- 1School of Life Science, Anhui Agricultural University, Hefei, China
- 2State Key Laboratory of Tea Plant Biology and Utilization, Anhui Agricultural University, Hefei, China
Polyphenols play an important role in the astringent taste of tea [Camellia sinensis (L.)] infusions; catechins in phenolic compounds are beneficial to health. The biosynthesis of gallic acid (GA), a precursor for polyphenol synthesis, in tea plants remains unknown. It is well known that 3-dehydroquinate dehydratase/shikimate dehydrogenase (DQD/SDH) is a key enzyme for catalyzing the conversion of 3-dehydroshikimate (3-DHS) to shikimate (SA); it also potentially participates in GA synthesis in a branch of the SA pathway. In this study, four CsDQD/SDH proteins were produced in Escherichia coli. Three CsDQD/SDHs had 3-DHS reduction and SA oxidation functions. Notably, three CsDQD/SDHs showed individual differences between the catalytic efficiency of 3-DHS reduction and SA oxidation; CsDQD/SDHa had higher catalytic efficiency for 3-DHS reduction than for SA oxidation, CsDQD/SDHd showed the opposite tendency, and CsDQD/SDHc had almost equal catalytic efficiency for 3-DHS reduction and SA oxidation. In vitro, GA was mainly generated from 3-DHS through nonenzymatic conversion. Quantitative reverse transcriptase polymerase chain reaction (qRT-PCR) analysis showed that CsDQD/SDHc and CsDQD/SDHd expression was correlated with GA and 1-O-galloyl-β-D-glucose accumulation in C. sinensis. These results revealed the CsDQD/SDHc and CsDQD/SDHd genes are involved in GA synthesis. Finally, site-directed mutagenesis exhibited the mutation of residues Ser-338 and NRT to Gly and DI/LD in the SDH unit is the reason for the low activity of CsDQD/SDHb for 3-DHS reduction and SA oxidation.
Introduction
The shikimate (SA) pathway contributes to the production of a wide range of intermediates and aromatic amino acids vital for secondary metabolites and protein biosynthesis of microorganisms and plants. The SA pathway in plants consists of seven enzymatic reaction steps, beginning with the condensation of phosphoenolpyruvate (PEP) and erythrose 4-phosphate to form 3-deoxy-d-arabino-heptulosonate 7-phosphate (DAHP) and ending with the synthesis of chorismate from 5-enolpyruvylshikimate 3-phosphate (EPSP) (Figure 1) (Sprenger, 2006; Bentley and Haslam, 2008). Chorismic acid, the end-product of SA pathway, is essential for the formation of aromatic amino acids (l-tyrosine, l-tryptophan, and l-phenylalanine), which can be further catalyzed into several secondary metabolites, such as phenylalanine, lignin, flavonoids, chlorogenic acid, indole acetic acid, and alkaloids (Sprenger, 2006; Maeda and Dudareva, 2012). Tyrosine and phenylalanine are two crucial precursors that can be catalyzed by phenylalanine ammonia-lyase (PAL) to activate the phenylalanine pathway and accumulate flavonoids, polyphenols, and lignin. Furthermore, increasing FaSKDH expression can activate the phenylpropanoid pathway to promote flavonoid and polyphenol accumulation in strawberry fruit (Xu et al., 2014; Nagpala et al., 2016).
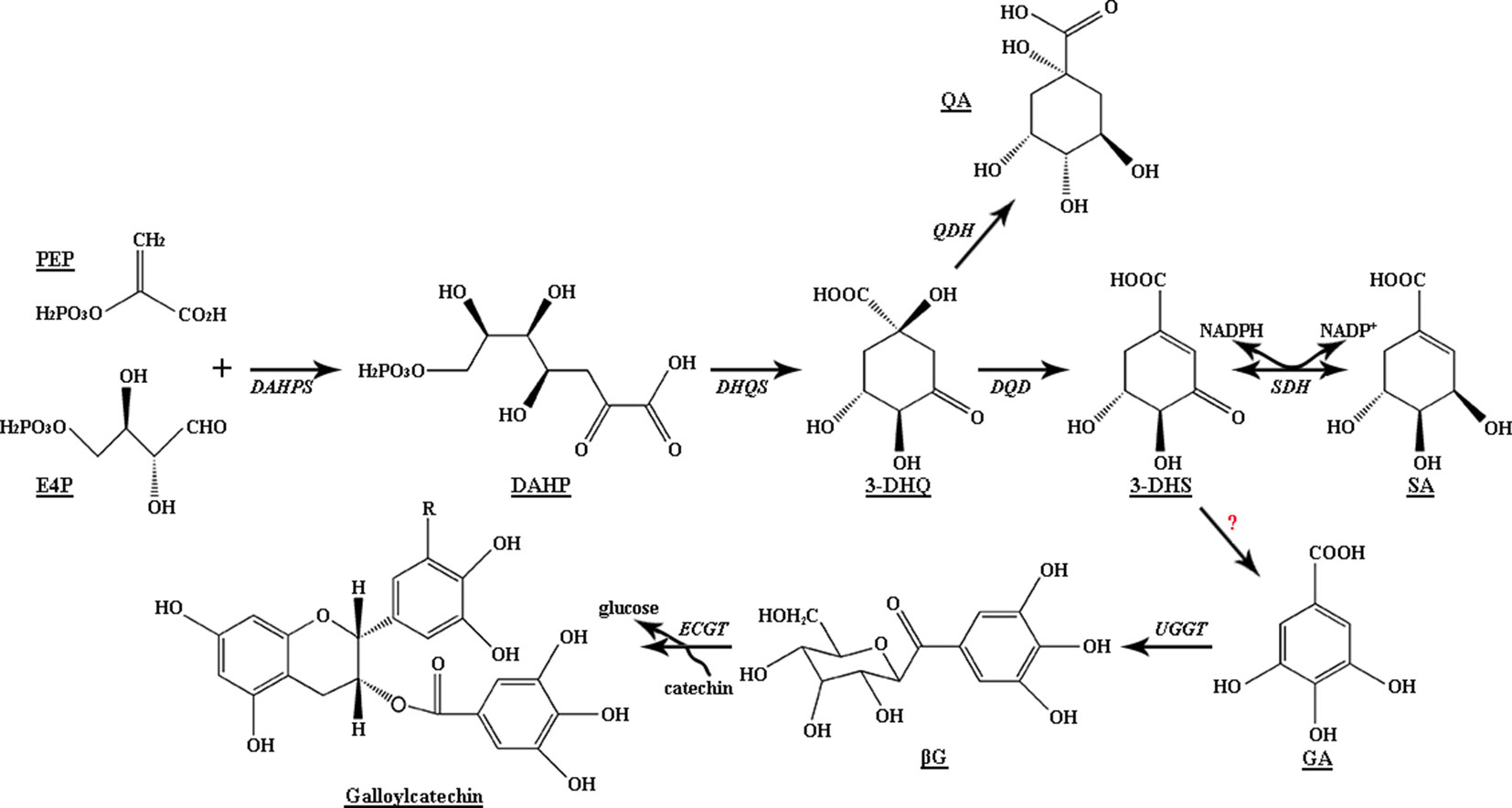
Figure 1 Biosynthetic pathway of gallic acid and shikimic acid in plants. DAHPS, 3-deoxy-D-arabino-heptulosonate 7-phosphate synthase; DHQS, 3-dehydroquinate synthase; DQD, 3-dehydroquinate dehydratase; SDH, SA dehydrogenase; QDH, quinate dehydrogenase; UGGT, UDP-glucose: gallate 1-O-galloyltransferase; ECGT, epicatechin: 1-O-galloyl-β-D-glucose O-galloyltransferase.
The SA pathway is important to plant growth, development, and defense; and silencing the DAHP synthase gene (DAHPS) or 3-dehydroquinate dehydratase/shikimate dehydrogenase (DQD/SDH) in potato, tomato, and tobacco plants results in slow growth and reduces the production of secondary metabolites, such as lignin and chlorogenic acid (Dyer et al., 1989; Kasama et al., 2007). In plants, DQD/SDH, a bifunctional enzyme, is crucial in the third and fourth reversible reactions in the SA pathway; SA can be formed from 3-dehydroshikimate (3-DHS) and NADPH under catalysis by DQD/SDH, and 3-DHS can also be generated from SA and NADP+ under catalysis by DQD/SDH (Kasama et al., 2007; Maeda and Dudareva, 2012). Analysis of the crystal structure of Arabidopsis DQD/SDH showed the functional difference between DQD and SDH sites, which bind with the substrates SA and tartrate to form complexes (Singh, 2006), respectively. Metabolic flux through the SA pathway can be controlled by increasing the effective concentration of the intermediate 3-DHS at each of the two sites. The ratio of SDH to DQD catalytic efficiency is 9:1; therefore, 3-DHS can be quickly converted to SA without accumulation in the stroma of spinach chloroplasts (Fiedler and Schultz, 1985).
Polyphenols in tea plants, including phenolic acids, catechins, and flavonol derivatives, not only determine the mouthfeel of tea infusions but also provide health benefits. Tea plants have abundant ester catechin and epigallocatechin gallate (EGCG) content, which range up to 12%. Gallic acid (GA) is an essential precursor for galloylated catechin biosynthesis (Figure 1), and the amount of GA is a key limiting factor for the formation of EGCG and epicatechin gallate (ECG) (Liu et al., 2012). In addition, GA derivatives, including hydrolysable tannins (HTs) and mainly galloylquinic acid (GQA), highly accumulate in vegetables, Rhus typhina, and Camellia sinensis (Haslam and Cai, 1994; Niemetz and Gross, 2005; Jiang et al., 2013). They are responsible for the unique flavor of plant-derived foods and show a wide range of biological activities, including antioxidant, antiviral, anti-inflammatory, antibacterial, anticancer, and immune-regulation activities (Vit et al., 2008; Steinmann et al., 2013).
