- 1Laboratoire de Biologie et Pathologie Végétales, Université de Nantes, Nantes, France
- 2Division of Biological Science, Graduate School of Science and Technology, Nara Institute of Science and Technology, Ikoma, Nara, Japan
- 3Institute for Research Initiatives, Division for Research Strategy, Nara Institute of Science and Technology, Ikoma, Nara, Japan
- 4RIKEN Center for Sustainable Resource Science, Yokohama, Japan
- 5Graduate School of Science, The University of Tokyo, Tokyo, Japan
Parasitic plants in the Orobanchaceae family include devastating weed species, such as Striga, Orobanche, and Phelipanche, which infest important crops and cause economic losses of over a billion US dollars worldwide, yet the molecular and cellular processes responsible for such parasitic relationships remain largely unknown. Parasitic species of the Orobanchaceae family form specialized invasion organs called haustoria on their roots to enable the invasion of host root tissues. The process of forming haustoria can be divided into two steps, prehaustorium formation and haustorium maturation, the processes occurring before and after host attachment, respectively. Prehaustorium formation is provoked by host-derived signal molecules, collectively called haustorium-inducing factors (HIFs). Cell wall-related quinones and phenolics have been known for a long time to induce haustoria in many Orobanchaceae species. Although such phenolics are widely produced in plants, structural specificities exist among these molecules that modulate their competency to induce haustoria in different parasitic plant species. In addition, the plant hormone cytokinins, structurally distinct from phenolic compounds, also trigger prehaustorium formation in Orobanchaceae. Recent findings demonstrate their involvement as rhizopsheric HIFs for Orobanche and Phelipanche species and thus address new activities for cytokinins in haustorium formation in Orobanchaceae, as well as in rhizospheric signaling. This review highlights haustorium-inducing signals in the Orobanchaceae family in the context of their host origin, action mechanisms, and species specificity.
Introduction
Limitations in soil fertility have influenced the diversification of nutrient acquisition strategies in plants (Zemunik et al., 2015). Parasitic plants develop haustoria (singular haustorium), specialized organs for nutrient acquisition from host plants (Yoshida et al., 2016). Although the term haustoria is commonly used in biotrophic plant-pathogenic fungi or oomycetes and both fungi and parasitic plant haustoria have functions for nutrient withdrawing, the morphology and organization of haustoria in these organisms are quite different. The fungal haustoria represent unicellular hyphae surrounded by host-derived extrahaustorial membrane, whereas haustoria of parasitic plants are multicellular organs that consist of different cell types and invade host tissues intercellularly (Yoshida et al., 2016). Among angiosperms, there are approximately 4,500 species of parasitic plants that have the ability to form haustoria and connect their vasculatures with those of their hosts to obtain water and nutrition. Parasitic plants have evolved independently at least 12 times and can be classified into two groups, root parasites and stem parasites, depending on which host tissue is parasitized (Westwood et al., 2010). The Orobanchaceae family contains the largest number of parasitic plants and consists of root parasites with various degrees of host dependency and photosynthetic activity, i.e., facultative parasites, obligate hemiparasites, and obligate holoparasites. Facultative parasites include species that have the ability for an autotrophic lifestyle and opportunistically parasitize host plants. Obligate parasites include species that cannot complete their life cycles without host plants; those who have photosynthetic activity are called hemiparasites, and those who have lost photosynthetic ability are called holoparasites (Westwood et al., 2010). Some obligate parasitic plants in the Orobanchaceae family, especially in the genera of Striga, Orobanche, and Phelipanche, parasitize crop species and cause devastating yield losses. Striga species infect maize, sorghum, and upland rice especially in sub-Saharan Africa with the economic damage caused by Striga estimated to be about 1 billion US dollars per year (Spallek et al., 2013). Orobanche and Phelipanche species infect economically important crops including pea, faba bean, sunflower, and oilseed rape (Fernández-Aparicio et al., 2016a). Germination of these obligate parasites depends on stimulating compounds including strigolactones and strigolactone-like compounds exuded by the roots of host plants (Yoneyama et al., 2010). Signaling components involved in strigolactone perception in Orobanchaceae are found to be associated with their germination (Lechat et al., 2015; Brun et al., 2018; Brun et al., 2019). In comparison, the molecular basis underlying haustorium formation remains mostly unknown. Nevertheless, recent studies have identified several haustorium-inducing chemicals for various Orobanchaceae members and have characterized their structural commonality and species specificity. This review will focus on the early developmental processes for haustorium formation in the Orobanchaceae family.
Haustorium Developmental Processes
According to the parasite species, two kinds of haustoria can be observed in the Orobanchaceae family: one is a “terminal haustorium” that develops on the radicle tip, and the other is a “lateral haustorium” that develops as a lateral extension of primary and lateral roots of parasitic plants (Joel, 2013; Yoshida et al., 2016). Most facultative parasites in the Orobanchaceae form lateral haustoria, while terminal haustoria are characteristic of several obligate holo- and hemiparasite clades, such as Orobanche, Phelipanche, Striga, and Alectra (Joel, 2013). Despite the structural variation among haustoria, haustorial developmental processes seem largely conserved among species.
Haustorium formation processes can be divided into two phases: prehaustorium formation induced by haustorium-inducing factors (HIFs) and haustorium maturation upon host infection. Except for some species that can form self-haustoria (Xiang et al., 2018), the initial step of haustorium formation is provoked by host-derived small compounds, collectively called HIFs. Several hours after recognition of HIFs, parasite roots or radicles start to show morphological changes including cell expansion, cell division, and differentiation of haustorial hairs in hemiparasites or papillae in holoparasitic genera Orobanche and Phelipanche spp., and semi-spherically shaped prehaustorial organs are formed within a few days (Riopel and Musselman, 1979; Baird and Riopel, 1985; Cui et al., 2016; Ishida et al., 2016; Goyet et al., 2017). The resultant organ is defined as “prehaustorium” or an “early haustorial structure” due to its premature architecture. Haustorium maturation occurs only after host attachment and is not solely promoted by HIFs, indicating the requirement of additional host signal(s) other than HIFs for structural maturation. Once the haustorium reaches host tissues, the epidermal cells of the parasite haustorium apex differentiate into intrusive cells, the specialized cells for host invasion with characteristic elongated shapes (Figure 1) (Stephens, 1912; Olivier et al., 1991). Intrusive cells grow inside host tissues toward host vasculatures. Upon reaching the host vasculature, some of the adjacent parasite cells differentiate into tracheary elements and form a xylem connection between the host and the parasite (Dorr, 1997), a structure called xylem bridge. Cell lineage analysis using the facultative parasite Phtheirospermum japonicum revealed dynamic cell fate transitions during haustorium formation (Wakatake et al., 2018). Intrusive cells originate from epidermal cells, and the central part of the haustorium has small cells with procambium-like cell identity as indicated by marker gene expression (Wakatake et al., 2018). Notably, development of such internal structures is only observed concomitant with host interaction; some incompatible hosts can interfere with the formation of intrusive cells and the subsequent xylem bridge (Figure 1) (Olivier et al., 1991; Yoshida and Shirasu, 2009), rendering these cells minimal prerequisites for establishing parasitism. Transcriptome analysis of P. japonicum revealed that YUCCA3, a gene encoding a key enzyme for auxin biosynthesis, is induced by HIF treatment in the epidermal cell layer of the haustorium-forming site (Ishida et al., 2016). Artificial induction of YUCCA3 expression within epidermal cells of P. japonicum roots can induce haustorium-like semi-spherical structures with haustorial hair proliferation (Ishida et al., 2016), indicating the involvement of auxin biosynthesis in organogenesis of the prehaustorium.
