signal measured again after full oxidation of P700 by illuminating far-red light.
Metabolite Analysis Using Gas Chromatography Mass Spectrometry (GC–MS)
Uppermost fully expanded leaves of 1-month-old WT and osplgg1 mutant plants grown in the paddy field were collected in the middle of the day, immediately frozen in liquid nitrogen, and stored at −80°C until further processing. Metabolites were analyzed via gas chromatography–mass spectrometry (GC–MS) using protocols adapted from Lisec et al. (2006) and Gu et al. (2012). Metabolites were extracted from 40–70 mg fresh weight of ground leaf materials using 1.5 ml CHCl3/CH3OH/H2O (1:2.5:1, v/v) mixture including 5 µM ribitol as internal standard pre-cooled to −20°C, then mixed on a rotator for 10 min and centrifuged at 20,000 g for 2 min at 4°C. A total of 50 µl of the supernatant were dried completely in a vacuum concentrator and derivatized in two steps via an MPS-Dual-head autosampler (Gerstel): (1) with 10 µl methoxyamine hydrochloride [Acros organics; freshly prepared at 20 mg ml−1 in pure pyridine (Sigma-Aldrich)] and shaking at 37°C for 90 min, (2) adding 90 µl N-Methyl-N-(trimethylsilyl)trifluoroacetamide (MSTFA; Macherey-Nagel) and shaking at 37°C for 30 min. After incubation for 2 h at room temperature, 1 µl of derivatized compounds was injected at a flow of 1 ml min−1 with an automatic liner exchange system in conjunction with a cold injection system (Gerstel) in splitless mode (ramping from 50 to 250°C at 12°C s−1) into the GC. Chromatography was performed using a 7890B GC system (Agilent Technologies) with a HP-5MS column with 5% phenyl methyl siloxane film (Agilent 19091S-433, 30 m length, 0.25 mm internal diameter, 0.25 µM film). The oven temperature was held constant at 70°C for 2 min and then ramped at 12.5°C min−1 to 320°C at which it was held constant for 5 min; resulting in a total run time of 27 min. Metabolites were ionized with an electron impact source at 70 V and 200°C source temperature and recorded in a mass range of m/z 60 to m/z 800 at 20 scans per sec with a 7200 GC-QTOF (Agilent Technologies). Metabolites were identified via MassHunter Qualitative (v b06, Agilent Technologies) by comparison of spectra to the NIST14 Mass Spectral Library (https://www.nist.gov/srd/nist-standard-reference-database-1a-v14). A standard mixture containing all target compounds at a concentration of 50 µM was processed in parallel to the samples as a response check and retention time reference. Peaks were integrated using MassHunter Quantitative (v b06, Agilent Technologies). For relative quantification, all metabolite peak areas were normalized to the corresponding fresh weight used for extraction and the peak area of the internal standard ribitol (Sigma-Aldrich). Three biological replicates with three technical replicates each were tested for this analysis. Student's t-test was performed to show statistically significant differences. Additional experimental details are provided in Data Sheet S1 following reporting standards suggested by Fernie et al. (2011).
Results
Identification of AtPLGG1 Homologs in Rice
To identify the PLGG1 candidate genes in the rice genome, we performed a BLAST search using the previously reported amino acid sequences for the AtPLGG1 (At1g32080) in Arabidopsis. We selected two candidates, named OsPLGG1 (LOC_Os01g32830) and OsPLGG2 (LOC_Os10g42780) from the NCBI database. While the annotation of OsPLGG1 was incorrect [MSU Rice Genome Annotation (Osa1) Release 7], the cDNA sequence (EEE54674.1) from a Chinese group appeared to correctly match that from our WT (cv. Dongjin) and aligned well with its homologs from many plant species (Yu et al., 2005; Wang and Bayles, 2013; Kang et al., 2016). Those two rice proteins showed identities of 76% and 69% to AtPLGG1, respectively (Figure S1). Heat map expression analysis of publicly available Affymetrix rice microarray data revealed leaf and shoot-preferential expression (Figure S2), suggesting their role in these organs/tissues.
Similar to AtPLGG1 which contains an N-terminal chloroplast targeting peptide followed by 12 transmembrane regions, SMART search (http://smart.embl-heidelberg.de/) indicated that OsPLGG1 and OsPLGG2 harbor 10 and 11 transmembrane helices, respectively. Kyoto Encyclopedia of Genes and Genomes search (KEGG, http://www.genome.jp/kegg/) also showed that like AtPLGG1, both contain LrgA and LrgB domains, components for bacterial cid/lrgAB operons which regulate bacterial programmed cell death and cell lysis (Brunskill and Bayles, 1996; Rice and Bayles, 2008). This suggests that OsPLGG1 and OsPLGG2 are likely membrane proteins. Moreover, TargetP Program (Emanuelsson et al., 2000; http://www.cbs.dtu.dk/services/TargetP) identified a predicted N-terminal chloroplast targeting peptide with 77 amino acids in OsPLGG1 but not in OsPLGG2. Thus, only OsPLGG1 appeared to retain the chloroplast targeting peptide that is absent in OsPLGG2 (Figure S1), suggesting that OsPLGG1 is a rice homolog of AtPLGG1, whereas OsPLGG2 may have a distinct function.
Characterization of OsPLGG1 and OsPLGG2 Mutants
To determine whether either OsPLGG1 or OsPLGG2 or both play a critical role in photorespiration, we isolated T-DNA insertion mutants for OsPLGG1 and OsPLGG2 from our T-DNA mutant population (Jeon et al., 2000; Jeong et al., 2002; Jeong et al., 2006). As a result, OsPLGG1 mutant, plgg1-1 carried a T-DNA insertion in the 5′-UTR (Figure 1A) and OsPLGG2 mutants, osplgg2-1 and osplgg2-2 carried T-DNA insertions at different positions in the third exon (Figure 1B). All homozygous mutants were isolated from their segregating progeny by genotyping using genomic DNA PCR with gene- and T-DNA-specific primers (Figure 1C). RT-PCR confirmed null expression of OsPLGG1 or OsPLGG2 in the homozygous mutants (Figure 1D).
