- 1Department of Biology, University of York, York, United Kingdom
- 2Department of Animal and Plant Sciences, University of Sheffield, Sheffield, United Kingdom
Controlled environment studies show that arbuscular mycorrhizal fungi (AMF) may contribute to plant nitrogen (N) uptake, but the role of these near-ubiquitous symbionts in crop plant N nutrition under natural field conditions remains largely unknown. In a field trial, we tested the effects of N fertilisation and barley (Hordeum vulgare L.) cultivar identity on the contribution of AMF to barley N uptake using 15N tracers added to rhizosphere soil compartments. AMF were shown capable of significantly increasing plant 15N acquisition from root exclusion zones, and this was influenced by nitrogen addition type, N fertiliser application rate and barley cultivar identity. Our data demonstrate a previously overlooked potential route of crop plant N uptake which may be influenced substantially and rapidly in response to shifting agricultural management practices.
Introduction
Nitrogen (N) is usually the most limiting mineral nutrient to plant growth (Agren et al., 2012) and maintaining modern agricultural production requires frequent and substantial application of fertiliser to farm soils. In various forms an estimated 50 MT year−1 fertiliser N is applied to agricultural land worldwide (Ladha et al., 2016). Assimilation of applied N by crops may be under 50% (Ladha et al., 2005, Masclaux-Daubresse et al., 2010); a significant fraction of this applied N is wasted — lost through processes including volatilisation, microbial immobilisation, runoff, and leaching (Ladha et al., 2016, Cameron et al., 2013). There is economic and ecological pressure on farmers to optimise the N uptake efficiency of crop plants (Hawkesford, 2014) and by reducing the reliance on non-renewable inputs, improve the sustainability of agriculture (Pretty, 2008). This progress will require the integration of biological and ecological processes into agriculture, and better understanding of soil microbial communities and their roles in nutrient cycling (Rillig et al., 2016, Pretty, 2018).
As near-ubiquitous symbionts of cereal crops, arbuscular mycorrhizal fungi (AMF) are prime targets to investigate the role of soil biota in improving agricultural sustainability (Gosling et al., 2006, Thirkell et al., 2017, Rillig et al., 2019). The majority of land plant species engage in symbiosis with these fungi, which may aid plants’ mineral nutrient uptake from soils, in exchange for photosynthetic carbon (C) from their plant hosts (Smith and Read, 2008). The influence that AMF mycelia may exert over nutrient dynamics in agricultural systems is not limited to direct effects on plant nutrient acquisition however; the presence of AMF has been shown to reduce mineral fertiliser leaching (Cavagnaro et al., 2015) and to influence greenhouse gas emissions (Storer et al., 2018). While the role of AMF in biogeochemical cycles is undoubtedly complex, of pressing need is to determine the extent to which plants rely on these symbionts for mineral nutrient acquisition.
It is well established that AMF can contribute to plant N uptake (Ames et al., 1983; Hodge et al., 2001; Leigh et al., 2009; Thirkell et al., 2016), but the extent to which this takes place, and whether it is ecologically or agriculturally relevant is unclear (Smith and Smith, 2011a). This is in part due to relatively little experimental attention. There remains in the literature a focus on the role of AMF in plant phosphorus (P) uptake (Smith and Smith, 2011a; Karasawa et al., 2012; Ezawa and Saito, 2018), and consideration of symbiotic N uptake is often restricted to diazotrophic bacteria while AMF are often overlooked (Garcia et al., 2016).
Improved access to poorly-mobile soil P is, in most instances, the primary benefit of AMF to their plant hosts (Smith and Read, 2008). The relative immobility of inorganic P (Pi) in soil means that plant uptake of Pi from the rhizosphere can outpace Pi diffusion from the surrounding bulk soil and the subsequent P-depletion zones that form around the root are narrow and sharply defined. By engaging in symbiosis with AMF, with a mycelium spreading several centimetres beyond the rhizosphere, the plant effectively increases the volume of soil from which it can acquire nutrients, particularly poorly mobile ions such as Pi (Sanders and Tinker, 1973; Hodge, 2017). Nitrate (NO3−) and ammonium (NH4+), the predominant forms in which plants and fungi acquire N (Marschner, 2011), are more mobile in soil than orthophosphate (Tinker and Nye, 2000). Despite this, a zone of N-depletion may still form around the root (Brackin et al., 2017), in which case AMF may facilitate improved N capture for their plant hosts. With smaller diameters than plant roots, AMF hyphae may also penetrate soil micropores more effectively than a plant root, and thereby be present when inorganic N forms are released through microbial decomposition processes and effectively scavenge for this released inorganic N (Hodge, 2014).
Results from microcosm studies are conflicting as to the importance of AMF in plant N uptake (Hodge and Storer, 2015). While a number of studies have shown no improvement of N uptake by AM plants versus non-mycorrhizal counterparts (Cui and Caldwell, 1996a; Cui and Caldwell, 1996b; Reynolds et al., 2005; Kahkola et al., 2012), it is possible that AMF make an invisible contribution to nutrient acquisition which cannot easily be identified without the use of isotope tracing techniques. Mycorrhizal downregulation of plant root phosphate transporters has been identified in a number of studies (Smith et al., 2003; Smith et al., 2004). In this situation, AMF may be responsible for the majority of a plant’s P acquisition, but root transporter downregulation may result in reduced plant P uptake compared to non-mycorrhizal control plants (Smith et al., 2003; Smith et al., 2004). Whether a similar phenomenon occurs in mycorrhizal root N uptake remains unclear. Isotope tracing data does, however, show that AMF can transfer substantial amount of N to a host plant (Leigh et al., 2009; Thirkell et al., 2016), while the contribution of AMF to field-grown plant N uptake is unknown.
AMF are capable of acquiring N from decomposing organic sources (Leigh et al., 2009; Hodge and Fitter, 2010; Herman et al 2012; Barrett et al., 2014; Thirkell et al., 2016) and even to acquire some organic N directly from the hyphosphere, notably as amino acids (Hawkins et al., 2000; Breuninger et al., 2004; Whiteside et al., 2012a; Whiteside et al., 2012b, Tisserant et al., 2012) and perhaps as dipeptides (Belmondo et al., 2014). As in plants however, the vast majority of N acquired by AMF is thought to be as NO3− or NH4+ (Govindarajulu et al., 2005; Bucking and Kafle, 2015). Greater N uptake as NO3− might be expected as it is usually more abundant than NH4+ because of rapid nitrification (Marschner, 2011). However, because N acquired as NO3− must be reduced to NH4+ before further assimilation, it should be energetically favourable for AMF to acquire N as NH4+ (Hodge et al., 2010; Courty et al., 2015). Corroborative data remains equivocal as to AMF “preference” for N types (Johansen et al., 1993; Hawkins and George, 2001). As NO3− and NH4+ are the most commonly-used forms of fertiliser in Western agriculture, the need to understand mycorrhizal plant acquisition of these N sources is pressing.
Nutrient trade between partners in AM symbioses shows considerable variation in response to biotic factors such as plant and fungal genotype (Smith et al., 2004), in addition to abiotic factors including soil nutrient status (Johnson, 2010; Johnson et al., 2015). Despite substantial experimental data, predictability of the extent to which plants benefit from AMF colonisation remains poor. For example, no universally beneficial fungal isolate has been identified and comparatively few plants are obligate symbionts with AMF.
Despite the widespread distribution of AMF (Smith and Read, 2008; Davison et al., 2015) and the readiness with which they colonise most staple crop plant roots (Smith and Smith, 2011a), little is understood about the function of AMF in the field (Lekberg and Helgason, 2018; Ryan and Graham, 2018). Most published material on the function of AMF is derived from studies conducted under controlled conditions, often comparing AM plants with non-AM controls. While such experiments have provided much valuable data and insight, their findings cannot directly be extrapolated to the field scale, as the occurrence of non-AM cereals in most arable soils is unlikely (Smith and Smith, 2011a). Despite disruptive practices such as tilling and the application of fungicides, there remains a substantial AMF spore bank (and therefore inoculum potential) in agricultural soils (Sosa-Hernandez et al., 2018) and it is very likely that plants in arable field soil will be colonised by AMF (Smith and Smith, 2011a). Further research is needed to begin to understand how AMF might affect crop plant nutrient uptake in situ.