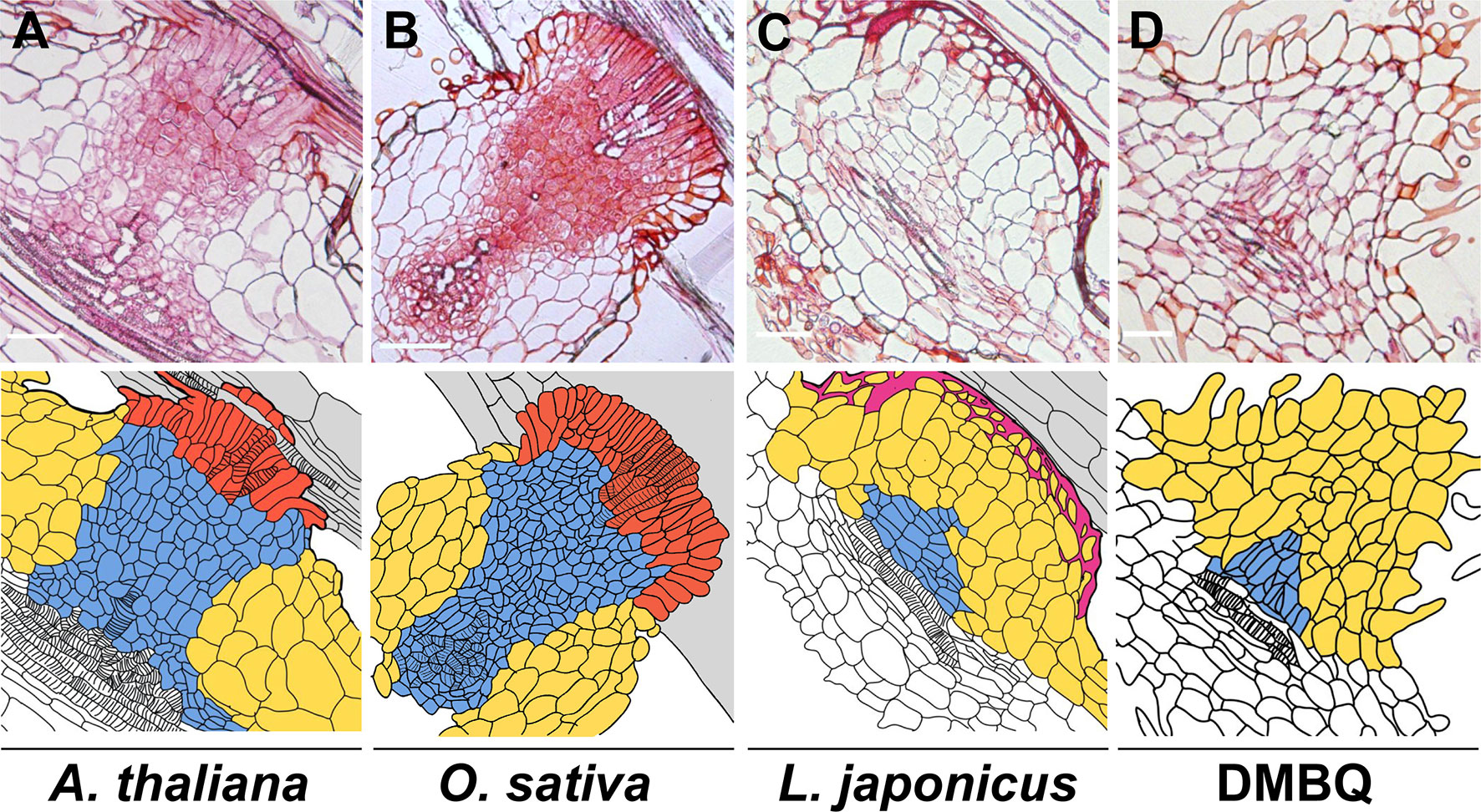
Figure 1 Haustorial cell structures formed upon infection of various plants or induced by DMBQ. The upper photos show the longitudinal sections of P. japonicum haustoria after infection of susceptible plants (Arabidopsis thaliana (A) and Oryza sativa (B)) and a resistant plant (Lotus japonicus (C)) or induction by DMBQ treatment (D). The lower drawings show the cell outlines of the upper sections. The colors denote cell types. Red, blue, yellow, purple, and gray indicate intrusive cells, small (procambium-like) cells, large cells, lignin accumulation, and host root cells, respectively. Stripes mark the location of xylem cells. Bars = 100 µm.
HIFs for Hemiparasites
Induction of prehaustoria by host-derived HIFs is an initial step for haustorium formation. Isolation of HIFs has been accomplished mainly using photosynthetic hemiparasitic species whose prehaustoria are easy to observe due to their characteristic shape and their proliferation of haustorial hairs. HIF activities have been recognized in plant exudates since the 1970s from studies showing that root exudates of numerous plant species were able to induce prehaustoria in the facultative parasites Agalinis purpurae and Castilleja exserta (previously known as Orthocarpus purpurascensi) (Atsatt et al., 1978; Riopel and Musselman, 1979). Several small molecules were afterwards reported to be HIFs (Figure 2). Two flavonoids, named xenognosin A and B, were isolated from gum tragacanth, an exudate of Astragalus spp., as HIFs for Agalinis (Lynn et al., 1981; Steffens et al., 1982). Further attempts to identify HIFs yielded a terpenoid soyasapogenol B from root exudates of Lespedeza sericea (Fabaceae), a host of Agalinis (Steffens et al., 1983); however, the activity was not sufficiently high to account for the entire host exudate activity (Lynn, 1985; Steffens et al., 1986). A few years later, a quinone 2,6-dimethoxy-1,4-benzoquinone (DMBQ) was isolated from root extracts of sorghum, a natural host for Striga, and found to be a HIF for Striga asiatica and Agalinis (Chang and Lynn, 1986). As DMBQ could not be detected from a healthy root exudate in that report, it has remained questionable whether DMBQ is a naturally occurring HIF. Nevertheless, DMBQ has the highest activity and widest parasite range among currently known HIFs. For example, DMBQ is able to induce haustoria in the obligate hemiparasite Striga spp., as well as in the facultative parasites P. japonicum, Triphysaria spp., and Agalinis spp.(Chang and Lynn, 1986; Ishida et al., 2011; Tomilov et al., 2004). Compounds with structures similar to DMBQ, such as phenolic acids (including syringic acid, vanillic acid, and ferulic acid), aldehydes (including syringaldehyde), and flavonoids (including peonidin), were reported also to induce haustoria in Triphysaria versicolor, P. japonicum, and Striga hermonthica (Albrecht et al., 1999; Cui et al., 2018). Castilleja tenuiflora was reported to react with vanillic acid as well as catechin and hydrogen peroxide (H2O2) (Salcedo-Morales et al., 2013), although H2O2 alone does not induce haustoria in S. hermonthica (Wada et al., 2019).
