- 1UMR Ecologie Microbienne, CNRS, INRA, VetAgro Sup, UCBL, Université de Lyon, Lyon, France
- 2Biocapteurs-Analyses-Environment, Universite de Perpignan Via Domitia, Perpignan, France
- 3Laboratoire de Biodiversite et Biotechnologies Microbiennes, USR 3579 Sorbonne Universites (UPMC) Paris 6 et CNRS Observatoire Oceanologique, Paris, France
The study of pathogenic agents in their natural niches allows for a better understanding of disease persistence and dissemination. Bacteria belonging to the Agrobacterium genus are soil-borne and can colonize the rhizosphere. These bacteria are also well known as phytopathogens as they can cause tumors (crown gall disease) by transferring a DNA region (T-DNA) into a wide range of plants. Most reviews on Agrobacterium are focused on virulence determinants, T-DNA integration, bacterial and plant factors influencing the efficiency of genetic transformation. Recent research papers have focused on the plant tumor environment on the one hand, and genetic traits potentially involved in bacterium-plant interactions on the other hand. The present review gathers current knowledge about the special conditions encountered in the tumor environment along with the Agrobacterium genetic determinants putatively involved in bacterial persistence inside a tumor. By integrating recent metabolomic and transcriptomic studies, we describe how tumors develop and how Agrobacterium can maintain itself in this nutrient-rich but stressful and competitive environment.
Introduction
The persistence of bacterial phytopathogens results from many factors including survival in natural habitats such as the soil, the rhizosphere, or the phyllosphere (van der Wolf and Boer, 2015), acclimation capacities to different lifestyles, and the ability to efficiently shift from one lifestyle to another (Sokurenko et al., 2006; Engering et al., 2013; Duprey et al., 2014; Wei et al., 2015). Survival in the rhizosphere implies abilities to resist both abiotic and biotic stress, and a high capacity to rapidly access nutritional sources (Ji and Wilson, 2002; Romanuk et al., 2009; Wei et al., 2015; Leonard et al., 2017). The pathogenic lifestyle generally involves bacterial growth inside the host and tight interactions with it. The success of the pathogen thus depends on its ability to face the conditions encountered inside the host, including plant defense mechanisms, availability of nutrient resources, and interactions with the host microbiota (Brader et al., 2017; Spanu and Panstruga, 2017; van der Does and Rep, 2017).
Members of the Agrobacterium genus are soil-borne bacteria able to live in the plant rhizosphere; they can be pathogenic when they harbor the Ti (Tumor-inducing) plasmid. This plasmid contains a DNA region (T-DNA) that can be transferred to plant cells and integrated into the plant genome. Briefly, the T-DNA genes are expressed by the infected plant, leading to hormone production which induces uncontrolled proliferation of plant cells (Drummond, 1979; Nester, 2014). Then the plant develops a tumor and is considered as suffering from crown gall disease. The T-DNA region also contains genes encoding opine biosynthesis. Opines are conjugates of amino acids and sugars or organic acids, and are specifically used as nutrients by agrobacteria harboring the Ti plasmid. Around 40 different types of opines have been characterized; some of them trigger the transfer of the Ti plasmid from one bacterium to another, enhancing pathogenicity and contributing to the persistence of pathogenic bacteria in the environment (Dessaux et al., 1998; Flores-Mireles et al., 2012). These properties confer opines a central role in the Agrobacterium-infected plant interaction (Guyon et al., 1980; Dessaux and Faure, 2018). According to the “opine concept,” the presence of opines improves the competitiveness of the bacteria able to catabolize them at the expense of the bacteria unable to do so. However, the ability to catabolize opines may not be sufficient to be competitive under detrimental environmental conditions. Tumor exploitation also implies dealing with plant defense mechanisms. Plant defense relies on signaling by chemical compounds such as salicylic acid (SA), jasmonic acid (JA), or ethylene that allow for the induction of pathogenesis-related proteins, some of which exhibit antimicrobial activities (Costet et al., 1999; Durrant and Dong, 2004). Even though plants initiate defense mechanisms against Agrobacterium (Deeken et al., 2006; Pitzschke, 2013; Shih et al., 2018), the bacterium is known to bypass those defenses to durably settle in tumors (Lee et al., 2009; Someya et al., 2013; Nonaka and Ezura, 2014; Nonaka et al., 2017). It should be emphasized that Agrobacterium is one of the only bacterial phytopathogens that exploits its host for niche construction instead of killing it (Leonard et al., 2017).
Non-pathogenic Agrobacterium strains have also been isolated from tumors (Bélanger et al., 1995; Llop et al., 2009; Shams et al., 2012). Some strains could be mutants of the initial tumor-inducing strain (e.g., following spontaneous deletions inside pTi), but most of these non-pathogenic isolates may have an environmental origin. Tumors have also been reported to host other microorganisms (Faist et al., 2016). Hence, even in an opine-rich tumor environment and beyond its ability to use opine as a nutrient, Agrobacterium needs additional determinants to compete with other microorganisms and durably settle.
The induction of the pathogenicity program, as well as the process of genetic transformation leading to tumor formation, has been extensively studied (Lacroix and Citovsky, 2013; Nester, 2014; Gelvin, 2017). The natural ability of Agrobacterium to genetically transform plants and its use as a tool for genetic engineering are also well documented (Hwang et al., 2015; Krenek et al., 2015). Moreover, the transition between the rhizospheric and tumor lifestyles, which represents a critical step in the establishment of crown gall disease, has recently been reviewed (Barton et al., 2018). As Agrobacterium pathogenicity and perennial soil contamination are directly linked to its success in maintaining a long-term association with its host plant, we chose to focus this review on the bacterial ability to durably exploit the specific tumor environment. We therefore summarized knowledge about Agrobacterium settlement and the subsequent changes triggered in the host plant, and focused on the molecular determinants that allow Agrobacterium to survive in tumors, exploit this environment, and compete with other microorganisms encountered in tumors.
Tumor Development Causes Stress in Bacterial and Plant Cells
Once the T-DNA is integrated, tumor development is initiated and the physico-chemical parameters of the tumor evolve with time and with the host plant biotic and abiotic environment (Hwang et al., 2015). We focused on the specific conditions encountered in tumors and on the bacterial genes that are likely relevant for thriving in that special environment and modifying it.
Role of Hormones
The role of jasmonic acid (JA) in tumor development is not formally established and seems to differ according to the plant species. No increase in JA production was observed during tumor development in Arabidopsis thaliana (Lee et al., 2009), whereas transient JA accumulation occurred in 1-week-old Ricinus communis tumors (Veselov et al., 2003). By contrast, the role of auxin, cytokinin, and ethylene in tumor proliferation and development has been established (for a review see Gohlke and Deeken, 2014). Native plant cells contain genes responsible for the synthesis of these types of hormones, but transformed cells contain additional T-DNA-encoded genes for auxin and cytokinin biosynthesis, i.e., iaaH and iaaM for auxin, and ipt for cytokinin (Skoog and Miller, 1957; Zhu et al., 2000). Tumors contain higher levels of auxin and cytokinin than non-infected plant stems; concentrations vary according to the plant and the age of the tumor (Veselov et al., 2003; Lee et al., 2009; Gohlke and Deeken, 2014). Agrobacterium cells can also produce hormones. Indeed, two genes have been proposed to be involved in cytokinin production of Agrobacterium: miaA (atu2039) located on the C58 circular chromosome (Gray et al., 1996) and tzs (atu6164) located on several pTi including the nopaline C58-pTi (Hwang et al., 2010). Both genes are expressed in C58-induced tumors (González-Mula et al., 2018), suggesting that bacteria produce cytokinin in that environment. Agrobacterium can synthesize another hormone, the indole-3-acetic acid (IAA of the auxin family). Although the genetic determinants of IAA synthesis remain unknown, this property is not T-DNA encoded, as the non-oncogenic strain GV3101 can also produce IAA (Lee et al., 2009).
High cytokinin and auxin levels enhance the activity of 1-aminocyclopropane-1-carboxylate (ACC) synthase, the key enzyme of ethylene synthesis in plants (Adams and Yang, 1979). Ethylene has been proposed to be involved in the high amount of vascularized tissues in tumors at the detriment of aerial part, leading to the water-flow priority to tumor cells over the host shoot (i.e., “Gall-constriction hypothesis”; Aloni, 1995; Aloni et al., 1998). Indeed, the comparison of tumors induced by A. tumefaciens strain C58 in wild-type tomato and in never-ripe mutants (sensitive and insensitive to ethylene, respectively) revealed that the mutant plants had smaller tumors and more vascularized tissues than the wild-type plants (Aloni et al., 1998). Thus, ethylene plays a central role in tumor water supply (Figure 1A). Agrobacterium growth is not affected by ethylene (strain C58; Nonaka and Ezura, 2014) and Agrobacterium cannot directly affect the ethylene content. While some plant-associated bacteria (either phytobeneficial or phytopathogenic) can degrade the immediate precursor of ethylene, ACC, Agrobacterium fails to do so, because it lacks the gene encoding the ACC-deaminase enzyme (Someya et al., 2013; Bruto et al., 2014; Nonaka and Ezura, 2014).
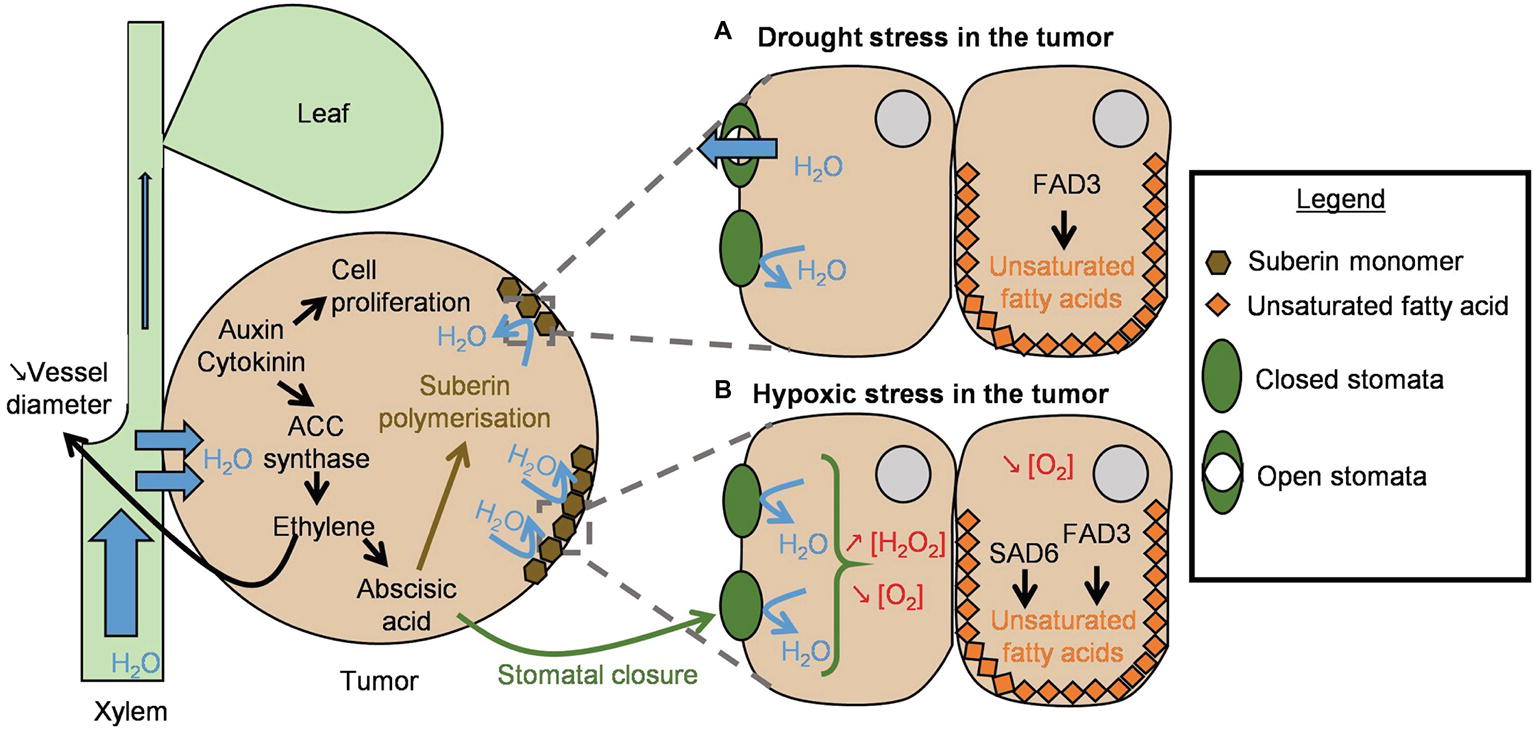
Figure 1. Schematic representation of the plant reactions to the stresses caused by tumor development. T-DNA integration into the plant genome induces auxin and cytokinin production. High concentrations of these two phytohormones accelerate cell proliferation and tumor growth. ACC synthase expression is also induced and triggers ethylene production. Ethylene has two main roles in the tumor: it reduces plant vessel diameter around the tumor to ensure its hydration, and it triggers abscisic acid synthesis. The latter induces the polymerization of suberin that forms a protective layer around the tumor. (A) Drought stress in the tumor: under drought stress, FAD3 produces α-linolenic acid, an unsaturated fatty acid, to maintain lipid membrane integrity. (B) Hypoxic stress in the tumor: when the drought stress is more severe (i.e., in old tumors), abscisic acid triggers stomatal closure. This implies H2O2 production and leads to a decreased oxygen rate. Under low oxygen, SAD6 also contributes to the production of unsaturated fatty acids to maintain lipid membrane integrity in the transformed cells.
