- 1Department of Organismic Interactions, Max Planck Institute of Terrestrial Microbiology, Marburg, Germany
- 2Department of Life Sciences and Systems Biology, University of Turin, Turin, Italy
- 3Botanical Institute, Cluster of Excellence on Plant Sciences (CEPLAS), University of Cologne, Cologne, Germany
- 4BPMP, Univ Montpellier, CNRS, INRAE, Montpellier SupAgro, Montpellier, France
Loss-of-function alleles of MLO (Mildew Resistance Locus O) confer broad-spectrum resistance to foliar infections by powdery mildew pathogens. Like pathogens, microbes that establish mutually beneficial relationships with their plant hosts, trigger the induction of some defense responses. Initially, barley colonization by the root endophyte Serendipita indica (syn. Piriformospora indica) is associated with enhanced defense gene expression and the formation of papillae at sites of hyphal penetration attempts. This phenotype is reminiscent of mlo-conditioned immunity in barley leaf tissue and raises the question whether MLO plays a regulatory role in the establishment of beneficial interactions. Here we show that S. indica colonization was significantly reduced in plants carrying mlo mutations compared to wild type controls. The reduction in fungal biomass was associated with the enhanced formation of papillae. Moreover, epidermal cells of S. indica-treated mlo plants displayed an early accumulation of iron in the epidermal layer suggesting increased basal defense activation in the barley mutant background. Correspondingly, the induction of host cell death during later colonization stages was impaired in mlo colonized plants, highlighting the importance of the early biotrophic growth phase for S. indica root colonization. In contrast, the arbuscular mycorrhizal fungus Funneliformis mosseae displayed a similar colonization morphology on mutant and wild type plants. However, the frequency of mycorrhization and number of arbuscules was higher in mlo-5 mutants. These findings suggest that MLO differentially regulates root colonization by endophytic and AM fungi.
Introduction
Plants establish diverse beneficial interactions with fungi from different taxa. Root endophytes belonging to the order Sebacinales establish long-lasting beneficial relationships with a broad range of plant species (Weiss et al., 2016). Root colonization by members of this order results in enhanced growth (Varma et al., 1999; Waller et al., 2005; Serfling et al., 2007; Ghimire et al., 2009; Franken, 2012), improved tolerance to abiotic stress (Waller et al., 2005; Baltruschat et al., 2008; Sherameti et al., 2008; Ghimire and Craven, 2011), as well as increased resistance to pathogens (Stein et al., 2008; Waller et al., 2008; Lahrmann et al., 2015; Sarkar et al., 2019). In some cases nutrient status has been reported to play a role in the interaction of the model sebacinoid fungus Serendipita indica with some plant species (Shahollari et al., 2005; Sherameti et al., 2005; Nautiyal et al., 2010; Yadav et al., 2010; Kumar et al., 2012). However, S. indica colonization of Nicotiana attenuata and barley had no effect on host phosphorus (P) and nitrogen (N) content (Barazani et al., 2005; Achatz et al., 2010), suggesting that nutrient exchange is not central to the beneficial effects conferred by sebacinoid fungi. S. indica colonizes the rhizodermis and outer root cortex (Deshmukh et al., 2006; Jacobs et al., 2011; Weiss et al., 2016). Following an initial biotrophic growth phase, during which the fungal hyphae remain surrounded by a plant-derived membrane, S. indica transitions to cell death-associated colonization that does not result in host disease (Deshmukh et al., 2006; Jacobs et al., 2011; Zuccaro et al., 2011; Lahrmann and Zuccaro, 2012; Qiang et al., 2012; Lahrmann et al., 2013).
Like sebacinoid fungi, arbuscular mycorrhizae (AM) establish beneficial interactions with many plant species (Bonfante and Genre, 2010). AM are obligate biotrophs that rely on their plant hosts as carbon sources in exchange for soil nutrients including P and N (Parniske, 2008). Additionally, AM colonization results in increased plant biomass and confers enhanced resistance to stress and pathogen infection (Khaosaad et al., 2007; Liu J et al., 2007; Pozo and Azcon-Aguilar, 2007). Following the mutual recognition between plant and microbe, AM form specialized hyphae, called hyphopodia, that adhere to the root epidermal surface where penetration hyphae emerge. In the inner root cortex, intracellular hyphae then establish so-called arbuscules, which represent the active interface for nutrient exchange (Genre et al., 2008).
The successful establishment of AM symbioses is genetically controlled by the ancestral common symbiosis pathway (CSP). In legumes, this pathway is required for the establishment of AM as well as root nodule symbiosis with rhizobacteria (Kistner et al., 2005; Gutjahr, 2014; Svistoonoff et al., 2014). In contrast, S. indica colonization and development is independent of Lotus japonicus and Arabidopsis thaliana CSP genes, suggesting that independent host pathways control AM symbiosis and endophytism (Banhara et al., 2015). However, in both cases transient and weak activation of defense responses have been reported during the early phases of colonization that are effectively suppressed by the fungi as the symbioses progress (Harrison, 2005; Schäfer et al., 2009; Camehl et al., 2011; Jacobs et al., 2011). These early defense responses include the formation of papillae at sites of hyphal penetration attempts of S. indica on barley (Lahrmann and Zuccaro, 2012). Papillae are dome-shaped cell wall appositions that play a vital role in resistance to plant pathogens (Hückelhoven, 2005; Hückelhoven, 2007; Albersheim et al., 2011; Hückelhoven, 2014). They generally consist of layers of callose, cellulose, arabinoxylan and phenolyic compounds (Chowdhury et al., 2014; Hückelhoven, 2014). Additionally, papillae contain reactive oxygen species (hydrogen peroxide H2O2) and in barley their formation appears to be dependent on iron (Fe3+) accumulation in the apoplast (Thordal-Christensen et al., 1997; Hückelhoven et al., 1999; Liu G et al., 2007). Depending on their size, composition and the degree of cross-linking of their constituent parts, papillae can be more or less efficient in halting penetration (Aist and Israel, 1977; Israel et al., 1980; von Röpenack et al., 1998; Asaad et al., 2004; Chowdhury et al., 2014; Hückelhoven, 2014).
In barley, natural as well as chemically induced mutant lines carrying recessive mlo (MILDEW RESISTANCE LOCUS O) alleles display broad-spectrum resistance to the obligate biotrophic pathogen Blumeria graminis f. sp. hordei (Bgh), causal agent of the foliar powdery mildew disease (Büschges et al., 1997), and have been successfully employed in agriculture since the late 1970s (Jørgensen, 1992; Lyngkjær et al., 2000; Kusch and Panstruga, 2017). Especially barley spring varieties that are now largely grown in central Europe are resistant to Bgh following the introgression of mlo alleles (Jørgensen, 1992; McGrann et al., 2014; Kusch and Panstruga, 2017). Compared to wild type susceptible cultivars, these lines display faster formation of larger papillae upon pathogen attack (Jørgensen, 1992; Lyngkjær et al., 2000; Chowdhury et al., 2014). Similar mutations in orthologous genes of wheat, tomato, pea, A. thaliana, and many other plant species have since confirmed the importance of mlo for resistance to various species of powdery mildew (Elliott et al., 2002; Consonni et al., 2006; Bai et al., 2008; Humphry et al., 2011; Wang et al., 2014; Kusch and Panstruga, 2017). In contrast, some hemibiotrophic and necrotrophic pathogens show enhanced infection on mlo mutant plants possibly profiting from the spontaneous induction of leaf cell death (Jarosch et al., 1999; Kumar et al., 2001; McGrann et al., 2014). The contribution of MLO to host resistance against root-colonizing microbes is less well understood. Recent evidence suggests that the mlo genetic background does not affect barley root infection by the oomycete pathogen Phytophthora palmivora (Le Fevre et al., 2016). Similarly, MLO does not seem to play a role in the establishment of the beneficial relationships between pea and the rhizobacterium Rhizobium leguminosarum bv. viciae or the AM fungus Rhizophagus irregularis (syn. Glomus intraradices) (Humphry et al., 2011). However, transcriptional analyses showed an upregulation of the Lotus japonicus MLO1-like (chr1.CM0150.1) gene in cortical cells containing arbuscules of Gigaspora margarita (Guether et al., 2009) suggesting that MLO may play a regulatory role in AM colonization.
In this study, we used the endophyte S. indica and the AM fungus F. mosseae, both of which have intracellular lifestyles, to investigate the role of MLO in barley root symbioses. Comparative colonization analyses showed differential regulation by MLO during endophytism and mycorrhization. The decreased colonization by S. indica coincided with enhanced defense responses and papillae formation, highlighting the importance of the biotrophic growth phase for the establishment of the long-term beneficial relationship between S. indica and its plant hosts.
Materials and Methods
Plant Material and S. indica Growth Conditions
Seeds of mlo-3, -4, and -5 mutant lines backcrossed to barley cv. Ingrid (Peterhänsel et al., 1997; Jarosch et al., 2003) were kindly provided by Ralph Panstruga. Wild type (WT) barley (Hordeum vulgare L. cv. Ingrid) and mutant seeds were surface-sterilized by washing in 70% ethanol for 1–5 min, rinsing in sterile distilled water and soaking in 4%–12% sodium hypochlorite for 1–1.5 h. Seeds were then thoroughly washed in sterile distilled water, placed onto sterile wet filter paper and kept in the dark at room temperature for 3–5 days to allow germination. S. indica Sav. Verma, Aj. Varma, Rexer, G. Kost & P. Franken (DSM11827, Deutsche Sammlung von Mikroorganismen und Zellkulturen, Braunschweig, Germany) was grown in liquid complex medium (CM) (Pham et al., 2004) at 130 rpm or on CM medium supplemented with 1.5% agar at 28°C.