GA biosynthesis has been studied for more than 50 years since the 1960s (Dewick and Haslam, 1969). By using the isotope-labeling method, studies have provided an indication regarding GA biosynthesis: GA is mainly derived from the dehydrogenation of 3-DHS by the action of SDH to produce 3,5-didehydroshikimate. This compound tautomerizes to form the redox equivalent GA. Studies have also verified this speculation in plants: GA could be synthesized from 3-DHS with NADP+ as the cofactor when the crude enzyme of birch leaf was used (Ossipov et al., 2003). Transgenic Nicotiana tabacum lines overexpressing Juglans regiaSDH exhibited a 500% increase in GA accumulation (Muir et al., 2011). In the persimmon fruits of pollination-constant and nonastringent (PCNA)-type mutants, downregulated SDH expression was correlated with the reduction in epigallocatechin content, which confirmed the correlation of SDH with proanthocyanidin (PA) content (Akagi et al., 2009). Recently, two of the four VvSDH genes, namely, VvSDH3 and VvSDH4, were validated to be involved in GA biosynthesis in Vitis vinifera (Bontpart et al., 2016).
Recently, an early intermediate of the SA pathway, 3-DHS, was identified to be a crucial precursor for GA synthesis in plants. Alternatively, a study found that GA could be produced through the SA pathway and phenylpropanoid pathway simultaneously. Ishikura and coworkers revealed that GA was derived from not only labeled SA in young leaves but also labeled l-phenylalanine in mature and autumn leaves of Acer buergerianum and Rhus succedanea (Ishikura et al., 1984); this finding indicated that the biosynthetic pathway of GA is diverse and changes according to the developmental stage of the plant.
Studies related to the key gene involved in GA biosynthesis in tea plants are scant. In this study, we screened out four CsDQD/SDH genes from the tea genome (designated as CsDQD/SDHa, CsDQD/SDHb, CsDQD/SDHc, and CsDQD/SDHd). The functions of CsDQD/SDHs expressed in Escherichia coli were surveyed, and after purification, the capacity of recombinant proteins in vitro to produce GA was determined using enzymatic analysis. We also evaluated the relationship between the expression patterns of these four CsDQD/SDH genes and the contents of GA and its derivatives in various organs of tea plants. The results may provide a basis for understanding the biosynthesis of GA and its derivatives.
Methods and Materials
Plant Material
Camellia sinensis cv. Shuchazao (variety approval number: CHN20022008) samples were obtained from the experimental tea garden of Anhui Agricultural University, Hefei, China. Leaves (bud, first leaf, and second leaf), young stems, and tender roots were immediately frozen in liquid nitrogen and stored at −80°C until use.
Chemicals
DL-Dithiothreitol (DTT), ethylenediaminetetraacetic acid (EDTA), NADP+, NADPH, GA, SA, 3-DHS, NaCl, NaOH, Tris, maltose, HCl, isopropyl β-D-thiogalactoside (IPTG), Bis-Tris propane HCl (BTP-HCl), tryphone, and yeast extract were obtained from Sigma Aldrich (St. Louis, MO, USA). Ultra-performance liquid chromatography (UPLC)-grade methanol, acetic acid, acetonitrile, and phosphoric acid were purchased from Tedia Co., Ltd. (Fairfield, OH, USA).
CsDQD/SDH Cloning and Sequence Analysis
Total RNA was isolated from 50 mg of various tea tissues using the RNAiso Plus kit and RNAiso-mate (Takara, Dalian, China), and cDNA was synthesized using the PrimeScript RT Reagent Kit (Takara, Dalian, China) according to the manufacturer’s protocol. The sequences of CsDQD/SDHa (National Center for Biotechnology Information (NCBI) accession number: MH000201, 1,605 bp), CsDQD/SDHb (NCBI accession number: MH000202, 1,560 bp), and CsDQD/SDHc (NCBI accession number: MH000203, 1,599 bp) were obtained from the NCBI database, and the sequence of CsDQD/SDHd (NCBI accession number: MH000204, 1,578 bp) was obtained from the Sequence Read Archive database at NCBI under Bioproject ID PRJNA283013 for Huangjinya. The open reading frames (ORFs) of CsDQD/SDHs were cloned with high-fidelity DNA polymerase using C. sinensis cv. Shuchazao cDNA as the template and were constructed into a pEASY-Blunt Simple Cloning Kit vector (New England Biolabs, MA, USA) to sequence the full-length gene. The forward and reverse primers for cloning into the pMAL-c2x expression vector (New England Biolabs) were inserted to incorporate the restriction site for BamHI and PstI before the start codon and after the stop codon, respectively (primers are listed in Supplementary Table S1).
Multiple DQD/SDH protein sequences (Supplementary Table S2) were obtained from Phytozome (https://phytozome.jgi.doe.gov/pz/portal.html) 12.0 and NCBI (https://www.ncbi.nlm.nih.gov/). Amino acid sequences were aligned, and phylogenetic analysis was performed using DNAMAN 6.0 (Lynnon Corporation, San Ramon, CA, USA), Primer 5.0 (Primer, Canada), and MEGA 5.0 (Mega, Raynham, MA, USA) (Tamura et al., 2011). The tree nodes were statistically evaluated using the bootstrap method, with 1,000 bootstrap replicates conducted. A neighbor-joining tree with the evolutionary distance was computed using the ρ-distance model and protein sequences alignment with a gap open penalty of 10 and a gap extension penalty of 0.2.
CsDQD/SDH Expression in Escherichia coli and Purification of Recombinant Proteins
CsDQD/SDH ORFs were cloned into the pMAL-c2x (New England Biolabs, MA, USA) expression vector and then expressed in E. coli NovaBlue (DE3) competent cells (Novagen, Schwalbach, Germany) to express recombinant proteins, and the proteins purified according to the manufacturer’s protocol (New England Biolabs). The recombinant E. coli strains expressing CsDQD/SDHs were shaken in Luria–Bertani (LB) medium (1 L of LB culture medium containing 5 g of yeast extract, 10 g of tryptone, and 10 g of NaCl, adjusted to pH 7.0 before sterilization) containing 1 µL·ml−1 of ampicillin to an OD600 of 0.6, and the cells were shaken at 37°C at 220 rpm. Thereafter, 1 mmol·L−1 of IPTG was added to the culture medium, and protein expression in strains was induced at 24°C for 24 h. After centrifugation at 7,200 rpm for 15 min, supernatants were discarded, and the precipitates were resuspended in column buffer (200 mM of NaCl, 20 mM of Tris, 1 mM of EDTA, and 1 mM of DTT, pH 7.5). The cells were ultrasonically disrupted for approximately 20 min and then centrifuged at 6,500 rpm for approximately 15 min at 4°C (SCIENTZ-llD, NingBo, China). Supernatants were purified using a maltose column; finally, the target proteins were eluted using maltose column buffer (20 mM of maltose in column buffer, pH 7.5). The target proteins were concentrated using 50-kD ultrafiltration concentration pipes. Recombinant protein concentrations were determined using Coomassie Brilliant Blue G-250, and their concentrations were confirmed by running the proteins on sodium dodecyl sulfate–polyacrylamide gel electrophoresis (SDS-PAGE) electrophoresis gel; they were then stored at −80°C in 50% glycerol.
Enzyme Activity and Product Analysis
To analyze the in vitro activity of the candidate CsDQD/SDHs for 3-DHS reduction and SA oxidation, reactions were conducted in a 100-µL reaction solution consisting of 100 mM of BTP-HCl buffer (pH 7.5), 1 mM of NADPH or NADP+ as the cofactor donor, 1 mM of 3-DHS or 1 mM of SA as the substrate, and 10 µg of purified recombinant CsDQD/SDHs protein at 30°C for 30 min. Reactions were stopped by mixing the reaction solutions with 2.3 M of HCl. Reaction samples lacking recombinant proteins were used as blank controls.
The high-performance liquid chromatography (HPLC) system from Agilent Technologies (RHMo Alto, CA, USA) was used in this study to detect 3-DHS and SA (UV maximum absorption wavelengths were 234 and 211 nm, respectively). The HPLC system comprised a Venusil XBP C18 reverse phase column (4.6 × 251 mm2, Agela Technologies), quaternary pump with a vacuum degasser, thermostated column compartment, and autosampler. The elution profile was as follows: 100% eluent A (1% phosphoric acid in water) for 0–24 min and termination at 25 min at 0.2 mL·min−1 flow rate (eluent B: 100% acetonitrile).
To analyze the in vitro activity of CsDQD/SDHs to produce GA, assays were performed in a buffer containing 100 mM of BTP-HCl buffer (pH 9.0), 1 mM of 3-DHS, 1 mM of NADP+, and 10 µg of purified recombinant CsDQD/SDHs protein at 30°C for 30 min. Reactions were stopped by mixing the reaction solutions with an equal volume of 100% methanol. GA (UV maximum absorption wavelengths were 280 nm) was analyzed using a reverse-phase HPLC LC10Avp system (Shimadzu, Kyoto, Japan). The column was eluted using a mobile phase consisting of eluent A (1% acetic acid) and eluent B (100% acetonitrile) at room temperature. The elution profile was as follows: starting with 100% A (1% acetic acid), a linear gradient from 1% to 5% B (100% acetonitrile) for 0–8 min, 5–10% B for 8–13 min, 10–1% B for 13–14 min, and termination at 15 min at a flow rate of 0.2 ml·min−1 (eluent B: 100% acetonitrile).