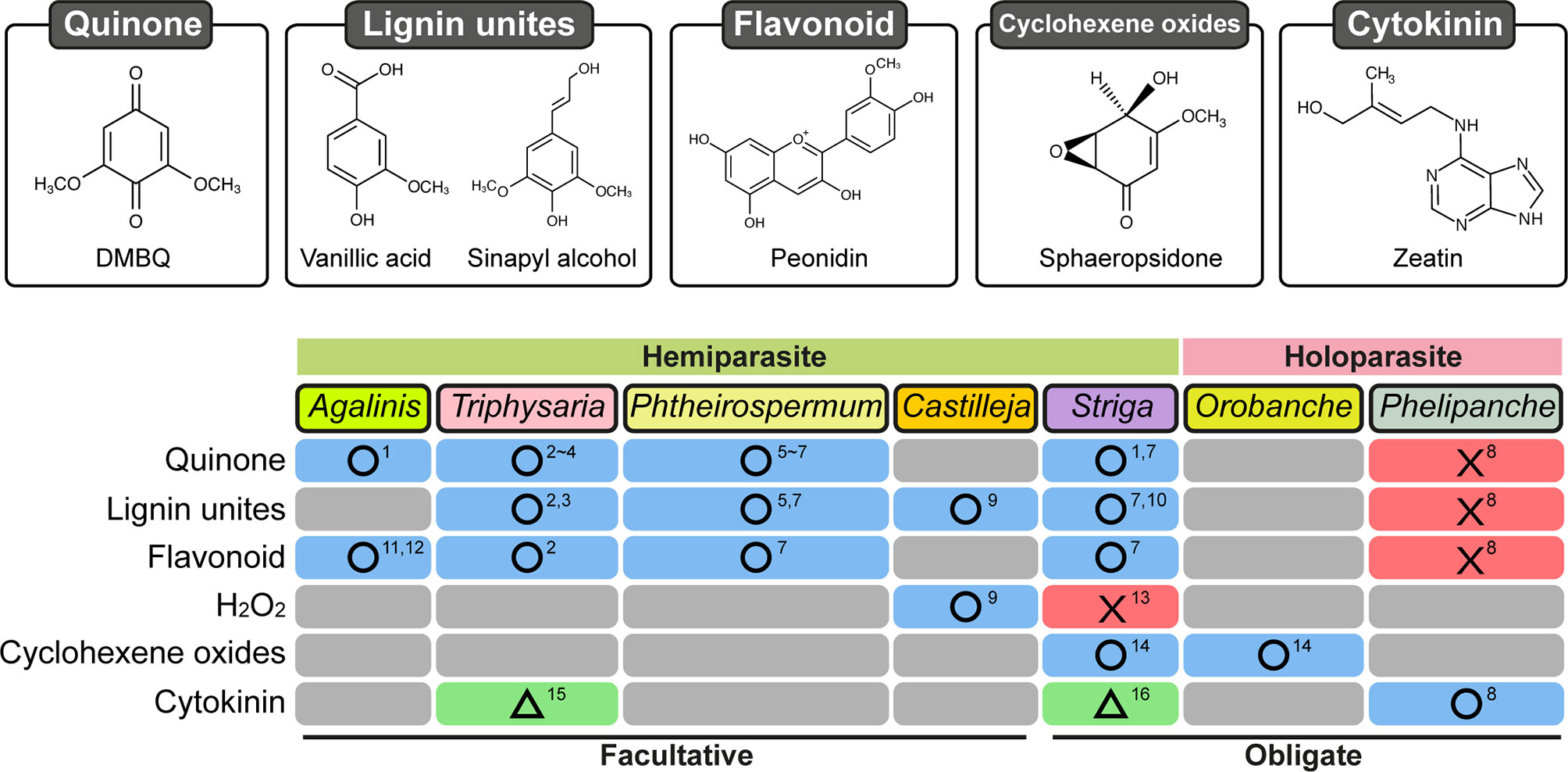
Figure 2 Response of Orobanchaceae parasitic plants treated with various chemicals for haustorium induction. Chemical categories previously reported as HIFs are shown on the left. Blue boxes with circles: at least one chemical was reported in the corresponding species. Red boxes with crosses: tested chemical(s) did not induce haustoria. Green boxes with triangles: haustoria-like structures were reported. Gray boxes: not reported. Numbers indicate references: 1, Chang and Lynn (1986); 2, Albrecht et al. (1999); 3, Bandaranayake et al. (2010); 4, Bandaranayake et al. (2012); 5, Cui et al. (2016); 6, Ishida et al. (2016); 7, Cui et al. (2018); 8, Goyet et al. (2017); 9, Salcedo-Morales et al. (2013); 10, Lynn and Chang (1990); 11, Lynn et al. (1981); 12, Steffens et al. (1982); 13, Wada et al. (2019); 14, Fernández-Aparicio et al. (2016b); 15, Wrobel and Yoder (2001); 16, Keyes et al. (2000).
Because highly active HIFs in host exudates have yet to be identified, localization of HIF production and mode of action of HIFs remain largely unknown. Although some reports suggest that HIF activity is related to the pectin fraction of host cell walls (Keyes et al., 2000), the structures of HIFs are more closely related with those of lignin monomers. Lignin is a complex polymer abundant in the secondary cell walls of vascular plants and is composed of aromatic monolignols, i.e., p-coumaryl alcohol, coniferyl alcohol, and sinapyl alcohol, derived from the phenylpropanoid pathway (Vanholme et al., 2010). Polymerization of monolignols results in three major types of generic lignin units, p-hydroxyphenyl (H), guaiacyl (G), and syringil (S) units, which respectively contain zero, one, and two methoxy groups on the aromatic rings. A series of lignin precursors and lignin-derived phenolics were tested for their ability to induce haustoria in S. hermonthica and P. japonicum (Cui et al., 2018). Interestingly, S-type compounds that have two methoxy groups at the 3 and 5 positions and a hydroxyl group at position 4 of the aromatic ring, e.g., sinapaldehyde, synapyl alcohol, syringic acid, and acetosyringone, can induce haustoria in both S. hermonthica and P. japonicum (Cui et al., 2018). In contrast, G-type compounds that have one methoxy group at the 3 or 5 position and a hydroxyl group at position 4, including ferulic acid, vanillic acid, vanillin, and apocynin, have a high capacity for inducing haustoria in S. hermonthica but not in P. japonicum. H-type compounds that do not have a methoxy group generally do not show haustorium-inducing activity for either species (Cui et al., 2018). These results suggest that there are certain species specificities for HIF recognition. Consistently, purified natural lignin polymers as well as artificially synthesized lignin polymers composed of only G-units can induce haustoria only in S. hermonthica, and those containing G and S units can induce haustoria in both P. japonicum and S. hermonthica (Cui et al., 2018). Because the prehaustorium-inducing activity of lignin polymers is greatly enhanced by application of white rot fungi-derived laccase, an enzyme that can produce monolignols and quinones via oxidative lignin depolymerization (Pollegioni et al., 2015), monomeric phenolics or quinones are more likely to be the active compounds in vivo (Cui et al., 2018). These results could also explain the high HIF activity for Striga in the pectin fraction from sorghum cell walls (Keyes et al., 2000). The primary cell wall (pectin-rich) fraction from Poaceae species is known to contain abundant amounts of ester-linked ferulic acid, which acts as a HIF for Striga (Harris and Trethewey, 2010). Alteration of the lignin monomer composition in a host using genetic modification affects the haustorium-induction activity of P. japonicum and S. hermonthica (Cui et al., 2018), indicating that HIFs naturally produced in hosts originate at least partly from lignin biosynthesis or degradation pathways, although details of the origin of HIFs need to be further investigated in the future.