Barley Inoculation With S. indica
Three-day-old seedlings were placed into sterile jars containing 1/10 plant nutrition medium (PNM) (Basiewicz et al., 2012). For inoculation, S. indica chlamydospores were collected from CM agar plates in 0.1% Tween20-water, filtered through Miracloth and pelleted by centrifugation at 3,500 g for 5 min. Spores were washed two more times in 0.1% Tween20-water and then re-suspended to a final concentration of 5x105 spores/ml. Mock treatment consisted of 0.1% Tween20-water only. Three milliliters of spore suspension was added onto roots of barley seedlings. Jars were transferred to a growth chamber with a 16 h/8 h day/night (light intensity of 108 µmol/m2/s) cycle at 22°C/18°C and 60% humidity. All experiments were prepared with three to four biological replicates consisting of pooled material from 4 plants/jar, and two to three independent replicate experiments.
Quantification of S. indica Colonization
Mock-treated and S. indica-colonized roots were harvested at 3, 5, 7, and 10 days post inoculation. Roots were washed in water and sections of the first 3 cm below the seed were cut and frozen in liquid nitrogen. Genomic DNA from 200 mg of freshly ground material was isolated according to (Doyle and Doyle, 1987). To remove contaminating RNA, samples were treated with 1 µL 10 mg/mL RNaseA (Thermo Fisher Scientific, Schwerte, Germany) and incubated at 37°C for 20 min. Quantitative PCR was performed with 10 ng gDNA template and primers targeting the S. indica TEF (SiTEF) the barley ubiquitin (HvUBI) genes (see Supplementary Table 1) in 10 µl SYBR green Supermix (BioRad, Munich, Germany) using the following amplification protocol: initial denaturation for 16 min at 95°C, followed by 40 cycles of 15 s at 95°C, 20 s at 59°C, and 30s at 72°C, and a melt curve analysis. The relative amount of fungal vs. plant gDNA was calculated according to the 2-ΔCt method (Schmittgen and Livak, 2008).
Vacuolar Processing Enzyme (VPE) Activity Assay
Mock-treated and S. indica-colonized roots of WT and mutant plants were harvested at 10 dpi. Vacuolar processing enzyme (VPE) activity was measured as described previously (Lahrmann et al., 2013). Briefly, roots were washed in water and sections of the first 4 cm below the seed were cut and frozen in liquid nitrogen. Extracts were prepared from 100 mg freshly ground root material ground in liquid nitrogen with 1 ml extraction buffer [10 mM sodium acetate pH 5.5, 100 mM NaCl, 1 mM EDTA, 2mM dithiothreitol (DTT) and 1mM phenylmethylsulfonyl fluoride (PMSF)]. Plant debris was pelleted by centrifugation at max speed and 4°C for 10 min. To measure VPE activity, 100 µM of the fluorescent VPE-specific substrate Ac-ESEN-MCA (Peptide Institute Inc., Osaka, Japan) was added to 100 µl of root extract supernatants aliquoted into a 96-well plate. Fluorescence intensities were measured in a TECAN Infinite microplate reader (TECAN, Männerdorf, Switzerland) with 360 and 465 nm excitation and emission wavelengths, respectively, at 10 min intervals for 1 h. Buffer with and without substrate was used as control.
Staining and Microscopy of S. indica-Inoculated Barley Root Sections
Confocal pictures were taken using a TCS-SP5 confocal microscope (Leica, Bensheim, Germany). Colonized root tissue of WT and mutant plants was collected at indicated time points, boiled for 2 min in 10% potassium hydroxide, washed three times in deionized water, and three additional times in 1x PBS (pH 7.4) for 30 min. Roots were stained by infiltrating colorants four times for 4 min at 260 mbar with 1 min atmospheric pressure breaks. To visualize fungal structures, roots were infiltrated with 10 μg/mL fluorescent Wheat Germ Agglutinin (WGA) AF488 (Invitrogen, Thermo Fisher Scientific, Schwerte, Germany) in 1x PBS. Papillae were visualized following infiltration of 10 μg/mL fluorescent concanavalin A (ConA) AF633 (Invitrogen, Thermo Fisher Scientific, Schwerte, Germany) in 1x PBS. For iron staining, samples were fixed, embedded in resin, sectioned and then stained with the Perls/DAB procedure, as described previously (Roschzttardtz et al., 2009). This method is a sensitive histological test for iron accumulation in plants (Roschzttardtz et al., 2013).
Barley Inoculation With the Mycorrhizal Fungus F. mosseae
Following germination on wet filter paper, seedlings were kept 3 days in continuous light (light intensity of 80 µmol/m2/s). For each experiment, four seedlings of barley WT and mlo-5 were placed into separate pots filled with a 7:3 mix of sterile quartz sand/granular F. mosseae inoculum (v/v). The F. mosseae inoculum was composed of newly formed spores and Sorghum root pieces already colonized by F. mosseae. The inoculum was purchased from MycAgro Lab (Dijon, France) and contained a minimum of 10 active propagules/g. Plants were transferred to a growth chamber with a 14 h/10 h day/night cycle at 23°C/21°C. After 2 months of cultivation, one half of the root apparatus was sampled from each plant and stained with Cotton Blue (0.1% in lactic acid) to evaluate the intraradical colonization. The other half was treated with WGA conjugated with the fluorescent probe fluorescein isothiocyanate (FITC) to analyze fungal penetration in the root epidermal cells by confocal microscopy. The experiment was performed twice.
Quantification of the AM Colonization
For each plant at least 1 m of root tissue was observed under an optical microscope to evaluate the degree of mycorrhizal colonization (Trouvelot et al., 1986). Four parameters were considered: F% (frequency of mycorrhization) reporting the percentage of segments showing internal colonization, M% (intensity of mycorrhization in the root cortex) indicating the average percentage of colonized root segments, a% (percentage of arbuscules in the infected areas) quantifying the average presence of arbuscules within the infected areas, A% (percentage of arbuscules in the entire root apparatus) quantifying the presence of arbuscules in the whole root system.
Details of the AM Penetration
To analyze the fungal penetration through the root epidermis, fifty 0.5 cm root segments were fixed in 4% paraformaldehyde in 0.05 M phosphate buffer pH 7.2, for 4 h at room temperature. Fixed segments were embedded in 8% agarose (Agarose type II-A, Sigma-Aldrich, Taufkirchen, Germany) and cut into 50 µm sections with a vibratome. The sections were incubated for 5 min in 1:30 commercial bleach/phosphate buffer (v/v), carefully rinsed with buffer and incubated for 2 h at room temperature in 1 mg/ml WGA-FITC. Sections were then observed with a Leica TCS SP2 confocal microscope equipped with an Ar/HeNe laser with 543 nm excitation and 580–650 nm emission wavelengths.
Statistical Analyses
Statistically significant effects of plant genotypes on VPE activity in colonized and mock-treated root tissue were determined with ANOVA using R (v3.3.2). Significant differences between treatments were determined with Tukey’s post hoc test from the Stats package (Miller, 1981; Yandell, 1997). Letters displaying similarities and differences were extracted using the multcompView package (v0.1-7) (Donoghue, 2004; Piepho, 2004). Statistically significant effects of plant genotypes on fungal colonization and activation of defense responses (papillae size and number) were determined using the Welch Two Sample t test from the Stats package in R. Asterisks indicate these differences.
Results and Discussion
MLO Loss-Of-Function Mutations Result in Reduced Barley Root Colonization by S. indica
Removal of host genes that are required by invading pathogens for plant colonization, termed susceptibility genes, has been shown to provide disease resistance (van Schie and Takken, 2014). Several natural and mutagen-induced changes in the barley MLO locus confer broad-spectrum resistance to the powdery mildew pathogen Blumeria graminis f. sp. hordei (Jørgensen, 1992; Büschges et al., 1997; Lyngkjær et al., 2000; Kusch and Panstruga, 2017). Similarly, TALEN- and CRISPR-Cas9-introduced targeted mutations in three MLO homeoalleles of bread wheat showed their requirement for resistance to wheat powdery mildew (Wang et al., 2014). However, the recessive loss-of-function mlo alleles in barley resulted in increased susceptibility to some other fungal pathogens (Jarosch et al., 1999; McGrann et al., 2014). To investigate the role of MLO in the establishment of the long-term beneficial relationship between barley and the root endophyte Serendipita indica, we used the mlo-5 allele backcrossed (BC) into barley cv. Ingrid (hereafter referred to as mlo-5), which carries a point mutation in the start codon and is a predicted null allele (Büschges et al., 1997). Mutant and WT plants were inoculated with S. indica spores suspended in Tween20-water or Tween20-water alone as mock treatment. To assess the effect of the mlo-5 mutation on S. indica colonization, we quantified the relative abundance of S. indica gDNA as a proxy for fungal biomass in roots of WT barley cv. Ingrid and mlo-5 plants by quantitative PCR at 3, 5, and 7 days post inoculation (dpi), representing early and late biotrophic interaction stages and the initial cell death-associated phase, respectively. We observed a significant reduction in fungal biomass in mutant compared to control plants from early through late colonization stages (Figure 1A). To confirm this phenotype, we also quantified S. indica biomass in roots of colonized mlo-3 and mlo-4 mutant lines, which display frame shift mutations in exon 11 and 4, respectively, and likewise are predicted null alleles. Similar to mlo-5, mlo-3 and -4 were less colonized by S. indica compared to WT control plants (Supplementary Figure 1), suggesting that MLO is required for barley root colonization by S. indica. This is in contrast to earlier findings where mlo triple mutants of A. thaliana displayed similar levels of S. indica colonization as wild type plants (Acevedo-Garcia et al., 2017). In A. thaliana there are 15 members of the MLO family. It could be that in A. thaliana roots other MLO members play a role in fungal accommodation than the three mutated genes. Alternatively, it has previously been shown that S. indica displays different colonization strategies on barley and A. thaliana host plants (Lahrmann et al., 2013). It is, therefore, conceivable that MLO-controlled defense does not play an important role in S. indica accommodation in A. thaliana roots.
