- 1VIB-KU Leuven Center for Microbiology, VIB, Leuven, Belgium
- 2Laboratory of Molecular Cell Biology, Institute of Botany and Microbiology, KU Leuven, Leuven, Belgium
- 3Department of Biology, College of Natural Sciences, Cantho University, Cantho, Vietnam
- 4Centro de Investigación en Dinámica Celular, Instituto de Investigación en Ciencias Básicas y Aplicadas, Universidad Autónoma del Estado de Morelos, Cuernavaca, Mexico
The function of trehalose metabolism in plants during growth and development has been extensively studied, mostly in the eudicot Arabidopsis thaliana. So far, however, not much is known about trehalose metabolism in the moss Physcomitrella patens. Here, we show that in P. patens, two active trehalose-6-phosphate synthase enzymes exist, PpTPS1 and PpTPS2. Expression of both enzymes in Saccharomyces cerevisiae can complement the glucose-growth defect of the yeast tps1∆ mutant. Truncation of N-terminal extension in PpTPS1 and PpTPS2 resulted in higher TPS activity and high trehalose levels, upon expression in yeast. Physcomitrella knockout plants were generated and analyzed in various conditions to functionally characterize these proteins. tps1∆ and tps2∆ knockouts displayed a lower amount of caulonema filaments and were significantly reduced in size of gametophores as compared to the wild type. These phenotypes were more pronounced in the tps1∆ tps2∆ mutant. Caulonema formation is induced by factors such as high energy and auxins. Only high amounts of supplied energy were able to induce caulonema filaments in the tps1∆ tps2∆ mutant. Furthermore, this mutant was less sensitive to auxins as NAA-induced caulonema development was arrested in the tps1∆ tps2∆ mutant. In contrast, formation of caulonema filaments is repressed by cytokinins. This effect was more severe in the tps1∆ and tps1∆ tps2∆ mutants. Our results demonstrate that PpTPS1 and PpTPS2 are essential for sensing and signaling sugars and plant hormones to monitor the balance between caulonema and chloronema development.
Introduction
Trehalose is a non-reducing sugar consisting of two glucose units in an α,α-1,1 configuration. It is now well known that highly accumulated trehalose in the so-called anhydrobiotic organisms, including yeasts, tardigrades, worms, and some specific plants such as the desert plant Selaginella lepidophylla, the resurrection plant Myrothamnus flabellifolius, and the grass Sporobolus spp, helps them survive during extreme dehydration, heat, or oxidative stress (Adams et al., 1990; Müller et al., 1995; Rebecchi et al., 2009; Wharton, 2011; Vilaça et al., 2012). In plants, trehalose is synthesized through two consecutive enzymatic reactions. The first step is mediated by trehalose-6-phosphate synthase (TPS), which results in the intermediate trehalose-6-phosphate (Tre-6P). A subsequent step results in the formation of the end product, trehalose, catalyzed by trehalose-6-phosphate phosphatase (TPP) (Cabib and Leloir, 1958).
The plant trehalose biosynthesis genes are grouped in three distinct subfamilies according to their similarity to the yeast homologues TPS1 and TPS2 in Saccharomyces cerevisiae (Leyman et al., 2001; Avonce et al., 2006). The yeast ScTPS1 gene encodes the TPS enzyme while ScTPS2 encodes the TPP enzyme (Bonini et al., 2004). In A. thaliana, class I TPS enzymes display the highest similarity to ScTps1 and contain four proteins (AtTPS1 – AtTPS4), with AtTPS1, AtTPS2, and AtTPS4 showing TPS activity (Blázquez et al., 1998; Van Dijck et al., 2002; Vandesteene et al., 2010; Delorge et al., 2015). The class II TPS proteins (AtTPS5–AtTPS11) are most similar to ScTps2, but they do not show any detectable TPS or TPP activity upon expression in yeast (Vogel et al., 2001; Ramon et al., 2009). The class III TPP proteins (AtTPPA – AtTPPJ) are smaller isoforms with a well-conserved TPP domain harboring the three L-2-haloacid dehydrogenase (HAD) motifs, and they all function as active trehalose-6-phosphate phosphatases (Vogel et al., 1998; Vandesteene et al., 2012). There are no orthologues of TPP proteins present in S. cerevisiae. Interestingly, phylogenetic analysis revealed that TPP proteins are closely related to genes present in Mycobacterium, indicating that the class III genes seem to be of bacterial origin (Avonce et al., 2006; Avonce et al., 2010).
Tre-6P acts as a sugar-signaling metabolite that regulates plant metabolism and affects many aspects of plant growth and development (Schluepmann et al., 2004; Lunn et al., 2006; Wahl et al., 2013; Lunn et al., 2014; Bledsoe et al., 2017). Tre-6P is considered as a specific sensor for sucrose availability. Upon carbon starvation Tre-6P levels were low in A. thaliana seedlings and they increase strongly by addition of sucrose (Lunn et al., 2006). There is a positive correlation between Tre-6P and sucrose levels under various growth conditions. Many studies have confirmed this correlation in A. thaliana rosettes (Lunn et al., 2006; Wingler et al., 2012; Carillo et al., 2013; Yadav et al., 2014), seedlings (Lunn et al., 2006; Yadav et al., 2014), developing seeds (Thiel et al., 2011), and shoot apex (Wahl et al., 2013), as well as in wheat grains (Martínez-Barajas et al., 2011), and developing potato tubers (Debast et al., 2011). Small changes in Tre-6P levels, as a result of increased or decreased gene expression/enzyme activity, can perturb metabolic signaling, leading to reprogramming of expression of hundreds of genes involved in growth and stress responses (Griffiths et al., 2016). The tight regulation of sucrose levels by Tre-6P might be a homeostatic mechanism of the plant ensuring levels of sucrose to be contained in a certain range, optimal for growth (Lunn et al., 2014). Tre-6P also stimulates starch synthesis by promoting thioredoxin-mediated AGPase redox activation (Kolbe et al., 2005), and promotes biosynthetic processes in seedlings in response to high levels of sucrose (Delatte et al., 2011). SNF1-related protein kinase 1 (SnRK1), which is known to inhibit plant growth (Baena-González et al., 2007), is inhibited by Tre-6P (Zhang et al., 2009) and thereby promotes survival of growing tissues of Arabidopsis under stress. Moreover, carbon utilization and carbon allocation over plant growth are regulated through the SnRK1 signaling pathway involving Tre-6P, SnRK1, and the sugar-regulated transcription factor basic region leucine zipper transcription factor11 (bZIP11) (Delatte et al., 2011). Tre-6P signaling plays a crucial role in regulation of floral transition in A. thaliana (Wahl et al., 2013) and is involved in stress responses. Overexpression of rice TPP1 in developing maize ears with reduced Tre-6P amounts led to an increase of crop yield in non-drought and mild or even severe drought conditions (Nuccio et al., 2015).
In most vascular plants, only minute amounts of trehalose are detected, which implies that trehalose could not act as an osmoprotectant during stress conditions, as seen in stress-resistant species such as Selaginella lepidophylla, where trehalose accumulates to high amounts (Vogel et al., 2001; Fernandez et al., 2010; Pampurova et al., 2014). Therefore, several initiatives were taken to increase trehalose levels by expressing microbial TPS and TPP genes in plants. Such transgenic model and crop plants showed improved stress tolerance, but unfortunately, also resulted in abnormal plant development. Transgenic plants experienced stunted growth, abnormally shaped leaves, delayed flowering, and many other phenotypic effects (Romero et al., 1997; Penna, 2003; Ge et al., 2008; Li et al., 2011; Delorge et al., 2014). These results suggested that trehalose metabolism and plant growth signaling pathways interfere with each other.
Physcomitrella patens is an important model plant in genetic studies due to numerous advantages such as a dominant haploid phase of the life cycle, which allows phenotype selection upon gene modification. Protoplasts can easily be isolated from gametophytes and regenerate directly into filamentous tissues. Furthermore, efficient homologous recombination allows precise inactivation or modification of genes (Cove, 2005). P. patens has been widely used as a model organism in fundamental research, developmental studies, and as a tool to produce biopharmaceuticals on a large scale (Cove et al., 1991; Baur et al., 2005; Weise et al., 2007; Reski et al., 2015).
In P. patens there are two alternative generations: the haploid gametophyte stage and the diploid sporophyte stage. The protonema filaments of the gametophyte are generated by spore germination. The protonema expands its filaments to form a colony comprising chloronema and caulonema. However, at first protonema only consists of chloronemal cells, which are densely packed with many chloroplasts and divide more slowly. Next, caulonemal cells are differentiated from the chloronemal apical cells. Caulonemal cells contain fewer chloroplasts and, due to their increased rate of cell division, cause rapid colony expansion, allowing the moss to grow (Cove, 2005). The balance between these two types of tissue depends on various factors including light, sugars, and plant hormones (Reski and Abel, 1998; Thelander et al., 2005; Jang and Dolan, 2011). In high-energy conditions, such as high light and increased sugar concentration in the medium, caulonema filaments are induced, whereas low energy, including low light and reduced levels of sugars, stimulates chloronema branching (Thelander et al., 2005).
So far, studies in sugar metabolic signaling, particularly in trehalose metabolism, are limited in P. patens (Nagao et al., 2005; Nagao et al., 2006; Rother et al., 2006; Erxleben et al., 2012; Arif et al., 2018). As a bryophyte, P. patens represents an ancient lineage of early-diverging land plants and it is an excellent model to study the role of the metabolism of trehalose in plants from an evolutionary perspective. Therefore, in this study, we generated class I TPS knockout plants including tps1∆, tps2∆, and tps1∆ tps2∆ mutants to functionally characterize these proteins, and to determine the role of class I trehalose biosynthesis enzymes in response to various factors in this moss.
Materials and Methods
Yeast Strains, Culture Conditions, Transformation, and Complementation Assay
S. cerevisiae wild-type strain, W303-1A (Thomas and Rothstein, 1989); TPS1 deletion strain, YSH290 (W303-1A, tps1∆::TRP1) (Hohmann et al., 1993); TPS2 deletion strain, YSH450 (W303-1A, tps2∆::LEU2) (Neves et al., 1995); and TPS1 TPS2 deletion strain, YSH652 (W303-1A, tps1∆::TRP1, tps2∆::LEU2) (Neves et al., 1995) were used in this study. Yeast cells were grown at 30°C or at 38°C in synthetic medium with the appropriate auxotrophic requirements and supplemented with 2% (w/v) glucose or 2% (w/v) galactose, as described previously (Blázquez and Gancedo, 1995).
Class I TPS genes of P. patens were cloned behind the CUP1 promoter in the yeast expression vector pSAL4 (Zentella et al., 1999) for the complementation assay. Primers used to make these constructs are listed in Supplementary Table 1. Plasmids were transformed using the LiAc/PEG method without heat shock (Gietz et al., 1995). Transformants were selected in medium without uracil. Plates were incubated at 30°C (for TPS complementation) or at 38°C (for TPP complementation). After 48 h, the growth of the colonies was analyzed. Growth curves were started with start OD600 of 0.05. Growth was monitored by OD600 measurements every 2 h while continuous shaking in the Automated Microbiology Growth Analysis System (Bioscreen C) for 3 days at 30°C or 38°C.
Plant Material and Culture Conditions
P. patens wild-type strain, “Gransden” isolate, collected from Gransden Wood, in Cambridgeshire (supplied by David Cove, University of Leeds) was used throughout the study, and knockout plants were generated in the Gransden background. Protonema or gametophores were grown axenically on agar plates containing sterile BCD medium (Ashton et al., 1979), supplemented with 1 mM CaCl2 and with or without 5 mM ammonium tartrate. The growth of chloronema is induced when ammonium tartrate is included, whereas caulonema formation is enhanced when ammonium tartrate is omitted. The agar plates were overlaid with sterile cellophane. Plants were cultivated in continuous light (45 μmol m-2s-1) at 25°C. Each week, plants were transferred to new plates. Subcultivation was performed by harvesting plants and homogenizing in sterile water using an ULTRA-TURRAX Tube Drive disperser (IKA).
For most assays, small pieces of protonema were cultivated on solid plates containing cellophane layers, supplied with or without exogenous additives (hormones, sugars). Following hormones were tested: BAP (concentrations of 0.1, 1, and 10 μM) and NAA (concentrations of 0.1, 1, and 10 μM). Following sugars were tested: glucose (25 and 150 mM), sucrose (25 and 150 mM). Expression of PpTPS1 and PpTPS2 was monitored by quantitative-PCR after 2, 4, 8, and 24 h (see below).
TPS Activity Measurements
TPS activity was measured by a coupled-enzyme assay as described by Hottiger et al. (1987). Specific activity was expressed as μkat/g protein. Protein concentration was determined by PIERCE™ 660 nm protein assay reagent (Thermo Scientific) and absorbance was measured at 660 nm using a bovine serum albumin (BSA) standard curve as reference.
Trehalose Determination Upon Expression in Yeast
Trehalose was determined by hydrolysis to glucose with trehalase (extracted from Humicola grisea) and was subsequently quantified by the glucose oxidase:peroxidase method, described by Zentella et al. (1999).
Metabolite Measurements
Tre-6P was extracted with chloroform-methanol and measured by plant extracts by LC-MS/MS (Lunn et al., 2006) with modifications as described in Figueroa et al. (2016). Trehalose was measured enzymatically in the same extracts using a fluorometric assay as described in Carillo et al. (2013).
Isolation and Cloning of Class I TPS Genes
Coding sequence (CDS) of the class I trehalose-6-phosphate synthase genes of P. patens was isolated via RT-PCR starting from cDNA of wild-type protonema tissues. To determine the sequence of 5'-end of PpTPS2V6.1 cDNA, the 5' RACE (Rapid amplification of cDNA ends) System was used, according to the manufacturer's instructions (Invitrogen). After cloning in the appropriate vectors, plasmids were prepared for sequencing (VIB Genetic Service Facility, Belgium). Used primers are listed in Supplementary Table 1.
Quantitative-PCR (qPCR) Analysis
RNA samples were extracted with Trizol (Invitrogen), according to the manufacturer's instructions. To synthesize cDNA, 2 µg total RNA was treated with 1 µl DNase (NEB) for 10 min at 37°C. The reaction was then stopped by denaturation at 75°C for 5 min. cDNA was synthesized from the DNase-treated RNA with the Reverse Transcription System (Promega).