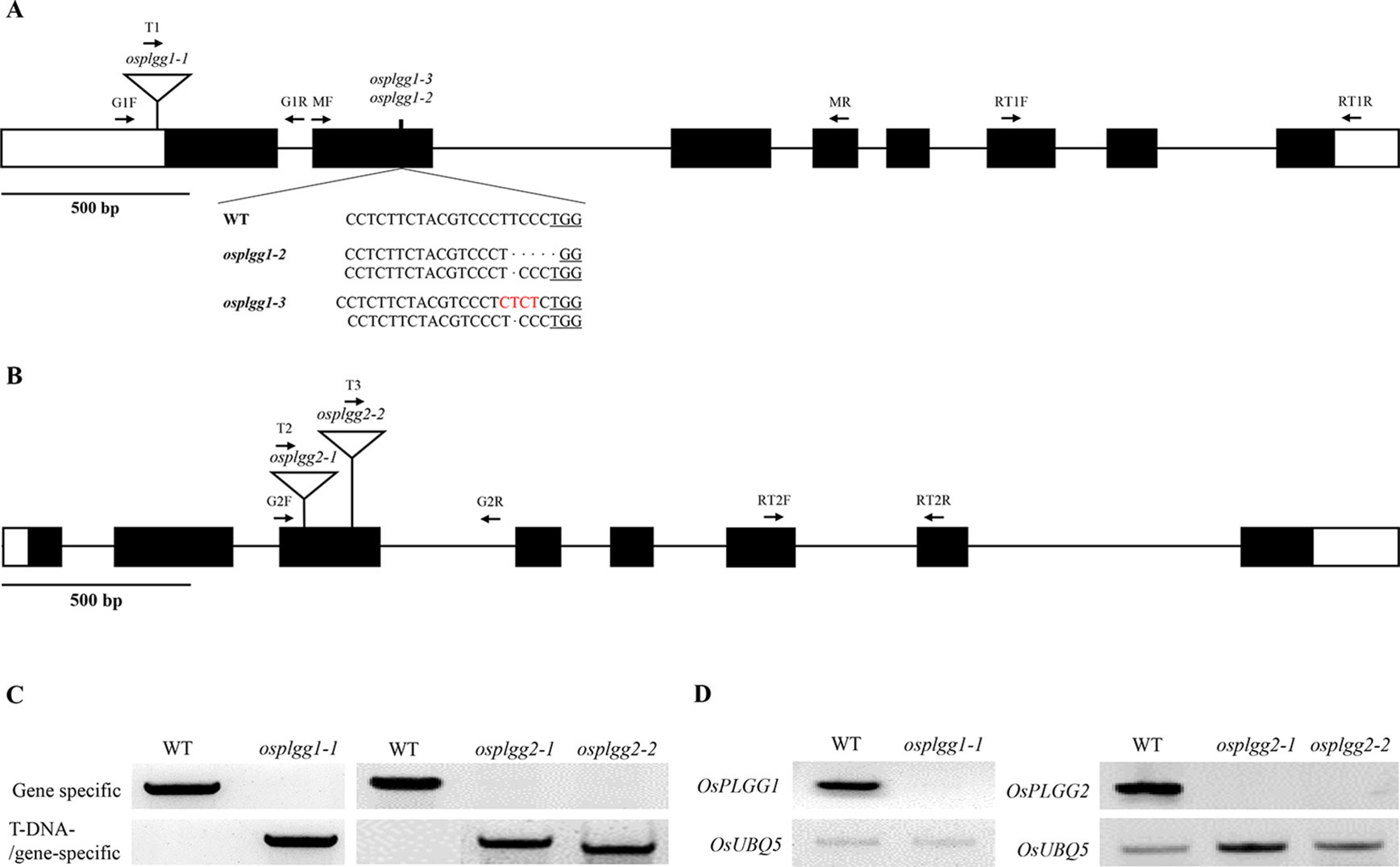
Figure 1 Characterization of the OsPLGG mutants. (A) Schematic representation of OsPLGG1 genomic structure with position of the T-DNA insertion (osplgg1-1) and Indel mutant alleles (osplgg1-2 and osplgg1-3) created by CRISPR/Cas9 in cv. Dongjin. The underlined sequence is the PAM target site. (B) Schematic representation of OsPLGG2 genomic structure with position of the T-DNA insertions (osplgg2-1 and osplgg2-2) in cv. Dongjin. Both OsPLGG1 and OsPLGG2 consist of eight exons (black boxes) and seven introns (line between the black boxes). Arrows indicate primer location for genomic DNA PCR and RT-PCR. (C) Genomic DNA PCR analysis of osplgg1-1 (left) and osplgg2-1 and osplgg2-2 (right). Gene specific primers (upper) amplify WT and T-DNA/gene-specific primers (lower) T-DNA insertion alleles. (D) RT-PCR analysis of osplgg1-1 (left) and osplgg2-1 and osplgg2-2 (right). OsUBQ5 was used as an internal control.
Only osplgg1-1 plants displayed pale green leaves from the early developmental stage and later the growth was severely retarded, yielding only a few tillers during maturation (Figures 2A, B). In osplgg1-1, the average number of tillers was about half (6.6) of WT (11.8) (Figure 2B), and 1000-grain weight was also reduced to 19.32 g compared to 23.37 g of WT (Figure 2C). Consistently with the pale green leaf, the photosynthetic carbon reduction rate was significantly reduced to 20% of WT in osplgg1-1 (Figure 2D). This growth retardation of osplgg1-1 mimicked that of atplgg1 grown under ambient air condition (Pick et al., 2013). Retarded growth phenotype of osplgg1-1 was rescued to levels comparable to WT under high CO2 (0.3%) in an environmental growth chamber (Figures 2E, F bottom), whereas the pale green leaves and growth retardation remained unchanged under ambient air condition (Figure 2F top). However, OsPLGG2 mutants, osplgg2-1 and osplgg2-2, did not exhibit any visible growth phenotype (Figure S3).