SA take part in plant defense mechanisms called systemic acquired resistance (SAR) and local acquired resistance (LAR) by triggering the synthesis of pathogenesis-related proteins (Costet et al., 1999; Durrant and Dong, 2004). However, transcriptomic analyses have demonstrated that genes involved in plant defense were under-expressed in the early stages of infection by Agrobacterium (Veena et al., 2003; Lee et al., 2009). In 5-week-old A. thaliana tumors, the activation of the SA-dependent defense signaling pathway was not triggered despite an increase in SA levels. This observation was attributed to the high level of auxin in these tumors (Lee et al., 2009). SA can inhibit bacterial growth when added to the culture medium (Yuan et al., 2007; Anand et al., 2008). The SA inhibitory concentration could vary according to bacterial growth conditions: 5 μM in a minimal culture medium (Yuan et al., 2007) as compared to 200 μM in an enriched one (Anand et al., 2008). As for IAA, its effect is dose-dependent: it promotes bacterial growth at low concentrations (0.8 μM) but shows deleterious effects at high concentrations (200 μM) (Liu and Nester, 2006; Gohlke and Deeken, 2014). Hence, Agrobacterium could first benefit from the presence of IAA, but could be locally sensitive to high levels of this compound likely to impede its development as the tumor grows (Gohlke and Deeken, 2014). Thus, in the tumor tissue, ethylene circumvents its classical role in defense response and highly participates in tumor development together with cytokinin and auxin.
Drought
The water advantage conferred by ethylene through its influence on xylem vessel diameter is further counterbalanced by an increased water loss: the massive proliferation of tumor cells causes epidermal rupture and uncontrolled evaporation of the water contained in the tumor (Figure 1; Schurr et al., 1996). In this case, ethylene also plays a crucial role in tumor drought tolerance, as proposed earlier (Efetova et al., 2007) and as summarized in Figure 1A. Cytokinins and auxins trigger ethylene synthesis that in turn induces abscisic acid (ABA) synthesis in the tumor. ABA induces suberin polymerization to create a protective layer around the tumor and avoid its desiccation (Veselov et al., 2003; Efetova et al., 2007; Gohlke and Deeken, 2014). In response to the drought stress mediated by ABA signaling, enhancement of fatty acid desaturase activity is also observed in tumor cells, to synthesize α-linolenic acid and ensure lipid membrane integrity (Klinkenberg et al., 2014). Fatty acid desaturase 3 (FAD3) plays an important role in tumors: infection of A. thaliana FAD3 mutants by Agrobacterium strain C58 at low humidity rates revealed that fad3-2 mutant tumors were smaller than wild-type ones. This demonstrates that FAD3-synthesized α-linolenic acid can maintain the lipid membrane integrity of plant cells under drought conditions (Figure 1; Klinkenberg et al., 2014).
In order to maintain homeostasis, bacteria use two main strategies, i.e., accumulation of osmoprotectants and production of exopolysaccharides (EPSs) (Weiner et al., 1995; Dandapath et al., 2017). Osmoprotectants are compounds that accumulate into the cell to protect it from different stresses such as drought, dehydration, or the presence of oxygen radicals. Agrobacterium can take up or synthesize a large range of compounds previously described as being involved in osmoprotection (Kempf and Bremer, 1998; Panikulangara et al., 2004; Bougouffa et al., 2014). These compounds include glycine betaine and choline (Boncompagni et al., 1999), gamma-butyrobetaine (Nobile and Deshusses, 1986), proline (Haudecoeur et al., 2009b), sucrose and trehalose (Ampomah et al., 2013), palatinose (De Costa et al., 2003), melibiose, raffinose, and stachyose (Meyer et al., 2018b). Bacterial genes allowing for melibiose, raffinose, and stachyose uptake are expressed in mature C58-induced tumors (González-Mula et al., 2018) where they could allow for bacterial osmoprotection. However, the role of these compounds in bacterial osmoprotection has not been assessed yet. A direct role of gamma-butyrobetaine, glycine betaine, and choline in Agrobacterium osmoprotection has been described only in vitro (notably for C58), but so far no study has investigated their role in planta (Nobile and Deshusses, 1986; Boncompagni et al., 1999).
Biosynthesis of EPS is the second way of resisting high or low environmental osmolarities. Agrobacterium can synthesize diverse kinds of EPS: β-1-2-glucan (atu2728–atu2730) (Cangelosi et al., 1990), curdlan (atu3355–atu3357) (Stasinopoulos et al., 1999; McIntosh et al., 2005; Lassalle et al., 2011; Ruffing and Chen, 2012), succinoglycan (atu4049–atu4060; atu4166; atu3325–atu3327) (Tomlinson et al., 2010), unipolar polysaccharide (atu1235–atu1240; atu0102), cellulose (atu3303–atu3309) (Matthysse, 2018). Among the EPS, only curdlan is specific to the “A. fabrum” species (Lassalle et al., 2011, 2017). A role in osmoprotection has only been described for β-1-2-glucan, which is produced in response to low-osmolarity conditions in different microorganisms including Agrobacterium (Cangelosi et al., 1990; Ingram-Smith and Miller, 1998; Domínguez-Ferreras et al., 2006). Some Agrobacterium genes involved in EPS (curdlan) production are expressed in C58-induced tumors (González-Mula et al., 2018) where they may serve as osmoprotectants. Alternatively, they could be useful for cell adhesion, as some of these EPSs are involved in that process (for a review see Matthysse, 2018). The precise role of EPS inside tumors thus remains to be deciphered.
Oxidative and Hypoxic Stress
One of the first plant responses to pathogen perception is the production of reactive oxygen species (ROS), like superoxide (O2−), or hydrogen peroxide (H2O2) (Torres et al., 2006; Lee et al., 2009). Agrobacterium strain C58 can detoxify these toxic compounds thanks to proteins encoded by chromosomal genes. Firstly, it harbors three genes encoding superoxide dismutase: sodBI (atu0876), sodBII (atu4583), and sodBIII (atu4726) (Saenkham et al., 2007). The functional analysis of these three enzymes revealed that SodBI is mainly responsible for transforming O2− into O2 and H2O2. The sodBI mutant and the triple superoxide dismutase mutant were less proficient than the wild-type in inducing vir genes and consequently displayed a reduced ability to induce tumor formation (Saenkham et al., 2007). Secondly, Agrobacterium can degrade H2O2 to H2O and O2 thanks to the KatA catalase (atu4642 carried by strain C58 linear chromid – i.e., linear chromosome) (Xu and Pan, 2000). A C58-derivative katA mutant was highly affected in its capacity to form tumors. It has been suggested that Agrobacterium catalase expression prevents the infected plant from activating the hypersensitive response (HR), allowing for the bacterium to settle and to form tumors without risking plant tissue necrosis (Xu and Pan, 2000; Gohlke and Deeken, 2014). katA expression is induced by OxyR upon H2O2 perception (Nakjarung et al., 2003). Conversely, in Agrobacterium strain C58, the global regulator LsrB was recently shown to negatively regulate katA transcription (Tang et al., 2018). The ability to cope with oxidative stress likely participates in the long-term efficient interaction. Five-week-old R. communis tumors were reported to contain hypertrophied non-functional stomata at the tumor surface that increased tumor water loss (Figure 1B; Schurr et al., 1996). The ABA drought stress pathway is involved in stomatal closure through its influence on H2O2 production (Zhang et al., 2001). Accordingly, although H2O2 accumulation was impeded by the bacteria at the beginning of the infection process (3 h and 6 days after inoculation), it accumulated later during tumor development (5 weeks after inoculation) (Lee et al., 2009). To determine if the differences in H2O2 levels are due to lower bacterial degradation and/or greater production by plants or by bacterial cells, it would be relevant to study the expression of the bacterial genes sodB, katA, and their regulator, and also H2O2 accumulation during tumor development.
The oxygen rate inside tumors decreases, and hypoxic stress has consequences on the plant metabolism, notably on the fatty acid metabolism. In these conditions, stearoyl ACP desaturase 6 (SAD6), which is synthesized only in a hypoxic environment, maintains plant cell membrane integrity by catalyzing the first step of fatty acid biosynthesis (Figure 1B; Klinkenberg et al., 2014; Kerpen et al., 2019). In accordance, in 5-week-old A. thaliana tumors, genes involved in photosynthesis were under-expressed, whereas genes involved in glycolysis and fermentation were over-expressed (Deeken et al., 2006). The authors suggested that transformed plant cells switch from an autotrophic metabolism to a heterotrophic one and that oxygen availability is limited, at least in some parts of the tumor. The low oxygen content of tumors has been recently confirmed in 14- and 28 day-old A. thaliana tumors induced by Agrobacterium strain B6 (Kerpen et al., 2019). Under low oxygen conditions, some Agrobacterium species are facultative denitrifiers (Baek and Shapleigh, 2005). A nitrate reductase (NapAB: Atu4408–Atu4409) allows them to reduce nitrate into nitrite (NO2−), which is further converted into nitric oxide (NO) by a nitrite reductase (NirK: Atu4382). Finally, an NO reductase (NorB: Atu4388) catalyzes the transformation of NO into nitrous oxide (N2O), which accumulates in the absence of an N2O reductase (encoded by the nosZ gene) (Kampschreur et al., 2012). The norB gene was expressed in a context of agroinfiltration in A. thaliana leaves, and a transcriptomic study comparing tumors and culture media suggests that partial denitrification occurs in tumors (Baek and Shapleigh, 2005; González-Mula et al., 2018). These results indicate that on the one hand, denitrification can participate in Agrobacterium survival in tumors by providing energy in the absence of oxygen, and on the other hand, NO degradation by Agrobacterium NO reductase can also participate in the bypassing of plant defenses mediated by NO (Delledonne et al., 1998; Leitner et al., 2009; Bellin et al., 2013).