Each qPCR reaction consisted of a 5 μl diluted cDNA sample (2 ng) in a mixture of 10 μl Platinum SYBR Green qPCR Supermix (Invitrogen), 0.8 μl primer-mix (10 μM each), and 4.2 μl H2O. The qPCR reactions were performed in a StepOnePlusTM Real-Time PCR System (Applied Biosystems). Amplification was performed according to the following protocol: denaturation step: 95°C, 15 s; annealing step: 58°C, 30 s; extension step: 72°C, 30 s; all steps repeated for 50 cycles. The housekeeping gene used as a reference is PpACT1. Used primers are listed in Supplementary Table 1.
Construction of Transgenic Lines
To completely disrupt the PpTPS1 or PpTPS2 gene, approximately 1 kb of flanking genomic regions of 5'-UTR and 3'-UTR of PpTPS1 or PpTPS2 was adjacent to the 5'- and 3'-ends of a selection marker in transformation vectors, respectively. Homologous recombination allows efficient integration of the selection cassette at the targeted genomic site, causing a full deletion of the PpTPS1 or PpTPS2 gene (Figure 1). PpTPS1 knockout constructs were generated in the pTN186 transformation vector (NIBB, kindly provided by Yukiko Kabeya from the Hasebe laboratory, Japan) that contains the aph4 gene for selection on hygromycin. PpTPS2 knockout constructs were made in pHIZ2 (NIBB, kindly provided by Yukiko Kabeya from the Hasebe laboratory, Japan), a plasmid that contains the ble gene, allowing selection on zeocin. The final transformation constructs were linearized by restriction digestion prior to PEG-based transformation in protoplasts. Used primers are listed in Supplementary Table 1. Furthermore, a double knockout was generated by transformation of the pHIZ2:TPS2 construct in a verified tps1Δ background.
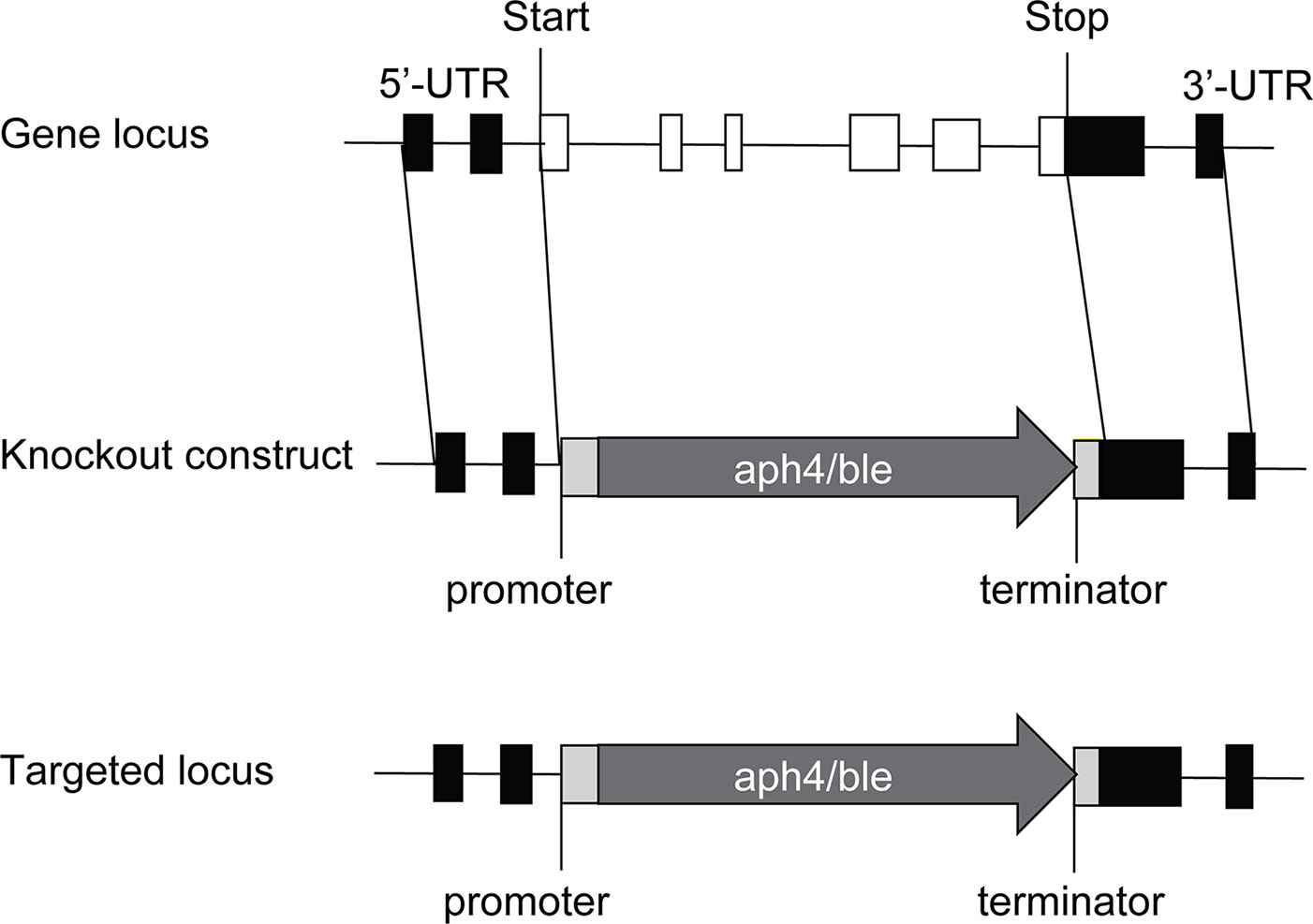
Figure 1 Disruption of PpTPS1/PpTPS2 genes in P. patens. The PpTPS1 or PpTPS2 genes are replaced by the antibiotic resistance genes aph4 or ble, respectively. White boxes and black lines between the boxes represent exons and introns, respectively. Black boxes indicate UTR (untranslated region). Light gray boxes indicate promoter and terminator of the resistance gene. The dark gray arrows show the zeocin expression cassette (aph4) or the hygromycin expression cassette (ble).
Isolation of P. patens Protoplasts
Five-day-old protonema tissues were digested in 10 ml 8% (w/v) D-mannitol, supplied with 1% driselase (Sigma) for 1–1.5 h at room temperature. Protoplasts were collected via filtration on a 100 μm Cell Dissociation Sieve (CD-1TM, Sigma-Aldrich, St. Louis, MO, USA). After several washing steps with 8% (w/v) D-mannitol, protoplast density was determined with a hemocytometer and re-adjusted to an optimal density of 1.6×106/ml.
Transformation of P. patens Protoplasts
Transformation started from 150 μl of protoplast suspension, which was subsequently mixed with 150 μl 2X MMg solution (100 ml contains 6.1 g MgCl2.6H2O, 8 g D-mannitol, 0.2 g MES, pH 5.6 adjusted with 4M KOH and filter sterilized). DNA (around 60 μg) was quickly added and the whole mixture was transferred to a fresh tube containing 300 μl of 40% PEG solution [10 ml contains 0.236 g Ca(NO3)2.4H2O, 0.047 g HEPES, 0.728 g D-mannitol, 4 g PEG6000, pH 7.5 adjusted with 4M KOH, overnight incubated at room temperature, filter sterilized]. The protoplast mixture was subjected to a 5 min heat shock at 45°C and was afterwards cooled down in a water bath at room temperature. Over the next hour, the transformation samples were step by step diluted with 8% (w/v) D-mannitol to allow full recovery. Finally, protoplasts were left recovering overnight in the dark in PRM-L (BCD medium, 5 mM ammonium tartrate, 6% D-mannitol, 10 mM CaCl2). The morning after, protoplasts were centrifuged, resuspended in 9 ml PRM-T [BCD medium, 5 mM ammonium tartrate, 6% mannitol, 10 mM CaCl2, 0.4% plant agar (Duchefa)], and plated on cellophane covered PRM-B plates [BCD medium, 5 mM ammonium tartrate, 6% mannitol, 10 mM CaCl2, 0.55% Plant agar (Duchefa)]. After 5 days of recovery, protoplasts were transferred to BCDAT plates containing the appropriate antibiotic (25 mgL-1 zeocin, Invitrogen or 20 mgL-1 hygromycin, Cayla). After 2 weeks of initial selection, plants were transferred to new BCDAT plates without antibiotics in order to avoid unstable transformants. At the end of this period, small parts of the grown protoplasts were again selected on BCDAT plates containing antibiotics. Two weeks of growth will eventually select stable transformants ready for genotyping.
Phenotypic Analysis
To induce formation of gametophytes, homogenized protonema was cultivated on BCD agar plates, supplemented with 1 mM CaCl2 and 5 mM ammonium tartrate (BCDAT medium) for 2 weeks at 25°C under continuous light. Next, small pieces of protonema were transferred to new BCDAT plates. After 1 month, gametophores were ready for phenotyping. Induction of sporophytes occurred in BCD medium, supplemented with 1 mM CaCl2 and without ammonium tartrate. Protonema colonies were incubated in baby glass jars containing thick layered agar medium at 25°C in continuous light. After a month, the moss was transferred to a growth chamber adjusted to 15°C under an 8 h light/16 h dark diurnal cycle. Around 14 days later, antheridia and archegonia were formed. To facilitate fertilization, sterile water was added on top of the gametophores. Two weeks later, sporophytes were formed. One month later, matured spores are generated and phenotyping was performed.
To assess the composition of chloronemal cells and caulonemal cells in protonema colonies, protonema filaments were grown on BCDAT plates at 25°C in continuous light. Discrimination of chloronemal and caulonemal cells are based on the angle of the septa. The septa of caulonemal cells are oblique and those of chloronemal cells are perpendicular. The numbers of caulonemal and chloronemal cells in protonema filaments were counted after the cultivation of protonema for 10 days. Growing filaments at the edge of protonema colonies were selected randomly to calculate the percentage of chloronemal and caulonemal cells.
Morphology of the moss was analyzed by a M165C binocular microscope (Leica). Size of protonema colonies, gametophores, and sporophytes were analyzed by ImageJ.
Results
P. patens Class I TPS Proteins PpTPS1 and PpTPS2 Are Catalytically Active
Phylogenetic analysis of plant trehalose biosynthesis class I genes showed that two homologues, which are annotated as PpTPS1 and PpTPS2, exist in P. patens (Avonce et al., 2010). BLAST analysis in the Genome Browser of Cosmoss database (The P. patens genome resource) predicted different splice variants for PpTPS1 and PpTPS2 genes. PpTPS1 has three splice variants, which are Pp1s240_110V6.1, Pp1s240_110V6.2, and Pp1s240_110V6.3 (Figure 2A). These splice variants mainly differed in the N-terminal end. Similarly, there are three splice variants of PpTPS2 (Pp1s116_157F3.1, Pp1s116_157V6.1, and Pp1s116_157V6.2) (Figure 2B). Alignment analysis with AtTPS1 elucidated an N-terminal extension in both full length PpTPS1 (Pp1s240_110V6.2) and PpTPS2 (Pp1s116_157F3.1). Interestingly, the splice variant Pp1s116_157V6.1 (PpTPS2V6.1) lacks this N-terminal extension.
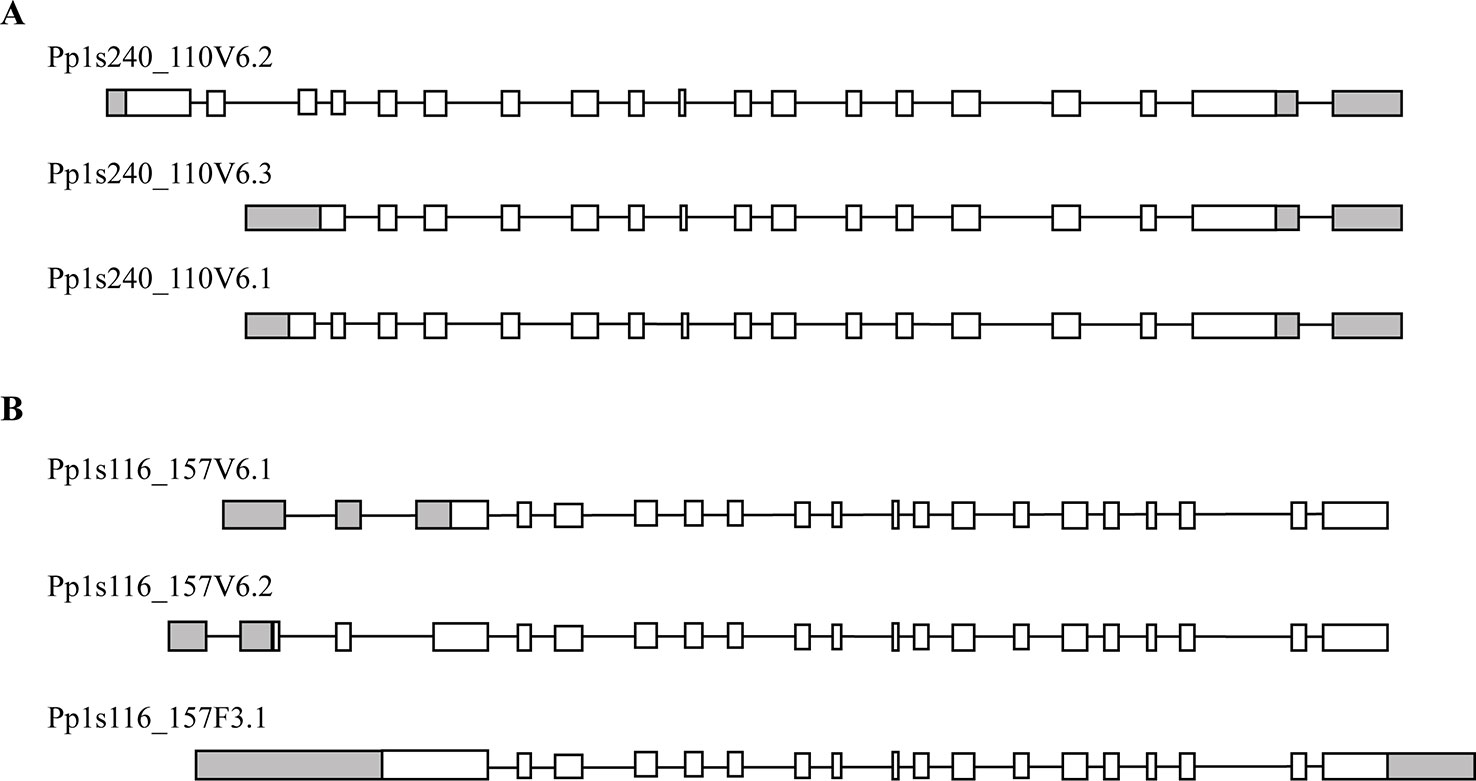
Figure 2 Predicted models by Cosmoss database of the PpTPS1 (A) and PpTPS2 (B) genes. Pp1s240_110V6.1, Pp1s240_110V6.2, Pp1s240_110V6.3 and Pp1s116_157V6.1, Pp1s116_157V6.2, Pp1s116_157F3.1 are different splice variants of PpTPS1 and PpTPS2, respectively. White boxes represent exons, black lines indicate introns, and gray boxes show UTR (untranslated region).
