- 1RIKEN Center for Sustainable Resource Science, Yokohama, Japan
- 2Graduate School of Science, University of Tokyo, Bunkyo, Japan
Plant-parasitic nematodes (PPNs), such as root-knot nematodes (RKNs) and cyst nematodes (CNs), are among the most devastating pests in agriculture. RKNs and CNs induce redifferentiation of root cells into feeding cells, which provide water and nutrients to these nematodes. Plants trigger immune responses to PPN infection by recognizing PPN invasion through several different but complementary systems. Plants recognize pathogen-associated molecular patterns (PAMPs) sderived from PPNs by cell surface–localized pattern recognition receptors (PRRs), leading to pattern-triggered immunity (PTI). Plants can also recognize tissue and cellular damage caused by invasion or migration of PPNs through PRR-based recognition of damage-associated molecular patterns (DAMPs). Resistant plants have the added ability to recognize PPN effectors via intracellular nucleotide-binding domain leucine-rich repeat (NLR)-type immune receptors, leading to NLR-triggered immunity. Some PRRs may also recognize apoplastic PPN effectors and induce PTI. Plant immune responses against PPNs include the secretion of anti-nematode enzymes, the production of anti-nematode compounds, cell wall reinforcement, production of reactive oxygen species and nitric oxide, and hypersensitive response–mediated cell death. In this review, we summarize the recognition mechanisms for PPN infection and what is known about PPN-induced immune responses in plants.
Introduction
Plant-parasitic nematodes (PPNs) are among the most devastating agricultural pests worldwide with an annual global crop loss estimated at about 80 billion USD (Jones et al., 2013). PPNs infect a broad host range of commercially important crop families such as the Solanaceae (tomato, potato, pepper), Fabaceae (soybean), Malvaceae (cotton), Amaranthaceae (sugar beet), and Poaceae (syn. Gramineae; rice, wheat, maize). In general, the economically important PPNs have a broad host range and are highly virulent. PPNs may possess sophisticated virulent strategy as they can infect many plants without inducing strong immune responses (Warmerdam et al., 2018). This characteristic feature makes it difficult to isolate mutants of Arabidopsis thaliana that are defective in immunity against PPNs. However, recent progress in plant and nematode genomics has opened a way to understanding the plant’s mechanisms for recognizing PPN infection. There is now a large body of work surrounding the immune, tolerance, and susceptible responses of plant species to nematode infection (summarized in Supplementary Table 1). In this review, we summarize the known plant recognition mechanisms for PPN infection, and the host immune responses to PPN. In addition, we discuss how different recognition systems activate different immune responses.
PPN Life Cycles
PPNs are divided into three major groups according to feeding behavior: ectoparasitic, semi-endoparasitic, and endoparasitic (Decraemer and Hunt, 2013; Palomares-Rius et al., 2017; Smant et al., 2018). Ectoparasitic nematodes spend their entire life cycle outside of the host, with the only physical contact being the insertion of a long and rigid feeding stylet (Figure 1A). Semi-endoparasitic nematodes penetrate roots to feed, with its posterior part remaining in the soil. Endoparasitic nematodes completely enter the root and feed on internal tissues. Each of these feeding types is further divided into either migratory or sedentary lifestyles. For example, migratory endoparasites (e.g., the root-lesion nematodes Pratylenchus spp., and the burrowing nematodes Radopholus spp.) migrate through root tissues to feed on plant cells, causing damage to tissues as they migrate (Figure 1B), whereas sedentary endoparasites move into the vascular cylinder and induce redifferentiation of host cells into multinucleate and hypertrophic feeding cells. The two main PPNs in the sedentary group are the root-knot nematodes (RKNs) in the genus Meloidogyne, and the cyst nematodes (CNs) including the genera Globodera and Heterodera (Figures 1C, D). RKNs and CNs are the most devastating nematodes in the world (Jones et al., 2013).
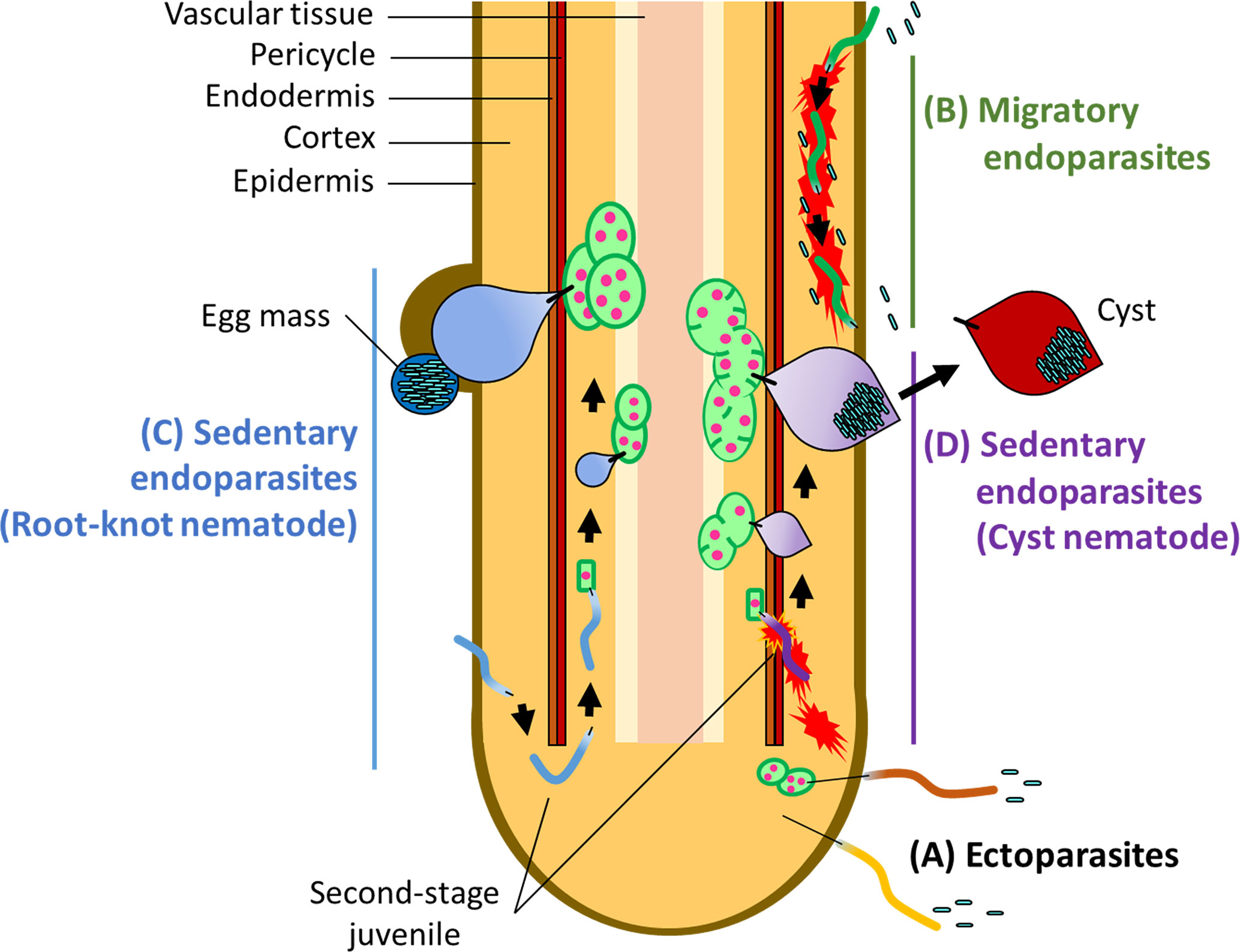
Figure 1 Infection strategies of PPNs (A) Ectoparasites take up nutrients from plant cells without invading the plant root. Some ectoparasites such as needle nematodes (Longidorus spp.) and dagger nematodes (Xiphinema spp.) induce the formation of nurse cells which extends the period of feeding. (B) Migratory endoparasites move through inside of the root tissues causing destruction en route and feed on plant tissues. Sedentary endoparasites include the root-knot nematodes (RKNs), Meloidogyne spp. and the cyst nematodes (CNs), including Globodera spp. and Heterodera spp. (C) Second-stage RKN juveniles enter the root near the root-tip then migrate intercellularly to the vascular cylinder where they reprogram root tissues into giant cells. After establishment of giant cells, RKN juveniles become sedentary and take up nutrients and water through a feeding stylet. Adult RKN females form an egg mass on or below the root surface. (D) Second-stage juveniles of the CNs move inside of the root intracellularly, causing destruction of plant tissues as they go, and establish syncytia in the vascular tissues as feeding cells. CN juveniles also become sedentary and start feeding from syncytia. Adult CN females retain eggs inside of the body, which forms a cyst after death.
Both RKNs and CNs induce host-cell redifferentiation to establish feeding cells for own development and reproduction, but in two different ways. Infective RKN juveniles enter near the root-tip and migrate intercellularly to the vascular cylinder where feeding cells are formed. Once RKNs enter a favorable location, they induce the redifferentiation of plant cells into multinucleate giant cells by repeated nuclear divisions without cytoplasmic division (Abad et al., 2009; Escobar et al., 2015). About 4–6 weeks after infection, the pear-shaped mature adult RKN female lays eggs in a gelatinous egg mass on or below the surface of the root (Abad et al., 2009; Escobar et al., 2015). RKNs exhibit variable reproduction modes such as amphimixis, facultative parthenogenesis and obligate parthenogenesis. In particular, the most devastating RKN species, Meloidogyne incognita, Meloidogyne arenaria, and Meloidogyne javanica, reproduce by obligate parthenogenesis and males appear to have no role in reproduction (Castagnone-Sereno, 2006). CN juveniles enter the root and move intracellularly into the vascular cylinder where, unlike RKNs, they induce syncytia through the local dissolution of cell walls and protoplast fusion of neighboring plant cells. Hundreds of eggs are produced inside of the female body after mating. When the female dies, its body forms a cyst, which can protect the eggs for many years in the soil (Bohlmann and Sobczak, 2014; Bohlmann, 2015). Both RKNs and CNs secrete virulence effectors through a stylet to manipulate host cells for establishing feeding cells. PPNs secrete effectors include cell wall degrading enzymes, inhibitors of anti-nematodal plant enzymes, plant immune signaling suppressors, and proteins required for the establishment of feeding cells (Davis et al., 2008; Gheysen and Mitchum, 2011; Hewezi and Baum, 2013; Goverse and Smant, 2014; Smant et al., 2018; Mejias et al., 2019).
Recognition of PPNs
In general, pathogens are perceived by several different recognition systems in plants (Jones and Dangl, 2006; Dodds and Rathjen, 2010). The first recognition system is mediated by the perception of pathogen-associated molecular patterns (PAMPs) (e.g., bacterial flagellin, fungal chitin) and damage-associated molecular patterns (DAMPs) released by the disrupted host plant tissues. PAMPs and DAMPs are perceived by cell surface–localized pattern recognition receptors (PRRs), leading to pattern-triggered immunity (PTI) (Boutrot and Zipfel, 2017; Hou et al., 2019). Plant PRRs are usually either receptor-like kinases (RLKs) or receptor-like proteins (Boutrot and Zipfel, 2017). Successful pathogens secrete effector proteins into host apoplast and cytoplasm to interfere with recognition and immune signaling. In resistant plants, however, these effectors are often recognized by intracellular nucleotide-binding domain leucine-rich repeat (NLR)-type immune sensors, leading to NLR-triggered immunity (Cui et al., 2015). The N-terminus of NLR proteins usually contains a toll-interleukin 1 receptor (TIR) domain or coiled coil (CC), which are used to classify NLR proteins into two subgroups TIR-NLRs and CC-NLRs. In addition, some PRRs in resistant plants also recognize apoplastic effectors to induce PTI.
