- 1Ufa Institute of Biology, Ufa Federal Research Centre (RAS), Ufa, Russia
- 2The Lancaster Environment Centre, Lancaster University, Lancaster, United Kingdom
The capacity of rhizoshere bacteria to influence plant hormonal status, by bacterial production or metabolism of hormones, is considered an important mechanism by which they promote plant growth, and productivity. Nevertheless, inoculating these bacteria into the plant rhizosphere may produce beneficial or detrimental results depending on bacterial effects on hormone composition and quantity in planta, and the environmental conditions under which the plants are growing. This review considers some effects of bacterial hormone production or metabolism on root growth and development and shoot physiological processes. We analyze how these changes in root and shoot growth and function help plants adapt to their growth conditions, especially as these change from optimal to stressful. Consistent effects are addressed, along with plant responses to specific environmental stresses: drought, salinity, and soil contamination (with petroleum in particular).
Introduction
The capacity of some rhizosphere bacteria (so-called plant growth promoting rhizobacteria—PGPR) to promote plant growth under stressful environments (drought, salinity, suboptimal temperature, toxic metals, pollution with organic substances of man-made origin) is attracting increasing attention (Belimov et al., 2009a; Gerhardt et al., 2009; Paul and Lade, 2014; Vejan et al., 2016; Backer et al., 2018). Microorganisms can directly influence plant growth by synthesizing growth-stimulating hormones (Spaepen and Vanderleyden, 2011; Kudoyarova et al., 2015a; Shi et al., 2017) and metabolizing growth-inhibitory hormones (Belimov et al., 2009a; Glick, 2014). The importance of hormones in mediating such plant/microbe interactions has been highlighted by experiments showing that inoculation with PGPR causes pivotal changes in plant expression of hormone-mediated genes (Lara-Chavez et al., 2015; Ambreetha et al., 2018; Jatan et al., 2018). Changes in plant hormonal status may result from either microbial consumption or production of hormones, or changes in plant hormone metabolism in planta (Dodd et al., 2010) that may be induced by volatile substances synthesized by microorganisms (Zhang et al., 2007). In turn, plant hormones such as auxins (Spaepen and Vanderleyden, 2011) affect microbial gene expression following their addition to culture media.
Hormones produced by PGPR are mostly related directly to plant growth promotion, while other effects of PGPR (their capacity to improve mineral nutrition and plant resistance to pathogens and abiotic stress) are considered independently from microbial effects on plant hormonal system in other reviews. Meanwhile, the effects of PGPR on plant hormonal regulation are important not only in directly promoting plant growth, but also in other aspects of PGPR action on plants, such as improving mineral nutrition or plant resistance to biotic stresses. Plant hormones regulate vitally important processes in plants such as mineral nutrition, water relations, resistance to pathogens, and antioxidant functions. Thus, hormone-mediated stimulation of root growth can improve mineral nutrition and water relations (Kudoyarova et al., 2015b and references therein). Furthermore, hormone-mediated stimulation of plant antioxidant systems, such as catalase, ascorbate peroxidase (Zavaleta-Mancera et al., 2007), and CuZn-superoxide dismutase (Tyburski et al., 2009) enzymes, helps protect plants against oxidative stress, which accompanies most detrimental environmental factors (Xia et al., 2015). PGPR can also improve plant mineral nutrition by fixing atmospheric nitrogen or solubilizing phosphates, thereby indirectly affecting phytohormone concentrations in planta, since plant hormonal status partially depends on the availability of mineral nutrients (Kudoyarova et al., 2015b and references therein). Thus the capacity of rhizosphere bacteria to influence plant hormonal status is likely involved in most known mechanisms of growth promoting action by PGPR.
However, PGPR effects on plant hormone systems may be either beneficial or detrimental for plants depending on their growth environment, with much literature indicating that inhibition (and not promotion) of plant growth protects plants against stress factors (Chapin, 1991; Chaves et al., 2003; Achard et al., 2006; Bechtold and Field, 2018). This review aims to assess whether the outcome of microbial effects on plant hormonal system changes according to whether plants are grown under either optimal or stressful environments. Special attention is paid to plant/microbe interactions when the soil dries, is salinized, or is contaminated with petroleum. This information may help choose optimal PGPR traits for their use in certain environments. PGPR effects on plant hormonal status is discussed with attention focused on auxins, cytokinins, abscisic acid (ABA) and ethylene. Although PGPRs can produce other plant hormones (e.g. gibberellins or jasmonates), those are not included in this review and interested readers may find information about them in corresponding reviews (Bottini et al., 2004; Van der Ent et al., 2009). Nevertheless, mechanisms of auxins, cytokinins, ABA, and ethylene action may inform general conclusions that are relevant to other plant hormones produced by PGPR.
Effects of Bacterial Auxins on Root Growth and Development
Changes in root growth and development are most important for adapting plants to either optimal or stressful environments. Microbial acceleration of root growth is very important, since some cultivars are characterized by weak root system development (Ehdaie et al., 2003; Waines and Ehdaie, 2007). New varieties with deeper root systems accessed more of the stored soil moisture at depth than current varieties, thus producing higher yields (Watt et al., 2013). Despite recent efforts, root growth and development still has not been fully exploited as a yield enhancement strategy (Den Herder et al., 2010). Germplasm from many breeding programs has traditionally been evaluated at high levels of mineral nutrition, where enhanced root development is not needed. However, since well-developed roots are needed under conditions of water deficit, many modern plant breeding efforts are focused on vigorous root systems (Comas et al., 2013). Nevertheless, selection of cultivars with specific root traits is a long process, while the use of bacterial preparations potentially enables fast results.
Rhizobacterial stimulation of root growth is mostly considered to be via their capacity to synthesize indole acetic acid (IAA—the most common, naturally occurring, plant hormone of the auxin class) (Spaepen and Vanderleyden, 2011), since stimulation of rhizogenesis is one of the best known effects of auxins. Many rhizosphere bacteria can synthesize auxins (Spaepen and Vanderleyden, 2011), with addition of tryptophan to bacterial culture mediа providing a simple method to determine bacterial auxin production (Blinkov et al., 2014). Inoculating canola (Brassica napus) plants with mutant strains of Pseudomomas putida with decreased synthesis of auxins diminished the growth promoting effect of inoculation on root growth (Patten and Glick, 2002). Similarly, inoculating wheat (Triticum aestivum) seedlings with IAA-deficient mutants of the salt tolerant Pseudomomas moraviensis decreased root surface area by 13%–38% compared to inoculating with the wild-type strain (Hassan and Bano, 2019). Furthermore, the importance of bacterial auxins for stimulating plant root proliferation was also confirmed in experiments showing that Azospirillum mutants deficient in auxin production did not enhance wheat root development (Dobbelaere et al., 1999). Thus mutational analyses of the significance of auxin in plant/microbe interactions gave consistent results across two bacterial genera and different plant species.
Experiments with exogenously applied synthetic auxins showed that their action on root growth may depend on the site of hormone application. Thus addition of auxins to the root tips enhanced lateral root initiation (Casimiro et al., 2001), while shoot application of auxin stimulated lateral root emergence (Reed et al., 1998). In accordance, different plant response may be expected depending on the site of PGPR application that may be achieved either through leaf spraying or seed inoculation (Gunes et al., 2015). Since our article mainly focuses on rhizobacteria, root zone inoculation is mainly considered.
Inoculating wheat plants with the auxin-producing Paenibacillus illinoisensis IB 1087 and Pseudomonas extremaustralis IB-К13-1А increased root mass and root auxin concentrations (Kudoyarova et al., 2017). Field experiments showed that pre-sowing bacterization of the wheat seeds with auxin-producing (P. extremaustralis IB-К13-1А) or phosphate solubilizing strains (Advenella kashmirensis IB-К1 and P. extremaustralis IB-К13-1А) increased crop yield by 10% to 36%, although the relative significance of each bacterial trait was not clear (Arkhipova et al., 2019). Inoculating seeds with all these strains increased the number of spike bearing tillers compared to uninoculated controls. The number of spikelets in the main spike was greater than in the controls only following P. extremaustralis IB-К13-1А inoculation. Treatment with all the strains (except A. kashmirensis IB-К1) increased the number of grains and their weight in the main spike. This effect was more pronounced in axillary spikes, where P. extremaustralis IB-К13-1А inoculation approximately doubled grain weight and number, with lesser effects following A. kashmirensis IB-К1 inoculation. These positive effects of bacterization on wheat productivity occurred following insufficient summer rainfall at the time of grain filling, in agreement with reports of bacterial preparations having greater effects on growth and yield of droughted plants (Kuzmina and Melentev, 2003; Rubin et al., 2017). The cost of seed bacterization per hectare [2400 roubles (about $37) with a sowing density of 3–4 million seeds ha−1] was overshadowed by the economic gain of enhanced wheat yield due to PGPR action [22,000 roubles (more than $300) per hectare], suggesting such treatments may be commercially appealing to farmers.
Interestingly, strains capable of either solubilizing phosphates (A. kashmirensis IB-К1) or synthesizing auxins (B. subtilis IB-21) were less effective than the strain that combined these traits (P. extremaustralis IB-К13-1А). Introducing P. extremaustralis IB-К13-1А increased soil soluble phosphate concentrations (0.5 N acetic acid extraction) by 10% during the vegetative period compared to uninoculated controls and when bacteria unable to solubilize phosphates were applied (Arkhipova et al, 2019). Inoculating soil with P. extremaustralis IB-К13-1А increased root phosphorus concentration by 30%, while increased shoot phosphorus content was due to enhanced shoot biomass and not phosphorus concentration (Kudoyarova et al., 2017). Increased phosphorus uptake by plants inoculated with auxin producing bacteria was due not only to more plant-available phosphorus in the soil, but also to better root development correlating with increased root auxin levels (Kudoyarova et al., 2017). Application of such bacterial preparations may also allow farmers to apply less fertilizers (Adesemoye et al., 2009), thereby decreasing the costs of production.