In order to functionally characterize these two genes, we isolated the coding sequence of class I genes by RT-PCR from protonemal cDNA. Among the three predicted variants of PpTPS1, only Pp1s240_110V6.2 (named as PpTPS1) could be isolated. In addition, the splice variant Pp1s116_157V6.2 (PpTPS2V6.2) might be a pseudogene as its sequence contains a premature stop codon and was therefore not included for further analysis. Next, the three variants of class I genes (PpTPS1, PpTPS2, and PpTPS2V6.1) were cloned in a yeast expression shuttle vector, pSAL4, controlled by the CUP1 promoter. N-terminally truncated versions of PpTPS1 and PpTPS2 were also cloned in pSAL4. The first 155 residues at the N-terminal end of PpTPS1 or PpTPS2 were removed, producing the truncated versions of PpTPS1 and PpTPS2. Final constructs were transformed in the yeast tps1∆ and tps1∆ tps2∆ mutants to determine whether the class I TPS enzymes can complement the growth defect on glucose-containing medium of the yeast mutants. The yeast tps1∆ and tps1∆ tps2∆ mutants are unable to grow on glucose as carbon source, as ScTps1 is required for regulating the flow of glucose into glycolysis (Blázquez et al., 1993; Thevelein and Hohmann, 1995) (Figure 3). Apart from plate assays on solid medium, liquid growth assays were performed starting from an initial OD600 of 0.05. Both on plates and in liquid media, growth of the yeast tps1∆ and tps1∆ tps2∆ mutants was restored on glucose containing medium (Figure 3) and therefore all three enzymes are considered as active trehalose-6-phosphate synthases.
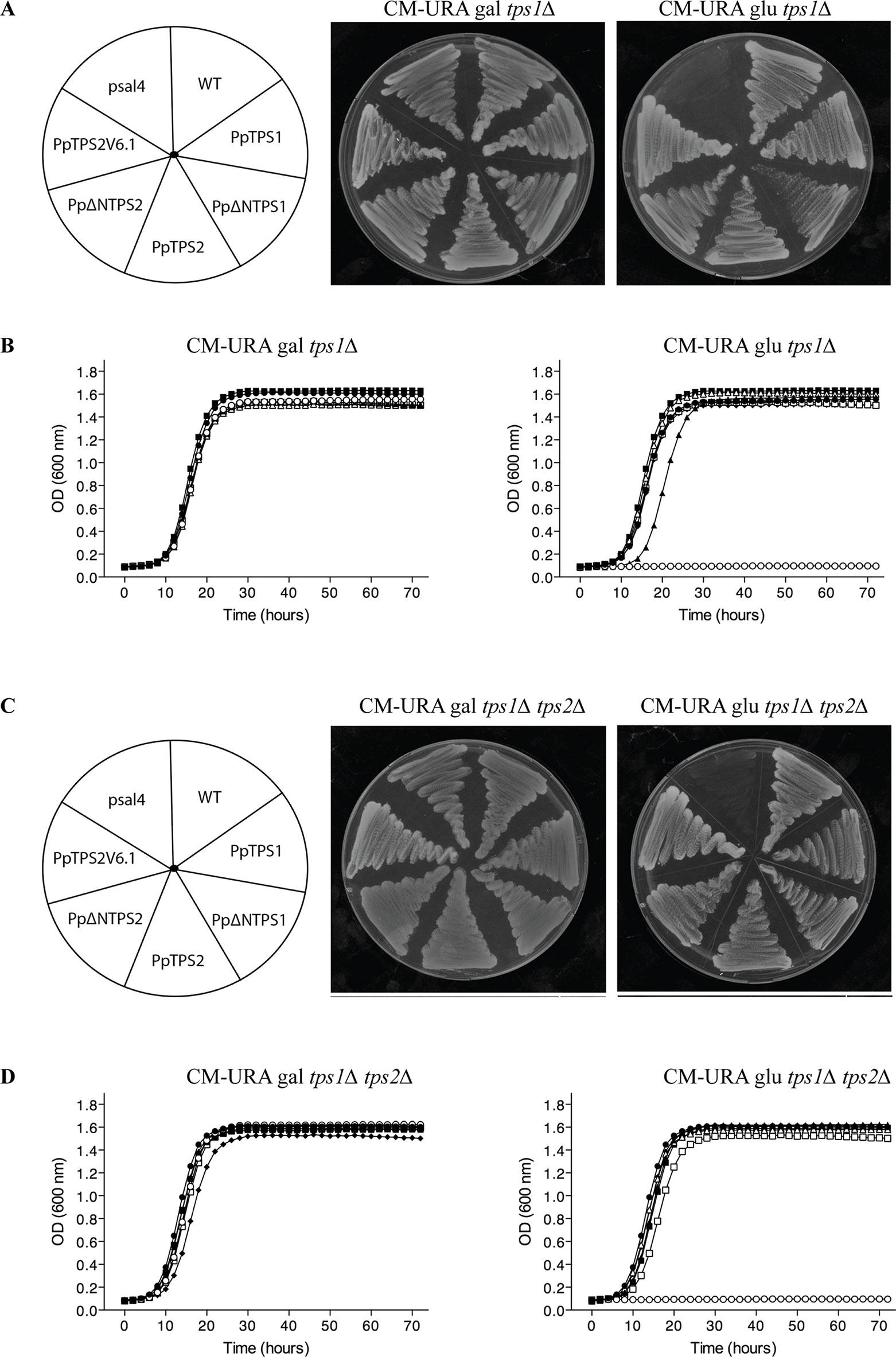
Figure 3 Complementation studies of PpTPS1, PpTPS2, and PpTPS2V6.1 in the yeast tps1∆ (A and B) and tps1∆ tps2∆ (C and D) mutants. (A and C) Complementation assay was performed on plates with galactose or glucose. Copper sulfate was added to a final concentration of 100 μM to induce expression. The wild-type yeast strain transformed with the empty vector was used as a control (WT). (B and D) Bioscreen analysis was done in liquid synthetic medium lacking uracil with galactose or glucose and 100 μM copper sulfate. Wild-type (WT) strain transformed with pSAL4 ( ● ), the deletion strain transformed with pSAL4 ( ○ ), or PpTPS1 ( ▲ ), or PpTPS2 ( △ ), or Pp∆NTPS1( ■ ), or Pp∆NTPS2 ( □ ), or PpTPS2V6.1 ( ◆ ).
Further evidence supporting the moss class I TPS proteins as active enzymes was provided by measuring TPS activity and trehalose levels upon expression in yeast. The full-length enzymes showed low activity compared to the ScTps1. Truncated PpTPS1 and PpTPS2 had a significantly higher activity, compared to their full-length version (Figure 4A). Interestingly, the activity of the splice variant PpTPS2V6.1 was higher than that of PpTPS2 since PpTPS2V6.1 lacks most of the N-terminal extension, which is present in the full length PpTPS2 and which is predicted to exert an inhibitory effect on the enzyme activity. This result is similar to results described by Van Dijck et al. (2002) for A. thaliana and for Ostreococcus tauri (Avonce et al., 2010). In addition, more trehalose accumulated in the yeast tps1∆ mutant strains expressing the truncated forms of PpTPS1 and PpTPS2 in comparison with the full-length versions (Figure 4B). Likewise, the yeast tps1∆ containing PpTPS2V6.1 also accumulated more trehalose, which is in accordance with the TPS activity data.
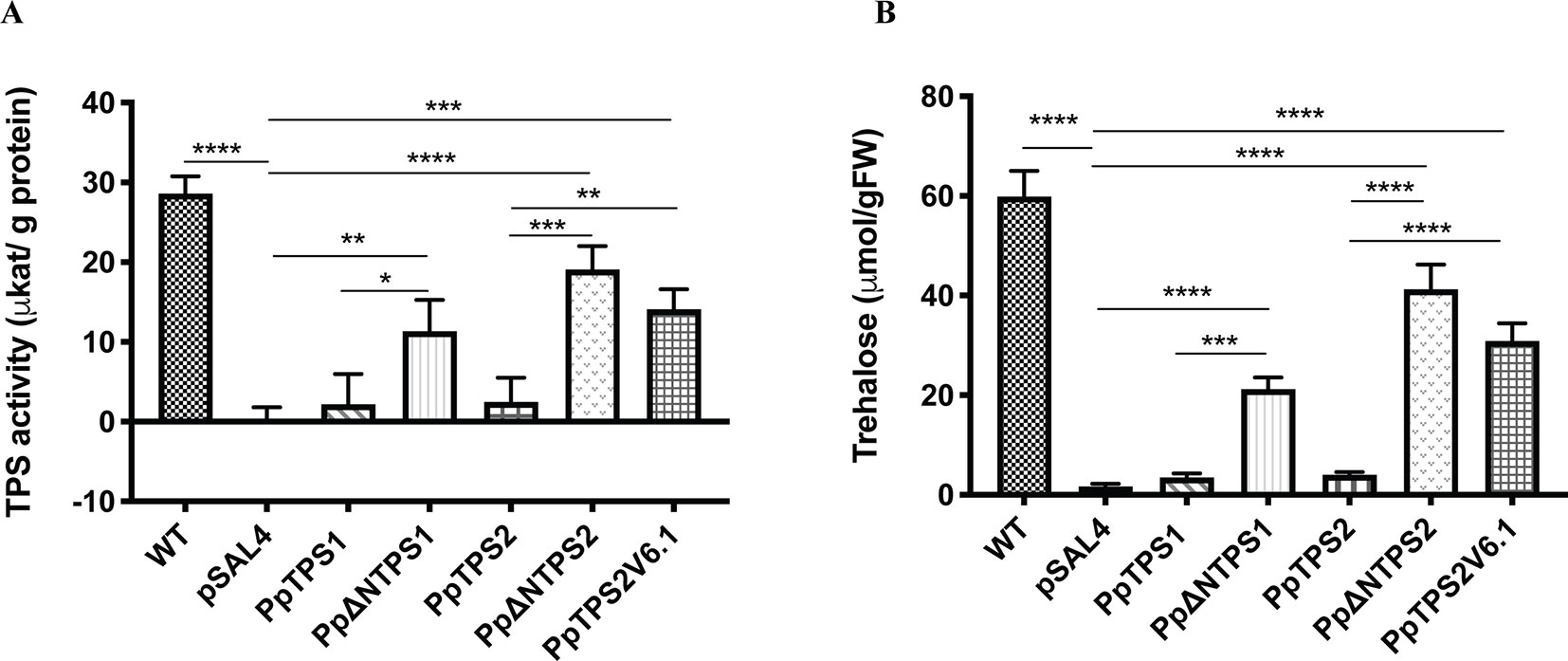
Figure 4 (A) TPS activity and (B) trehalose measurements in the yeast tps1Δ mutant transformed with PpTPS1, PpTPS2, or PpTPS2V6.1. Cells were grown on synthetic medium lacking uracil supplied with 2% galactose. Data represent the mean ± SD of three biological repeats. Statistical analysis with one-way ANOVA, *p ≤ 0.05, **p ≤ 0.01, ***p ≤ 0.001, ****p ≤ 0.0001.
P. patens Class I TPS Proteins Do Not Show TPP Enzymatic Activity
Plant class I TPS proteins harbor a C-terminal part, which is homologous to the TPP domain of S. cerevisiae ScTps2 and E. coli OtsB (Goddijn and van Dun, 1999). TPP functionality can therefore be tested by complementation studies in the yeast tps2Δ mutant. This mutant displays a thermosensitive phenotype since lack of trehalose and accumulating Tre-6P result in a stress-sensitive phenotype at high temperatures. In our hands, none of the class I TPS enzymes of P. patens were able to complement the phenotype of the tps2Δ mutant at 38°C (Figure 5). This result was expected since the TPP domains of these proteins lack the conserved motifs of the phosphatase active site (Leyman et al., 2001; Eastmond et al., 2003; Vandesteene et al., 2010) and none of the previously tested plant class I TPS enzymes have displayed bifunctional enzymatic activity (Avonce et al., 2010).