PPNs are known to induce PTI in plants. For example, ascaroside, an evolutionarily conserved nematode pheromone, is the first and only nematode PAMP identified so far (Manosalva et al., 2015). Ascr#18, the most abundant ascaroside in PPNs, activates typical plant immune responses, such as mitogen-activated protein kinases, PTI-marker gene expression, and salicylic acid– and jasmonic acid (JA)–mediated defense signaling pathways. Importantly, treatment with Ascr#18 increases resistance to both RKNs and CNs in Arabidopsis. Moreover, Ascr#18 is also recognized by tomato, potato, and barley, suggesting that the recognition of Ascr#18 is well conserved in both monocots and dicots. However, the corresponding PRR for recognizing Ascr#18 has not yet been identified. The first identified PRR involved in the induction of PTI in response to a PPN-derived molecule is a leucine-rich repeat (LRR)-RLK encoded by Arabidopsis Nilr1 (nematode-induced LRR-RLK 1) (Mendy et al., 2017). NILR1 was isolated as an essential component for recognizing “NemaWater,” an aqueous solution incubated with infective-stage juveniles of CN (Heterodera schachtii) and RKN (M. incognita) as PTI inducers. Interestingly, the extracellular receptor domain of NILR1 is widely conserved among dicots and monocots, which is consistent with the fact that NemaWater activates immune responses in tomato, sugar beet, tobacco, and rice. However, the corresponding PAMP molecule recognized by NILR1 has not been identified. The importance of PTI in immunity against PPNs has also been demonstrated in Arabidopsis PTI-deficient mutants (Teixeira et al., 2016; Mendy et al., 2017). The susceptibility of Arabidopsis to RKNs was enhanced in bak1–5 and bik1 mutants (Teixeira et al., 2016). BAK1 is a co-receptor for many PRRs inducing PTI, and in the BIK1 mutant, it is a required receptor-like cytoplasmic kinase for PTI signaling. bak1–5 and bak1–5 bkk1 (BKK1 is the closest homolog of BAK1) mutants are more susceptible to CNs (Mendy et al., 2017). Importantly, RKNs and CNs have multiple virulence effectors that are able to suppress PTI responses (Chen et al., 2013; Jaouannet et al., 2013; Lin et al., 2016; Chen et al., 2018; Naalden et al., 2018; Kud et al., 2019; Yang et al., 2019). PPN infections induce host-cell damage, thus they likely produce DAMP(s), which results in PTI induction. For example, CNs migrate intracellularly, thus their migration results in the release of oligogalacturonides (OGs) from plant cell walls. Fungal pathogens produce cell wall degrading enzymes like polygalacturonase (PG) to digest plant cell wall materials (D’Ovidio et al., 2004), and most plants have polygalacturonase inhibitor proteins (PGIPs) that attenuate pectin degradation by PGs, resulting in OG release. The released long-chain OGs activate PTI (Bishop et al., 1981; Hahn et al., 1981; Nothnagel et al., 1983; Benedetti et al., 2015). Arabidopsis has two PGIPs, PGIP1, and PGIP2, both of which are rapidly expressed during the migratory stage of CNs. A genetic study showed that PGIP1 activates plant camalexin and indole-glucosinolate pathways, thus attenuating CN infection (Shah et al., 2017). In addition, exogenous treatment with OGs enhances resistance against CNs. These results suggest that upon CN infection, Arabidopsis PGIP1 releases OGs, triggering PTI (Shah et al., 2017). Furthermore, CN infection induces ethylene production by the host, a signaling step that delays establishment of the syncytial-phase, indicating that damage-induced ethylene responses contribute to immunity against CNs (Marhavý et al., 2019). In contrast, there is as yet no clear evidence for damage-induced immunity against RKNs, which migrate intercellularly and are thus less-destructive than CNs. For example, neither PGIP1 nor PGIP2 are induced during the migratory stages of RKNs, and PGIP-mediated DAMP responses are not required for resistance against RKNs (Shah et al., 2017). Similarly, the loss of other DAMP receptors, PEPR1 and PEPR2 for plant elicitor peptides or DORN1 for extracellular ATP, fails to affect susceptibility to RKNs (Teixeira et al., 2016). However, it is possible that unknown DAMPs might be important for inducing immunity against RKNs, as PTI activation by exogenous application of known DAMPs is quite effective for suppressing the reproduction of RKNs (Lee et al., 2018).
NLR proteins also play critical roles in recognizing PPNs. NLRs involved in PPN recognition are mostly encoded by resistance (R) genes (Kaloshian et al., 2011). Well-studied R genes include tomato Mi-1.2, Mi-9, and Hero-A; potato Gpa2 and Gro1–4; pepper CaMi; and prune Ma (Milligan et al., 1998; van der Vossen et al., 2000; Ernst et al., 2002; Paal et al., 2004; Chen et al., 2007; Jablonska et al., 2007; Claverie et al., 2011). Mi-1.2, Mi-9, CaMi, and Ma confer resistance against RKNs, while Hero-A, Gpa2, and Gro1–4 provide resistance against CNs. Gro1–4 and Ma encode TIR-NLRs, whereas the others encode CC-NLRs. Interestingly, Ma protein has a large and highly polymorphic C-terminal post-LRR region that is thought to be important for the recognition of PPNs (Claverie et al., 2011). Few examples of PPN avirulence factors recognized by NLRs are known. Gp-RBP-1, one of the secreted SP1a and RYanodine receptor (SPRY) domain (SPRYSEC) proteins from CN Globodera pallida, is an effector that induces hypersensitive response (HR)–cell death in the presence of GPA2 and Ran GTPase-activating protein 2 (RanGAP2) (Blanchard et al., 2005; Sacco et al., 2009). The proline residue at position 187 in the SPRY domain of Gp-RBP-1 is required for recognition by GPA2, whereas the virulent type Gp-RBP-1 variant allele has a mutation at this position, allowing it to avoid host recognition. Moreover, RanGAP2 interacts with the CC domain of GPA2 (Tameling and Baulcombe, 2007), suggesting that the RanGAP2-GPA2 complex is required for the recognition of the SPRY domain of Gp-RBP-1. Other example of an avirulence factor recognized by plants is Cg-1 in M. javanica, an RKN. The Cg-1 gene is present in an Mi-1.2-avirulent population, but virulent RKN strains carry a deletion of Cg-1 (Gleason et al., 2008; Gross and Williamson, 2011). Moreover, silencing of Cg-1 in an avirulent strain increased virulence on Mi-1.2-containing tomato, suggesting a possible role for Cg-1 as a factor recognized by R protein Mi-1.2, although its signal transduction mechanism is unclear.
Surface-localized PRRs are also known to recognize PPN effectors. Venom allergen–like protein Gr-VAP1 from the CN Globodera rostochiensis interacts with apoplastic papain-like cysteine protease (PLCP) RCR3pim in tomato to suppress host immunity (Lozano-Torres et al., 2012). However, Cf-2, a plasma membrane-localized receptor-like protein with extracellular LRRs, recognizes the interaction of Gr-VAP1 with RCR3pim, triggering HR-cell death in resistant hosts. Notably, Cf-2 was originally identified as a resistance gene against the fungal pathogen Cladosporium fulvum (Rooney et al., 2005). Similar to Gr-VAP1, C. fulvum secretes AVR2, which interacts with and inhibits RCR3pim, and this interaction is recognized by Cf-2 protein. Thus, Cf-2 recognizes both fungal and nematode pathogens by monitoring RCR3pim.
Secretion of Anti-Nematode Enzymes Into the Apoplast
The fact that the PPN effector Gr-VAP1 inhibits RCR3pim, a PLCP, implies that its enzymatic activity is important in immunity against PPNs (Figure 2A). Indeed, the absence of RCR3pim homologs in Arabidopsis results in enhanced susceptibility to CN (Lozano-Torres et al., 2014). In addition to Gr-VAP1, Mc1194, an effector of RKN Meloidogyne chitwoodi targets another PLCP, RD21A in Arabidopsis (Davies et al., 2015b). Lack of RD21A leads to hyper-susceptibility to M. chitwoodi, showing that this PLCP also plays a positive role in immunity against RKN. However, it is not yet known how these PLCPs inhibit PPN infection.
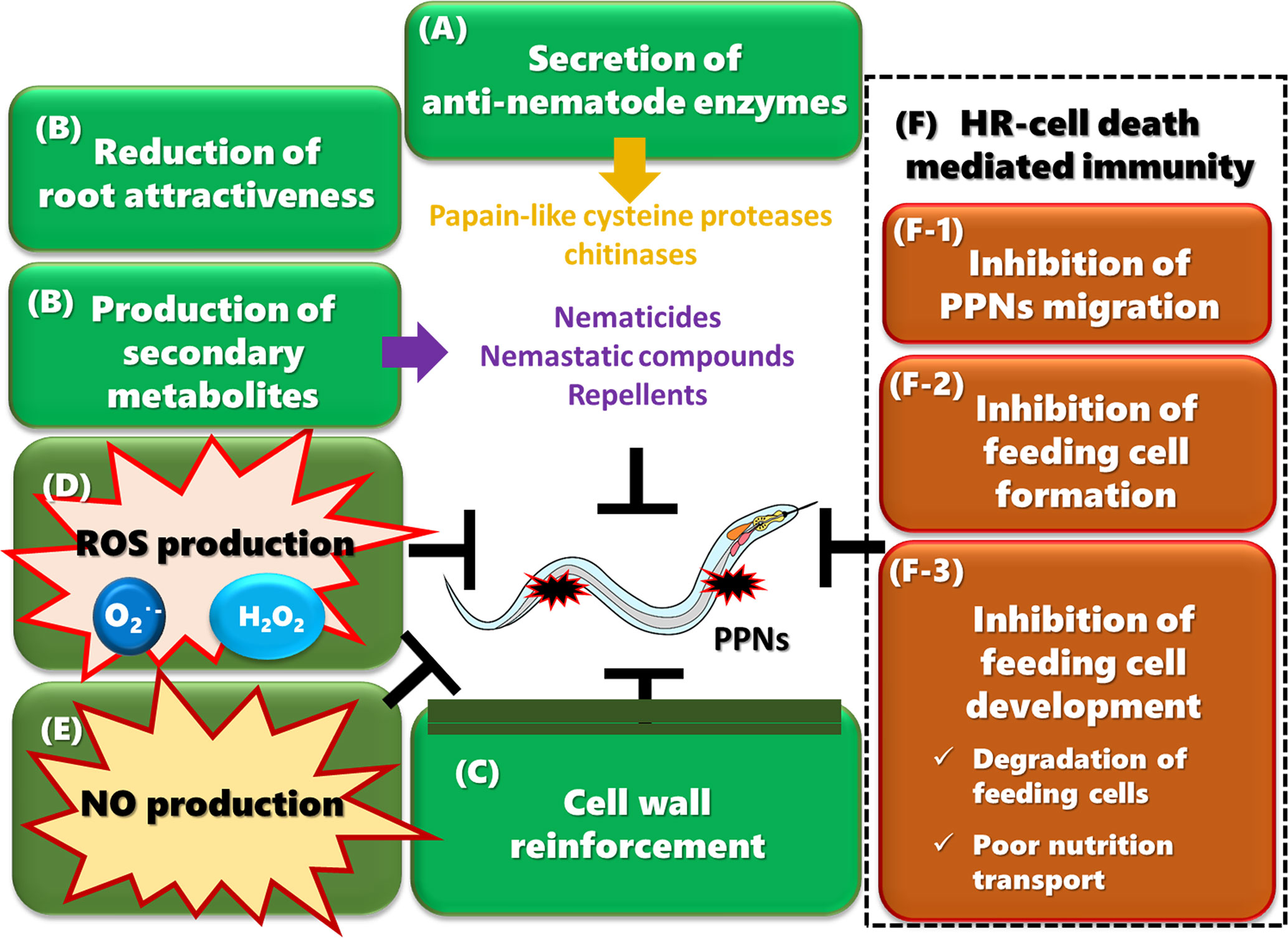
Figure 2 Multiple plant immune responses against PPNs (A) Plants secrete anti-nematode enzymes such as papain-like cysteine proteases (PLCPs) and chitinases into the apoplast to attack PPNs. (B) Resistant plants produce a wide range of secondary metabolites in response to PPN infection. Some metabolites inhibit egg hatching, suppress the motility of migrating PPNs, arrest growth and development, or kill nematodes. Plants may also reduce chemoattraction by secreting less amounts of attractants or more repellents. (C) Plants reinforce their cell walls by accumulating lignin, suberin, and callose, which strengthen the physical barrier to PPNs. (D) PPN infection induces the production of ROS, which may be directly toxic to PPNs. Hydrogen peroxide plays a role in cell wall cross-linking. ROS may also work as a transducing signal to activate immune responses and to control HR-cell death. (E) NO production is induced upon PPN infection and may play a role in JA-mediated defense responses, possibly through the production of protease inhibitor 2. (F) HR-cell death is crucial for limiting PPN movement and completing the life cycle. (F-1) HR-cell death occurs during penetration and migration of PPNs in cortical and epidermal tissues, contributing to inhibition of migration. (F-2) HR-cell death is induced in cells infected by RKNs or CNs, which inhibit the formation of feeding cells. (F-3) HR-cell death is also induced in cells surrounding feeding cells, often resulting in degeneration of feeding cells. Even if some feeding cells survive, the nutrient transport from surrounding tissues to the feeding cells is limited, causing a reduction in the number of eggs, and production of relatively more males. Some resistant plants induce the deterioration of feeding cells without any HR-cell death of surrounding cells.
Chitinases are also potentially important apoplastic enzymes in immunity against PPNs (Figure 2A). Upon fungal infection, plants often secrete chitinases, which degrade chitin in the fungal cell walls (Kumar et al., 2018; Pusztahelyi, 2018). In nematodes, chitin is the main component of the egg shell (Clarke et al., 1967; McClure and Bird, 1976; Perry and Trett, 1986) and makes up part of the pharyngeal lumen walls of Caenorhabditis elegans (Zhang et al., 2005), suggesting that chitinases may have anti-nematodal activity and thus contribute to immunity against PPNs. Consistent with this idea, chitinase activity and transcript levels are upregulated after PPN infection in resistant plants (Qiu et al., 1997; de-Deus Barbosa et al., 2009; Bagnaresi et al., 2013). However, there is currently no genetic evidence connecting plant chitinases to resistance against PPNs.