Increased root mass following PGPR inoculation is also important for phytoremediation of soils contaminated with inorganic and organic pollutants such as toxic metals (Safronova et al., 2006), salinity (Cheng et al., 2007; Habib et al., 2016), and petroleum hydrocarbons (Greenberg et al., 2006). Plants are relatively tolerant of various environmental contaminants and are often used within phytoremediation strategies, but their biomass accumulation (and thus contaminant removal) may be limited in the presence of high contaminant levels (Glick, 2006). PGPRs increase plant tolerance to petroleum pollutants and other stresses (Gerhardt et al., 2009). Alongside the degradation of organic pollutants (Chetverikov et al., 2017, Korshunova et al., 2017), they vigorously promote plant biomass accumulation (Glick, 2003), allowing faster remediation than without PGPR application (Glick et al., 1998). Inoculating oat (Avena sativa) plants with Acinetobacter sp. increased degradation of total petroleum hydrocarbons in contaminated soil from about 33% to 45% (Xun et al., 2015), while hydrocarbon degradation was more than doubled by inoculating alfalfa (Medicago sativa) with Bacillus sp. PVMX4 (Benson et al., 2017). These effects are attributed to microbial production and provision of auxins to plants (Huang et al., 2005; Glick, 2006; Benson et al., 2017). Thus microbial auxin production may also offer environmental benefits in remediation programs.
While enhancing root mass may be an appropriate strategy to increase resource acquisition in stressful environments, optimizing root architecture (with the same carbon allocation to the roots) may be a more effective strategy. Here, the complexity of applying auxin-producing bacteria becomes apparent. Auxins stimulate root branching (Laskowski, 2013) to allow capture of light rainfall that fails to infiltrate the soil to a great depth (Hodge, 2010). Although fast root elongation into deeper, moister soil layers is important during soil drying (Xiong et al., 2006), high auxin concentrations may inhibit this process. Rhizobacterial IAA accumulation was significantly correlated with decreased elongation of sugar beet (Beta vulgaris) roots following PGPR application (Loper and Schroth, 1986). Applying auxin producing pseudomonad bacteria decreased Arabidopsis thaliana root elongation (Zamioudis et al., 2013). Indeed, treating canola seedlings with the IAA-deficient mutant of Pseudomonas putida GR12-2 accelerated root elongation, thereby confirming that high auxin concentrations decrease root length (Patten and Glick, 2002). Such root growth inhibition may be detrimental when plants grow in drying soil. Since auxin concentrations decrease in stressed plants due to increased activity of the GH3 gene involved in conjugating active free auxins (Seo et al., 2009) and activation of IAA-oxidase (Li et al., 2014), drought may sensitize plants to microbial-produced auxins. Thus inoculating common bean (Phaseolus vulgaris) with intermediate concentrations (107 CFU ml−1) of the auxin-producing Azospirillum brasilense strain Cd increased root length of plants grown in drying soil, but not in well-watered plants (German et al., 2000). The effect disappeared at higher inoculum concentrations (108 CFU ml−1), suggesting that high concentrations of microbial auxins inhibited root elongation. Thus the optimal level of microbial auxin production to enhance plant growth in drying soil may depend on the relative importance of root branching versus elongation in mediating water uptake.
Interestingly, monocotyledonous plants are less sensitive to auxins than dicotyledonous plants (Fedtke, 1982). Morgan and Hall (1962) proposed that the differential sensitivity of monocots and dicots to the synthetic auxin (and herbicide) 2,4-D was caused by differences in the rates of ethylene production following 2,4-D application. Furthermore, root growth of monocotyledonous plants (barley, wheat, and oats) was generally less sensitive than dicotyledonous plants [canola, lettuce (Lactuca sativa), tomato (Solanum lycopersicum)] to seed treatment with the 1-aminocyclopropane-1-carboxylic (ACC) deaminase containing PGPR P. putida GR12-2 or application of the chemical ethylene generator (2-chloroethyl) phosphonic acid (ethephon) (Hall et al., 1996). Differential sensitivity to bacterial auxins has been most clearly shown via inhibition of primary root elongation of the dicotyledonous plant A. thaliana (Zamioudis et al., 2013), whereas auxin producing bacteria of the Bacillus, Enterobacter, Moraxella, and Pseudomonas genera stimulated wheat (monocotyledonous plant) root elongation (Raheem et al., 2017). Nevertheless, auxin producing bacteria may also inhibit wheat root growth (Ali et al., 2009). This diversity of response may result from differences in endogenous auxin content in the studied wheat cultivars. Still, although inhibition of root elongation by auxin producing bacteria is less likely in monocotyledonous than in dicotyledonous plants, root growth inhibition is possible in both cases, which makes plants less drought-tolerant.
Inhibition of root elongation by auxins may seem surprising, since these hormones stimulate shoot cell extension. Auxin effects on root elongation may be explained by their dose-dependent capacity (increasing with auxin concentration—Arteca and Arteca, 2008) to stimulate production of the growth inhibitor ethylene (Glick, 2014). Increased ethylene production may be prevented by bacterial ACC deaminase activity, since ACC is a direct precursor of ethylene (Chen et al., 2013). Bacteria with this enzyme decreased soil and root ACC concentrations (Belimov et al., 2015), thereby lowering ethylene production throughout the plant (Chen et al., 2013) and decreasing its concentration in planta. Inoculating pea (Pisum sativum) plants with a strain of Variovorax paradoxus with high activity of this enzyme attenuated a soil-drying induced increase in xylem ACC concentration (Belimov et al., 2009b). The ACC deaminase mediated decrease in ethylene synthesis enhances root elongation, despite potentially inhibitory high concentrations of auxins (Figure 1). Enterobacter cloacae UW4 capable of synthesizing both IAA and ACC deaminase promoted root elongation of canola plants, while its ACC deaminase minus mutant failed to influence root growth suggesting this enzyme is required for bacteria to exert beneficial effects on root elongation (Li et al., 2000). Patten and Glick (2002) suggested that IAA and ACC deaminase work in concert to stimulate root elongation. Thus the capacity of rhizobacteria to produce both auxins and ACC deaminase becomes especially important under unfavorable conditions (Glick, 2014).
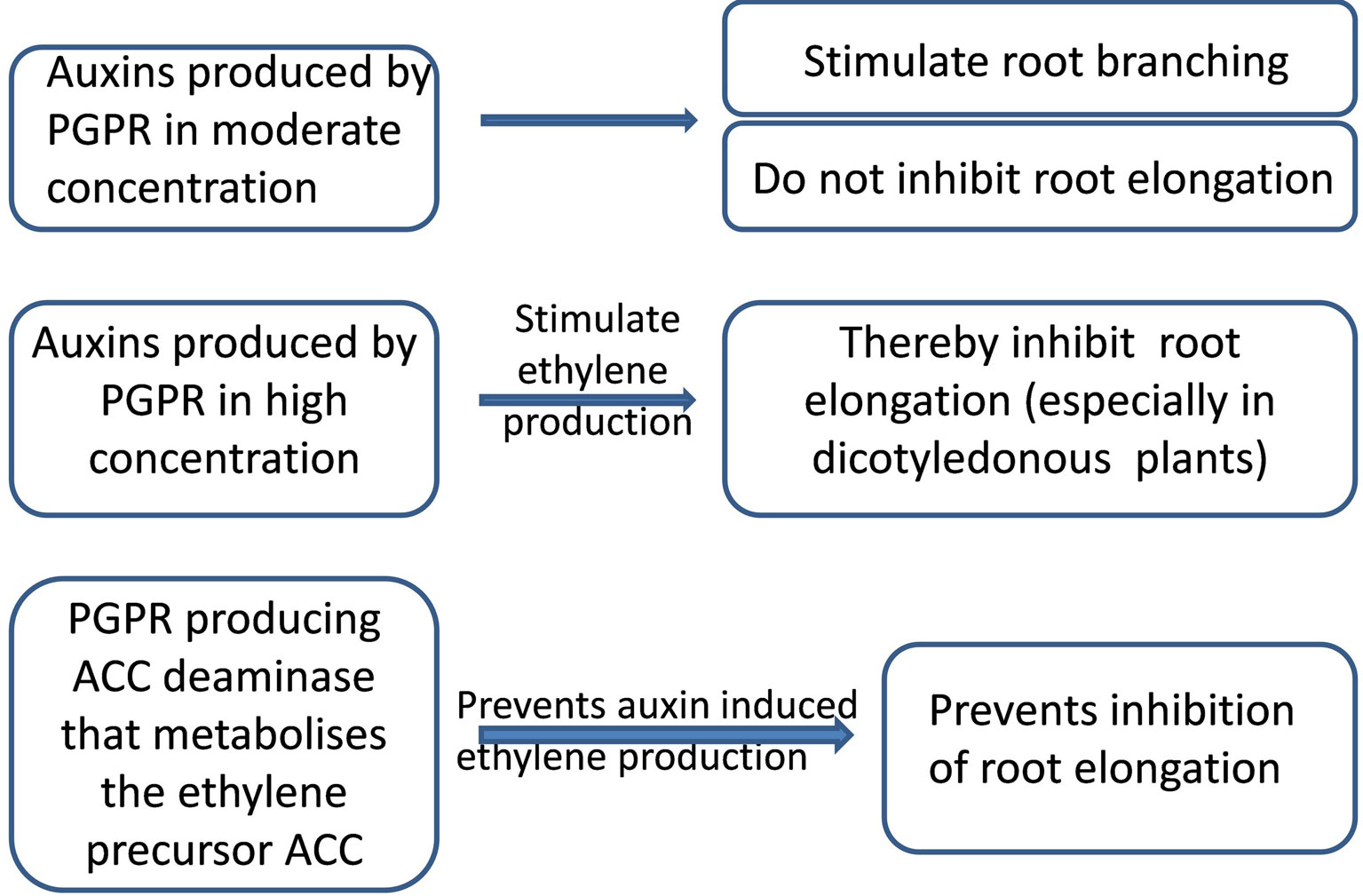
Figure 1 Scheme of bacterial action depending on the level of auxin production and presence of 1-aminocyclopropane-1-carboxylic (ACC) deaminase activity.
Bacteria producing ACC deaminase prevent not only auxin-induced, but also stress-induced, synthesis of ethylene, by consuming ACC. In practice, ACC deaminase-containing plant growth promoting bacteria have been used to protect plants against growth inhibition caused by the presence of organic toxicants and total petroleum hydrocarbons (Huang et al., 2005; Hong et al., 2011), a variety of different metals (Tiwari and Lata, 2018), high salt (Cheng et al., 2007; Singh and Jha, 2016), and drought (Mayak et al., 2004; Niu et al., 2017).
Thus applying auxin-producing PGPR may produce different outcomes under stressful and optimal conditions. Although microbial auxins can stimulate root branching, which should enhance water and nutrient capture, high concentrations of microbial auxins can inhibit root elongation which may be detrimental under drought, when long roots are necessary for extracting water from deep soil layers. Nevertheless, this potentially negative side effect of microbial auxins may be prevented by bacteria also having ACC deaminase activity.