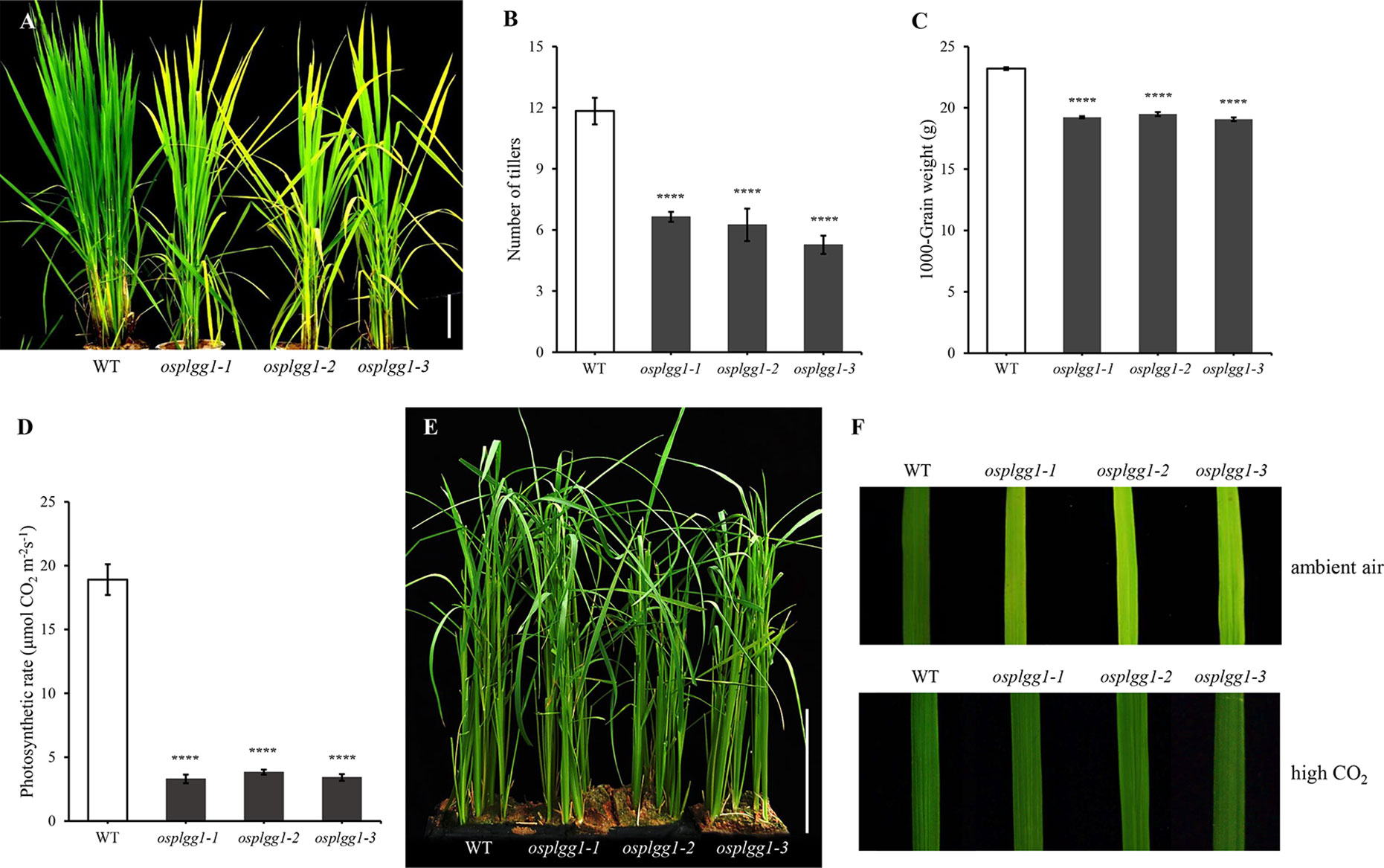
Figure 2 Phenotype analysis of osplgg1 mutants. (A) Mature plants of WT, osplgg1-1, osplgg1-2 and osplgg1-3 mutants grown for 2 months in the paddy field. Bar = 10 cm. (B, C) Number of tillers (B) and 1000-grain weight (C) of WT, osplgg1-1, osplgg1-2, and osplgg1-3 plants. The experiment consisted of five independent plants per each line. Error bars indicate the standard error of the mean (SEM). ****P < 0.0001. Student's t-test was used to show statistical differences. (D) Photosynthetic carbon reduction rate of WT, osplgg1-1, osplgg1-2, and osplgg1-3 in leaves of 1-month-old WT and osplgg1 mutant plants grown in the paddy field. Four independent biological replicates were used in the experiment, each having three technical replicates. Error bars indicate SEM. ****P < 0.0001. (E) Phenotypic recovery of osplgg1-1, osplgg1-2, and osplgg1-3 mutant plants grown under high CO₂ condition for 1 month in an environmental growth chamber. Bar = 10 cm. (F) Leaf blades of WT, osplgg1-1, osplgg1-2, and osplgg1-3 plants grown under ambient air (top) or high CO₂ condition (bottom) for 3 weeks in an environmental growth chamber.
To further confirm the growth retardation in osplgg1-1, we generated two additional OsPLGG1 mutant alleles via the CRISPR/Cas9 system. The PAM site in the second exon was selected as the target site. Among more than 30 homozygous mutant lines of OsPLGG1, only 10 survived as early growth of most of the primary mutant plants was very poor. We randomly selected two biallelic mutant plants for further experiments and designated them as osplgg1-2 (T deletion and TCCCT deletion relative to WT) and osplgg1-3 (T deletion and TCC to CTCT substitution) (Figure 1A). We predicted that T deletion and TCCCT deletion in osplgg1-2 induce severe changes after the 180th amino acid and premature stop codons at the 221st and the 232nd amino acid, respectively. In osplgg1-3, T deletion and TCC to CTCT substitution also cause severe changes after the 180th amino acid and premature stop codons at the 221st and 233rd amino acid, respectively.
Osplgg1-2 and osplgg1-3 both showed the same morphological phenotype with osplgg1-1. They displayed pale green leaves and growth retardation and had fewer tillers (Figures 2A, B). Similar to osplgg1-1, osplgg1-2 and osplgg1-3 had only five to six tillers (Figure 2B) and reduced 1000-grain weight (Figure 2C). We also found a similarly great reduction of the photosynthetic carbon reduction rate in osplgg1-2 and osplgg1-3 (Figure 2D). osplgg1-2 and osplgg1-3 also rescued plant growth to levels comparable to WT under high CO2 (0.3%) in an environmental growth chamber, whereas the pale green leaves and growth retardation remained unchanged under ambient air condition (Figures 2E, F). This result supports that OsPLGG1 deficiency resulted in plant growth retardation in rice.
The great reduction in photosynthetic carbon fixation rates per leaf area in OsPLGG1 mutants (Figure 2D) can partly be explained by the inactivation of PS I and/or PS II. Experiments under two different photoinhibitory illumination conditions (900 µmol m−2 s−1 for 20 min or 1,800 µmol m−2 s−1 for 3 h) revealed that PS IIs of OsPLGG1 mutants, osplgg1-1, osplgg1-2, and osplgg1-3, were very sensitive to high light stress, and PS IIs of the mutants were partly damaged even after overnight dark-adaptation (Table 1). Under actinic light illumination, the effective photochemical quantum yield of PS II, Y(II), was low and its recovery under darkness was slow in the mutants (Figure 3A). The osplgg1 mutants showed fast Y(NPQ) development kinetics in the light and slow decay kinetics in darkness (Figure 3B), and these differences were compensated in Y(NO), because the sum of these three parameters is equal to 0 (Figure 3C). The effective photochemical quantum yield of PS I, Y(I), was also low in the mutants (Figure 3D), and the low activities of PS II and PS I resulted in higher donor-side limitation, Y(ND), in the mutants compared with WT (Figure 3E). However, there was no significant difference in the degree of acceptor-side limitation, Y(NA), between WT and mutants (Figure 3F).