Bacteria Find Trophic Resources and Toxic Compounds Inside Tumors
Tumor Metabolite Content
Nutrient availability is crucial for bacterial development inside tumors. A tumor is a highly vascularized metabolite sink supplied by water and minerals (mainly conducted via the xylem) and by sugars and other biosynthesized carbonated compounds (transported via the phloem) (Gohlke and Deeken, 2014). Apart from hormones, ABA and opines, whose presence in the tumor has been specifically analyzed at different times after inoculation, only a few studies have dealt with metabolite analyses (Deeken et al., 2006; Simoh et al., 2009; González-Mula et al., 2019). Moreover, these studies used different bacterial strains, plant models (A. thaliana, Brassica napa, Solanum lycopersicum), and analytical methods (targeted or global analyses based on NMR or GC-TOF-MS). Overall, as in non-infected tissues, the main primary metabolites in tumors were sugars, organic acids, and amino acids (Deeken et al., 2006; González-Mula et al., 2019). In addition to sucrose and glucose, and as compared to non-infected stems, 5-week-old A. thaliana tumors contained a high concentration of amino acids (glutamine, serine, asparagine, glutamic acid, threonine, proline, aspartic acid, alanine, valine, isoleucine, leucine, histidine, and arginine) (Deeken et al., 2006). Among sugars, organic acids and amino acids, and in contrast to A. thaliana tumors, only pyruvate and proline significantly accumulated in S. lycopersicum tumors (González-Mula et al., 2019). γ-hydroxybutyric acid (GHB) also slightly accumulated in S. lycopersicum tumors (González-Mula et al., 2019). Among nitrogen compounds, GABA and α-aminoadipinic acid were more abundant in infected tissues (Deeken et al., 2006; González-Mula et al., 2019). Secondary metabolites, some of which can limit bacterial growth (Del Valle et al., 2016; Wang et al., 2018) can also be detected in tumors: flavonoids such as quercetin and kaempferol, phenylpropanoids and its derivatives (ferulate, sinapoyl- and coumaroyl-malate, 3-caffeoylquinate), and glucosinolate or its derivatives are also accumulated in B. napa or S. lycopersicum tumors (Simoh et al., 2009; González-Mula et al., 2019).
Metabolites are not uniformly distributed across tumors, and both their amount and their localization vary during tumor development. For example, the hexose level was found higher in the center of 3-week-old R. communis tumors than in their periphery. Conversely, sucrose and proline were more abundant in the periphery of the tumor than in its center in 6-week-old tumors (Wächter et al., 2003). This makes sense since sucrose is more easily transportable and assimilable under drought conditions (Singh and Maclachlan, 1983; Wobus and Weber, 1999; Deeken et al., 2006). Those gradients are proposed to be linked mainly to tumor expansion and also to turgor pressure and osmoprotection. The relative abundance of metabolites also depends on the opine-type of the Agrobacterium strain (Simoh et al., 2009): B. napa tumors induced by strains harboring a “nopaline-type” or a “octopine-type” Ti plasmid differed in flavonoids, phenylpropanoid derivatives, sugar, organic and amino acid contents. However, no difference was reported for the precursors of nopaline and octopine (i.e, arginine and respectively α-ketoglutarate and pyruvate; Simoh et al., 2009). Additional metabolomic studies of tumors would provide further insights into the Agrobacterium-plant interaction. Nevertheless, it appears that bacteria can use plenty of metabolites as nutrient resources in tumors and have to cope with toxic compounds.
Opine Catabolism and Tumor Colonization
Pathogenic agrobacteria can catabolize opines using genes carried by their Ti plasmid. Opines are mainly used as carbon and nitrogen sources, but can also be used as phosphate or sulfur sources (Dessaux et al., 1998; Flores-Mireles et al., 2012; Dessaux and Faure, 2018). One of the features of the Ti plasmid is that it confers the bacterium that harbors it the possibility to catabolize a specific type of opine determined by the Ti plasmid-type (Gordon and Christie, 2014). The genes involved in the uptake of the well-described opines are actively studied (Marty et al., 2016, 2019; Dessaux and Faure, 2018). Among them, in Agrobacterium strain C58, nocT (atu6027) encodes a periplasmic binding protein (PBP) involved in the binding of nopaline and pyronopaline (Lang et al., 2014). The ocd (atu6016) gene encodes an ornithine cyclodeaminase involved in nopaline and pyronopaline degradation (Sans et al., 1987). nocT and ocd mutant strains are unable to use and assimilate these two opines. In competition assays for tumor niche occupancy, the wild-type strain outcompeted the two mutant strains, indicating that opines favor the survival of bacteria able to catabolize them under competitive conditions (Lang et al., 2014).
Interestingly, after individual inoculation, the wild-type and the two nocT and ocd mutants reached the same population level (Lang et al., 2014). This finding implies that the tumor provided other carbon sources that supported the growth of the two mutants. Accordingly, metabolomics studies and the Agrobacterium tumor transcriptome revealed a large diversity of available carbon sources together with the up-regulation of numerous and diverse genes involved in metabolism (Deeken et al., 2006; Simoh et al., 2009; González-Mula et al., 2019).
Additional Nutrient Resources
Annotation of the Agrobacterium strain C58 genome predicted a large set of transporters putatively involved in the import of plant compounds, composed of 190 ABC (ATP-binding cassette transporters) and 3 TRAP (Tripartite ATP-independent periplasmic) transporters (Goodner et al., 2001; Wood et al., 2001). However, only a few of them has been characterized. The presence of multiple transporters for one substrate could be beneficial for the fitness of Agrobacterium in competition with other microorganisms and a redundancy of transporters importing plant substrates is found in Agrobacterium. For example, the gguABC-chvE (atu2345–atu2348) and the gxySBA (atu3574–atu3576) clusters are two distinct ABC transporters allowing for D-glucose, D-xylose, D-fucose, D-galactose, D-glucosamine, and L-arabinose uptake in Agrobacterium (Zhao and Binns, 2011, 2014). D-glucosamine, D-fucose, and D-glucose transport were shown experimentally dependent on both transporters (Zhao and Binns, 2014). The presence of additional transporters was suggested for L-arabinose, D-xylose, and D-galactose, as the strains deleted for genes encoding both transporters (gguABC-chvE and gxySBA) could still grow on these compounds (Zhao and Binns, 2014). In the case of D-galactose, the mel operon (encoding an ABC transporter) was suggested as the additional transport system in Agrobacterium (Meyer et al., 2018b). Expression of the Agrobacterium mel operon was induced inside the tumor (González-Mula et al., 2018) and a deletion mutant of the PBP uptake gene of this operon (responsible for melibiose, galactinol, raffinose, and stachyose uptake) was less competitive than the wild-type strain (Meyer et al., 2018b). However, it is not yet known whether the mel-imported compounds are used for osmoprotection and/or nutrition.
Sugars such as glucose, fructose, sucrose, xylose, fucose, and arabinose are commonly degraded by plant-associated bacteria (Lugtenberg et al., 1999; Gunina and Kuzyakov, 2015). Only a few catabolic pathways involved in plant compound assimilation have been described in Agrobacterium, mainly in strain C58. The pycA gene (atu2726) encodes a glucose-6P isomerase essential for Agrobacterium growth on sucrose, fructose, and glucose, which are abundant compounds in the tumor (González-Mula et al., 2019). Accordingly, a pycA deletion mutant was affected in its capacity to compete with a wild-type strain in the tumor. A similar result is obtained with a mutant strain unable to degrade GHB. This compound is degraded in Agrobacterium by BlcRABC (formerly known as the AttJKLM system, Atu5136-Atu5139; Carlier et al., 2004). The BlcRABC mutant was outcompeted by the wild-type strain in tumors (Haudecoeur et al., 2009a; González-Mula et al., 2019).
Some bacterial pathogens feed on plant cell wall degradation products (Reverchon and Nasser, 2013). Agrobacterium strain C58 was suggested to be able to degrade plant cell wall. For example, thanks to XynA (Atu2371), C58 strain is able to grow on xylan, a common component of plant cell wall (Mathews et al., 2019). The polygalacturonase PglA (Atu3129) and PglB (Atu4560) are involved in polygalacturonic acid degradation (Mathews et al., 2019). The pglA gene was suggested to form an operon with picA, a gene that is induced by plant extracts (Rong et al., 1991; Mathews et al., 2019). Interestingly, although galacturonic acid is detected in tumors (González-Mula et al., 2019), only picA is overexpressed (González-Mula et al., 2018). Agrobacterium can import galacturonic acid owing to the gaaPQM operon (atu3135-atu3137) which also allows for glucuronic acid uptake, yet to a lesser extent. Both organic acids can be used in vitro for growth (Zhao and Binns, 2016). Galacturonic acid is transformed into α-ketoglutarate by enzymes encoded by atu3138-atu3143 (for details about galacturonic acid degradation, see Boer et al., 2010; Andberg et al., 2012; Taberman et al., 2014a,b; Zhao and Binns, 2016).
Agrobacterium strain C58 possesses other genetic determinants that allow it to grow on plant compounds but whose role and importance in tumor colonization have not yet been specifically studied. For example, Agrobacterium possesses a functional palatinose and trehalose uptake and degradation system (Ampomah et al., 2013). The thuEFGKAB operon (also known as the palEFGKAB operon; atu3338-atu3343) is composed of genes encoding an ABC transporter (thuFGK) linked to a gene encoding a PBP (thuE), and the thuB and thuA genes are those involved in the degradation process (Ampomah et al., 2013). Deletion of the genes coding for the ABC transporter abolished Agrobacterium growth on palatinose (De Costa et al., 2003), while maltitol, trehalose, and isomeric forms of sucrose were still imported and used for growth, again suggesting transporter redundancy. The thuEFGK deletion mutant is not required for tumor formation but as often, its role in the competitive colonization of the tumor has not been assessed yet. In addition to the above-described genes, other genes appear to be up-regulated in the tumor as compared to minimal media, and could be involved in the metabolism of carbon sources according to their annotation (González-Mula et al., 2018, 2019). Thus, Agrobacterium seems to be capable of using a large set of plant compounds to sustain its growth inside the tumor. Besides the functional characterization of genes involved in the uptake and catabolism of these plant compounds, additional efforts to decipher their roles not only in vitro, but also in planta and in competitive plant colonization are needed. Finally, whether Agrobacterium displays a preference for opines rather than for other carbon sources available in the tumor remains an open question.
Toxic Compounds
The host plant also produces potential antibacterial compounds in the tumor, including phenolic compounds (Schwalm et al., 2003; Cushnie and Lamb, 2005; Deeken et al., 2006; Simoh et al., 2009; Shi et al., 2016; González-Mula et al., 2019). The VirH2 protein, an O-demethylase whose pTi-located gene is highly expressed in tumors, plays a role in the transformation of ferulic acid (highly present in tumors) into caffeic acid, a less toxic phenolic compound (Brencic et al., 2004; González-Mula et al., 2019). Some chromosomal genes are also involved in phenolic compound degradation. In “A. fabrum” species, the SpG8-1b genomic region is involved in ferulic acid, caffeic acid, and p-coumaric acid degradation (Lassalle et al., 2011; Campillo et al., 2014; Baude et al., 2016). In addition to detoxifying these phenolic compounds, some of these genetic determinants extend the metabolic versatility of “A. fabrum” by weakly sustaining growth (Campillo et al., 2014). Their expression is tightly regulated by the HcaR repressor, and this regulation is important for bacterial fitness in the tumor (Meyer et al., 2018a). Other non-characterized pathways putatively annotated as phenolic compound degradation pathways are present in other Agrobacterium species, highlighting the importance of this function (Lassalle et al., 2017).