HIFs for Holoparasites
HIFs have been less studied in holoparasitic Orobanchaceae partly because their prehaustorial structures are less apparent than hemiparasites that proliferate haustorial hairs. Prehaustorium induction in Orobanche and Phelipanche is nevertheless characterized by radicle growth arrest, radicle tip swelling, and extension of epidermal cells to form secretory papillae bearing a host-attachment function similar to that of haustorial hairs in hemiparasites (Baird and Riopel, 1985; Joel and Losner-Goshen, 1994; Fernández-Aparicio et al., 2016a; Fernández-Aparicio et al., 2016b; Goyet et al., 2017). Because a study reported in vitro host-independent prehaustorium induction in the species Orobanche cumana and Phelipanche aegyptiaca (Joel and Losner-Goshen, 1994), prehaustorium induction in holoparasites has been considered for a long time as a host-independent process. However, recent findings in the species Phelipanche ramosa demonstrate that root exudates collected from healthy oilseed rape plants induce prehaustorium formation in vitro (Goyet et al., 2017). A similar observation has been made for the species O. cumana in response to sunflower root exudates (Montiel & Simier, personal communication). These recent findings therefore imply that prehaustorium induction by host-derived chemical signals or molecules occurring in the rhizosphere is a common process in both hemiparasitic and holoparasitic Orobanchaceae.
However, the currently known HIFs for hemiparasites, including DMBQ, syringic acid, and vanillic acids, are inefficient for inducing prehaustoria in P. ramosa (Goyet et al., 2017). In addition, DMBQ is also inactive towards P. aegyptiaca (Westwood et al., 2010). Recently, the mycotoxins sphaeropsidone and epi-sphaeropsidone, which are cyclohexene oxides isolated from Diplodia cupressi (Figure 2), the causal agent for cypress (Cupressus sempervirens L.) canker (Evidente et al., 1998), were shown to bear HIF activity in vitro towards Orobanche crenata and O. cumana (Fernández-Aparicio et al., 2016a; Fernández-Aparicio et al., 2016b). Moreover, according to structure-activity relationship analyses, structural modifications of sphaeropsidone and epi-sphaeropsidone affect differently prehaustorium induction in O. crenata and in O. cumana (Fernández-Aparicio et al., 2016b). As reported in the hemiparasitic Orobanchaceae, these findings suggest that certain species specificities for HIF recognition also occur in the holoparasite Orobanche. Nevertheless, these compounds are also active in vitro towards Striga while being structurally different from the currently known HIFs for hemiparasites. Although activity towards Striga could be possibly due to the conversion of sphaeropsidone and its derivatives to active 3-methoxyquinones (Fernández-Aparicio et al., 2016b), this eventually suggests that Orobanche may have a more strict specificity for HIF compounds than Striga.
Cytokinins As HIFs
Cytokinins are known to be important phytohormones acting in a vast array of plant development processes including root development as well as nodule formation during plant-bacteria symbiosis (Pacifici et al., 2015). Cytokinins were detected in the rhizosphere of many plants including maize, rice, and tomato (Davey and van Staden, 1976; van Staden and Dimalla, 1976; Soejima et al., 1992; Yang et al., 2002; Kirwa et al., 2018), and interestingly, cytokinins are also effective in vitro for inducing prehaustorium-like structures in hemiparasites and prehaustoria in holoparasites (Figure 2). Kinetin, a synthetic cytokinin, and 6-benzylaminopurine (BAP) were indeed reported to induce prehaustoria-like structures in S. asiatica and in T. versicolor, respectively (Keyes et al., 2000; Wrobel and Yoder, 2001). However, some morphological differences between phenolic HIF-induced and cytokinin-induced prehaustoria were underlined; e.g., in T. versicolor, cytokinin-induced prehaustoria have smaller swelled structures than those induced by DMBQ. All these findings made questionable the involvement of cytokinins in cooperation with phenolic HIFs as prehaustorium inducers for these hemiparasitic species (Estabrook and Yoder, 1998).
However, recent analyses on prehaustorium formation in the holoparasite P. ramosa parasitizing oilseed rape showed clear evidences of the existence of a signal carrying both cytokinin and HIF activities in oilseed rape rhizosphere (Goyet et al., 2017). Bioguided ultra-performance liquid chromatography-electrospray tandem mass spectrometry (UPLC-ESI(+)-/MS/MS) analyses showed that oilseed rape root constitutively exudes a cytokinin with dihydrozeatin characteristics. In parallel, cytokinins at physiological levels (10−7–10−8 M) were effective for prehaustorium induction in P. ramosa. Moreover, as expected from its antagonist activity toward cytokinins, auxin treatment prevented prehaustorium formation in response to cytokinins or root exudates. Gene expression analysis after treatment with oilseed rape root exudates or exogenous t/zeatine confirmed that plant hormone-related genes, including cytokinin-related genes (notably PrRR5, PrCKX2 and PrCKX4), were up-regulated during prehaustoria induction (Goyet et al., 2017). These findings highlight cytokinins as bona fide rhizosphere signals for holoparasites and that the possible roles for hemiparasite prehaustorium induction need to be re-investigated.
Action Mechanisms of HIFs in Prehaustorium Induction
Most of the currently known phenolic HIFs for hemiparasites contain an aromatic ring with a hydroxyl group at position 4 and one or two methoxy groups at positions 3 and/or 5 (Figure 2). Variation of the functional group at position 1 could affect the haustorium induction activity (Cui et al., 2018), probably due to modification of the redox range of the molecules (Smith et al., 1996), but a structural requirement at position 1 is not apparent. Lignin-related HIFs, syringic acid and syringaldehyde, were reported to be oxidized to produce DMBQ in the presence of peroxidase or laccase in vitro (Frick et al., 1996; Ibrahim et al., 2013). Kim et al. (1998) identified peroxidase activity in a Striga cell wall fraction that is able to convert syringic acid to DMBQ. Furthermore, quinone oxidoreductase (QR) genes were upregulated in the parasite after DMBQ treatment and/or host infection. Two types of QR (QR1 and QR2) were isolated from T. versicolor, P. japonicum, and S. hermonthica. QR1 reduces one electron from quinone and converts it to a semiquinone, whereas QR2 is thought to reduce two electrons from quinone to hydroquinone (Bandaranayake et al., 2010; Ishida et al., 2017). In T. versicolor, QR1 expression is upregulated by DMBQ and host exudates, whereas QR2 is induced by DMBQ but not by host exudates (Matvienko et al., 2001). In P. japonicum and S. hermonthica, QR2 but not QR1 expression is upregulated upon both DMBQ treatment and host infection (Ishida et al., 2017). Knockdown of QR1 expression in T. versicolor and QR2 expression in P. japonicum resulted in reduced haustoria formation (Bandaranayake et al., 2010; Ishida et al., 2017). Although it is uncertain why the different types of QRs are involved in haustoria formation in different plant species, the likely scenario is that electron transfer by a QR function is crucial for haustorium initiation.