Plant Response to Cytokinins Produced by Bacteria
The capacity of PGPR to synthesize cytokinins has been studied much less frequently than auxin production, although almost every review on PGPR mentions microbial production of cytokinins. While cytokinins undoubtedly have direct impacts on various plant processes (e.g. stimulating cell division), often the balance between auxin and cytokinin levels is considered a key regulator of plant organogenesis and root architecture. Since some PGPR are able to produce both of these hormones (Vacheron et al., 2013), tissue auxin to cytokinin ratio can be important in determining plant response to rhizobacterial inoculation.
Almost all known cytokinins were identified in the growth media of Paenibacillus polymyxa after their separation by immunoaffinity chromatography and final identification by gas chromatography–mass spectrometry (Timmusk et al., 1999). Microbial cytokinin production was suggested to stimulate plant growth, although not directly studied (Timmusk et al., 1999). A quarter of the pseudomonads isolated from rhizospheres of different crops (Pennisetum glaucum, Helianthus annuus, Zea mays) grown under 25 arid and semi-arid locations across India were capable of producing cytokinins, when grown under osmotic stress (25% PEG 6000) (Sandhya et al., 2010). Of 70 rhizobacterial strains isolated from the Coleus rhizosphere, three (Pseudomonas stutzeri, Stenotrophomonas maltophilia, and P. putida) produced cytokinins (Patel and Saraf, 2017). Since these bacteria also synthesized other hormones (auxins and gibberellins), it was difficult to determine the mechanistic basis of plant growth promotion. More convincing evidence of cytokinin involvement in the plant growth promoting effect of Bacillus megaterium was obtained using the triple cytokinin receptor CRE1-12/AHK2-2/AHK3-3 knockout mutant of Arabidopsis, in which plant development was insensitive to inoculation (Ortíz-Castro et al., 2008). Microbial cytokinin production was identified as a key determinant of the ability of Pseudomonas fluorescens G20-18 to regulate Arabidopsis development, since inoculation with G20-18 cytokinin deficient loss-of-function mutants had no effect on the plant development (Großkinsky et al., 2016). Thus microbial cytokinin production and plant sensitivity to cytokinins are both necessary for microbial stimulation of plant growth in certain plant/microbe interactions.
Introducing B. subtilis IB 22 (with high cytokinin production) into wheat and lettuce rhizospheres increased leaf area (Arkhipova et al., 2005), since cytokinins stimulate shoot cell division and elongation (Werner et al., 2003). At the same time, cytokinins can inhibit root growth (Werner et al., 2010). Bacillus amyloliquefaciens UCMB5113 inhibited primary root growth of Arabidopsis, which may be due to bacterial cytokinin production and increased root cytokinin levels, or the increased auxin levels that were also detected in colonized roots (Asari et al., 2017). Nevertheless, introducing a bacterial suspension of B. subtilis IB 22 into wheat rhizospheres did not decrease root biomass accumulation (Kudoyarova et al., 2014a). Following rhizobacterial inoculation, cytokinins (predominantly zeatin riboside) appeared in the roots, but later shoot cytokinin accumulation occurred while root cytokinin concentration declined (Arkhipova et al., 2005). Since B. subtilis IB 22 produced ribosylated cytokinin forms that are readily transported out of the roots, root cytokinin accumulation did not occur and the roots grew normally. Thus introducing cytokinin-producing microbes into the rhizosphere may not necessarily inhibit root growth if they are transported to the shoots (Figure 2).
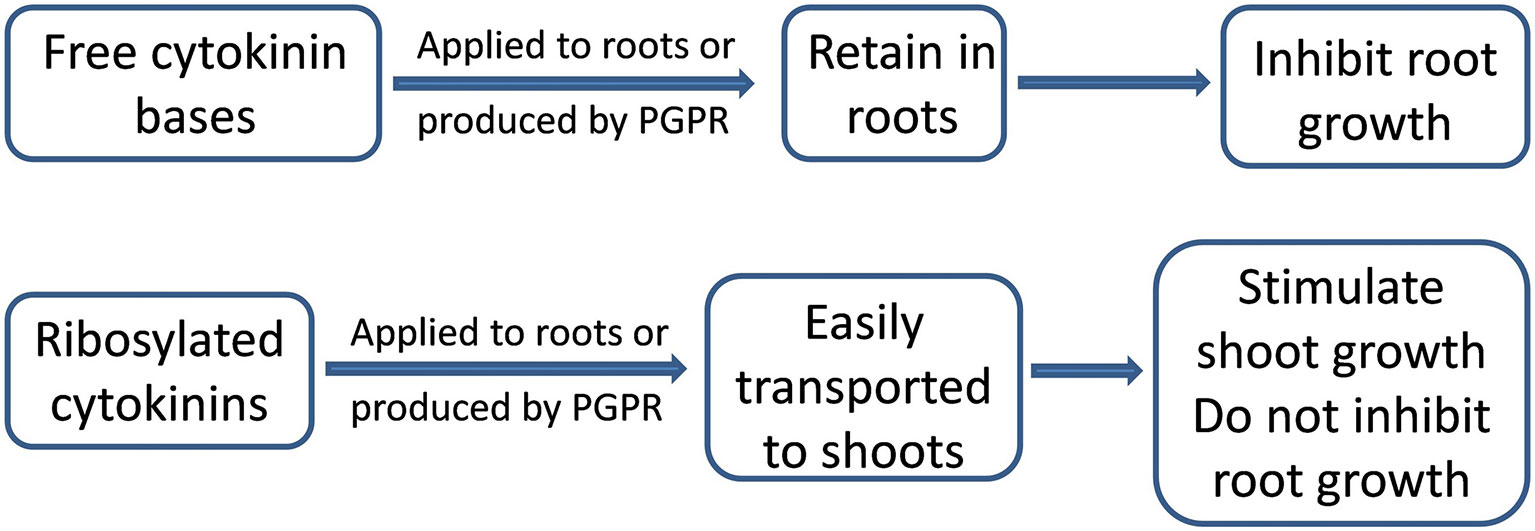
Figure 2 Effects cytokinins on root and shoot growth. Free cytokinin bases retain in the roots and inhibit their growth, while ribosylated cytokinins flow to the shoots and stimulate their growth without inhibiting root growth.
Comparing the root/shoot cytokinin distribution of wheat plants, to which either free cytokinin bases or their ribosides were root-applied, explained how cytokinins were not retained in the roots of inoculated plants (Korobova et al., 2013). Moreover, inhibiting root cell cytokinin uptake by the protonophore, carbonyl cyanide m-chlorophenylhydrazone; increased xylem sap cytokinin concentrations and their flow to the shoot (Kudoyarova et al., 2014b). Thus free bases of cytokinins were actively absorbed by root cells (Korobova et al., 2013). Unlike free zeatin, more cytokinins accumulated in the shoots than in the roots of plants treated with zeatin riboside applied to their roots. The results, showing rapid export of root-derived ribosides of cytokinins to the shoot (Korobova et al., 2013), explain how riboside-producing bacteria do not inhibit root growth, but instead stimulate leaf growth (Arkhipova et al., 2005).
Although cytokinin-producing bacteria may limit root proliferation thereby minimizing salt uptake, the role of bacterial cytokinins in salt stress tolerance is largely unknown as there have been few studies (Ilangumaran and Smith, 2017). Nevertheless, whether rhizobacterial cytokinin production influences plant drought response was evaluated, since increased leaf area and greater stomatal opening mediated by cytokinins could accelerate soil moisture depletion (Davies et al., 2005). Inoculating well-watered plants with different cytokinin producing B. subtilis strains increased shoot ABA concentrations of lettuce (Arkhipova et al., 2007) and Platycladus orientalis (Liu et al., 2013) by 2.1-fold and 1.8-fold respectively. Shoot total cytokinin concentrations were increased by 1.8-fold and 2-fold respectively. With similar fold-changes in these two phytohormones having antagonistic effects on stomatal opening (Dodd, 2003), stomatal conductance of well-watered, inoculated plants was similar or slightly (15%) increased compared to uninoculated plants. Since inoculation increased shoot biomass, these plants were exposed to more rapid soil drying (due to greater transpiration by larger leaves) after withholding water, then once a threshold soil water content was achieved, it was maintained via daily, suboptimal irrigation. Inoculation alleviated the impacts of soil drying on shoot total cytokinin concentrations in both studies, but eliminated (Arkhipova et al., 2007) or magnified (Liu et al., 2013) the effects of soil water deficit on shoot ABA accumulation. Despite these contrasting effects on shoot ABA accumulation, inoculation generally had no effect on soil-drying induced stomatal closure. Nevertheless, both studies demonstrated that inoculation with cytokinin-producing bacteria enhanced plant growth in drying soil, even if the relative effects of changes in shoot ABA and cytokinin concentrations were not explored.
Both salinity (Albacete et al., 2008) and drought (Kudoyarova et al., 2007) decreased foliar cytokinin concentrations, which may inhibit leaf growth. Introducing cytokinin producing bacteria of B. subtilis IB 22 strain into the rhizosphere of lettuce plants increased cytokinin contents (and leaf area) of both well-watered and droughted plants (Arkhipova et al., 2007). Although leaf growth inhibition can protect plants against terminal drought stress (Avramova et al., 2015) by conserving water for use during reproductive development, it almost inevitably decreases crop yield under more moderate conditions. Moreover, leaf growth inhibition may delay canopy closure which limits direct evaporation from moistened soil (Tardieu, 2005). Consequently, accelerated leaf growth by microbial cytokinins may be advantageous in dryland agriculture if the soil is rapidly covered following planting to minimize evaporation from the soil. Under conditions of moderate drought, a pre-sowing treatment of wheat seeds with the cytokinin-producing B. subtilis IB 22 promoted early canopy closure and increased yield by 40% (Wilkinson et al., 2012). This effect of B. subtilis IB 22 on wheat yield was repeated in several (dry) years and comparable to the effects of auxin producing bacteria (Arkhipova et al., 2019). Thus microbial cytokinin production may provide agronomically useful seed treatments under conditions of moderate drought.
Alfalfa tolerance to severe drought stress (watering ceased for 2 weeks until non-inoculated plants died) was increased by inoculating plants with engineered Sinorhizobium strains overproducing cytokinins due to expression of the Agrobacterium ipt gene under the control of different promoters (Xu et al., 2012). Most of the alfalfa plants inoculated with engineered strains survived under conditions of severe stress, which was attributed to increased expression of antioxidant enzymes and decreased level of reactive oxygen in inoculated stressed plants. These findings suggest that engineered Sinorhizobium strains synthesizing more cytokinin could improve the tolerance of alfalfa to severe drought stress.