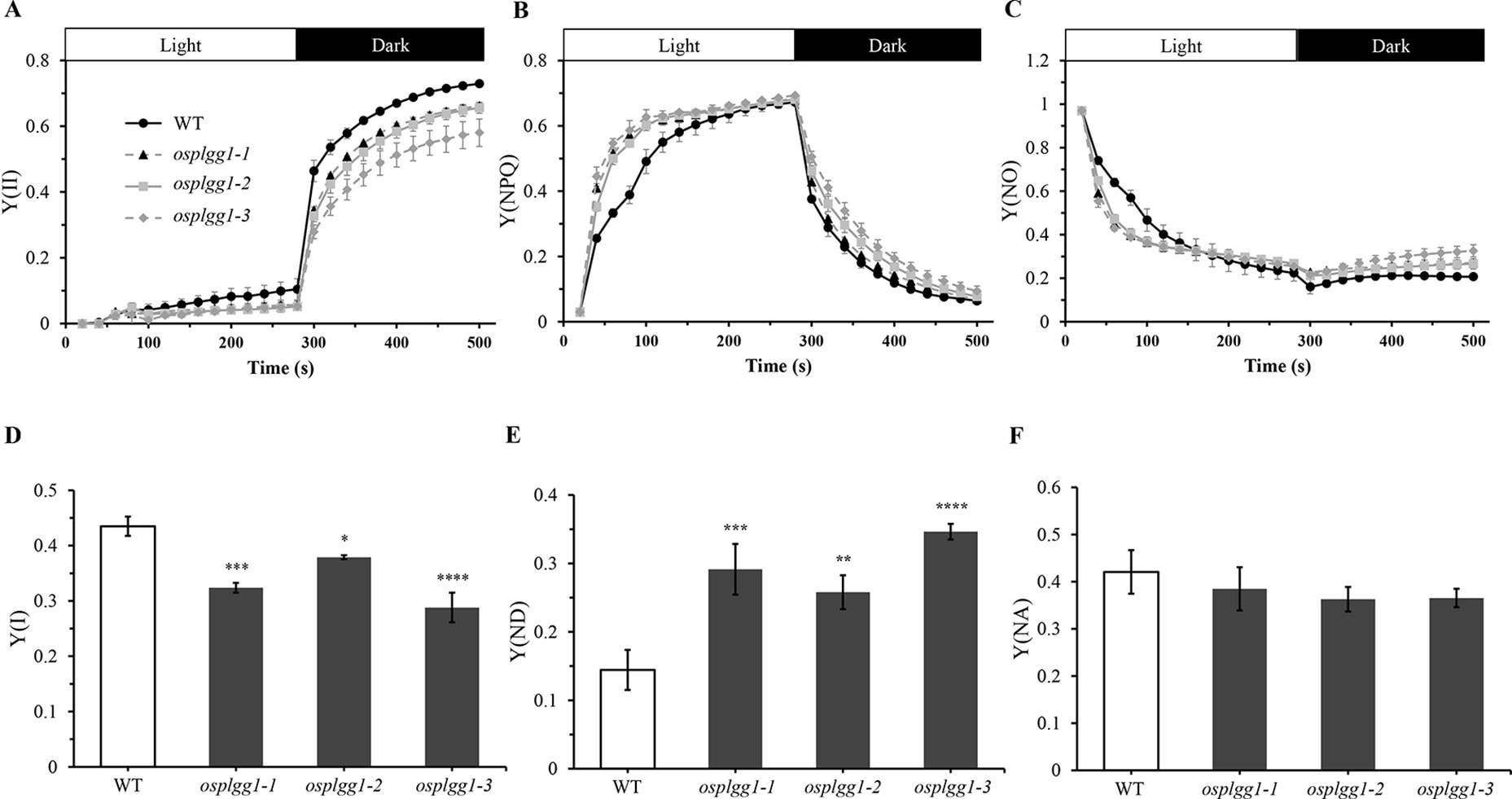
Figure 3 Analysis of PS II and PS I activities of osplgg1 mutants, osplgg1-1, osplgg1-2, and osplgg1-3. (A) Effective photochemical quantum yield of PS II, Y(II). (B) Quantum yield of regulated energy dissipation of PS II, Y(NPQ). (C) Quantum yield of non-regulated energy dissipation of PS II, Y(NO). Detached leaves were illuminated with actinic light (550 µmol m−2 s−1) for 280 s, followed by recovery in darkness for 280 s. Saturation pulse was applied in every 20 s. (D) Quantum yield of photochemical energy conversion in PS I, Y(I). (E) Quantum yield of non-photochemical energy dissipation due to donor-side limitation of PS I, Y(ND). (F) Quantum yield of non-photochemical energy dissipation due to acceptor-side limitation of PS I, Y(NA). Detached leaves were illuminated with actinic light (120 µmol m−2 s−1) for 5 min for the activation of Calvin–Benson–Bassham cycle before the measurement of maximal P700 signals. Three independent biological replicates were used in the experiment, each having four technical replicates. Error bars indicate SEM. Student's t-test was used to show statistical differences. *P < 0.05; **P < 0.01; ***P < 0.001; **** < 0.0001.
Subcellular Localization of OsPLGG1
According to the subcellular localization prediction, only OsPLGG1 retained an N-terminal chloroplast targeting peptide and its mutant mimicked the atplgg1 phenotype. To determine its in vivo subcellular localization, a full-length OsPLGG1 cDNA without stop codon was fused in-frame to GFP gene under the control of the CaMV35S promoter of a plant expression vector (Figure 4A). The resulting construct was transiently expressed in maize protoplasts. The green fluorescence signal observed by microscopy clearly overlapped with the outer layer of chlorophyll autofluorescence. This result supports the chloroplast membrane localization of OsPLGG1 (Figure 4B).
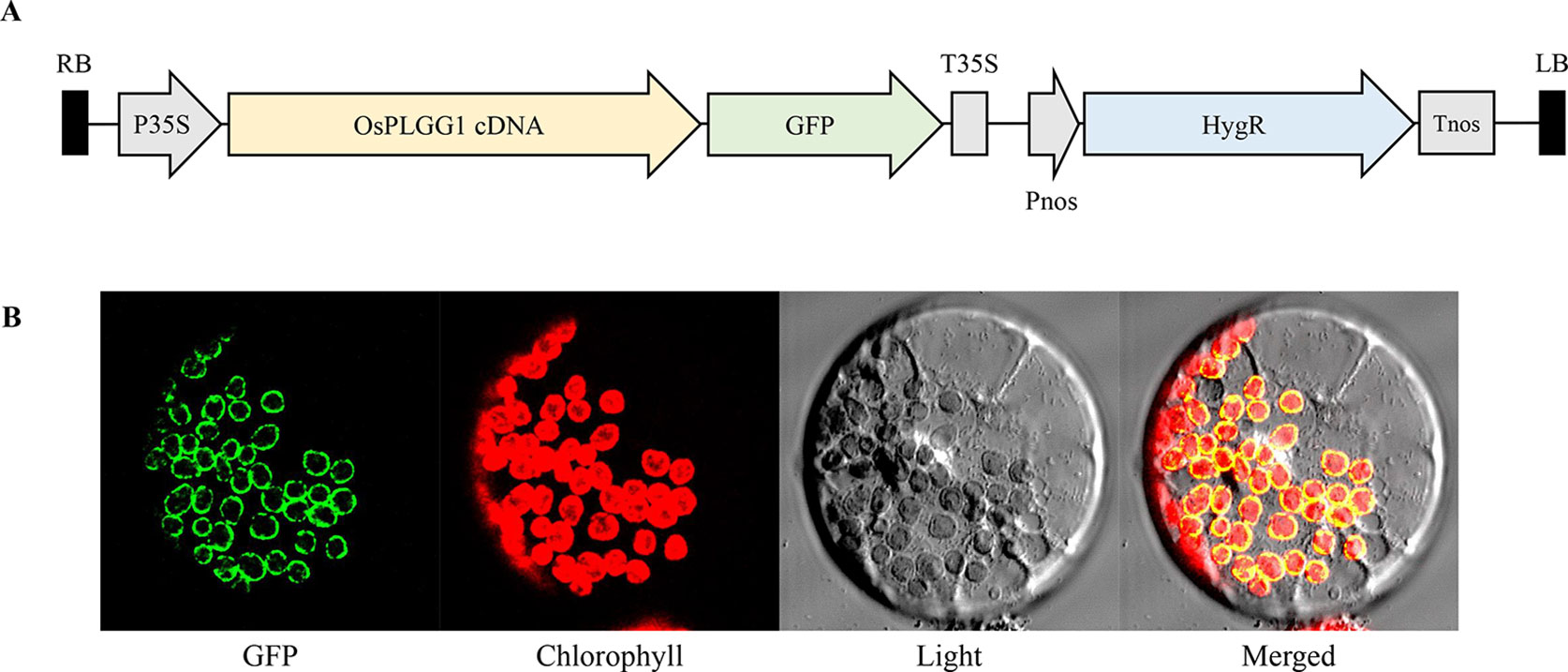
Figure 4 Subcellular localization of OsPLGG1-GFP in maize protoplast. (A) Schematic diagram of OsPLGG1-GFP fusion construct. P35S, CaMV35S promoter; T35S, CaMV35S terminator; Pnos, Nopaline synthase promoter; Tnos, Nopaline synthase terminator. HygR, Hygromycin phosphotransferase. (B) GFP localization in maize protoplast. Fluorescent GFP signals, chlorophyll autofluorescence, light microscope view, and a merged image are shown from left to right.