All products were analyzed using UPLC–triple quadrupole mass spectrometry (QQQ)–tandem mass spectrometry (MS/MS) with an Agilent 20RBAX RRHD Eclipse Plus C18 column (particle size, 1.8 mm; length, 100 mm; and internal diameter, 2.1 mm) at a flow rate of 0.4 ml·min−1 following previously published protocols (Jiang et al., 2013; Zhao et al., 2017). All reactions in each experiment were three bio-replications.
Enzymatic Kinetic Analysis of Recombinant CsDQD/SDH Proteins
Optimal pH analysis was performed using 3-DHS and SA as substrates at varying pH. The buffers for the pH test were 100 mM of citric acid with pH ranging from 4 to 7, 100 mM of BTP-HCl buffer with pH ranging from 6 to 9, and 100 mM of sodium carbonate with pH ranging from 8 to 11. The reaction was maintained at 30°C for 30 min, and the reaction was stopped by adding 40 µL of 2.3 M of HCl.
In vitro, the kinetic parameters of the recombinant enzymes were obtained from hyperbolic Michaelis–Menten saturation curves for substrates. For the measurement of the KM and Vmax of CsDQD/SDHs, 3-DHS, SA, NADP+, and NADPH were used as acceptor substrates. The linear phase of the reaction was conducted in BTP-HCl buffer (pH 8.5) with 1.5 mM of 3-DHS and NADP+ (0–500 µM), 1.5 mM of NADP+ and 3-DHS (0–500 µM), 1.5 mM of SA and NADPH (0–500 µM), and 1.5 mM of NADPH and SA (0–500 µM) at 30°C for 3 min. Three biological replicates were used per reaction.
Functional Unit Reorganization and Site-Directed Mutagenesis
To identify the key unit and amino acid residues responsible for 3-DHS reduction and SA oxidation, CsDQD/SDHa was categorized as two mutant proteins named CsDQDa (from Lys-91 to Phe-316 in the AtSDH protein sequence) and CsSDHa (from IIe-328 to Gly-588 in the AtSDH protein sequence). Referring to the model of Arabidopsis thaliana protein crystal structure, the amino acid residue sites of Gly-338, Gly-381, Asp-483, Leu-484, and Asp-485 in CsDQD/SDHb were mutated to Ser-338, Thr-381, Asn-483, Arg-484, and Thr-485, respectively; the mutant protein was named MTCsDQD/SDHb.
Site-directed mutagenesis was performed using a gene site-directed mutagenesis kit. The plasmid pMAL-c2X harboring CsDQD/SDHs was used as templates to obtain the site-directed mutants of CsDQDa, CsSDHa, and MTCsDQD/SDHb. Oligonucleotide sequences specifically designed for mutagenesis are listed in Supplementary Table S1.
Purification and analysis of the mutant recombinant proteins were performed using the same protocols as those used for native proteins. The quantitative measurement of the recombinant enzyme products was performed using the aforementioned method, and three biological replicates were used for each experiment.
Expression of GA and GA-Related Compounds and Their Accumulation in Tea Plants
GA, βG, ECG, and EGCG were extracted from various organs of tea plants as follows: 1 g of the dry weight sample (bud, first leaf, second leaf, stem, and root) was ground in liquid nitrogen; it was then extracted with 2 ml of the extraction solution (0.2% HCl added to 80% methanol and 20% water) at room temperature through ultrasonic extraction for 10 min and centrifugation for 15 min at 4,000 rpm (Wang et al., 2018). The precipitate was resuspended in the extraction solution and re-extracted twice as previously described; supernatants were filtered through a 0.22-µm filter membrane. Three biological replicates were analyzed using quantitative reverse transcriptase polymerase chain reaction (qRT-PCR).
GA, βG, ECG, and EGCG contents were detected using an UPLC–MS/MS system equipped with a quaternary pump with a vacuum degasser, thermostated column compartment, autosampler, diode array detector (DAD), and QQQ purchased from Agilent Technologies (Palo Alto, CA, USA). Samples were analyzed using UPLC–QQQ–MS/MS with an Agilent 20RBAX RRHD Eclipse Plus C18 column (particle size, 1.8 mm; length, 100 mm; and internal diameter, 2.1 mm) at a flow rate of 0.4 mL·min−1 following previously published protocols (Jiang et al., 2013; Zhao et al., 2017).
Expression Pattern of CsDQD/SDHs in Tea Plants
The expression levels of glyceraldehyde-3-phosphate dehydrogenase gene (GAPDH), as the reference gene, were standardized against CsDQD/SDHs expression levels. Total RNA was extracted using TRIzol and then reverse transcribed into cDNA. All primers were designed using Primer 5.0 and were detected using PCR. The qRT-PCR system contained 200 ng of cDNA template, 10 µL of IQ SYBR Green Supermix (Takara), and 0.8 µL of each gene-specific primer, and the reaction volume of 20 µL was attained through the addition of RNase-free H2O. The expression levels are represented as a mean value of three replicates. Relative CsDQD/SDH expression was deduced from the cycle threshold (CT) based on the 2−ΔΔCt method. ΔCT = CTtarget − CTinternal standard and −ΔΔCT = −(ΔCTtarget − ΔCTcontrol), where CTtarget and CTinternal standard are the cycle threshold (CT) values for the target and housekeeping genes, respectively. The Pearson correlation coefficient of the CsDQD/SDH expression profiles was also calculated.
Results
Cloning and Protein Sequence Analysis of Four CsDQD/SDH Genes
The four CsDQD/SDH genes were screened from the NCBI and tea genome database and successfully cloned from the tea plant cDNA library. Their ORF lengths are 1,605, 1,560, 1,599, and 1,578 nucleotides, and their encoded proteins are 534, 519, 532, and 525 amino acid residues in length, respectively, with predicted molecular weights of 57.568, 56.411, 58.241, and 56.776 kDa, respectively, and calculated isoelectric points (pIs) of 6.26, 6.1, 6.73, and 6.8, respectively.
Analysis using DNAMAN 6.0 showed that the protein sequence of DQD/SDHs in plants shared 54.17% consistency (Supplementary Figure 1). The amino acid sequence of CsDQD/SDHa shared 64.11% and 66.79% identity with CsDQD/SDHc and CsDQD/SDHd. However, CsDQD/SDHb showed only 49.44%, 48.41%, and 48.67% identity with CsDQD/SDHa, CsDQD/SDHc, and CsDQD/SDHd, respectively.
Some characterized DQD/SDH protein sequences were extracted from the NCBI database and Phytozome 12.0, and a phylogenetic tree was constructed using MEGA 5.0 (Figure 2A).The result showed that the plant DQD/SDH proteins can be divided into five groups. Bontpart et al. (2016) divided VvSDH proteins into four groups. The CsDQD/SDH proteins in this study were divided into four groups, similar to VvSDH proteins. Figure 2B presents the enzyme characteristics of each DQD/SDH protein identified from Arabidopsis thaliana (Singh, 2006), Nicotiana tabacum (Kasama et al., 2007), Vitis vinifera (Bontpart et al., 2016), and Populus trichocarpa (Guo et al., 2014). Relevant studies have indicated that the DQD/SDH proteins in the first, third, and fourth groups derived from showed both 3-DHS reduction and SA oxidation activities. However, the enzymatic activities of several DQD/SDH proteins in the second group could not been detected. VvSDH2 only showed a very low SA oxidation activity (Bontpart et al., 2016). Whether DQD/SDH proteins participate in GA production needs further investigation and supporting evidence, although research has proved that VvSDH3 and VvSDH4 are involved in GA production in vitro (Bontpart et al., 2016).