Thus cytokinins produced by PGPR are likely to promote plant growth, thereby enhancing productivity either under normal or stress conditions. The potential of cytokinins to augment stomatal opening is counteracted by plant ABA accumulation to prevent excessive water losses, while cytokinins-induced increase in leaf size accelerates canopy closure to prevent evaporation of water from the soil. Nevertheless, under severe drought the capacity of cytokinins to inhibit root elongation (as with auxins) may limit water extraction from deep soil layers. Still, the inhibitory action of bacterial cytokinins on the root growth may be prevented, when they are produced in ribosylated forms that are readily exported to the shoots and not retained in the roots.
The Role of ABA in Plant/Bacteria Interactions
Changes in plant ABA status may also be important in mediating plant/microbe interactions, by antagonizing effects of microbial cytokinin production on stomatal conductance as discussed above (Arkhipova et al., 2007; Liu et al., 2013) and also having direct effects. Moreover, plant ABA status can mediate the outcome of interactions with PGPR, as when B. megaterium inoculation stimulated growth of wild type tomato (S. lycopersicum), but inhibited shoot biomass of the ABA-deficient tomato mutants flacca and sitiens (Porcel et al., 2014). In the absence of microbial effects on stomatal conductance (and presumably photosynthesis) in all genotypes, changes in growth were attributed to microbial stimulation of shoot ethylene production. Microbial inoculation increased ethylene production of WT and flacca plants by 2.2-fold and 4.1-fold respectively, with the excessive ethylene accumulation triggered by the PGPR in flacca associated with growth inhibition rather than growth promotion. Interestingly, uninoculated flacca plants produce at least twice as much ethylene as WT plants (Sharp et al., 2000; Dodd et al., 2009), further suggesting the importance of ABA/ethylene interactions in growth regulation. In contrast, the ABA-metabolizing Rhodococcus sp. P1Y and Novosphingobium sp. P6W had similar effects on root and shoot biomass of ABA-deficient mutant flacca and WT plants grown in vitro, probably since ethylene was not involved in regulating these plant/microbe interactions (Belimov et al., 2014). Based on these studies with ABA-deficient mutants, it is difficult to be certain whether ABA status is the primary determinant of these plant/microbe interactions.
Moreover, microorganisms can synthesize ABA (Cohen et al., 2009; Cohen et al., 2015; Shahzad et al., 2017) that should alter ABA-mediated processes due to plant uptake of microbially produced hormone. Otherwise microbes can influence expression of plant genes (e.g. NCED3, ABA2 and ABA3) responsible for ABA biosynthesis in planta (Vargas et al., 2014). Thus inoculation of sugarcane (Saccharum officinarum) roots with Gluconacetobacter diazotrophicus had different transcriptome profiles (for genes responsible for ABA biosynthesis and signaling) from uninoculated plants, with G. diazotrophicus activating ABA-dependent signaling genes in the shoots, which may confer drought resistance (Vargas et al., 2014). Nevertheless, it can be difficult to ascribe changes in plant ABA status to direct microbial ABA biosynthesis or to changes in ABA synthesis in planta due to changes in plant water status resulting from plant growth promotion. In some reports, ABA concentration in bacterial culture media was related to actual changes in ABA content in planta (Yasmin et al., 2017). Bacillus pumilus produced five times more ABA than Pseudomonas sp. in vitro, thereby greatly increasing relative water content and osmotic potential of inoculated plants. Microbial ABA production by Azospirillum lipoferum increased ABA concentrations in planta (Cohen et al., 2009; Cohen et al., 2015), preventing a drought-induced decline in relative water content of inoculated maize (Z. mays) seedlings. Although ABA accumulation was related to some physiological effects associated with PGPR inoculation (see below), a causal relation between them was not frequently supported experimentally.
ABA protects plants from dehydration by stimulating expression of dehydrins (Shakirova et al., 2016). In accordance with this effect of ABA, genes involved in the synthesis of dehydrins [late embryogenesis abundant (LEA) proteins] were upregulated in maize plants inoculated with P. putida strain FBKV2 (SkZ et al., 2018). FBKV2 inoculation enhanced SnRK2 family proteins, which facilitated transcription of ABA-responsive genes, thereby conferring drought tolerance. Arabidopsis plants inoculated with ABA producing A. brasilense showed less lipid damage quantified by malondialdehyde levels. Although this effect was attributed to ABA-induced antioxidative defense mechanisms (e.g. increased content of phenolic compounds that scavenge free radicals), a simpler explanation may be that ABA-induced stomatal closure improved leaf water relations and thus minimized oxidative stress (Cohen et al., 2015). Thus it can be difficult to separate direct molecular effects of microbial ABA production from indirect effects of enhancing shoot water status.
Furthermore, soil drying can increase ABA concentrations in the soil solution (Hartung et al., 2002) and stimulate root-to-shoot signaling of ABA prior to any changes in shoot water status (Davies et al., 2005). Bacteria may alter this signaling, by synthesizing ABA under stress conditions (e.g. in some strains of B. pumilus) (Forchetti et al., 2007) or metabolizing ABA present in the soil solution, thereby decreasing ABA concentrations in planta (Belimov et al., 2014). Despite being unable to metabolize ABA in vitro, V. paradoxus 5C-2 decreased root ABA concentrations and accumulation by 40%–60% in pea, which was related to bacterial ACC deaminase (Jiang et al., 2012). Since an important function of ABA is to restrict ethylene production in planta (Sharp et al., 2000), microbial limitation of plant ethylene production seems to diminish the need for high ABA levels.
Since plant growth promotion by rhizobacteria occurred with both increased [e.g. Bacillus licheniformis Rt4M10 and P. fluorescens Rt6M10 (Salomon et al., 2014)] and decreased ABA concentration [B. subtilis GB03 (Zhang et al., 2008)], it is difficult to conclude that ABA was involved in growth regulation. The growth response to the microbial induced changes in ABA content may vary according to tissue water status: with increased ABA concentration maintaining root growth under low water potential, but decreased ABA concentrations maintaining root growth under high water potential (Sharp et al., 1994). Nevertheless, any bacterial-mediated changes in ABA level may affect stomatal conductance. Stomatal closure in inoculated plants maintained leaf water relations under soil water deficit (Salomon et al., 2014). In contrast, stomatal conductance increased under optimal conditions due to decreased ABA content in planta (due to emission of volatile compounds by B. subtilis GB03), thereby maintaining photosynthesis (Zhang et al., 2008). This contradiction (beneficial effect of both increased and decreased stomatal conductance induced by PGPR) is because stomatal closure both restricts water loss while inhibiting photosynthesis (Figure 3). The agronomic outcome of this trade-off between economizing water use and utilizing CO2 depends on soil water availability (Ewers, 2013).
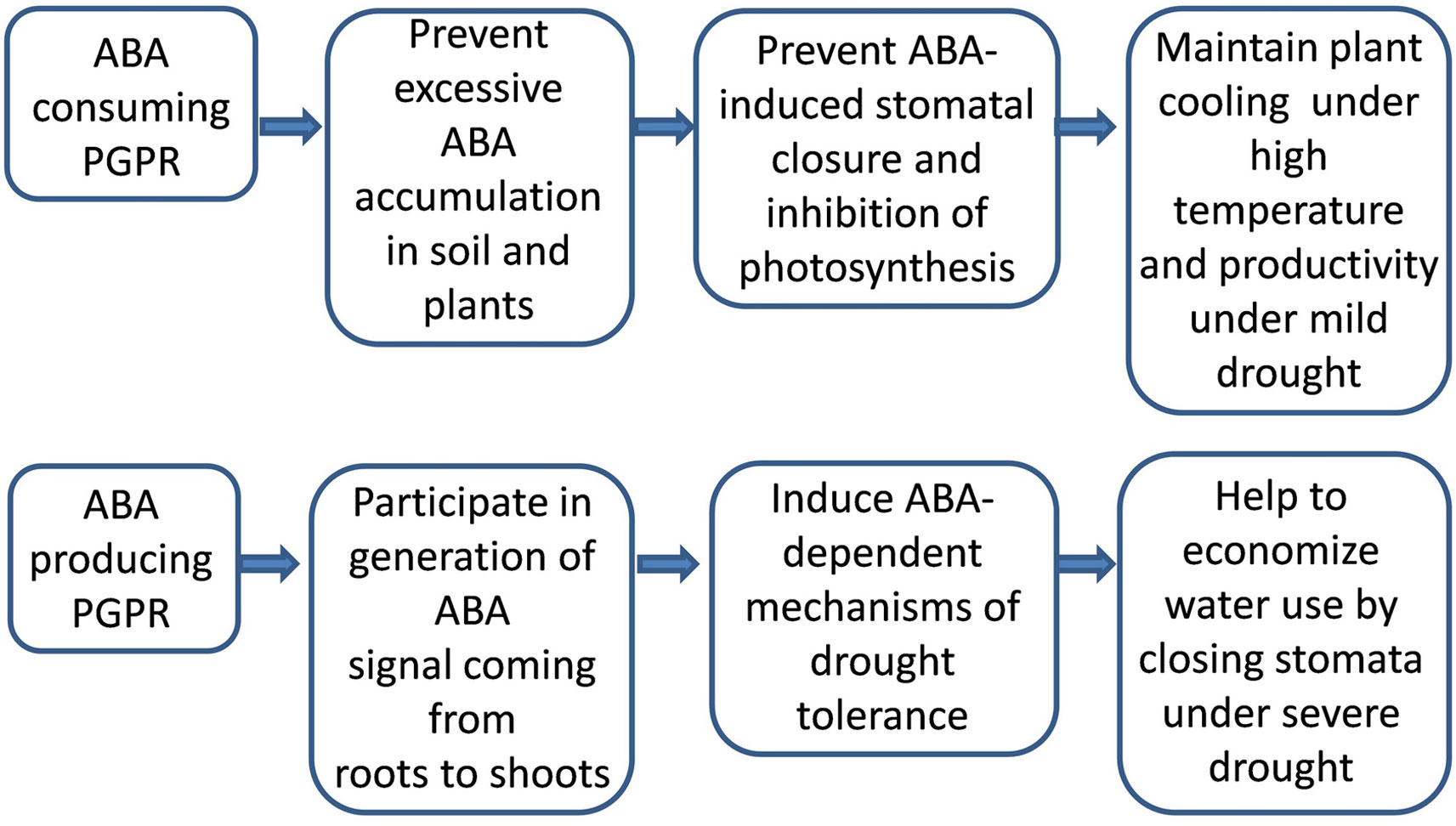
Figure 3 Outcome of action of PGPRs either producing or consuming abscisic acid (ABA) depends on conditions of plant growth.