The action of efflux pumps is another mechanism allowing Agrobacterium survival in the presence of toxic compounds. In Agrobacterium strain C58, three operons have been described, all located on the linear chromid. The AcrABR efflux system (atu3003–atu3001) was shown to export numerous toxic compounds and to confer resistance to high concentrations of these compounds (Nuonming et al., 2018). The EmrBAR efflux pump (atu4479–atu4476) has recently been shown to confer resistance to toxic compounds and to be induced by indole and flavonoids among which quercetin, a compound detected in tumor tissues (see section “Tumor Metabolite Content”; Simoh et al., 2009; Lee et al., 2015; Khemthong et al., 2019). Both of these systems could be useful for bacterial survival in tumors. However, the corresponding genes (acrABR and emrBAR) were not up-regulated in 3-week-old A. thaliana tumors as compared to the culture medium (González-Mula et al., 2018). This study rather revealed overexpression of tetR/tetA (atu4205–atu4206), which encodes an efflux pump conferring resistance to tetracycline (González-Mula et al., 2018). To date, the plant compounds genuinely exported by TetR/TetA remain unknown (Luo and Farrand, 1999; Lassalle et al., 2011).
Agrobacterium Faces Microbial Competition in the Tumor
Agrobacterium long-term colonization of the tumor is partly dependent on competitions for opine nutritive resource, either between strains of Agrobacterium or with other microorganisms. Inside tumors, opines are public goods that are produced by the infected plant cells elicited by virulent Agrobacterium. However, opines can be shared within all the opines-catabolizing populations, not necessarily the virulent strains that originally induced tumors (For a review, see Platt et al., 2014). Tumors were shown to contain avirulent but opines-catabolizing Agrobacterium strains (either from environmental origin or derived from the strain inducing the tumor) (Bélanger et al., 1995; Llop et al., 2009). Those strains can be considered as cheaters (not expressing virulence genes but using opines), that may outcompete the Agrobacterium virulent population, burdened by the cost of infecting the plant due to the expression of virulence genes (Platt et al., 2012a,b). Virulence genes are still highly expressed in A. thaliana tumors, even 3 weeks post inoculation (González-Mula et al., 2018). The cost of the pTi plasmid could thus lead to plasmidless-genotypes dominance inside tumors. However, in the opine-rich tumor environment, the cost of the Ti plasmid is counterbalanced by opine benefits (as reviewed by Platt et al., 2014). In any case, competition for opine and tumor colonization occurs between Agrobacterium strains. Such a competition would have consequences on the persistence of the pTi rather than on Agrobacterium itself.
Besides, the presence of different Agrobacterium strains, it was recently reported that the microbial community of natural Vitis vinifera tumors caused by Allorhizobium vitis (another tumorigenic Rhizobiaceae species previously known as Agrobacterium vitis) contains more than 150 species, among which members of the Pseudomonas, Sphingomonas, Erwinia, and Bradyrhizobium genera (Faist et al., 2016). Unfortunately, no such global analyses have been conducted for Agrobacterium-induced tumors. Nonetheless, some studies report that tumors can harbor bacteria belonging to the Corynebacterium or the Arthrobacter genera or even to the E. meliloti species, a well-known plant symbiont (Tremblay et al., 1987; Nautiyal and Dion, 1990; Moore et al., 1997). The tumor environment can also shelter fungal species that are also opine degraders, such as Cylindrocarpon destructans, C. heteronema, and Fusarium solani (Beauchamp et al., 1990). Moreover, in the tumor, some Pseudomonas putida strains can catabolize mannopine, and some fungi catabolize mannopine and succinamopine (Nautiyal and Dion, 1990). Pseudomonas spp. can take the advantage over Agrobacterium when co-cultured in a medium supplemented with octopine, indicating that Pseudomonas spp. could use octopine more efficiently than Agrobacterium in vitro (Bell et al., 1990). In addition to metabolic abilities, other genetic determinants may allow Agrobacterium to compete with the tumor microbiota to durably settle in this environment.
The Type VI Secretion System (T6SS) described in several Agrobacterium strains could represent one such feature (Wu et al., 2012, 2019; Lin et al., 2013; Ma et al., 2014; Bondage et al., 2016). T6SS is a molecular syringe that injects effectors such as DNAse and amidase into target cells to kill them (Ma et al., 2014; Bondage et al., 2016). In the Agrobacterium C58 strain, this system is induced in acidic conditions by the ChvG/I two-component system and repressed by ExoR (Wu et al., 2012; Heckel et al., 2014). The T6SS expression is also induced upon high level of intracellular cyclic di-GMP (McCarthy et al., 2019). Interestingly, a P. aeruginosa T6SS was shown to inhibit “A. fabrum” growth in vitro (Ma et al., 2014). However, after co-infiltration in leaves, wild-type “A. fabrum” cells of strain C58 outcompeted P. aeruginosa whereas T6SS mutants were unable to do so (Ma et al., 2014). Thus, “A. fabrum” T6SS is efficient in planta but not in vitro. This suggests that plant compounds induce the expression of “A. fabrum” T6SS genes and/or affect P. aeruginosa competitive properties.
The ability to catch and/or sequester iron is important for competition under low-iron conditions, and iron scavenging is known as a plant defense mechanism against phytopathogens (Aznar et al., 2014; Niehus et al., 2017). In this context, siderophores, the most widespread bacterial system for iron acquisition, are certainly determining for the competitive colonization of tumors (Aznar et al., 2014; Verbon et al., 2017). The different Agrobacterium species seem able to produce distinct siderophores, but an operon involved in siderophore biosynthesis has so far only been characterized in Agrobacterium strain C58 (Rondon et al., 2004). Deletion of this large gene cluster (nearly 50 kb) abolished the bacterial capacity to survive in an iron-limited medium (Rondon et al., 2004; Liu et al., 2016). However, a tight control of siderophore gene expression is needed because this expression can be metabolically costly (Miethke and Marahiel, 2007; Harrison et al., 2008; Verbon et al., 2017). Regulatory proteins controlling siderophore biosynthesis are RirA, Irr, and SigI, a sigma factor influenced by heme and Fe-S concentrations (Figure 2; Qi et al., 1999; Ngok-Ngam et al., 2009; Hibbing and Fuqua, 2011). The exact mechanism of regulation by SigI and the structure of “A. fabrum” C58 siderophore remain unknown. The global regulator LsrB was also recently shown to be involved in iron homeostasis by positively regulating siderophore biosynthesis genes (Tang et al., 2018). Among the microorganisms highly adapted to the plant environment, some possess multiple receptors and ABC transporters; some of these make the uptake of heterologous siderophores possible (Loper and Buyer, 1991; Lemanceau et al., 2009; Berendsen et al., 2015). The Agrobacterium strain C58 genome contains three ABC transporters annotated as useful for iron uptake (atu0408–atu0406; atu2473–atu2476; atu5311–atu5316; respectively on circular chromosome, linear chromid, and At plasmid); they appear to be up-regulated in tumors as compared to culture media (González-Mula et al., 2018). Information about the compound actually transported in Agrobacterium or about the specificity of those transporters is unavailable, but it is tempting to speculate that these compounds are important for the uptake of heterologous siderophores and for bacterial fitness within tumors.
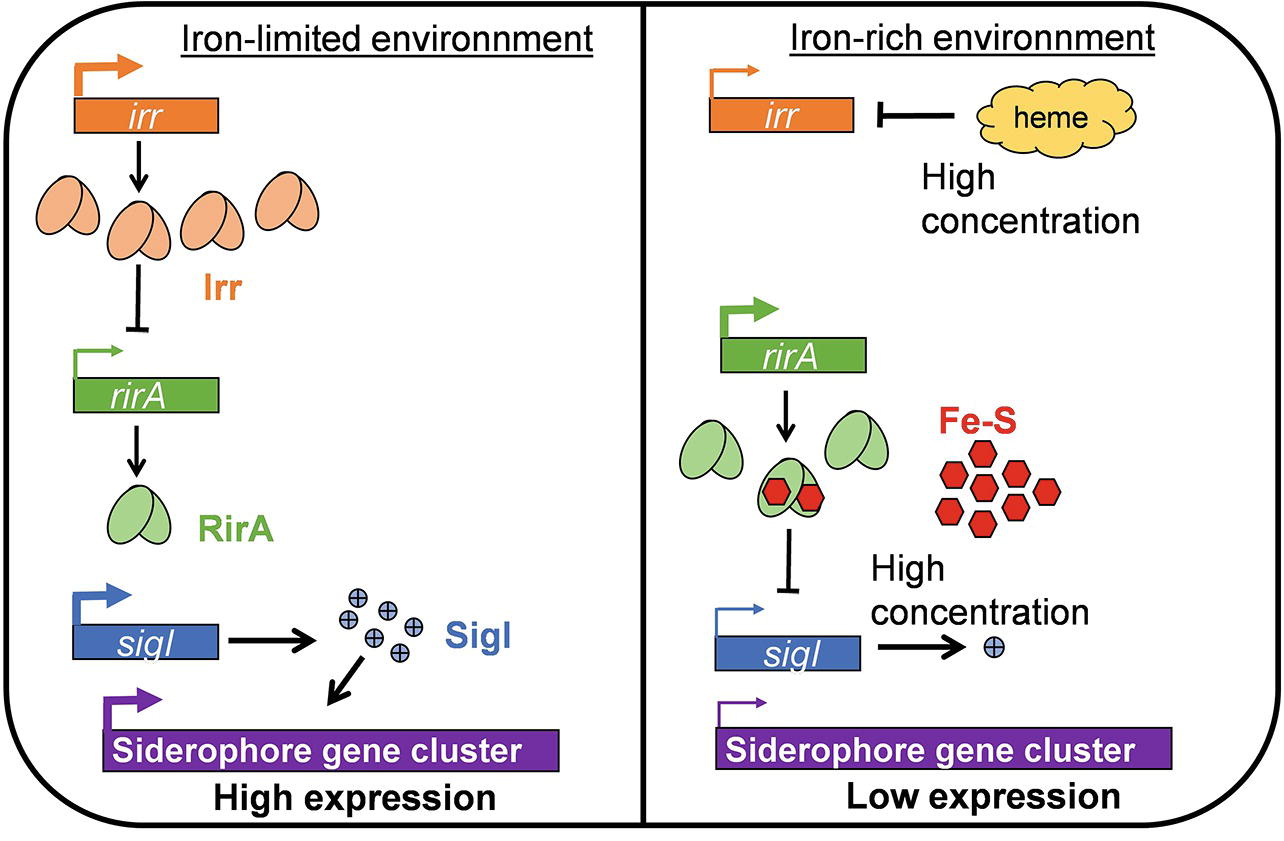
Figure 2. Model of the regulation of siderophore gene cluster expression according to iron availability. In an iron-limited environment, due to the low heme and Fe-S concentrations, the Irr protein is abundant and represses rirA. Consequently, SigI, which is under the negative control of RirA, is expressed and can induce siderophore synthesis. In an iron-rich environment, Irr is negatively regulated by the high heme concentration. In these conditions, RirA is abundant and its binding to Fe-S allows for the repression of sigI, which leads to the very low expression of siderophore biosynthesis genes.
Concluding Remarks
In tumors, agrobacteria are exposed to plant defense reactions, hypoxic and drought conditions, as well as competition with other tumor dwellers. Even if the opine concept explains a major part of the Agrobacterium-plant interaction, it cannot solely justify Agrobacterium maintenance and competitiveness in tumors. The high tumor-colonizing capacity of Agrobacterium is likely to be conferred by its ability to survive stresses encountered in tumors, to kill competitors, its metabolic capacities, and efficient resource uptake.
Unfortunately, the tumor metabolites content is sparsely described. To date, only a few untargeted metabolites studies provide valuable but limited information since they were performed at different stages (i.e., 4- to 5-week-old tumors) on different plant species (B. rapa, A. thaliana, S. lycopersicum) and with different analytical methods (Deeken et al., 2006; Simoh et al., 2009; González-Mula et al., 2019). The integration of metabolite and plant gene expression analyses contributes to a better understanding of the changes that occur during tumor development and of the way the plant faces such modifications (Deeken et al., 2006). Moreover, integrated analyses of the two partners would be essential to understand the different aspects of the long-term interaction inside the tumor.