Oxidation-reduction cycles produce reactive oxygen species (ROS), which may act as signals for haustorium induction (Bandaranayake et al., 2010). To investigate the roles of ROS in haustorium formation, pharmacological analyses were conducted. Application of catalase, a scavenger of H2O2, reduced haustorium induction of S. asiatica by syringic acid but not DMBQ, suggesting that H2O2 is important for conversion of syringic acid to DMBQ (Keyes et al., 2000). However, a similar experiment using S. hermonthica showed that catalase reduces haustorium induction by both DMBQ and syringic acid (Wada et al., 2019), indicating that ROS are also necessary downstream of DMBQ. Application of a series of ROS inhibitors and scavengers revealed that reduced nicotinamide adenine dinucleotide phosphate (NADPH) oxidase inhibitors, especially diphenyleneiodonium (DPI), could efficiently reduce haustorium formation in S. hermonthica (Wada et al., 2019). NADPH oxidase is an enzyme that produces O2−, which is further converted to H2O2 by the superoxide dismutase (SOD) enzyme. An inhibitor of SOD also reduced haustorium formation, indicating that H2O2 production plays a pivotal role for prehaustorium induction in response to DMBQ. Furthermore, peroxidase inhibitors also reduced the haustorium formation rates, whereas exogenous application of peroxidase increased the haustorium formation rates (Wada et al., 2019). These results suggest that ROS modulation via peroxidase may regulate HIF-derived signaling, but how ROS and peroxidases interact with HIF perception and signal transduction in the context of haustorium initiation remains to be elucidated.
In the holoparasite P. ramosa, transcriptomic analysis focusing on time points surrounding prehaustorium formation in response to HIF-containing host root exudates did not reveal any over-representation of ROS-related Gene Ontology (GO) terms. Therefore, involvement of ROS in prehaustorium induction in holoparasites remains obscure.
Roles of HIFs in Host-Parasitic Plant Interactions After Prehaustorium Induction
The roles of HIFs in other functions during the host plant-parasitic plant interaction besides prehaustorium induction have not been well studied yet. Cytokinins may have roles during invasion by P. ramosa because pre-treatment of germinated seeds of P. ramosa with cytokinins increased the number of parasites invading host tissues. Furthermore, pre-treatment with PI-55, an inhibitor of cytokinin signaling (Spíchal et al., 2009), reduced the number of invading parasites (Goyet et al., 2017). Increased successful invasion rates, regarded as P. ramosa aggressiveness, upon cytokinin treatment could be due to either modification of prehaustoria formation rates or modification of haustorium functioning. In addition, cytokinins have been reported to manipulate host physiology after successful host penetration (Spallek et al., 2017). Upon infection, trans-zeatin-type cytokinins accumulate in both P. japonicum and Arabidopsis thaliana. Analysis of Arabidopsis mutants defective in cytokinin biosynthesis indicated that the accumulated cytokinins in host plants were delivered by the parasites (Spallek et al., 2017). Cytokinin accumulation in the host causes hypertrophy of the infected area of the host and is characterized by an increase in vascular diameter and cell number (Spallek et al., 2017). These morphological and physiological changes of host vascular would lead to increased sink strength at haustorium attachment site, which could contribute to nutrient uptake by the parasitic plant.
Many phenolic HIFs are lignin precursors and, therefore, can be incorporated into lignin polymers in the secondary cell walls of hosts or parasitic plants. Lignin is known to be involved in physical defense against various pathogens including bacteria, fungi, plant-parasitic nematodes, and parasitic plants (Goldwasser et al., 1999; Bhuiyan et al., 2009; Miedes et al., 2014; Khanam et al., 2018; Mutuku et al., 2019). Lignin is deposited at the Striga infection site in a Striga-resistant rice cultivar, and lignin modification in the rice host affects resistance against Striga (Mutuku et al., 2019). Thus, phenolic HIFs may be used by hosts for forming a physical barrier against parasitic plants after parasite attack.
Redox cycling between quinones and hydroquinones occurs in the rhizosphere with a great impact on plant-microbe interactions (Taran et al., 2019). Such redox cycling is known to be used as a lignocellulolytic agent by wood-decaying brown rot fungi. A variation of DMBQ, 2,5-dimethoxy-p-benzoquinone (2,5-DMBQ) and its reduced form 2,5-dimethoxy-p-hydroquinone (DMHQ) were detected in wafers of aspen wood colonized by the brown rot fungus Serpula lacrymans (Korripally et al., 2013). Oxidation of DMHQ to 2,5-DMBQ drives a Fenton reaction: H2O2 + Fe2+ + H+ → H2O + Fe3+ + •OH (Suzuki et al., 2006; Korripally et al., 2013). The resulting hydroxyl radical (·OH) is highly active and non-enzymatically deconstructs the lignocellulose structure (Cragg et al., 2015). During intrusion, parasitic members of the Orobanchaceae family need to pass through lignified endodermal cell layers (Yoshida and Shirasu, 2009), and intrusive cells in some species, such as Striga spp., can penetrate into lignified host xylem vessels (Dorr, 1997). It is tempting to hypothesize that parasitic plants may employ the Fenton reaction to depolymerize host lignin during invasion, and quinone-type HIFs may act as driving forces of the reaction. Understanding the physiological roles of HIF molecules beyond prehaustorium induction will help us to know why parasitic plants use these molecules as host signals.
Conclusions
Evidences collected on hemi- and holoparasites indicate a commonality and specificity of HIFs in parasitic members of the Orobanchaceae family. In addition to cytokinins, ROS-producing quinones or phenolics are commonly recognized as HIFs. For a long time, redox cycling has been suggested to manipulate prehaustorium induction, but the detailed mechanisms of this activity are yet to be discovered. Future studies to understand the common and specific signaling pathways from different types of HIFs should reveal how parasitic plants sense the presence of a host and begin their parasitic lifestyles.
Contribution to the Field Statement
Parasitic plants in the Orobanchaceae family are among world’s most devastating weed pests, yet the molecular processes underlying their parasitism have not been well understood. Parasitic plants develop haustoria, specialized invasive organs, upon recognition of host-derived chemical signals, the so-called haustorium-inducing factors (HIFs). This review summarizes past work and recent advances in the research of HIFs for parasitic plants in the Orobanchaceae family. Recent research efforts have begun to reveal the origins, species specificity, and action mechanisms of HIFs.