ABA not only closes stomata, but also can also increase hydraulic conductance of plant tissues, which can be explained by ABA’s effect on the activity of water channels aquaporins (Kaldenhoff et al., 2008). Although inoculation with B. megaterium activated expression of the genes coding for water channels (Marulanda et al., 2010), it is uncertain whether this change was ABA-mediated as ABA levels were not quantified. Indeed, B. megaterium decreased root ABA concentrations of the flacca mutant (Porcel et al., 2014). Nevertheless, immunohistochemistry of ABA and aquaporin contents in root epidermal cells showed that ABA application to nutrient solution increased the level of HvPIP2;2 and HvPIP2;1 aquaporins in these cells, coincident with increased ABA concentrations (Sharipova et al., 2016). Thus bacterial ABA production (known to increase under osmotic stress—Forchetti et al., 2007) may theoretically mediate root aquaporin activity, although whether the PGPR enhance root ABA concentrations sufficiently to allow this has not been assessed.
Conclusion
To conclude, the benefits of applying rhizosphere bacteria capable of synthesizing or consuming plant hormones will depend on soil conditions. Bacterial auxin synthesis may stimulate root growth and increase plant productivity under favorable conditions or mild stress. Under more severe stress, this trait should be combined with ACC deaminase activity in the same strain or another bacterial species in the consortium. To prevent inhibitory effects of bacterial cytokinins on root growth, it is important that microbes produce cytokinins in ribosylated forms that are readily exported to the shoot to stimulate cell division and expansion. Bacterial mediation of plant ABA levels merits further study, but decreasing ABA content seems beneficial under moderate stress, while ABA-producing bacteria are beneficial under severe stress. Inter-cultivar variation in intrinsic or stress-induced hormone status (Quarrie, 1981; Sanguineti et al., 1999; Valluru et al., 2016) or hormone sensitivity (Ibort et al., 2017) should be considered when choosing optimal traits for bacterial preparations. There is still limited knowledge about how plants integrate their intracellular signaling in response to multiple phytohormones produced by PGPR, and how these interact with endogenous plant pathways. Such information seems necessary to predict the outcomes of PGPR inoculation, especially when such organisms produce multiple phytohormones. Moreover, the role of hormones in mediating plant/microbe interactions will be important in determining the success (or otherwise) of phytoremediation of salt-affected or oil contaminated soils. However, these interactions require further study to determine whether such biotechnological approaches can deliver predictable outcomes.
Author Contributions
All authors listed have made a substantial, direct, and intellectual contribution to the work, and approved it for publication. GK – arranged the plan of the review, was responsible for sections concerning hormonal aspects of the review, collected and integrated sections written by other authors, TA was responsible for the material concerning salt stress, TK was responsible for microbial aspects of the review, MB added information about remediation of soils contaminated with iol, OL integrated microbial components of the review, ID was responsible for physiological aspects of water relations and drought resistance and checked English of the text.
Funding
The work was granted by Russian Foundation for Basic Research N 18-29-05025/18 and N 18-04-00577/18.
Conflict of Interest
The authors declare that the research was conducted in the absence of any commercial or financial relationships that could be construed as a potential conflict of interest.
References
Achard, P., Cheng, H., De Grauwe, L., Decat, J., Schoutteten, H., Moritz, T., et al. (2006). Integration of plant responses to environmentally activated phytohormonal signals. Science 311, 91–94. doi: 10.1126/science.1118642
Adesemoye, A., Torbert, H., Kloepper, J. (2009). Plant growth promoting rhizobacteria allows reduced application rates of chemical fertilizers. Microb. Ecol. 58, 921–929. doi: 10.1007/s00248-009-9531-y
Albacete, A., Ghanem, M. E., Martínez-Andújar, C., Acosta, M., Sánchez-Bravo, J., et al. (2008). Hormonal changes in relation to biomass partitioning and shoot growth impairment in salinized tomato (Solanum lycopersicum L.) plants. J. Exp. Bot. 59, 4119–4131. doi: 10.1093/jxb/ern251
Ali, B., Sabri, A. N., Ljung, K., Hasnain, S. (2009). Auxin production by plant associated bacteria: impact on endogenous IAA content and growth of Triticum aestivum L. Lett. Appl. Microbiol. 48, 542–547. doi: 10.1111/j.1472-765X.2009.02565.x
Ambreetha, S., Chinnadurai, C., Marimuthu, P., Balachandar, D. (2018). Plant-associated Bacillus modulates the expression of auxin-responsive genes of rice and modifies the root architecture. Rhizosphere 5, 57–66. doi: 10.1016/j.rhisph.2017.12.001
Arkhipova, T. N., Veselov, S. U., Melentiev, A. I., Martynenko, E. V., Kudoyarova, G. R. (2005). Ability of bacterium Bacillus subtilis to produce cytokinins and to influence the growth and endogenous hormone content of lettuce plants. Plant Soil 272, 201–209. doi: 10.1007/s11104-004-5047-x
Arkhipova, T. N., Prinsen, E., Veselov, S. U., Martinenko, E. V., Melentiev, A. I., Kudoyarova, G. R. (2007). Cytokinin producing bacteria enhance plant growth in drying soil. Plant Soil 292, 305–315. doi: 10.1007/s11104-007-9233-5
Arkhipova, T. N., Galimsyanova, N. F., Yu, K. L., Vysotskaya, L. B., Sidorova, L. V., Gabbasova, I. M., et al. (2019). Effect of seed bacterization withplant growth-promoting bacteria on wheat productivity and phosphorus mobility in the rhizospere. Plant Soil Environ. 65, I.6, 313–319. doi: 10.17221/752/2018-PSE
Arteca, R. N., Arteca, J. M. (2008). Effects of brassinosteroid, auxin, and cytokinin on ethylene production in Arabidopsis thaliana plants. J. Exp. Bot. 59, 3019–3026. doi: 10.1093/jxb/ern159
Asari, S., Tarkowská, D., Rolčík, J., Novák, O., Palmero, D. V., Bejai, S., et al. (2017). Analysis of plant growth-promoting properties of Bacillus amyloliquefaciens UCMB5113 using Arabidopsis thaliana as host plant. Planta. 245, 15–30. doi: 10.1007/s00425-016-2580-9
Avramova, V., AbdElgawad, H., Zhang, Z., Fotschki, B., Casadevall, R., Vergauwen, L., et al. (2015). Drought Induces Distinct Growth Response, Protection, and Recovery Mechanisms in the Maize Leaf Growth Zone. Plant Physiol. 169, 1382–1396. doi: 10.1104/pp.15.00276
Backer, R., Rokem, J. S., Ilangumaran, G., Lamont, J., Praslickova, D., Ricci, E., et al. (2018). Plant growth-promoting rhizobacteria: context, mechanisms of action, and roadmap to commercialization of biostimulants for sustainable agriculture. Front. Plant Sci. 9, 1473. doi: 10.3389/fpls.2018.01473
Bechtold, U., Field, B. (2018). Molecular mechanisms controlling plant growth during abiotic stress. J. Exp. Bot. 69, 2753–2758. doi: 10.1093/jxb/ery157
Belimov, A., Dodd, I., Safronova, V., Davies, W. (2009a). ACC deaminase-containing rhizobacteria improve vegetative development and yield of potato plants grown under water-limited conditions. Annals. Appl. Biol. 98, 163–169. doi: 10.1111/aab.12203
Belimov, A., Dodd, I. C., Hontzeas, N., Theobald, J. C., Safronova, V. I., Davies, W. J. (2009b). Rhizosphere bacteria containing 1-aminocyclopropane-1-carboxylate deaminase increase yield of plants grown in drying soil via both local and systemic hormone signaling. New Phytol. 181, 413–423. doi: 10.1111/j.1469-8137.2008.02657.x
Belimov, A. A., Dodd, I. C., Safronova, V. I., Dumova, V. A., Shaposhnikov, A. I., Ladatko, A. G., et al. (2014). Abscisic acid metabolizing rhizobacteria decrease ABA concentrations in planta and alter plant growth. Plant Physiol. Biochem. 74, 84–91. doi: 10.1016/j.plaphy.2013.10.032
Belimov, A. A., Dodd, I. C., Safronova, V. I., Shaposhnikov, A. I., Azarova, T. S., Makarova, N. M., et al. (2015). Rhizobacteria that produce auxins and contain 1-amino-cyclopropane-1-carboxylic acid deaminase decrease amino acid concentrations in the rhizosphere and improve growth and yield of well-atered and water-limited potato (Solanum tuberosum). Ann. Appl Biol. 167, 11–25. doi: 10.1111/aab.12203
Benson, A., Ram, G., John, A., Joe, M. M. (2017). Inoculation of 1-aminocyclopropane-1-carboxylate deaminase-producing bacteria along with biosurfactant application enhances the phytoremediation efficiency of Medicago sativa in hydrocarbon-contaminated soils. Bioremediat. J. 21, 20–29. doi: 10.1080/10889868.2017.1282934
Blinkov, E. A., Selitskaya, O. V., Tsavkelova, E. A. (2014). Auxin production by the Klebsiella planticola strain TSKhA-91 and its effect on development of cucumber (Cucumis sativus L.) seeds. Microbiology 83, 531–538. doi: 10.1134/S0026261714050063