Even if Agrobacterium has been the subject of many functional studies, genetic characterizations have mostly been focused on virulence genes, the factors influencing T-DNA integration, and the efficiency of plant genetic transformation. There is growing interest in the characterization of the genetic determinants that allow for rhizosphere colonization, and more recently for the transition between the rhizosphere and pathogenic lifestyles. Many bacterial functions highlighted in this review are indeed found in non-tumor environments. EPS and siderophores are usually described as essential for root colonization (as reviewed by Dessaux and Faure, 2018; Matthysse, 2018). Moreover, the import and catabolism of melibiose, raffinose, stachyose, and galactinol have recently been showed to confer a competitive advantage for rhizosphere colonization (Meyer et al., 2018b). The ability of Agrobacterium to synthesize hormones might modify the root architecture, as observed for plant-growth-promoting rhizobacteria (Vacheron et al., 2013), thus providing a favorable habitat to the bacterium. Therefore, besides the characterization of new genetic clusters, an additional effort is needed not only to decipher the role of these clusters in bacterial virulence (their tumor-inducing ability) as often tested, but also to evaluate their role in colonization, persistence, and in the rhizosphere-to-tumor transition. This would provide further insights into key determinants of Agrobacterium ecology, as well as valuable information on disease persistence and dissemination.
Author Contributions
CL, LV, TM, and CT-M conceived the idea and designed the outlines of the article. TM, CT-M, LV, FW-D, and CL wrote the manuscript. GC and IK contributed in revising manuscript. All authors listed have made a substantial, direct, and intellectual contribution to the work, and approved it for publication.
Funding
TM, LV, IK, and CL were supported by the CNRS (Mission pour l’interdisciplinarité, Agromics 2014-2016) and by the French national program EC2CO-Biohefect/Ecodyn/Dril/MicrobiEn (IBAD). TM received a doctoral grant from the French Ministère de l’Education Nationale, de l’Enseignement Supérieur et de la Recherche.
Conflict of Interest Statement
The authors declare that the research was conducted in the absence of any commercial or financial relationships that could be construed as a potential conflict of interest.
Acknowledgments
We thank A. Buchwalter for reading the manuscript and providing suggestions.
References
Adams, D. O., and Yang, S. F. (1979). Ethylene biosynthesis: identification of 1-aminocyclopropane-1-carboxylic acid as an intermediate in the conversion of methionine to ethylene. Proc. Natl. Acad. Sci. USA 76, 170–174. doi: 10.1073/pnas.76.1.170
Aloni, R. (1995). “The induction of vascular tissues by auxin and cytokinin” in Plant hormones: Physiology, biochemistry and molecular biology. ed. P. J. Davies (Dordrecht, Netherlands: Springer), 531–546.
Aloni, R., Wolf, A., Feigenbaum, P., Avni, A., and Klee, H. J. (1998). The never ripe mutant provides evidence that tumor-induced ethylene controls the morphogenesis of Agrobacterium tumefaciens-induced crown galls on tomato stems. Plant Physiol. 117, 841–849.
Ampomah, O. Y., Avetisyan, A., Hansen, E., Svenson, J., Huser, T., Jensen, J. B., et al. (2013). The thuEFGKAB operon of rhizobia and Agrobacterium tumefaciens codes for transport of trehalose, maltitol, and isomers of sucrose and their assimilation through the formation of their 3-keto derivatives. J. Bacteriol. 195, 3797–3807. doi: 10.1128/JB.00478-13
Anand, A., Uppalapati, S. R., Ryu, C.-M., Allen, S. N., Kang, L., Tang, Y., et al. (2008). Salicylic acid and systemic acquired resistance play a role in attenuating crown gall disease caused by Agrobacterium tumefaciens. Plant Physiol. 146, 703–715. doi: 10.1104/pp.107.111302
Andberg, M., Maaheimo, H., Boer, H., Penttilä, M., Koivula, A., and Richard, P. (2012). Characterization of a novel Agrobacterium tumefaciens galactarolactone cycloisomerase enzyme for direct conversion of D-galactarolactone to 3-deoxy-2-keto-L-threo-hexarate. J. Biol. Chem. 287, 17662–17671. doi: 10.1074/jbc.M111.335240
Aznar, A., Chen, N. W. G., Rigault, M., Riache, N., Joseph, D., Desmaële, D., et al. (2014). Scavenging iron: a novel mechanism of plant immunity activation by microbial siderophores. Plant Physiol. 164, 2167–2183. doi: 10.1104/pp.113.233585
Baek, S.-H., and Shapleigh, J. P. (2005). Expression of nitrite and nitric oxide reductases in free-living and plant-associated Agrobacterium tumefaciens C58 sells. Appl. Environ. Microbiol. 71, 4427–4436. doi: 10.1128/AEM.71.8.4427-4436.2005
Barton, I. S., Fuqua, C., and Platt, T. G. (2018). Ecological and evolutionary dynamics of a model facultative pathogen: Agrobacterium and crown gall disease of plants. Environ. Microbiol. 20, 16–29. doi: 10.1111/1462-2920.13976
Baude, J., Vial, L., Villard, C., Campillo, T., Lavire, C., Nesme, X., et al. (2016). Coordinated regulation of species-specific hydroxycinnamic acid degradation and siderophore biosynthesis pathways in Agrobacterium fabrum. Appl. Environ. Microbiol. 82, 3515–3524. doi: 10.1128/AEM.00419-16
Beauchamp, C. J., Chilton, W. S., Dion, P., and Antoun, H. (1990). Fungal catabolism of crown gall opines. Appl. Environ. Microbiol. 56, 150–155.
Bélanger, C., Canfield, M. L., Moore, L. W., and Dion, P. (1995). Genetic analysis of nonpathogenic Agrobacterium tumefaciens mutants arising in crown gall tumors. J. Bacteriol. 177, 3752–3757. doi: 10.1128/jb.177.13.3752-3757.1995
Bell, C. R., Cummings, N. E., Canfield, M. L., and Moore, L. W. (1990). Competition of octopine-catabolizing Pseudomonas spp. and octopine-type Agrobacterium tumefaciens for octopine in chemostats. Appl. Environ. Microbiol. 56, 2840–2846.
Bellin, D., Asai, S., Delledonne, M., and Yoshioka, H. (2013). Nitric oxide as a mediator for defense responses. Mol. Plant-Microbe Interact. 26, 271–277. doi: 10.1094/MPMI-09-12-0214-CR
Berendsen, R. L., van Verk, M. C., Stringlis, I. A., Zamioudis, C., Tommassen, J., Pieterse, C. M. J., et al. (2015). Unearthing the genomes of plant-beneficial Pseudomonas model strains WCS358, WCS374 and WCS417. BMC Genomics 16:539. doi: 10.1186/s12864-015-1632-z
Boer, H., Maaheimo, H., Koivula, A., Penttilä, M., and Richard, P. (2010). Identification in Agrobacterium tumefaciens of the D-galacturonic acid dehydrogenase gene. Appl. Microbiol. Biotechnol. 86, 901–909. doi: 10.1007/s00253-009-2333-9
Boncompagni, E., Østerås, M., Poggi, M.-C., and le Rudulier, D. (1999). Occurrence of choline and glycine betaine uptake and metabolism in the family Rhizobiaceae and rheir roles in osmoprotection. Appl. Environ. Microbiol. 65, 2072–2077.
Bondage, D. D., Lin, J.-S., Ma, L.-S., Kuo, C.-H., and Lai, E.-M. (2016). VgrG C terminus confers the type VI effector transport specificity and is required for binding with PAAR and adaptor–effector complex. Proc. Natl. Acad. Sci. USA 113, E3931–E3940. doi: 10.1073/pnas.1600428113
Bougouffa, S., Radovanovic, A., Essack, M., and Bajic, V. B. (2014). DEOP: a database on osmoprotectants and associated pathways. Database 2014:bau100. doi: 10.1093/database/bau100
Brader, G., Compant, S., Vescio, K., Mitter, B., Trognitz, F., Ma, L.-J., et al. (2017). Ecology and genomic insights into plant-pathogenic and plant-nonpathogenic endophytes. Annu. Rev. Phytopathol. 55, 61–83. doi: 10.1146/annurev-phyto-080516-035641
Brencic, A., Eberhard, A., and Winans, S. C. (2004). Signal quenching, detoxification and mineralization of vir gene-inducing phenolics by the VirH2 protein of Agrobacterium tumefaciens. Mol. Microbiol. 51, 1103–1115. doi: 10.1046/j.1365-2958.2003.03887.x
Bruto, M., Prigent-Combaret, C., Muller, D., and Moënne-Loccoz, Y. (2014). Analysis of genes contributing to plant-beneficial functions in plant growth-promoting rhizobacteria and related. Proteobacteria. Sci. Rep. 4. doi: 10.1038/srep06261
Campillo, T., Renoud, S., Kerzaon, I., Vial, L., Baude, J., Gaillard, V., et al. (2014). Analysis of hydroxycinnamic acid degradation in Agrobacterium fabrum reveals a coenzyme A-dependent, beta-oxidative deacetylation pathway. Appl. Environ. Microbiol. 80, 3341–3349. doi: 10.1128/AEM.00475-14
Cangelosi, G. A., Martinetti, G., and Nester, E. W. (1990). Osmosensitivity phenotypes of Agrobacterium tumefaciens mutants that lack periplasmic beta-1,2-glucan. J. Bacteriol. 172, 2172–2174. doi: 10.1128/jb.172.4.2172-2174.1990
Carlier, A., Chevrot, R., Dessaux, Y., and Faure, D. (2004). The assimilation of gamma-butyrolactone in Agrobacterium tumefaciens C58 interferes with the accumulation of the N-acyl-homoserine lactone signal. Mol. Plant-Microbe Interact. 17, 951–957. doi: 10.1094/MPMI.2004.17.9.951
Costet, L., Cordelier, S., Dorey, S., Baillieul, F., Fritig, B., and Kauffmann, S. (1999). Relationship between localized acquired resistance (LAR) and the hypersensitive response (HR): HR is necessary for LAR to occur and salicylic acid is not sufficient to trigger LAR. Mol. Plant-Microbe Interact. 12, 655–662. doi: 10.1094/MPMI.1999.12.8.655
Cushnie, T. P. T., and Lamb, A. J. (2005). Antimicrobial activity of flavonoids. Int. J. Antimicrob. Agents 26, 343–356. doi: 10.1016/j.ijantimicag.2005.09.002
Dandapath, I., Chatterjee, M., Sarkar, D., Gupta, A., Rabbani, G., and Minakshi, R. (2017). “Bacterial osmolyte system and its physiological roles” in Cellular osmolytes: From chaperoning protein folding to clinical perspectives. eds. L. Rajendrakumar Singh and T. A. Dar (Singapore: Springer Singapore), 229–249.
De Costa, D. M., Suzuki, K., and Yoshida, K. (2003). Structural and functional analysis of a putative gene cluster for palatinose transport on the linear chromosome of Agrobacterium tumefaciens MAFF301001. J. Bacteriol. 185, 2369–2373. doi: 10.1128/jb.185.7.2369-2373.2003
Deeken, R., Engelmann, J. C., Efetova, M., Czirjak, T., Müller, T., Kaiser, W. M., et al. (2006). An integrated view of gene expression and solute profiles of Arabidopsis tumors: a genome-wide approach. Plant Cell 18, 3617–3634. doi: 10.1105/tpc.106.044743
Delledonne, M., Xia, Y., Dixon, R. A., and Lamb, C. (1998). Nitric oxide functions as a signal in plant disease resistance. Nature 394, 585–588. doi: 10.1038/29087
Del Valle, P., García-Armesto, M. R., de Arriaga, D., González-Donquiles, C., Rodríguez-Fernández, P., and Rúa, J. (2016). Antimicrobial activity of kaempferol and resveratrol in binary combinations with parabens or propyl gallate against Enterococcus faecalis. Food Control 61, 213–220. doi: 10.1016/j.foodcont.2015.10.001
Dessaux, Y., and Faure, D. (2018). “Niche construction and exploitation by Agrobacterium: how to survive and face competition in soil and plant habitats” in Agrobacterium Biology: From Basic Science to Biotechnology Current Topics in Microbiology and Immunology. ed. S. B. Gelvin (Cham: Springer International Publishing), 55–86.