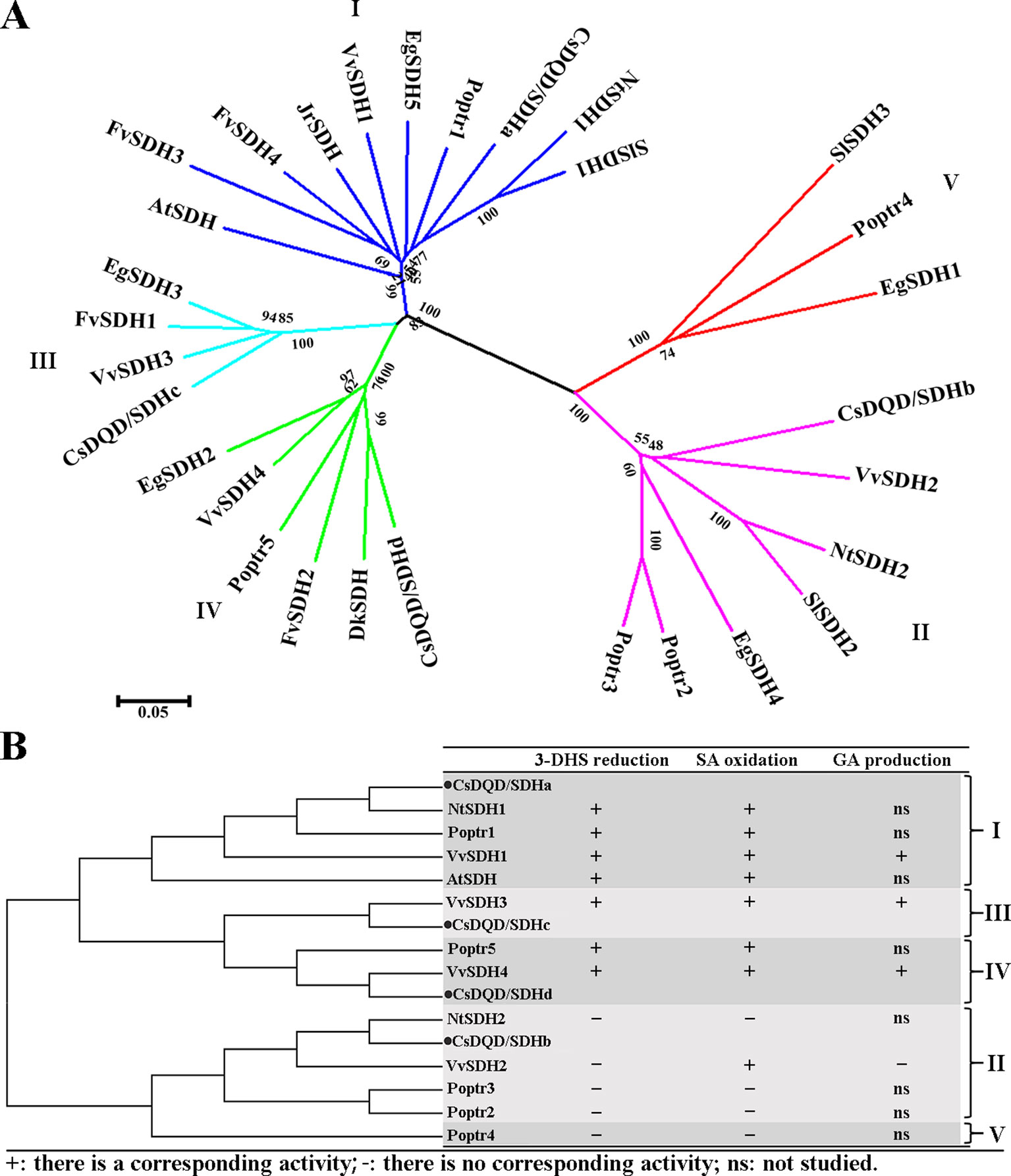
Figure 2 Neighbor-joining tree analysis. (A) A neighbor-joining tree was constructed from the four CsDQD/SDHs sequenced in this study and the 26 sequences available in public databases (NCBI and Phytozome 12) using MEGA 5.0 software. (B) A simplified neighbor-joining tree was constructed to display the enzyme characteristics of each DQD/SDH protein from Arabidopsis thaliana, Nicotiana tabacum, Vitis vinifera, and Populus trichocarpa. NCBI, National Center for Biotechnology Information; DQD/SDH, 3-dehydroquinate dehydratase/shikimate dehydrogenase.
The DQD/SDH protein sequence alignments in Figure 2 show that the plant DQD/SDH proteins consist of two functional units: the DQD unit (Supplementary Figure 2A) and the SDH unit. The DQD unit can catalyze the conversion of 3-dehydroquinate to 3-DHS (Michel et al., 2003; Vogan, 2003), whereas the SDH unit can catalyze the NADPH-dependent reduction of 3-DHS to SA (Padyana and Burley, 2003; Ye et al., 2003). According to the structure of AtDQD/SDH (Singh, 2006), residues Lys-241 and His-214 as well as Arg-279 in the DQD unit function as key catalytic groups and a binding group, respectively. Residues Lys-385 and Asp-423 have been proposed to be a catalytic dyad in the SDH unit, and Ser-336 has been proposed to be its key binding group. Moreover, Asn-483, Arg-484, and Thr-485 formed the NRT motif responsible for binding the cofactor NADP(H). The Ser-338 Ala mutant of AtSDH affected substrate binding and its catalysis. These amino acid residues were conserved in plant DQD/SDHs of the first, third, and fourth groups, except for CsDQD/SDHc (where His-214 was replaced by Gln).
Notably, the corresponding catalytic residues His-214 and Arg-279 in the DQD unit and Ser-338 in the SDH unit of CsDQD/SDHb and VvSDH2 belonging to the second group were replaced by Tyr, Gln, and Gly, respectively. The position of NRT was replaced by DI/LD in CsDQD/SDHb and VvSDH2. These results may contribute to the difficulty in detecting the enzymatic activities of DQD/SDH proteins in the second group.
Enzyme Assays and Product Identification
To detect a recombinant protein activity, the four CsDQD/SDHs were fused to maltose-binding protein and expressed in NovaBlue (DE3) strains. The NADPH-dependent reduction of 3-DHS (Supplementary Figure 3A) and the NADP+-dependent oxidation of SA (Supplementary Figure 3B) by the recombinant proteins of CsDQD/SDHa were measured, and the corresponding products in these reactions were detected quantitatively through UPLC–QQQ–MS/MS (Supplementary Figure 3C). Quantitative results indicated that among these four recombinant proteins, CsDQD/SDHa exhibited the highest 3-DHS reduction and SA oxidation activities, and CsDQD/SDHb exhibited the lowest SA oxidation activity but had no 3-DHS reduction activity (Figure 3).
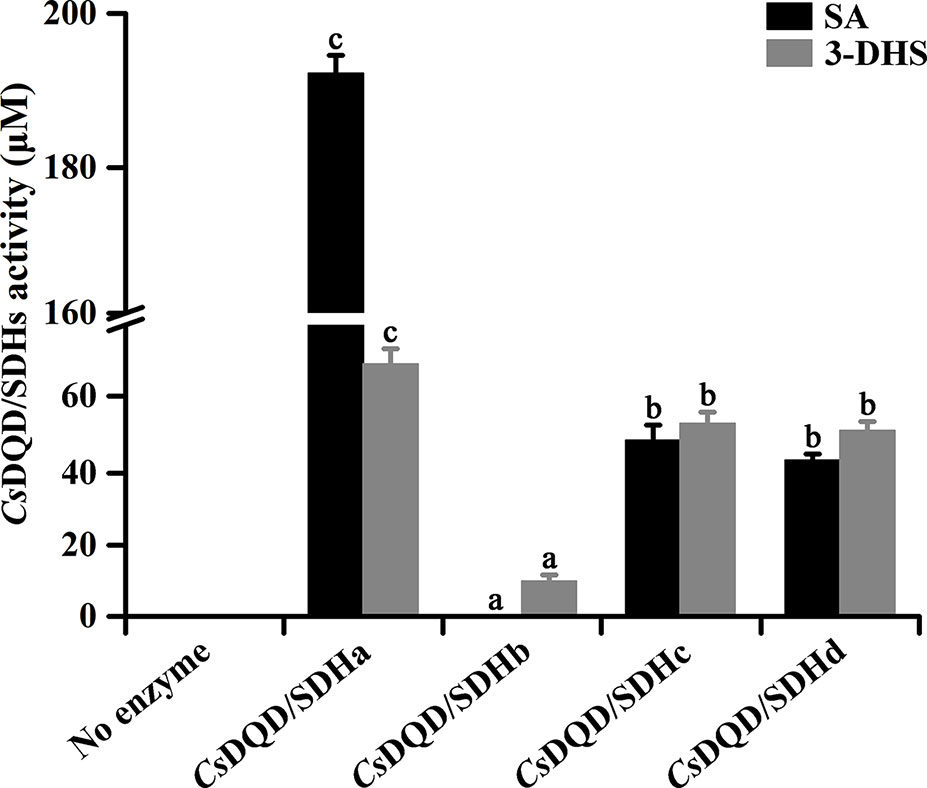
Figure 3 Quantification of 3-DHS and SA production catalyzed by recombinant CsDQD/SDHs in vitro. The reaction products in enzymatic assays were quantified using HPLC (λ3-DHS = 234 nm, λSA = 211 nm). Data are shown as the mean of three replicates ± SD. Different letters represent statistically differ at P < 0.05 according to one-way ANOVA of Duncan’s test. 3-DHS, 3-dehydroshikimate; SA, shikimate; HPLC, high-performance liquid chromatography; ANOVA, analysis of variance.
GA production was found in CsDQD/SDHs assays. SA generation occurred immediately in the 3-DHS reduction reaction catalyzed by CsDQD/SDHa and reached its maximum value after 15 min, whereas GA was detected after 20 min in time course of the reaction (Figure 4A). A similar pattern was observed for SA oxidation by CsDQD/SDHa; the increase in GA content was consistent with the decrease in the product 3-DHS (Figure 4B). On the basis of these results, we speculate that GA may be directly generated from 3-DHS with NADP+ as the coenzyme. However, a significant increase in GA production was not detected in the time course of the CsDQD/SDHa assay when using 3-DHS and the coenzyme NADP+ as substrates (Figure 4C). To further verify that GA was directly generated from 3-DHS, enzymatic or nonenzymatic CsDQD/SDH assay was conducted using 3-DHS and the coenzyme NADP+ as substrates, and the assay revealed GA was directly generated from 3-DHS in the enzymatic SA oxidation reaction (Figure 5).