Production of Anti-Nematode Compounds
Plants produce secondary metabolites in response to PPN invasion (Figure 2B). For instance, chlorogenic acid, a phenolic compound, is produced in various plants including solanaceous plants (Milne et al., 1965; Hung and Rohde, 1973; Pegard et al., 2005), carrots (Knypl et al., 1975), and rice (Plowright et al., 1996), suggesting a common defense response against PPN infection. Although the production of chlorogenic acid is well-correlated with PPN resistance levels, chlorogenic acid itself is only weakly nematicidal for M. incognita (Mahajan et al., 1985; D’Addabbo et al., 2013) with moderate activity against Nacobbus aberrans, a false root-knot nematode (López-Martínez et al., 2011). One possible explanation for this lack of correlation between response and effectiveness is that metabolized products of chlorogenic acid have higher nematicidal activity in the target organism, but those compounds may be unstable or highly toxic in plants. Chlorogenic acid can be hydrolyzed to quinic acid and caffeic acid, with the latter being further oxidized to orthoquinone, which is toxic to PPNs (Mahajan et al., 1985). However, the roles of caffeic acid and orthoquinone in resistance against PPNs need to be further established.
Another phenolic compound, phenylphenalenone anigorufone accumulates at the infection sites of the burrowing nematode Radopholus similis in a resistant banana cultivar (Musa sp.) (Dhakshinamoorthy et al., 2014; Hölscher et al., 2014). Anigorufone has high nematicidal activity because of the formation of large lipid–anigorufone complexes in the bodies of R. similis. Anigorufone is also known as an antifungal phytoalexin, and its synthesis is activated by infection with the pathogenic fungus Fusarium oxysporum (Luis et al., 1995). Interestingly, anigorufone also kills the human protozoan parasite Leishmania through the inhibition of succinate dehydrogenase in the mitochondrial respiratory complex II (Luque-Ortega et al., 2004). However, the toxic mechanism of anigorufone in PPNs and its relationship to the formation of large lipid–anigorufone complexes remains to be determined.
Flavonoids constitute a large class of secondary metabolites in plants. Some flavonoids play important roles in PPN resistance by functioning as nematicides, nemastatic compounds (which do not kill but inhibit their movement), repellents, or inhibitors of egg hatching (Chin et al., 2018). These flavonoids that have anti-nematodal activity mostly belong to the classes of flavonols (e.g., kaempferol, quercetin, myricetin), isoflavonoids, and pterocarpans (e.g., medicarpin, glyceollin). Kaempferol inhibits egg hatching of R. similis (Wuyts et al., 2006b). Kaempferol, quercetin, and myricetin are repellents and nemastatic to M. incognita juveniles (Wuyts et al., 2006b), and medicarpin also inhibits the motility of Pratylenchus penetrans in a concentration-dependent manner (Baldridge et al., 1998). Similarly, patuletin, patulitrin, quercetin, and rutin are nematicidal for infective juveniles of Heterodera zeae, a CN (Faizi et al., 2011). The synthesis of some flavonoids is also induced during infection in resistant plants. For instance, M. incognita–resistant soybean cultivars accumulate glyceollins, a group of soybean-specific prenylated pterocarpan phytoalexins that are expressed upon infection (Kaplan et al., 1980). Interestingly, glyceollin inhibits the motility of M. incognita (Kaplan et al., 1979; Kaplan et al., 1980). Glyceollin accumulation is also higher in CN-resistant soybean cultivars than in susceptible ones. One of the glyceollin isomers, glyceollin I accumulates in tissues adjacent to the head of the CN in resistant soybean roots (Huang and Barker, 1991), suggesting accumulation of glyceollin is spacio-temporally specific to the infection site.
Apart from phenolic compounds, other nematicidal chemicals are produced by several nematode-antagonistic plants, such as marigold and asparagus, which have been used for reducing nematode populations in soil. Marigold roots secrete α-terthienyl (Gommers and Bakker, 1988; Wang et al., 2007; Faizi et al., 2011), an oxidative stress-inducing chemical that effectively penetrates the nematode hypodermis and exerts nematicidal activity (Nivsarkar et al., 2001; Hamaguchi et al., 2019). Similarly, asparagus produces asparagusic acid, which inhibits hatching of two important CNs, Heterodera glycines and G. rostochiensis (Takasugi et al., 1975).
In Brassicaceae family plants, the broad spectrum antimicrobial isothiocyanates and indole glucosinolates are considered as anti-PPN compounds. Isothiocyanates effectively inhibit hatching of CNs and RKNs (Brown et al., 1997; Yu et al., 2005) and also have toxicity to RKNs and the semi-endoparasitic nematode Tylenchulus semipenetrans (Zasada and Ferris, 2003). In Arabidopsis, the synthesis of camalexin, an indole alkaloid glucosinolate-type phytoalexin, is catalyzed by three cytochrome P450-dependent monooxygenases, CYP79B2, CYP79B3 (Hull et al., 2000; Mikkelsen et al., 2000; Bak et al., 2001; Mikkelsen et al., 2004), and PAD3 (phytoalexin-deficient 3, CYP71B15). Double mutants cyp79b2/b3 which do not accumulate indolic glucosinolates are more susceptible to CNs (Shah et al., 2017), while pad3, camalexin-deficient mutants are more susceptible to RKNs than wild type (Teixeira et al., 2016). These results suggest that some indole glucosinolates including camalexin have some inhibitory effects on PPNs, but there have so far been no reports of direct toxicity of indolic glucosinolates on PPNs.
In addition to nematicides and nemastatic compounds, interruption of PPN chemotaxis may also be an effective plant response for inhibiting or limiting PPN infection. Ethylene, which is normally produced after wounding as well as during pathogen invasion, reduces PPN attraction to the root (Booker and DeLong, 2015; Guan et al., 2015; Marhavý et al., 2019). An ethylene-overproducing Arabidopsis mutant is less attractive for PPNs, and attractiveness is greater in plants treated with ethylene-synthesis inhibitors or in ethylene-insensitive mutants (Fudali et al., 2013; Hu et al., 2017). These results suggest that PPN infection induces ethylene production, which possibly prevents secondary PPN invasion by reducing attractiveness. The reduced attractiveness could be due to a reduction in attractant secretion or an increase in repellents. However, the molecular basis of the attractiveness for PPNs is still largely unknown. Several groups have tried to identify RKN attractants from root tips (Čepulytė et al., 2018) and seed-coat mucilage (Tsai et al., 2019). The identification of chemoattractants and chemorepellents may offer some insight into how plants respond to nematodes in the rhizosphere both before and during PPN infection.
Reinforcement of Cell Wall as a Physical Barrier
Since all PPNs must penetrate the cell wall for feeding, reinforcement of cell wall structure has been implicated as an effective defense as a physical barrier (Figure 2C). For instance, PPN infection often induces accumulation of lignin in resistant plants (Balhadère and Evans, 1995a; Balhadère and Evans, 1995b; Andres et al., 2001; Dhakshinamoorthy et al., 2014). Moreover, Arabidopsis mutants with increased levels of syringyl lignin have reduced M. incognita reproduction rates (Wuyts et al., 2006a). These results suggest that lignin accumulation in roots is an effective antagonist to PPN infection.
The effectiveness of lignin accumulation for suppressing nematode infection is also supported by plant immune inducers such as β-aminobutyric acid (BABA), thiamine, and sclareol. BABA, a non-protein amino acid, has broad efficacy against viruses, bacteria, fungi, and oomycetes in various plants (Alexandersson et al., 2016; Cohen et al., 2016). Treatment with BABA inhibits RKN invasion, delays giant cell formation, and retards RKN development. Interestingly, BABA induces lignin accumulation in roots, and callose accumulation in galls (Ji et al., 2015). Thiamine (vitamin B1) treatment also induces lignin accumulation in roots; enhances the expression of phenylalanine ammonia-lyase, a key enzyme of the phenylpropanoid biosynthesis pathway; reduces PPN penetration; and delays PPN development (Huang et al., 2016). An inhibitor of phenylalanine ammonia-lyase suppresses thiamin-mediated immunity, indicating that activation of the phenylpropanoid pathway with subsequent lignin accumulation is important for thiamin-mediated immunity against nematodes. Treatment with sclareol, an antimicrobial compound with activity against some plant-pathogenic bacteria and fungi (Bailey et al., 1975; Kennedy et al., 1992; Seo et al., 2012), also induces lignin accumulation and suppresses RKN penetration (Fujimoto et al., 2015). Importantly, an Arabidopsis mutant of cinnamoyl-coA reductase (ccr2) defective in lignin accumulation cannot induce sclareol-mediated suppression of RKN penetration, suggesting that lignin accumulation is important for the sclareol-mediated immunity.
Similar to lignin accumulation, callose deposition and suberin accumulation may also reinforce cell walls and contribute to immunity against PPNs. The RKN Meloidogyne naasi induces callose deposition at an early infection stage, and suberin accumulation at a later stage in the resistant grass plant Aegilops variabilis (Balhadère and Evans, 1995a; Balhadère and Evans, 1995b). Infection of Arabidopsis by RKN or CN also induces transcriptional activation of suberin biosynthesis genes at the site of infection (Holbein et al., 2019). Overexpression of the transcription factor RAP2.6 in Arabidopsis leads to enhanced callose deposition at syncytia and results in higher resistance to CN (Ali et al., 2013). RAP2.6 is strongly downregulated in syncytia compared to uninfected root; therefore, it is possible that CN suppresses RAP2.6 expression to inhibit callose deposition within syncytia.
Lignin and suberin in suberin lamellae and casparian strips at the endodermis are also important basal physical barriers to RKNs. RKNs are not able to directly cross the endodermis because of the reinforcement of cell walls by suberin lamellae and casparian strips (Wyss et al., 1992; Abad et al., 2009). Indeed, Arabidopsis mutants defective in casparian strips are more susceptible to RKNs (Holbein et al., 2019).
Reactive Oxygen Species (ROS)
The rapid production of ROS, such as superoxide anion and hydrogen peroxide, is a conserved signaling response across kingdoms, and in plants, it is induced at an early stage of PPN infection (Figure 2D). ROS have direct antimicrobial properties but also serve as signaling molecules to activate additional and complementary immune outputs such as strengthening cell walls by cross-linking polymers, amplifying and propagating intra- and intercellular defense signals, and regulating HR-cell death (Torres et al., 2006; Kadota et al., 2015). Resistant tomato plants carrying the Mi-1.2 gene respond to RKN infection with a strong and prolonged induction of ROS. On the other hand, susceptible tomato plants have weak and transient ROS induction in response to nematode infection (Melillo et al., 2006; Melillo et al., 2011; Zhou et al., 2018). Similarly, strong ROS production is induced in Arabidopsis roots during incompatible interactions with the soybean CN H. glycines (Waetzig et al., 1999). Histochemical studies showed that hydrogen peroxide accumulates in the apoplast after infection of the avirulent RKNs or CNs (Waetzig et al., 1999; Melillo et al., 2006).
The plasma membrane-bound NADPH oxidase respiratory burst oxidase homologs (RBOHs) are important for the production of apoplastic ROS (Kadota et al., 2015). In tomato, whitefly-induced 1 (WFI1), an RBOH homolog, is required for Mi-1.2-mediated ROS accumulation during RKN infection. Consistently, HSFA1, a class-A heat-shock factor that regulates Wfi1 transcription by binding to the Wfi1 promoter, is also critical for Mi-1.2-mediated ROS production (Zhou et al., 2018). In Arabidopsis, which has 10 RBOHs, RBOHD is the primary source of ROS production during PTI and NLR-triggered immunity. RBOHF may also work redundantly with RBOHD in some responses, because the rbohD rbohF double mutant has a stronger defense response phenotype against bacterial pathogens (Torres and Dangl, 2005; Torres et al., 2006). Similarly, rbohD rbohF produces more galls after RKN infection than the wild type (Teixeira et al., 2016), indicating a positive role for RBOHD and RBOHF ROS production in immunity against RKNs. Interestingly, fewer CNs develop in rbohD rbohF double mutant, suggesting that CNs require a different level of ROS control by RBOH for successful establishment of infection. Furthermore, rbohD rbohF exhibits larger regions of HR-cell death and less syncytium formation upon CN infection, suggesting that CNs utilize RBOHD- and RBOHF-mediated ROS to suppress HR-cell death in the host (Siddique et al., 2014).
To protect themselves from the toxicity of produced ROS by the host, endoparasitic nematodes may have evolved a number of antioxidant enzymes on their surface and in the hypodermis (Henkle-Dührsen and Kampkötter, 2001). For example, both CNs and RKNs produce peroxiredoxins; some of the most abundant detoxifying antioxidant enzymes, which remove hydrogen peroxides from the apoplast of host plants by thioredoxin (TRX) cysteine thiol-disulfide exchange (Robertson et al., 2000; Henkle-Dührsen and Kampkötter, 2001; Dubreuil et al., 2011). PRX2.1, a clade B peroxiredoxin in M. incognita, is expressed upon infection, and knock-down of the gene reduces resistance against oxidative stress, resulting in fewer galls. This interaction suggests a critical role for PRX2.1 in infection. CNs also secrete GPX-1, a glutathione peroxidase variant, from the hypodermis to scavenge host-derived ROS, thereby protecting external cell membranes from oxidation (Jones et al., 2004). M. incognita glutathione-S-transferases are delivered into the host apoplast to detoxify the products of oxidative stress (Dubreuil et al., 2007). Indeed, freshly hatched infective juveniles of M. incognita are much more resistant to exogenous treatment with hydrogen peroxide than C. elegans (Isermann et al., 2004).