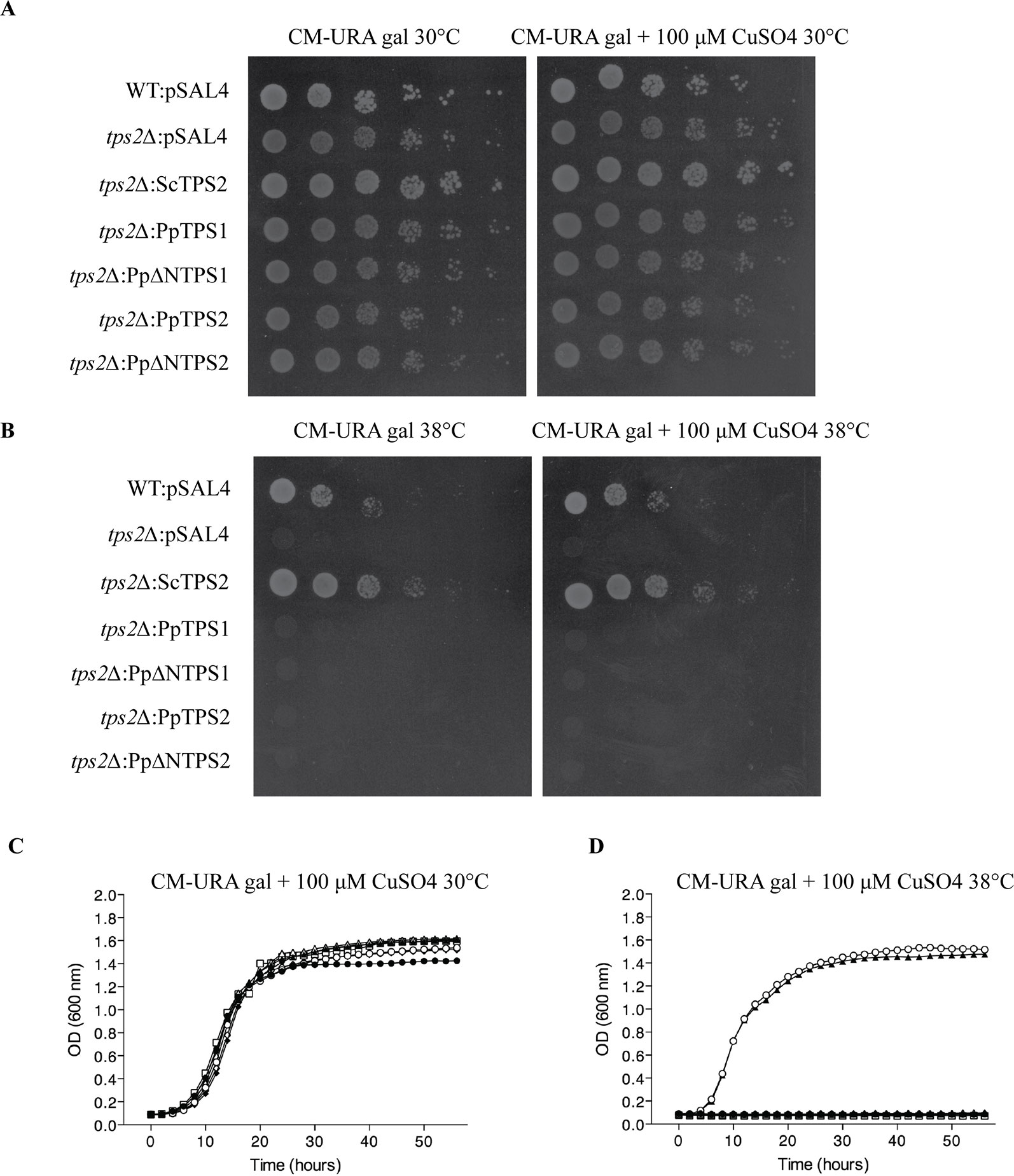
Figure 5 Complementation studies of PpTPS1 and PpTPS2 in the yeast tps2∆ mutant at 30°C or 38°C. Complementation assay was performed on plates supplemented with 2% galactose with or without 100 μM copper sulfate at 30°C (A) and 38°C (B). Bioscreen analysis was done at 30°C (C) and 38°C (D). Wild-type (WT) strain transformed with pSAL4 ( ▲ ), the deletion strain transformed with ScTps2 ( ○ ), pSAL4 ( ● ), PpTPS1 ( △ ), PpTPS2 ( ◆ ), PpΔNTPS1 ( □ ), or PpΔNTPS2 ( ◇ ).
Expression Profiling of Class I Trehalose Biosynthesis Genes of P. patens
The expression of class I TPS genes was analyzed by quantitative-PCR (qPCR). Since no specific primers could be designed for PpTPS2V6.1, qPCR analysis for this splice variant was excluded. As shown in Figure 6, both PpTPS1 and PpTPS2 were expressed in protonema and gametophores. In both tissues PpTPS2 was expressed at higher levels as compared to PpTPS1. In the case of PpTPS1, this gene was expressed highly in protonema, whereas its expression was low in gametophores.
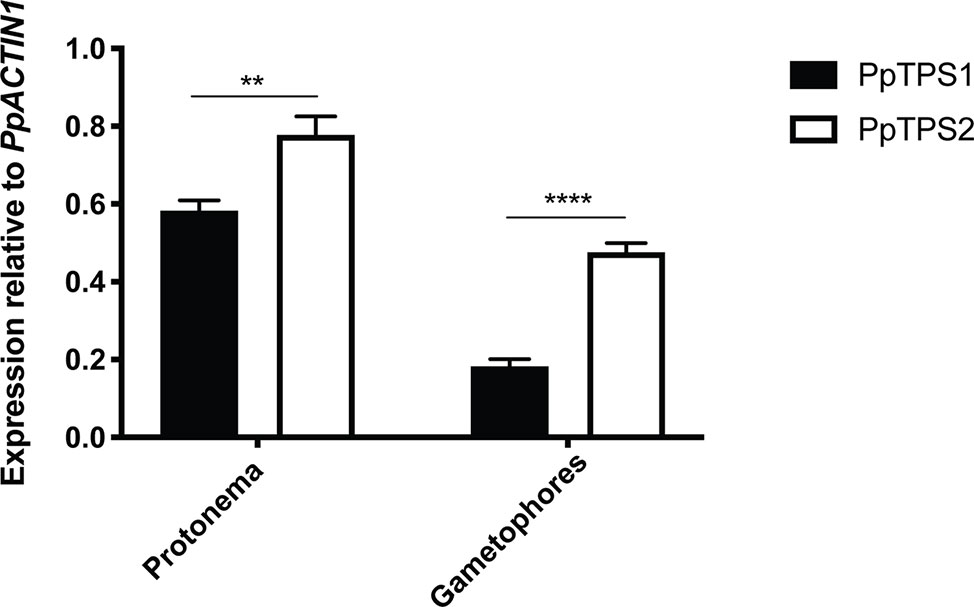
Figure 6 qPCR analysis of PpTPS1 and PpTPS2 in different tissues. Expression of PpTPS1 and PpTPS2 was measured in protonema and gametophores. Data represent the mean ± SD of three biological repeats after normalization with the housekeeping gene PpACT1. Statistical analysis with multiple t tests, **p ≤ 0.01, ****p ≤ 0.0001.
Functional Characterization of Transgenic P. patens
To understand the roles of PpTPS1 and PpTPS2 in plant metabolism and development, transgenic single and double knockout plants (tps1∆, tps2∆, and tps1∆ tps2∆ mutants) were generated. Genotyping by PCR was performed to confirm correct deletions. In addition, southern blot analysis was also performed to identify single insertion mutants (Supplementary Figure 1). Phenotypic analysis of transgenic plants was performed. A few days after protoplasting, filaments started growing and, after approximately 1 month, plants were ready for subcultivation. In continuous light, moss tends to develop many caulonema filaments in order to stimulate expansion and gametophores are found randomly spread in the plant. In the knockout mutants, caulonema outgrowth seemed to be impaired and gametophores were densely packed together, which might indicate a slower growth rate or altered distribution of energy in the two tissue types, especially in the double mutant as compared to the wild type (Figures 7A–G). In addition, the differentiation of chloronema to caulonema was also repressed in the single mutants. This phenomenon was more pronounced in the double tps1∆ tps2∆ mutant (Figure 7H and Supplementary Figure 2). These results imply that PpTPS1 and PpTPS2 play an important role in growth and development of P. patens. This is similar to A. thaliana, where AtTPS1 is essential during embryo development (Eastmond et al., 2002).
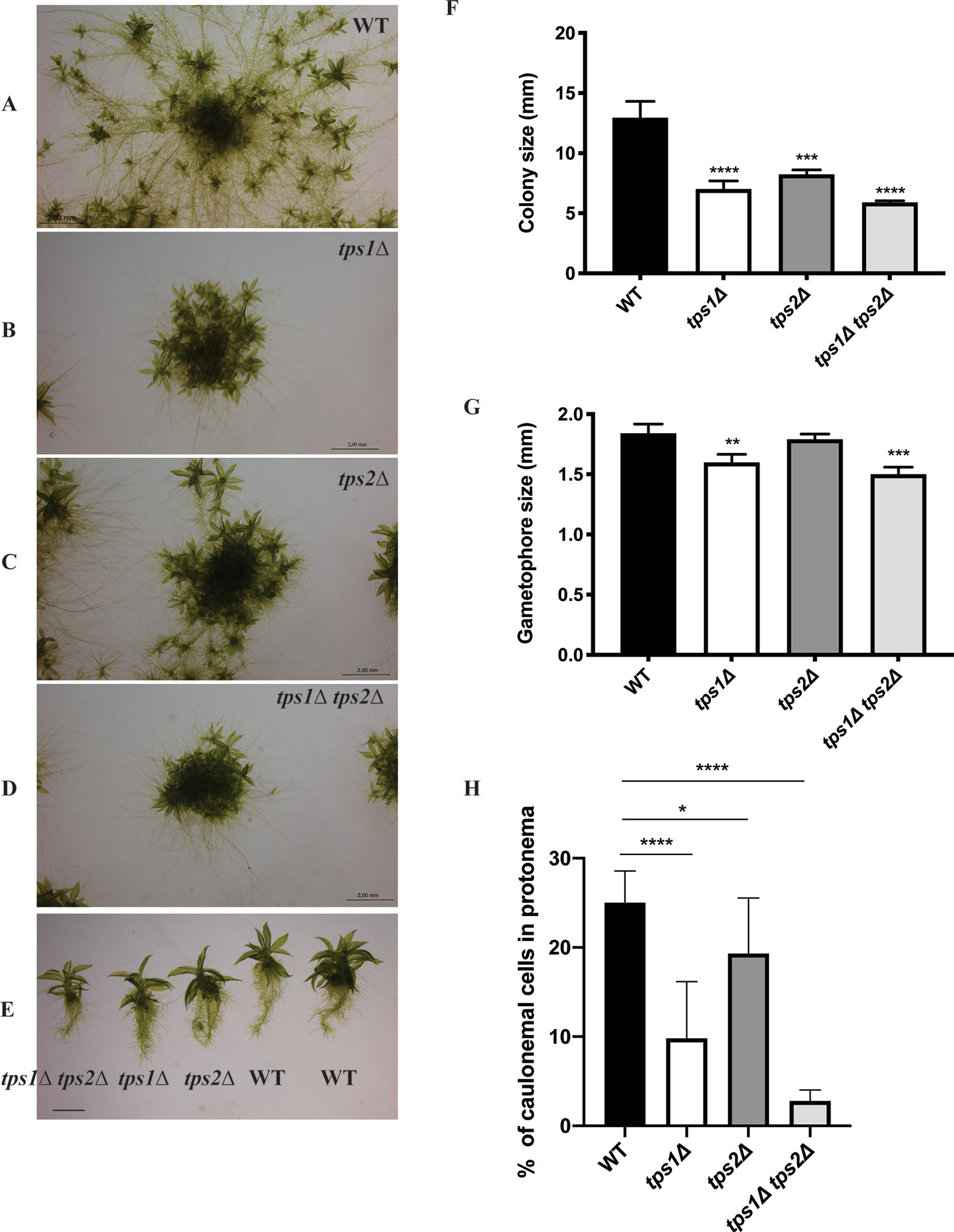
Figure 7 Morphology of protonema of wild-type (WT) and transgenic plants. (A–E) Representative images of protonema morphology of WT and knockout lines grown on BCDAT medium in continuous light at 25°C for 1 month. Bars represent 2 mm. Measurement of colony size (F) and gametophore size (G) of WT and transgenic plants. Data represent mean ± SD of three individuals of protonema colonies or gametophores. (H) The percentage of caulonemal cells in protonema colonies of WT and mutant lines. Thirteen protonema colonies of each line were selected randomly to count the numbers of caulonemal cells. Statistical analysis with one-way ANOVA, *p ≤ 0.05, **p ≤ 0.01, ***p ≤ 0.001, ****p ≤ 0.0001.
The effect of class I trehalose biosynthesis proteins on sporophytes was also investigated. Sporulation was initiated by growing the moss plants on BCD agar plates without ammonium tartrate for 1 month under continuous light at 25°C. After that, the plants were grown in the condition of 8 h light/16 h dark light cycle at 15°C for another 4 weeks; sporophytes were formed and ready for analysis. The double mutant (tps1∆ tps2∆) failed to produce sporophytes, while single mutants (tps1∆ and tps2∆) developed sporophytes and viable spores. However, no significant difference in sizes of spores was detected between single mutants and wild type (Figure 8). The result reveals that PpTPS1 and PpTPS2 are necessary, but redundant, for sporophyte production in P. patens.
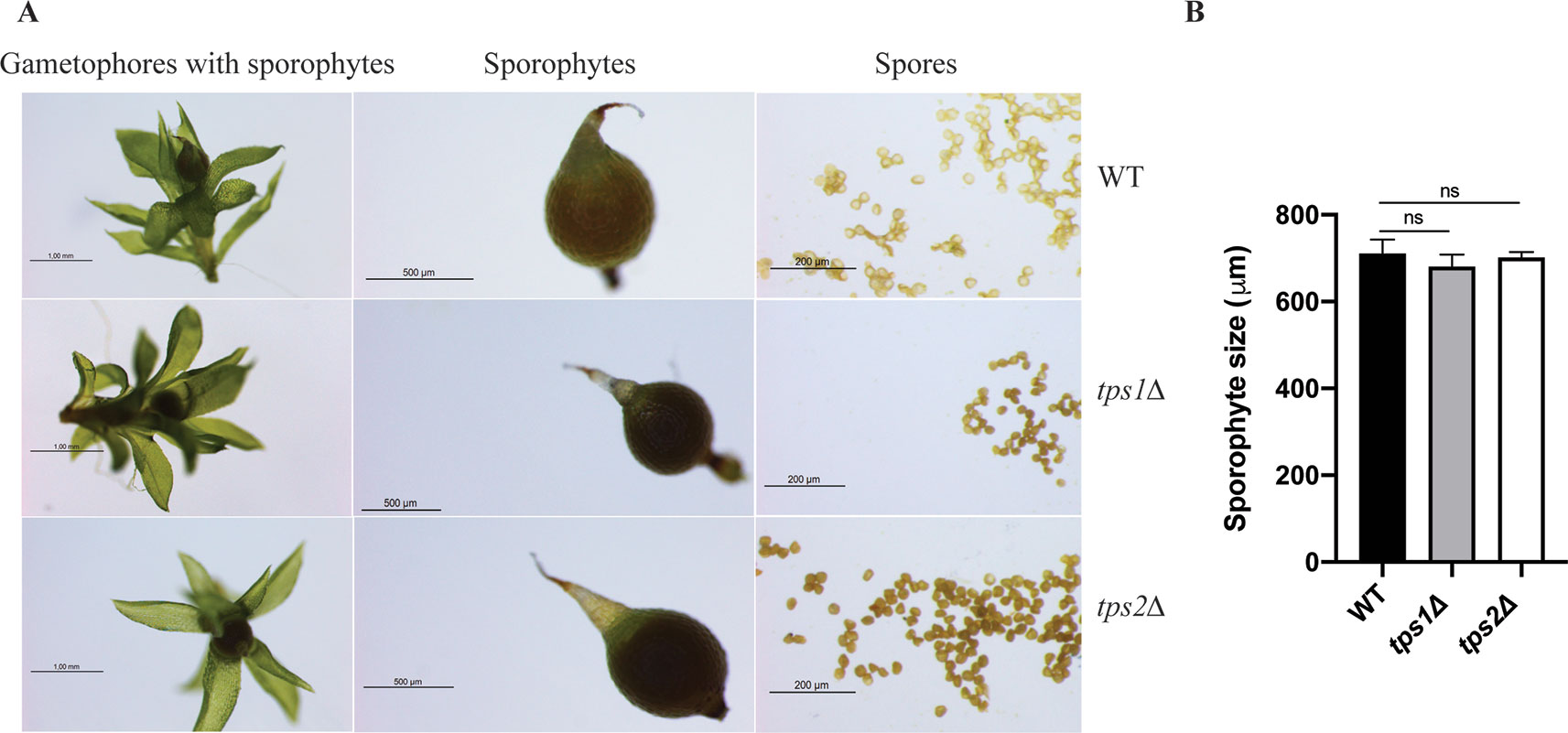
Figure 8 Morphology of sporophytes of wild-type (WT), tps1Δ, and tps2Δ mutants (A). Measurement of sporophyte size of WT and mutants (B). Data represent mean ± SD of six sporophytes for each line. Statistical analysis with one-way ANOVA, ns, no significant difference.