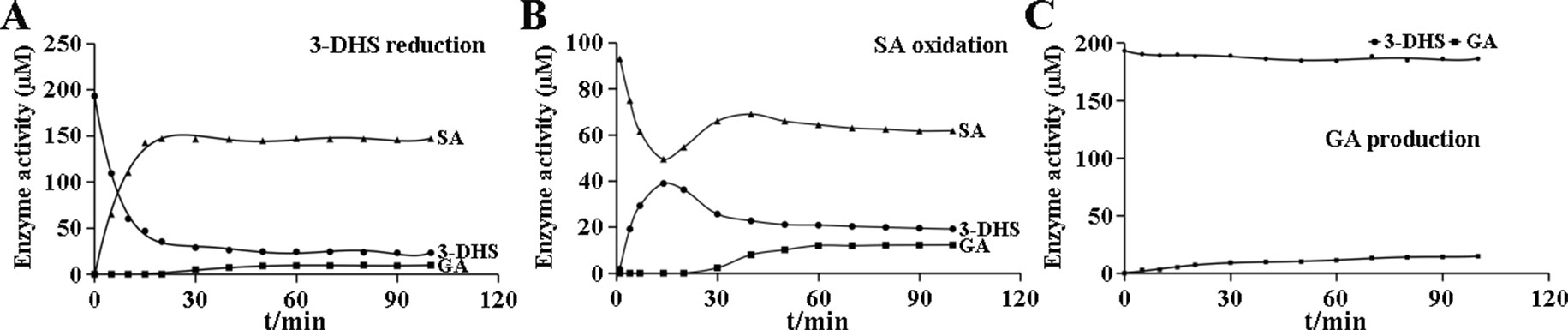
Figure 4 Enzymatic reaction time of the recombinant protein CsDQD/SDHa in vitro. (A) The time course of the 3-DHS reduction reaction catalyzed by the CsDQD/SDHa enzyme. (B) The time course of the SA oxidation catalyzed by the CsDQD/SDHa enzyme. (C) GA production in a reaction catalyzed by the CsDQD/SDHa enzyme. 3-DHS, 3-dehydroshikimate; SA, shikimate; GA, gallic acid.
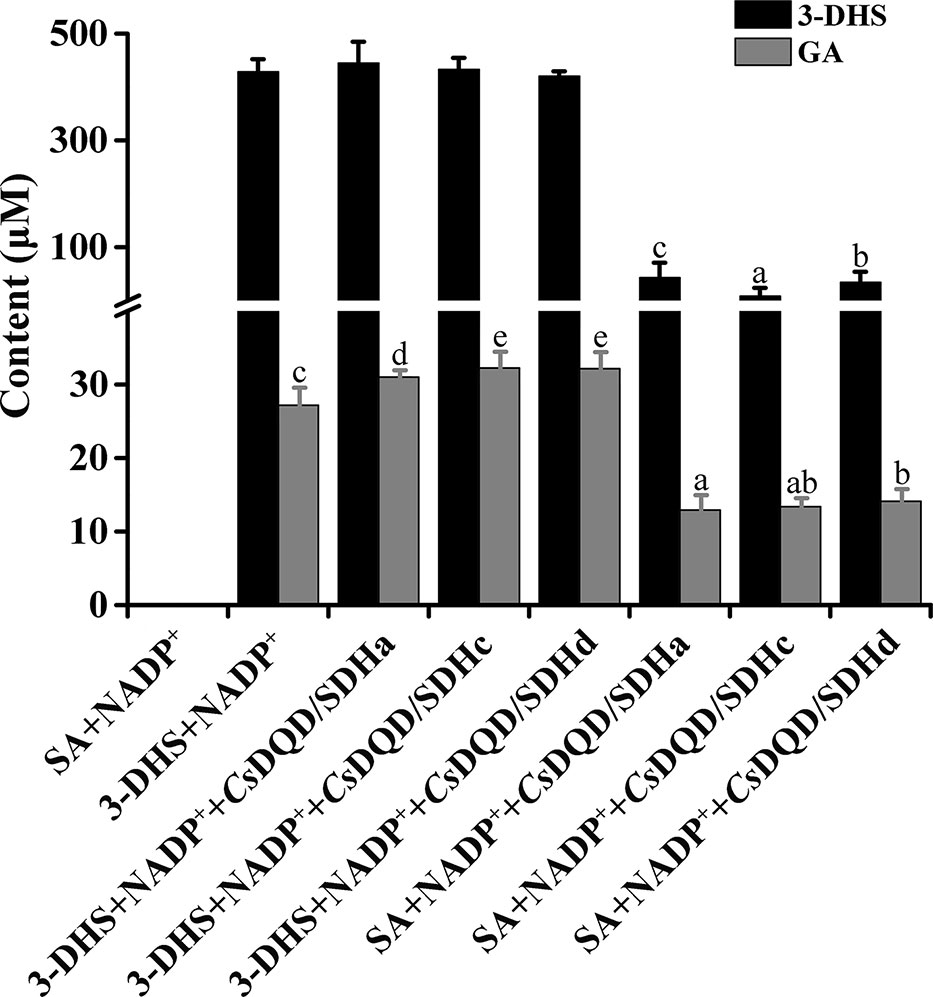
Figure 5 GA generation capacity from CsDQD/SDHa, CsDQD/SDHc, and CsDQD/SDHd and without enzyme as the control. The reaction products in the enzymatic assays were quantified using HPLC (λ3-DHS = 234 nm, λGA = 280 nm). Data are shown as the mean of three replicates ± SD. Different letters represent statistically differ at P < 0.05 according to one-way ANOVA of Duncan’s test. GA, gallic acid; HPLC, high-performance liquid chromatography; ANOVA, analysis of variance.
Determination of Kinetic Parameters
To determine the optimum pH, assays were performed at 30°C for 30 min with citric acid buffer (100 mM, pH 4–7), BTP-HCl buffer (100 mM, pH 6–9), and sodium carbonate buffer (100 mM, pH 8–11), individually. The results showed that the 3-DHS reduction and SA oxidation activities of CsDQD/SDHa, CsDQD/SDHc, and CsDQD/SDHd were higher in alkaline buffer than in acidic buffer (Supplementary Figure 4).
In vitro, the kinetic parameters of both substrates (3-DHS and SA) and cofactors (NADP+ and NADPH) were measured at 30°C for 3 min in a buffer with pH 8.5 (Table 1). For 3-DHS reduction, compared with CsDQD/SDHc (KM(3-DHS) = 330.933 µM) and CsDQD/SDHd (KM(3-DHS = 465.971 µM), CsDQD/SDHa (KM(3-DHS) = 286.576 µM) had the highest affinity for 3-DHS. The catalytic efficiency of CsDQD/SDHa (kcat/KM(3-DHS) = 1,412.281 S−1·M−1) was almost three times more than that of CsDQD/SDHc (kcat/KM(3-DHS) = 506.596 S−1·M−1) and 14 times more than that of CsDQD/SDHd (kcat/KM(3-DHS) = 99.546 S−1·M−1). For SA oxidation, CsDQD/SDHc (KM(SA) = 199.653 µM) showed the highest affinity for the substrate SA in comparison with CsDQD/SDHa (KM(SA) = 272.782 µM) and CsDQD/SDHd (KM(SA) = 610.643 µM). However, the catalytic efficiency of CsDQD/SDHa (kcat/KM(SA) = 562.168 S−1·M−1) and CsDQD/SDHc (kcat/KM(SA) = 535.744 S−1·M−1) were comparable and approximately four times more than that of CsDQD/SDHd (kcat/KM(SA) = 138.482 S−1·M−1). In summary, CsDQD/SDHa compared with CsDQD/SDHc and CsDQD/SDHd had the highest catalytic efficiency for 3-DHS reduction and SA oxidation.
Notably, CsDQD/SDHs showed considerable individual differences between the catalytic efficiency of 3-DHS reduction and SA oxidation; CsDQD/SDHa had higher catalytic efficiency for 3-DHS reduction than for SA oxidation, CsDQD/SDHd showed the opposite tendency, and CsDQD/SDHc had almost equal catalytic efficiency for 3-DHS reduction and SA oxidation. In addition, CsDQD/SDHd had higher affinity for NADP+. These results suggest that CsDQD/SDHc and CsDQD/SDHd function differently in tea plants, such as efficient GA generation.
Truncation and Site-Directed Mutagenesis
To determine the function of the SDH unit and the DQD unit, two units were expressed in Escherichia coli, respectively, and the recombinant proteins were named CsDQDa and CsSDHa. The results of the enzyme assay indicated that compared with the CsDQD/SDHa recombinant protein, the CsSDHa recombinant protein had an almost identical enzymatic activity for NADPH-dependent reduction of 3-DHS and a lower enzymatic activity for NADP+-dependent oxidation of SA, whereas the CsDQDa recombinant protein was completely inactive (Figure 6A).