Adding 15N isotope tracers to mesh-walled soil compartments in a field trial, we examined the role of AMF in the N acquisition by barley (Hordeum vulgare L.) cultivars “Meridian” and “Maris Otter”. Isotopic 15N labelling was carried out in plots receiving contrasting N rates to test the impact of N availability on nutrient transfer in the symbiosis. We tested the hypothesis that increased N fertilisation would result in more AMF transfer of N to host plants because AMF, by virtue of their size, would be better able than roots to compete with the soil microbiome for the added N held in physically small microsites. N tracers were added as NH4+ or NO3− to investigate the relative uptake and transfer of different N sources by AMF.
Materials and Methods
Field Trial Design
Data were gathered from a larger field trial, designed and implemented at Sancton, East Riding of Yorkshire (co-ordinates 53°51′10.2″N 0°35′29.1″W), by ADAS (Pendeford, Wolverhampton, UK). The ADAS trial was set up to test how barley yield compares among 6 application rates of ammonium nitrate (NH4NO3) fertiliser (Nitram, CF Fertiliser, Ince, Cheshire, UK) ranging from 0–300 kg ha−1. The soil at the trial site comprises a silty rendzina, with a significant proportion of chalk fragments (UKSO, 2016). Soil mineral N, quantified shortly before sowing, was 29.9 kg N ha−1, of which 28 kg was nitrate-N and 1.9 kg ammonium-N. The field site on which the trial was based is a commercial arable farm, with barley (Hordeum vulgare L.), oilseed rape (Brassica napus L.) and wheat (Triticum aestivum L.) grown in a rotation.
The ADAS trial used plots measuring 12 m × 1.5 m, clustered in groups of 6 by N application rate, with each variety represented once per cluster. Each N application rate was applied to 3 replicate clusters, of 6 varieties, meaning 18 clusters in total, with a combined area of 1944 m2. Experimental clusters of N application rates were separated to each side by buffer zones 6 m wide, and at each end by buffer zones 3 m long (Figure 1). Owing to the logistical challenges of sampling the entire trial, the experimental work presented here is gathered from two of the N application rates (60 kg ha−1 (N rate 2 in Figure 1), and 280 kg ha−1 (N rate 5 in Figure 1)), and two of the barley cultivars: KWS Meridian (KWS UK Ltd, Thriplow, Hertfordshire, UK), a 6-row feedstock barley; and Maris Otter (Robin Appel, Waltham Chase, Hampshire, UK), a 2-row malting barley, giving 4 treatment groups, with 3 replicate plots per treatment. Meridian and Maris Otter were chosen from the panel of 6 cultivars available in the trial as they represent contrasting ages of barley varieties, developed in the 1960s and 2000s respectively. Further, Maris Otter is a malting barley, characterised by a low grain protein content, while Meridian was developed as a feedstock barley, with a higher grain protein (and therefore N) content. Experimental sampling and isotope labelling were carried out during the post-anthesis, grain filling period — approximate growth stages 70–80 (Zadoks et al., 1974).
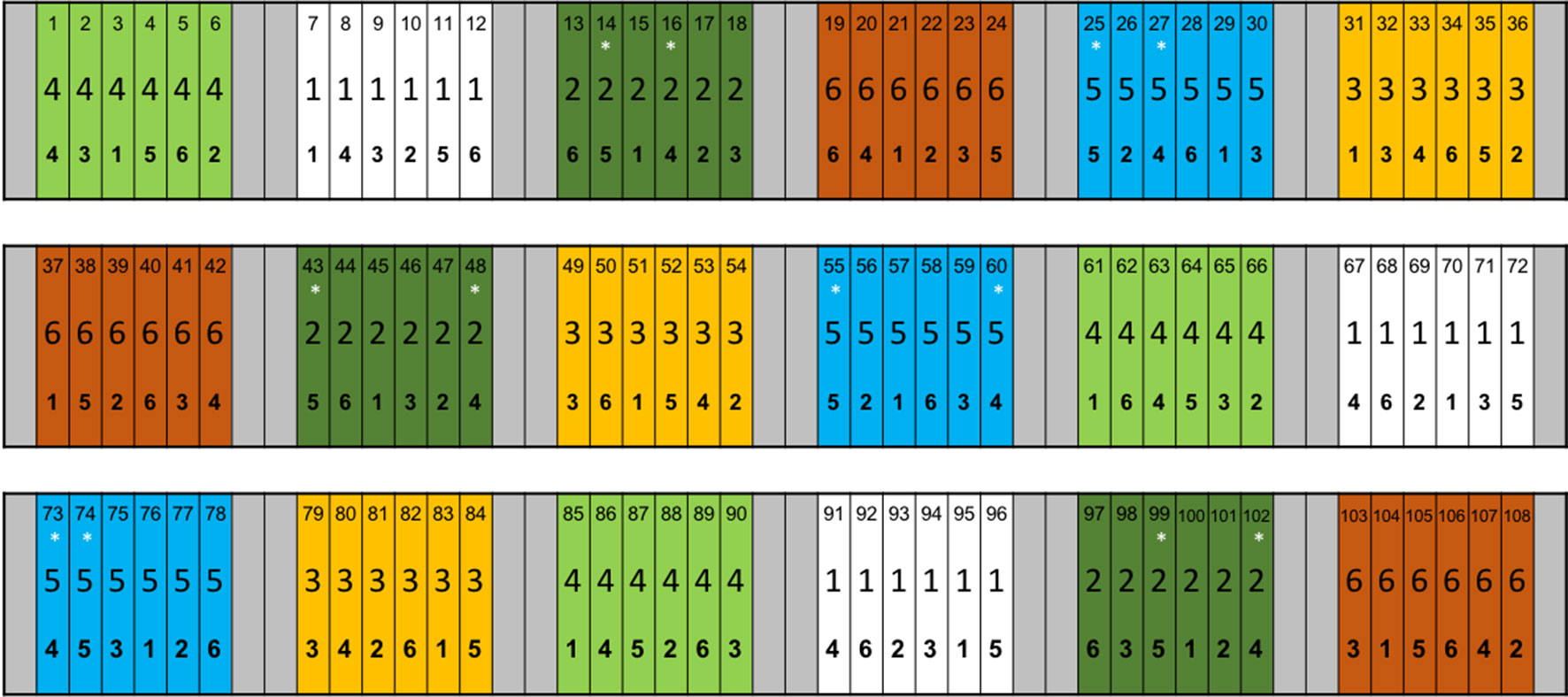
Figure 1 ADAS experiment established at Sancton, East Riding of Yorkshire, UK. Six barley (Hordeum vulgare L.) cultivars were planted at the trial site, and received one of 6 N addition rates, ranging from 0 to 300 kg ha−1. Each combination of barley cultivar and N rate was replicated 3 times. Each plot has 3 numbers, denoting: plot identity, N addition rate and barley cultivar, reading top to bottom. Nitrogen addition rate “2” represents 60 kg ha−1 and “5” is 280 kg ha−1. Plot colours also represent N addition rate. Meridian barley is denoted by “4” and Maris Otter by “5”. Asterisks (*) represent plots from which root samples were taken for analysis of root length colonisation and to which 15N tracer was added. Reproduced with permission from Pete Berry and Kate Storer, ADAS.
Intraradical and Extraradical AMF Quantification
AMF colonisation of both barley varieties was confirmed and then quantified by staining of roots collected from the trial plots. Roots were collected from between 5 and 15 cm below the surface. After clearing in 10% (w/v) KOH for 20 min at 70°C, roots were rinsed in de-ionised water, acidified in 1% (v/v) HCl at 25°C for 10 min and then stained in Trypan Blue at 25°C for 20 min. Roots were then rinsed again in de-ionised water before being left in a 50% (v/v) glycerol solution for 24 h, before being mounted onto microscope slides to allow quantification of root length colonisation (RLC) using the gridline intersect method (McGonigle et al., 1990).
Soil samples were collected from between 5 and 15 cm below the soil surface. As AMF hyphal turnover can be rapid (Staddon et al., 2003), hyphal extraction took place within 6 h of collection to minimise loss due to decomposition. Extraradical hyphal quantity in the plots was determined using an adapted method from Staddon et al. (1999). Briefly, samples of known mass (5–10 g) were suspended in 500 mL of de-ionised water and agitated with a magnetic stirrer plate in order to free the hyphae from soil particles. From this, 200 mL was decanted to a smaller beaker on a magnetic stirrer. Aliquots (10 mL) were removed and vacuum filtered through 0.45 µm nylon mesh (Anachem, Bedfordshire, UK) and hyphal length density (HLD) was quantified using the gridline intersect method (Hodge, 2001).