Next, metabolite levels were determined in the knockout lines to examine whether altered expression levels of class I TPS genes affect trehalose and Tre-6P contents. All plants were grown on BCDAT medium for 1 month under continuous light at 25°C. As expected, trehalose levels were significantly reduced in all the knockout lines (Figure 9A). Comparison of Tre-6P levels in all knockout lines, compared to the wild type, showed an expected reduction in tps1∆ and tps1∆ tps2∆ knockout lines, but no significant reduction in the tps2∆ line (Figure 9B).
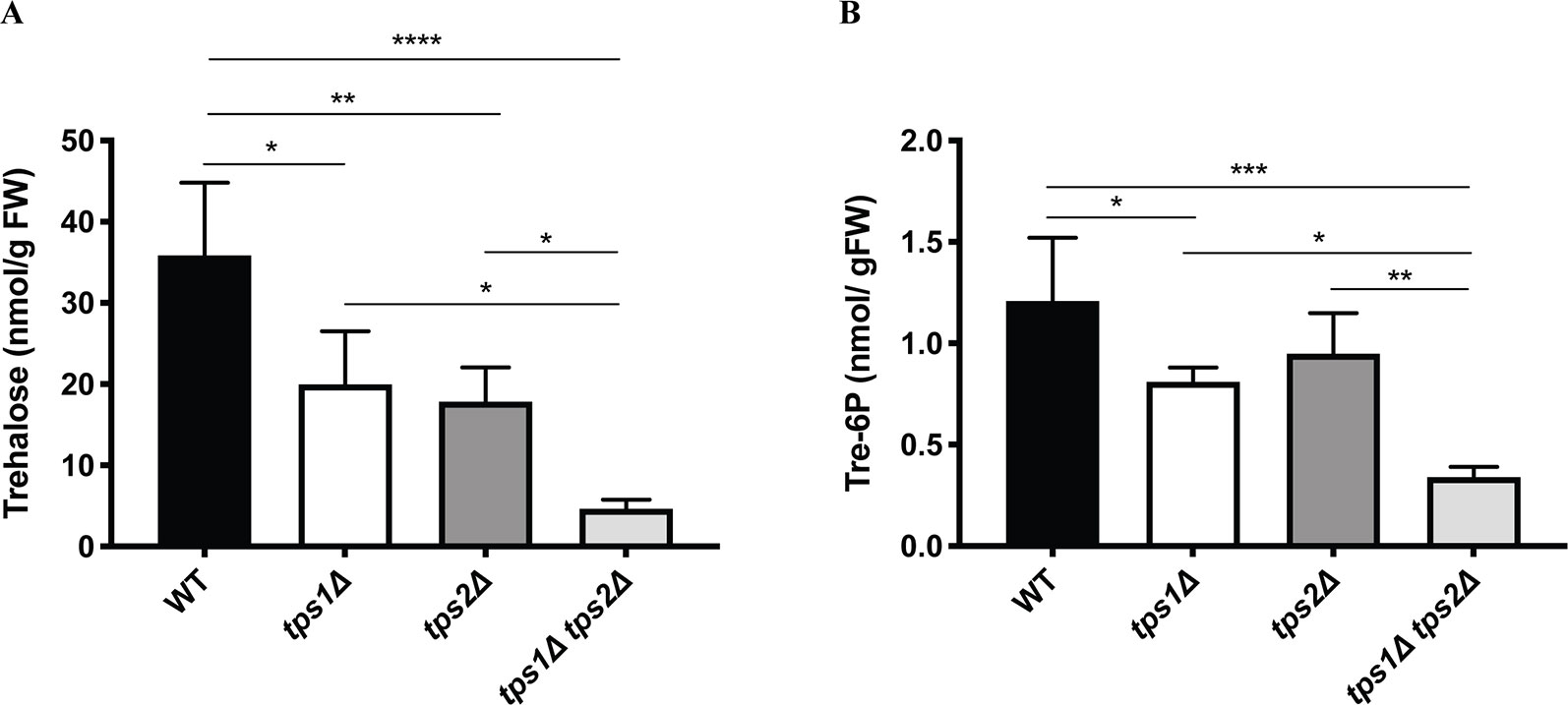
Figure 9 Measurement of trehalose (A) and trehalose-6-phosphate (B) levels in wild-type (WT) and transgenic plants. Data represent mean ± SD of four biological replicates. Statistical analysis with one-way ANOVA. *p ≤ 0.05, **p ≤ 0.01, ***p ≤ 0.001, ****p ≤ 0.0001.
Transgenic Lines in Sugar Growth Conditions
Tre-6P has been reported as a key regulator of carbon utilization for growth and development in plants. Disturbance of Tre-6P levels strongly affects plant metabolic signaling (Eastmond et al., 2002; Schluepmann et al., 2003; Avonce et al., 2004; Schluepmann et al., 2004; Martins et al., 2013; Lunn et al., 2014; Griffiths et al., 2016). In P. patens it has been reported that caulonema formation is stimulated in the presence of high levels of sugars or high light, which induce the plant to grow rapidly. In contrast, low light or low levels of sugars will force the plant to produce more energy by formation of photosynthetic tissues, such as chloronema and gametophores (Thelander et al., 2005). Moreover, Olsson et al. (2003) showed that supplied sugars stimulate caulonema filament formation. Therefore, to investigate the relationship of trehalose metabolism and energy availability, we grew the plants on BCDAT agar plates with externally supplied glucose (25 and 150 mM) and sucrose (25 and 150 mM). Addition of glucose or sucrose, even at low levels (25 mM), induced the formation of caulonema in the wild type and in the tps1Δ and tps2Δ mutants. The double mutant, tps1∆ tps2∆, seemed to be less susceptible to the supplied energy (Figure 10). When grown on 25 mM glucose, caulonema formation was less induced compared to the wild type and the single mutants. However, increasing the concentration of glucose to 150 mM was able to induce caulonema in the tps1∆ tps2∆ mutant (Figure 10). The double mutant appeared less sensitive to the effect of exogenous sucrose as compared to the effect of glucose.
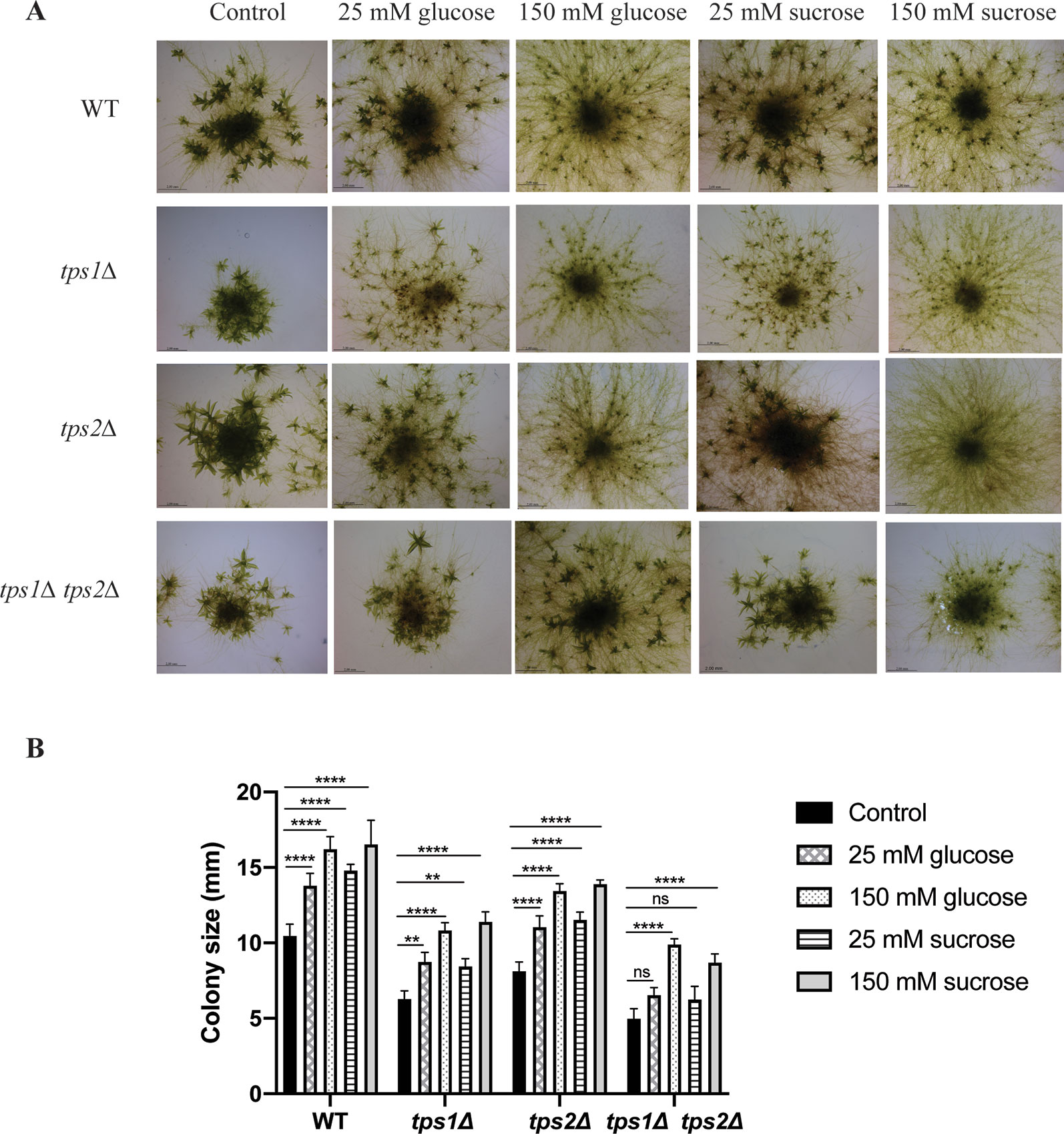
Figure 10 Effect of sugars on growth of wild-type (WT) and transgenic plants. (A) Morphology of wild-type (WT) and knockout plants grown on plain BCDAT medium or BCDAT medium supplemented with different sugars (glucose and sucrose). Bars represent 2 mm. (B) Measurement of colony size of WT and knockout plants. Data represent mean ± SD of three individuals of protonema colonies. Statistical analysis with two-way ANOVA, **p ≤ 0.01, ****p ≤ 0.0001, ns, no significant difference.
Besides energy availability, plant hormones also affect many signaling pathways and have pronounced effects on growth and development. The balance between caulonema and chloronema is regulated by hormones. For instance, auxins induce the formation of caulonema, while cytokinins show the opposite effect (Ashton et al., 1979; Reski and Abel, 1985). Thus, we studied the effect of auxin (NAA) and cytokinin (BAP) on trehalose metabolism in P. patens. For this purpose, transgenic plants were grown on media containing different concentrations of NAA or BAP, and after 1 month, the effect on morphology was analyzed by microscopy analysis.
As expected, BAP reduced the size of colonies and induced the formation of callus-like tissues in the wild type and all mutants (Figures 11A, B). This effect was more severe in the tps1∆ and tps1∆ tps2∆ mutants. Whereas, NAA-induced caulonema formation was clearly observed in wild-type plants, and in the tps2∆ line, there was only a very small effect of NAA on the tps1∆ and tps1∆ tps2∆ knockout lines as no well-defined outward growth was observed (Figures 11C, D).