Another PPN strategy for protection against host ROS is to activate the host ROS-scavenging system by the secretion of virulence effectors. For example, CN effector 10A06 interacts with host spermidine synthase 2 and increases spermidine content in infected tissues (Hewezi et al., 2010). Spermidine in higher concentrations functions as a ROS scavenger, and in lower concentrations, it indirectly decreases oxidative stress by activating cellular antioxidant systems (Kasukabe et al., 2004). Indeed, ectopic expression of 10A06 in Arabidopsis increases the expression of several genes encoding antioxidant enzymes. Similarly, MjTTL5, a virulence effector from M. javanica interacts with the Arabidopsis ferredoxin:TRX reductase (FTR) catalytic subunit (FTRc) in plastids (Lin et al., 2016). FTR activates TRXs in chloroplasts or plastids by receiving reducing equivalents from reduced ferredoxin (Balmer et al., 2006; Kirchsteiger et al., 2012). The interaction of MjTTL5 with FTRc drastically increases host ROS-scavenging activity, thus modulating the plant immune reaction. Because peroxiredoxins use TRX to reduce hydrogen peroxide (Broin et al., 2002; Kotze, 2003), it is possible that FTRc works in part with peroxiredoxins by providing reduced TRX to lower ROS production in plants.
Nitric Oxide (NO) and Protease Inhibitor-Based Immunity
NO is an essential signaling molecule that has multiple functions in plants (Delledonne et al., 1998; Torres et al., 2006; Bellin et al., 2013; Mur et al., 2013; Scheler et al., 2013) (Figure 2E). After infection with M. incognita, resistant tomato plants carrying Mi-1.2 produce more NO than susceptible cultivars (Melillo et al., 2011). Application of an exogenous NO donor, sodium nitroprusside (SNP), to susceptible tomato plants significantly enhances immunity against RKNs (Zhou et al., 2015). Treatment with SNP reduces the number of egg masses and restores the growth inhibition associated with PPNs, suggesting that NO plays a positive role in immunity. NO may be involved in the JA-dependent RKN defense pathway, as an NO scavenger partially inhibitis JA-induced RKN defense responses. Moreover, the inhibition of JA biosynthesis by chemical inhibitors significantly increased susceptibility to RKNs, but resistance was effectively restored by exogenous SNP application. Because both JA- and SNP-induced RKN defense responses are compromised by silencing protease inhibitor 2 (PI2), the NO- and JA-pathways likely converge to induce immunity against PPNs (Zhou et al., 2015). However, it remains unclear which proteases PI2 inhibits. Since PPNs use a variety of proteases for their virulence and for their development (Urwin et al., 1997; Neveu et al., 2003), these activities can be inhibited by PI2. Interestingly, heterologous expression of various protease inhibitors, including trypsin inhibitors and cysteine protease inhibitors, confer resistance against PPNs, showing the effectiveness of protease inhibitor-based immunity against PPNs (Hepher and Atkinson, 1992; Urwin et al., 2000; Urwin et al., 2003).
HR-Cell Death-Based Inhibition of Nematode Development
HR-cell death, a type of programmed cell death that is induced after the invasion of avirulent pathogens to prevent the spread of biotrophic pathogens (Huysmans et al., 2017), also plays a crucial role in PPN immunity (Figure 2F). HR-cell death has been observed at three different phases of PPN infection in resistant plants: (1) in the cortex and epidermis during PPN penetration and migration (Hung and Rohde, 1973; Thomason et al., 1976; Finetti Sialer, 1990; Balhadère and Evans, 1995b; Pegard et al., 2005; Proite et al., 2008; Albuquerque et al., 2010; Khallouk et al., 2011; Cabasan et al., 2014; Davies et al., 2015a), (2) in vascular tissues during the initiation of feeding cell formation (Paulson and Webster, 1972; Melillo et al., 2006), and (3) in cells adjacent to developing feeding cells (Kim et al., 1987; Rice et al., 1987; Sobczak et al., 2005; Kim et al., 2010; Kim et al., 2012; Cabasan et al., 2014; Seo et al., 2014; Ye et al., 2017).
During PPN penetration and migration, cell death is also often observed in susceptible plants, but it is less rapid and less frequent than in resistant varieties (Endo and Veech, 1970; Thomason et al., 1976; Sobczak et al., 2005). HR-cell death may inhibit nematode migration, but it is not clear if HR-cell death stops PPN movement directly, or indirectly by releasing nemastatic or nematicidal chemicals or DAMPs to activate other immune responses. HR-cell death is also induced during the initiation of feeding cell development. For instance, Mi-1.2-resistant tomato plants induce HR-cell death during the RKN induction of giant cells, thus inhibiting the development of feeding cells (Paulson and Webster, 1972; Melillo et al., 2006). Another possible function of HR-cell death is to create a physical gap between feeding cells and surrounding cells to block nutrient and water supplies. For example, in resistant tomato lines carrying the Hero gene, potato CN (G. rostochiensis) makes syncytia, but HR-cell death is induced in surrounding cells, which resulted in the separation of the syncytium from stelar conductive tissues (Sobczak et al., 2005). Disconnection of feeding cells from surrounding tissue also occurs in resistant plants after infection with RKNs (Seo et al., 2014; Ye et al., 2017). Disassociation of surrounding tissue leads to poor nutrient supply, thereby inhibiting growth or causing the death of feeding cells, reducing fecundity in females, and increasing male development (Acedo et al., 1984; Rice et al., 1987; Kouassi et al., 2004; Sobczak et al., 2005). Increased male development coincides with a reduced number of females, resulting in the reduction of PPN eggs. In some resistant plants, death of feeding cells is also induced without HR-cell death of surrounding cells. For example, death of syncytia is induced in resistant soybeans (Yan and Baidoo, 2018), and deterioration of giant cells is induced in resistant cowpea carrying Rk gene without typical HR-cell death in surrounding cells (Das et al., 2008). These differences in HR-cell death initiation site may depend on the specific expression pattern of host R genes (Yan and Baidoo, 2018) and PPN effectors.
The importance of HR-cell death is supported by the observation that both RKNs and CNs have effectors that suppress HR-cell death. The M. incognita effector MiISE5, a zinc-finger protein, suppresses HR-cell death induced by the non-host bacterial pathogen, Burkholderia glumae in N. benthamiana, possibly through reprogramming of the host transcriptome (Shi et al., 2018). The RKN effector MeTCTP from Meloidogyne enterolobii also suppresses HR-cell death triggered by the mouse pro-apoptotic protein, Bcl2 associated X protein (Zhuo et al., 2017). CNs also have HR-cell death suppression effectors such as SPRYSEC effectors (Ali et al., 2015b), RHA1B, an E3 ubiquitin ligase (Kud et al., 2019), and GrEXPB2, an expansin-like protein (Ali et al., 2015a). However, these CN effectors do not specifically inhibit HR-cell death but also inhibit other defense responses.
Conclusions and Future Directions
As a result of the identification of several NLR-type and PRR-type receptors involved in immunity against PPNs, we have gradually begun to understand how plants recognize and respond to nematode infection at the molecular level. However, PPN effectors and PAMPs are still largely unknown, and the corresponding receptors remain unidentified. Similarly, various immune responses against nematodes in a wide range of resistant crop and model plants have been recognized (Figure 2 and Supplementary Table 1), but there is still much that is unknown between the phenomena of PPN recognition and the triggering of specific immune responses (Figure 3). Thus, significant challenges for future research in the field of plant and nematode interactions would be to identify immune receptor-ligands pairs (PAMPs, DAMPs, and effectors), to clarify the molecular bases of signaling pathways leading to individual immune responses, to understand the interactions of these components and signaling pathways in PPN immunity, and to identify the molecular components that define host specificity. Loss of significant agricultural productivity in a burgeoning global population goes beyond monetary losses. The absence of truly effective strategies for controlling nematode populations and infection has serious and worsening consequences for sustainable agriculture. Understanding the molecular mechanisms of PPN recognition and immune signaling networks will provide a knowledge base for much-needed PPN disease control strategies in the future.
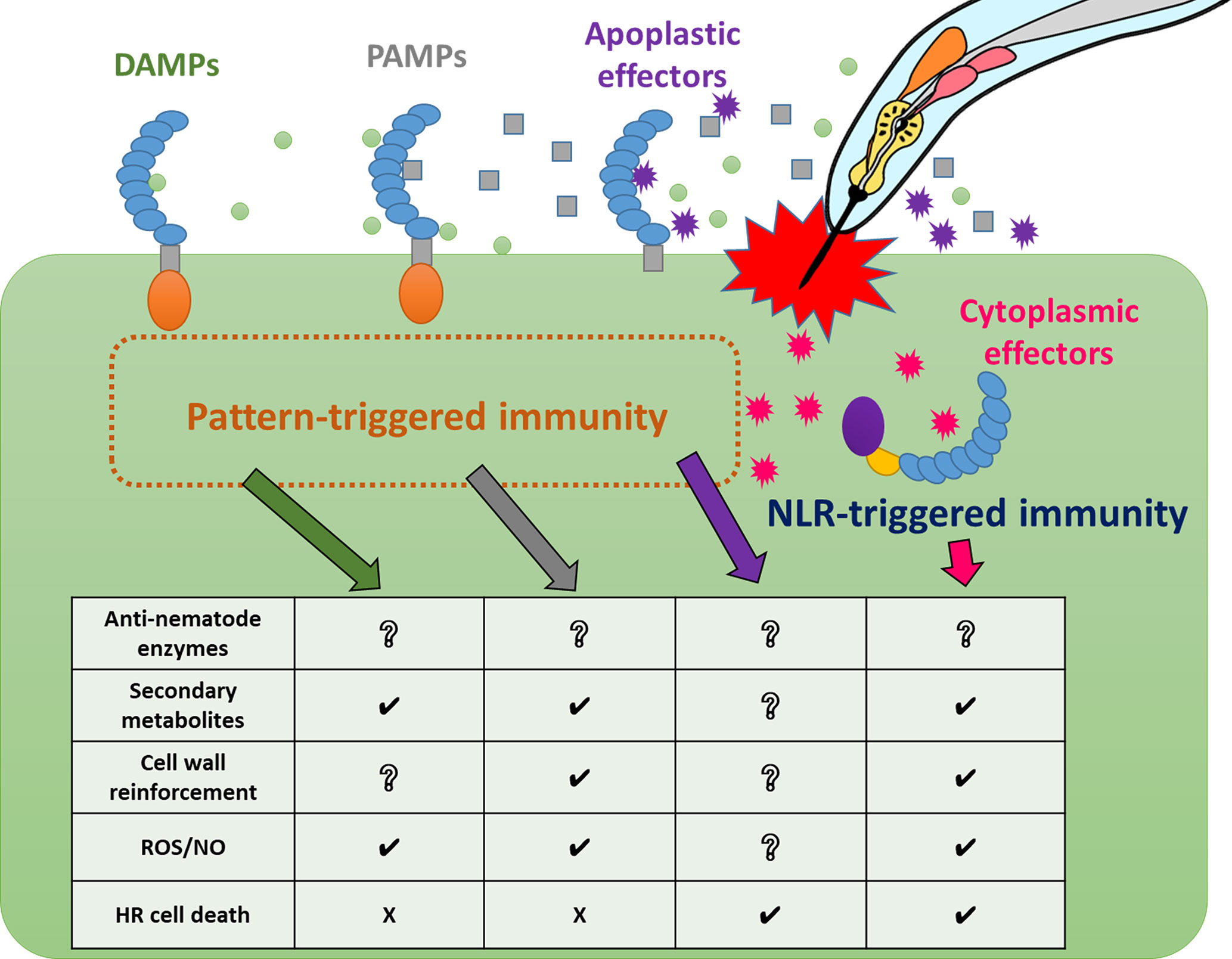
Figure 3 Relationships between nematode recognition and immune responses Plants activate pattern-triggered immunity and NLR-triggered immunity against PPN infection using different immune receptors. These receptors trigger a variety of defense responses. Some immune responses, such as ROS/NO production, are induced in common by some immune receptors with different kinetics, while other responses, such as cell death, are induced by specific immune receptors.
Author Contributions
All authors listed have made a substantial, direct and intellectual contribution to the work, and approved it for publication.
Funding
This work was supported by Grant-in-Aid for JSPS Fellows Grant number JP19J00655 (to KSa), and MEXT/JSPS KAKENHI Grant Number, JP16H06186, JP16KT0037 and JP19H02962 (to YK), JP15H05959 and JP17H06172 (to KSh).