Changes in Photorespiratory Metabolites in Osplgg1 Mutants
To identify the role of OsPLGG1 in photorespiration, we analyzed photorespiratory metabolites in WT and three osplgg1 mutants. Since photorespiration occurs only during the day, all leaf samples were harvested at the middle of the day. Most of the photorespiratory intermediates, including glycerate and glycolate, were highly accumulated in osplgg1 mutants, which is well consistent with the observation in atplgg1 (Figure 5). In the mutants, significantly increased abundances of the photorespiratory metabolites glycerate (2.6-, 3.41-, and 3.69-fold), glycolate (25.87-, 32.43-, and 35.74-fold), glycine (2.5-, 1.2-, and 1.88-fold), and serine (14.73-, 6.19-, and 15.14-fold) were determined relative to WT for osplgg1-1, osplgg1-2, and osplgg1-3, respectively (Figure 5).
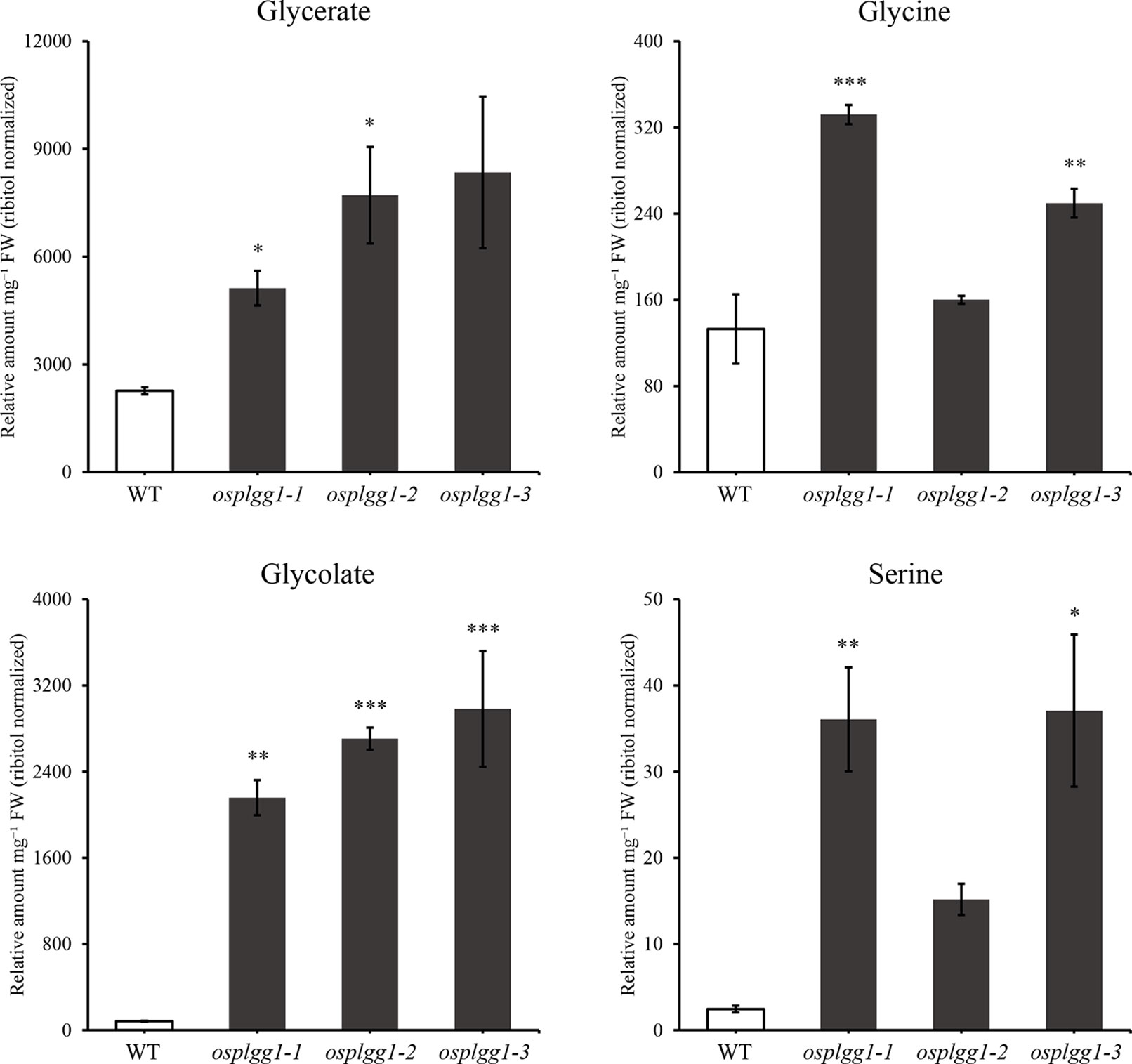
Figure 5 Contents of representative photorespiratory metabolites, glycerate, glycine, glycolate, and serine in leaves of 1-month-old WT and osplgg1 mutant plants grown in the paddy field. The relative metabolite levels were normalized to an internal standard (ribitol) and the fresh weight of the samples. Three independent biological replicates were used in the experiment, each having three technical replicates. Error bars indicate SEM. Student's t-test was used to show statistical differences. *P < 0.05; **P < 0.01; ***P < 0.001.