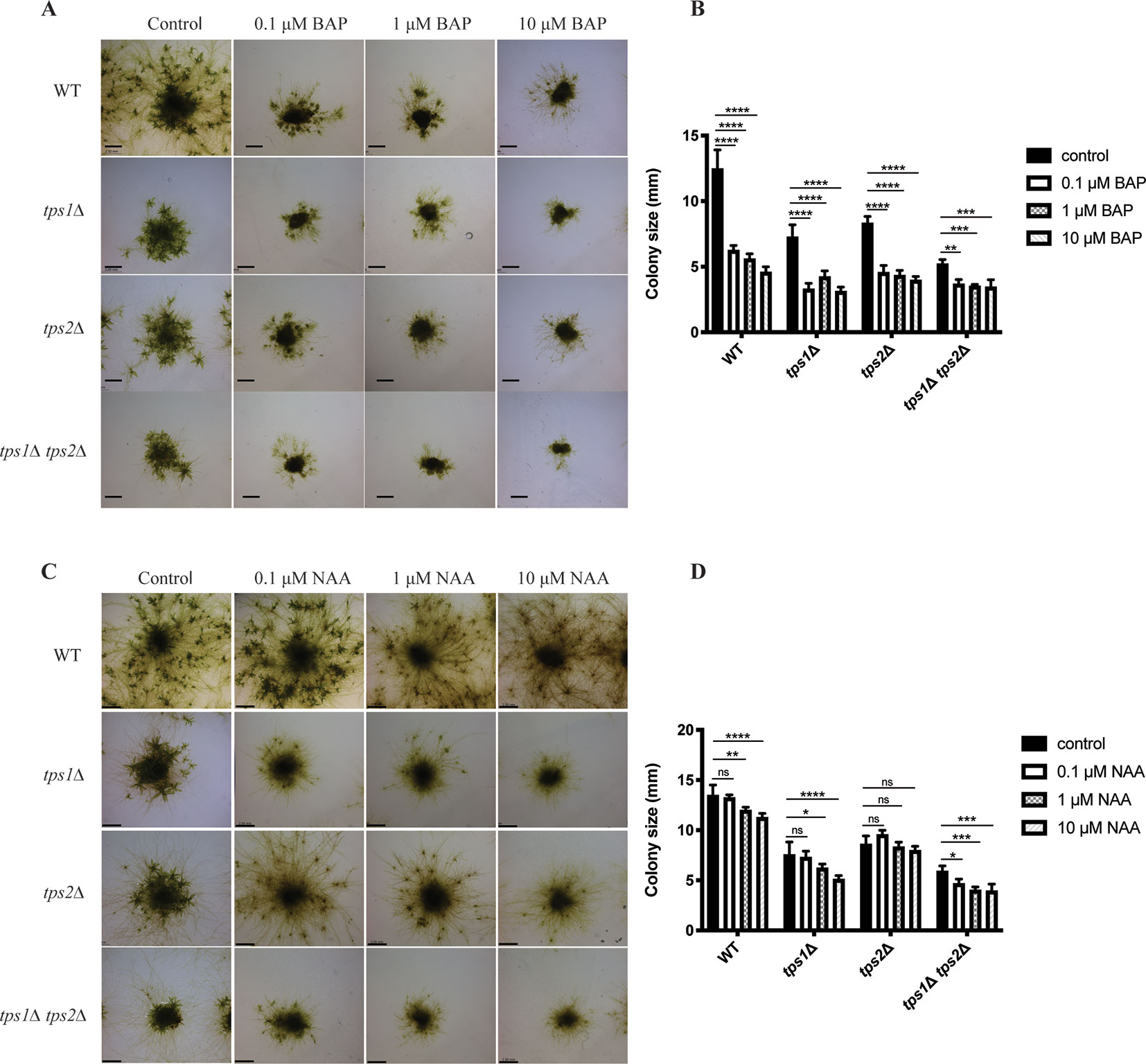
Figure 11 Morphology of wild-type (WT) and transgenic plants grown on different concentrations of cytokinin and auxin. (A) Representative images of protonema morphology of plants grown on plain BCDAT medium (control) or BCDAT medium added with different concentrations of BAP (0.1, 1, 10 µM). Bars represent 2 mm. (B) Measurement of colony size of WT and mutants after 1-month growth on the medium added with BAP. Data represent mean ± SD of four individuals of protonema colonies. (C) Representative of protonema morphology of plants grown on plain BCDAT medium (control) or BCDAT medium added with different concentrations of NAA (0.1, 1, 10 µM). Bars represent 2 mm. (D) Measurement of colony size of WT and mutants after 1-month growth on the medium added with NAA. Data represent mean ± SD of four individuals of protonema colonies. Statistical analysis with two-way ANOVA, *p ≤ 0.05, **p ≤ 0.01, ***p ≤ 0.001, ****p ≤ 0.0001, ns, no significant difference.
Discussion
In this study, we present the molecular and functional characterization of the class I TPS proteins in P. patens. By BLAST analysis, using the Cosmoss database, two genes were subsequently found and annotated as PpTPS1 and PpTPS2. By using yeast complementation studies, we show that both genes have TPS enzymatic activity, but no TPP activity, similar to other plant class I enzymes. Moreover, P. patens together with A. thaliana (Delorge et al., 2015) are the only two plant species described so far with more than one catalytically active TPS enzyme. Interestingly and different from all characterized plants is that P. patens has two TPS enzymes with an N-terminal extension. Truncation of the N-terminus of PpTPS1 and PpTPS2 strongly enhanced their enzymatic activity as well as increased trehalose levels when expressed in yeast. Interestingly, a splice variant of PpTPS2 (PpTPS2V6.1) lacking the N-terminus has been predicted in the database. This might be the first time by which such a natural splice variant of the plant class I TPS proteins has been described, suggesting that harboring this variant may allow the plant to modulate rapidly TPS activity and control tightly Tre-6P production in order to respond to environmental stimuli.
Lower trehalose and Tre-6P levels were detected in knockout plants in comparison with the wild type. However, there was no significant reduction in Tre-6P levels in the tps2∆ line as compared to the wild type. This is possibly due to TPS1, which is still capable of synthesizing Tre-6P and which is still present in the tps2∆ mutant. Interestingly, Tre-6P levels were significantly lower in the single tps1∆ mutant, which harbors TPS2. This indicates that TPS1 might be more active than TPS2 as its expression was lower compared to TPS2 (Figure 6). Remarkably, low amounts of Tre-6P were still present in the tps1∆ tps2∆ mutant. This indicates that other active trehalose-6-phosphate synthase(s) might be present in P. patens. Four class II TPS proteins (PpTPS3-PpTPS6) were described in P. patens (Avonce et al., 2010). Hence, Tre-6P in the tps1∆ tps2∆ mutant might be synthesized by the class II proteins. Therefore, further research on the functional characterization of class II proteins needs to be performed to confirm this assumption.
Different factors including sugars, light, and plant hormones define the balance between chloronema and caulonema growth in Physcomitrella. Conditions such as high light, or the presence of glucose or sucrose that provide energy for plants induce caulonema formation (Thelander et al., 2005). The same was observed in the presence of auxins. Conversely, when conditions are less energy favorable, chloronema branching is stimulated in order to invest in photosynthetic tissues. Furthermore, in the presence of cytokinins, caulonema is inhibited and chloronema is enhanced. It is likely that factors inducing the formation of one type of filaments inhibit the development of the other. Here we demonstrated that caulonema filaments were significantly reduced in knockout lines compared to wild-type plants. Tre-6P was reported to regulate sugar utilization in normal growth (Schluepmann et al., 2003); therefore, a dramatic decrease in Tre-6P levels in the tps1∆ tps2∆ mutant might lead to a disturbance in carbon allocation, resulting in a reduced growth rate. Additionally, the double mutant was unable to produce sporophytes. Sporophytes largely depend on gametophytes for energy and nutrients. Sporophytes require more energy than they can supply themselves. In P. patens, transfer of sugars from gametophores to sporophytes is facilitated through transfer cells, which are present at the boundary of the gametophores and sporophytes (Courtice et al., 1978). It is possible that in the double tps1∆ tps2∆ mutant the allocation of energy supply towards the sporophytes from gametophores is somehow hampered, leading to a failure in sexual reproduction. We then tested the effect of hormones on plant growth. We show that single knockout lines, especially the tps1∆ mutant, were less susceptible to NAA-induced caulonema formation. This phenotype was more pronounced in the tps1∆ tps2∆ mutant, which could explain the lack of caulonema in this mutant. Moreover, it was demonstrated that the transition from chloronema to caulonema is promoted by auxin (Imaizumi et al., 2002; Decker et al., 2006; Jang and Dolan, 2011). We revealed that the differentiation of chloronemal cells to caulonemal cells is significantly decreased in the knockout mutants, especially in the tps1∆ tps2∆ mutant. These results illustrate that auxin-induced caulonema formation relies on the PpTPS1/PpTPS2-dependent pathway. The link between trehalose metabolism and auxin signaling has also been revealed previously. A. thaliana seedlings expressing the E. coli TPS gene (OtsA) with elevated Tre-6P levels displayed a down-regulation of auxin/IAA genes involved in auxin response (Paul et al., 2010). Opposite to auxin, cytokinins did not have a profound effect compared to the effect of NAA, but there was a slight indication towards higher sensitivity in knockout plants. Remarkably, caulonema production in the tps1Δ mutant was less than that in the tps2Δ mutant under all treatment conditions. Moreover, the former was also less sensitive to sugars and plant hormones than the latter. This suggests that PpTPS1 might be more preferable than PpTPS2 for sensing the growth factors.
Interestingly, an increase in caulonema formation was observed in single and double mutants when high glucose or sucrose was supplied, implying that these plants are still able to sense the supplied energy. Tre-6P is a central metabolic sensor, which regulates plant growth and development. Adequate Tre-6P levels are required for carbon utilization during normal growth. It has been demonstrated previously that Arabidopsis plants with reduced Tre-6P levels experienced a growth inhibition when exogenous sugars were added (Schluepmann et al., 2003). This brings us to a question of how energy is sensed in P. patens. Sensing energy levels in the cell could be the task of PpSNF1a and/or PpSNF1b (Thelander et al., 2004). A strong connection between trehalose metabolism and SnRK1-signaling has been indicated. SnRK1 is a sucrose-non fermenting related kinase 1, and is part of a serine/threonine kinase family that acts as a metabolite sensor to adapt metabolism accordingly (Jossier et al., 2009). Upon activation by starvation conditions, SnRK1 represses energy-consuming anabolic processes, whereas it induces catabolism, in order to ensure plant survival and stress tolerance. It has been revealed that Tre-6P inhibits SnRK1 (Zhang et al., 2009; Lunn et al., 2014). Recently, it was reported that Tre-6P interacts directly with a catalytic SnRK1α subunit (KIN10) in vitro (Zhai et al., 2018). In P. patens, PpSNF1a and PpSNF1b have been shown to be involved in the maintenance of sufficient energy levels, clearly demonstrated by the inability of snf1a∆ snf1b∆ to grow in low light or darkness (Thelander et al., 2004). Interestingly, the phenotype of the snf1a∆ snf1b∆ mutant is opposite to what is seen in the tps1∆ tps2∆ mutant. In the former, caulonema formation was highly induced and chloronema branching was reduced, which suggest that the plant experiences a constitutive high energy growth mode. In the tps1∆ tps2∆ mutant, caulonema filaments were reduced. However, the tps1∆ tps2∆ mutant was still able to develop caulonema, although to a lesser extent, in high light conditions (Supplementary Figure 3). Previously, the link between sucrose and Tre-6P was not fully clear. Nonetheless, it was recently elucidated that Tre-6P regulates sucrose levels by inhibiting the cleavage of sucrose by sucrose synthase (SUS) in castor beans (Ricinus communis) (Fedosejevs et al., 2018). This feedback inhibition may control sucrolytic flux from the source to the sink. It was reported that SUS expression in potato is dependent on SnRK1 (Purcell et al., 1998). A possibility is that in the tps1∆ tps2∆ mutant a relief of Tre-6P-mediated SUS repression might occur, in combination with an increase of PpSNF1a and/or PpSNF1b-induced SUS expression, leading to changes in hexose levels and altered gene expression. In A. thaliana, it was demonstrated that sucrose promotes hypocotyl elongation in the light by activating auxin signaling (Lilley et al., 2012). Recently, sucrose-induced hypocotyl elongation was reported to involve the SnRK1/Tre-6P system as either KIN10 overexpression or a tps1Δ mutant shows a defect in hypocotyl elongation, which is induced by sucrose under light/dark cycles (Simon et al., 2018). All taken together, it suggests that Tre-6P/sucrose signaling, PpSNF1, and the auxin pathway might be working together to control growth rate in P. patens. The precise mechanism by which external factors and growth regulators affect downstream signaling pathways in Physcomitrella needs to be further investigated.
In S. cerevisiae, there is a clear connection between trehalose biosynthesis and the activity of hexokinase (Bonini et al., 2003). The S. cerevisiae tps1Δ mutant is unable to grow on glucose-containing medium, which is caused by an overactive influx of sugar into glycolysis, mainly due to an overactive hexokinase (Blázquez et al., 1993; Thevelein and Hohmann, 1995). In A. thaliana, AtTPS1 is very lowly expressed in HXK1-antisense plants, suggesting that expression of AtTPS1 is dependent on HXK1 and that AtTPS1 might participate downstream of HXK1 (Avonce et al., 2004). If this hypothesis occurs in P. patens, we might expect similar phenotypes of hxk1 and class I TPS mutants. Indeed, caulonema filaments and size of colonies were reduced in the hxk1 knockout mutant compared to the wild type (Thelander et al., 2005). Furthermore, the inhibition of cytokinins on caulonema formation was more pronounced in the hxk1 mutant. These phenotypes are similar to what we observed in the tps1∆ tps2∆ mutant in our study. Therefore, the class I TPS proteins and hexokinase might work in the same pathway of sensing growth factors to monitor the balance between chloronema and caulonema growth in Physcomitrella.
Conclusions
This work demonstrates the importance of class I TPS proteins in regulating growth and development as well as sexual reproduction of the moss P. patens. The growth of caulonema filaments is regulated by PpTPS1 and PpTPS2 as a double knockout mutant displayed a failure in caulonema expansion. Furthermore, the moss is unable to produce sporophytes when the class I TPS genes are absent. Additionally, the presence of PpTPS1 and PpTPS2 is essential for sensing and signaling growth factors including sugars and plant hormones. Disruption in Tre-6P production led to a failure in the use of supplied sugar and hormones. These findings are consistent with studies in trehalose metabolism in angiosperms. This indicates that the trehalose metabolism is crucial in regulating plant responses over environmental conditions and that are roles conserved through the plant kingdom. Moreover, it is likely that class I TPS proteins sense energy availability and phytohormones through a pathway involving PpSNF1 and HXK1. Further investigation needs to be performed to support this assumption.
Data Availability Statement
The raw data and generated strains supporting the conclusions of this article will be made available by the authors, without undue reservation, to any qualified researcher.
Author Contributions
ID conceived the experiments. ID and TP analyzed the data. NA helped in the generation of moss mutants and contributed to data analysis. TP, ID, and PD wrote the paper. PD supervised the work.
Funding
This research was supported by the Fund for Scientific Research Flanders (FWO: grant number G.0859.10) and the fund from Vietnam International Education Development (VIED). Ines Delorge was supported by a grant from the Flemish Institute for Science and Technology (IWT).
Conflict of Interest
The authors declare that the research was conducted in the absence of any commercial or financial relationships that could be construed as a potential conflict of interest.
Acknowledgments
We would like to thank Nico Van Goethem (VIB-KU Leuven Center for Microbiology) for assistance with preparation of the figures. We thank John Lunn and Regina Feil (Max Planck Golm) for Tre-6P measurements.