Author Contributions
VG, SW, and SY conceived the paper and drafted the manuscript. TW and SC drew the figures. SC, TW, KS, GM, PS, and SY edited and finalized the manuscript.
Funding
This work is partly supported by a PhD fellowship from the French Ministry of Education and Research to VG, and MEXT KAKENHI grants No. 18H02464 and 18H04838 to SY, 15H05959 to KS, and 19K16169 to SC. This study was also partly supported by the International Atomic Energy Agency Research Contract Number 20645.
Conflict of Interest Statement
The authors declare that the research was conducted in the absence of any commercial or financial relationships that could be construed as a potential conflict of interest.
References
Albrecht, H., Yoder, J. I., Phillips, D. A. (1999). Flavonoids promote haustoria formation in the root parasite Triphysaria versicolor. Plant Physiol. 119, 585–592. doi: 10.1104/pp.119.2.585
Atsatt, P. R., Hearn, T. F., Nelson, R. L., Heinman, R. T. (1978). Chemical induction and repression of haustoria in Orthocarpus pwpurascens (Scrophulariaceae). Ann. Bot. 42, 1177–1184. doi: 10.1093/oxfordjournals.aob.a085559
Baird, W. M. V., Riopel, J. M. (1985). Surface characteristics of root and haustorial hairs of parasitic Scrophlariaceae. Bot. Gaz. 146, 63–69. doi: 10.1086/337501
Bandaranayake, P. C. G., Filappova, T., Tomilov, A., Tomilova, N. B., Jamison-McClung, D., Ngo, Q., et al. (2010). A single-electron reducing quinone oxidoreductase is necessary to induce haustorium development in the root parasitic plant Triphysaria. Plant Cell 22, 1404–1419. doi: 10.1105/tpc.110.074831
Bandaranayake, P. C. G., Tomilov, A., Tomilova, N. B., Ngo, Q., Wickett, N., Pamphilis, C. W., et al. (2012). The TvPirin gene is necessary for haustorium development in the parasitic plant Triphysaria versicolor. Plant Physiol. 158, 1046–1053. doi: 10.1104/pp.111.186858
Bhuiyan, N. H., Selvaraj, G., Wei, Y., King, J. (2009). Gene expression profiling and silencing reveal that monolignol biosynthesis plays a critical role in penetration defence in wheat against powdery mildew invasion. J. Exp. Bot. 60, 509–521. doi: 10.1093/jxb/ern290
Brun, G., Braem, L., Thoiron, S., Gevaert, K., Goormachtig, S., Delavault, P. (2018). Seed germination in parasitic plants: what insights can we expect from strigolactone research? J. Exp. Bot. 69, 2265–2280. doi: 10.1093/jxb/erx472
Brun, G., Thoiron, S., Braem, L., Pouvreau, J., Monatiel, G., Lechat, M., et al. (2019). CYP707As are effectors of karrikin and strigolactone signaling pathways in Arabidopsis thaliana and parasitic plants. Plant. Cell Environ. 10, pce.13594. doi: 10.1111/pce.13594
Chang, M., Lynn, D. G. (1986). The haustorium and the chemistry of host recognition in parasitic angiosperms. J. Chem. Ecol. 12, 561–579. doi: 10.1007/BF01020572
Cragg, S. M., Beckham, G. T., Bruce, N. C., Bugg, T. D. H., Distel, D. L., Dupree, P., et al. (2015). Lignocellulose degradation mechanisms across the tree of life. Curr. Opin. Chem. Biol. 29, 108–119. doi: 10.1016/j.cbpa.2015.10.018
Cui, S., Wada, S., Tobimatsu, Y., Takeda, Y., Saucet, S. B., Takano, T., et al. (2018). Host lignin composition affects haustorium induction in the parasitic plants Phtheirospermum japonicum and Striga hermonthica. New Phytol. 218, 710–723. doi: 10.1111/nph.15033
Cui, S., Wakatake, T., Hashimoto, K., Saucet, S., Toyooka, K., Yoshida, S., et al. (2016). Haustorial hairs are specialized root hairs that support parasitism in the facultative parasitic plant, Phtheirospermum japonicum. Plant Physiol. 170, pp.01786.2015. doi: 10.1104/pp.15.01786
Davey, J. E., van Staden, J. (1976). Cytokinin translocation: changes in zeatin and zeatin-riboside levels in the root exudate of tomato plants during their development. Planta 130, 69–72. doi: 10.1007/BF00390846
Dorr, I. (1997). How Striga parasitizes its host: a TEM and SEM study. Ann. Bot 79, 463–472. doi: 10.1006/anbo.1996.0385
Estabrook, E. M., Yoder, J. I., (1998). Update on plant-plant communication plant-plant communications: rhizosphere signaling between parasitic angiosperms and their hosts. Plant Physiol. 116 (1), 1–7. doi: 10.1104/pp.116.1.1
Evidente, A., Sparapano, L., Fierro, O., Bruno, G., Giordano, F., Motta, A. (1998). Sphaeropsidone and episphaeropsidone, phytotoxic dimedone methyl ethers produced by Sphaeropsis sapinea f. sp. cupressi grown in liquid culture. Phytochemistry 48, 1139–1143. doi: 10.1016/S0031-9422(97)00897-2
Fernández-Aparicio, M., Reboud, X., Gibot-Leclerc, S. (2016a). Broomrape weeds. Underground mechanisms of parasitism and associated strategies for their control: a review. Front. Plant Sci. 7, 135. doi: 10.3389/fpls.2016.00135
Fernández-Aparicio, M., Masi, M., Maddau, L., Cimmino, A., Evidente, M., Rubiales, D., et al. (2016b). Induction of haustorium development by sphaeropsidones in radicles of the parasitic weeds Striga and Orobanche. A structure-activity relationship study. J. Agric. Food Chem. 64, 5188–5196. doi: 10.1021/acs.jafc.6b01910
Frick, E., Frahne, D., Wegmann, K. (1996). Biochemical synthesis of 2,6-dimethoxy-para-benzoquinone—a haustorial stimulant of Striga asiatica (L.) Kuntze. Nat. Prod. Lett. 9, 153–159. doi: 10.1080/10575639608044939
Goldwasser, Y., Hershenhorn, J., Plakhine, D., Kleifeld, Y., Rubin, B. (1999). Biochemical factors involved in vetch resistance to Orobanche aegyptiaca. Physiol. Mol. Plant Pathol. 54, 87–96. doi: 10.1006/pmpp.1998.0191
Goyet, V., Billard, E., Pouvreau, J.-B., Lechat, M.-M., Pelletier, S., Bahut, M., et al. (2017). Haustorium initiation in the obligate parasitic plant Phelipanche ramosa involves a host-exudated cytokinin signal. J. Exp. Bot. 68, 5539–5552. doi: 10.1093/jxb/erx359