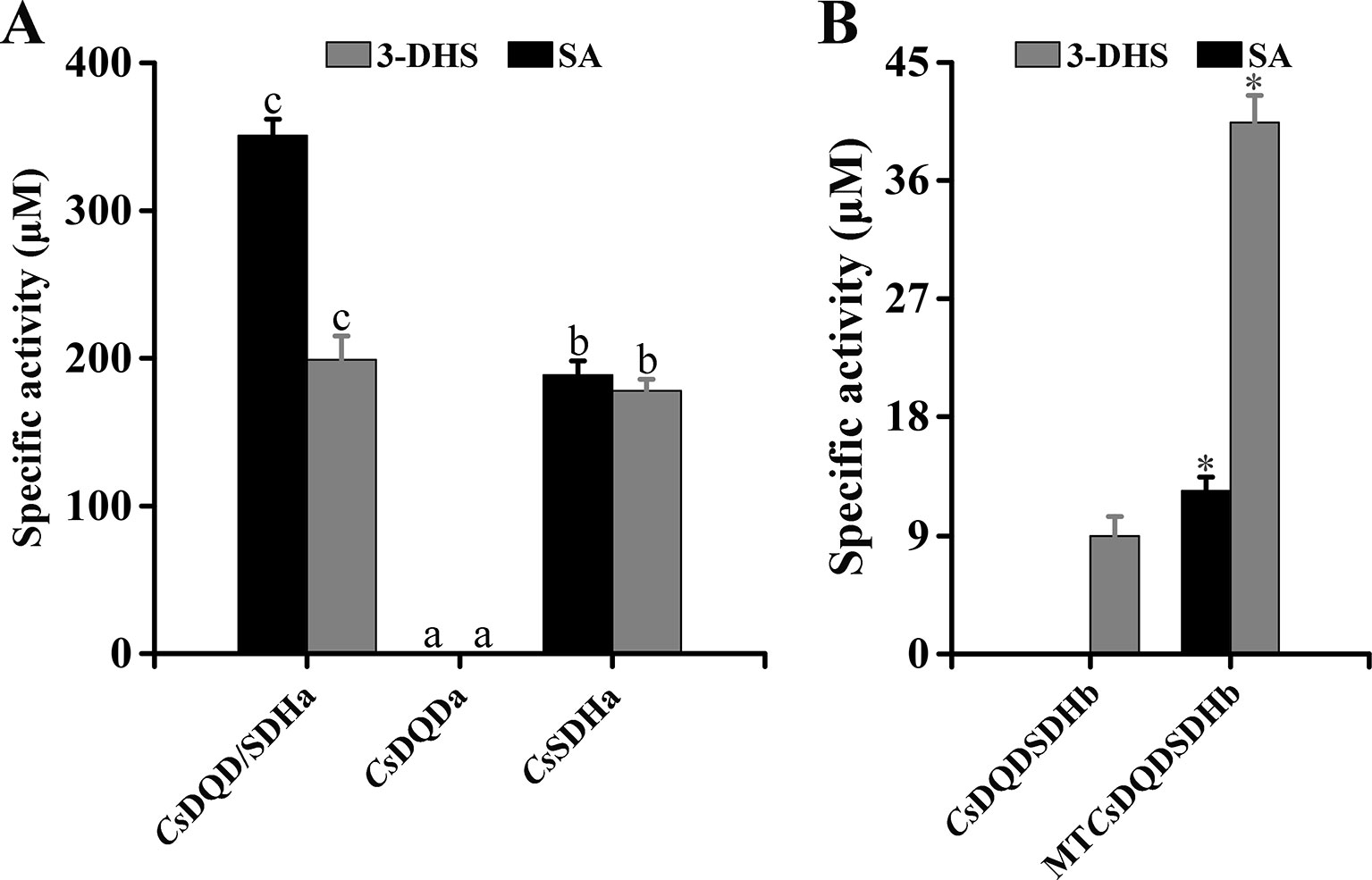
Figure 6 Effect of key unit and site-directed mutagenesis on the activity of CsDQD/SDHa and CsDQD/SDHb, respectively. (A) Specific activity analysis of the CsDQDa and CsSDHa truncated mutant proteins. (B) Specific activity analysis of the purified MTCsDQD/SDHb mutant protein. Data are presented as the means of three independent assays. Data are shown as the mean of three replicates ± SD. Different letters in (A) are statistically different at P < 0.05 according to one-way ANOVA of Duncan’s test. Asterisks in (B) indicate significant difference based on Tukey’s test (P < 0.05). ANOVA, analysis of variance.
The sequence alignments in Supplementary Figure 2 showed that the key residues Ser-338 and NRT in the SDH unit of CsDQD/SDHb were replaced by Gly and DI/LD, respectively; this may be a reason that the enzymatic activities of DQD/SDH proteins in the second group were difficult to detect. To verify this postulation, the substrate and cofactor binding sites of CsDQD/SDHb were analyzed using site-directed mutagenesis. Referring to the model of A. thaliana protein crystal structure, the residue sites of Gly-338, Gly-381, Asp-483, Leu-484, and Asp-485 in CsDQD/SDHb were mutated to Ser-338, Thr-381, Asn-483, Arg-484, and Thr-485, respectively; the mutant protein was named MTCsDQD/SDHb. The results showed that MTCsDQD/SDHb had a similar reduction activity of 3-DHS and had six times higher oxidation activity of SA than CsDQD/SDHb (Figure 6B), suggesting that the mutation of residues Ser-338 and NRT to Gly and DI/LD in the SDH unit is the reason for the low activity of CsDQD/SDHb, respectively.
Expression Pattern of CsDQD/SDHs and Accumulation of GA, βG, ECG, and EGCG in Tea Plants
The relative expression pattern of the four CsDQD/SDHs in diverse plant parts (bud, first leaf, second leaf, stem, and root) was determined using qRT-PCR (Figure 7A). The results revealed that CsDQD/SDHa and CsDQD/SDHb showed similar expression patterns in buds, young leaves, stems, and roots and showed the highest expression in first leaves. CsDQD/SDHc and CsDQD/SDHd were highly expressed in the bud and tender leaves, and their expression decreased from the bud to the root, with the least expression in roots.
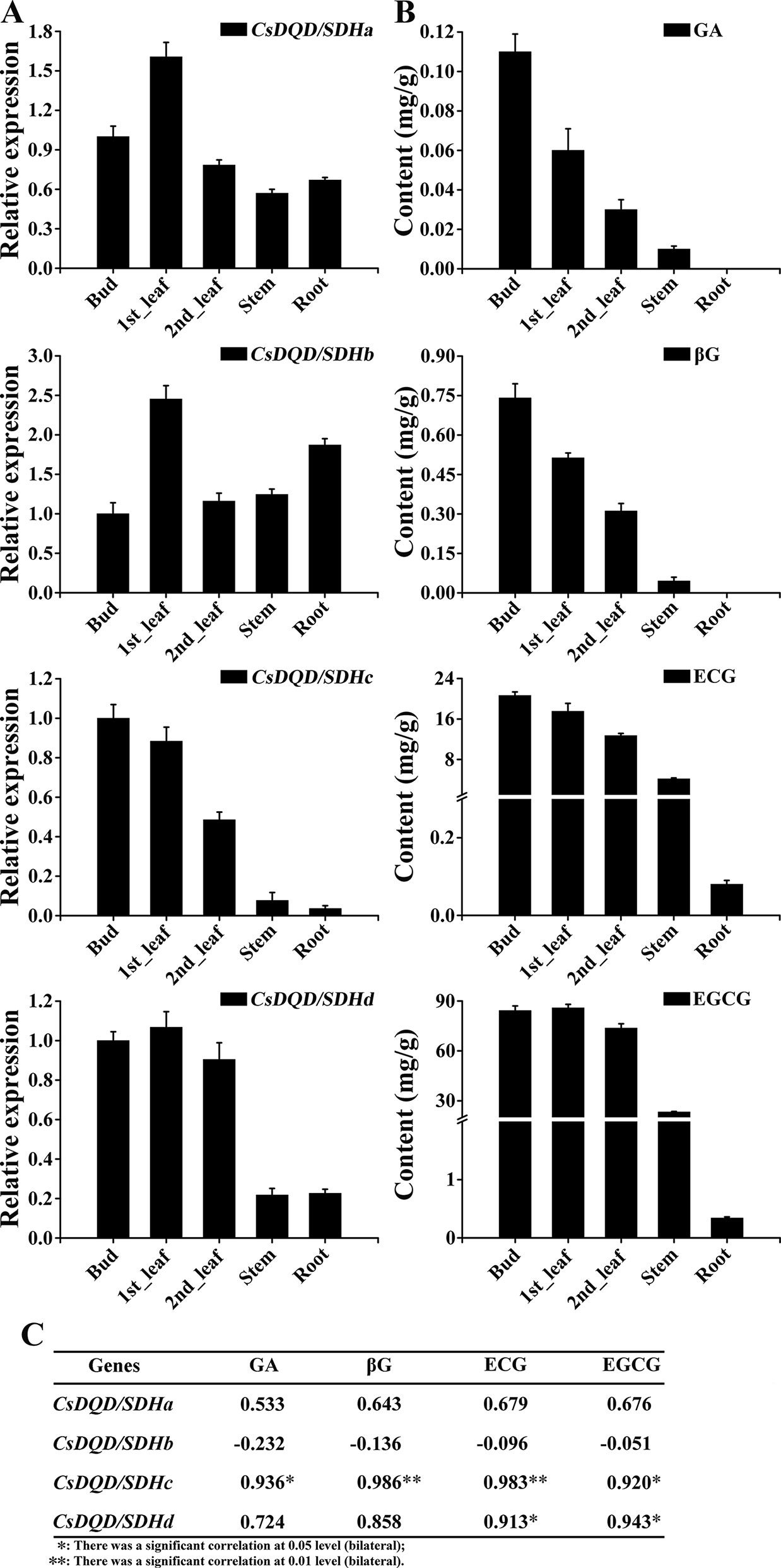
Figure 7 Expression patterns of four CsDQD/SDH genes and the accumulation profiles of GA and its derivatives in different tissues of tea plants. (A) Expression profiles of the CsDQD/SDHa, CsDQD/SDHb, CsDQD/SDHc, and CsDQD/SDHd genes in different organs. (B) Quantitative analysis of GA, βG, ECG, and EGCG contents in different tea organs. Data are presented as the means of three independent assays. (C) Analysis of the Pearson correlation coefficient of the expression pattern of CsDQD/SDHs and the content of GA, βG, ECG, and EGCG. GA, gallic acid; ECG, epicatechin gallate; EGCG, epigallocatechin gallate.
The contents of GA and its glucose ester, βG, were higher in buds and young leaves than in other tissues. A positive correlation was observed between the expression pattern of CsDQD/SDHc and CsDQD/SDHd and the accumulation of GA, βG, ECG, and EGCG (Figure 7B). The Pearson correlation coefficient (Figure 7C) indicating that the expression pattern of CsDQD/SDHc was more positively related to the synthesis of GA, βG, and galloylated catechins.
