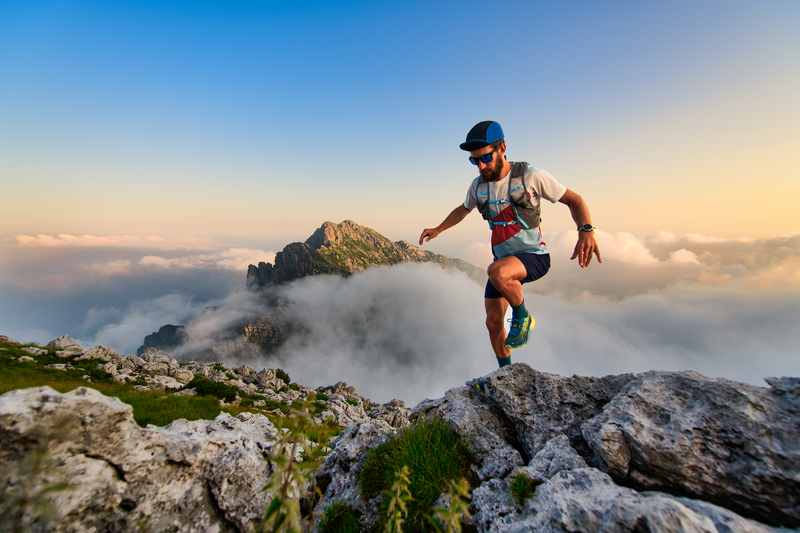
95% of researchers rate our articles as excellent or good
Learn more about the work of our research integrity team to safeguard the quality of each article we publish.
Find out more
REVIEW article
Front. Plant Sci. , 24 July 2019
Sec. Plant Nutrition
Volume 10 - 2019 | https://doi.org/10.3389/fpls.2019.00923
Soilless cultivation represent a valid opportunity for the agricultural production sector, especially in areas characterized by severe soil degradation and limited water availability. Furthermore, this agronomic practice embodies a favorable response toward an environment-friendly agriculture and a promising tool in the vision of a general challenge in terms of food security. This review aims therefore at unraveling limitations and opportunities of hydroponic solutions used in soilless cropping systems focusing on the plant mineral nutrition process. In particular, this review provides information (1) on the processes and mechanisms occurring in the hydroponic solutions that ensure an adequate nutrient concentration and thus an optimal nutrient acquisition without leading to nutritional disorders influencing ultimately also crop quality (e.g., solubilization/precipitation of nutrients/elements in the hydroponic solution, substrate specificity in the nutrient uptake process, nutrient competition/antagonism and interactions among nutrients); (2) on new emerging technologies that might improve the management of soilless cropping systems such as the use of nanoparticles and beneficial microorganism like plant growth-promoting rhizobacteria (PGPRs); (3) on tools (multi-element sensors and interpretation algorithms based on machine learning logics to analyze such data) that might be exploited in a smart agriculture approach to monitor the availability of nutrients/elements in the hydroponic solution and to modify its composition in realtime. These aspects are discussed considering what has been recently demonstrated at the scientific level and applied in the industrial context.
Considering that human world population will reach about 9 billion by the year 2050 (Tilman et al., 2002), it appears clear that food security is one of the pivotal themes of the new millennium and, reasonably, the most urgent challenge for the agricultural sector. However, it should be considered that the progressive drop of fertile soil surface, due to environmental pollution and urbanization phenomena (Chen, 2007), greatly complicates the context. In this regard, the intensification of the production cycles and the monoculture approach, which favored the diffusion of many pathogens and the development of the corresponding pathologies (Jones et al., 1991; Shafique et al., 2016; Tittarelli et al., 2016), should also be taken into account. Moreover, the strict dependency of agricultural practice on water availability (Verones et al., 2017) in an age of drastic climate changes (desertification) makes the scenario even more complex. In this respect, the possibility of exploiting surfaces not anymore fertile (due to pollution or pathogen problems) for agricultural purposes and also limiting at the same time the water consumption (see also Table 1; Pignata et al., 2017; Müller et al., 2017) makes the soilless system cultivation surely a valid opportunity. Moreover, it should be highlighted that this cultivation approach also represents a favorable response toward a more environmentally friendly agriculture (Benke and Tomkins, 2017; Maucieri et al., 2017; Tajudeen and Taiwo, 2018) as well as a promising tool also in the vision of the general challenge of food security.