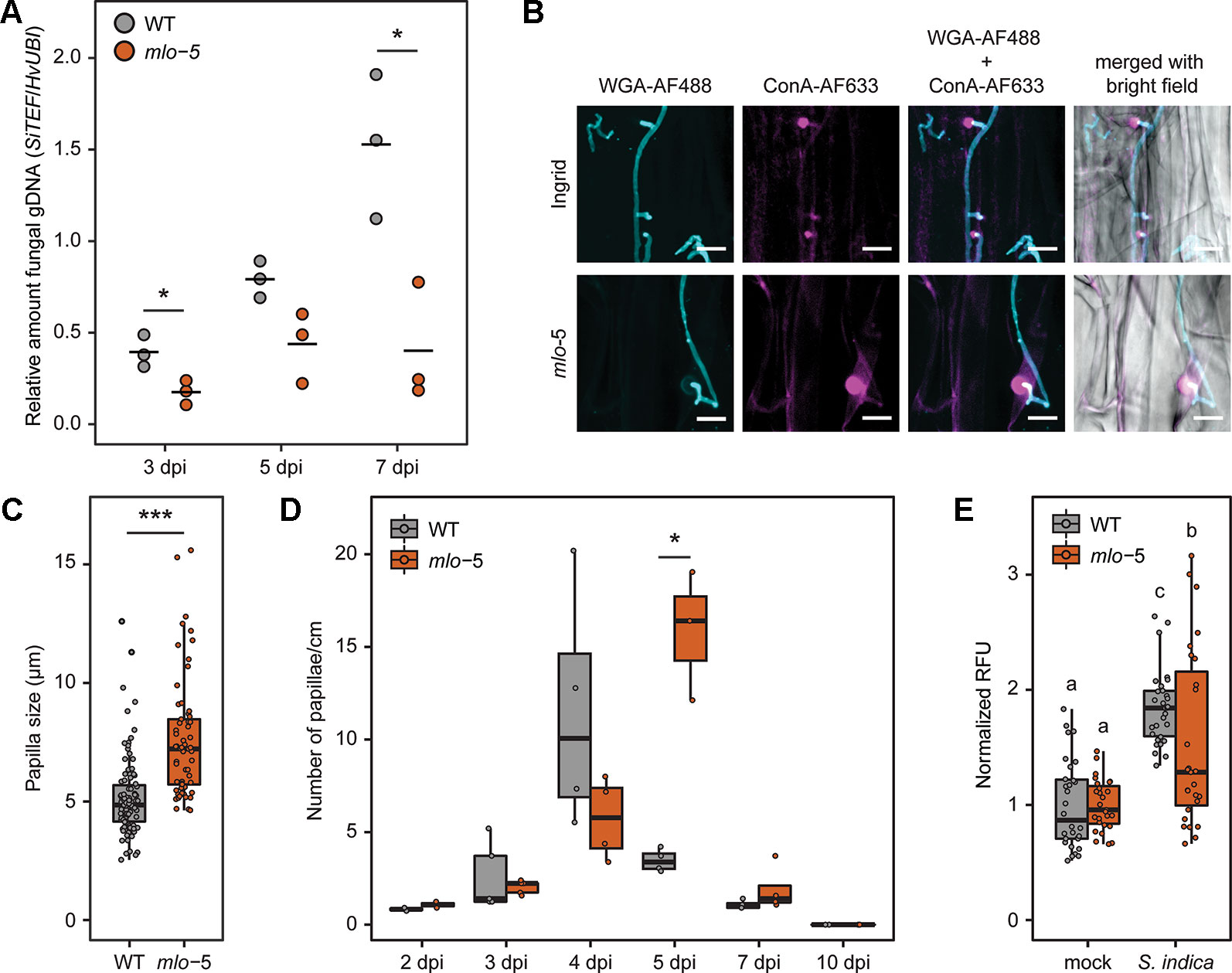
Figure 1 Reduced S. indica colonization of barley mlo-5 coincides with enhanced root defense. Three-day-old barley seedlings were inoculated with a S. indica chlamydospore suspension at a final concentration of 5x105 spores/ml in Tween20-water or Tween20-water alone as mock treatment. (A) At 3, 5, and 7 days post inoculation (dpi) seedlings were removed from jars and gDNA was extracted from inoculated root sections as described in the Materials and Methods. Fungal colonization in each biological replicate was confirmed by quantitative PCR (n = 3). Statistically significant differences in the relative abundance of fungal gDNA during colonization of wild type (WT) and mutant mlo-5 plants were determined with Welch two sample t tests (*p < 0.5). (B) Roots of inoculated WT and mlo-5 plants were collected and stained with 10 μg/ml Wheat Germ Agglutinin (WGA-AF488, cyan) and 10 μg/ml concanavalin A (ConA-AF633, magenta) for visualization of fungal structures and papillae, respectively. Confocal microscopy shows extraradical growth of S. indica and hyphal penetration attempts of WT and mlo-5 root tissue. At these sites, the barley host responds with papillae formation. (C) Sizes of papillae formed in colonized WT (n=95) and mlo-5 (n = 61) roots were determined based on confocal microscopy pictures taken at 3, 4, 5 and 7 dpi. The statistically significant difference in papilla size between wild type (WT) and mutant mlo-5 plants throughout colonization was determined with the Welch two sample t test (***p < 0.001). (D) The number of papillae formed in colonized WT and mlo-5 roots was quantified based on confocal microscopy pictures taken at 2, 3, 4, 5, 7, and 10 dpi (n = 2-4). Statistically significant differences in papilla quantity between barley genotypes were determined with Welch two sample t tests (*p < 0.5). (E) Mock-treated and S. indica-colonized roots of WT and mutant plants were harvested at 10 dpi. Root cell death was quantified by measuring vacuolar processing enzyme (VPE) activity-dependent fluorescence (Qiang et al., 2012). Letters represent statistically significant differences in VPE activity according to two-way ANOVA (F(1,110) = 7.077, p < 0.01) and Tukey’s post hoc test.
S. indica-Colonized mlo-5 Mutant Plants Display Enhanced Papilla Formation During Biotrophic Growth
Enhanced barley resistance to powdery mildew in mlo mutants has been associated with enhanced cell wall apposition, or papillae, formation (Skou et al., 1984; Bayles et al., 1990; Wolter et al., 1993). Due to the reduction in S. indica colonization in mlo plants, we compared papillae formation in inoculated mlo-5 mutant and WT roots. To visualize fungal structures and papillae, colonized root tissue was stained with the chitin-specific wheat germ agglutinin (WGA-AF488) and α-mannopyranosyl-/α-glucopyanosyl- specific concanavalin A (ConA-AF633) for confocal microscopy, respectively. While both barley genotypes responded with papillae formation to S. indica hyphal penetration attempts, these cell wall appositions were significantly bigger in mlo-5 plants compared to the controls (Figures 1B, C). The number of papillae increased over time both in WT and mlo-5 plants but was significantly higher at 5 dpi in mutant plants (Figure 1D). These phenotypes are reminiscent of mlo-mediated resistance to Bgh in leaves, where the fungus is arrested at the prehaustorial stage when papillae formation occurs in epidermal cells (Skou et al., 1984), highlighting an interesting parallel between mlo-dependent leaf and root responses to fungal colonization.
At early stages of WT barley root colonization by S. indica, transient papillae development has previously been observed at penetration sites and coincides with the activation of weak barley defense responses at the transcriptional level during the fungus’ biotrophic growth phase (Schäfer et al., 2009; Zuccaro et al., 2011; Lahrmann and Zuccaro, 2012). However, most of the papillae formed during the biotrophic colonization of the rhizodermis do not prevent S. indica hyphal penetration and disappear once the fungus reaches the root cortex (Zuccaro et al., 2011; Lahrmann and Zuccaro, 2012). The role of papillae in resistance against host cell penetration has been discussed for decades (Zeyen et al., 2002). Recent evidence suggests that so-called effective papillae [cell wall appositions that are formed in response to a local colonization attempt and that cannot be penetrated; (Hückelhoven, 2014)] contain higher quantities of callose, arabinoxylan and cellulose than ineffective papillae in barley (Chowdhury et al., 2014). Noncovalent bonds between arabinoxylan and cellulose have been proposed to maintain the barley cell wall, potentially forming a network of highly cross-linked polysaccharides (McNeil et al., 1975; Chowdhury et al., 2014). Phenolic compounds present in papillae could further enhance the degree of cross-linking giving rise to a tight structure resistant to mechanical penetration. The resistance to mechanical force, however, is unlikely to play a role in the interaction between S. indica and barley, since S. indica does not form appressorium-like structures. The genome of S. indica, like the genome of its orchid mycorrhizal relative S. vermifera, harbors a large number of genes encoding hydrolytic enzymes comparable to genomes of hemibiotrophic and necrotrophic pathogens, as well as several white rot saprotrophs (Lahrmann et al., 2013; Lahrmann et al., 2015). The up-regulation of genes encoding putative cell wall degrading enzymes during the pre-penetration stage (Zuccaro et al., 2011) suggests that S. indica uses hydrolytic enzymes for host cell penetration. The phenotypic alteration of papillae formed in mlo-5 plant roots suggests a change in composition similar to that proposed for papillae in leaves of resistant mlo barley plants. Considering that S. indica colonization is efficiently arrested in mlo-5 mutant plants, we hypothesize that the arsenal of hydrolytic enzymes and effector proteins produced by S. indica is not sufficient to overcome altered defenses in mlo-5 plants. Additionally, accumulation of toxic compounds at the penetration site in the mlo-5 background could decrease the capability of S. indica to penetrate the host cell.