Differential Changes of Additional Metabolites Induced in Osplgg1 Mutants
In osplgg1 mutant leaves, we further analyzed a number of additional metabolites and found significant changes for some metabolites which are not intermediates of photorespiration (Figure S4). The levels of hydroxyglutarate, mannitol, and xylose were highly elevated, while those of GABA (gamma-aminobutyric acid), maltose, methionine, quinate, raffinose, shikimate, succinate, and tyrosine were significantly decreased in all three osplgg1 mutants (Figure S4). Hydroxyglutarate, mannitol, and xylose were increased to average 8.6-, 2.5-, and 2.2-fold, respectively in osplgg1 mutants. In contrast, osplgg1 plants showed declines of 3.7-, 2.8-, 4.4-, 8.2-, 6.8-, 2.3-, 2.5-, and 12.8-fold each in GABA, maltose, methionine, quinate, raffinose, shikimate, succinate, and tyrosine compared with WT (Figure S4).
Discussion
Photorespiration requires various transmembrane transporters residing in three organelles, chloroplasts, peroxisomes, and mitochondria, none of which have been identified in rice. Here, we report OsPLGG1 that functions as the plastidic glycolate/glycerate translocator in rice. In silico analysis of publicly available data proposed two candidates for the rice plastidic glycolate/glycerate translocator, OsPLGG1 and OsPLGG2 (Figure S1). Among the mutant plants for both genes, osplgg1 mutants only displayed severe growth retardation with pale-green leaves, less tillers, lower grain weight as well as reduced photosynthesis ability (Figures 2 and 3), which mimics the bleached lesions on the leaf lamina and growth retardation of Arabidopsis plgg1 mutant (Pick et al., 2013). The plant growth retardation in osplgg1 mutants was rescued under high (0.3%) CO2 condition (Figure 2). These results proposed OsPLGG1 as a functional homolog of AtPLGG1. In contrast, OsPLGG2 mutants, osplgg2-1 and osplgg2-2, did not show any visible phenotype (Figure S3). Chloroplast membrane localization was confirmed by detection of OsPLGG1-GFP in maize protoplasts (Figure 4). Metabolite analysis in osplgg1 mutants revealed significant accumulation of photorespiratory metabolites including glycolate and glycerate that have been shown to be transported by the Arabidopsis PLGG1 (Pick et al., 2013) (Figure 5). Therefore, accumulated glycolate and glycerate resulted from the blocked OsPLGG1 transporter most likely support that OsPLGG1 functions as glycolate/glycerate translocator across the chloroplast membrane. We speculate that reduced consumption of 2-PG in osplgg1 mutants leads to harmful effects on plants and eventually limited reduction of 2-PG to 3-PGA decreases the photosynthetic rate. In this regard, we could not observe any significant increase in the acceptor-side limitations in the mutants. Instead, there were significant decrease in effective photochemical quantum yield of PS II and PS I in the mutants (Figure 3). Changes in PS II photochemical efficiency or Fv/Fm revealed that PS II of the mutants was sensitive to photoinhibitory illumination (Table 1).
In osplgg1 mutant leaves, we found increased relative abundances of the photorespiratory metabolites, glycine and serine, and also significant changes for a number of metabolites which are not intermediates of photorespiration (Figure 5 and Figure S4). Photorespiration has been known to interact with associated processes such as nitrogen assimilation, one-carbon (C1) metabolism, sulfur metabolism, and the GABA shunt (Bauwe et al., 2010; Bloom et al., 2010; Peterhansel et al., 2010; Bauwe et al., 2012; Hodges et al., 2016; Obata et al., 2016; Samuilov et al., 2018; Eisenhut et al., 2019). Glycine decarboxylation by GDC releases ammonia and CO2 that are reassimilated. Thus, the accumulated glycine in osplgg1 mutants affected nitrogen metabolism. Serine is supplied for the cytosolic production of C1-compounds that are in turn required for C1 metabolism, which is essential for the synthesis of nucleic acids, proteins, pantothenate, and methylated molecules (Hanson and Roje, 2001). It is also probable that the accumulated serine may affect sulfur metabolism including cysteine biosynthesis in osplgg1 mutants. GABA, an intermediate of the GABA shunt pathway, was decreased in all three osplgg1 mutants (Figure S4). In addition, our metabolite analysis suggests that some metabolites associated with the TCA cycle, the pentose phosphate pathway, starch pathway, methionine synthesis, and the shikimate pathway (Figure S4) were affected likely as compensation for retarded plant growth. Nevertheless, to better understand the core photorespiratory pathway and its metabolic interdependency, detailed investigation including kinetic analysis of important metabolites is a prerequisite in photorespiratory mutants.
Improvement of photosynthetic rate is one of the main approaches for increased crop productivity. In this regard, a synthetic route for photorespiratory bypass produces more biomass and leads to increased crop yield (Kebeish et al., 2007; Shen et al., 2019). Interestingly, when a synthetic glycolate catabolic pathway was introduced into chloroplasts of WT tobacco and a line displaying reduced expression of PLGG1, the latter showed significantly enhanced plant growth (South et al., 2019). Identification of photorespiratory membrane transporters is a prerequisite for similarly engineering photorespiratory bypass routes to improve yield potential in crop plants including rice that can contribute to feed an increasing world population. Therefore, the OsPLGG1 discovered here may provide a good opportunity to improve productivity in rice as well as to understand photorespiration in rice.
Data Availability Statement
All datasets generated for this study are included in the article/Supplementary Material.
Author Contributions
J-SJ conceived and designed the experiments. S-HS, S-KL, D-WL, GW, and SK performed the experiments. S-HS, S-KL, D-WL, DB, C-HL, AW, and J-SJ analyzed the data. S-HS, S-KL, DB, C-HL, AW, and J-SJ wrote the paper.
Funding
This work was supported by a grant from the Next Generation BioGreen 21 Program of the Rural Development Administration of Korea (PJ013172). AW appreciates funding by the Deutsche Forschungsgemeinschaft (DFG, German Research Foundation) under Germany's Excellence Strategy—EXC-2048/1—Project ID: 390686111, and the Federal Ministry of Education and Research, Project FormatPlant (FKZ: 031B0194).
Conflict of Interest
The authors declare that the research was conducted in the absence of any commercial or financial relationships that could be construed as a potential conflict of interest.
Acknowledgments
We acknowledge the excellent technical assistance of Elisabeth Klemp, Katrin Weber, and Maria Graf for GC–MS measurements as well as Jessica Albers for manual data curation.