Dessaux, Y., Petit, A., Farrand, S. K., and Murphy, P. J. (1998). “Opines and opine-like molecules involved in plant-rhizobiaceae interactions” in The rhizobiaceae: Molecular biology of model plant-associated bacteria. eds. H. P. Spaink, A. Kondorosi, and P. J. J. Hooykaas (Dordrecht, Netherlands: Springer), 173–197.
Domínguez-Ferreras, A., Pérez-Arnedo, R., Becker, A., Olivares, J., Soto, M. J., and Sanjuán, J. (2006). Transcriptome profiling reveals the importance of plasmid pSymB for osmoadaptation of Sinorhizobium meliloti. J. Bacteriol. 188, 7617–7625. doi: 10.1128/JB.00719-06
Duprey, A., Reverchon, S., and Nasser, W. (2014). Bacterial virulence and Fis: adapting regulatory networks to the host environment. Trends Microbiol. 22, 92–99. doi: 10.1016/j.tim.2013.11.008
Durrant, W. E., and Dong, X. (2004). Systemic acquired resistance. Annu. Rev. Phytopathol. 42, 185–209. doi: 10.1146/annurev.phyto.42.040803.140421
Efetova, M., Zeier, J., Riederer, M., Lee, C.-W., Stingl, N., Mueller, M., et al. (2007). A central role of abscisic acid in drought stress protection of Agrobacterium-induced tumors on Arabidopsis. Plant Physiol. 145, 853–862. doi: 10.1104/pp.107.104851
Engering, A., Hogerwerf, L., and Slingenbergh, J. (2013). Pathogen–host–environment interplay and disease emergence. Emerging Microbes Infect. 2:e5. doi: 10.1038/emi.2013.5
Faist, H., Keller, A., Hentschel, U., and Deeken, R. (2016). Grapevine (Vitis vinifera) crown galls host distinct microbiota. Appl. Environ. Microbiol. 82, 5542–5552. doi: 10.1128/AEM.01131-16
Flores-Mireles, A. L., Eberhard, A., and Winans, S. C. (2012). Agrobacterium tumefaciens can obtain sulphur from an opine that is synthesized by octopine synthase using S-methylmethionine as a substrate. Mol. Microbiol. 84, 845–856. doi: 10.1111/j.1365-2958.2012.08061.x
Gelvin, S. B. (2017). Integration of Agrobacterium T-DNA into the plant genome. Annu. Rev. Genet. 51, 195–217. doi: 10.1146/annurev-genet-120215-035320
Gohlke, J., and Deeken, R. (2014). Plant responses to Agrobacterium tumefaciens and crown gall development. Front. Plant Sci. 5:155. doi: 10.3389/fpls.2014.00155
González-Mula, A., Lachat, J., Mathias, L., Naquin, D., Lamouche, F., Mergaert, P., et al. (2019). The biotroph Agrobacterium tumefaciens thrives in tumors by exploiting a wide spectrum of plant host metabolites. New Phytol. 222, 455–467. doi: 10.1111/nph.15598
González-Mula, A., Lang, J., Grandclément, C., Naquin, D., Ahmar, M., Soulère, L., et al. (2018). Lifestyle of the biotroph Agrobacterium tumefaciens in the ecological niche constructed on its host plant. New Phytol. 219, 350–362. doi: 10.1111/nph.15164
Goodner, B., Hinkle, G., Gattung, S., Miller, N., Blanchard, M., Qurollo, B., et al. (2001). Genome sequence of the plant pathogen and biotechnology agent Agrobacterium tumefaciens C58. Science 294, 2323–2328. doi: 10.1126/science.1066803
Gordon, J. E., and Christie, P. J. (2014). The Agrobacterium Ti plasmids. Microbiol. Spectr. 2:PLAS-0010-2013. doi: 10.1128/microbiolspec.PLAS-0010-2013
Gray, J., Gelvin, S. B., Meilan, R., and Morris, R. O. (1996). Transfer RNA is the source of extracellular isopentenyladenine in a Ti-plasmidless strain of Agrobacterium tumefaciens. Plant Physiol. 110, 431–438. doi: 10.1104/pp.110.2.431
Gunina, A., and Kuzyakov, Y. (2015). Sugars in soil and sweets for microorganisms: review of origin, content, composition and fate. Soil Biol. Biochem. 90, 87–100. doi: 10.1016/j.soilbio.2015.07.021
Guyon, P., Chilton, M. D., Petit, A., and Tempé, J. (1980). Agropine in “null-type” crown gall tumors: evidence for generality of the opine concept. Proc. Natl. Acad. Sci. USA 77, 2693–2697.
Harrison, F., Paul, J., Massey, R. C., and Buckling, A. (2008). Interspecific competition and siderophore-mediated cooperation in Pseudomonas aeruginosa. ISME J. 2, 49–55. doi: 10.1038/ismej.2007.96
Haudecoeur, E., Planamente, S., Cirou, A., Tannières, M., Shelp, B. J., Moréra, S., et al. (2009b). Proline antagonizes GABA-induced quenching of quorum-sensing in Agrobacterium tumefaciens. Proc. Natl. Acad. Sci. USA 106, 14587–14592. doi: 10.1073/pnas.0808005106
Haudecoeur, E., Tannières, M., Cirou, A., Raffoux, A., Dessaux, Y., and Faure, D. (2009a). Different regulation and roles of lactonases AiiB and AttM in Agrobacterium tumefaciens C58. Mol. Plant-Microbe Interact. 22, 529–537. doi: 10.1094/MPMI-22-5-0529
Heckel, B. C., Tomlinson, A. D., Morton, E. R., Choi, J.-H., and Fuqua, C. (2014). Agrobacterium tumefaciens exoR controls acid response genes and impacts exopolysaccharide synthesis, horizontal gene transfer, and virulence gene expression. J. Bacteriol. 196, 3221–3233. doi: 10.1128/JB.01751-14
Hibbing, M. E., and Fuqua, C. (2011). Antiparallel and interlinked control of cellular iron levels by the Irr and RirA regulators of Agrobacterium tumefaciens. J. Bacteriol. 193, 3461–3472. doi: 10.1128/JB.00317-11
Hwang, H.-H., Gelvin, S. B., and Lai, E.-M. (2015). Editorial: Agrobacterium biology and its application to transgenic plant production. Front. Plant Sci. 6:265. doi: 10.3389/fpls.2015.00265
Hwang, H.-H., Wang, M.-H., Lee, Y.-L., Tsai, Y.-L., Li, Y.-H., Yang, F.-J., et al. (2010). Agrobacterium-produced and exogenous cytokinin-modulated Agrobacterium-mediated plant transformation. Mol. Plant Pathol. 11, 677–690. doi: 10.1111/j.1364-3703.2010.00637.x
Ingram-Smith, C., and Miller, K. J. (1998). Effects of ionic and osmotic strength on the glucosyltransferase of Rhizobium meliloti responsible for cyclic β-(1,2)-glucan biosynthesis. Appl. Environ. Microbiol. 64, 1290–1297.
Ji, P., and Wilson, M. (2002). Assessment of the importance of similarity in carbon source utilization profiles between the biological control agent and the pathogen in biological control of bacterial speck of tomato. Appl. Environ. Microbiol. 68, 4383–4389. doi: 10.1128/AEM.68.9.4383-4389.2002
Kampschreur, M. J., Kleerebezem, R., Picioreanu, C., Bakken, L., Bergaust, L., de Vries, S., et al. (2012). Metabolic modeling of denitrification in Agrobacterium tumefaciens: a tool to study inhibiting and activating compounds for the denitrification pathway. Front. Microbiol. 3:370. doi: 10.3389/fmicb.2012.00370
Kempf, B., and Bremer, E. (1998). Uptake and synthesis of compatible solutes as microbial stress responses to high-osmolality environments. Arch. Microbiol. 170, 319–330.
Kerpen, L., Niccolini, L., Licausi, F., van Dongen, J. T., and Weits, D. A. (2019). Hypoxic conditions in crown galls induce plant anaerobic responses that support tumor proliferation. Front. Plant Sci. 10:56. doi: 10.3389/fpls.2019.00056
Khemthong, S., Nuonming, P., Dokpikul, T., Sukchawalit, R., and Mongkolsuk, S. (2019). Regulation and function of the flavonoid-inducible efflux system, emrR-emrAB, in Agrobacterium tumefaciens C58. Appl. Microbiol. Biotechnol. doi: 10.1007/s00253-019-09899-5
Klinkenberg, J., Faist, H., Saupe, S., Lambertz, S., Krischke, M., Stingl, N., et al. (2014). Two fatty acid desaturases, STEAROYL-ACYL CARRIER PROTEIN Δ9-DESATURASE6 and FATTY ACID DESATURASE3, are involved in drought and hypoxia stress signaling in Arabidopsis crown galls. Plant Physiol. 164, 570–583. doi: 10.1104/pp.113.230326
Krenek, P., Samajova, O., Luptovciak, I., Doskocilova, A., Komis, G., and Samaj, J. (2015). Transient plant transformation mediated by Agrobacterium tumefaciens: principles, methods and applications. Biotechnol. Adv. 33, 1024–1042. doi: 10.1016/j.biotechadv.2015.03.012
Lacroix, B., and Citovsky, V. (2013). The roles of bacterial and host plant factors in Agrobacterium-mediated genetic transformation. Int. J. Dev. Biol. 57, 467–481. doi: 10.1387/ijdb.130199bl
Lang, J., Vigouroux, A., Planamente, S., El Sahili, A., Blin, P., Aumont-Nicaise, M., et al. (2014). Agrobacterium uses a unique ligand-binding mode for trapping opines and acquiring a competitive advantage in the niche construction on plant host. PLoS Pathog. 10:e1004444. doi: 10.1371/journal.ppat.1004444
Lassalle, F., Campillo, T., Vial, L., Baude, J., Costechareyre, D., Chapulliot, D., et al. (2011). Genomic species are ecological species as revealed by comparative genomics in Agrobacterium tumefaciens. Genome Biol. Evol. 3, 762–781. doi: 10.1093/gbe/evr070
Lassalle, F., Planel, R., Penel, S., Chapulliot, D., Barbe, V., Dubost, A., et al. (2017). Ancestral genome estimation reveals the history of ecological diversification in Agrobacterium. Genome Biol. Evol. 9, 3413–3431. doi: 10.1093/gbe/evx255
Lee, C.-W., Efetova, M., Engelmann, J. C., Kramell, R., Wasternack, C., Ludwig-Müller, J., et al. (2009). Agrobacterium tumefaciens promotes tumor induction by modulating pathogen defense in Arabidopsis thaliana. Plant Cell 21, 2948–2962. doi: 10.1105/tpc.108.064576
Lee, J.-H., Kim, Y.-G., Baek, K.-H., Cho, M. H., and Lee, J. (2015). The multifaceted roles of the interspecies signalling molecule indole in Agrobacterium tumefaciens. Environ. Microbiol. 17, 1234–1244. doi: 10.1111/1462-2920.12560
Leitner, M., Vandelle, E., Gaupels, F., Bellin, D., and Delledonne, M. (2009). NO signals in the haze: nitric oxide signalling in plant defence. Curr. Opin. Plant Biol. 12, 451–458. doi: 10.1016/j.pbi.2009.05.012
Lemanceau, P., Expert, D., Gaymard, F., Bakker, P. A. H. M., and Briat, J.-F. (2009). “Chapter 12 role of iron in plant–microbe interactions” in Advances in botanical research advances in botanical research. ed. L. C. Van Loon (Amsterdam, Nederlands: Academic Press), 491–549.