Discussion
Early studies have also uncovered the importance of the SA pathway in plant secondary compound synthesis, including lignin and pigments such as anthocyanins. Research related to the key gene involved in GA biosynthesis in tea plants is scant but indicates that DQD/SDHs are the candidate target genes. GA serves as a precursor for the biosynthesis of galloyl-type HTs and galloylated PAs (Haslam and Cai, 1994). HTs play crucial roles in tea plant herbivore deterrence and influence tea’s bitterness and astringency. A critical step in HT production is βG generation. For βG formation, CsUGT84A22 requires GA as a substrate (Cui et al., 2016), which is produced from 3-DHS of the SA pathway. In addition, UGT84A23, UGT84A24, and DQD/SDH are localized in the cytoplasm in Punica granatum (Ono, 2016); therefore, it is conceivable that GA production in cytosolic from CsDQD/SDHs may be supplied to CsUGT84A22-catalyzed reactions. PAs, also known as condensed tannins, are polymers of flavan-3-ol units such as catechins and galloylated catechins (Winkel-Shirley, 2001). The galloylated catechins EGCG and PA polymers are the dominant flavonoids in tea leaves and roots, respectively (Jiang et al., 2013; Jiang et al., 2015). Previous studies have shown that a UDP-glycosyltransferases and a serine carboxypeptidase-like (SCPL) acyltransferase participate in the biosynthesis of galloylated flavanol-3-ol and galloyl-type HTs (Gross, 2008).
In plants, DQD/SDH is an essential bifunctional enzyme involved in the SA pathway producing chorismic acid, which is converted into several secondary metabolites (Herrmann, 1995; Maeda and Dudareva, 2012). As a bi-functional or tri-functional enzyme, the DQD/SDH protein plays vital roles in controlling 3-DHS reduction, SA oxidation, and GA synthesis. Plant DQD/SDH belongs to the multigene family and may play different roles in 3-DHS reduction, SA oxidation, and GA synthesis (Kasama et al., 2007). Enzyme–substrate kinetic analysis indicated that CsDQD/SDHa had higher catalytic efficiency for 3-DHS reduction than for SA oxidation; therefore, we can assume that CsDQD/SDHa directs carbon flux towards aromatic acids and biosynthesis in the main trunk of the SA pathway, effectively converting 3-DHS into SA. Ding et al. revealed that RNAi-mediated reduction of NtDHD/SHD-1 (in the first group with CsDQD/SDHa) expression resulted in severe metabolic and phenotypic alterations in tobacco plants (Kasama et al., 2007), highlighting the essentiality of DQD/SDHs (from the first group) for plant growth and development. CsDQD/SDHd showed the opposite tendency compared with CsDQD/SDHa, whereas CsDQD/SDHc had almost equal catalytic efficiency for 3-DHS reduction and SA oxidation. All these findings imply that CsDQD/SDHc and CsDQD/SDHd have different functions compared with CsDQD/SDHa in vivo; for instance, they might be more suitable for SA oxidation and result in continuous GA generation. In grape berry, the highest expression of VvSDH3 and VvSDH4 occurred in parallel with galloylated PA accumulation (Bogs et al., 2005; Kennedy et al., 2010). CsDQD/SDHc and CsDQD/SDHd generate high amounts of galloylated flavan-3-ols in tea plants (Jiang et al., 2013). Moreover, the phenomenon of the consistency of the expression pattern of CsDQD/SDHc and CsDQD/SDHd with the accumulation pattern of GA, βG, gallocatechin gallate (GCG), and EGCG in tea plants showed that the CsDQD/SDHc and CsDQD/SDHd genes are involved in GA synthesis. In addition, DkSDH, which clustered with CsDQD/SDHd into the fourth group, was downregulated in nonastringent persimmon fruits (with low galloylated PA levels) compared with astringent persimmon fruits (Ikegami et al., 2005).
The function of CsDQD/SDH in GA synthesis remains unclear. GA could be spontaneously generated from 3-DHS in the enzymatic or nonenzymatic CsDQD/SDHs assay when using 3-DHS and the coenzyme NADP+ as substrates.
Plant DQD/SDH belongs to the second group, which is unique. In tea plants, among the four proteins, the CsDQD/SDHa, CsDQD/SDHc, and CsDQD/SDHd proteins exhibited a mainstream activity for the substrates 3-DHS and SA with the cofactors of NADPH and NADP+, respectively, whereas CsDQD/SDHb exhibited a very low mainstream activity. Site-directed mutagenesis suggested that mutation of residues Ser-338 and NRT to Gly and DI/LD in the SDH unit, respectively, is the reason for the low activity of CsDQD/SDHb for 3-DHS reduction and SA oxidation. Notably, the Poptr2 and Poptr3 proteins from P. trichocarpa belonging to the second group quinate dehydrogenase (QDH) activity; these proteins are involved in the synthesis of quinate and its derivatives (Guo et al., 2014). GQA, a conjugate of quinic acid (QA) and GA, highly accumulates in tea plants (Jiang et al., 2013). Chlorogenic acid, a QA derivative, is involved in antimicrobial and antiherbivore activities, and it mainly accumulates in the roots of carrot (Cole, 2010b), sweet potato (Mcclure, 1960), and lettuce plants (Cole, 2010a); it is induced by microbe and herbivore infestation. Because of its high sequence identity with Poptr2 and Poptr3, CsDQD/SDHb must be a favorable candidate enzyme for the biosynthesis of QA and its derivatives, and a study examining this postulation is ongoing.
In conclusion, experimental evidence from enzyme assays and kinetic analysis indicates that CsDQD/SDHc and CsDQD/SDHd are favorable candidate genes for GA biosynthesis in tea plants. This study advances our understanding of GA, and its metabolism is tightly connected to the SA pathway.
Data Availability Statement
All datasets for this study are included in the manuscript/Supplementary Files.
Author Contributions
KH, ML, LG, and TX conceived and designed research. KH, ML, YL, and YZ performed the real-time PCR experiments. KH, MZ and GZ analyzed the data. YW, LZ, and XD revised the English writing of the manuscript. YW, LZ, and LG revised the English writing of the manuscript. All authors read and approved the manuscript.
Funding
This work was supported by the National Natural Science Foundation of China (31870676, 31570694, 31470689), National Key Research and Development Program of China (2018YFD1000601), and the Natural Science Foundation of Anhui Province, China (1908085MC100).
Conflict of Interest
The authors declare that the research was conducted in the absence of any commercial or financial relationships that could be construed as a potential conflict of interest.
Supplementary Material
The Supplementary Material for this article can be found online at: https://www.frontiersin.org/articles/10.3389/fpls.2019.01268/full#supplementary-material
References
Akagi, T., Ikegami, A., Suzuki, Y., Yoshida, J., Yamada, M., Sato, A., et al. (2009). Expression balances of structural genes in shikimate and flavonoid biosynthesis cause a difference in proanthocyanidin accumulation in persimmon (Diospyros kaki Thunb.) fruit. Planta 230 (5), 899–915. doi: 10.1007/s00425-009-0991-6
Bentley, R., Haslam, E. (2008). The shipmate pathway—a metabolic tree with many branches. Ccr Crit. Rev. Biochem. 25, 307–384. doi: 10.3109/10409239009090615
Bogs, J., Downey, M. O., Harvey, J. S., Ashton, A. R., Tanner, G. J., Robinson, S. P. (2005). Proanthocyanidin synthesis and expression of genes encoding leucoanthocyanidin reductase and anthocyanidin reductase in developing grape berries and grapevine leaves. Plant Physiol. 139 (2), 652–663. doi: 10.1104/pp.105.064238
Bontpart, T., Marlin, T., Vialet, S., Guiraud, J. L., Pinasseau, L., Meudec, E., et al.(2016). Two shikimate dehydrogenases, VvSDH3 and VvSDH4, are involved in gallic acid biosynthesis in grapevine. J. Exp. Bot. 67 (11), 3537–3550. doi: 10.1093/jxb/erw184
Cole, R. A. (2010a). Phenolic acids associated with the resistance of lettuce cultivars to the lettuce root aphid. Ann. Appl. Biol. 105 (1), 129–145. doi: 10.1111/j.1744-7348.1984.tb02809.x
Cole, R. A. (2010b). Relationship between the concentration of chlorogenic acid in carrot roots and the incidence of carrot fly larval damage. Ann. Appl. Biol. 106 (2), 211–217. doi: 10.1111/j.1744-7348.1985.tb03110.x
Cui, L., Yao, S., Dai, X., Yin, Q., Liu, Y., Jiang, X., et al. (2016). Identification of UDP-glycosyltransferases involved in the biosynthesis of astringent taste compounds in tea (Camellia sinensis). J. Exp. Bot. 67 (8), 2285–2297. doi: 10.1093/jxb/erw053
Dewick, P. M., Haslam, E. (1969). Phenol biosynthesis in higher plants. Gallic acid. Biochem. J. 113 (3), 537. doi: 10.1042/bj1130537