Currently, about 3.5% of the worldwide area cultivated under tunnels and greenhouses for vegetables production adopts the soilless agriculture techniques based on hydroponic solution (such as floating systems, nutrient film technique (named also NFT) – or aeroponics, Hickman (2016)). This significant diffusion at the field scale undoubtedly highlights the presence of many advantages of this production approach in addition to the more efficient use of the nutritional resources including water (Kinoshita et al., 2016; Rodriguez-Ortega et al., 2017). In fact, there is a variety of examples where hydroponic solution can be efficiently used for biofortification programs with oligo elements (e.g., iodine (I), selenium (Se), silicon (Si), and calcium (Ca), see also Table 2; Schiavon et al., 2013; Tomasi et al., 2013, 2015a,b; Smoleń et al., 2016; D’Imperio et al., 2016a,b; Mimmo et al., 2017) as well as to improve vegetable quality and its shelf life according to the market and consumer needs (Giuffrida et al., 2014, 2017; Amalfitano et al., 2017; Giro and Ferrante, 2017; Yasuor et al., 2017; Islam et al., 2018). However, the extensive research activity aimed at fine-tuning fertilizers’/nutrients’ concentration in the hydroponic solutions, particularly (1) to restrain nitrate content in edible plant tissues (Fageria, 2010), (2) to guarantee vegetables’ safety, and (3) to improve the nutritional quality of the yields (Fallovo et al., 2009; Gruda, 2009; Putra and Yuliando, 2015), point out that there is still much room for improvement. This is particularly true in the light of the recent scientific pieces of evidence about the mechanisms underlying the mineral nutrition in plants and their regulation (competition/antagonism/interaction among nutrients) and about the bio-geochemical cycles of nutrients in the soil solution (solubilization/precipitation). This knowledge is fundamental when the development of different strategies (including approaches and tools such as beneficial microorganisms-PGPRs and nanoparticles) for the optimization of the hydroponic production of vegetables is pursued.
Table 2. Mineral biofortification in soilless vegetables species (research activities are represented by coloured cells).
In the industrial context, a new paradigm labeled as Industry 4.0 or Smart Manufacturing and based on cyber-physical manufacturing systems and Internet of Things is emerging (Kagermann et al., 2013). It is interesting to note that hydroponic production of vegetables carried out in limited and well-controlled environments is going to reduce the most relevant differences between agricultural and industrial processes and, in turn, to improve the quality control. However, to achieve this goal, a set of additional monitoring tools specifically for the hydroponic-based cultivation approach are necessary. In this regard, sensors for realtime monitoring of the hydroponic solutions composition (i.e., availability of nutrients/elements) as well as interpretation algorithms also based on machine learning logics to analyze such data play a pivotal role. In fact, only thanks to these tools, that can be borrowed from the industrial context, it might be possible to maintain/adapt in realtime the composition of a hydroponic solution in order to achieve products of a desired quality.
This review is aimed at analyzing the open questions of hydroponic systems and at highlighting opportunities in their applicative use in a field scale, considering also what has recently been demonstrated at the scientific level and applied in industrial context. In particular, from the scientific point of view, we would like to unravel the topics and problems that have been adequately studied and the ones that instead still require significant research efforts, all these pieces of information being essential for a better management of crop nutrient acquisition in soilless systems. Moreover, from the practical point of view, the potential to use new forms of nutrients and/or bioeffectors as well as new technologies to collect/analyze data could be a viable tool at the field scale for farmers in a context of a smart agriculture.