Harris, P. J., Trethewey, J. A. K. (2010). The distribution of ester-linked ferulic acid in the cell walls of angiosperms. Phytochem. Rev. 9, 19–33. doi: 10.1007/s11101-009-9146-4
Ibrahim, V., Volkova, N., Pyo, S. H., Mamo, G., Hatti-Kaul, R. (2013). Laccase catalysed modification of lignin subunits and coupling to p-aminobenzoic acid. J. Mol. Catal. B Enzym. 97, 45–53. doi: 10.1016/j.molcatb.2013.07.014
Ishida, J. K., Wakatake, T., Yoshida, S., Takebayashi, Y., Kasahara, H., Wafula, E., et al. (2016). Local auxin biosynthesis mediated by a YUCCA flavin monooxygenase regulates haustorium development in the parasitic plant Phtheirospermum japonicum. Plant Cell 28, 1795–1814. doi: 10.1105/tpc.16.00310
Ishida, J. K., Yoshida, S., Ito, M., Namba, S., Shirasu, K. (2011). Agrobacterium rhizogenes-mediated transformation of the parasitic plant Phtheirospermum japonicum. PLoS One 6, e25802. doi: 10.1371/journal.pone.0025802
Ishida, J. K., Yoshida, S., Shirasu, K. (2017). Quinone oxidoreductase 2 is involved in haustorium development of the parasitic plant Phtheirospermum japonicum. Plant Signal. Behav. 12, 1–4. doi: 10.1080/15592324.2017.1319029
Joel, D. M. (2013). “Functional structure of the mature haustorium,” in Parasitic Orobanchaceae. Eds. Joel, D. M., Gressel, J., Musselman, L. J. (Springer), Berlin, Heidelberg 25–56. doi: 10.1007/978-3-642-38146-1_3
Joel, D. M., Losner-Goshen, D. (1994). The attachment organ of the parasitic angiosperms Orobanche cumana and O. aegyptiaca and its development. Can. J. Bot. 72, 564–574. doi: 10.1139/b94-075
Keyes, W. J., O’Malley, R. C., Kim, D., Lynn, D. G. (2000). Signaling organogenesis in parasitic angiosperms: xenognosin generation, perception, and response. J. Plant Growth Regul. 19, 217–231. doi: 10.1007/s003440000024
Khanam, S., Bauters, L., Singh, R. R., Verbeek, R., Haeck, A., Sultan, S. M. D., et al. (2018). Mechanisms of resistance in the rice cultivar Manikpukha to the rice stem nematode Ditylenchus angustus. Mol. Plant Pathol. 19, 1391–1402. doi: 10.1111/mpp.12622
Kim, D., Kocz, R., Boone, L., Keyes, W. J., Lynn, D. G. (1998). On becoming a parasite: evaluating the role of wall oxidases in parasitic plant development. Chem. Biol. 5, 103–117. doi: 10.1016/S1074-5521(98)90144-2
Kirwa, H. K., Murungi, L. K., Beck, J. J., Torto, B. (2018). Elicitation of differential responses in the root-knot nematode Meloidogyne incognita to tomato root exudate cytokinin, flavonoids, and alkaloids. J. Agric. Food Chem. 66, 11291–11300. doi: 10.1021/acs.jafc.8b05101
Korripally, P., Timokhin, V. I., Houtman, C. J., Mozuch, M. D., Hammel, K. E. (2013). Evidence from Serpula lacrymans that 2,5-dimethoxyhydroquinone is a lignocellulolytic agent of divergent brown rot basidiomycetes. Appl. Environ. Microbiol. 79, 2377–2383. doi: 10.1128/AEM.03880-12
Lechat, M. M., Brun, G., Montiel, G., Véronési, C., Simier, P., Thoiron, S., et al. (2015). Seed response to strigolactone is controlled by abscisic acid-independent DNA methylation in the obligate root parasitic plant, Phelipanche ramosa L. Pomel. J. Exp. Bot. 66, 3129–3140. doi: 10.1093/jxb/erv119
Lynn, D. G. (1985). The involvement of allelochemicals in the host selection of parasitic angiosperms. Acs Symp. Ser. 268, 55–81. doi: 10.1021/bk-1985-0268.ch005
Lynn, D. G., Chang, M. (1990). Phenolic signals in cohabitation: implications for plant development. Annual Rev. Plant Phys. Plant Mol. Biol. 41, 497–526. doi: 10.1146/annurev.pp.41.060190.002433
Lynn, D. G., Steffens, J. C., Kamut, V. S., Graden, D. W., Shabanowitz, J., Riopel, J. L. (1981). Isolation and characterization of the 1st host recognition substance for parasitic angiosperms. J. Am. Chem. Soc. 103, 1868–1870. doi: 10.1021/ja00397a062
Matvienko, M., Wojtowicz, A., Wrobel, R., Jamison, D., Goldwasser, Y., Yoder, J I. (2001). Quinone oxidoreductase message levels are differentially regulated in parasitic and non-parasitic plants exposed to allelopathic quinones. Plant J. 25, 375–387. doi: 10.1046/j.1365-313x.2001.00971.x
Miedes, E., Vanholme, R., Boerjan, W., Molina, A. (2014). The role of the secondary cell wall in plant resistance to pathogens. Front. Plant Sci. 5, 1–13. doi: 10.3389/fpls.2014.00358
Mutuku, J. M., Cui, S., Hori, C., Takeda, Y., Tobimatsu, Y., Nakabayashi, R., et al. (2019). The structural integrity of lignin is crucial for resistance against Striga hermonthica parasitism in rice. Plant Physiol. 179, pp.01133.2018. doi: 10.1104/pp.18.01133
Olivier, A., Benhamou, N., Leroux, G. D. (1991). Cell surface interactions between sorghum roots and the parasitic weed Striga hermonthica : cytochemical aspects of cellulose distribution in resistant and susceptible host tissues. Can. J. Bot. 69, 1679–1690. doi: 10.1139/b91-213
Pacifici, E., Polverari, L., Sabatini, S. (2015). Plant hormone cross-talk: the pivot of root growth. J. Exp. Bot. 66, 1113–1121. doi: 10.1093/jxb/eru534
Pollegioni, L., Tonin, F., Rosini, E. (2015). Lignin-degrading enzymes. FEBS J. 282, 1190–1213. doi: 10.1111/febs.13224
Riopel, J. L., Musselman, L. J. (1979). Experimental initiation of haustoria in Agalinis purpurea (Scrophulariaceae). Am. J. Bot. 66, 570–575. doi: 10.1002/j.1537-2197.1979.tb06259.x
Salcedo-Morales, G., Jiménez-Aparicio, A. R., Cruz-Sosa, F., Trejo-Tapia, G. (2013). Anatomical and histochemical characterization of in vitro haustorium from roots of Castilleja tenuiflora. Biol. Plant. 58, 164–168. doi: 10.1007/s10535-013-0369-2
Smith, C. E., Ruttledge, T., Zeng, Z., O’Malley, R. C., Lynn, D. G. (1996). A mechanism for inducing plant development: the genesis of a specific inhibitor. Proc. Natl. Acad. Sci. U.S.A. 93, 6986–6991. doi: 10.1073/pnas.93.14.6986