Conflict of Interest
The authors declare that the research was conducted in the absence of any commercial or financial relationships that could be construed as a potential conflict of interest.
Abbreviations
BABA, β-aminobutyric acid; CC, coiled-coil; CN, Cyst nematode; FTR, ferredoxin:thioredoxin reductase; HR, hypersensitive response; JA, jasmonic acid; LRR, leucine-rich repeat; NLR, nucleotide-binding domain leucine-rich repeat; NO, nitric oxide; OG, oligogalacturonides; PAMP, pathogen-associated molecular pattern; PG, polygalacturonase; PGIP, polygalacturonase inhibitor proteins; PLCP, papain-like cysteine protease; PPN, plant parasitic nematode; PRR, pattern recognition receptor; PTI, pattern-triggered immunity; RBOH, respiratory burst oxidase homolog; RKN, root-knot nematode; RLK, receptor-like kinase; ROS, reactive oxygen species; SNP, sodium nitroprusside; SPRYSEC, secreted SP1a and ryanodine receptor (SPRY) domain; TIR, toll-interleukin 1 receptor; TRX, thioredoxin.
Acknowledgments
We thank all the members of the Shirasu laboratory in RIKEN and Dr. Taketo Uehara in Central Region Agricultural Research Center, NARO for fruitful discussions about this review. Due to space constraints, a limited number of studies have been cited. We recognize the important contributions of many others to the understanding of plant responses to nematode infection, and therefore apologize to all our colleagues whose works are not referenced here.
Supplementary Material
The Supplementary Material for this article can be found online at: https://www.frontiersin.org/articles/10.3389/fpls.2019.01165/full#supplementary-material
References
Abad, P., Castagnone-Sereno, P., Rosso, M. N., Engler, J.d.A., Favery, B. (2009). “Invasion, feeding and development,” in Root-knot nematodes. Eds. Perry, R. N., Moens, M., Starr, J. L. (Cambridge: CABI International Press), 163–181. doi: 10.1079/9781845934927.0163
Acedo, J. R., Dropkin, V. H., Luedders, V. D. (1984). Nematode population attrition and histopathology of Heterodera glycines-soybean associations. J. Nematol. 16 (1), 48–56.
Albuquerque, E., Carneiro, R., Costa, P., Gomes, A., Santos, M., Pereira, A., et al. (2010). Resistance to Meloidogyne incognita expresses a hypersensitive-like response in Coffea arabica. Eur. J. Plant Pathol. 127 (3), 365–373. doi: 10.1007/s10658-010-9603-3
Alexandersson, E., Mulugeta, T., Lankinen, Å., Liljeroth, E., Andreasson, E. (2016). Plant resistance inducers against pathogens in Solanaceae species-from molecular mechanisms to field application. Int. J. Mol. Sci. 17 (10), 1673. doi: 10.3390/ijms17101673
Ali, M. A., Abbas, A., Kreil, D. P., Bohlmann, H. (2013). Overexpression of the transcription factor RAP2.6 leads to enhanced callose deposition in syncytia and enhanced resistance against the beet cyst nematode Heterodera schachtii in Arabidopsis roots. BMC Plant Biol. 13, 47. doi: 10.1186/1471-2229-13-47
Ali, S., Magne, M., Chen, S., Cote, O., Stare, B. G., Obradovic, N., et al. (2015a). Analysis of putative apoplastic effectors from the nematode, Globodera rostochiensis, and identification of an expansin-like protein that can induce and suppress host defenses. PLoS One 10 (1), e0115042. doi: 10.1371/journal.pone.0115042
Ali, S., Magne, M., Chen, S., Obradovic, N., Jamshaid, L., Wang, X., et al. (2015b). Analysis of Globodera rostochiensis effectors reveals conserved functions of SPRYSEC proteins in suppressing and eliciting plant immune responses. Front. Plant Sci. 6, 623. doi: 10.3389/fpls.2015.00623
Andres, M. F., Melillo, M. T., Delibes, A., Romero, M. D., Bleve-Zacheo, T. (2001). Changes in wheat root enzymes correlated with resistance to cereal cyst nematodes. New Phytol. 152 (2), 343–354. doi: 10.1111/j.0028-646X.2001.00258.x
Bagnaresi, P., Sala, T., Irdani, T., Scotto, C., Lamontanara, A., Beretta, M., et al. (2013). Solanum torvum responses to the root-knot nematode Meloidogyne incognita. BMC Genomics 14, 540. doi: 10.1186/1471-2164-14-540
Bailey, J., Carter, G., Burden, R., Wain, R. (1975). Control of rust diseases by diterpenes from Nicotiana glutinosa. Nature 255 (5506), 328–329. doi: 10.1038/255328a0
Bak, S., Tax, F. E., Feldmann, K. A., Galbraith, D. W., Feyereisen, R. (2001). CYP83B1, a cytochrome P450 at the metabolic branch point in auxin and indole glucosinolate biosynthesis in Arabidopsis. Plant Cell 13 (1), 101–111. doi: 10.1105/tpc.13.1.101
Baldridge, G. D., O’Neill, N. R., Samac, D. A. (1998). Alfalfa (Medicago sativa L.) resistance to the root-lesion nematode, Pratylenchus penetrans: defense-response gene mRNA and isoflavonoid phytoalexin levels in roots. Plant Mol. Biol. 38 (6), 999–1010. doi: 10.1023/A:1006182908528
Balhadère, P., Evans, A. (1995a). Cytochemical investigation of resistance to root-knot nematode Meloidogyne naasi in cereals and grasses using cryosections of roots. Fund. Appl. Nematol. 18 (6), 539–547.
Balhadère, P., Evans, A. (1995b). Histopathogenesis of susceptible and resistant responses of wheat, barley and wild grasses to Meloidogyne naasi.Fund. Appl. Nematol. 18 (6), 531–538.
Balmer, Y., Vensel, W. H., Cai, N., Manieri, W., Schürmann, P., Hurkman, W. J., et al. (2006). A complete ferredoxin/thioredoxin system regulates fundamental processes in amyloplasts. Proc. Natl. Acad. Sci. U S A 103 (8), 2988–2993. doi: 10.1073/pnas.0511040103
Bellin, D., Asai, S., Delledonne, M., Yoshioka, H. (2013). Nitric oxide as a mediator for defense responses. Mol. Plant Microbe Interact. 26 (3), 271–277. doi: 10.1094/MPMI-09-12-0214-CR
Benedetti, M., Pontiggia, D., Raggi, S., Cheng, Z., Scaloni, F., Ferrari, S., et al. (2015). Plant immunity triggered by engineered in vivo release of oligogalacturonides, damage-associated molecular patterns. Proc. Natl. Acad. Sci. U S A 112 (17), 5533–5538. doi: 10.1073/pnas.1504154112
Bishop, P. D., Makus, D. J., Pearce, G., Ryan, C. A. (1981). Proteinase inhibitor-inducing factor activity in tomato leaves resides in oligosaccharides enzymically released from cell walls. Proc. Natl. Acad. Sci. U S A 78 (6), 3536–3540. doi: 10.1073/pnas.78.6.3536
Blanchard, A., Esquibet, M., Fouville, D., Grenier, E. (2005). Ranbpm homologue genes characterised in the cyst nematodes Globodera pallida and Globodera ‘mexicana’. Physiol. Mol. Plant Pathol. 67 (1), 15–22. doi: 10.1016/j.pmpp.2005.09.001
Bohlmann, H. (2015). “Introductory chapter on the basic biology of cyst nematodes,” in Plant nematode interactions: a view on compatible interrelationships, vol 73, Advances in Botanical Research. Eds. Escobar, C., Fenoll, C. (Oxford, UK: Elsevier), 33–60. doi: 10.1016/bs.abr.2014.12.001
Bohlmann, H., Sobczak, M. (2014). The plant cell wall in the feeding sites of cyst nematodes. Front. Plant Sci. 5, 89. doi: 10.3389/fpls.2014.00089
Booker, M. A., DeLong, A. (2015). Producing the ethylene signal: regulation and diversification of ethylene biosynthetic enzymes. Plant Physiol. 169 (1), 42–50. doi: 10.1104/pp.15.00672
Boutrot, F., Zipfel, C. (2017). Function, discovery, and exploitation of plant pattern recognition receptors for broad-spectrum disease resistance. Annu. Rev. Phytopathol. 55, 257–286. doi: 10.1146/annurev-phyto-080614-120106
Broin, M., Cuiné, S., Eymery, F., Rey, P. (2002). The plastidic 2-cysteine peroxiredoxin is a target for a thioredoxin involved in the protection of the photosynthetic apparatus against oxidative damage. Plant Cell 14 (6), 1417–1432. doi: 10.1105/tpc.001644
Brown, P. D., Morra, M. J., Sparks, D. L. (1997). Control of soil-borne plant pests using glucosinolate-containing plants. Adv. Agron. 61, 167–231. doi: 10.1016/S0065-2113(08)60664-1
Cabasan, M., Kumar, A., Bellafiore, S., De Waele, D. (2014). Histopathology of the rice root-knot nematode, Meloidogyne graminicola, on Oryza sativa and O. glaberrima. Nematology 16, 73–81. doi: 10.1163/15685411-00002746
Castagnone-Sereno, P. (2006). Genetic variability and adaptive evolution in parthenogenetic root-knot nematodes. Heredity 96 (4), 282–289. doi: 10.1038/sj.hdy.6800794
Čepulytė, R., Danquah, W. B., Bruening, G., Williamson, V. M. (2018). Potent attractant for root-knot nematodes in exudates from seedling root tips of two host species. Sci. Rep. 8 (1), 10847. doi: 10.1038/s41598-018-29165-4
Chen, J., Hu, L., Sun, L., Lin, B., Huang, K., Zhuo, K., et al. (2018). A novel Meloidogyne graminicola effector, MgMO237, interacts with multiple host defence-related proteins to manipulate plant basal immunity and promote parasitism. Mol. Plant Pathol. 19 (8), 1942–1955. doi: 10.1111/mpp.12671
Chen, R., Li, H., Zhang, L., Zhang, J., Xiao, J., Ye, Z. (2007). CaMi, a root-knot nematode resistance gene from hot pepper (Capsium annuum L.) confers nematode resistance in tomato. Plant Cell Rep. 26 (7), 895–905. doi: 10.1007/s00299-007-0304-0
Chen, S., Chronis, D., Wang, X. (2013). The novel GrCEP12 peptide from the plant-parasitic nematode Globodera rostochiensis suppresses flg22-mediated PTI. Plant Signal Behav. 8 (9), e25359. doi: 10.4161/psb.25359
Chin, S., Behm, C. A., Mathesius, U. (2018). Functions of flavonoids in plant–nematode interactions. Plants (Basel) 7 (4), 85. doi: 10.3390/plants7040085
Clarke, A. J., Cox, P. M., Shepherd, A. M. (1967). The chemical composition of the egg shells of the potato cyst-nematode, Heterodera rostochiensis Woll. Biochem. J. 104 (3), 1056–1060. doi: 10.1042/bj1041056
Claverie, M., Dirlewanger, E., Bosselut, N., Van Ghelder, C., Voisin, R., Kleinhentz, M., et al. (2011). The Ma gene for complete-spectrum resistance to Meloidogyne species in Prunus is a TNL with a huge repeated C-terminal post-LRR region. Plant Physiol. 156 (2), 779–792. doi: 10.1104/pp.111.176230
Cohen, Y., Vaknin, M., Mauch-Mani, B. (2016). BABA-induced resistance: milestones along a 55-year journey. Phytoparasitica 44 (4), 513–538. doi: 10.1007/s12600-016-0546-x
Cui, H., Tsuda, K., Parker, J. E. (2015). Effector-triggered immunity: from pathogen perception to robust defense. Annu. Rev. Plant Biol. 66, 487–511. doi: 10.1146/annurev-arplant-050213-040012
D’Addabbo, T., Carbonara, T., Argentieri, M., Radicci, V., Leonetti, P., Villanova, L., et al. (2013). Nematicidal potential of Artemisia annua and its main metabolites. Eur. J. Plant Pathol. 137 (2), 295–304. doi: 10.1007/s10658-013-0240-5
D’Ovidio, R., Mattei, B., Roberti, S., Bellincampi, D. (2004). Polygalacturonases, polygalacturonase-inhibiting proteins and pectic oligomers in plant-pathogen interactions. Biochim. Biophys. Acta 1696 (2), 237–244. doi: 10.1016/j.bbapap.2003.08.012
Das, S., DeMason, D., Ehlers, J., Close, T., Roberts, P. (2008). Histological characterization of root-knot nematode resistance in cowpea and its relation to reactive oxygen species modulation. J. Exp. Bot. 59 (6), 1305–1313. doi: 10.1093/jxb/ern036