Leonard, S., Hommais, F., Nasser, W., and Reverchon, S. (2017). Plant-phytopathogen interactions: bacterial responses to environmental and plant stimuli. Environ. Microbiol. 19, 1689–1716. doi: 10.1111/1462-2920.13611
Lin, J.-S., Ma, L.-S., and Lai, E.-M. (2013). Systematic dissection of the Agrobacterium type VI secretion system reveals machinery and secreted components for subcomplex formation. PLoS One 8:e67647. doi: 10.1371/journal.pone.0067647
Liu, P., and Nester, E. W. (2006). Indoleacetic acid, a product of transferred DNA, inhibits vir gene expression and growth of Agrobacterium tumefaciens C58. Proc. Natl. Acad. Sci. USA 103, 4658–4662. doi: 10.1073/pnas.0600366103
Liu, Z., Xie, Y., Zhang, X., Hu, X., Li, Y., Ding, X., et al. (2016). Efficient construction of large genomic deletion in Agrobacterium tumefaciens by combination of Cre/loxP system and triple recombineering. Curr. Microbiol. 72, 465–472. doi: 10.1007/s00284-015-0977-5
Llop, P., Murillo, J., Lastra, B., and López, M. M. (2009). Recovery of nonpathogenic mutant bacteria from tumors caused by several Agrobacterium tumefaciens strains: a frequent event? Appl. Environ. Microbiol. 75, 6504–6514. doi: 10.1128/AEM.01867-08
Loper, J. E., and Buyer, J. S. (1991). Siderophores in microbial interactions on plant surfaces. Mol. Plant-Microbe Interact. 4, 5–13. doi: 10.1094/MPMI-4-005
Lugtenberg, B. J., Kravchenko, L. V., and Simons, M. (1999). Tomato seed and root exudate sugars: composition, utilization by Pseudomonas biocontrol strains and role in rhizosphere colonization. Environ. Microbiol. 1, 439–446. doi: 10.1046/j.1462-2920.1999.00054.x
Luo, Z. Q., and Farrand, S. K. (1999). Cloning and characterization of a tetracycline resistance determinant present in Agrobacterium tumefaciens C58. J. Bacteriol. 181, 618–626.
Ma, L.-S., Hachani, A., Lin, J.-S., Filloux, A., and Lai, E.-M. (2014). Agrobacterium tumefaciens deploys a superfamily of type VI secretion DNase effectors as weapons for interbacterial competition in planta. Cell Host Microbe 16, 94–104. doi: 10.1016/j.chom.2014.06.002
Marty, L., Vigouroux, A., Aumont-Nicaise, M., Dessaux, Y., Faure, D., and Moréra, S. (2016). Structural basis for high specificity of amadori compound and mannopine opine binding in bacterial pathogens. J. Biol. Chem. 291, 22638–22649. doi: 10.1074/jbc.M116.745562
Marty, L., Vigouroux, A., Aumont-Nicaise, M., Pelissier, F., Meyer, T., Lavire, C., et al. (2019). Structural basis for two efficient modes of agropinic acid opine import into the bacterial pathogen Agrobacterium tumefaciens. Biochem. J. 476, 165–178. doi: 10.1042/BCJ20180861
Mathews, S. L., Hannah, H., Samagaio, H., Martin, C., Rodriguez-Rassi, E., and Matthysse, A. G. (2019). Glycoside hydrolase genes are required for virulence of Agrobacterium tumefaciens on Bryophyllum daigremontiana and tomato. Appl. Environ. Microbiol. 85:e00603-19. doi: 10.1128/AEM.00603-19
Matthysse, A. G. (2018). “Exopolysaccharides of Agrobacterium tumefaciens” in Agrobacterium biology: From basic science to biotechnology current topics in microbiology and immunology. ed. S. B. Gelvin (Cham: Springer International Publishing), 111–141.
McCarthy, R. R., Yu, M., Eilers, K., Wang, Y.-C., Lai, E.-M., and Filloux, A. (2019). Cyclic di-GMP inactivates T6SS and T4SS activity in Agrobacterium tumefaciens. Mol. Microbiol. doi: 10.1111/mmi.14279
McIntosh, M., Stone, B. A., and Stanisich, V. A. (2005). Curdlan and other bacterial (1→3)-β-d-glucans. Appl. Microbiol. Biotechnol. 68, 163–173. doi: 10.1007/s00253-005-1959-5
Meyer, T., Renoud, S., Vigouroux, A., Miomandre, A., Gaillard, V., Kerzaon, I., et al. (2018a). Regulation of hydroxycinnamic acid degradation drives Agrobacterium fabrum lifestyles. Mol. Plant-Microbe Interact. 31, 814–822. doi: 10.1094/MPMI-10-17-0236-R
Meyer, T., Vigouroux, A., Aumont-Nicaise, M., Comte, G., Vial, L., Lavire, C., et al. (2018b). The plant defense signal galactinol is specifically used as a nutrient by the bacterial pathogen Agrobacterium fabrum. J. Biol. Chem. 293, 7930–7941. doi: 10.1074/jbc.RA118.001856
Miethke, M., and Marahiel, M. A. (2007). Siderophore-based iron acquisition and pathogen control. Microbiol. Mol. Biol. Rev. 71, 413–451. doi: 10.1128/MMBR.00012-07
Moore, L. W., Chilton, W. S., and Canfield, M. L. (1997). Diversity of opines and opine-catabolizing bacteria isolated from naturally occurring crown gall tumors. Appl. Environ. Microbiol. 63, 201–207.
Nakjarung, K., Mongkolsuk, S., and Vattanaviboon, P. (2003). The oxyR from Agrobacterium tumefaciens: evaluation of its role in the regulation of catalase and peroxide responses. Biochem. Biophys. Res. Commun. 304, 41–47. doi: 10.1016/S0006-291X(03)00535-7
Nautiyal, C. S., and Dion, P. (1990). Characterization of the opine-utilizing microflora associated with samples of soil and plants. Appl. Environ. Microbiol. 56, 2576–2579.
Nester, E. W. (2014). Agrobacterium: nature’s genetic engineer. Front. Plant Sci. 5:730. doi: 10.3389/fpls.2014.00730
Ngok-Ngam, P., Ruangkiattikul, N., Mahavihakanont, A., Virgem, S. S., Sukchawalit, R., and Mongkolsuk, S. (2009). Roles of Agrobacterium tumefaciens RirA in iron regulation, oxidative stress response, and virulence. J. Bacteriol. 191, 2083–2090. doi: 10.1128/JB.01380-08
Niehus, R., Picot, A., Oliveira, N. M., Mitri, S., and Foster, K. R. (2017). The evolution of siderophore production as a competitive trait. Evol. Int. J. Org. Evol. 71, 1443–1455. doi: 10.1111/evo.13230
Nobile, S., and Deshusses, J. (1986). Transport of gamma-butyrobetaine in an Agrobacterium species isolated from soil. J. Bacteriol. 168, 780–784. doi: 10.1128/jb.168.2.780-784.1986
Nonaka, S., and Ezura, H. (2014). Plant-Agrobacterium interaction mediated by ethylene and super-Agrobacterium conferring efficient gene transfer. Front. Plant Sci. 5:681. doi: 10.3389/fpls.2014.00681
Nonaka, S., Someya, T., Zhou, S., Takayama, M., Nakamura, K., and Ezura, H. (2017). An Agrobacterium tumefaciens strain with gamma-aminobutyric acid transaminase activity shows an enhanced genetic transformation ability in plants. Sci. Rep. 7:42649. doi: 10.1038/srep42649
Nuonming, P., Khemthong, S., Dokpikul, T., Sukchawalit, R., and Mongkolsuk, S. (2018). Characterization and regulation of AcrABR, a RND-type multidrug efflux system, in Agrobacterium tumefaciens C58. Microbiol. Res. 214, 146–155. doi: 10.1016/j.micres.2018.06.014
Panikulangara, T. J., Eggers-Schumacher, G., Wunderlich, M., Stransky, H., and Schöffl, F. (2004). Galactinol synthase1. A novel heat shock factor target gene responsible for heat-induced synthesis of raffinose family oligosaccharides in Arabidopsis. Plant Physiol. 136, 3148–3158. doi: 10.1104/pp.104.042606
Pitzschke, A. (2013). Agrobacterium infection and plant defense—transformation success hangs by a thread. Front. Plant Sci. 4:519. doi: 10.3389/fpls.2013.00519
Platt, T. G., Bever, J. D., and Fuqua, C. (2012a). A cooperative virulence plasmid imposes a high fitness cost under conditions that induce pathogenesis. Proc. Biol. Sci. 279, 1691–1699. doi: 10.1098/rspb.2011.2002
Platt, T. G., Fuqua, C., and Bever, J. D. (2012b). Resource and competitive dynamics shape the benefits of public goods cooperation in a plant pathogen. Evol. Int. J. Org. Evol. 66, 1953–1965. doi: 10.1111/j.1558-5646.2011.01571.x
Platt, T. G., Morton, E. R., Barton, I. S., Bever, J. D., and Fuqua, C. (2014). Ecological dynamics and complex interactions of Agrobacterium megaplasmids. Front. Plant Sci. 5:635. doi: 10.3389/fpls.2014.00635
Qi, Z., Hamza, I., and O’Brian, M. R. (1999). Heme is an effector molecule for iron-dependent degradation of the bacterial iron response regulator (Irr) protein. Proc. Natl. Acad. Sci. USA 96, 13056–13061. doi: 10.1073/pnas.96.23.13056
Reverchon, S., and Nasser, W. (2013). Dickeya ecology, environment sensing and regulation of virulence programme. Environ. Microbiol. Rep. 5, 622–636. doi: 10.1111/1758-2229.12073
Romanuk, T. N., Zhou, Y., Brose, U., Berlow, E. L., Williams, R. J., and Martinez, N. D. (2009). Predicting invasion success in complex ecological networks. Philos. Trans. R. Soc. Lond. Ser. B Biol. Sci. 364, 1743–1754. doi: 10.1098/rstb.2008.0286
Rondon, M. R., Ballering, K. S., and Thomas, M. G. (2004). Identification and analysis of a siderophore biosynthetic gene cluster from Agrobacterium tumefaciens C58. Microbiology 150, 3857–3866. doi: 10.1099/mic.0.27319-0
Rong, L. J., Karcher, S. J., and Gelvin, S. B. (1991). Genetic and molecular analyses of picA, a plant-inducible locus on the Agrobacterium tumefaciens chromosome. J. Bacteriol. 173, 5110–5120. doi: 10.1128/jb.173.16.5110-5120.1991
Ruffing, A. M., and Chen, R. R. (2012). Transcriptome profiling of a curdlan-producing Agrobacterium reveals conserved regulatory mechanisms of exopolysaccharide biosynthesis. Microb. Cell Factories 11:17. doi: 10.1186/1475-2859-11-17
Saenkham, P., Eiamphungporn, W., Farrand, S. K., Vattanaviboon, P., and Mongkolsuk, S. (2007). Multiple superoxide dismutases in Agrobacterium tumefaciens: functional analysis, gene regulation, and influence on tumorigenesis. J. Bacteriol. 189, 8807–8817. doi: 10.1128/JB.00960-07
Sans, N., Schröder, G., and Schröder, J. (1987). The Noc region of Ti plasmid C58 codes for arginase and ornithine cyclodeaminase. Eur. J. Biochem. 167, 81–87.