15N Stable Isotope Labelling
The AMF contribution to barley N uptake was investigated by adding a solution of 15N (as either (15NH4)2SO4 or K15NO3), into mesh-walled cores, into which AMF hyphae could access but plant roots could not, or (as controls for diffusion and mass flow of the added N) cores into which neither AMF hyphae or roots could access. Isotopic 15N was added in the form of Long Ashton nutrient solution (LAS) (Smith et al., 1983), which can be prepared variously to provide 15N as 15NH4+ or 15NO3− in equimolar concentrations. The LAS was made to the standard protocols except N being 300% the original concentrations. Each core received 5 mL of LAS, containing 0.683 mg 15N. (Long Ashton nutrient solution protocol is included in Supplementary Information Document 1).
Hyphal access cores were constructed following an adapted method from Johnson et al. (2001). Lengths of PVC tubing (length 85 mm, internal diameter 13 mm, external diameter 16 mm; internal volume 9.9 cm3) with 2 windows cut in the sides of the lower 2/3 of the tube so that 50% of the side area was open, were wrapped in a 20 µm nylon mesh (John Stanier and Co., Whitefield, Manchester, UK), fixed with Tensol adhesive cement (Bostik Inc., Wauwatosa, Wisconsin, USA). The open bottom end of each tube was covered with the same size mesh. Control cores, which allowed diffusion and mass flow of solutes but prevent hyphal ingrowth, were covered with 0.45 µm nitrocellulose membrane mesh to prevent root and hyphal ingrowth. Cores were filled with a 1/1 (v/v) mixture of silica sand and TerraGreen® (calcinated attapulgite clay, Oil-Dri, Cambridgeshire, UK), which had been sterilised by autoclaving (121°C for 44 min), providing a uniform substrate into which the 15N solutions could be added.
Each of these cores was then placed inside another, slightly larger core, constructed in the same manner (length 75 mm internal diameter 18 mm, external diameter 21 mm). These cores were also covered in a 20 µm nylon mesh. Such a “core in a core” design allows the placement of zones of defined and uniform size into the soil, to which 15N label solutions could be added. A small (approximately 1 mm) air gap is made between the external mesh wall of one core and the internal mesh wall of the other, which should reduce the rapid diffusion of N from the site of addition, which has been a problem in studies where 15N has been added (Smith and Smith, 2011b). Diffusion and mass flow are unlikely to be prevented entirely, as the pressure of soil on the sides of the core may push the mesh together so that the two layers of mesh make contact. However, the system provides a more stable labelling zone than using a single core, where one mesh layer may be easily damaged (Johnson et al., 2001).
Each of the 12 experimental plots received four cores (1. No AMF Access + 15NH4+; 2. AMF Access + 15NH4+; 3. No AMF Access + 15NO3−; 4. AMF Access + 15NO3−), spaced 3 m apart to avoid contamination of 15N from neighbouring cores (Figure 2). Placement of cores took place 8 weeks before label addition to allow hyphal ingrowth from the bulk soil. A piece of tape was placed over the top of cores to minimise contamination. This tape was removed for 15N addition and then replaced.
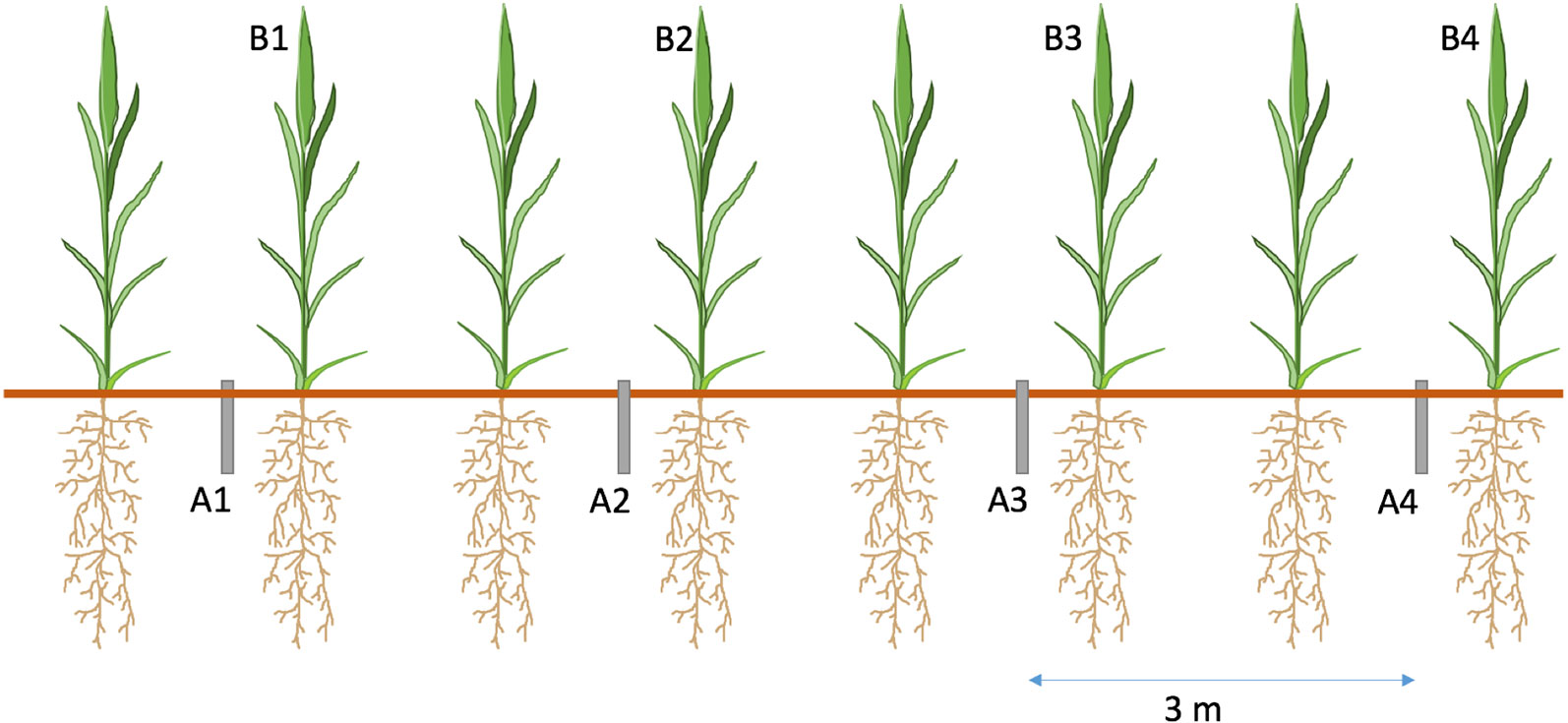
Figure 2 Diagram of 15N addition experiment. PVC cores were inserted adjacent to barley (Hordeum vulgare L.) plants, four cores per plot, spaced 3 m apart. Cores were organised as follows A1 – AMF Access + Ammonium (NH4+); A2 - No AMF Access + Nitrate (NO3−); A3 - AMF Access + NO3−; A4 - No AMF Access + NH4+ Each core received 0.683 mg 15N added as Long Ashtons nutrient solution. Plant shoots closest to the core (B1-4) were removed, dried and homogenised for N analysis. B1-B4 denote shoot samples taken.
Sample Collection and Preparation
After 7 days, the nearest plant to each labelling core was cut at ground level and removed, dried at 70°C for 48 h and homogenised in a kitchen blender (Morphy Richards, Mexborough, South Yorkshire, UK) then in a ball mill (MM400 Ball Mill, Retsch GmbH, Haan, Germany). Homogenised shoot samples of known mass (3 mg ± 0.5 mg) were used to quantify 15N and N content, performed by isotope ratio mass spectrometry (IRMS) (PDZ 2020, Sercon Ltd, Crewe, UK).
Statistical Analysis
For all data, statistical analysis was performed using the “R 3.1.0” statistical package, through the “RStudio” integrated development environment (R foundation for Statistical Computing, Vienna, Austria). Data were tested for normality using Shapiro-Wilk and Kolmogorov-Smirnov tests, and Levene’s test was used to confirm homogeneity of variance. Where these tests suggested data did not match test assumptions, data were square-root or log-transformed prior to analysis. Data for root length colonisation, hyphal length density, barley N concentration and biomass were tested by two-way ANOVA, using N addition rate and barley variety as explanatory variables. As two additional explanatory variables were added in the trial for 15N uptake (N addition type, ammonium/nitrate; AM treatment, access/no access), and the small number of replicates in the ADAS field trial, it was not possible to test these factors and the N addition rate and barley cultivar at once. As such, data were split into barley cultivar and N application rate for the 15N data and tested by two-way ANOVA. Here, 15N enrichment was the response variable, while N type and AMF access treatment were the explanatory variables.