Davies, L. J., Brown, C. R., Elling, A. A. (2015a). Calcium is involved in the RMc1(blb)-mediated hypersensitive response against Meloidogyne chitwoodi in potato. Plant Cell Rep. 34 (1), 167–177. doi: 10.1007/s00299-014-1697-1
Davies, L. J., Zhang, L., Elling, A. A. (2015b). The Arabidopsis thaliana papain-like cysteine protease RD21 interacts with a root-knot nematode effector protein. Nematology 17 (6), 655–666. doi: 10.1163/15685411-00002897
Davis, E. L., Hussey, R. S., Mitchum, M. G., Baum, T. J. (2008). Parasitism proteins in nematode-plant interactions. Curr. Opin. Plant Biol. 11 (4), 360–366. doi: 10.1016/j.pbi.2008.04.003
Decraemer, W., Hunt, D. J. (2013). “Structure and classification,” in Plant Nematology, 2 ed. Eds. Perry, R. N., Moens, M. (Wallingford, UK: CABI Publishing), 3–39. doi: 10.1079/9781780641515.0003
de-Deus Barbosa, A. E. A., Rocha, F. R., de Lima e Souza, D. D. S., Freire, É., de Oliveira Neto, O. B., Viana, A. A. B., et al. (2009). Differentially expressed genes in cotton plant genotypes infected with Meloidogyne incognita. Plant Sci. 177 (5), 492–497. doi: 10.1016/j.plantsci.2009.07.013
Delledonne, M., Xia, Y., Dixon, R. A., Lamb, C. (1998). Nitric oxide functions as a signal in plant disease resistance. Nature 394 (6693), 585–588. doi: 10.1038/29087
Dhakshinamoorthy, S., Mariama, K., Elsen, A., De Waele, D. (2014). Phenols and lignin are involved in the defence response of banana (Musa) plants to Radopholus similis infection. Nematology 16, 565–576. doi: 10.1163/15685411-00002788
Dodds, P. N., Rathjen, J. P. (2010). Plant immunity: towards an integrated view of plant-pathogen interactions. Nat. Rev. Genet. 11 (8), 539–548. doi: 10.1038/nrg2812
Dubreuil, G., Deleury, E., Magliano, M., Jaouannet, M., Abad, P., Rosso, M. N. (2011). Peroxiredoxins from the plant parasitic root-knot nematode, Meloidogyne incognita, are required for successful development within the host. Int. J. Parasitol. 41 (3-4), 385–396. doi: 10.1016/j.ijpara.2010.10.008
Dubreuil, G., Magliano, M., Deleury, E., Abad, P., Rosso, M. N. (2007). Transcriptome analysis of root-knot nematode functions induced in the early stages of parasitism. New Phytol. 176 (2), 426–436. doi: 10.1111/j.1469-8137.2007.02181.x
Endo, B., Veech, J. (1970). Morphology and histochemistry of soybean roots infected with Heterodera glycines. Phytopathology 60 (10), 1493–1498. doi: 10.1094/Phyto-60-1493
Ernst, K., Kumar, A., Kriseleit, D., Kloos, D. U., Phillips, M. S., Ganal, M. W. (2002). The broad-spectrum potato cyst nematode resistance gene (Hero) from tomato is the only member of a large gene family of NBS-LRR genes with an unusual amino acid repeat in the LRR region. Plant J. 31 (2), 127–136. doi: 10.1046/j.1365-313X.2002.01341.x
Escobar, C., Barcala, M., Cabrera, J., Fenoll, C. (2015). “Overview of root-knot nematodes and giant cells,” in Plant nematode interactions: a view on compatible interrelationships, vol 73, Advances in Botanical Research. Eds. Escobar, C., Fenoll, C. (Oxford, UK: Elsevier), 1–32. doi: 10.1016/bs.abr.2015.01.001
Faizi, S., Fayyaz, S., Bano, S., Iqbal, E. Y., Lubna, Siddiqi, H., et al. (2011). Isolation of nematicidal compounds from Tagetes patula L. yellow flowers: structure–activity relationship studies against cyst nematode Heterodera zeae infective stage larvae. J. Agric. Food Chem. 59 (17), 9080–9093. doi: 10.1021/jf201611b
Finetti Sialer, M. (1990). Histopathological changes induced by Nacobbus aberrans resistant and susceptible potato roots. Rev. Nématol. 13 (2), 155–160.
Fudali, S. L., Wang, C., Williamson, V. M. (2013). Ethylene signaling pathway modulates attractiveness of host roots to the root-knot nematode Meloidogyne hapla. Mol. Plant Microbe Interact. 26 (1), 75–86. doi: 10.1094/MPMI-05-12-0107-R
Fujimoto, T., Mizukubo, T., Abe, H., Seo, S. (2015). Sclareol induces plant resistance to root-knot nematode partially through ethylene-dependent enhancement of lignin accumulation. Mol. Plant Microbe Interact. 28 (4), 398–407. doi: 10.1094/MPMI-10-14-0320-R
Gheysen, G., Mitchum, M. G. (2011). How nematodes manipulate plant development pathways for infection. Curr. Opin. Plant Biol. 14 (4), 415–421. doi: 10.1016/j.pbi.2011.03.012
Gleason, C. A., Liu, Q. L., Williamson, V. M. (2008). Silencing a candidate nematode effector gene corresponding to the tomato resistance gene Mi-1 leads to acquisition of virulence. Mol. Plant Microbe Interact. 21 (5), 576–585. doi: 10.1094/MPMI-21-5-0576
Gommers, F. J., Bakker, J. (1988). Mode of action of alpha-terthienyl and related compounds may explain the suppressant effects of Tagetes species on populations of free living endoparasitic plant nematodes. Bioact. Mol. 7, 61–69.
Goverse, A., Smant, G. (2014). The activation and suppression of plant innate immunity by parasitic nematodes. Annu. Rev. Phytopathol. 52, 243–265. doi: 10.1146/annurev-phyto-102313-050118
Gross, S. M., Williamson, V. M. (2011). Tm1: a mutator/foldback transposable element family in root-knot nematodes. PLoS One 6 (9), e24534. doi: 10.1371/journal.pone.0024534
Guan, R., Su, J., Meng, X., Li, S., Liu, Y., Xu, J., et al. (2015). Multilayered regulation of ethylene induction plays a positive role in Arabidopsis resistance against Pseudomonas syringae. Plant Physiol. 169 (1), 299–312. doi: 10.1104/pp.15.00659
Hölscher, D., Dhakshinamoorthy, S., Alexandrov, T., Becker, M., Bretschneider, T., Buerkert, A., et al. (2014). Phenalenone-type phytoalexins mediate resistance of banana plants (Musa spp.) to the burrowing nematode Radopholus similis. Proc. Natl. Acad. Sci. U S A 111 (1), 105–110. doi: 10.1073/pnas.1314168110
Hahn, M. G., Darvill, A. G., Albersheim, P. (1981). Host-pathogen interactions: XIX. The endogenous elicitor, a fragment of a plant cell wall polysaccharide that elicits phytoalexin accumulation in soybeans. Plant Physiol. 68 (5), 1161–1169. doi: 10.1104/pp.68.5.1161
Hamaguchi, T., Sato, K., Vicente, C. S. L., Hasegawa, K. (2019). Nematicidal actions of the marigold exudate α-terthienyl: oxidative stress-inducing compound penetrates nematode hypodermis. Biol. Open 8 (4) (bio038646). doi: 10.1242/bio.038646
Henkle-Dührsen, K., Kampkötter, A. (2001). Antioxidant enzyme families in parasitic nematodes. Mol. Biochem. Parasitol. 114 (2), 129–142. doi: 10.1016/S0166-6851(01)00252-3
Hewezi, T., Baum, T. J. (2013). Manipulation of plant cells by cyst and root-knot nematode effectors. Mol. Plant Microbe Interact. 26 (1), 9–16. doi: 10.1094/MPMI-05-12-0106-FI
Hewezi, T., Howe, P. J., Maier, T. R., Hussey, R. S., Mitchum, M. G., Davis, E. L., et al. (2010). Arabidopsis spermidine synthase is targeted by an effector protein of the cyst nematode Heterodera schachtii. Plant Physiol. 152 (2), 968–984. doi: 10.1104/pp.109.150557
Holbein, J., Franke, R. B., Marhavý, P., Fujita, S., Górecka, M., Sobczak, M., et al. (2019). Root endodermal barrier system contributes to defence against plant-parasitic cyst and root-knot nematodes. Plant J. doi: 10.1111/tpj.14459
Hou, S., Liu, Z., Shen, H., Wu, D. (2019). Damage-associated molecular pattern-triggered immunity in plants. Front. Plant Sci. 10, 646. doi: 10.3389/fpls.2019.00646
Hu, Y., You, J., Li, C., Williamson, V. M., Wang, C. (2017). Ethylene response pathway modulates attractiveness of plant roots to soybean cyst nematode Heterodera glycines. Sci. Rep. 7, 41282. doi: 10.1038/srep41282
Huang, J. S., Barker, K. R. (1991). Glyceollin I in soybean-cyst nematode interactions: spatial and temporal distribution in roots of resistant and susceptible soybeans. Plant Physiol. 96 (4), 1302–1307. doi: 10.1104/pp.96.4.1302
Huang, W. K., Ji, H. L., Gheysen, G., Kyndt, T. (2016). Thiamine-induced priming against root-knot nematode infection in rice involves lignification and hydrogen peroxide generation. Mol. Plant Pathol. 17 (4), 614–624. doi: 10.1111/mpp.12316
Hull, A. K., Vij, R., Celenza, J. L. (2000). Arabidopsis cytochrome P450s that catalyze the first step of tryptophan-dependent indole-3-acetic acid biosynthesis. Proc. Natl. Acad. Sci. U S A 97 (5), 2379–2384. doi: 10.1073/pnas.040569997
Hung, C., Rohde, R. A. (1973). Phenol accumulation related to resistance in tomato to infection by root-knot and lesion nematodes. J. Nematol. 5 (4), 253–258.
Huysmans, M., Lema, A, S., Coll, N. S., Nowack, M. K. (2017). Dying two deaths—programmed cell death regulation in development and disease. Curr. Opin. Plant Biol. 35, 37–44. doi: 10.1016/j.pbi.2016.11.005
Isermann, K., Liebau, E., Roeder, T., Bruchhaus, I. (2004). A peroxiredoxin specifically expressed in two types of pharyngeal neurons is required for normal growth and egg production in Caenorhabditis elegans. J. Mol. Biol. 338 (4), 745–755. doi: 10.1016/j.jmb.2004.03.021
Jablonska, B., Ammiraju, J. S., Bhattarai, K. K., Mantelin, S., Martinez de Ilarduya, O., Roberts, P. A., et al. (2007). The Mi-9 gene from Solanum arcanum conferring heat-stable resistance to root-knot nematodes is a homolog of Mi-1. Plant Physiol. 143 (2), 1044–1054. doi: 10.1104/pp.106.089615
Jaouannet, M., Magliano, M., Arguel, M. J., Gourgues, M., Evangelisti, E., Abad, P., et al. (2013). The root-knot nematode calreticulin Mi-CRT is a key effector in plant defense suppression. Mol. Plant Microbe Interact. 26 (1), 97–105. doi: 10.1094/MPMI-05-12-0130-R
Ji, H., Kyndt, T., He, W., Vanholme, B., Gheysen, G. (2015). β-aminobutyric acid-induced resistance against root-knot nematodes in rice is based on increased basal defense. Mol. Plant Microbe Interact. 28 (5), 519–533. doi: 10.1094/MPMI-09-14-0260-R
Jones, J. D., Dangl, J. L. (2006). The plant immune system. Nature 444 (7117), 323–329. doi: 10.1038/nature05286
Jones, J. T., Haegeman, A., Danchin, E. G., Gaur, H. S., Helder, J., Jones, M. G., et al. (2013). Top 10 plant-parasitic nematodes in molecular plant pathology. Mol. Plant Pathol. 14 (9), 946–961. doi: 10.1111/mpp.12057
Jones, J. T., Reavy, B., Smant, G., Prior, A. E. (2004). Glutathione peroxidases of the potato cyst nematode Globodera rostochiensis. Gene 324, 47–54. doi: 10.1016/j.gene.2003.09.051
Kadota, Y., Shirasu, K., Zipfel, C. (2015). Regulation of the NADPH oxidase RBOHD during plant immunity. Plant Cell Physiol. 56 (8), 1472–1480. doi: 10.1093/pcp/pcv063
Kaloshian, I., Desmond, O. J., Atamian, H. S. (2011). “Disease resistance-genes and defense responses during incompatible interactions,” in Genomics and molecular genetics of plant-nematode interactions. Eds. Jones, J., Gheysen, G., Fenoll, C. (Dordrecht, Netherlands: Springer), 309–324. doi: 10.1007/978-94-007-0434-3_15
Kaplan, D. T., Keen, N. T., Thomason, I. J. (1980). Association of glyceollin with the incompatible response of soybean roots to Meloidogyne incognita. Physiol. Plant Pathol. 16 (3), 309–318. doi: 10.1016/S0048-4059(80)80002-6
Kaplan, D. T., Thomason, I. J., Van Gundy, S. D. (1979). Histological study of the compatible and incompatible interaction of soybeans and Meloidogyne incognita. J. Nematol. 11 (4), 338–343.