Schurr, U., Schuberth, B., Aloni, R., Pradel, K. S., Schmundt, D., Jähne, B., et al. (1996). Structural and functional evidence for xylem-mediated water transport and high transpiration in Agrobacterium tumefaciens-induced tumors of Ricinus communis. Bot. Acta 109, 405–411. doi: 10.1111/j.1438-8677.1996.tb00590.x
Schwalm, K., Aloni, R., Langhans, M., Heller, W., Stich, S., and Ullrich, C. I. (2003). Flavonoid-related regulation of auxin accumulation in Agrobacterium tumefaciens-induced plant tumors. Planta 218, 163–178. doi: 10.1007/s00425-003-1104-6
Shams, M., Campillo, T., Lavire, C., Muller, D., Nesme, X., and Vial, L. (2012). Rapid and efficient methods to isolate, type strains and determine species of Agrobacterium spp. in pure culture and complex environments. Biochem. Test. 3–20. doi: 10.5772/36068
Shi, C., Zhang, X., Sun, Y., Yang, M., Song, K., Zheng, Z., et al. (2016). Antimicrobial activity of ferulic acid against Cronobacter sakazakii and possible mechanism of action. Foodborne Pathog. Dis. 13, 196–204. doi: 10.1089/fpd.2015.1992
Shih, P.-Y., Chou, S.-J., Müller, C., Halkier, B. A., Deeken, R., and Lai, E.-M. (2018). Differential roles of glucosinolates and camalexin at different stages of Agrobacterium-mediated transformation. Mol. Plant Pathol. 19, 1956–1970. doi: 10.1111/mpp.12672
Simoh, S., Quintana, N., Kim, H. K., Choi, Y. H., and Verpoorte, R. (2009). Metabolic changes in Agrobacterium tumefaciens-infected Brassica rapa. J. Plant Physiol. 166, 1005–1014. doi: 10.1016/j.jplph.2008.11.015
Singh, R., and Maclachlan, G. (1983). Transport and metabolism of sucrose versus hexoses in relation to growth in etiolated pea stem. Plant Physiol. 71, 531–535. doi: 10.1104/pp.71.3.531
Skoog, F., and Miller, C. O. (1957). Chemical regulation of growth and organ formation in plant tissues cultured in vitro. Symp. Soc. Exp. Biol. 11, 118–130.
Sokurenko, E. V., Gomulkiewicz, R., and Dykhuizen, D. E. (2006). Source–sink dynamics of virulence evolution. Nat. Rev. Microbiol. 4, 548–555. doi: 10.1038/nrmicro1446
Someya, T., Nonaka, S., Nakamura, K., and Ezura, H. (2013). Increased 1-aminocyclopropane-1-carboxylate deaminase activity enhances Agrobacterium tumefaciens-mediated gene delivery into plant cells. MicrobiologyOpen 2, 873–880. doi: 10.1002/mbo3.123
Spanu, P. D., and Panstruga, R. (2017). Editorial: Biotrophic Plant-Microbe Interactions. Front. Plant Sci. 8:192. doi: 10.3389/fpls.2017.00192
Stasinopoulos, S. J., Fisher, P. R., Stone, B. A., and Stanisich, V. A. (1999). Detection of two loci involved in (1→3)-β-glucan (curdlan) biosynthesis by Agrobacterium sp. ATCC31749, and comparative sequence analysis of the putative curdlan synthase gene. Glycobiology 9, 31–41. doi: 10.1093/glycob/9.1.31
Taberman, H., Andberg, M., Parkkinen, T., Jänis, J., Penttilä, M., Hakulinen, N., et al. (2014a). Structure and function of a decarboxylating Agrobacterium tumefaciens keto-deoxy-d-galactarate dehydratase. Biochemistry 53, 8052–8060. doi: 10.1021/bi501290k
Taberman, H., Andberg, M., Parkkinen, T., Richard, P., Hakulinen, N., Koivula, A., et al. (2014b). Purification, crystallization and preliminary X-ray diffraction analysis of a novel keto-deoxy-D-galactarate (KDG) dehydratase from Agrobacterium tumefaciens. Acta Crystallogr. Sect. F: Struct. Biol. Commun. 70, 49–52. doi: 10.1107/S2053230X13031361
Tang, G., Li, Q., Xing, S., Li, N., Tang, Z., Yu, L., et al. (2018). The LsrB protein is required for Agrobacterium tumefaciens interaction with host plants. Mol. Plant-Microbe Interact. 31, 951–961. doi: 10.1094/MPMI-02-18-0041-R
Tomlinson, A. D., Ramey-Hartung, B., Day, T. W., Merritt, P. M., and Fuqua, C. (2010). Agrobacterium tumefaciens ExoR represses succinoglycan biosynthesis and is required for biofilm formation and motility. Microbiology 156, 2670–2681. doi: 10.1099/mic.0.039032-0
Torres, M. A., Jones, J. D. G., and Dangl, J. L. (2006). Reactive oxygen species signaling in response to pathogens. Plant Physiol. 141, 373–378. doi: 10.1104/pp.106.079467
Tremblay, G., Gagliardo, R., Chilton, W. S., and Dion, P. (1987). Diversity among opine-utilizing bacteria: identification of coryneform isolates. Appl. Environ. Microbiol. 53, 1519–1524.
Vacheron, J., Desbrosses, G., Bouffaud, M.-L., Touraine, B., Moënne-Loccoz, Y., Muller, D., et al. (2013). Plant growth-promoting rhizobacteria and root system functioning. Front. Plant Sci. 4:356. doi: 10.3389/fpls.2013.00356
van der Does, H. C., and Rep, M. (2017). Adaptation to the host environment by plant-pathogenic fungi. Annu. Rev. Phytopathol. 55, 427–450. doi: 10.1146/annurev-phyto-080516-035551
van der Wolf, J., and De Boer, S. H. (2015). “Phytopathogenic bacteria” in Principles of plant-microbe interactions: Microbes for Sustainable Agriculture. ed. B. Lugtenberg (Cham: Springer International Publishing), 65–77.
Veena,, Jiang, H., Doerge, R. W., and Gelvin, S. B. (2003). Transfer of T-DNA and Vir proteins to plant cells by Agrobacterium tumefaciens induces expression of host genes involved in mediating transformation and suppresses host defense gene expression. Plant J. Cell Mol. Biol. 35, 219–236. doi: 10.1046/j.1365-313X.2003.01796.x
Verbon, E. H., Trapet, P. L., Stringlis, I. A., Kruijs, S., Bakker, P. A. H. M., and Pieterse, C. M. J. (2017). Iron and immunity. Annu. Rev. Phytopathol. 55, 355–375. doi: 10.1146/annurev-phyto-080516-035537
Veselov, D., Langhans, M., Hartung, W., Aloni, R., Feussner, I., Götz, C., et al. (2003). Development of Agrobacterium tumefaciens C58-induced plant tumors and impact on host shoots are controlled by a cascade of jasmonic acid, auxin, cytokinin, ethylene and abscisic acid. Planta 216, 512–522. doi: 10.1007/s00425-002-0883-5
Wächter, R., Langhans, M., Aloni, R., Götz, S., Weilmünster, A., Koops, A., et al. (2003). Vascularization, high-volume solution flow, and localized roles for enzymes of sucrose metabolism during tumorigenesis by Agrobacterium tumefaciens. Plant Physiol. 133, 1024–1037. doi: 10.1104/pp.103.028142
Wang, S., Yao, J., Zhou, B., Yang, J., Chaudry, M. T., Wang, M., et al. (2018). Bacteriostatic effect of quercetin as an antibiotic alternative in vivo and its antibacterial mechanism in vitro. J. Food Prot. 81, 68–78. doi: 10.4315/0362-028X.JFP-17-214
Wei, Z., Yang, T., Friman, V.-P., Xu, Y., Shen, Q., and Jousset, A. (2015). Trophic network architecture of root-associated bacterial communities determines pathogen invasion and plant health. Nat. Commun. 6:8413. doi: 10.1038/ncomms9413
Weiner, R., Langille, S., and Quintero, E. (1995). Structure, function and immunochemistry of bacterial exopolysaccharides. J. Ind. Microbiol. 15, 339–346. doi: 10.1007/BF01569989
Wobus, U., and Weber, H. (1999). Sugars as signal molecules in plant seed development. Biol. Chem. 380, 937–944. doi: 10.1515/BC.1999.116
Wood, D. W., Setubal, J. C., Kaul, R., Monks, D. E., Kitajima, J. P., Okura, V. K., et al. (2001). The genome of the natural genetic engineer Agrobacterium tumefaciens C58. Science 294, 2317–2323. doi: 10.1126/science.1066804
Wu, C.-F., Lin, J.-S., Shaw, G.-C., and Lai, E.-M. (2012). Acid-induced type VI secretion system is regulated by ExoR-ChvG/ChvI signaling cascade in Agrobacterium tumefaciens. PLoS Pathog. 8:e1002938. doi: 10.1371/journal.ppat.1002938
Wu, C.-F., Santos, M. N. M., Cho, S.-T., Chang, H.-H., Tsai, Y.-M., Smith, D. A., et al. (2019). Plant-Pathogenic Agrobacterium tumefaciens strains have diverse type VI effector-immunity pairs and vary in In-Planta competitiveness. Mol. Plant. Microbe Interact. MPMI-01-19-0021-R. doi: 10.1094/MPMI-01-19-0021-R
Xu, X. Q., and Pan, S. Q. (2000). An Agrobacterium catalase is a virulence factor involved in tumorigenesis. Mol. Microbiol. 35, 407–414. doi: 10.1046/j.1365-2958.2000.01709.x
Yuan, Z.-C., Edlind, M. P., Liu, P., Saenkham, P., Banta, L. M., Wise, A. A., et al. (2007). The plant signal salicylic acid shuts down expression of the vir regulon and activates quormone-quenching genes in Agrobacterium. Proc. Natl. Acad. Sci. U. S. A. 104, 11790–11795. doi: 10.1073/pnas.0704866104
Zhang, X., Zhang, L., Dong, F., Gao, J., Galbraith, D. W., and Song, C. P. (2001). Hydrogen peroxide is involved in abscisic acid-induced stomatal closure in Vicia faba. Plant Physiol. 126, 1438–1448. doi: 10.1104/pp.126.4.1438
Zhao, J., and Binns, A. N. (2011). Characterization of the mmsAB-araD1 (gguABC) genes of Agrobacterium tumefaciens. J. Bacteriol. 193, 6586–6596. doi: 10.1128/JB.05790-11
Zhao, J., and Binns, A. N. (2014). GxySBA ABC transporter of Agrobacterium tumefaciens and its role in sugar utilization and vir gene expression. J. Bacteriol. 196, 3150–3159. doi: 10.1128/JB.01648-14
Zhao, J., and Binns, A. N. (2016). Involvement of Agrobacterium tumefaciens galacturonate tripartite ATP-independent periplasmic (TRAP) transporter GaaPQM in virulence gene expression. Appl. Environ. Microbiol. 82, 1136–1146. doi: 10.1128/AEM.02891-15
Keywords: Agrobacterium tumefaciens, tumor lifestyle, crown gall, molecular traits, competition, plant defense
Citation: Meyer T, Thiour-Mauprivez C, Wisniewski-Dyé F, Kerzaon I, Comte G, Vial L and Lavire C (2019) Ecological Conditions and Molecular Determinants Involved in Agrobacterium Lifestyle in Tumors. Front. Plant Sci. 10:978. doi: 10.3389/fpls.2019.00978
Edited by:
Essaid Ait Barka, Université de Reims Champagne-Ardenne, FranceCopyright © 2019 Meyer, Thiour-Mauprivez, Wisniewski-Dyé, Kerzaon, Comte, Vial and Lavire. This is an open-access article distributed under the terms of the Creative Commons Attribution License (CC BY). The use, distribution or reproduction in other forums is permitted, provided the original author(s) and the copyright owner(s) are credited and that the original publication in this journal is cited, in accordance with accepted academic practice. No use, distribution or reproduction is permitted which does not comply with these terms.
*Correspondence: Céline Lavire, celine.lavire@univ-lyon1.fr
†These authors have contributed equally to this work