Bottini, R., Cassán, F., Piccoli, P. (2004). Gibberellin production by bacteria and its involvement in plant growth promotion and yield increase. Appl. Microbiol. Biotechnol. 65, 497–503. doi: 10.1007/s00253-004-1696-1
Casimiro, I., Marchant, A., Bhalerao, R. P., Beeckman, T., Dhooge, S., Swarup, R., et al. (2001). Auxin transport promotes Arabidopsis lateral root initiation. Plant Cell. 13, 843–852. doi: 10.1105/tpc.13.4.843
Chapin, F.S., III. (1991). Integrated responses of plants to stress: a centralized system of physiological responses. BioScience 41, 29–36. doi: 10.2307/1311538
Chaves, M. M., Maroco, J. P., Pereira, J. S. (2003). Understanding plant responses to drought – from genes to the whole plant. Funct. Plant Biol. 30, 239–264. doi: 10.1071/FP02076
Chen, L., Dodd, I. C., Theobald, J. C., Davies, W. J., Belimov, A. A. (2013). The rhizobacterium Variovorax paradoxus 5C-2, containing ACC deaminase, promotes growth and development of Arabidopsis thaliana via an ethylene-dependent pathway. J. Exp. Bot. 64, 1565–1573. doi: 10.1093/jxb/ert031
Cheng, Z., Park, E., Glick, B. R. (2007). 1-Aminocyclopropane-1-carboxylate deaminase from Pseudomonas putida UW4 facilitates the growth of canola in the presence of salt. Can. J. Microbiol. 53, 912–918. doi: 10.1139/W07-050
Chetverikov, S. P., Sharipov, D. A., Yu, K. T., Loginov, O. N. (2017). Degradation of perfluorooctanyl sulfonate by strain Pseudomonas plecoglossicida 2,4-D. Appl. Biochem. Microbiol. 53, 533–538. doi: 10.1134/S0003683817050027
Cohen, A. C., Travaglia, C., Bottini, R., Piccoli, P. (2009). Participation of abscisic acid and gibberellins produced by entophytic Azospirillum in the alleviation of drought effects in maize. Botany 87, 455–462. doi: 10.1139/B09-023
Cohen, A. C., Bottini, R., Pontin, M., Berli, F. J., Moreno, D., Boccanlandro, H., et al. (2015). Azospirillum brasilense ameliorates the response of Arabidopsis thaliana to drought mainly via enhancement of ABA levels. Physiol. Plant. 153, 79–90. doi: 10.1111/ppl.12221
Comas, L. H., Becker, S. R., Cruz, V. M., Byrne, P. F., Dierig, D. A. (2013). Root traits contributing to plant productivity under drought. Front. Plant Sci. 4, 442. doi: 10.3389/fpls.2013.00442
Davies, W. J., Kudoyarova, G., Hartung, W. (2005). Long-distance ABA signaling and its relation to other signaling pathways in the detection of soil drying and the mediation of the plant’s response to drought. J. Plant Growth Regul. 24, 285–295. doi: 10.1007/s00344-005-0103-1
Den Herder, G., Van Isterdael, G., Beeckman, T., De Smet, I. (2010). The roots of a new green revolution. Trends Plant Sci. 15, 600–607. doi: 10.1016/j.tplants.2010.08.009
Dobbelaere, S., Croonenborghs, A., Thys, A., Vande, B. A., Vanderleyden, J. (1999). Phytostimulatory effect of Azospirillum brasilense wild type and mutant strains altered IAA production on wheat. Plant Soil 212, 153–162. doi: 10.1023/A:1004658000815
Dodd, I. C. (2003). Hormonal interactions and stomatal responses. J. Plant Growth Regul. 22, 32–46. doi: 10.1007/s00344-003-0023-x
Dodd, I. C., Theobald, J. C., Richer, S. K., Davies, W. J. (2009). Partial phenotypic reversion of ABA-deficient flacca tomato (Solanum lycopersicum) scions by a wild-type rootstock: normalising shoot ethylene relations promotes leaf area but does not diminish whole plant transpiration rate. J. Exp. Bot. 60, 4029–4039. doi: 10.1093/jxb/erp236
Dodd, I. C., Zinovkina, N. Y., Safronova, V. I., Belimov, A. A. (2010). Rhizobacterial mediation of plant hormone status. Ann. Appl. Biol. 157, 361–379. doi: 10.1111/j.1744-7348.2010.00439.x
Ehdaie, B., Whitkus, R. W., Waines, J. G. (2003). Root biomass, water-use efficiency and performance of wheat-rye translocations of chromosomes 1 and 2 in spring wheat Pavon. Crop Sci. 43, 710–717. doi: 10.2135/cropsci2003.0710
Ewers, B. E. (2013). Understanding stomatal conductance responses to long-term environmental changes: a Bayesian framework that combines patterns and processes. Tree Physiol. 33, 119–122. doi: 10.1093/treephys/tpt008
Forchetti, G., Masciarelli, O., Alemano, S., Alvarez, D., Abdala, G. (2007). Endophytic bacteria in sunflower (Helianthus annuus L.): isolation, characterization, and production of jasmonates and abscisic acid in culture medium. Appl. Microbiol. Biotechnol. 76, 1145–1152. doi: 10.1007/s00253-007-1077-7
Gerhardt, K. E., Huang, X.-D., Glick, B. R., Greenberg, B. M. (2009). Phytoremediation and rhizoremediation of organic soil contaminants: Potential and challenges. Plant Sci. 176, 20–30. doi: 10.1016/j.plantsci.2008.09.014
German, M. A., Burdman, S., Okon, Y., Kigel, J. (2000). Effects of Azospirillum brasilense on root morphology of common bean (Phaseolus vulgaris L.) under different water regimes. Biol. Fertil. Soils. 32, 259–264. doi: 10.1007/s003740000245
Glick, B. R., Penrose, D. M., Li, J. (1998). A model for the lowering of plant ethylene concentrations by plant growth-promoting bacteria. J. Theor. Biol. 190, 63–68. doi: 10.1006/jtbi.1997.0532
Glick, B. R. (2003). Phytoremediation: synergistic use of plants and bacteria to clean up the environment. Biotechnol. Adv. 21, 383–393. doi: 10.1016/S0734-9750(03)00055-7
Glick, B. R. (2006). “Modifying a plant’s response to stress by decreasing ethylene production,” in Phytoremediation and Rhizoremediation. Eds. Mackova, M., Dowling, D., Macek, T. (Dordrecht: Springer), 227–236.
Glick, B. R. (2014). Bacteria with ACC deaminase can promote plant growth and help to feed the world. Microbiol. Res. 169, 30–39. doi: 10.1016/j.micres.2013.09.009
Greenberg, B. M., Huang, X.-D., Gurska, Y., Gerhardt, K. E., Lampi, M. A., Khalid, A., et al. (2006). “Development and successful field tests of a multi-process phytoremediation system for decontamination of persistent petroleum and organic contaminants in soils,” in Reclamation and Remediation: Policy and Practice. Eds. Tisch, B., Zimmerman, K., White, P., Beckett, P., Guenther, L., Macleod, A. (Calgary: Canadian Land Reclamation Association (CLRA)), 124–133.
Großkinsky, D. K., Tafner, R., Moreno, M. V., Stenglein, S. A., García de Salamone, I. E., Nelson, et al. (2016). Cytokinin production by Pseudomonas fluorescens G20-18 determines biocontrol activity against Pseudomonas syringae in Arabidopsis. Sci. Rep. 6, 23310. doi: 10.1038/srep23310
Gunes, A., Karagoz, K., Turan, M., Kotan, R., Yildirim, E., Ramazan, R., et al. (2015). Fertilizer efficiency of some plant growth promoting rhizobacteria for plant growth. Research Journal of Soil Biology. 7, 28–45. doi: 10.3923/rjsb.2015.28.45
Habib, S. H., Kausar, H., Saud, H. M. (2016). Plant growth-promoting rhizobacteria enhance salinity stress tolerance in okra through ROS-scavenging enzymes. Biomed. Res. Int. 2016, 6284547. doi: 10.1155/2016/6284547
Hall, J. A., Peirson, D., Ghosh, S., Glick, B. R. (1996). Root elongation in various agronomic crops by the plant growth promoting rhizobacterium Pseudomonas putida GR12-2. Isr. J. Plant Sci. 44, 37–42. doi: 10.1080/07929978.1996.10676631
Hassan, T. U., Bano, A. (2019). Construction of IAA-deficient mutants of Pseudomonas moraviensis and their comparative effects with wild type strains as bio-inoculant on wheat in saline sodic soil. Geomicrobiol. J. 36, 376–384. doi: 10.1080/01490451.2018.1562498
Hartung, W., Sauter, A., Hose, E. (2002). Abscisic acid in the xylem: where does it come from, where does it go to? J. Exp. Bot. 53, 27–32. doi: 10.1093/jexbot/53.366.27
Hodge, A. (2010). “Roots: the acquisition of water and nutrients from the heterogeneous soil environment,” in Progress in Botany, vol. 71. Eds. Lüttge, U., Beyschlag, W., Büdel, B., Francis, D. (Berlin, Heidelberg: Springer), 307–337. doi: 10.1007/978-3-642-02167-1_12
Hong, S. H., Ryu, H., Kim, J., Cho, K. S. (2011). Rhizoremediation of diesel-contaminated soil using the plant growth-promoting rhizobacterium Gordonia sp. S2RP-17. Biodegradation 22, 593–601. doi: 10.1007/s10532-010-9432-2
Huang, X.-D., El-Alawi, Y., Gurska, J., Glick, B. R., Greenberg, B. M. (2005). A multi-process phytoremediation system for decontamination of persistent total petroleum hydrocarbons (TPHs) from soils. Microchem. J. 81, 139–147. doi: 10.1016/j.microc.2005.01.009
Ibort, P., Molina, S., Núñez, R., Zamarreño, Á. M., García-Mina, J. M., Ruiz-Lozano, J. M., et al. (2017). Tomato ethylene sensitivity determines interaction with plant growth-promoting bacteria. Ann. Bot. 120, 101–122. doi: 10.1093/aob/mcx052
Ilangumaran, G., Smith, D. L. (2017). Plant growth promoting rhizobacteria in amelioration of salinity stress: a systems biology perspective. Front. Plant Sci. 8, 1768. doi: 10.3389/fpls.2017.01768
Jatan, R., Chauhan, P. S., Lata, C. (2019). Pseudomonas putida modulates the expression of miRNAs and their target genes in response to drought and salt stresses in chickpea (Cicer arietinum L.). Genomics. 111, 509–519. doi: 10.1016/j.ygeno.2018.01.007
Jiang, F., Chen, L., Belimov, A. A., Shaposhnikov, A. I., Gong, F., Meng, X., et al. (2012). Multiple impacts of the plant growth-promoting rhizobacterium Variovorax paradoxus 5C-2 on nutrient and ABA relations of Pisum sativum. J. Exp. Bot. 63, 6421–6430. doi: 10.1093/jxb/ers301