The early arrest of S. indica colonization in plants carrying the mlo-5 allele indicates that the biotrophic phase plays an important role for the establishment of the beneficial barley-endophyte interaction. To test whether a reduction in biotrophic colonization would have an effect on fungal development, we assessed the induction of host root cell death, which is a hallmark of the S. indica-plant interaction during later colonization stages, using a well-established vacuolar processing enzyme (VPE) activity assay (Deshmukh et al., 2006; Qiang et al., 2012; Lahrmann et al., 2013). At 10 dpi, root sections of colonized WT and mlo-5 plants were collected for total protein extraction. Protein extracts were then incubated with the fluorescent VPE-specific substrate Ac-ESEN-MCA to measure VPE-mediated proteolytic cleavage of MCA. We observed a significant reduction in VPE activity in S. indica-treated mlo-5 plants compared to WT controls (Figure 1E) suggesting a decrease in host cell death. This finding is in agreement with lower colonization levels in mlo mutant plants (Figure 1A, Supplementary Figure 1), Thus, the recessive mlo allele effect on the early biotrophic fungal growth also affects the cell death phase.
Reduced S. indica Colonization Correlates With an Early Accumulation of Iron At the Epidermal Layer of mlo-5 Mutant Plants
The production of reactive oxygen species (ROS) represents a key factor in pathogen resistance, and has been reported to play a role in a number of beneficial associations (Enkerli et al., 1997; Hückelhoven et al., 1999; Fenster and Hause, 2005; Zuccaro et al., 2011; Lahrmann and Zuccaro, 2012). In addition to their direct antimicrobial effects, ROS have been implicated in the fortification of papillae and are emerging as signaling components during plant immunity (Thordal-Christensen et al., 1997; Torres et al., 2006; Baxter et al., 2014).
In cereals, the extracellular production of H2O2 and the resulting oxidative burst in response to biotic stress are dependent on the accumulation of iron (Liu G et al., 2007). To visualize iron accumulation, root tissue of infected and mock-treated plants was stained with Perls/DAB. Consistent with our observations on papillae formation in mlo-5 mutant plants, iron accumulation was apparent at 5 dpi in S. indica-colonized mutant plants (Figure 2A), whereas iron depositions started to appear at 6 dpi in WT plants (Figure 2B). Iron accumulated at the cell periphery throughout the rhizodermal cell layer, suggesting a systemic reaction of this layer. In leaves it was shown that accumulation of Fe3+ occurs specifically around the cell wall appositions just below the site of Bgh penetration attempts, where it facilitates H2O2 production (Greenshields et al., 2007; Liu G et al., 2007), suggesting that the mechanisms underlying iron accumulation and its role in ROS generation may be different in the rhizodermal cell layer. Together, these findings indicate that defense responses are enhanced and occur earlier in the mlo-5 mutant resulting in reduced S. indica colonization.
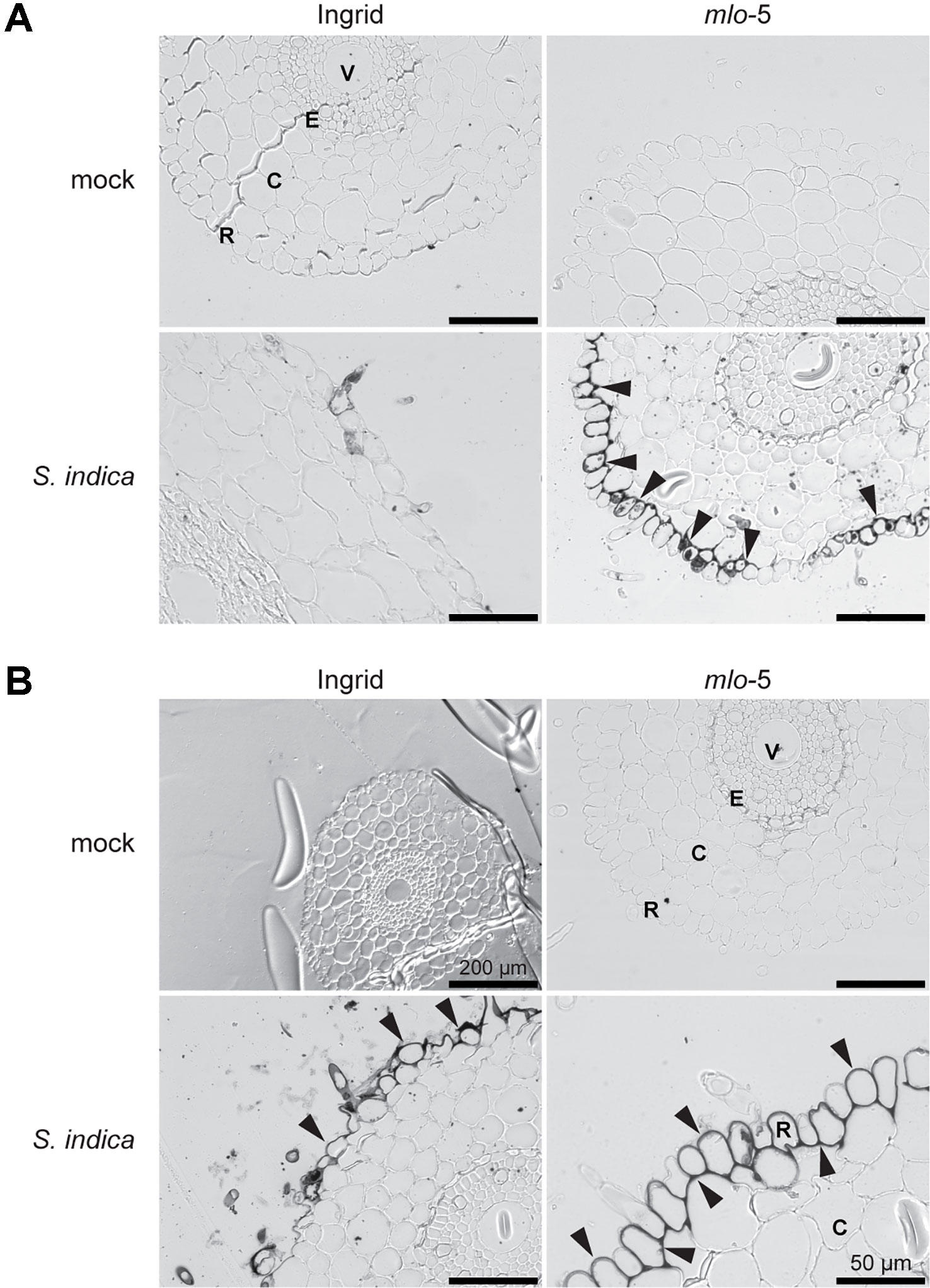
Figure 2 Early accumulation of iron in the epidermal cells of the Ingrid mlo-5 mutant upon S. indica colonization. Light microscopic pictures show root sections stained with Perls/DAB at 5 (A) and 6 dpi (B). Black precipitates, indicative of iron accumulation, appear around root epidermal cells in mlo-5 at 5 dpi, whereas iron accumulation in WT plants is only visible after 6 days of S. indica colonization (black arrowheads). Size bar = 100 µm unless otherwise indicated. R, rhizodermis; C, cortex; E, endodermis; V, vasculature.
Mlo Loss-Of-Function Mutations Result in Enhanced Mycorrhization
Like S. indica, F. mosseae has an intracellular lifestyle. Transcriptome studies comparing mycorrhizal to non-mycorrhizal conditions in Lotus japonicus suggested that MLO might play a regulatory role in AM colonization (Guether et al., 2009). To test this hypothesis, WT and mlo-5 barley plants were inoculated with F. mosseae and the rate of AM colonization was determined after 2 months of co-cultivation. Microscopically, we did not observe evident differences in the mycorrhizal phenotype. The extraradical mycelia developed in both plant genotypes (Figure 3A, upper panel) and the hyphopodia (Figure 3A, middle panel) consisted of similarly swollen hyphae at places where hyphae were in direct contact with the plant epidermal cell. Moreover, arbuscules were produced in cortical cells with a typical branched appearance in colonized WT and mlo-5 mutant plants (Figure 3A, lower panel). These findings indicate that MLO is not essential for the establishment of AM symbiosis and cannot be listed as one of the genes of the AM symbiosis signaling module that are required for the perception of AM-derived signaling molecules (Vigneron et al., 2018). To analyze the first step of the colonization (i.e. the penetration of the epidermal cells) in greater detail, we stained vibratome sections of colonized mlo-5 root tissue with WGA-FITC. Confocal microscopy of stained sections revealed that F. mosseae follows an intracellular penetration mechanism in the mutant background (Supplementary Figure 2), as has been described for G. margarita on Medicago truncatula roots (Genre et al., 2005; Genre et al., 2008). Similar to G. margarita, F. mosseae hyphae proliferating from the hyphopodium directly cross the wall of the epidermal cells to which the hyphopodium is adhering (Supplementary Figure 2).