Kasukabe, Y., He, L., Nada, K., Misawa, S., Ihara, I., Tachibana, S. (2004). Overexpression of spermidine synthase enhances tolerance to multiple environmental stresses and up-regulates the expression of various stress-regulated genes in transgenic Arabidopsis thaliana. Plant Cell Physiol. 45 (6), 712–722. doi: 10.1093/pcp/pch083
Kennedy, B. S., Nielsen, M. T., Severson, R. F., Sisson, V. A., Stephenson, M. K., Jackson, D. M. (1992). Leaf surface chemicals from Nicotiana affecting germination of Peronospora tabacina (adam) sporangia. J. Chem. Ecol. 18 (9), 1467–1479. doi: 10.1007/BF00993221
Khallouk, S., Voisin, R., Van Ghelder, C., Engler, G., Amiri, S., Esmenjaud, D. (2011). Histological mechanisms of the resistance conferred by the Ma gene against Meloidogyne incognita in Prunus spp. Phytopathology 101 (8), 945–951. doi: 10.1094/PHYTO-01-11-0004
Kim, Y., Kim, K., Riggs, R. (2010). Differential subcellular responses in resistance soybeans infected with soybean cyst nematode races. Plant Pathol. J. 26 (2), 154–158. doi: 10.5423/PPJ.2010.26.2.154
Kim, Y., Kim, K., Riggs, R. (2012). Initial subcellular responses of susceptible and resistant soybeans infected with the soybean cyst nematode. Plant Pathol. J. 28 (4), 401–408. doi: 10.5423/PPJ.OA.04.2012.0054
Kim, Y. H., Riggs, R. D., Kim, K. S. (1987). Structural changes associated with resistance of soybean to Heterodera glycines. J. Nematol. 19 (2), 177–187.
Kirchsteiger, K., Ferrández, J., Pascual, M. B., González, M., Cejudo, F. J. (2012). NADPH thioredoxin reductase C is localized in plastids of photosynthetic and nonphotosynthetic tissues and is involved in lateral root formation in Arabidopsis. Plant Cell 24 (4), 1534–1548. doi: 10.1105/tpc.111.092304
Knypl, J. S., Chylinska, K. M., Brzeski, M. W. (1975). Increased level of chlorogenic acid and inhibitors of indoyl-3-acetic acid oxidase in roots of carrot infested with the northern root-knot nematode. Physiol. Plant Pathol. 6 (1), 51–64. doi: 10.1016/0048-4059(75)90104-6
Kotze, A. C. (2003). Catalase induction protects Haemonchus contortus against hydrogen peroxide in vitro. Int. J. Parasitol. 33 (4), 393–400. doi: 10.1016/S0020-7519(03)00012-2
Kouassi, A., Kerlan, M., Sobczak, M., Dantec, J., Rouaux, C., Ellisseche, D., et al. (2004). Resistance to the root-knot nematode Meloidogyne fallax in Solanum sparsipilum: analysis of the mechanisms. Nematology 6, 389–400. doi: 10.1163/1568541042360519
Kud, J., Wang, W., Gross, R., Fan, Y., Huang, L., Yuan, Y., et al. (2019). The potato cyst nematode effector RHA1B is a ubiquitin ligase and uses two distinct mechanisms to suppress plant immune signaling. PLoS Pathog. 15 (4), e1007720. doi: 10.1371/journal.ppat.1007720
Kumar, M., Brar, A., Yadav, M., Chawade, A., Vivekanand, V., Pareek, N. (2018). Chitinases—potential candidates for enhanced plant resistance towards fungal pathogens. Agriculture 8 (7), 88. doi: 10.3390/agriculture8070088
Lee, M. W., Huffaker, A., Crippen, D., Robbins, R. T., Goggin, F. L. (2018). Plant elicitor peptides promote plant defences against nematodes in soybean. Mol. Plant Pathol. 19 (4), 858–869. doi: 10.1111/mpp.12570
López-Martínez, N., Colinas-León, M., Peña-Valdivia, C., Salinas-Moreno, Y., Fuentes-Montiel, P., Biesaga, M., et al. (2011). Alterations in peroxidase activity and phenylpropanoid metabolism induced by Nacobbus aberrans Thorne and Allen, 1944 in chilli (Capsicum annuum L.) CM334 resistant to Phytophthora capsici Leo. Plant Soil 338 (1-2), 399–409. doi: 10.1007/s11104-010-0553-5
Lin, B., Zhuo, K., Chen, S., Hu, L., Sun, L., Wang, X., et al. (2016). A novel nematode effector suppresses plant immunity by activating host reactive oxygen species-scavenging system. New Phytol. 209 (3), 1159–1173. doi: 10.1111/nph.13701
Lozano-Torres, J. L., Wilbers, R. H., Gawronski, P., Boshoven, J. C., Finkers-Tomczak, A., Cordewener, J. H., et al. (2012). Dual disease resistance mediated by the immune receptor Cf-2 in tomato requires a common virulence target of a fungus and a nematode. Proc. Natl. Acad. Sci. U S A 109 (25), 10119–10124. doi: 10.1073/pnas.1202867109
Lozano-Torres, J. L., Wilbers, R. H., Warmerdam, S., Finkers-Tomczak, A., Diaz-Granados, A., van Schaik, C. C., et al. (2014). Apoplastic venom allergen-like proteins of cyst nematodes modulate the activation of basal plant innate immunity by cell surface receptors. PLoS Pathog. 10 (12), e1004569. doi: 10.1371/journal.ppat.1004569
Luis, J. G., Fletcher, W. Q., Echeverri, F., Abad, T., Kishi, M. P., Perales, A. (1995). New phenalenone-type phytoalexins from Musa acuminata (Colla AAA) grand nain. Nat. Prod. Lett. 6 (1), 23–30. doi: 10.1080/10575639508044083
Luque-Ortega, J. R., Martínez, S., Saugar, J. M., Izquierdo, L. R., Abad, T., Luis, J. G., et al. (2004). Fungus-elicited metabolites from plants as an enriched source for new leishmanicidal agents: antifungal phenyl-phenalenone phytoalexins from the banana plant (Musa acuminata) target mitochondria of Leishmania donovani promastigotes. Antimicrob. Agents Chemother. 48 (5), 1534–1540. doi: 10.1128/AAC.48.5.1534-1540.2004
Mahajan, R., Singh, P., Bajaj, K. (1985). Nematicidal activity of some phenolic compounds against Meloidogyne incognita. Rev. Nématol. 8 (2), 161–164.
Manosalva, P., Manohar, M., von Reuss, S. H., Chen, S., Koch, A., Kaplan, F., et al. (2015). Conserved nematode signalling molecules elicit plant defenses and pathogen resistance. Nat. Commun. 6, 7795. doi: 10.1038/ncomms8795
Marhavý, P., Kurenda, A., Siddique, S., Denervaud Tendon, V., Zhou, F., Holbein, J., et al. (2019). Single-cell damage elicits regional, nematode-restricting ethylene responses in roots. EMBO J. 38 (10), e100972. doi: 10.15252/embj.2018100972
McClure, M. A., Bird, A. F. (1976). The tylenchid (Nematoda) egg-shell: formation of the egg-shell in Meloidogyne javanica. Parasitology 72 (1), 29–39. doi: 10.1017/S003118200004316X
Mejias, J., Truong, N. M., Abad, P., Favery, B., Quentin, M. (2019). Plant proteins and processes targeted by parasitic nematode effectors. Front. Plant Sci. 10, 970. doi: 10.3389/fpls.2019.00970
Melillo, M., Leonetti, P., Leone, A., Veronico, P., Bleve-Zacheo, T. (2011). ROS and NO production in compatible and incompatible tomato-Meloidogyne incognita interactions. Eur. J. Plant Pathol. 130 (4), 489–502. doi: 10.1007/s10658-011-9768-4
Melillo, M. T., Leonetti, P., Bongiovanni, M., Castagnone-Sereno, P., Bleve-Zacheo, T. (2006). Modulation of reactive oxygen species activities and H2O2 accumulation during compatible and incompatible tomato-root-knot nematode interactions. New Phytol. 170 (3), 501–512. doi: 10.1111/j.1469-8137.2006.01724.x
Mendy, B., Wang’ombe, M. W., Radakovic, Z. S., Holbein, J., Ilyas, M., Chopra, D., et al. (2017). Arabidopsis leucine-rich repeat receptor-like kinase NILR1 is required for induction of innate immunity to parasitic nematodes. PLoS Pathog. 13 (4), e1006284. doi: 10.1371/journal.ppat.1006284
Mikkelsen, M. D., Hansen, C. H., Wittstock, U., Halkier, B. A. (2000). Cytochrome P450 CYP79B2 from Arabidopsis catalyzes the conversion of tryptophan to indole-3-acetaldoxime, a precursor of indole glucosinolates and indole-3-acetic acid. J. Biol. Chem. 275 (43), 33712–33717. doi: 10.1074/jbc.M001667200
Mikkelsen, M. D., Naur, P., Halkier, B. A. (2004). Arabidopsis mutants in the C-S lyase of glucosinolate biosynthesis establish a critical role for indole-3-acetaldoxime in auxin homeostasis. Plant J. 37 (5), 770–777. doi: 10.1111/j.1365-313X.2004.02002.x
Milligan, S. B., Bodeau, J., Yaghoobi, J., Kaloshian, I., Zabel, P., Williamson, V. M. (1998). The root knot nematode resistance gene Mi from tomato is a member of the leucine zipper, nucleotide binding, leucine-rich repeat family of plant genes. Plant Cell 10 (8), 1307–1319. doi: 10.1105/tpc.10.8.1307
Milne, D. L., Boshoff, D. N., Buchan, P. W. W. (1965). The nature of resistance of Nicotiana repanda to the root-knot nematode, Meloidogyne javanica. S. Afr. J. Agric. Sci. 8, 557–570.
Mur, L. A., Mandon, J., Persijn, S., Cristescu, S. M., Moshkov, I. E., Novikova, G. V., et al. (2013). Nitric oxide in plants: an assessment of the current state of knowledge. AoB Plants 5 pls052. doi: 10.1093/aobpla/pls052
Naalden, D., Haegeman, A., de Almeida-Engler, J., Birhane Eshetu, F., Bauters, L., Gheysen, G. (2018). The Meloidogyne graminicola effector Mg16820 is secreted in the apoplast and cytoplasm to suppress plant host defense responses. Mol. Plant Pathol. 19 (11), 2416–2430. doi: 10.1111/mpp.12719
Neveu, C., Abad, P., Castagnone-Sereno, P. (2003). Molecular cloning and characterization of an intestinal cathepsin L protease from the root-knot nematode Meloidogyne incognita. Physiol. Mol. Plant Pathol. 63 (3), 159–165. doi: 10.1016/j.pmpp.2003.10.005
Nivsarkar, M., Cherian, B., Padh, H. (2001). Alpha-terthienyl: a plant-derived new generation insecticide. Curr .Sci. 81 (6), 667–672.
Nothnagel, E. A., McNeil, M., Albersheim, P., Dell, A. (1983). Host-pathogen interactions: XXII. A galacturonic acid oligosaccharide from plant cell walls elicits phytoalexins. Plant Physiol. 71 (4), 916–926. doi: 10.1104/pp.71.4.916
Paal, J., Henselewski, H., Muth, J., Meksem, K., Menendez, C. M., Salamini, F., et al. (2004). Molecular cloning of the potato Gro1-4 gene conferring resistance to pathotype Ro1 of the root cyst nematode Globodera rostochiensis, based on a candidate gene approach. Plant J. 38 (2), 285–297. doi: 10.1111/j.1365-313X.2004.02047.x
Palomares-Rius, J. E., Escobar, C., Cabrera, J., Vovlas, A., Castillo, P. (2017). Anatomical alterations in plant tissues induced by plant-parasitic nematodes. Front. Plant Sci. 8, 1987. doi: 10.3389/fpls.2017.01987
Paulson, R. E., Webster, J. M. (1972). Ultrastructure of the hypersensitive reaction in roots of tomato, Lycopersicon esculentum L., to infection by the root-knot nematode, Meloidogyne incognita. Physiol. Plant Pathol. 2 (3), 227–234. doi: 10.1016/0048-4059(72)90005-7
Pegard, A., Brizzard, G., Fazari, A., Soucaze, O., Abad, P., Djian-Caporalino, C. (2005). Histological characterization of resistance to different root-knot nematode species related to phenolics accumulation in Capsicum annuum. Phytopathology 95 (2), 158–165. doi: 10.1094/PHYTO-95-0158
Perry, R. N., Trett, M. W. (1986). Ultrastructure of the eggshell of Heterodera schachtii and H. glycines (Nematoda: Tylenchida). Rev. Nématol. 9 (4), 399–403.