Supplementary Material
The Supplementary Material for this article can be found online at: https://www.frontiersin.org/articles/10.3389/fpls.2019.01694/full#supplementary-material
Supplementary Figure 1 | Southern blot analysis of tps1∆ mutant (A-C) and tps2∆ mutant (D-F). Genomic DNA of several independent tps1∆ lines (1-5) was digested once by BamHI or XbaI (A), generating a band of approximately 5.2 kb (B) or 4.7 kb (C), respectively. Genomic DNA of several independent tps2∆ lines (1-6) was double digested by KpnI and XhoI or digested once by HindIII (D), generating a band of approximately 7.5 kb (E) or 4.5 kb (F), respectively. A positive control (+) was included to verify correct recognition of the probe. Ladder (L) is shown twice but differently exposed. Arrows show the position of correct digested bands.
Supplementary Figure 2 | Caulonemal cells and chloronemal cells in protonema filaments of wild-type (WT) and transgenic plants grown on BCDAT agar medium at 25%°C in continuous light for 10 days. (A) Representative of protonema filaments at the edge of a colony. Magnification 10X. (B) Representative of chloronemal and caulonemal cells. Magnification 40X.
Supplementary Figure 3 | Effect of high light (50 µmol m-2s-1) in combination with sugar on growth of wild-type (WT) and the tps1∆ tps2∆ mutant. (A) Morphology of WT and the tps1∆ tps2∆ mutant grown on BCDAT medium, supplied with sugar (either 25 mM glucose or 25 mM sucrose), in high light for one month. (B) Measurement of colony size of WT and the tps1∆ tps2∆ mutant. Data represent mean ± SD of four individuals of protonema colonies. Statistical analysis with two-way ANOVA, *p≤0.05, **p≤0.01, ****p≤0.0001.
Supplementary Table 1 | List of primers used in the study. Abbreviations: FW (forward), RV (reverse), GSP (gene-specific primer), CDS (coding sequence), qPCR (quantitative PCR).
References
Adams, R. P., Kendall, E., Kartha, K. K. (1990). Comparison of free sugars in growing and desiccated plants of Selaginella lepidophylla. Biochem. Syst. Ecol. 18, 107–110. doi: 10.1016/0305-1978(90)90044-G
Arif, M. A., Alseekh, S., Harb, J., Fernie, A., Frank, W. (2018). Abscisic acid, cold and salt stimulate conserved metabolic regulation in the moss Physcomitrella patens. Plant Biol. 20 (6), 1014–1022. doi: 10.1111/plb.12871
Ashton, N. W., Grimsley, N. H., Cove, D. J. (1979). Analysis of gametophytic development in the moss, Physcomitrella patens, using auxin and cytokinin resistant mutants. Planta 144 (5), 427–435. doi: 10.1007/BF00380118
Avonce, N., Leyman, B., Mascorro-Gallardo, J. O., Van Dijck, P., Thevelein, J. M., Iturriaga, G. (2004). The Arabidopsis trehalose-6-P-synthase AtTPS1 gene is a regulator of glucose, abscisic acid and stress signaling. Plant Physiol. 136, 3649–3659. doi: 10.1104/pp.104.052084
Avonce, N., Mendoza-Vargas, A., Morett, E., Iturriaga, G. (2006). Insights on the evolution of trehalose biosynthesis. BMC Evol. Biol. 6, 109. doi: 10.1186/1471-2148-6-109
Avonce, N., Wuyts, J., Verschooten, K., Vandesteene, L., Van Dijck, P. (2010). The Cytophaga hutchinsonii ChTPSP: first characterized bifunctional TPS-TPP protein as putative ancestor of all eukaryotic trehalose biosynthesis proteins. Mol. Biol. Evol. 27, 359–369. doi: 10.1093/molbev/msp241
Baena-González, E., Rolland, F., Thevelein, J. M., Sheen, J. (2007). A central integrator of transcription networks in plant stress and energy signalling. Nature 448, 938–942. doi: 10.1038/nature06069
Baur, A., Reski, R., Gorr, G. (2005). Enhanced recovery of a secreted recombinant human growth factor using stabilizing additives and by co-expression of human serum albumin in the moss Physcomitrella patens. Plant Biotechnol. J. 3, 331– 340. doi: 10.1111/j.1467-7652.2005.00127.x
Blázquez, M. A., Gancedo, C. (1995). Mode of action of the qcr9 and cat3 mutations in the suppression of the lack of growth in glucose of Saccharomyces cerevisiae tps1 mutants. Mol. Gen. Genet. 249, 655–664.
Blázquez, M. A., Lagunas, R., Gancedo, C., Gancedo, J. M. (1993). Trehalose-6-phosphate, a new regulator of yeast glycolysis that inhibits hexokinases. FEBS Lett. 329, 51–54. doi: 10.1007/BF00418035
Blázquez, M. A., Santos, E., Flores, C. L., Martinez Zapater, J. M., Salinas, J., Gancedo, C. (1998). Isolation and molecular characterization of the Arabidopsis TPS1 gene, encoding trehalose-6-phosphate synthase. Plant J. 13, 685–689. doi: 10.1016/0014-5793(93)80191-V
Bledsoe, S. W., Henry, C., Griffiths, C. A., Paul, M. J., Feil, R., Lunn, J. E., Stitt, M., Lagrimini, L. M. (2017) The role of Tre6P and SnRK1 in maize early kernel development and events leading to stress-induced kernel abortion. BMC Plant Biol 17(1):74. doi: 10.1186/s12870-017-1018-2
Bonini, B. M., Van Dijck, P., Thevelein, J. M. (2003). Uncoupling of the glucose growth defect and the deregulation of glycolysis in Saccharomyces cerevisiae Tps1 mutants expressing trehalose-6-phosphate-insensitive hexokinase from Schizosaccharomyces pombe. Biochim. Biophys. Acta 1606, 83–93. doi: 10.1016/S0005-2728(03)00086-0
Bonini, B. M., Van Dijck, P., Thevelein, J. M. (2004). “Trehalose metabolism: enzymatic pathways and physiological functions,” in The Mycota, A treatise on the Biology of fungi with emphasis on systems for fundamental and applied research, 2nd ed., vol. 291-332 . Eds. Esser, K., Lemke, G. A. (Berlin-Heidelberg: Springer Verlag). doi: 10.1007/978-3-662-06064-3_15
Cabib, E., Leloir, L. F. (1958). The biosynthesis of trehalose phosphate. J. Biol. Chem. 231, 259–275.
Carillo, P., Feil, R., Gibon, Y., Satoh-Nagasawa, N., Jackson, D., Bläsing, O. E., et al. (2013). A fluorometric assay for trehalose in the picomole range. Plant Methods 9, 21. doi: 10.1186/1746-4811-9-21
Courtice, G. R. M., Ashton, N. W., Cove, D. J. (1978). Evidence for the restricted passage of metabolites into the sporophyte of the moss Physcomitrella patens (Hedw.) . Br. Eur. J. Bryol. 10, 191–198. doi: 10.1179/jbr.1978.10.2.191
Cove, D. J., Kammerer, W., Knight, C. D., Leech, M. J., Martin, C. R., Wang, T. L. (1991). Developmental genetic studies of the moss, Physcomitrella patens. Symp. Soc Exp. Biol. 45, 31–43.
Cove, D. J. (2005). The moss Physcomitrella patens. Annu. Rev. Genet. 39, 339–358. doi: 10.1146/annurev.genet.39.073003.110214
Debast, S., Nunes-Nesi, A., Hajirezaei, M. R., Hofmann, J., Sonnewald, U., Fernie, A. R., et al. (2011). Altering trehalose-6-phosphate content in transgenic potato tubers affects tuber growth and alters responsiveness to hormones during sprouting. Plant Physiol. 156, 1754–1771. doi: 10.1104/pp.111.179903
Decker, E. L., Frank, W., Sarnighausen, E., Reski, R. (2006). Moss systems biology en route: phytohormones in Physcomitrella development. Plant Biol. (Stuttg) 8 (3), 397–405. doi: 10.1055/s-2006-923952
Delatte, T. L., Sedijani, P., Kondou, Y., Matsui, M., de Jong, G. J., Somsen, G. W., et al. (2011). Growth arrest by trehalose-6-phosphate: an astonishing case of primary metabolite control over growth by way of the SnRK1 signaling pathway. Plant Physiol. 157, 160–174. doi: 10.1104/pp.111.180422
Delorge, I., Janiak, M., Carpentier, S. C., Van Dijck, P. (2014). Fine tuning of trehalose biosynthesis and hydrolysis as novel tools for the generation of abiotic stress tolerant plants. Front. Plant Sci. 5, 147. doi: 10.3389/fpls.2014.00147
Delorge, I., Figueroa, C. M., Feil, R., Lunn, J. E., Van Dijck, P. (2015). Trehalose-6-phosphate synthase 1 is not the only active TPS in Arabidopsis thaliana. Biochem. J. 466 (2), 283–290. doi: 10.1042/BJ20141322
Eastmond, P. J., van Dijken, A. J. H., Spielman, M., Kerr, A., Tissier, A. F., Dickinson, H. G., et al. (2002). Trehalose-6-phosphate synthase 1, which catalyses the first step in trehalose synthesis, is essential for Arabidopsis embryo maturation. Plant J. 29, 225–235. doi: 10.1046/j.1365-313x.2002.01220.x
Eastmond, P. J., Li, Y., Graham, I. A. (2003). Is trehalose-6-phosphate a regulator of sugar metabolism in plants? J. Exp. Bot. 54, 533–537. doi: 10.1093/jxb/erg039
Erxleben, A., Gessler, A., Vervliet-Scheebaum, M., Reski, R. (2012). Metabolite profiling of the moss Physcomitrella patens reveals evolutionary conservation of osmoprotective substances. Plant Cell Rep. 31 (2), 427–436. doi: 10.1007/s00299-011-1177-9
Fedosejevs, E. T., Feil, R., Lunn, J. E., Plaxton, W. C. (2018). The signal metabolite trehalose-6-phosphate inhibits the sucrolytic activity of sucrose synthase from developing castor beans. FEBS Lett. 592 (15), 2525–2532. doi: 10.1002/1873-3468.13197
Fernandez, O., Béthencourt, L., Quero, A., Sangwan, R. S., Clément, C. (2010). Trehalose and plant stress responses: friend or foe? Trends Plant Sci. 15, 409–417. doi: 10.1016/j.tplants.2010.04.004
Figueroa, C. M., Feil, R., Ishihara, H., Watanabe, M., Kolling, K., Krause, U., et al. (2016). Trehalose 6-phosphate coordinates organic and amino acid metabolism with carbon availability. Plant J. 85, 410–423. doi: 10.1111/tpj.13114
Ge, L. F., Chao, D. Y., Shi, M., Zhu, M. Z., Gao, J. P., Lin, H. X. (2008). Overexpression of the trehalose-6-phosphate phosphatase gene OsTPP1 confers stress tolerance in rice and results in the activation of stress responsive genes. Planta 228, 191– 201. doi: 10.1007/s00425-008-0729-x
Gietz, R. D., Schliestl, R. H., Willems, A. R., Woods, R. A. (1995). Studies on the transformation of intact yeast cells by the LiAc/SS-DNA/PEG procedure. Yeast 11, 355–360. doi: 10.1002/yea.320110408
Goddijn, O. J. M., van Dun, K. (1999). Trehalose metabolism in plants. Trends Plant Sci. 4, 315–319. doi: 10.1016/S1360-1385(99)01446-6
Griffiths, C. A., Paul, M. J., Foyer, C. H. (2016). Metabolite transport and associated sugar signalling systems underpinning source/sink interactions. Biochim. Biophys. Acta 1857, 1715–1725. doi: 10.1016/j.bbabio.2016.07.007
Hohmann, S., Neves, M. J., de Koning, W., Alijo, R., Ramos, J., Thevelein, J. M. (1993). The growth and signalling defects of the ggs1 (fdp1/byp1) deletion mutant on glucose are suppressed by a deletion of the gene encoding hexokinase PII. Curr. Genet. 23, 281–289. doi: 10.1007/BF00310888
Hottiger, T., Schmutz, P., Wiemken, A. (1987). Heat-induced accumulation and futile cycling of trehalose in Saccharomyces cerevisiae. J. Bacteriol. 169, 5518–5522. doi: 10.1128/jb.169.12.5518-5522.1987
Imaizumi, T., Kadota, A., Hasebe, M., Wada, M. (2002). Cryptochrome light signals control development to suppress auxin sensitivity in the moss Physcomitrella patens. Plant Cell. 14 (2), 373–386. doi: /10.1105/tpc.010388
Jang, G., Dolan, L. (2011). Auxin promotes the transition from chloronema to caulonema in moss protonema by positively regulating PpRSL1 and PpRSL2 in Physcomitrella patens. New Phytol. 192, 319–327. doi: 10.1111/j.1469-8137.2011.03805.x
Jossier, M., Bouly, J.-P., Meimoun, P., Arjmand, A., Lessard, P., Hawley, S., et al. (2009). SnRK1 (SNF1-related kinase 1) has a central role in sugar and ABA signalling in Arabidopsis thaliana. Plant J. 59, 316–328. doi: 10.1111/j.1365-313X.2009.03871.x
Kolbe, A., Tiessen, A., Schluepmann, H., Paul, M., Ulrich, S., Geigenberger, P. (2005). Trehalose-6-phosphate regulates starch synthesis via posttranslational redox activation of ADP-glucose pyrophosphorylase. Proc. Natl. Acad. Sci. U.S.A. 102, 11118–11123. doi: 10.1073/pnas.0503410102
Leyman, B., Van Dijck, P., Thevelein, J. M. (2001). An unexpected plethora of trehalose biosynthesis genes in Arabidopsis thaliana. Trends Plant Sci. 6 (11), 510–513. doi: 10.1016/S1360-1385(01)02125-2
Li, H. W., Zang, B. S., Deng, X. W., Wang, X. P. (2011). Overexpression of the trehalose-6-phosphate synthase gene OsTPS1 enhances abiotic stress tolerance in rice. Planta 234, 1007–1018. doi: 10.1007/s00425-011-1458-0