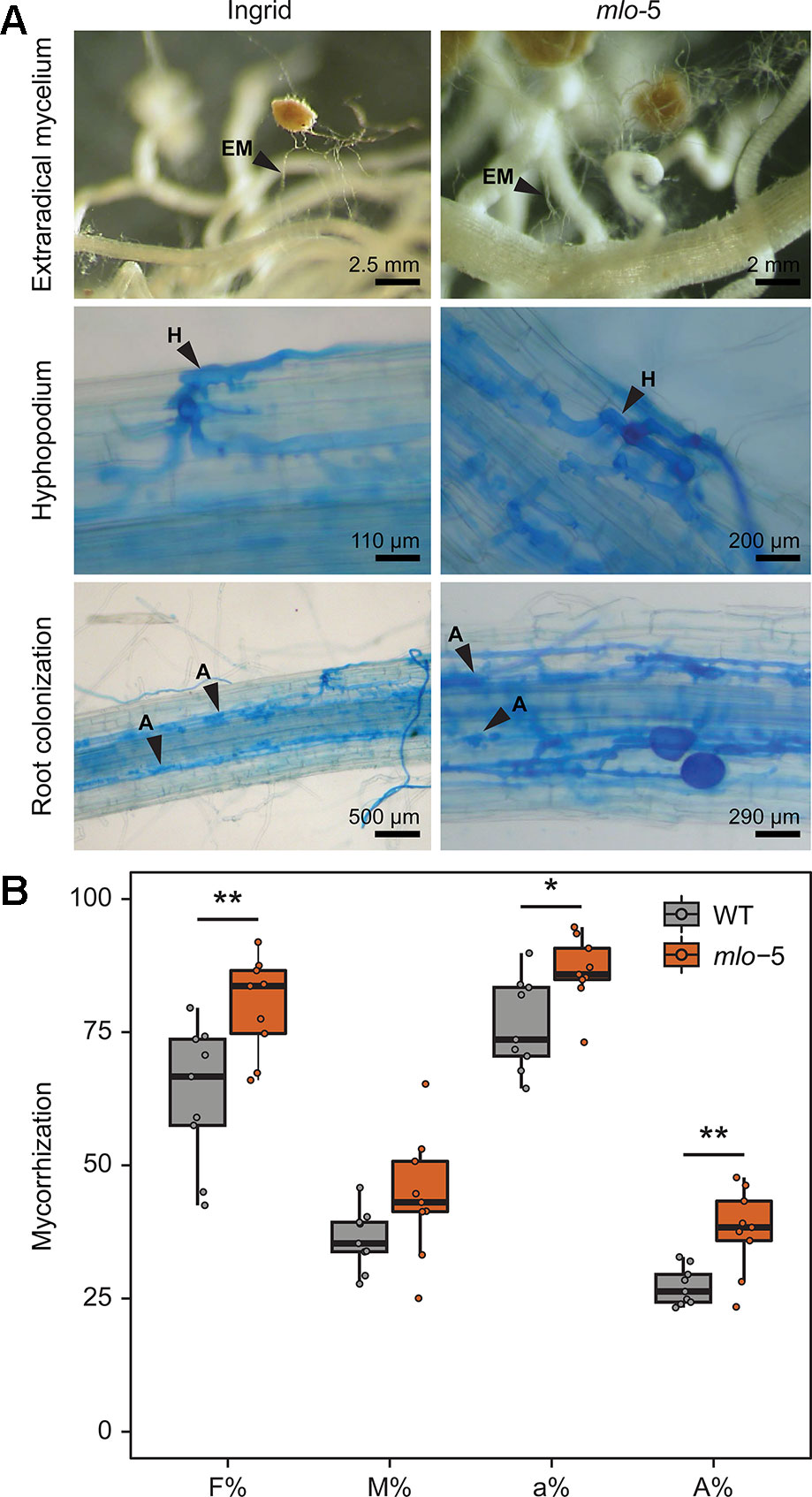
Figure 3 The barley mlo-5 allele promotes mycorrhization. Three-day-old seedlings were inoculated with F. mosseae inoculum with ≥10 active propagules/g mixed into the soil. After 2 months of cultivation, intraradical colonization was assessed by light microscopy of Cotton blue-stained root tissue in WT and mlo-5 plants (A). Black arrowheads indicate extraradical mycelia (EM; upper panel), hyphopodia (H; middle panel), and arbuscules (A; lower panel). (B) Mycorrhization was quantified using root tissue stained with fluorescein isothiocyanate-conjugated Wheat Germ Agglutinin (WGA-FITC) following confocal microscopy (n = 2). F% reports the percentage of segments showing internal colonization, M% indicates the average percent colonization of root segments, a% quantifies the average presence of arbuscules within the infected areas, A% quantifies the presence of arbuscules in the whole root system. Statistically significant differences in mycorrhization between barley genotypes were determined with Welch two sample t tests (*p < 0.5, **p < 0.01).
We then assessed the success of F. mosseae colonization by quantifying the frequency of mycorrhization (F%), the intensity of mycorrhization in the root cortex (M%), the percentage of arbuscules in infected areas (a%), and the presence of arbuscules in the entire root system (A%). In our experiments, F%, a%, and A% were higher in mlo-5 mutants compared to WT control plants, while the intensity of mycorrhization did not seem to be affected by the plant phenotype (Figure 3B). This is in contrast to the results presented by Ruiz-Lozano and colleagues (1999) who described a negative effect of the mlo-5 mutation on F. mosseae colonization at 6 weeks. This discrepancy is likely due to differences in inoculum efficiency and the large difference in root material analyzed here (1 m/plant) compared to the earlier study (30 cm/3 plants), or in the time points analyzed. We cannot exclude that colonization of the mlo-5 mutant plants would be negatively affected at earlier stages, but our data indicate that the absence of this gene is not negatively affecting the symbiosis in the long run. Our observations suggest that MLO may be required for optimal AM colonization levels and could be grouped with other so-called downstream genes, which are involved in the re-organization of the plant host cell and facilitate nutrient exchange (Vigneron et al., 2018). While mutations in many of these downstream genes lead to a decrease in colonization success (Wang et al., 2019), the mlo-5 allele enhances host susceptibility. MLO genes encode plant-specific proteins with seven membrane-spanning domains (Büschges et al., 1997; Devoto et al., 1999; Elliott et al., 2005; Kusch et al., 2016). Despite considerable effort, our knowledge on the biochemical functions of this group of proteins remains limited. However, it has been demonstrated that mlo-mediated resistance against Bgh relies on actin cytoskeleton function in epidermal cells (Miklis et al., 2007). Similarly, the cytoskeleton is likely involved in the biogenesis of the perifungal membrane, which is an extension of the host plasmalemma surrounding the growing AM intracellular hyphae (Genre and Bonfante, 2002; Genre et al., 2012). Thus, MLO may exert control over the number of produced arbuscules through the regulation of cytoskeletal assembly.
Conclusions
In this study, we show that MLO differentially regulates the establishment of beneficial symbioses with the endophyte S. indica and the AM fungus F. mosseae in barley. During WT colonization by S. indica and F. mosseae, non-specific defense responses are largely repressed. In contrast, inoculation of mlo-5 mutant plants with S. indica results in an early activation of strong defense responses, such as accumulation of iron and papillae formation, limiting S. indica colonization at the epidermal layer during biotrophic growth and at the onset of the cell death-associated phase, while F. mosseae mycorrhization is enhanced in cortex cells at a late colonization stage. Based on these findings, we conclude that the regulatory role of MLO may be cell type specific. This hypothesis is in accordance with the recent finding that the mlo-5 mutation diminishes P. palmivora infection only in young leaf tissue (Le Fevre et al., 2016). Moreover, there seems to be a difference in mlo contribution to resistance between monocot and dicot plant species since mlo barley and A. thaliana mutants display contrasting phenotypes during the interaction with S. indica. One explanation could be the prominent role of iron (Fe3+)-mediated oxidative (H2O2) cross-linking of cell wall appositions in barley and other cereals, which does not occur during the A. thaliana-S. indica interaction (Greenshields et al., 2007; Liu G et al., 2007; Lahrmann et al., 2013). Additionally, the results presented here corroborate the pleiotropic effects of mlo in various plant-microbe interactions. Other genes have been shown to display variable regulative roles depending on the colonization strategy of the microbe. For example, in M. truncatula the gene Nod Factor Perception (MtNFP) is essential for the establishment of N-fixing symbiosis, and, as a consequence Mtnfp mutants cannot establish the beneficial interaction (Rey et al., 2013). However, Mtnfp mutant lines are more susceptible to pathogens including Aphanomyces euteiches and Colletotrichum trifolii, indicating that the regulation of plant-microbe symbioses is often dependent on the interacting microbe.
Data Availability Statement
All datasets generated for this study are included in the article/Supplementary Material.
Author Contributions
MH, MN, PB, and AZ conceived and designed experiments. MH, MN, SM, and CG carried out experiments and analyzed the data. MN, HR, PB, and AZ wrote the manuscript.
Funding
AZ acknowledges support from the Max-Planck-Gesellschaft and the Cluster of Excellence on Plant Sciences (CEPLAS) under Germany’s Excellence Strategy—EXC 2048/1—Project ID: 390686111. Research in Torino was supported by the project 60% (local project of University of Torino) to PB.
Conflict of Interest
The authors declare that the research was conducted in the absence of any commercial or financial relationships that could be construed as a potential conflict of interest.
The reviewer [RP] declared providing the plant material for this research and confirms the absence of any other collaboration with the authors to the handling editor.
Acknowledgments
The authors thank Ralph Panstruga for kindly providing seeds of BC Ingrid mlo allele genotypes.
Supplementary Material
The Supplementary Material for this article can be found online at: https://www.frontiersin.org/articles/10.3389/fpls.2019.01678/full#supplementary-material
References
Acevedo-Garcia, J., Gruner, K., Reinstädler, A., Kemen, A., Kemen, E., Cao, L., et al. (2017). The powdery mildew-resistant Arabidopsis mlo2 mlo6 mlo12 triple mutant displays altered infection phenotypes with diverse types of phytopathogens. Sci. Rep. 7, 9319. doi: 10.1038/s41598-017-07188-7
Achatz, B., Kogel, K.-H., Franken, P., Waller, F. (2010). Piriformospora indica mycorrhization increases grain yield by accelerating early development of barley plants. Plant Signal Behav. 5, 1685–1687. doi: 10.4161/psb.5.12.14112
Aist, J. R., Israel, H. W. (1977). Papilla formation - timing and significance during penetration of barley coleoptiles by Erysiphe graminis hordei. Physiol. Plant Pathol. 67, 455–461. doi: 10.1016/0048-4059(77)90003-0
Albersheim, P., Darvill, A. G., Roberts, K. A., Sederoff, R., Staehlin, A. (2011). Cell walls and plant-microbe interactions (New York, NY, USA: Garland Science, Taylor & Francis).