Soejima, H., Sugiyama, T., Ishihara, K. (1992). Changes in cytokinin activities and mass spectrometric analysis of cytokinins in root exudates of rice plant (Oryza sativa L.) 1. Plant Physiol. 100, 1724–1729. doi: 10.1104/pp.100.4.1724
Spallek, T., Melnyk, C. W., Wakatake, T., Zhang, J., Sakamoto, Y., Kiba, T., et al. (2017). Interspecies hormonal control of host root morphology by parasitic plants. Proc. Natl. Acad. Sci. U.S.A. 114, 5283–5288. doi: 10.1073/pnas.1619078114
Spallek, T., Mutuku, M., Shirasu, K. (2013). The genus Striga: a witch profile. Mol. Plant Pathol. 14, 861–869. doi: 10.1111/mpp.12058
Spíchal, L., Werner, T., Popa, I., Riefler, M., Schmülling, T., Strnad, M. (2009). The purine derivative PI-55 blocks cytokinin action via receptor inhibition. FEBS J. 276, 244–253. doi: 10.1111/j.1742-4658.2008.06777.x
Steffens, J. C., Lynn, D. G., Kamut, V. S., Riopel, J. L. (1982). Molecular specificity of haustorial induction in Agalinis purpurea (L.) Raf. (Scrophulariaceae). Ann. Bot. 50, 1–7. doi: 10.1093/oxfordjournals.aob.a086332
Steffens, J. C., Lynn, D. G., Riopel, J. L. (1986). An haustoria inducer for the root parasite Agalinis purpurea. Phytochemistry 25, 2291–2298. doi: 10.1016/S0031-9422(00)81682-9
Steffens, J. C., Roark, J. L., Lynn, D. G., Riopel, J. L. (1983). Host recognition in parasitic angiosperms: use of correlation spectroscopy to identify long-range coupling in an haustorial inducer. J. Am. Chem. Soc. 105, 1669–1671. doi: 10.1021/ja00344a052
Stephens, E. L. (1912). The structure and development of the haustorium of Striga lutea. Ann. Bot. 26, 1067–1076. doi: 10.1093/oxfordjournals.aob.a089431
Suzuki, M. R., Hunt, C. G., Houtman, C. J., Dalebroux, Z. D., Hammel, K. E. (2006). Fungal hydroquinones contribute to brown rot of wood. Environ. Microbiol. 8, 2214–2223. doi: 10.1111/j.1462-2920.2006.01160.x
Taran, O., Patel, V., Lynn, D. G. (2019). Small molecule reaction networks that model the ROS dynamics of the rhizosphere. Chem. Commun. (Camb.) 55, 3602–3605. doi: 10.1039/C8CC08940J
Tomilov, A., Tomilova, N., Yoder, J. I. (2004). In vitro haustorium development in roots and root cultures of the hemiparasitic plant Triphysaria versicolor. Plant Cell Tissue Organ Cult. 77, 257–265. doi: 10.1023/B:TICU.0000018392.62980.41
van Staden, J., Dimalla, G. G. (1976). Cytokinins from soils. Planta 130, 85–87. doi: 10.1007/BF00390849
Vanholme, R., Demedts, B., Morreel, K., Ralph, J., Boerjan, W. (2010). Lignin biosynthesis and structure. Plant Physiol. 153, 895–905. doi: 10.1104/pp.110.155119
Wada, S., Cui, S., Yoshida, S. (2019). Reactive oxygen species (ROS) generation is indispensable for haustorium formation of the root parasitic plant Striga hermonthica. Front. Plant Sci. 10, 1–12. doi: 10.3389/fpls.2019.00328
Wakatake, T., Yoshida, S., Shirasu, K., (2018). Induced cell fate transitions at multiple cell layers configure haustorium development in parasitic plants. Dev. 145, dev164848. doi: 10.1242/dev.164848
Westwood, J. H., Yoder, J. I., Timko, M. P., dePamphilis, C. W. (2010). The evolution of parasitism in plants. Trends Plant Sci. 15, 227–235. doi: 10.1016/j.tplants.2010.01.004
Wrobel, R. L., Yoder, J. I. (2001). Differential RNA expression of alpha-expansin gene family members in the parasitic angiosperm Triphysaria versicolor (Scrophulariaceae). Gene 266, 85–93. doi: 10.1016/S0378-1119(01)00376-6
Xiang, L., Li, Y., Sui, X., Li, A. (2018). Fast and abundant in vitro spontaneous haustorium formation in root hemiparasitic plant Pedicularis kansuensis Maxim. (Orobanchaceae). Plant Divers. 40, 226–231. doi: 10.1016/j.pld.2018.07.005
Yang, J., Zhang, J., Wang, Z., Zhu, Q., Liu, L. (2002). Abscisic acid and cytokinins in the root exudates and leaves and their relationship to senescence and remobilization of carbon reserves in rice subjected to water stress during grain filling. Planta 215, 645–652. doi: 10.1007/s00425-002-0789-2
Yoneyama, K. K., Awad, A. A., Xie, X., Takeuchi, Y., Yoneyama, K. K., Takeuchi, Y. (2010). Strigolactones as germination stimulants for root parasitic plants. Plant Cell Physiol. 51, 1095–1103. doi: 10.1093/pcp/pcq055
Yoshida, S., Cui, S., Ichihashi, Y., Shirasu, K. (2016). The haustorium, aspecialized invasive organ in parasitic plants. Annu. Rev. Plant Biol. 67, 643–667. doi: 10.1146/annurev-arplant-043015-111702
Yoshida, S., Shirasu, K. (2009). Multiple layers of incompatibility to parasitic witch weed, Striga hermonthica. New Phytol. 183, 180–189. doi: 10.1111/j.1469-8137.2009.02840.x
Keywords: haustorium, haustorium-inducing factor, Orobanchaceae, parasitic plants, Striga, quinone, cytokinin, lignin
Citation: Goyet V, Wada S, Cui S, Wakatake T, Shirasu K, Montiel G, Simier P and Yoshida S (2019) Haustorium Inducing Factors for Parasitic Orobanchaceae. Front. Plant Sci. 10:1056. doi: 10.3389/fpls.2019.01056
Received: 20 May 2019; Accepted: 30 July 2019;
Published: 29 August 2019.
Edited by:
Masa H. Sato, Kyoto Prefectural University, JapanReviewed by:
Koh Aoki, Osaka Prefecture University, JapanLiliana M. Cano, University of Florida, United States
Copyright © 2019 Goyet, Wada, Cui, Wakatake, Shirasu, Montiel, Simier and Yoshida. This is an open-access article distributed under the terms of the Creative Commons Attribution License (CC BY). The use, distribution or reproduction in other forums is permitted, provided the original author(s) and the copyright owner(s) are credited and that the original publication in this journal is cited, in accordance with accepted academic practice. No use, distribution or reproduction is permitted which does not comply with these terms.
*Correspondence: Satoko Yoshida, U2F0b2tveUBicy5uYWlzdC5qcA==
†These authors have contributed equally to this work.