Lilley, J. L., Gee, C. W., Sairanen, I., Ljung, K., Nemhauser, J. L. (2012). An endogenous carbon-sensing pathway triggers increased auxin flux and hypocotyl elongation. Plant Physiol. 160 (4), 2261–2270. doi: 10.1104/pp.112.205575
Lunn, J. E., Feil, R., Hendriks, J. H. M., Gibon, Y., Morcuende, R., Osuna, D., et al. (2006). Sugar-induced increases in trehalose-6-phosphate are correlated with redox activation of ADP-glucose pyrophosphorylase and higher rates of starch synthesis in Arabidopsis thaliana. Biochem. J. 397, 139–148. doi: 10.1042/BJ20060083
Lunn, J. E., Delorge, I., Figueroa, C. M., Van Dijck, P., Stitt, M. (2014). Trehalose metabolism in plants. Plant J. 79, 544–567. doi: 10.1111/tpj.12509
Martínez-Barajas, E., Delatte, T., Schluepmann, H., de Jong, G. J., Somsen, G. W., Nunes, C., et al. (2011). Wheat grain development is characterized by remarkable trehalose 6-phosphate accumulation pregrain filling: tissue distribution and relationship to SNF1-related protein kinase1 activity. Plant Physiol. 156 (1), 373–381. doi: 10.1104/pp.111.174524
Martins, M. C., Hejazi, M., Fettke, J., Steup, M., Feil, R., Krause, U., et al. (2013). Feedback inhibition of starch degradation in Arabidopsis leaves mediated by trehalose 6-phosphate. Plant Physiol. 163, 1142–1163.doi: 10.1104/pp.113.226787
Müller, J., Boller, T., Wiemken, A. (1995). Trehalose and trehalase in plants: recent developments. Plant Sci. 112, 1–9. doi:10.1016/0168-9452(95)04218-J
Nagao, M., Minami, A., Arakawa, K., Fujikawa, S., Takezawa, D. (2005). Rapid degradation of starch in chloroplasts and concomitant accumulation of soluble sugars associated with ABA-induced freezing tolerance in the moss Physcomitrella patens. J. Plant Physiol. 162 (2), 169–180. doi: 10.1016/j.jplph.2004.06.012
Nagao, M., Oku, K., Minami, A., Mizuno, K., Sakurai, M., Arakawa, K., et al. (2006). Accumulation of theanderose in association with development of freezing tolerance in the moss Physcomitrella patens. Phytochemistry 67 (7), 702–709. doi: 10.1016/j.phytochem.2006.01.031
Neves, M. J., Hohmann, S., Bell, W., Dumortier, F., Luyten, K., Ramos, J., et al. (1995). Control of glucose influx into glycolysis and pleiotropic effects studied in different isogenic sets of Saccharomyces cerevisiae mutants in trehalose biosynthesis. Curr. Genet. 27, 110–122. doi: 10.1007/BF00313424
Nuccio, M. L., Wu, J., Mowers, R., Zhou, H. P., Meghji, M., Primavesi, L. F., et al. (2015). Expression of trehalose-6-phosphate phosphatase in maize ears improves yield in well-watered and drought conditions. Nat. Biotechnol. 33 (8), 862–869. doi: 10.1038/nbt.3277
Olsson, T., Thelander, M., Ronne, H. (2003). A Novel Type of Chloroplast Stromal Hexokinase Is the Major Glucose-phosphorylating Enzyme in the Moss Physcomitrella patens. J. Biol. Chem. 278 (45), 44439–44447. doi: 10.1074/jbc.M30626520
Pampurova, S., Verschooten, K., Avonce, N., Van Dijck, P. (2014). Functional screening of a cDNA library from teh desiccation-tolerant plant Selaginella lepidophylla in yeast mutants identifies trehalose biosynthesis genes of plant and microbial origin. J. Plant Res. 127 (6), 803–813. doi: 10.1007/s10265-014-0663-x
Paul, M. J., Jhurreea, D., Zhang, Y., Primavesi, L. F., Delatte, T., Schluepmann, H., et al. (2010). Upregulation of biosynthetic processes associated with growth by trehalose-6-phosphate. Plant Signal Behav. 5, 386–392. doi: 10.4161/psb.5.4.10792
Penna, S. (2003). Building stress tolerance through over-producing trehalose in transgenic plants. Trends Plant Sci. 8, 355–357. doi: 10.1016/S1360-1385(03)00159-6
Purcell, P. C., Smith, A. M., Halford, N. G. (1998). Antisense expression of a sucrose nonfermenting-1-related protein kinase sequence in potato results in decreased expression of sucrose synthase in tubers and loss of sucrose-inducibility of sucrose synthase transcripts in leaves. Plant J. 14 (2), 195–202. doi: 10.1046/j.1365-313X.1998.00108.x
Ramon, M., De Smet, I., Vandesteene, L., Naudts, M., Leyman, B., Van Dijck, P., et al. (2009). Extensive expression regulation and lack of heterologous enzymatic acivity of the Class II trehalose metabolism proteins from Arabidopsis thaliana. Plant Cell Environ. 32, 1015–1032. doi: 10.1111/j.1365-3040.2009.01985.x
Rebecchi, L., Cesari, M., Altiero, T., Frigieri, A., Guidetti, R. (2009). Survival and DNA degradation in anhydrobiotic tardigrades. J. Exp. Biol. 212, 4033– 4039. doi: 10.1242/jeb.033266
Reski, R., Abel, W. O. (1985). Induction of budding on chloronemata and caulonemata of the moss, Physcomitrella patens, using isopentenyladenine. Planta 165 (3), 354–358. doi: 10.1007/BF00392232
Reski, R., Abel, W. O. (1998). Development, Genetics and Molecular Biology of Mosses. Bot. Acta 111, 1– 15. doi: 10.1111/j.1438-8677.1998.tb00670.x
Reski, R., Parsons, J., Decker, E. L. (2015). Moss-made pharmaceuticals: from bench to bedside. Plant Biotechnol. J. 13 (8), 1191–1198. doi: 10.1111/pbi.12401
Romero, C., Bellés, J. M., Vay, J. L., Serrano, R., Culiáñez-Macià, F. A. (1997). Expression of the yeast trehalose-6-phosphate synthase gene in transgenic tobacco plants: Pleiotropic phenotypes include drought tolerance. Planta 201 (3), 293–297. doi: 10.1007/s004250050069
Rother, M., Krauss, G. J., Grass, G., Wesenberg, D. (2006). Sulphate assimilation under Cd2+ stress in Physcomitrella patens - combined transcript, enzyme and metabolite profiling. Plant Cell Environ. 29 (9), 1801–1811. doi: 10.1111/j.1365-3040.2006.01557.x
Schluepmann, H., Pellny, T., van Dijken, A., Smeekens, S., Paul, M. (2003). Trehalose 6-phosphate is indispensible for carbohydrate utilization and growth in Arabidopsis thaliana. Proc. Natl. Acad. Sci. U.S.A. 100, 6849–6854. doi: 10.1073/pnas.1132018100
Schluepmann, H., van Dijken, A., Aghdasi, M., Wobbes, B., Paul, M., Smeekens, S. (2004). Trehalose mediated growth inhibition of Arabidopsis seedlings is due to trehalose-6-phosphate accumulation. Plant Physiol. 135, 879–890. doi: 10.1104/pp.104.039503
Simon, N. M. L., Kusakina, J., Fernández-López, Á., Chembath, A., Belbin, F. E., Dodd, A. N. (2018). The Energy-Signaling Hub SnRK1 Is Important for Sucrose-Induced Hypocotyl Elongation. Plant Physiol. 176 (2), 1299–1310. doi: 10.1104/pp.17.01395
Thelander, M., Olsson, T., Ronne, H. (2004). Snf1-related protein kinase 1 is needed for growth in a normal day-night light cycle. EMBO J. 23, 1900–1910. doi: 10.1038/sj.emboj.7600182
Thelander, M., Olsson, T., Ronne, H. (2005). Effect of the energy supply on filamentous growth and development in Physcomitrella patens. J. Exp. Bot. 56, 653–662. doi: 10.1093/jxb/eri040
Thevelein, J. M., Hohmann, S. (1995). Trehalose synthase: Guard to the gate of glycolysis in yeast? Trends Biochem. Sci. 20, 3–10. doi: 10.1016/S0968-0004(00)88938-0
Thiel, J., Rolletschek, H., Friedel, S., Lunn, J. E., Nguyen, T. H., Feil, R., et al. (2011). Seed-specific elevation of non-symbiotic hemoglobin AtHb1: beneficial effects and underlying molecular networks in Arabidopsis thaliana. BMC Plant Biol. 11, 48. doi: 10.1186/1471-2229-11-48
Thomas, B. J., Rothstein, R. J. (1989). Elevated recombination rates in transcriptionally active DNA. Cell 56, 619–630. doi: 10.1016/0092-8674(89)90584-9
Van Dijck, P., Mascorro-Gallardo, J. O., De Bus, M., Royackers, K., Iturriaga, G., Thevelein, J. M. (2002). Truncation of Arabidopsis thaliana and Selaginella lepidophylla trehalose-6-phosphate synthase (TPS) unlocks high catalytic activity and supports high trehalose levels upon expression in yeast. Biochem. J. 366, 63–71. doi: 10.1042/bj20020517
Vandesteene, L., Ramon, M., Le Roy, K., Van Dijck, P., Rolland, F. (2010). A single active trehalose-6-P synthase (TPS) and a family of putative regulatory TPS-like proteins in Arabidopsis. Mol. Plant 3, 406–419. doi: 10.1093/mp/ssp114
Vandesteene, L., Lopez-Galvis, L., Vanneste, K., Feil, R., Maere, S., Lammens, W., et al. (2012). Expansive evolution of the TREHALOSE-6-PHOSPHATE PHOSPHATASE gene family in Arabidopsis thaliana. Plant Physiol. 160, 884–896. doi: 10.1104/pp.112.201400
Vilaça, R., Mendes, V., Mendes, M. V., Carreto, L., Amorim, M. A., de Freitas, V., et al. (2012). Quercetin Protects Saccharomyces cerevisiae against Oxidative Stress by Inducing Trehalose Biosynthesis and the Cell Wall Integrity Pathway. PloS One 7 (9), e45494. doi: 10.1371/journal.pone.0045494
Vogel, G., Aeschbacher, R. A., Muller, J., Boller, T., Wiemken, A. (1998). Trehalose-6-phosphate phosphatases from Arabidopsis thaliana: identification by functional complementation of the yeast tps2 mutant. Plant J. 13, 673–683. doi: 10.1046/j.1365-313X.1998.00064.x
Vogel, G., Fiehn, O., Jean-Richard-dit-Bressel, L., Boller, T., Wiemken, A., Aeschbacher, R. A., et al. (2001). Trehalose metabolism in Arabidopsis: occurence of trehalose and molecular cloning and characterization of trehalose-6-phosphate synthase homologues. J. Exp. Bot. 52 (362), 1817–1826. doi: 10.1093/jexbot/52.362.1817
Wahl, V., Ponnu, J., Schlereth, A., Arrivault, S., Langenecker, T., Franke, A., et al. (2013). Regulation of flowering by trehalose-6-phosphate signaling in Arabidopsis thaliana. Science 339 (6120), 704–707. doi: 10.1126/science.1230406
Weise, A., Altmann, F., Rodriguez-Franco, M., Sjoberg, E. R., Bäumer, W., Launhardt, H., et al. (2007). High-level expression of secreted complex glycosylated recombinant human erythropoietin in the Physcomitrella ∆-fuct ∆-xyl-t mutant. Plant Biotechnol. J. 5, 389– 401. doi: 10.1111/j.1467-7652.2007.00248.x
Wharton, D. A. (2011). Anhydrobiosis: The Model Worm as a Model? Curr. Biol. 21 (15), R578–R579. doi: 10.1016/j.cub.2011.06.040
Wingler, A., Delatte, T. L., O'Hara, L. E., Primavesi, L. F., Jhurreea, D., Paul, M. J., et al. (2012). Trehalose-6-phosphate is required for the onset of leaf senescence associated with high carbon availability. Plant Physiol. 158, 1241–1251. doi: 10.1104/pp.111.191908
Yadav, U. P., Ivakov, A., Feil, R., Duan, G. Y., Walthe, D., Giavalisco, P., et al. (2014). The sucrose-trehalose 6-phosphate (Tre6P) nexus: specificity and mechanisms of sucrose signalling by Tre6P. J. Exp. Bot. 65, 1051–1068. doi: 10.1093/jxb/ert457
Zentella, R., Mascorro-Gallardo, J. O., Van Dijck, P., Folch-Mallol, J., Bonini, B., Van Vaeck, C., et al. (1999). A Selaginella lepidophylla trehalose-6-phosphate synthase complements growth and stress-tolerance defects in a yeast tps1 mutant. Plant Physiol. 119, 1473–1482. doi: 10.1104/pp.119.4.1473
Zhai, Z., Keereetaweep, J., Liu, H., Feil, R., Lunn, J. E., Shanklin, J. (2018). Trehalose 6-phosphate positively regulates fatty acid synthesis by stabilizing WRINKLED1. Plant Cell. 30 (10), 2616–2627. doi: 10.1105/tpc.18.00521
Keywords: Physcomitrella patens, trehalose metabolism, PpTPS1, PpTPS2, protonema, gametophytes, sugar signaling, plant hormones
Citation: Phan TLCHB, Delorge I, Avonce N and Van Dijck P (2020) Functional Characterization of Class I Trehalose Biosynthesis Genes in Physcomitrella patens. Front. Plant Sci. 10:1694. doi: 10.3389/fpls.2019.01694
Received: 16 September 2019; Accepted: 29 November 2019;
Published: 20 January 2020.
Edited by:
Stefan A. Rensing, University of Marburg, GermanyReviewed by:
Yuji Hiwatashi, Miyagi University, JapanCaspar Christian Cedric Chater, University of Sheffield, United Kingdom
Copyright © 2020 Phan, Delorge, Avonce and Van Dijck. This is an open-access article distributed under the terms of the Creative Commons Attribution License (CC BY). The use, distribution or reproduction in other forums is permitted, provided the original author(s) and the copyright owner(s) are credited and that the original publication in this journal is cited, in accordance with accepted academic practice. No use, distribution or reproduction is permitted which does not comply with these terms.
*Correspondence: *Correspondence:Nelson Avonce, bmVsc29uLmF2b25jZUB1YWVtLm14; Patrick Van Dijck, UGF0cmljay52YW5kaWpja0BrdWxldXZlbi52aWIuYmU=