Asaad, N., den Otter, M. J., Engberts, J. B. (2004). Aqueous solutions that model the cytosol: studies on polarity, chemical reactivity and enzyme kinetics. Org. Biomol. Chem. 2, 1404–1412. doi: 10.1039/b402886d
Büschges, R., Hollricher, K., Panstruga, R., Simons, G., Wolter, M., Frijters, A., et al. (1997). The barley Mlo gene: a novel control element of plant pathogen resistance. Cell. 88, 695–705. doi: 10.1016/s0092-8674(00)81912-1
Bai, Y., Pavan, S., Zheng, Z., Zappel, N. F., Reinstadler, A., Lotti, C., et al. (2008). Naturally occurring broad-spectrum powdery mildew resistance in a Central American tomato accession is caused by loss of mlo function. Mol. Plant Microbe Interact. 21, 30–39. doi: 10.1094/MPMI-21-1-0030
Baltruschat, H., Fodor, J., Harrach, B. D., Niemczyk, E., Barna, B., Gullner, G., et al. (2008). Salt tolerance of barley induced by the root endophyte Piriformospora indica is associated with a strong increase in antioxidants. New Phytol. 180, 501–510. doi: 10.1111/j.1469-8137.2008.02583.x
Banhara, A., Ding, Y., Kuhner, R., Zuccaro, A., Parniske, M. (2015). Colonization of root cells and plant growth promotion by Piriformospora indica occurs independently of plant common symbiosis genes. Front. Plant Sci. 6, 667. doi: 10.3389/fpls.2015.00667
Barazani, O., Benderoth, M., Groten, K., Kuhlemeier, C., Baldwin, I. T. (2005). Piriformospora indica and Sebacina vermifera increase growth performance at the expense of herbivore resistance in Nicotiana attenuata. Oecologia. 146, 234–243. doi: 10.1007/s00442-005-0193-2
Basiewicz, M., Weiss, M., Kogel, K.-H., Langen, G., Zorn, H., Zuccaro, A. (2012). Molecular and phenotypic characterization of Sebacina vermifera strains associated with orchids, and the description of Piriformospora williamsii sp. nov. Fungal Biol. 116, 204–213. doi: 10.1016/j.funbio.2011.11.003
Baxter, A., Mittler, R., Suzuki, N. (2014). ROS as key players in plant stress signalling. J. Exp. Bot. 65, 1229–1240. doi: 10.1093/jxb/ert375
Bayles, C. J., Ghemawat, M. S., Aist, J. R. (1990). Inhibition by 2-deoxy-D-glucose of callose formation, papilla deposition, and resistance to powdery mildew in an ml-o barley mutant. Physiol. Mol. Plant Pathol. 36, 63–72. doi: 10.1016/0885-5765(90)90092-C
Bonfante, P., Genre, A. (2010). Mechanisms underlying beneficial plant-fungus interactions in mycorrhizal symbiosis. Nat. Commun. 1, 48. doi: 10.1038/ncomms1046
Camehl, I., Drzewiecki, C., Vadassery, J., Shahollari, B., Sherameti, I., Forzani, C., et al. (2011). The OXI1 kinase pathway mediates Piriformospora indica-induced growth promotion in Arabidopsis. PloS Pathog. 7, e1002051. doi: 10.1371/journal.ppat.1002051
Chowdhury, J., Henderson, M., Schweizer, P., Burton, R. A., Fincher, G. B., Little, A. (2014). Differential accumulation of callose, arabinoxylan and cellulose in nonpenetrated versus penetrated papillae on leaves of barley infected with Blumeria graminis f. sp. hordei. New Phytol. 204, 650–660. doi: 10.1111/nph.12974
Consonni, C., Humphry, M. E., Hartmann, H. A., Livaja, M., Durner, J., Westphal, L., et al. (2006). Conserved requirement for a plant host cell protein in powdery mildew pathogenesis. Nat. Genet. 38, 716–720. doi: 10.1038/ng1806
Deshmukh, S., Hückelhoven, R., Schäfer, P., Imani, J., Sharma, M., Weiss, M., et al. (2006). The root endophytic fungus Piriformospora indica requires host cell death for proliferation during mutualistic symbiosis with barley. Proc. Natl. Acad. Sci. U.S.A. 103, 18450–18457. doi: 10.1073/pnas.0605697103
Devoto, A., Piffanelli, P., Nilsson, I., Wallin, E., Panstruga, R., von Heijne, G., et al. (1999). Topology, subcellular localization, and sequence diversity of the Mlo family in plants. J. Biol. Chem. 274, 34993–35004. doi: 10.1074/jbc.274.49.34993
Donoghue, J. R. (2004). “Implementing Schaffer's multiple comparison procedure for a large number of groups,” Recent Developments in multiple comparison procedures. (OH, USA: Institute of Mathematical Statistics), 1–23. doi: 10.1214/lnms/1196285622
Doyle, J. J., Doyle, J. L. (1987). A rapid DNA isolation procedure for small quantities of fresh leaf tissue. Phytochem. Bull. 19, 11–15.
Elliott, C., Zhou, F., Spielmeyer, W., Panstruga, R., Schulze-Lefert, P. (2002). Functional conservation of wheat and rice Mlo orthologs in defense modulation to the powdery mildew fungus. Mol. Plant Microbe Interact. 15, 1069–1077. doi: 10.1094/MPMI.2002.15.101069
Elliott, C., Muller, J., Miklis, M., Bhat, R. A., Schulze-Lefert, P., Panstruga, R. (2005). Conserved extracellular cysteine residues and cytoplasmic loop-loop interplay are required for functionality of the heptahelical MLO protein. Biochem. J. 385, 243–254. doi: 10.1042/BJ20040993
Enkerli, J., Bhatt, G., Covert, S. F. (1997). Nht1, a transposable element cloned from a dispensable chromosome in Nectria haematococca. Mol. Plant Microbe Interact. 10, 742–749. doi: 10.1094/MPMI.1997.10.6.742
Fenster, T., Hause, G. (2005). Accumulation of reactive oxygen species in arbuscular mycorrhizal roots. Mycorrhiza. 15, 3743–3779. doi: 10.1007/s00572-005-0363-4
Franken, P. (2012). The plant strengthening root endophyte Piriformospora indica: potential application and the biology behind. Appl. Microbiol. Biotechnol. 96, 1455–1464. doi: 10.1007/s00253-012-4506-1
Genre, A., Bonfante, P. (2002). Epidermal cells of a symbiosis-defective mutant of Lotus japonicus show altered cytoskeleton organisation in the presence of a mycorrhizal fungus. Protoplasma. 219, 43–50. doi: 10.1007/s007090200004
Genre, A., Chabaud, M., Timmers, T., Bonfante, P., Barker, D. G. (2005). Arbuscular mycorrhizal fungi elicit a novel intracellular apparatus in Medicago truncatula root epidermal cells before infection. Plant Cell. 17, 3489–3499. doi: 10.1105/tpc.105.035410
Genre, A., Chabaud, M., Faccio, A., Barker, D. G., Bonfante, P. (2008). Prepenetration apparatus assembly precedes and predicts the colonization patterns of arbuscular mycorrhizal fungi within the root cortex of both Medicago truncatula and Daucus carota. Plant Cell. 20, 1407–1420. doi: 10.1105/tpc.108.059014
Genre, A., Ivanov, S., Fendrych, M., Faccio, A., Zarsky, V., Bisseling, T., et al. (2012). Multiple exocytotic markers accumulate at the sites of perifungal membrane biogenesis in arbuscular mycorrhizas. Plant Cell Physiol. 53, 244–255. doi: 10.1093/pcp/pcr170
Ghimire, S. R., Craven, K. D. (2011). Enhancement of switchgrass (Panicum virgatum L.) biomass production under drought conditions by the ectomycorrhizal fungus Sebacina vermifera. Appl. Environ. Microbiol. 77, 7063–7067. doi: 10.1128/AEM.05225-11
Ghimire, S. R., Charlton, N. D., Craven, K. D. (2009). The mycorrhizal fungus, Sebacina vermifera, enhances seed germination and biomass production in swtichgrass (Panicum virgatum L.). Bioenergy Res. 2, 51–58. doi: 10.1007/s12155-009-9033-2
Greenshields, D. L., Liu, G., Wei, Y. (2007). Roles of iron in plant defence and fungal virulence. Plant Signal Behav. 2, 300–302. doi: 10.4161/psb.2.44042
Guether, M., Balestrini, R., Hannah, M., He, J., Udvardi, M. K., Bonfante, P. (2009). Genome-wide reprogramming of regulatory networks, transport, cell wall and membrane biogenesis during arbuscular mycorrhizal symbiosis in Lotus japonicus. New Phytol. 182, 200–212. doi: 10.1111/j.1469-8137.2008.02725.x
Gutjahr, C. (2014). Phytohormone signaling in arbuscular mycorhiza development. Curr. Opin. Plant Biol. 20, 26–34. doi: 10.1016/j.pbi.2014.04.003