Supplementary Material
The Supplementary Material for this article can be found online at: https://www.frontiersin.org/articles/10.3389/fpls.2019.01726/full#supplementary-material
References
Anderson, L. E. (1971). Chloroplast and cytoplasmic enzymes II. Pea leaf triose phosphate isomerases. Biochim. Biophys. Acta - Enzymol. 235, 237–244. doi: 10.1016/0005-2744(71)90051-9
Bauwe, H., Hagemann, M., Fernie, A. R. (2010). Photorespiration: players, partners and origin. Trends Plant Sci. 15, 330–336. doi: 10.1016/j.tplants.2010.03.006
Bauwe, H., Hagemann, M., Kern, R., Timm, S. (2012). Photorespiration has a dual origin and manifold links to central metabolism. Curr. Opin. Plant Biol. 15, 269–275. doi: 10.1016/j.pbi.2012.01.008
Bloom, A. J., Burger, M., Asensio, J. S. R., Cousins, A. B. (2010). Carbon dioxide enrichment inhibits nitrate assimilation in wheat and Arabidopsis. Science 328, 899–904. doi: 10.1126/science.1186440
Brunskill, E. W., Bayles, K. W. (1996). Identification of LytSR-regulated genes from Staphylococcus aureus. J. Bacteriol. 178, 5810–5812. doi: 10.1128/jb.178.19.5810-5812.1996
Cho, J. I., Ryoo, N., Eom, J. S., Lee, D. W., Kim, H. B., Jeong, S. W., et al. (2009). Role of the rice hexokinases OsHXK5 and OsHXK6 as glucose sensors. Plant Physiol. 149, 745–759. doi: 10.1104/pp.108.131227
Eisenhut, M., Planchais, S., Cabassa, C., Guivarc'H, A., Justin, A. M., Taconnat, L., et al. (2013). Arabidopsis A BOUT de SOUFFLE is a putative mitochondrial transporter involved in photorespiratory metabolism and is required for meristem growth at ambient CO2 levels. Plant J. 73, 836–849. doi: 10.1111/tpj.12082
Eisenhut, M., Roell, M., Weber, A. P. (2019). Mechanistic understanding of photorespiration paves the way to a new green revolution. New Phytol. 223, 1762–1769. doi: 10.1111/nph.15872
Emanuelsson, O., Nielsen, H., Brunak, S., Von Heijne, G. (2000). Predicting subcellular localization of proteins based on their N-terminal amino acid sequence. J. Mol. Biol. 300, 1005–1016. doi: 10.1006/jmbi.2000.3903
Eom, J.-S., Cho, J.-I., Reinders, A., Lee, S.-W., Yoo, Y., Tuan, P. Q., et al. (2011). Impaired function of the tonoplast-localized sucrose transporter in Rice, OsSUT2, limits the transport of vacuolar reserve sucrose and affects plant growth. Plant Physiol. 157, 109–119. doi: 10.1104/pp.111.176982
Fernie, A. R., Aharoni, A., Willmitzer, L., Stitt, M., Tohge, T., Kopka, J., et al. (2011). Recommendations for reporting metabolite data. Plant Cell 23, 2477–2482. doi: 10.1105/tpc.111.086272
Gu, J., Weber, K., Klemp, E., Winters, G., Franssen, S. U., Wienpahl, I., et al. (2012). Identifying core features of adaptive metabolic mechanisms for chronic heat stress attenuation contributing to systems robustness. Integr. Biol. 4, 480–493. doi: 10.1039/c2ib00109h
Hanson, A. D., Roje, S. (2001). One-carbon metabolism in higher plants. Annu. Rev. Plant Physiol. Plant Mol. Biol. 52, 119–137. doi: 10.1146/annurev.arplant.52.1.119
Hodges, M., Dellero, Y., Keech, O., Betti, M., Raghavendra, A. S., Sage, R., et al. (2016). Perspectives for a better understanding of the metabolic integration of photorespiration within a complex plant primary metabolism network. J. Exp. Bot. 67, 3015–3026. doi: 10.1093/jxb/erw145
Jeon, J. S., Lee, S., Jung, K. H., Jun, S. H., Jeong, D. H., Lee, J., et al. (2000). T-DNA insertional mutagenesis for functional genomics in rice. Plant J. 22, 561–570. doi: 10.1046/j.1365-313x.2000.00767.x
Jeong, D.-H., An, S., Kang, H.-G., Moon, S., Han, J.-J., Park, S., et al. (2002). T-DNA insertional mutagenesis for activation tagging in rice. Plant Physiol. 130, 1636–1644. doi: 10.1111/j.1365-313X.2005.02610.x
Jeong, D. H., An, S., Park, S., Kang, H. G., Park, G. G., Kim, S. R., et al. (2006). Generation of a flanking sequence-tag database for activation-tagging lines in japonica rice. Plant J. 45, 123–132. doi: 10.1104/pp.014357
Kang, X., Huang, W., Tang, N., Li, Z. (2016). Overexpression of tomato homolog of glycolate/glycerate transporter gene PLGG1/AtLrgB leads to reduced chlorophyll biosynthesis. J. Plant Growth Regul. 35, 792–802. doi: 10.1007/s00344-016-9576-3
Karimi, M., De Meyer, B., Hilson, P. (2005). Modular cloning in plant cells. Trends Plant Sci. 10, 103–105. doi: 10.1016/j.tplants.2005.01.008
Kebeish, R., Niessen, M., Thiruveedhi, K., Bari, R., Hirsch, H. J., Rosenkranz, R., et al. (2007). Chloroplastic photorespiratory bypass increases photosynthesis and biomass production in Arabidopsis thaliana. Nat. Biotechnol. 25, 593–599. doi: 10.1038/nbt1299
Klughammer, C., Schreiber, U. (2008a). Complementary PS II quantum yields calculated from simple fluorescence parameters measured by PAM fluorometry and the Saturation Pulse method. PAM Appl. Notes 1, 27–35.
Klughammer, C., Schreiber, U. (2008b). Saturation pulse method for assessment of energy conversion in PS I.