Kaldenhoff, R., Ribas-Carbo, M., Sans, J. F., Lovisolo, C., Heckwolf, M., Uehlein, N. (2008). Aquaporins and plant water balance. Plant Cell Environ. 31, 658–666. doi: 10.1111/j.1365-3040.2008.01792.x
Korobova, A. V., Vasinskaya, A. N., Akhiyarova, G. R., Kudoyarova, G. R., Veselov, S. Y., Hartung, W. (2013). Dependence of cytokinin distribution in plants on their physical and chemical properties and transpiration rate. Russ. J. Plant Physiol. 60, 193–199. doi: 10.1134/S1021443713020131
Korshunova, T.Yu., Mukhamatdyarova, S. R., Loginov, O. N. (2017). Molecular-genetic and chemotaxonomic identification of the bacterium of the genus Оhrobactrum possessing oil-oxidizing and nitrogen-fixing activity. Biol. Bull. 44, 493–500. doi: 10.1134/S1062359017050090
Kudoyarova, G. R., Vysotskaya, L. B., Cherkozyanova, A., Dodd, I. C. (2007). Effect of partial rootzone drying on the concentration of zeatintype cytokinins in tomato (Solanum lycopersicum L.) xylem sap and leaves. J. Exp. Bot. 58, 161–168. doi: 10.1093/jxb/erl116
Kudoyarova, G. R., Melentiev, A. I., Martynenko, E. V., Arkhipova, T. N., Shendel, G. V., Yu, K. L., et al. (2014a). Cytokinin producing bacteria stimulate amino acid deposition by wheat roots. Plant Physiol. Biochem. 83, 285–291. doi: 10.1016/j.plaphy.2014.08.015
Kudoyarova, G. R., Korobova, A. V., Akhiyarova, G. R., Arkhipova, T. N., Zaytsev, D. Y., Prinsen, E., et al. (2014b). Accumulation of cytokinins in roots and their export to the shoots of durum wheat plants treated with the protonophore carbonyl cyanide m-chlorophenylhydrazone (CCCP). J. Exp. Bot. 65, 2287–2294. doi: 10.1093/jxb/eru113
Kudoyarova, G. R., Arkhipova, T. N., Melent’ev, A. I (2015a). “Role of bacterial phytohormones in plant growth regulation and their development,” in Bacterial Metabolites in Sustainable Agroecosystem. Sustainable Development and Biodiversity, vol. 12. Ed. Maheshwari, D. K. (Cham: Springer), 69–86. doi: 10.1007/978-3-319-24654-3_4
Kudoyarova, G. R., Dodd, I. C., Veselov, D. S., Rothwell, S. A., Veselov, S. Y. (2015b). Common and specific responses to availability of mineral nutrients and water. J. Exp. Bot. 66, 2133–2144. doi: 10.1093/jxb/erv017
Kudoyarova, G. R., Vysotskaya, L. B., Arkhipova, T. N., Yu, K. L., Galimsyanova, N. F., Sidorova, L. V., et al. (2017). Effect of auxin producing and phosphate solubilizing bacteria on mobility of soil phosphorus, growth rate, and P acquisition by wheat plants. Acta Physiol. Plant. 39, 253. doi: 10.1007/s11738-017-2556-9
Kuzmina, L. Y., Melentev, A. I. (2003). The effect of seed bacterization by Bacillus Cohn bacteria on their colonization of the spring wheat rhizosphere. Microbiology 72, 230–235. doi: 10.1023/A:1023280300908
Lara-Chavez, A., Lowman, S., Kim, S., Tang, Y., Zhang, J., Udvardi, M., et al. (2015). Global gene expression profiling of two switchgrass cultivars following inoculation with Burkholderia phytofirmans strain PsJN. J. Exp. Bot. 66, 4337–4350. doi: 10.1093/jxb/erv096
Laskowski, M. (2013). Lateral root initiation is a probabilistic event whose frequency is set by fluctuating levels of auxin response. J. Exp. Bot. 64, 2609–2617. doi: 10.1093/jxb/ert155
Li, J., Ovakim, D. H., Charles, T. C., Glick, B. R. (2000). An ACC deaminase minus mutant of Enterobacter cloacae UW4 no longer promotes root elongation. Curr. Microbiol. 41, 101–105. doi: 10.1007/s002840010101
Li, S. W., Leng, Y., Feng, L., Zeng, X. Y. (2014). Involvement of abscisic acid in regulating antioxidative defense systems and IAA-oxidase activity and improving adventitious rooting in mung bean [Vigna radiata (L.) Wilczek] seedlings under cadmium stress. Environ. Sci. Pollut. Res. Int. 21, 525–537. doi: 10.1007/s11356-013-1942-0
Liu, F., Xing, S., Ma, H., Du, Z., Ma, B. (2013). Cytokinin-producing, plant growth-promoting rhizobacteria that confer resistance to drought stress in Platycladus orientalis container seedlings. Appl. Microbiol. Biotechnol. 97, 9155–9164. doi: 10.1007/s00253-013-5193-2
Loper, J. E., Schroth, M. N. (1986). Influence of bacterial sources of indole-3-acetic acid on root elongation of sugar beet. Phytopathology. 76, 386–389. doi: 10.1094/Phyto-76-386
Marulanda, A., Azcón, R., Chaumont, F., Ruiz-Lozano, J. M., Aroca, R. (2010). Regulation of plasma membrane aquaporins by inoculation with a Bacillus megaterium strain in maize (Zea mays L.) plants under unstressed and salt-stressed conditions. Planta. 232, 533–543. doi: 10.1007/s00425-010-1196-8
Mayak, S., Tirosh, T., Glick, B. R. (2004). Plant growthpromoting bacteria that confer resistance to water stress in tomato and pepper. Plant Sci. 166, 525–530. doi: 10.1016/j.plantsci.2003.10.025
Morgan, P. W., Hall, W. C. (1962). Effect of 2,4-dichlorophenoxyacetic acid on the production of ethylene by cotton and grain sorghum. Physiol. Plant. 15, 420–427. doi: 10.1111/j.1399-3054.1962.tb08045.x
Niu, X., Song, L., Xiao, Y., Weide Ge, W. (2017). Drought-tolerant plant growth-promoting rhizobacteria associated with foxtail millet in a semi-arid agroecosystem and their potential in alleviating drought stress. Front. Microbiol. 8, 2580. doi: 10.3389/fmicb.2017.02580
Ortíz-Castro, R., Valencia-Cantero, E., López-Bucio, J. (2008). Plant growth promotion by Bacillus megaterium involves cytokinin signaling. Plant Signal Behav. 3, 263–265. doi: 10.4161/psb.3.4.5204
Patel, T., Saraf, M. (2017). Biosynthesis of phytohormones from novel rhizobacterial isolates and their in vitro plant growth-promoting efficacy. J. Plant Interact. 12, 480–487. doi: 10.1080/17429145.2017.1392625
Patten, C. L., Glick, B. R. (2002). Role of Pseudomonas putida indoleacetic acid in development of the host plant root system. Appl. Environ. Microbiol. 68, 3795–3801. doi: 10.1128/AEM.68.8.3795-3801.2002
Paul, D., Lade, H. (2014). Plant-growth-promoting rhizobacteria to improve crop growth in saline soils: a review. Agron. Sustain. Dev. 34, 737–752. doi: 10.1007/s13593-014-0233-6
Porcel, R., Zamarreno, A. M., Garcia-Mina, J. M., Aroca, R. (2014). Involvement of plant endogenous ABA in Bacillus megaterium PGPR activity in tomato plants. BMC Plant Biol. 14, 36. doi: 10.1186/1471–2229–14–36
Quarrie, S. A. (1981). Genetic variability and heritability of droughtinduced abscisic acid accumulation in spring wheat. Plant Cell Environ. 4, 147–151. doi: 10.1111/j.1365-3040.1981.tb01036.x
Raheem, A., Shaposhnikov, A., Belimov, A. A., Dodd, I. C., Ali, B. (2017). Auxin production by rhizobacteria was associated with improved yield of wheat (Triticum aestivum L.) under drought stress. Arch. Agron. Soil Sci. 64, 574–587. doi: 10.1080/03650340.2017.1362105
Reed, R. C., Brady, S. R., Muday, G. K. (1998). Inhibition of auxin movement from the shoot into the root inhibits lateral root development in Arabidopsis. Plant Physiol. 118, 1369–1378. doi: 10.1104/pp.118.4.1369
Rubin, R. L., Van Groenigen, K. J., Hungate, B. A. (2017). Plant growth promoting rhizobacteria are more effective under drought: a meta-analysis. Plant Soil. 416, 309–323. doi: 10.1007/s11104-017-3199-8
Safronova, V. I., Stepanok, V. V., Engqvist, G. L., Alekseyev, Y. V., Belimov, A. A. (2006). Root-associated bacteria containing 1-aminocyclopropane-1-carboxylate deaminase improve growth and nutrient uptake by pea genotypes cultivated in cadmium supplemented soil. Biol. Fert. Soils. 42, 267–272. doi: 10.1007/s00374-005-0024-y
Salomon, M. V., Bottini, R., de Souza Filho, G. A., Cohen, A. C., Moreno, D., Gil, M., et al. (2014). Bacteria isolated from roots and rhizosphere of Vitis vinifera retard water losses, induce abscisic acid accumulation and synthesis of defense-related terpenes in in vitro cultured grapevine. Physiol. Plant. 151, 359–374. doi: 10.1111/ppl.12117
Sandhya, V., Ali, S. Z., Venkateswarlu, B., Reddy, G., Grover, M. (2010). Effect of osmotic stress on plant growth promoting Pseudomonas spp. Arch. Microbiol. 192, 867–876. doi: 10.1007/s00203-010-0613-5
Sanguineti, M. C., Tuberosa, R., Landi, P., Salvi, S., Maccaferri, M., Casarini, E., et al. (1999). QTL analysis of drought-related traits and grain yield in relation to genetic variation for leaf abscisic acid concentration in field-grown maize. J. Exp. Bot. 50, 1289–1297. doi: 10.1093/jxb/50.337.1289
Seo, P. J., Xiang, F., Qiao, M., Park, J. Y., Lee, Y. N., Kim, S. G., et al. (2009). The MYB96 transcription factor mediates abscisic acid signaling during drought stress response in Arabidopsis. Plant Physiol. 151, 275–289. doi: 10.1104/pp.109.144220
Shahzad, R., Khan, A. L., Bilal, S., Waqas, M., Kanga, S.-M., Lee, I.-J. (2017). Inoculation of abscisic acid-producing endophytic bacteria enhances salinity stress tolerance in Oryza sativa. Environ. Exp. Bot. 136, 68–77. doi: 10.1016/j.envexpbot.2017.01.010