Results
Shoot acquisition of 15N added to mesh cores was significantly improved by allowing AMF access into cores, but only when added as 15NO3−, and only in the High-N plots of Meridian barley (Figure 3). T-tests indicate that only in High-N Meridian plots receiving 15NO3− were 15N enrichment levels greater in the AM access treatment than in the no access controls (T2 = 4.48, p = 0.023)(Supplementary Information, Figure 1). A two-way ANOVA showed that in High-N Meridian, there was a significant effect of N source (F1,8 = 12.73, p = 0.007) and AMF access to cores (F1,8 = 27.86, p = 0.007). There was also a significant interaction between N source and AMF access (F1,8 = 14.25, p = 0.005) (Figure 3). In High-N Meridian with AMF access, the harvested plants, i.e. those individuals closest to the core to which the isotope label was added, acquired on average 1.62% of the 15N supplied. Other treatment groups saw no greater plant uptake of 15N where AMF could access the isotope label than in no-access controls. Excepting High-N Meridian plots, mean shoot 15N content did not differ among treatments and controls, indicating similar plant acquisition of N following diffusion/mass flow out and into the soil, but minimal fungal-mediated uptake.
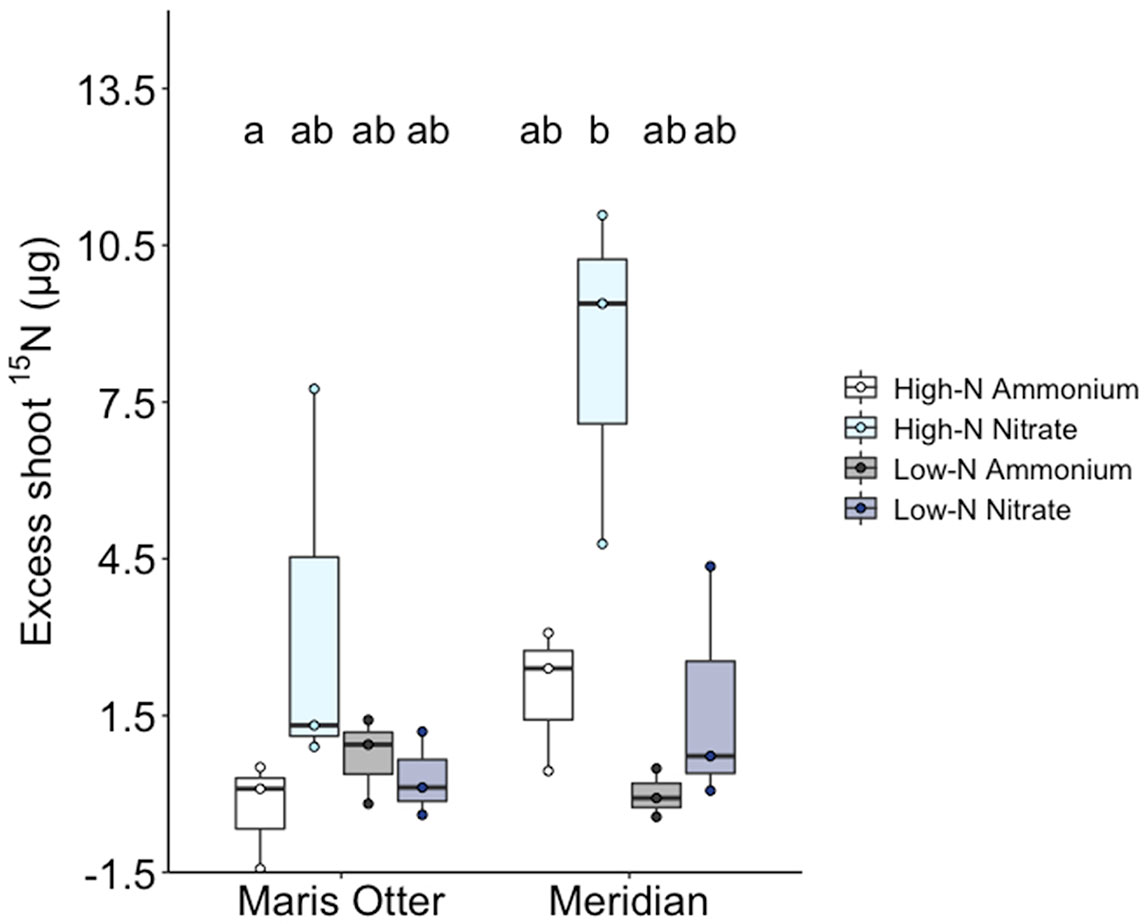
Figure 3 Excess 15N content in Maris Otter and Meridian shoots (calculated by subtracting shoot 15N content in each “Access” unit from the mean of the corresponding values in the “No Access” units). Shoot 15N enrichment was significantly higher than “no access” controls when supplied as nitrate to Meridian barley in High-N plots. Circles represent individual data points, boxplot centre bars represent the median values. High-N + ammonium groups are represented by white bars, High-N + nitrate by light blue bars, Low-N + ammonium by dark grey bars and Low-N + nitrate by dark blue bars. Data shown are means ± SEM, n = 3. Bars sharing the same letter are not significantly different.
All plant roots studied were found to be colonised by AMF, indicating a substantial inoculum potential of the soil at the trial site, although no differences were found between cultivar or N-rate treatments (p > 0.05). Mean colonisation was 33.7% (± 3.52% SEM) across all treatment groups (Figure 4). Extraradical mycelium (ERM) hyphal densities, measured in the zones to which 15N was added, were not different among treatment groups (p > 0.05). Mean ERM hyphal density across all treatments was 2.49 m g−1 DW soil (± 0.31 m g−1 SEM). In both cultivars, High-N plots supported ∼ 60% higher shoot N content than Low-N plots (F1,8 = 74.55, p < 0.001), and shoot N concentration was significantly higher in High-N than Low-N plots (F1,8 = 84.28, p < 0.001). Mean shoot N concentration was 9.30 mg g−1 DW in Low-N blocks of Maris Otter, and 14.75 mg g−1 DW in the High-N. Meridian showed a very similar trend, as N concentration increased from 9.57 mg g−1 DW in Low-N plots to 14.38 mg g−1 DW in the High N. Shoot N concentration and content did not differ between the two cultivars tested. Shoot DW did not differ between the varieties or the N addition rates.
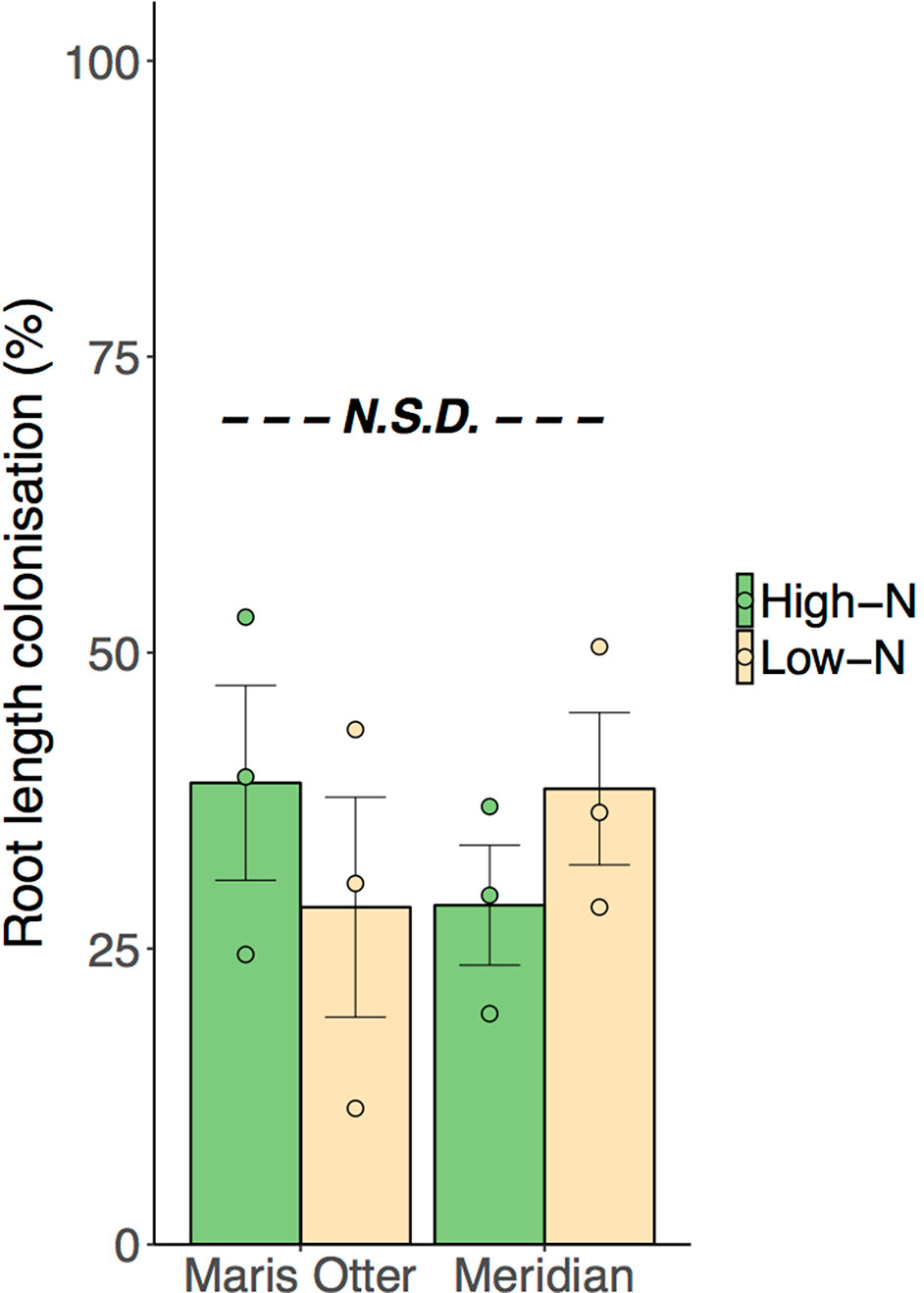
Figure 4 Percentage root length colonisation, as determined by Trypan Blue staining, was not significantly different between treatments. All inspected plants were colonised by arbuscular mycorrhizal fungi (AMF), confirmed by presence of characteristic structures, arbuscules and vesicles. Mean colonisation ranged from 28.5% in Maris Otter in Low N, to 38.0% in Meridian Low-N, but no groups were significantly different. Circles represent individual data points. High-N groups are denoted by green bars, Low-N groups are denoted by yellow bars. “N.S.D.” indicates that there were no significant differences among treatment means. Data shown are means ± SEM, n = 3.