Lisec, J., Schauer, N., Kopka, J., Willmitzer, L., Fernie, A. R. (2006). Gas chromatography mass spectrometry-based metabolite profiling in plants. Nat. Protoc. 1, 387–396. doi: 10.1038/nprot.2006.59
Maier, A., Fahnenstich, H., von Caemmerer, S., Engqvist, M. K. M., Weber, A. P. M., Flügge, U.-I., et al. (2012). Transgenic introduction of a glycolate oxidative cycle into A. thaliana chloroplasts leads to growth improvement. Front. Plant Sci. 3, 1–12. doi: 10.3389/fpls.2012.00038
Miao, J., Guo, D., Zhang, J., Huang, Q., Qin, G., Zhang, X., et al. (2013). Targeted mutagenesis in rice using CRISPR-Cas system. Cell Res. 23, 1233–1236. doi: 10.1038/cr.2013.123
Monné, M., Daddabbo, L., Gagneul, D., Obata, T., Hielscher, B., Palmieri, L., et al. (2018). Uncoupling proteins 1 and 2 (UCP1 and UCP2) from Arabidopsis thaliana are mitochondrial transporters of aspartate, glutamate, and dicarboxylates. J. Biol. Chem. 293, 4213–4227. doi: 10.1074/jbc.RA117.000771
Naito, Y., Hino, K., Bono, H., Ui-Tei, K. (2015). CRISPRdirect: software for designing CRISPR/Cas guide RNA with reduced off-target sites. Bioinformatics 31, 1120–1123. doi: 10.1093/bioinformatics/btu743
Obata, T., Florian, A., Timm, S., Bauwe, H., Fernie, A. R. (2016). On the metabolic interactions of (photo)respiration. J. Exp. Bot. 67, 3003–3014. doi: 10.1093/jxb/erw128
Ogren, W. L. (1984). Photorespiration: pathways, regulation, and modification. Annu. Rev. Plant Physiol. 35, 415–442. doi: 10.1146/annurev.pp.35.060184.002215
Peterhansel, C., Horst, I., Niessen, M., Blume, C., Kebeish, R., Kürkcüoglu, S., et al. (2010). Photorespiration. Arab. B. e0130, 1–24. doi: 10.1199/tab.0130
Pick, T. R., Brautigam, A., Schulz, M. A., Obata, T., Fernie, A. R., Weber, A. P. M. (2013). PLGG1, a plastidic glycolate glycerate transporter, is required for photorespiration and defines a unique class of metabolite transporters. Proc. Natl. Acad. Sci. 110, 3185–3190. doi: 10.1073/pnas.1215142110
Porcelli, V., Vozza, A., Calcagnile, V., Gorgoglione, R., Arrigoni, R., Fontanesi, F., et al. (2018). Molecular identification and functional characterization of a novel glutamate transporter in yeast and plant mitochondria. Biochim. Biophys. Acta - Bioenerg. 1859, 1249–1258. doi: 10.1016/j.bbabio.2018.08.001
Renné, P., Dreßen, U., Hebbeker, U., Hille, D., Flügge, U. I., Westhoff, P., et al. (2003). The Arabidopsis mutant dct is deficient in the plastidic glutamate/malate translocator DiT2. Plant J. 35, 316–331. doi: 10.1046/j.1365-313x.2003.01806.x
Reumann, S., Weber, A. P. M. (2006). Plant peroxisomes respire in the light: some gaps of the photorespiratory C2 cycle have become filled—others remain. Biochim. Biophys. Acta - Mol. Cell Res. 1763, 1496–1510. doi: 10.1016/j.bbamcr.2006.09.008
Rice, K. C., Bayles, K. W. (2008). Molecular control of bacterial death and lysis. Microbiol. Mol. Biol. Rev. 72, 85–109. doi: 10.1128/MMBR.00030-07
Samuilov, S., Brilhaus, D., Rademacher, N., Flachbart, S., Arab, L., Alfarraj, S., et al. (2018). The photorespiratory BOU gene mutation alters sulfur assimilation and its crosstalk with carbon and nitrogen metabolism in Arabidopsis thaliana. Front. Plant Sci. 871, 1–21. doi: 10.3389/fpls.2018.01709
Schneidereit, J., Häusler, R. E., Fiene, G., Kaiser, W. M., Weber, A. P. M. (2006). Antisense repression reveals a crucial role of the plastidic 2-oxoglutarate/malate translocator DiT1 at the interface between carbon and nitrogen metabolism. Plant J. 45, 206–224. doi: 10.1111/j.1365-313X.2005.02594.x
Sharkey, T. D. (1988). Estimating the rate of photorespiration in leaves. Physiol. Plant 73, 147–152. doi: 10.1111/j.1399-3054.1988.tb09205.x
Shen, B.-R., Wang, L.-M., Lin, X.-L., Yao, Z., Xu, H.-W., Zhu, C.-H., et al. (2019). Engineering a new chloroplastic photorespiratory bypass to increase photosynthetic efficiency and productivity in rice. Mol. Plant 12, 1–16. doi: 10.1016/j.molp.2018.11.013
Somerville, S. C., Ogren, W. L. (1983). An Arabidopsis thaliana mutant defective in chloroplast dicarboxylate transport. Proc. Natl. Acad. Sci. 80, 1290–1294. doi: 10.1073/pnas.80.5.1290
Somerville, S. C., Somerville, C. R. (1985). A mutant of Arabidopsis deficient in chloroplast dicarboxylate transport is missing an envelope protein. Plant Sci. Lett. 37, 217–220. doi: 10.1016/0304-4211(85)90007-0
South, P. F., Walker, B. J., Cavanagh, A. P., Rolland, V., Badger, M., Ort, D. R. (2017). Bile acid sodium symporter BASS6 can transport glycolate and is involved in photorespiratory metabolism in Arabidopsis thaliana. Plant Cell 29, 808–823. doi: 10.1126/science.aat9077
South, P. F., Cavanagh, A. P., Liu, H. W., Ort, D. R. (2019). Synthetic glycolate metabolism pathways stimulate crop growth and productivity in the field. Science 363, eaat9077. doi: 10.1105/tpc.16.00775
Wang, J., Bayles, K. W. (2013). Programmed cell death in plants: lessons from bacteria? Trends Plant Sci. 18, 133–139. doi: 10.1016/j.tplants.2012.09.004
Yamaguchi, M., Takechi, K., Myouga, F., Imura, S., Sato, H., Takio, S., et al. (2012). Loss of the plastid envelope protein AtLrgB causes spontaneous chlorotic cell death in Arabidopsis thaliana. Plant Cell Physiol. 53, 125–134. doi: 10.1093/pcp/pcr180
Yang, Y., Jin, H., Chen, Y., Lin, W., Wang, C., Chen, Z., et al. (2012). A chloroplast envelope membrane protein containing a putative LrgB domain related to the control of bacterial death and lysis is required for chloroplast development in Arabidopsis thaliana. New Phytol. 193, 81–95. doi: 10.1111/j.1469-8137.2011.03867.x
Keywords: OsPLGG1, photorespiration, photosynthesis, plastidic glycolate/glycerate translocator, rice
Citation: Shim S-H, Lee S-K, Lee D-W, Brilhaus D, Wu G, Ko S, Lee C-H, Weber APM and Jeon J-S (2020) Loss of Function of Rice Plastidic Glycolate/Glycerate Translocator 1 Impairs Photorespiration and Plant Growth. Front. Plant Sci. 10:1726. doi: 10.3389/fpls.2019.01726
Received: 22 May 2019; Accepted: 09 December 2019;
Published: 24 January 2020.
Edited by:
Wanchai De-Eknamkul, Chulalongkorn University, ThailandReviewed by:
Agepati S. Raghavendra, University of Hyderabad, IndiaToshihiro Obata, University of Nebraska–Lincoln, United States
Copyright © 2020 Shim, Lee, Lee, Brilhaus, Wu, Ko, Lee, Weber and Jeon. This is an open-access article distributed under the terms of the Creative Commons Attribution License (CC BY). The use, distribution or reproduction in other forums is permitted, provided the original author(s) and the copyright owner(s) are credited and that the original publication in this journal is cited, in accordance with accepted academic practice. No use, distribution or reproduction is permitted which does not comply with these terms.
*Correspondence: Jong-Seong Jeon, amplb25Aa2h1LmFjLmty
†These authors have contributed equally to this work.