Hückelhoven, R., Fodor, J., Preis, C., Kogel, K.-H. (1999). Hypersensitive cell death and papilla formation in barley attacked by the powdery mildew fungus are associated with hydrogen peroxide but not with salicylic acid accumulation. Plant Physiol. 119, 1251–1260. doi: 10.1104/pp.119.41251
Hückelhoven, R. (2005). Powdery mildew susceptibility and biotrophic infection strategies. FEMS Microbiol. Lett. 245, 9–17. doi: 10.1016/j.femsle.2005.03.001
Hückelhoven, R. (2007). Cell wall-associated mechanisms of disease resistance and susceptibility. Annu. Rev. Phytopathol. 45, 101–127. doi: 10.1146/annurev.phyto.45.062806.094325
Hückelhoven, R. (2014). The effective papilla hypothesis. New Phytol. 204, 438–440. doi: 10.1111/nph.13026
Harrison, M. J. (2005). Signaling in the arbuscular mycorrhizal symbiosis. Annu. Rev. Microbiol. 59, 19–42. doi: 10.1146/annurev.micro.58.030603.123749
Humphry, M., Reinstadler, A., Ivanov, S., Bisseling, T., Panstruga, R. (2011). Durable broad-spectrum powdery mildew resistance in pea er1 plants is conferred by natural loss-of-function mutations in PsMLO1. Mol. Plant Pathol. 12, 866–878. doi: 10.1111/j.1364-3703.2011.00718.x
Israel, H. W., Wilson, R. G., Aist, J. R., Kunoh, H. (1980). Cell wall appositions and plant disease resistance: acoustic microscopy of papillae that block fungal ingress. Proc. Natl. Acad. Sci. U.S.A. 77, 2046–2049. doi: 10.1073/pnas.77.42046
Jørgensen, I. H. (1992). Discovery, characterization and exploitation of Mlo powdery mildew resistance in barley. Euphytica. 63, 141–152. doi: 10.1007/BF00023919
Jacobs, S., Zechmann, B., Molitor, A., Trujillo, M., Petutschnig, E., Lipka, V., et al. (2011). Broad-spectrum suppression of innate immunity is required for colonization of Arabidopsis roots by the fungus Piriformospora indica. Plant Physiol. 156, 726–740. doi: 10.1104/pp.111.176446
Jarosch, B., Kogel, K.-H., Schaffrath, U. (1999). The ambivalence of the barley Mlo locus: Mutations conferring resistance against powdery mildew (Blumeria graminis f. sp. hordei) enhance susceptibility to the rice blast fungus Magnaporthe grisea. Mol. Plant Microbe Interact. 12, 508–514. doi: 10.1094/MPMI.1999.12.6.508
Jarosch, B., Jansen, M., Schaffrath, U. (2003). Acquired resistance functions in mlo barley, which is hypersusceptible to Magnaporthe grisea. Mol. Plant Microbe Interact. 16, 107–114. doi: 10.1094/MPMI.2003.16.2.107
Khaosaad, T., García-Garrido, J. M., Steinkellner, S., Vierheilig, H. (2007). Take-all disease is systemically reduced in roots of mycorrhizal barley plants. Soil Biol. Biochem. 39, 727–734. doi: 10.1016/j.soilbio.2006.09.014
Kistner, C., Winzer, T., Pitzschke, A., Mulder, L., Sato, S., Kaneko, T., et al. (2005). Seven Lotus japonicus genes required for transcriptional reprogramming of the root during fungal and bacterial symbiosis. Plant Cell. 17, 2217–2229. doi: 10.1105/tpc.105.032714
Kumar, J., Hückelhoven, R., Beckhove, U., Nagarajan, S., Kogel, K.-H. (2001). A compromised Mlo pathway affects the response of barley to the necrotrophic fungus Bipolaris sorokiniana (teleomorph: Cochliobolus sativus) and its toxins. Phytopathol. 91, 127–133. doi: 10.1094/PHYTO.2001.91.2.127
Kumar, V., Sarma, M. V. R. K., Saharan, K., Srivastava, R., Kumar, L., Sahai, V., et al. (2012). Effect of formulated root endophytic fungus Piriformospora indica and plant growth promoting rhizobacteria fluorescent pseudomonads R62 and R81 on Vigno mungo. World J. Microbiol. Biotechnol. 28, 595–603. doi: 10.1007/s11274-011-0852-x
Kusch, S., Panstruga, R. (2017). mlo-based resistance: an apparently universal “weapon” to defeat powdery mildew disease. Mol. Plant Microbe Interact. 30, 179–189. doi: 10.1094/MPMI-12-16-0255-CR
Kusch, S., Pesch, L., Panstruga, R. (2016). Comprehensive phylogenetic analysis sheds light on the diversity and origin of the MLO family of integral membrane proteins. Genome Biol. Evol. 8, 878–895. doi: 10.1093/gbe/evw036
Lahrmann, U., Zuccaro, A. (2012). Opprimo ergo sum–evasion and suppression in the root endophytic fungus Piriformospora indica. Mol. Plant Microbe Interact. 25, 727–737. doi: 10.1094/MPMI-11-11-0291
Lahrmann, U., Ding, Y., Banhara, A., Rath, M., Hajirezaei, M. R., Dohlemann, S., et al. (2013). Host-related metabolic cues affect colonization strategies of a root endophyte. Proc. Natl. Acad. Sci. U.S.A. 110, 13965–13970. doi: 10.1073/pnas.1301653110
Lahrmann, U., Strehmel, N., Langen, G., Frerigmann, H., Leson, L., Ding, Y., et al. (2015). Mutualistic root endophytism is not associated with the reduction of saprotrophic traits and requires a noncompromised plant innate immunity. New Phytol. 207, 841–857. doi: 10.1111/nph.13411
Le Fevre, R., O’Boyle, B., Moscou, M. J., Schornack, S. (2016). Colonization of barley by the broad-host hemibiotrophic pathogen Phytophthora palmivora uncovers a leaf development-dependent involvement of Mlo. Mol. Plant Microbe Interact. 29, 385–395. doi: 10.1094/MPMI-12-15-0276-R
Liu, G., Greenshields, D. L., Sammynaiken, R., Hirji, R. N., Selvaraj, G., Wei, Y. (2007). Targeted alterations in iron homeostasis underlie plant defense responses. J. Cell Sci. 120, 596–605. doi: 10.1242/jcs.001362
Liu, J., Maldonado-Mendoza, I., Lopez-Meyer, M., Cheung, F., Town, C. D., Harrison, M. J. (2007). Arbuscular mycorrhizal symbiosis is accompanied by local and systemic alterations in gene expression and an increase in disease resistance in the shoots. Plant J. 50, 529–544. doi: 10.1111/j.1365-313X.2007.03069.x
Lyngkjær, M., Newton, A., Atzema, J., Baker, S. (2000). The barley mlo-gene: an important powdery mildew resistance source. Agronomie. 20, 745–756. doi: 10.1051/agro:2000173
McGrann, G. R., Stavrinides, A., Russell, J., Corbitt, M. M., Booth, A., Chartrain, L., et al. (2014). A trade off between mlo resistance to powdery mildew and increased susceptibility of barley to a newly important disease, Ramularia leaf spot. J. Exp. Bot. 65, 1025–1037. doi: 10.1093/jxb/ert452
McNeil, M., Albersheim, P., Taiz, L., Jones, R. L. (1975). The structure of plant cell walls: VII. Barley Aleurone Cells Plant Physiol. 55, 64–68. doi: 10.1104/pp.55.1.64
Miklis, M., Consonni, C., Bhat, R. A., Lipka, V., Schulze-Lefert, P., Panstruga, R. (2007). Barley MLO modulates actin-dependent and actin-independent antifungal defense pathways at the cell periphery. Plant Physiol. 144, 1132–1143. doi: 10.1104/pp.107.098897
Miller, R. G. (1981). Simultaneous statistical inference. (New York, NY: Springer), doi: 10.1007/978-1-4613-8122-8
Nautiyal, C. S., Chauhan, P. S., DasGupta, S. M., Seem, K., Varma, A., Staddon, W. J. (2010). Tripartite interactions among Paenibacillus lentimorbus NRRL B-30488, Piriformospora indica DSM 11827, and Cicer arietinum L. World J. Microbiol. Biotechnol. 26, 1393–1399. doi: 10.1007/s11274-010-0312-z
Parniske, M. (2008). Arbuscular mycorrhiza: the mother of plant root endosymbioses. Nat. Rev. Microbiol. 6, 763–775. doi: 10.1038/nrmicro1987
Peterhänsel, C., Freialdenhoven, A., Kurth, J., Kolsch, R., Schulze-Lefert, P. (1997). Interaction analyses of genes required for resistance responses to powdery mildew in barley reveal distinct pathways leading to leaf cell death. Plant Cell. 9, 1397–1409. doi: 10.1105/tpc.9.81397
Pham, G. H., Singh, A., Malla, R., Kumari, R., Prasad, R., Sachdev, M., et al. (2004). “Interaction of P. indica with other microorganisms and plants,” in Plant Surface Microbiology. Eds. Varma, A., Abbott, L., Werner, D., Hampp, R. (Heidelberg, Germany: Springer), 237–265.