Discussion
The enrichment of 15N in barley shoots suggests a role for AMF-facilitated N acquisition by crop plants, a phenomenon not previously observed in a field setting. Moreover, our data suggest this route of N uptake is dependent upon barley cultivar identity, the N form added and the rate at which N has previously been applied to the plots. AMF have been shown to transfer substantial quantities of N to plants in root organ culture experiments (Jin et al., 2005) although caution must be exercised before extrapolating these values to crop plant systems as they are far-removed from realistic mycorrhizal physiology. Whole-plant microcosm studies conducted under greenhouse conditions have given mixed results as to whether AMF may contribute to plant N nutrition (Hodge and Storer, 2015). Our data provide the first suggestion that AMF may have a role in cereal crop N uptake in the field. Our data also suggest that short-term changes in N fertilisation regimes can elicit shifts in AM functioning.
While our data suggest a preference for AMF to transfer N to plants when provided to this system as NO3− rather than NH4+, previous experimental evidence as to inorganic N source preference by AMF is equivocal (Johansen et al., 1993; Hawkins and George, 2001). Higher uptake of NO3− than NH4+ is contrary to models which suggest NH4+ acquisition should be less energetically expensive (Govindarajulu et al., 2005). Hyphal NH4+ uptake may be retarded by problems of charge balancing that are perhaps not encountered when N is acquired as NO3−. Simultaneous uptake of NO3− and cations such as K+, Ca2+ or Mg2+ from the soil may avoid changes in electrochemical potential across exchange surfaces, allowing N acquisition. Meanwhile, NH4+ uptake would require proton secretion (or anion uptake), which may shift soil pH making further NH4+ uptake more difficult. Nitrate-N comprised over 90% of the available N in the soil before the trial was planted, a trend which is not unusual, as NO3− often dominates inorganic N pools in arable soils (Marschner, 2011). These relative abundances of N sources may have led to AMF hyphal physiology being acclimated to nitrate uptake (Garraway and Evans, 1984), meaning suddenly-available NH4+ could not be acquired effectively. Although the movement and cycling of nitrate and ammonium are known to be influenced by soil moisture (Homyak et al., 2017), precipitation data for the site (Supplementary Information, Table 1) indicates no extraordinary rainfall in the weeks over which the experiment took place, suggesting this was of minor importance here.
While recovery of only 1.6% of the 15N label seems low, total 15N recovery is likely to have been greater than the data suggests. Our data are derived from the aboveground tissue of one plant proximal to the mesh-walled core into which isotopes were added, and it is probable that the roots of numerous plants would have been in close proximity to the core. As such, further 15N is likely to have been acquired by multiple plants. Furthermore, greater 15N uptake into plant shoots may have been recorded if the shoot tissue samples had been taken longer after 15N addition to the mesh-walled cores.
Mesh-walled exclusion cores have been used to quantify AMF-plant nutrient dynamics in a number of studies (Johnson et al., 2001; Field et al., 2012; Field et al., 2016), and are of particular utility where the establishment of truly non-mycorrhizal control plants is not feasible, as in this study. The use of a 0.45 µm nitrocellulose membrane to exclude AMF in-growth to soil compartments is a well-established methodology in the literature (Hodge et al., 2001; Leigh et al., 2009; Thirkell et al., 2016; Storer et al., 2018), although some concerns arise in relation to the effects of such small pore sizes on solute movement, although in the case of studies investigating mycorrhizal P uptake, such effects have been determined to be insignificant (see Zhang et al., 2016; Svenningsen et al., 2018). Our data show increased plant 15N uptake in plots only where N was supplied as nitrate, to Meridian barley, and in plots which had received high rates of N fertiliser (Figure 3). Were the movement of N through these systems determined by the porosity of the membranes used in “no access” treatments, we might expect 15N enrichment in all plots which received 15NO3−, which is not the case. Alternative control treatments to disentangle the effects of AMF on plant nutrition might be tested further in future studies to determine the relative merits of each method. Non-mycorrhiza-forming mutants of a number of cereals have been developed (Paszkowski et al., 2006; Watts-Williams and Cavagnaro, 2015) but to date no mycorrhizal-defective barley mutants are available against which data from hyphal exclusion experiments can be compared. Furthermore, an AMF-colonised plant is morphologically (Gutjahr et al., 2009) and physiologically (Luginbuehl and Oldroyd, 2017) distinct from one which remains uncolonised, and comparisons between AM and mycorrhiza-defective mutants may erroneously conflate these differences and ascribe all contrasts to the lack of mycorrhizas. Combinations of experimental approaches may be employed here to improve the rigour of field experimentation, although the logistics of such trials may prove represent a significant challenge.
Identifying the mechanisms responsible for differential nitrogen transfer from fungus to plant are beyond the scope of this study, but a number of possibilities may be considered. Numerous studies have demonstrated shifts in AMF community composition or structure following N fertilisation, in grassland (Egerton-Warburton and Allen, 2000; Egerton-Warburton et al., 2007; Antoninka et al., 2011; Jiang et al., 2018) and arable systems (Verbruggen et al., 2010; Avio et al., 2013; Liu et al., 2014; Williams et al., 2017). As AMF isolates are known to be functionally different (Avio et al., 2006; Mensah et al., 2015) any N-driven shifts in AMF community have the potential to influence the N cycling in the system (Herman et al., 2012). Future experimental testing of the AMF community composition within cereal roots, combined with isotopic tracer studies may elucidate any link between the structure and function of AMF communities in agronomic systems.
Conclusions
Our data show that AMF transfer of N to plant hosts is influenced by agricultural management decisions, here the cultivar of barley and the rate at which inorganic N fertiliser is supplied. The extent to which symbiotic soil microbes might enhance total nutrient uptake in the field remains to be tested; despite demonstrating a mechanism by which plants acquire N, our data cannot indicate whether non-AMF plants in the same field conditions might show enhanced nutrition. Further experimental investigation is required for a wider perspective on the influence of these fungi on their crop plant hosts, and therefore their importance in agricultural systems.
Data Availability Statement
The datasets generated for this study are included within the supplementary information of this manuscript.
Author Contributions
TT, DC, and AH designed the study. TT carried out experimental work and data analysis and wrote the initial draft of the manuscript. All authors contributed to revisions of the manuscript, and read and approved the final submitted version.
Funding
This work was supported by a BBSRC White Rose DTP grant: BB/J014443/1.