Dyer, W. E., Henstrand, J. M., Handa, A. K., Herrmann, K. M. (1989). Wounding induces the first enzyme of the shikimate pathway in Solanaceae. Proc. Nat. Acad. Sci. U.S.A. 86 (19), 7370–7373. doi: 10.1073/pnas.86.19.7370
Fiedler, E., Schultz, G. (1985). Localization, purification, and characterization of shikimate oxidoreductase-dehydroquinate hydrolyase from stroma of spinach chloroplasts. Plant Physiol. 79 (1), 212–218. doi: 10.1104/pp.79.1.212
Gross, G. G. (2008). From lignins to tannins: Forty years of enzyme studies on the biosynthesis of phenolic compounds. Phytochemistry 69 (18), 3018–3031. doi: 10.1016/j.phytochem.2007.04.031
Guo, J., Carrington, Y., Alber, A., Ehlting, J. (2014). Molecular characterization of quinate and shikimate metabolism in Populus trichocarpa. J. Biol. Chem. 289 (34), 23846–23858. doi: 10.1074/jbc.M114.558536
Haslam, E., Cai, Y. (1994). Plant polyphenols (vegetable tannins): gallic acid metabolism. Nat. Prod. Rep. 11 (1), 41–66. doi: 10.1039/np9941100041
Herrmann, K. M. (1995). The shikimate pathway: early steps in the biosynthesis of aromatic compounds. Plant Cell 7 (7), 907–919. doi: 10.2307/3870046
Ikegami, A., Yonemori, K., Kitajima, A., Sato, A., Yamada, M. (2005). Expression of genes involved in proanthocyanidin biosynthesis during fruit development in a Chinese pollination-constant, nonastringent (PCNA) persimmon, ‘Luo Tian Tian Shi’. J. Am. Soc. Hortic. Sci. Am. Soc. Hortic. Sci. 41 (130), 830. doi: 10.21273/JASHS.130.6.830
Ishikura, N., Hayashida, S., Tazaki, K. (1984). Biosynthesis of gallic and ellagic acids with 14C-labeled compounds in Acer and Rhus leaves. Bot. Mag. = Shokubutsu-gaku-zasshi 97 (3), 355–367. doi: 10.1007/BF02488668
Jiang, X., Liu, Y., Li, W., Zhao, L., Meng, F., Wang, Y., et al. (2013). Tissue-specific, development-dependent phenolic compounds accumulation profile and gene expression pattern in tea plant [Camellia sinensis]. Plos One 8 (4), e62315. doi: 10.1371/journal.pone.0062315
Jiang, X., Liu, Y., Wu, Y., Tan, H., Meng, F., Wang, Y. S., et al. (2015). Analysis of accumulation patterns and preliminary study on the condensation mechanism of proanthocyanidins in the tea plant [Camellia sinensis]. Sci. Rep. 5, 8742. doi: 10.1038/srep08742
Kasama, J. S. T., Ohishi, M., Ding, L., Hofus, D., Hajirezaei, M-R., Ferni, A. R., et al. (2007). Functional analysis of the essential bifunctional tobacco enzyme 3-dehydroquinate dehydratase/shikimate dehydrogenase in transgenic tobacco plants. J. Exp. Bot. 58, 2053–2067. doi: 10.1093/jxb/erm059
Kennedy, J. A., Troup, G. J., Pilbrow, J. R., Hutton, D. R., Hewitt, D., Hunter, C. R., et al. (2010). Development of seed polyphenols in berries from Vitis vinifera L. cv. Shiraz. Aust. J. Grape Wine Res. 6 (3), 244–254. doi: 10.1111/j.1755-0238.2000.tb00185.x
Liu, Y., Gao, L., Liu, L., Yang, Q., Lu, Z., Nie, Z., et al. (2012). Purification and characterization of a novel galloyltransferase involved in catechin galloylation in the tea plant. J. Biol. Chem. 287. doi: 10.1074/jbc.M112.403071
Maeda, H., Dudareva, N. (2012). The shikimate pathway and aromatic amino acid biosynthesis in plants. Annu. Rev. Plant Biol. 63, 73–105. doi: 10.1146/annurev-arplant-042811-105439
Mcclure, T. T. (1960). Chlorogenic acid accumulation and wound healing in sweet potato roots. Am. J. Bot. 47 (4), 277–280. doi: 10.2307/2439607
Michel, G., Roszak, A. W., Sauvé, V., Maclean, J., Matte, A., Coggins, J. R., et al. (2003). Structures of shikimate dehydrogenase AroE and its paralog YdiB. A common structural framework for different activities. J. Biol. Chem. 278 (21), 19463. doi: 10.1074/jbc.M300794200
Muir, R. M., Ibáñez, A. M., Uratsu, S. L., Ingham, E. S., Leslie, C. A., Mcgranahan, G. H., et al. (2011). Mechanism of gallic acid biosynthesis in bacteria (Escherichia coli) and walnut (Juglans regia). Plant Mol. Biol. 75 (6), 555–565. doi: 10.1007/s11103-011-9739-3
Nagpala, E. G., Guidarelli, M., Gasperotti, M., Masuero, D., Bertolini, P., Vrhovsek, U., et al. (2016). Polyphenols variation in fruits of the susceptible strawberry cultivar Alba during ripening and upon fungal pathogen interaction and possible involvement in unripe fruit tolerance. J. Agric. Food Chem. 64, 1869–1878. doi: 10.1021/acs.jafc.5b06005
Niemetz, R., Gross, G. G. (2005). Enzymology of gallotannin and ellagitannin biosynthesis. Phytochemistry 66 (17), 2001–2011. doi: 10.1016/j.phytochem.2005.01.009
Ono, N. N., Xiaoqiong, Q., Wilson, A. E., Gang, L., Li, T., et al. (2016). Two UGT84 family glycosyltransferases catalyze a critical reaction of hydrolyzable tannin biosynthesis in pomegranate (Punica granatum). PLoS One 1–25. doi: 10.1371/journal.pone.0156319
Ossipov, V., Salminen, J. P., Ossipova, S. E., Pihlaja, K. (2003). Gallic acid and hydrolysable tannins are formed in birch leaves from an intermediate compound of the shikimate pathway. Biochem. Syst. Ecol. 31 (1), 3–16. doi: 10.1016/S0305-1978(02)00081-9
Padyana, A. K., Burley, S. K. (2003). Crystal structure of shikimate 5-dehydrogenase (SDH) bound to NADP: insights into function and evolution. Structure 11 (8), 1005–1013. doi: 10.1016/S0969-2126(03)00159-X
Singh, C. A. (2006). Structure of Arabidopsis dehydroquinate dehydratase-shikimate dehydrogenase and implications for metabolic channeling in the shikimate pathway†,‡. Biochemistry 45 (25), 7787. doi: 10.1021/bi060366
Steinmann, J., Buer, J., Pietschmann, T., Steinmann, E. (2013). Anti-infective properties of epigallocatechin-3-gallate (EGCG), a component of green tea. Br. J. Pharmacol. 168 (5), 1059–1073. doi: 10.1111/bph.12009
Tamura, K., Peterson, D., Peterson, N., Stecher, G., Nei, M., Kumar, S. (2011). MEGA5: molecular evolutionary genetics analysis using maximum likelihood, evolutionary distance, and maximum parsimony methods. Mol. Biol. Evol. 28 (10), 2731. doi: 10.1007/0-306-48380-7_2546
Vit, K., Katerina, K., Zuzana, R., Kamil, K., Daniel, J., Ludek, J., et al. (2008). Condensed and hydrolysable tannins as antioxidants influencing the health. Mini Rev. Med. Chem. 8 (5), 436–447. doi: 10.2174/138955708784223486
Vogan, E. (2003). Shikimate dehydrogenase structure reveals novel fold. Structure 11 (8), 902–903. doi: 10.1016/S0969-2126(03)00165-5
Wang, P., Zhang, L., Jiang, X., Dai, X., Xu, L., Li, T., et al. (2018). Evolutionary and functional characterization of leucoanthocyanidin reductases from Camellia sinensis. Planta 247 (1), 139–154. doi: 10.1007/s00425-017-2771-z
Winkel-Shirley, B. (2001). Flavonoid biosynthesis. A colorful model for genetics, biochemistry, cell biology, and biotechnology. Plant Physiol. 126 (2), 485–493. doi: 10.1104/pp.126.2.485
Xu, F., Cao, S. F., Shi, L. Y., Chen, W., Su, X. G., Yang, Z. F. (2014). Blue light irradiation affects anthocyanin content and enzyme activities involved in postharvest strawberry fruit. J. Agric. Food Chem. 62, 4778–4783. doi: 10.1021/jf501120u
Ye, S., Von, D. F., Brooun, A., Knuth, M. W., Swanson, R. V., Mcree, D. E. (2003). The crystal structure of shikimate dehydrogenase (AroE) reveals a unique NADPH binding mode. J. Bacteriol. 185 (14), 4144. doi: 10.1128/JB.185.14.4144-4151.2003
Keywords: Camellia sinensis, 3-dehydroquinate dehydratase/shikimate dehydrogenase, gallic acid, shikimate pathway, site-directed mutagenesis
Citation: Huang K, Li M, Liu Y, Zhu M, Zhao G, Zhou Y, Zhang L, Wu Y, Dai X, Xia T and Gao L (2019) Functional Analysis of 3-Dehydroquinate Dehydratase/Shikimate Dehydrogenases Involved in Shikimate Pathway in Camellia sinensis. Front. Plant Sci. 10:1268. doi: 10.3389/fpls.2019.01268
Received: 17 April 2019; Accepted: 11 September 2019;
Published: 11 October 2019.
Edited by:
Marco Landi, University of Pisa, ItalyReviewed by:
Golam Jalal Ahammed, Henan University of Science and Technology, ChinaJinchi Tang, Guangdong Academy of Agricultural Sciences, China
Antonella Castagna, University of Pisa, Italy
Copyright © 2019 Huang, Li, Liu, Zhu, Zhao, Zhou, Zhang, Wu, Dai, Xia and Gao. This is an open-access article distributed under the terms of the Creative Commons Attribution License (CC BY). The use, distribution or reproduction in other forums is permitted, provided the original author(s) and the copyright owner(s) are credited and that the original publication in this journal is cited, in accordance with accepted academic practice. No use, distribution or reproduction is permitted which does not comply with these terms.
*Correspondence: Tao Xia, eGlhdGFvNjJAMTI2LmNvbQ==; Liping Gao, Z2FvbHA2MkAxMjYuY29t
†These authors have contributed equally to this work and share first authorship