Piepho, H.-P. (2004). An algorithm for letter-based representation of all-pairwise comparisons. J. Comp. Graph. Stat. 13, 456–466. doi: 10.1198/1061860043515
Pozo, M. J., Azcon-Aguilar, C. (2007). Unraveling mycorrhiza-induced resistance. Curr. Opin. Plant Biol. 10, 393–398. doi: 10.1016/j.pbi.2007.05.004
Qiang, X., Zechmann, B., Reitz, M. U., Kogel, K.-H., Schäfer, P. (2012). The mutualistic fungus Piriformospora indica colonizes Arabidopsis roots by inducing an endoplasmic reticulum stress-triggered caspase-dependent cell death. Plant Cell. 24, 794–809. doi: 10.1105/tpc.111.093260
Rey, T., Nars, A., Bonhomme, M., Bottin, A., Huguet, S., Balzergue, S., et al. (2013). NFP, a LysM protein controlling Nod factor perception, also intervenes in Medicago truncatula resistance to pathogens. New Phytol. 198, 875–886. doi: 10.1111/nph.12198
Roschzttardtz, H., Conejero, G., Curie, C., Mari, S. (2009). Identification of the endodermal vacuole as the iron storage compartment in the Arabidopsis embryo. Plant Physiol. 151, 1329–1338. doi: 10.1104/pp.109.144444
Roschzttardtz, H., Conejero, G., Divol, F., Alcon, C., Verdeil, J. L., Curie, C., et al. (2013). New insights into Fe localization in plant tissues. Front. Plant Sci. 4, 350. doi: 10.3389/fpls.2013.00350
Ruiz-Lozano, J. M., Gianinazzi, S., Gianinazzi-Pearson, V. (1999). Genes involved in resistance to powdery mildew in barley differentially modulate root colonization by the mycorrhizal fungus Glomus mosseae. Mycorrhiza 9, 237–240. doi: 10.1007/s005720050273
Sarkar, D., Rovenich, H., Jeena, G., Nizam, S., Tissier, A., Balcke, G. U., et al. (2019). The inconspicuous gatekeeper: endophytic Serendipita vermifera acts as extended plant protection barrier in the rhizosphere. New Phytol. 224, 886–901. doi: 10.1111/nph.15904
Schäfer, P., Pfiffi, S., Voll, L. M., Zajic, D., Chandler, P. M., Waller, F., et al. (2009). Manipulation of plant innate immunity and gibberellin as factor of compatibility in the mutualistic association of barley roots with Piriformospora indica. Plant J. 59, 461–474. doi: 10.1111/j.1365-313X.2009.03887.x
Schmittgen, T. D., Livak, K. J. (2008). Analyzing real-time PCR data by the comparative C(T) method. Nat. Protoc. 3, 1101–1108. doi: 10.1038/nprot.2008.73
Serfling, A., Wirsel, S. G., Lind, V., Deising, H. B. (2007). Performance of the biocontrol fungus Piriformospora indica on wheat under greenhouse and field conditions. Phytopathol 97, 523–531. doi: 10.1094/PHYTO-97-4-0523
Shahollari, B., Varma, A., Oelmuller, R. (2005). Expression of a receptor kinase in Arabidopsis roots is stimulated by the basidiomycete Piriformospora indica and the protein accumulates in Triton X-100 insoluble plasma membrane microdomains. J. Plant Physiol. 162, 945–958. 10.1016/j.jplph.2004.08.012
Sherameti, I., Shahollari, B., Venus, Y., Altschmied, L., Varma, A., Oelmuller, R. (2005). The endophytic fungus Piriformospora indica stimulates the expression of nitrate reductase and the starch-degrading enzyme glucan-water dikinase in tobacco and Arabidopsis roots through a homeodomain transcription factor that binds to a conserved motif in their promoters. J. Biol. Chem. 280, 26241–26247. doi: 10.1074/jbc.M500447200
Sherameti, I., Tripathi, S., Varma, A., Oelmuller, R. (2008). The root-colonizing endophyte Pirifomospora indica confers drought tolerance in Arabidopsis by stimulating the expression of drought stress-related genes in leaves. Mol. Plant Microbe Interact. 21, 799–807. doi: 10.1094/MPMI-21-6-0799
Skou, J. P., Jørgensen, J. H., Lilholt, U. (1984). Comparative studies on callose formation in powdery mildew compatible and incompatible barley. J. Phytopathol. 109, 147–168. doi: 10.1111/j.1439-0434.1984.tb00702.x
Stein, E., Molitor, A., Kogel, K.-H., Waller, F. (2008). Systemic resistance in Arabidopsis conferred by the mycorrhizal fungus Piriformospora indica requires jasmonic acid signaling and the cytoplasmic function of NPR1. Plant Cell Physiol. 49, 1747–1751. doi: 10.1093/pcp/pcn147
Svistoonoff, S., Hocher, V., Gherbi, H. (2014). Actinorhizal root nodule symbioses: what is signalling telling on the origins of nodulation? Curr. Opin. Plant Biol. 20, 11–18. doi: 10.1016/j.pbi.2014.03.001
Thordal-Christensen, H., Zhang, Z., Yangdou, W., Collinge, D. B. (1997). Subcellular localization of H2O2 in plants. H2O2 accumulation in papillae and hypersensitive response during barley-powdery mildew infection. Plant J. 11, 1187–1194. doi: 10.1046/j.1365-313X.1997.11061187.x
Torres, M. A., Jones, J. D., Dangl, J. L. (2006). Reactive oxygen species signaling in response to pathogens. Plant Physiol. 141, 373–378. doi: 10.1104/pp.106.079467
Trouvelot, A., Kough, J., Gianinazzi-Pearson, V. (1986). “Measuring the rate of VA mycorrhization of root systems. Research methods for estimating having a functional significance,” in Proceedings of the 1st European symposium on mycorrhizae: Physiological and genetical aspects of mycorrhizae. Eds. Gianinazzi-Pearson, V., Gianinazzi, S. (Dijon, Paris: INRA), 217–222.
van Schie, C. C., Takken, F. L. (2014). Susceptibility genes 101: how to be a good host. Annu. Rev. Phytopathol. 52, 551–581. doi: 10.1146/annurev-phyto-102313-045854
Varma, A., Savita, V., Sudha, Sahay, N., Butehorn, B., Franken, P. (1999). Piriformospora indica, a cultivable plant-growth-promoting root endophyte. Appl. Environ. Microbiol. 65, 2741–2744.
Vigneron, N., Radhakrishnan, G. V., Delaux, P. M. (2018). What have we learnt from studying the evolution of the arbuscular mycorrhizal symbiosis? Curr. Opin. Plant Biol. 44, 49–56. doi: 10.1016/j.pbi.2018.02.004
von Röpenack, E., Parr, A., Schulze-Lefert, P. (1998). Structural analyses and dynamics of soluble and cell wall-bound phenolics in a broad spectrum resistance to the powdery mildew fungus in barley. J. Biol. Chem. 273, 9013–9022. doi: 10.1074/jbc.273.159013
Waller, F., Achatz, B., Baltruschat, H., Fodor, J., Becker, K., Fischer, M., et al. (2005). The endophytic fungus Piriformospora indica reprograms barley to salt-stress tolerance, disease resistance, and higher yield. Proc. Natl. Acad. Sci. U.S.A. 102, 13386–13391. doi: 10.1073/pnas.0504423102
Waller, F., Mukherjee, K., Deshmukh, S. D., Achatz, B., Sharma, M., Schäfer, P., et al. (2008). Systemic and local modulation of plant responses by Piriformospora indica and related Sebacinales species. J. Plant Physiol. 165, 60–70. doi: 10.1016/j.jplph.2007.05.017
Wang, Y., Cheng, X., Shan, Q., Zhang, Y., Liu, J., Gao, C., et al. (2014). Simultaneous editing of three homoeoalleles in hexaploid bread wheat confers heritable resistance to powdery mildew. Nat. Biotechnol. 32, 947–951. doi: 10.1038/nbt2969
Wang, J. Y., Haider, I., Jamil, M., Fiorilli, V., Saito, Y., Mi, J., et al. (2019). The apocarotenoid metabolite zaxinone regulates growth and strigolactone biosynthesis in rice. Nat. Commun. 10, 810. doi: 10.1038/s41467-019-08461-1
Weiss, M., Waller, F., Zuccaro, A., Selosse, M. A. (2016). Sebacinales - one thousand and one interactions with land plants. New Phytol. 211, 20–40. doi: 10.1111/nph.13977
Wolter, M., Hollricher, K., Salamini, F., Schulze-Lefert, P. (1993). The mlo resistance alleles to powdery mildew infection in barley trigger a developmentally controlled defence mimic phenotype. Mol. Gen. Genet. 239, 122–128. doi: 10.1007/bf00281610
Yadav, V., Kumar, M., Deep, D. K., Kumar, H., Sharma, R., Tripathi, T., et al. (2010). A phosphate transporter from the root endophytic fungus Piriformospora indica plays a role in phosphate transport to the host plant. J. Biol. Chem. 285, 26532–26544. doi: 10.1074/jbc.M110.111021
Yandell, B. S. (1997). Practical data analysis for designed experiments. (New York, NY: Chapman and Hall/CRC). doi: 10.1201/97802037425
Zeyen, R. J., Carver, T. L. W., Lyngkjær, M. F. (2002). “Epidermal cell papillae,” in The powdery mildews: a comprehensive treatise. Eds. Bélanger, R. R., Bushnell, W. R., Dik, A. J., Carver, T. L. W. (St Paul, MN, USA: APS Press), 107–125.
Keywords: biotrophy, cell death, fungal-root interactions, mutualism, cell wall appositions, Perls/DAB, VPE activity, susceptibility gene
Citation: Hilbert M, Novero M, Rovenich H, Mari S, Grimm C, Bonfante P and Zuccaro A (2020) MLO Differentially Regulates Barley Root Colonization by Beneficial Endophytic and Mycorrhizal Fungi. Front. Plant Sci. 10:1678. doi: 10.3389/fpls.2019.01678
Received: 09 August 2019; Accepted: 28 November 2019;
Published: 16 January 2020.
Edited by:
Pierre-Emmanuel Courty, INRA Centre Dijon Bourgogne Franche-Comté, FranceReviewed by:
Ralph Panstruga, RWTH Aachen University, GermanyHannah Kuhn, RWTH Aachen University, Germany
Brigitte Mauch-Mani, Université de Neuchâtel, Switzerland
Copyright © 2020 Hilbert, Novero, Rovenich, Mari, Grimm, Bonfante and Zuccaro. This is an open-access article distributed under the terms of the Creative Commons Attribution License (CC BY). The use, distribution or reproduction in other forums is permitted, provided the original author(s) and the copyright owner(s) are credited and that the original publication in this journal is cited, in accordance with accepted academic practice. No use, distribution or reproduction is permitted which does not comply with these terms.
*Correspondence: Alga Zuccaro, YXp1Y2Nhcm9AdW5pLWtvZWxuLmRl
†These authors have contributed equally to this work