Conflict of Interest
The authors declare that the research was conducted in the absence of any commercial or financial relationships that could be construed as a potential conflict of interest.
Acknowledgments
We thank Kate Storer and Pete Berry from ADAS for permission to use their field trial, and for discussion about the experiment. The barley trial used here was jointly funded by AHDB and CF Fertilisers. We thank Andrew Manfield, on whose land the trial was sited. We thank Heather Walker for help with the IRMS. This manuscript is an adapted and revised version of a thesis chapter submitted by Tom Thirkell for the degree of Doctor of Philosophy at the University of York, UK, in 2017. Experimental data is presented in the Supplementary Material.
Supplementary Material
The Supplementary Material for this article can be found online at: https://www.frontiersin.org/articles/10.3389/fpls.2019.01312/full#supplementary-material
References
Agren, G. I., Wetterstedt, J. A. M., Billberger, M. F. K. (2012). Nutrient limitation on terrestrial plant growth - modeling the interaction between nitrogen and phosphorus. New Phytol. 194, 953–960. doi: 10.1111/j.1469-8137.2012.04116.x
Ames, R. N., Reid, C. P. P., Porter, L. K., Cambardella, C. (1983). Hyphal uptake and transport of nitrogen from 2 15N-labeled sources by Glomus mosseae, a vesicular arbuscular mycorrhizal fungus. New Phytol. 95, 381–396. doi: 10.1111/j.1469-8137.1983.tb03506.x
Antoninka, A., Reich, P. B., Johnson, N. C. (2011). Seven years of carbon dioxide enrichment, nitrogen fertilization and plant diversity influence arbuscular mycorrhizal fungi in a grassland ecosystem. New Phytol. 192, 200–214. doi: 10.1111/j.1469-8137.2011.03776.x
Avio, L., Castaldini, M., Fabiani, A., Bedini, S., Sbrana, C., Turrini, A., et al. (2013). Impact of nitrogen fertilization and soil tillage on arbuscular mycorrhizal fungal communities in a mediterranean agroecosystem. Soil Biol. Biochem. 67, 285–294. doi: 10.1016/j.soilbio.2013.09.005
Avio, L., Pellegrino, E., Bonari, E., Giovannetti, M. (2006). Functional diversity of arbuscular mycorrhizal fungal isolates in relation to extraradical mycelial networks. New Phytol. 172, 347–357. doi: 10.1111/j.1469-8137.2006.01839.x
Barrett, G., Campbell, C. D., Hodge, A. (2014). The direct response of the external mycelium of arbuscular mycorrhizal fungi to temperature and the implications for nutrient transfer. Soil Biol. Biochem. 78, 109–117. doi: 10.1016/j.soilbio.2014.07.025
Belmondo, S., Fiorilli, V., Perez-Tienda, J., Ferrol, N., Marmeisse, R., Lanfranco, L. (2014). A dipeptide transporter from the arbuscular mycorrhizal fungus Rhizophagus irregularis is upregulated in the intraradical phase. Front. Plant Sci. 5, 1–11. doi: 10.3389/fpls.2014.00436
Brackin, R., Atkinson, B. S., Sturrock, C. J., Rasmussen, A. (2017). Roots-eye view: Using microdialysis and microct to non-destructively map root nutrient depletion and accumulation zones. Plant Cell Environ. 40, 3135–3142. doi: 10.1111/pce.13072
Breuninger, M., Trujillo, C. G., Serrano, E., Fischer, R., Requena, N. (2004). Different nitrogen sources modulate activity but not expression of glutamine sythetase in arbuscular mycorrhizal fungi. Fungal Genet. Biol. 41 (5), 542–552. doi: 10.1016/j.fgb.2004.01.003
Bucking, H., Kafle, A. (2015). Role of arbuscular mycorrhizal fungi in the nitrogen uptake of plants: current knowledge and research gaps. Agronomy-Basel 5, 587–612. doi: 10.3390/agronomy5040587
Cameron, K. C., Di, H. J., Moir, J. L. (2013). Nitrogen losses from the soil/plant system: a review. Ann. Appl. Biol. 162, 145–173. doi: 10.1111/aab.12014
Cavagnaro, T. R., Bender, S. F., Asghari, H. R., Van Der Heijden, M. G. A. (2015). The role of arbuscular mycorrhizas in reducing soil nutrient loss. Trends Plant Sci. 20, 283–290. doi: 10.1016/j.tplants.2015.03.004
Courty, P. E., Smith, P., Koegel, S., Redecker, D., Wipf, D. (2015). Inorganic nitrogen uptake and transport in beneficial plant root-microbe interactions. Critic. Rev. Plant Sci. 34, 4–16. doi: 10.1080/07352689.2014.897897
Cui, M., Caldwell, M. M. (1996a). Facilitation of plant phosphate acquisition by arbuscular mycorrhizas from enriched soil patches.1. Roots and hyphae exploiting the same soil volume. New Phytol. 133, 453–460. doi: 10.1111/j.1469-8137.1996.tb01912.x
Cui, M. Y., Caldwell, M. M. (1996b). Facilitation of plant phosphate acquisition by arbuscular mycorrhizas from enriched soil patches.2. Hyphae exploiting root-free soil. New Phytol. 133, 461–467. doi: 10.1111/j.1469-8137.1996.tb01913.x
Davison, J., Moora, M., Opik, M., Adholeya, A., Ainsaar, L., Ba, A., et al. (2015). Global assessment of arbuscular mycorrhizal fungus diversity reveals very low endemism. Science 349, 970–973. doi: 10.1126/science.aab1161
Egerton-Warburton, L. M., Allen, E. B. (2000). Shifts in arbuscular mycorrhizal communities along an anthropogenic nitrogen deposition gradient. Ecol. Appl. 10, 484–496. doi: 10.1890/1051-0761(2000)010[0484:SIAMCA]2.0.CO;2
Egerton-Warburton, L. M., Johnson, N. C., Allen, E. B. (2007). Mycorrhizal community dynamics following nitrogen fertilization: a cross-site test in five grasslands. Ecol. Monogr. 77, 527–544. doi: 10.1890/06-1772.1
Ezawa, T., Saito, K. (2018). How do arbuscular mycorrhizal fungi handle phosphate? New insight into fine-tuning of phosphate metabolism. New Phytol. 220, 1116–1121. doi: 10.1111/nph.15187
Field, K. J., Cameron, D. D., Leake, J. R., Tille, S., Bidartondo, M. I., Beerling, D. J. (2012). Contrasting arbuscular mycorrhizal responses of vascular and non-vascular plants to a simulated palaeozoic CO2 decline. Nat. Commun. 3, 1–8. doi: 10.1038/ncomms1831
Field, K. J., Rimington, W. R., Bidartondo, M. I., Allinson, K. E., Beerling, D. J., Cameron, D. D., et al. (2016). Functional analysis of liverworts in dual symbiosis with Glomeromycota and Mucoromycotina fungi under a simulated palaeozoic CO2 decline. ISME J. 10, 1514–1526. doi: 10.1038/ismej.2015.204
Garcia, K., Doidy, J., Zimmermann, S. D., Wipf, D., Courty, P. E. (2016). Take a trip through the plant and fungal transportome of mycorrhiza. Trends Plant Sci. 21, 937–950. doi: 10.1016/j.tplants.2016.07.010
Garraway, M. O., Evans, R. C. (1984). Fungal nutrition and physiology. USA, John Wiley & Sons: New York.