Shakirova, F., Allagulova, C., Maslennikova, D., Fedorova, K., Yuldashev, R., Lubyanova, A., et al. (2016). Involvement of dehydrins in 24-epibrassinolide-induced protection of wheat plants against drought stress. Plant Physiol. Biochem. 108, 539–548. doi: 10.1016/j.plaphy.2016.07.013
Sharipova, G., Veselov, D., Kudoyarova, G., Fricke, W., Dodd, I., Katsuhara, M., et al. (2016). Exogenous application of abscisic acid (ABA) increases root and cell hydraulic conductivity and abundance of some aquaporin isoforms in the ABA deficient barley mutant Az34. Ann. Bot. 118, 777–785. doi: 10.1093/aob/mcw117
Sharp, R. E., LeNoble, M. E., Else, M. A., Thorne, E. T., Gherardi, F. (2000). Endogenous ABA maintains shoot growth in tomato independently of effects on plant water balance: evidence for an interaction with ethylene. J. Exp. Bot. 51, 1575–1584. doi: 10.1093/jexbot/51.350.1575
Sharp, R. E., Wu, Y., Voetberg, G. S., Saab, I. N., LeNoble, M. E. (1994). Confirmation that abscisic acid accumulation is required for maize primary root elongation at low water potentials. J. Exp. Bot. 45, 1743–1751. doi: 10.1093/jxb/45
Shi, T.-Q., Peng, H., Zeng, S.-Y., Ji, R.-Y., Shi, K., Huang, H., et al. (2017). Microbial production of plant hormones: opportunities and challenges. Bioengineered. 8, 124–128. doi: 10.1080/21655979.2016.1212138
Singh, R. P., Jha, P. N. (2016). Alleviation of salinity-induced damage on wheat plant by an ACC deaminase-producing halophilic bacterium Serratia sp. SL-12 isolated from a salt lake. Symbiosis 69, 101–111. doi: 10.1007/s13199-016-0387-x
Siyar, S., Inayat, N., Hussain, F. (2019). Plant growth promoting rhizobacteria and plants’ improvement: a mini-review. PSM Biol. Res. 41, 1–5. https://www.journals.psmpublishers.org/index.php/biolres/article/view/214
Spaepen, S., Vanderleyden, J. (2011). Auxin and plant-microbe interactions. Cold Spring Harb. Perspect. Biol. 3. doi: 10.1101/cshperspect.a001438
SkZ, A., Vardharajula, S., Vurukonda, P. S. K. P. (2018). Transcriptomic profiling of maize (Zea mays L.) seedlings in response to Pseudomonas putida stain FBKV2 inoculation under drought stress. Ann. Microbiol. 68, 331–349. doi: 10.1007/s13213-018-1341-3
Tardieu, F. (2005). Plant tolerance to water deficit: physical limits and possibilities for progress. Comptes Rendus Géoscience. 337, 57–67. doi: 10.1016/j.crte.2004.09.015
Timmusk, S., Nicander, B., Granhall, U., Tillberg, E. (1999). Cytokinin production by Paenibacillus polymyxa. Soil Biol. Biochem. 31, 1847–1852. doi: 10.1016/S0038-0717(99)00113-3
Tiwari, S., Lata, C. (2018). Heavy metal stress, signaling, and tolerance due to plant-associated microbes: an overview. Front. Plant Sci. 9, 452. doi: 10.3389/fpls.2018.00452
Tyburski, J., Dunajska, K., Mazurek, P., Piotrowska, B., Tretyn, A. (2009). Exogenous auxin regulates H2O2 metabolism in roots of tomato (Lycopersicon esculentum Mill.) seedlings affecting the expression and activity of CuZn-superoxide dismutase, catalase, and peroxidase. Acta Physiol. Plant. 31, 249–260. doi: 10.1007/s11738-008-0225-8
Vacheron, J., Desbrosses, G., Bouffaud, M.-L., Touraine, B., Moënne-Loccoz, Y., Muller, D., et al. (2013). Plant growth-promoting rhizobacteria and root system functioning. Front Plant Sci. 4, 356. doi: 10.3389/fpls.2013.00356
Valluru, R., Davies, W. J., Reynolds, M. P., Dodd, I. C. (2016). Foliar abscisic acid-to-ethylene accumulation and response regulate shoot growth sensitivity to mild drought in wheat. Front. Plant Sci. 7, 461. doi: 10.3389/fpls.2016.00461
Van der Ent, S., Van Wees, S. C., Pieterse, C. M. (2009). Jasmonate signaling in plant interactions with resistance-inducing beneficial microbes. Phytochemistry. 70, 1581–1588. doi: 10.1016/j.phytochem.2009.06.009
Vargas, L., Santa Brígida, A. B., Mota Filho, J. P., de Carvalho, T. G., Rojas, C. A., Vaneechoutte, D., et al. (2014). Drought tolerance conferred to sugarcane by association with Gluconacetobacter diazotrophicus: a transcriptomic view of hormone pathways. PLoS One. 9, e114744. doi: 10.1371/journal.pone.0114744
Vejan, P., Abdullah, R., Khadiran, T., Ismail, S., Nasrulhaq Boyce, A. (2016). Role of plant growth promoting rhizobacteria in agricultural sustainability – a review. Molecules 21, E573. doi: 10.3390/molecules21050573
Waines, J. G., Ehdaie, B. (2007). Domestication and crop physiology: roots of green-revolution wheat. Ann. Bot. 100, 991–998. doi: 10.1093/aob/mcm180
Watt, M., Moosavi, S., Cunningham, S. C., Kirkegaard, J. A., Rebetzke, G. J., Richards, R. A. (2013). A rapid, controlled-environment seedling root screen for wheat correlates well with rooting depths at vegetative, but not reproductive, stages at two field sites. Ann. Bot. 112, 447–455. doi: 10.1093/aob/mct122
Werner, T., Motyka, V., Laucou, V., Smets, R., Van Onckelen, H., Schmulling, T. (2003). Cytokinin-deficient transgenic Arabidopsis plants show multiple developmental alterations indicating opposite functions of cytokinins in the regulation of shoot and root meristem activity. Plant Cell. 15, 2532–2550. doi: 10.1105/tpc.014928
Werner, T., Nehnevajova, E., Kollmer, I., Novak, O., Strnad, M., Kramer, U., et al. (2010). Root-specific reduction of cytokinin causes enhanced root growth, drought tolerance, and leaf mineral enrichment in Arabidopsis and tobacco. Plant Cell. 22, 3905–3920. doi: 10.1105/tpc.109.072694
Wilkinson, S., Kudoyarova, G. R., Veselov, D. S., Arkhipova, T. N., Davies, W. J. (2012). Plant hormone interactions: innovative targets for crop breeding and management. J. Exp. Bot. 63, 3499–3509. doi: 10.1093/jxb/ers148
Xia, X. J., Zhou, Y. H., Shi, K., Zhou, J., Foyer, C. H., Yu, J. Q. (2015). Interplay between reactive oxygen species and hormones in the control of plant development and stress tolerance. J. Exp. Bot. 66, 2839–2856. doi: 10.1093/jxb/erv089
Xiong, L., Wang, R. G., Mao, G., Koczan, J. M. (2006). Identification of drought tolerance determinants by genetic analysis of root response to drought stress and abscisic acid. Plant Physiol. 142, 1065–1074. doi: 10.1104/pp.106.084632
Xu, J., Li, X.-L., Luo, L. (2012). Effects of engineered Sinorhizobium meliloti on cytokinin synthesis and tolerance of alfalfa to extreme drought stress. Appl. Environ. Microbiol. 78, 8056–8061. doi: 10.1128/AEM.01276-12
Xun, F., Xie, B., Liu, S., Guo, C. (2015). Effect of plant growth-promoting bacteria (PGPR) and arbuscular mycorrhizal fungi (AMF) inoculation on oats in saline-alkali soil contaminated by petroleum to enhance phytoremediation. Environ. Sci. Pollut. Res. Int. 22, 598–608. doi: 10.1007/s11356-014-3396-4
Yasmin, H., Nosheen, A., Naz, R., Bano, A., Keyani, R. (2017). L-tryptophan-assisted PGPR-mediated induction of drought tolerance in maize (Zea mays L.). J. Plant Interact. 12, 567–578. doi: 10.1080/17429145.2017.1402212
Zamioudis, C., Mastranesti, P., Dhonukshe, P., Blilou, I., Pieterse, C. M. (2013). Unraveling root developmental programs initiated by beneficial Pseudomonas spp. Bacteria Plant Physiol. 162, 304–318. doi: 10.1104/pp.112.212597
Zhang, H., Kim, M. S., Krishnamachari, V., Payton, P., Sun, Y., Grimso, M., et al. (2007). Rhizobacterial volatile emissions regulate auxin homeostasis and cell expansion in Arabidopsis. Planta. 226, 839–851. doi: 10.1007/s00425-007-0530-2
Zhang, H., Xie, X., Kim, M. S., Kornyeyev, D. A., Holaday, S., Paré, P. W. (2008). Soil bacteria augment Arabidopsis photosynthesis by decreasing glucose sensing and abscisic acid levels in planta. Plant J. 56, 264–273. doi: 10.1111/j.1365-313X.2008.03593.x
Zavaleta-Mancera, H. A., Lopez-Delgado, H., Loza-Tavera, H., Mora-Herrera, M., Trevilla-Garcia, C., Vargas-Suarez, M., et al. (2007). Cytokinin promotes catalase and ascorbate peroxidase activities and preserves the chloroplast integrity during dark-senescence. J. Plant Physiol. 164, 1572–1582. doi: 10.1016/j.jplph.2007.02.003
Keywords: plant growth promoting rhizobacteria, plant hormones, drought, salinity, petroleum pollution
Citation: Kudoyarova G, Arkhipova T, Korshunova T, Bakaeva M, Loginov O and Dodd IC (2019) Phytohormone Mediation of Interactions Between Plants and Non-Symbiotic Growth Promoting Bacteria Under Edaphic Stresses. Front. Plant Sci. 10:1368. doi: 10.3389/fpls.2019.01368
Received: 05 July 2019; Accepted: 04 October 2019;
Published: 29 October 2019.
Edited by:
Ying Ma, University of Coimbra, PortugalReviewed by:
Collin M. Timm, Johns Hopkins University, United StatesFrancisco Perez-Alfocea, Spanish National Research Council, Spain
Copyright © 2019 Kudoyarova, Arkhipova, Korshunova, Bakaeva, Loginov and Dodd. This is an open-access article distributed under the terms of the Creative Commons Attribution License (CC BY). The use, distribution or reproduction in other forums is permitted, provided the original author(s) and the copyright owner(s) are credited and that the original publication in this journal is cited, in accordance with accepted academic practice. No use, distribution or reproduction is permitted which does not comply with these terms.
*Correspondence: Guzel Kudoyarova, Z3V6ZWxAYW5yYi5ydQ==