Plowright, R., Grayer, R., Gill, J., Rahman, M., Harborne, J. (1996). The induction of phenolic compounds in rice after infection by the stem nematode Ditylenchus angustus. Nematologica 42 (5), 564–578. doi: 10.1163/004625996X00063
Proite, K., Carneiro, R., Falcão, R., Gomes, A., Leal-Bertioli, S., Guimarães, P., et al. (2008). Post-infection development and histopathology of Meloidogyne arenaria race 1 on Arachis spp. Plant Pathol. 57, 974–980. doi: 10.1111/j.1365-3059.2008.01861.x
Pusztahelyi, T. (2018). Chitin and chitin-related compounds in plant–fungal interactions. Mycology 9 (3), 189–201. doi: 10.1080/21501203.2018.1473299
Qiu, J., Hallmann, J., Kokalis-Burelle, N., Weaver, D. B., Rodríguez-Kábana, R., Tuzun, S. (1997). Activity and differential induction of chitinase isozymes in soybean cultivars resistant or susceptible to root-knot nematodes. J. Nematol. 29 (4), 523–530.
Rice, S. L., Stone, A. R., Leadbeater, B. S. C. (1987). Changes in cell structure in roots of resistant potatoes parasitized by potato cyst nematodes. 2. Potatoes with resistance derived from Solanum vernei. Physiol. Mol. Plant Pathol. 31 (1), 1–14. doi: 10.1016/0885-5765(87)90002-6
Robertson, L., Robertson, W. M., Sobczak, M., Helder, J., Tetaud, E., Ariyanayagam, M. R., et al. (2000). Cloning, expression and functional characterisation of a peroxiredoxin from the potato cyst nematode Globodera rostochiensis. Mol. Biochem. Parasitol. 111 (1), 41–49. doi: 10.1016/S0166-6851(00)00295-4
Rooney, H. C., Van’t Klooster, J. W., van der Hoorn, R. A., Joosten, M. H., Jones, J. D., de Wit, P. J. (2005). Cladosporium Avr2 inhibits tomato Rcr3 protease required for Cf-2-dependent disease resistance. Science 308 (5729), 1783–1786. doi: 10.1126/science.1111404
Sacco, M. A., Koropacka, K., Grenier, E., Jaubert, M. J., Blanchard, A., Goverse, A., et al. (2009). The cyst nematode SPRYSEC protein RBP-1 elicits Gpa2- and RanGAP2-dependent plant cell death. PLoS Pathog. 5 (8), e1000564. doi: 10.1371/journal.ppat.1000564
Scheler, C., Durner, J., Astier, J. (2013). Nitric oxide and reactive oxygen species in plant biotic interactions. Curr. Opin. Plant Biol. 16 (4), 534–539. doi: 10.1016/j.pbi.2013.06.020
Seo, S., Gomi, K., Kaku, H., Abe, H., Seto, H., Nakatsu, S., et al. (2012). Identification of natural diterpenes that inhibit bacterial wilt disease in tobacco, tomato and Arabidopsis. Plant Cell Physiol. 53 (8), 1432–1444. doi: 10.1093/pcp/pcs085
Seo, Y., Park, J., Kim, Y., Park, Y., Kim, Y. (2014). Screening and histopathological characterization of Korean carrot lines for resistance to the root-knot nematode Meloidogyne incognita. Plant Pathol. J. 30 (1), 75–81. doi: 10.5423/PPJ.OA.08.2013.0082
Shah, S. J., Anjam, M. S., Mendy, B., Anwer, M. A., Habash, S. S., Lozano-Torres, J. L., et al. (2017). Damage-associated responses of the host contribute to defence against cyst nematodes but not root-knot nematodes. J. Exp. Bot. 68 (21-22), 5949–5960. doi: 10.1093/jxb/erx374
Shi, Q., Mao, Z., Zhang, X., Wang, Y., Ling, J., Lin, R., et al. (2018). A Meloidogyne incognita effector MiISE5 suppresses programmed cell death to promote parasitism in host plant. Sci. Rep. 8 (1), 7256. doi: 10.1038/s41598-018-24999-4
Siddique, S., Matera, C., Radakovic, Z. S., Hasan, M. S., Gutbrod, P., Rozanska, E., et al. (2014). Parasitic worms stimulate host NADPH oxidases to produce reactive oxygen species that limit plant cell death and promote infection. Sci. Signal 7 (320), ra33. doi: 10.1126/scisignal.2004777
Smant, G., Helder, J., Goverse, A. (2018). Parallel adaptations and common host cell responses enabling feeding of obligate and facultative plant parasitic nematodes. Plant J. 93 (4), 686–702. doi: 10.1111/tpj.13811
Sobczak, M., Avrova, A., Jupowicz, J., Phillips, M. S., Ernst, K., Kumar, A. (2005). Characterization of susceptibility and resistance responses to potato cyst nematode (Globodera spp.) infection of tomato lines in the absence and presence of the broad-spectrum nematode resistance Hero gene. Mol. Plant Microbe Interact. 18 (2), 158–168. doi: 10.1094/MPMI-18-0158
Takasugi, M., Yachida, Y., Anetai, M., Masamune, T., Kegasawa, K. (1975). Identification of asparagusic acid as a nematicide occuring naturally in the roots of asparagus. Chem. Lett. 1, 43–44. doi: 10.1246/cl.1975.43
Tameling, W. I., Baulcombe, D. C. (2007). Physical association of the NB-LRR resistance protein Rx with a Ran GTPase-activating protein is required for extreme resistance to Potato virus X. Plant Cell 19 (5), 1682–1694. doi: 10.1105/tpc.107.050880
Teixeira, M. A., Wei, L., Kaloshian, I. (2016). Root-knot nematodes induce pattern-triggered immunity in Arabidopsis thaliana roots. New Phytol. 211 (1), 276–287. doi: 10.1111/nph.13893
Thomason, I. J., Rich, J. R., O’Melia, F. C. (1976). Pathology and histopathology of Pratylenchus scribneri infecting snap bean and lima bean. J. Nematol. 8 (4), 347–352.
Torres, M. A., Dangl, J. L. (2005). Functions of the respiratory burst oxidase in biotic interactions, abiotic stress and development. Curr. Opin. Plant Biol. 8 (4), 397–403. doi: 10.1016/j.pbi.2005.05.014
Torres, M. A., Jones, J. D., Dangl, J. L. (2006). Reactive oxygen species signaling in response to pathogens. Plant Physiol. 141 (2), 373–378. doi: 10.1104/pp.106.079467
Tsai, A. Y., Higaki, T., Nguyen, C. N., Perfus-Barbeoch, L., Favery, B., Sawa, S. (2019). Regulation of root-knot nematode behavior by seed-coat mucilage-derived attractants. Mol. Plant 12 (1), 99–112. doi: 10.1016/j.molp.2018.11.008
Urwin, P. E., Lilley, C. J., McPherson, M. J., Atkinson, H. J. (1997). Characterization of two cDNAs encoding cysteine proteinases from the soybean cyst nematode Heterodera glycines. Parasitology 114 (6), 605–613. doi: 10.1017/S0031182097008858
Urwin, P. E., Levesley, A., Michael, J., McPherson, M. J., Atkinson, H. J. (2000). Transgenic resistance to the nematode Rotylenchulus reniformis conferred by Arabidopsis thaliana plants expressing proteinase inhibitors. Mol. Breed 6 (3), 257–264. doi: 10.1023/A:1009669325944
Urwin, P. E., Green, J., Atkinson, H. J. (2003). Expression of a plant cystatin confers partial resistance to Globodera, full resistance is achieved by pyramiding a cystatin with natural resistance. Mol. Breed 12 (3), 263–269. doi: 10.1023/A:1026352620308
van der Vossen, E. A., van der Voort, J. N., Kanyuka, K., Bendahmane, A., Sandbrink, H., Baulcombe, D. C., et al. (2000). Homologues of a single resistance-gene cluster in potato confer resistance to distinct pathogens: a virus and a nematode. Plant J. 23 (5), 567–576. doi: 10.1046/j.1365-313x.2000.00814.x
Waetzig, G., Sobczak, M., Grundler, F. (1999). Localization of hydrogen peroxide during the defence response of Arabidopsis thaliana against the plant-parasitic nematode Heterodera glycines. Nematology 1, 681–686. doi: 10.1163/156854199508702
Wang, K.-H., Hooks, C. R., Ploeg, A. (2007). “Protecting crops from nematode pests: using marigold as an alternative to chemical nematicides,” in Plant Disease; PD-35 (Honolulu: University of Hawaii).
Warmerdam, S., Sterken, M. G., van Schaik, C., Oortwijn, M. E. P., Sukarta, O. C. A., Lozano-Torres, J. L., et al. (2018). Genome-wide association mapping of the architecture of susceptibility to the root-knot nematode Meloidogyne incognita in Arabidopsis thaliana. New Phytol. 218 (2), 724–737. doi: 10.1111/nph.15034
Wuyts, N., Lognay, G., Swennen, R., De Waele, D. (2006a). Nematode infection and reproduction in transgenic and mutant Arabidopsis and tobacco with an altered phenylpropanoid metabolism. J. Exp. Bot. 57 (11), 2825–2835. doi: 10.1093/jxb/erl044
Wuyts, N., Swennen, R., De Waele, D. (2006b). Effects of plant phenylpropanoid pathway products and selected terpenoids and alkaloids on the behaviour of the plant-parasitic nematodes Radopholus similis, Pratylenchus penetrans and Meloidogyne incognita. Nematology 8, 89–101. doi: 10.1163/156854106776179953
Wyss, U., Grundler, F. M. W., Munch, A. (1992). The parasitic behaviour of second-stage juveniles of Meloidogyne incognita in roots of Arabidopsis thaliana. Nematologica 38, 98–111. doi: 10.1163/187529292X00081
Yan, G. P., Baidoo, R. (2018). Current research status of Heterodera glycines resistance and its implication on soybean breeding. Engineering 4 (4), 534–541. doi: 10.1016/j.eng.2018.07.009
Yang, S., Dai, Y., Chen, Y., Yang, J., Yang, D., Liu, Q., et al. (2019). A novel G16B09-like effector from Heterodera avenae suppresses plant defenses and promotes parasitism. Front. Plant Sci. 10, 66. doi: 10.3389/fpls.2019.00066
Ye, D. Y., Qi, Y. H., Cao, S. F., Wei, B. Q., Zhang, H. S. (2017). Histopathology combined with transcriptome analyses reveals the mechanism of resistance to Meloidogyne incognita in Cucumis metuliferus. J. Plant Physiol. 212, 115–124. doi: 10.1016/j.jplph.2017.02.002
Yu, Q., Tsao, R., Chiba, M., Potter, J. (2005). Selective nematicidal activity of allyl isothiocyanate. J. Food Agr. Environ. 3 (2), 218–221.
Zasada, I. A., Ferris, H. (2003). Sensitivity of Meloidogyne javanica and Tylenchulus semipenetrans to isothiocyanates in laboratory assays. Phytopathology 93 (6), 747–750. doi: 10.1094/PHYTO.2003.93.6.747
Zhang, Y., Foster, J. M., Nelson, L. S., Ma, D., Carlow, C. K. (2005). The chitin synthase genes chs-1 and chs-2 are essential for C. elegans development and responsible for chitin deposition in the eggshell and pharynx, respectively. Dev. Biol. 285 (2), 330–339. doi: 10.1016/j.ydbio.2005.06.037
Zhou, J., Jia, F., Shao, S., Zhang, H., Li, G., Xia, X., et al. (2015). Involvement of nitric oxide in the jasmonate-dependent basal defense against root-knot nematode in tomato plants. Front. Plant Sci. 6, 193. doi: 10.3389/fpls.2015.00193
Zhou, J., Xu, X. C., Cao, J. J., Yin, L. L., Xia, X. J., Shi, K., et al. (2018). Heat shock factor HsfA1a is essential for R gene-mediated nematode resistance and triggers H2O2 production. Plant Physiol. 176 (3), 2456–2471. doi: 10.1104/pp.17.01281
Keywords: pattern-triggered immunity, NLR-triggered immunity, anti-nematode enzymes, anti-nematode compounds, cell wall reinforcement, reactive oxygen species, nitric oxide, hypersensitive response cell death
Citation: Sato K, Kadota Y and Shirasu K (2019) Plant Immune Responses to Parasitic Nematodes. Front. Plant Sci. 10:1165. doi: 10.3389/fpls.2019.01165
Received: 20 June 2019; Accepted: 26 August 2019;
Published: 26 September 2019.
Edited by:
Bruno Favery, Institut National de la Recherche Agronomique (INRA), FranceReviewed by:
Shahid Siddique, University of Bonn, GermanyCécile Segonzac, Seoul National University, South Korea
Copyright © 2019 Sato, Kadota and Shirasu. This is an open-access article distributed under the terms of the Creative Commons Attribution License (CC BY). The use, distribution or reproduction in other forums is permitted, provided the original author(s) and the copyright owner(s) are credited and that the original publication in this journal is cited, in accordance with accepted academic practice. No use, distribution or reproduction is permitted which does not comply with these terms.
*Correspondence: Yasuhiro Kadota, eWFzdWhpcm8ua2Fkb3RhQHJpa2VuLmpw; Ken Shirasu, a2VuLnNoaXJhc3VAcmlrZW4uanA=