Gosling, P., Hodge, A., Goodlass, G., Bending, G. D. (2006). Arbuscular mycorrhizal fungi and organic farming. Agric. Ecosyst. Environ. 113, 17–35. doi: 10.1016/j.agee.2005.09.009
Govindarajulu, M., Pfeffer, P. E., Jin, H. R., Abubaker, J., Douds, D. D., Allen, J. W., et al. (2005). Nitrogen transfer in the arbuscular mycorrhizal symbiosis. Nature 435, 819–823. doi: 10.1038/nature03610
Gutjahr, C., Casieri, L., Paszkowski, U. (2009). Glomus intraradices induces changes in root system architecture of rice independently of common symbiosis signaling. New Phytol. 182, 829–837. doi: 10.1111/j.1469-8137.2009.02839.x
Hawkesford, M. J. (2014). Reducing the reliance on nitrogen fertilizer for wheat production. J. Cereal Sci. 59, 276–283. doi: 10.1016/j.jcs.2013.12.001
Hawkins, H. J., George, E. (2001). Reduced N-15-nitrogen transport through arbuscular mycorrhizal hyphae to Triticum aestivum L. Supplied with ammonium vs. nitrate nutrition. Ann. Bot. 87, 303–311. doi: 10.1006/anbo.2000.1305
Hawkins, H. J., Johansen, A., George, E. (2000). Uptake and transport of organic and inorganic nitrogen by arbuscular mycorrhizal fungi. Plant Soil 226, 275–285. doi: 10.1023/A:1026500810385
Herman, D. J., Firestone, M. K., Nuccio, E., Hodge, A. (2012). Interactions between an arbuscular mycorrhizal fungus and a soil microbial community mediating litter decomposition. FEMS Microbiol. Ecol. 80, 236–247.10. doi: 10.1111/j.1574-6941.2011.01292.x
Hodge, A. (2001). Arbuscular mycorrhizal fungi influence decomposition of, but not plant nutrient capture from, glycine patches in soil. New Phytol. 151, 725–734. doi: 10.1046/j.0028-646x.2001.00200.x
Hodge, A. (2014). Interactions between arbuscular mycorrhizal fungi and organic material substrates. Adv. Appl. Microbiol. 89, 47–99. doi: 10.1016/B978-0-12-800259-9.00002-0
Hodge, A. (2017). “Accessibility of inorganic and organic nutrients for mycorrhizas,” in Mycorrhizal mediation of soil fertility, structure and carbon storage. Eds. Johnson, N., Gehring, C., Jansa, J. (Amsterdam, Netherlands: Elsevier), 129–148. doi: 10.1016/B978-0-12-804312-7.00008-5
Hodge, A., Campbell, C. D., Fitter, A. H. (2001). An arbuscular mycorrhizal fungus accelerates decomposition and acquires nitrogen directly from organic material. Nature 413, 297–299. doi: 10.1038/35095041
Hodge, A., Fitter, A. H. (2010). Substantial nitrogen acquisition by arbuscular mycorrhizal fungi from organic material has implications for N cycling. Proc. Natl. Acad. Sci. U.S.A. 107, 13754–13759. doi: 10.1073/pnas.1005874107
Hodge, A., Helgason, T., Fitter, A. H. (2010). Nutritional ecology of arbuscular mycorrhizal fungi. Fungal Ecol. 3, 267–273. doi: 10.1016/j.funeco.2010.02.002
Hodge, A., Storer, K. (2015). Arbuscular mycorrhiza and nitrogen: implications for individual plants through to ecosystems. Plant Soil 386, 1–19. doi: 10.1007/s11104-014-2162-1
Homyak, P. M., Allison, S. D., Huxman, T. E., Goulden, M. L., Treseder, K. K. (2017). Effects of drought manipulation on soil nitrogen cycling: a meta-analysis. J. Geophys. Res. Biogeosci. 122, 3260–3272. doi: 10.1002/2017JG004146
Jiang, S. J., Liu, Y. J., Luo, J. J., Qin, M. S., Johnson, N. C., Opik, M., et al. (2018). Dynamics of arbuscular mycorrhizal fungal community structure and functioning along a nitrogen enrichment gradient in an alpine meadow ecosystem. New Phytol. 220, 1222–1235. doi: 10.1111/nph.15112
Jin, H., Pfeffer, P. E., Douds, D. D., Piotrowski, E., Lammers, P. J., Shachar-Hill, Y. (2005). The uptake, metabolism, transport and transfer of nitrogen in an arbuscular mycorrhizal symbiosis. New Phytol. 168, 687–696. doi: 10.1111/j.1469-8137.2005.01536.x
Johansen, A., Jakobsen, I., Jensen, E. S. (1993). Hyphal transport by a vesicular-arbuscular mycorrhizal fungus of N applied to the soil as ammonium or nitrate. Biol. Fertil. Soils 16, 66–70. doi: 10.1007/BF00336518
Johnson, D., Leake, J. R., Read, D. J. (2001). Novel in-growth core system enables functional studies of grassland mycorrhizal mycelial networks. New Phytol. 152, 555–562. doi: 10.1046/j.0028-646X.2001.00273.x
Johnson, N. C. (2010). Resource stoichiometry elucidates the structure and function of arbuscular mycorrhizas across scales. New Phytol. 185, 631–647. doi: 10.1111/j.1469-8137.2009.03110.x
Johnson, N. C., Wilson, G. W. T., Wilson, J. A., Miller, R. M., Bowker, M. A. (2015). Mycorrhizal phenotypes and the law of the minimum. New Phytol. 205, 1473–1484. doi: 10.1111/nph.13172
Kahkola, A. K., Nygren, P., Leblanc, H. A., Pennanen, T., Pietikainen, J. (2012). Leaf and root litter of a legume tree as nitrogen sources for cacaos with different root colonisation by arbuscular mycorrhizae. Nutr Cycl Agroecosys 92, 51–65. doi: 10.1007/s10705-011-9471-z
Karasawa, T., Hodge, A., Fitter, A. H. (2012). Growth, respiration and nutrient acquisition by the arbuscular mycorrhizal fungus glomus mosseae and its host plant Plantago lanceolata in cooled soil. Plant Cell Environ. 35, 819–828. doi: 10.1111/j.1365-3040.2011.02455.x
Ladha, J. K., Pathak, H., Krupnik, T. J., Six, J., Van Kessel, C. (2005). Efficiency of fertilizer nitrogen in cereal production: retrospects and prospects. Adv. Agro. 87, 85–156. doi: 10.1016/S0065-2113(05)87003-8
Ladha, J. K., Tirol-Padre, A., Reddy, C. K., Cassman, K. G., Verma, S., Powlson, D. S., et al. (2016). Global nitrogen budgets in cereals: a 50-year assessment for maize, rice, and wheat production systems. Sci. Rep. 6, 1–9. doi: 10.1038/srep19355
Leigh, J., Hodge, A., Fitter, A. H. (2009). Arbuscular mycorrhizal fungi can transfer substantial amounts of nitrogen to their host plant from organic material. New Phytol. 181, 199–207. doi: 10.1111/j.1469-8137.2008.02630.x
Lekberg, Y., Helgason, T. (2018). In situ mycorrhizal function - knowledge gaps and future directions. New Phytol. 220, 957–962. doi: 10.1111/nph.15064
Liu, W., Jiang, S. S., Zhang, Y. L., Yue, S. C., Christie, P., Murray, P. J., et al. (2014). Spatiotemporal changes in arbuscular mycorrhizal fungal communities under different nitrogen inputs over a 5-year period in intensive agricultural ecosystems on the north china plain. FEMS Microbiol. Ecol. 90, 436–453. doi: 10.1111/1574-6941.12405
Luginbuehl, L. H., Oldroyd, G. E. D. (2017). Understanding the arbuscule at the heart of endomycorrhizal symbioses in plants. Curr. Biol. 27, R952–R963. doi: 10.1016/j.cub.2017.06.042
Masclaux-Daubresse, C., Daniel-Vedele, F., Dechorgnat, J., Chardon, F., Gaufichon, L., Suzuki, A. (2010). Nitrogen uptake, assimilation and remobilization in plants: challenges for sustainable and productive agriculture. Ann. Bot. 105, 1141–1157. doi: 10.1093/aob/mcq028
McGonigle, T. P., Miller, M. H., Evans, D. G., Fairchild, G. L., Swan, J. A. (1990). A new method which gives an objective-measure of colonization of roots by vesicular arbuscular mycorrhizal fungi. New Phytol. 115, 495–501. doi: 10.1111/j.1469-8137.1990.tb00476.x
Mensah, J. A., Koch, A. M., Antunes, P. M., Kiers, E. T., Hart, M., Bucking, H. (2015). High functional diversity within species of arbuscular mycorrhizal fungi is associated with differences in phosphate and nitrogen uptake and fungal phosphate metabolism. Mycorrhiza 25, 533–546. doi: 10.1007/s00572-015-0631-x
Paszkowski, U., Jakovleva, L., Boller, T. (2006). Maize mutants affected at distinct stages of the arbuscular mycorrhizal symbiosis. Plant J. 47, 165–173. doi: 10.1111/j.1365-313X.2006.02785.x
Pretty, J. (2008). Agricultural sustainability: concepts, principles and evidence. Philos. Trans. R. Soc. Lond., B, Biol. Sci. 363, 447–465. doi: 10.1098/rstb.2007.2163
Pretty, J. (2018). Intensification for redesigned and sustainable agricultural systems. Science 362, 908–90+. doi: 10.1126/science.aav0294
Reynolds, H. L., Hartley, A. E., Vogelsang, K. M., Bever, J. D., Schultz, P. A. (2005). Arbuscular mycorrhizal fungi do not enhance nitrogen acquisition and growth of old-field perennials under low nitrogen supply in glasshouse culture. New Phytol. 167, 869–880. doi: 10.1111/j.1469-8137.2005.01455.x
Rillig, M. C., Aguilar-Trigueros, C. A., Camenzind, T., Cavagnaro, T. R., Degrune, F., Hohmann, P., et al. (2019). Why farmers should manage the arbuscular mycorrhizal symbiosis. New Phytol. 222, 1171–1175. doi: 10.1111/nph.15602
Rillig, M. C., Sosa-Hernandez, M. A., Roy, J., Aguilar-Trigueros, C. A., Valyi, K., Lehmann, A. (2016). Towards an integrated mycorrhizal technology: harnessing mycorrhiza for sustainable intensification in agriculture. Front. Plant Sci. 7, 1–5. doi: 10.3389/fpls.2016.01625
Ryan, M. H., Graham, J. H. (2018). Little evidence that farmers should consider abundance or diversity of arbuscular mycorrhizal fungi when managing crops. New Phytol. 220, 1092–1107. doi: 10.1111/nph.15308
Sanders, F. E., Tinker, P. B. (1973). Phosphate inflow into mycorrhizal roots. Pestic. Sci. 4, 385–395. doi: 10.1002/ps.2780040316
Smith, F. A., Smith, S. E. (2011a). What is the significance of the arbuscular mycorrhizal colonisation of many economically important crop plants? Plant Soil 348, 63–79. doi: 10.1007/s11104-011-0865-0
Smith, G. S., Johnston, C. M., Cornforth, I. S. (1983). Comparison of nutrient solutions for growth of plants in sand culture. New Phytol. 94, 537–548. doi: 10.1111/j.1469-8137.1983.tb04863.x
Smith, S. E., Smith, F. A. (2011b). Roles of arbuscular mycorrhizas in plant nutrition and growth: new paradigms from cellular to ecosystem scales. Annu. Rev. Plant Biol. 62, 227–250. doi: 10.1146/annurev-arplant-042110-103846
Smith, S. E., Smith, F. A., Jakobsen, I. (2003). Mycorrhizal fungi can dominate phosphate supply to plants irrespective of growth responses. Plant Physiol. 133, 16–20. doi: 10.1104/pp.103.024380
Smith, S. E., Smith, F. A., Jakobsen, I. (2004). Functional diversity in arbuscular mycorrhizal (AM) symbioses: the contribution of the mycorrhizal P uptake pathway is not correlated with mycorrhizal responses in growth or total P uptake. New Phytol. 162, 511–524. doi: 10.1111/j.1469-8137.2004.01039.x
Sosa-Hernandez, M. A., Roy, J., Hempel, S., Kautz, T., Kopke, U., Uksa, M., et al. (2018). Subsoil arbuscular mycorrhizal fungal communities in arable soil differ from those in topsoil. Soil Biol. Biochem. 117, 83–86. doi: 10.1016/j.soilbio.2017.11.009
Staddon, P. L., Fitter, A. H., Graves, J. D. (1999). Effect of elevated atmospheric co2 on mycorrhizal colonization, external mycorrhizal hyphal production and phosphorus inflow in Plantago lanceolata and Trifolium repens in association with the arbuscular mycorrhizal fungus Glomus mosseae. Global Change Biol. 5, 347–358. doi: 10.1046/j.1365-2486.1999.00230.x
Staddon, P. L., Ramsey, C. B., Ostle, N., Ineson, P., Fitter, A. H. (2003). Rapid turnover of hyphae of mycorrhizal fungi determined by AMS microanalysis of 14C. Science 300 (5622), 1138–1140. doi: 10.1126/science.1084269
Storer, K., Coggan, A., Ineson, P., Hodge, A. (2018). Arbuscular mycorrhizal fungi reduce nitrous oxide emissions from N2O hotspots. New Phytol. 220, 1285–1295. doi: 10.1111/nph.14931
Svenningsen, N. B., Watts-Williams, S. J., Joner, E. J., Battini, F., Efthymiou, A., Cruz-Paredes, C., et al. (2018). Suppression of the activity of arbuscular mycorrhizal fungi by the soil microbiota. ISME J. 12, 1296–1307. doi: 10.1038/s41396-018-0059-3
Thirkell, T. J., Cameron, D. D., Hodge, A. (2016). Resolving the ‘nitrogen paradox’ of arbuscular mycorrhizas: fertilization with organic matter brings considerable benefits for plant nutrition and growth. Plant Cell Environ. 39, 1683–1690. doi: 10.1111/pce.12667
Thirkell, T. J., Charters, M. D., Elliott, A. J., Sait, S. M., Field, K. J. (2017). Are mycorrhizal fungi our sustainable saviours? Considerations for achieving food security. J. Ecol. 105, 921–929, 2745.12788. doi: 10.1111/1365-2745.12788
Tinker, P. B., Nye, P. H. (2000). Solute movement in the rhizosphere. Oxford University Press: Oxford.
Tisserant, E., Kohler, A., Dozolme-Seddas, P., Balestrini, R., Benabdellah, K., Colard, A., et al. (2012). The transcriptome of the arbuscular mycorrhizal fungus Glomus intraradices (DAOM 197198) reveals functional tradeoffs in an obligate symbiont. New Phytol. 193, 755–769. doi: 10.1111/j.1469-8137.2011.03948.x
UKSO. 2016. Soilscapes for England and Wales [Online]. Available: http://mapapps2.bgs.ac.uk/ukso/home.html [Accessed 20/08 2016].
Verbruggen, E., Roling, W. F. M., Gamper, H. A., Kowalchuk, G. A., Verhoef, H. A., Van Der Heijden, M. G. A. (2010). Positive effects of organic farming on below-ground mutualists: large-scale comparison of mycorrhizal fungal communities in agricultural soils. New Phytol. 186, 968–979. doi: 10.1111/j.1469-8137.2010.03230.x
Watts-Williams, S. J., Cavagnaro, T. R. (2015). Using mycorrhiza-defective mutant genotypes of non-legume plant species to study the formation and functioning of arbuscular mycorrhiza: a review. Mycorrhiza 25, 587–597. doi: 10.1007/s00572-015-0639-2
Whiteside, M. D., Digman, M. A., Gratton, E., Treseder, K. K. (2012a). Organic nitrogen uptake by arbuscular mycorrhizal fungi in a boreal forest. Soil Biol. Biochem. 55, 7–13. doi: 10.1016/j.soilbio.2012.06.001
Whiteside, M. D., Garcia, M. O., Treseder, K. K. (2012b). Amino acid uptake in arbuscular mycorrhizal plants. Plos One 7, 1–4. doi: 10.1371/journal.pone.0047643
Williams, A., Manoharan, L., Rosenstock, N. P., Olsson, P. A., Hedlund, K. (2017). Long-term agricultural fertilization alters arbuscular mycorrhizal fungal community composition and barley (Hordeum vulgare) mycorrhizal carbon and phosphorus exchange. New Phytol. 213, 874–885. doi: 10.1111/nph.14196
Zadoks, J. C., Chang, T. T., Konzak, C. F,. (1974). A decimal code for the growth stages of cereals. Weed Res. 14 (6), 415–421. doi: 10.1111/j.1365-3180.1974.tb01084.x
Keywords: arbuscular mycorrhiza, nitrogen, barley, field trial, plant ecophysiology
Citation: Thirkell T, Cameron D and Hodge A (2019) Contrasting Nitrogen Fertilisation Rates Alter Mycorrhizal Contribution to Barley Nutrition in a Field Trial. Front. Plant Sci. 10:1312. doi: 10.3389/fpls.2019.01312
Received: 20 May 2019; Accepted: 20 September 2019;
Published: 30 October 2019.
Edited by:
Paola Bonfante, University of Turin, ItalyReviewed by:
Jan Jansa, Institute of Microbiology (ASCR), CzechiaErik Verbruggen, University of Antwerp, Belgium
Copyright © 2019 Thirkell, Cameron and Hodge. This is an open-access article distributed under the terms of the Creative Commons Attribution License (CC BY). The use, distribution or reproduction in other forums is permitted, provided the original author(s) and the copyright owner(s) are credited and that the original publication in this journal is cited, in accordance with accepted academic practice. No use, distribution or reproduction is permitted which does not comply with these terms.
*Correspondence: Tom Thirkell, dC5qLnRoaXJrZWxsQGxlZWRzLmFjLnVr
†Present address: Tom Thirkell, Centre for Plant Sciences, University of Leeds, United Kingdom