It is widely known that the productivity and quality of crops grown in hydroponic systems are markedly dependent on the extent of the plant nutrients acquisition from the growing medium (Valentinuzzi et al., 2015). It is interesting to highlight that this root physiological process is not only affected by the availability levels of the nutrients (i.e., by their soluble forms) in the medium (solubilization/precipitation: section “Chemical Management of Nutrient Availability in the Hydroponic Solution”), but also by the nutrient sources (nutrient chemical forms: section “Nutrient Chemical Forms and Uptake Processes”) and/or by the interactions among the different nutrients (e.g., competition/antagonism: section “Nutrient Interactions”).
When dealing with hydroponic cultures, solution chemistry is fundamental to ensure adequate nutrient concentrations for plant uptake. In particular, multiple chemical equilibria must be taken into account when preparing nutrient solutions using salts or concentrated liquid stocks, especially solubilization/precipitation equilibria (De Rijck and Schrevens, 1998b). In fact, a number of physical-chemical phenomena can alter the nutrient availability for plants, the most important of which are (1) precipitation, (2) co-precipitation, and (3) complexation. In this respect, it should be highlighted that the temperature of the nutrient solution, affecting the chemical equilibria in solution, may considerably influence these processes. This is particularly crucial for areas where the overwarming of the nutrient solution often occurs, impacting also at the physiological level of crops (Lee and Takakura, 1995 – spinach; Fazlil Ilahi et al., 2017 – butterhead lettuce).
Precipitation reactions may occur when cations and anions in aqueous solution combine to form an insoluble ionic solid (the precipitate). Such conditions, called saturation, occur when the concentrations of certain cations and anions in solution reach a maximum limit value (solubility). The concentrations of ions in equilibrium with the precipitate (i.e., solubility) can be calculated using a specific equilibrium constant called solubility product, which is tabulated for many chemical compounds and depends on temperature. Besides temperature, precipitation equilibria can be also influenced by other parameters such as pH and ionic strength (a parameter that considers the sum of the concentrations of all the ionic compounds in solution and their charge). Cations may form insoluble hydroxides at alkaline pH (by combining with OH− anions) or other insoluble precipitates by reacting with other anionic nutrients; thus, they must be carefully balanced and optimized to avoid losses from solution. In such cases, the values of pH and those of redox potential (Eh) must be continuously monitored or controlled. In this regard, pH values above 7 and positive Eh values may cause the precipitation of nutrients like iron (Fe), zinc (Zn), copper (Cu), nickel (Ni), and manganese (MnII) as insoluble (hydr)oxides. Precipitation of FeIII may occur already at pH well below the neutrality (Takeno, 2005). At negative Eh values and acidic pH, e.g., in uncontrolled hydroponic systems under anoxic conditions, the same elements might also precipitate as insoluble sulfides, when sulfate is reduced to sulfide. At high pH values and high dissolved CO2 concentrations, macronutrients like Ca and magnesium (Mg) can precipitate as carbonates. Precipitation of phosphates (mostly hydrogen phosphates) is another process to avoid in hydroponic solutions. This process, besides depleting phosphorus (P) from nutrient solution, may also reduce the solubility of other nutrients such as Ca, Mg, Fe, and MnII. It is known that phosphate availability can be reduced at pH above 7 mostly due to precipitation with Ca. Different Ca-phosphate minerals can potentially form above this pH such as hydroxylapatite [Ca5(PO4)3OH], amorphous tricalcium phosphate [Ca3(PO4)2], and Ca4H(PO4)3·3H2O (Lee et al., 2017). Also sulfur (S) availability can be limited by precipitation with Ca, as Ca-sulfate minerals (Packter, 1974). Silicon solubility is usually reduced at acidic pH, where SiO2 precipitates may be produced (Takeno, 2005). Precipitation/dissolution phenomena are often promoted by pH changes and therefore pH must be continuously controlled or buffered. Addition of nutrients in the form of salts to hydroponic solutions may lead to hydrolysis reactions, which may result in the acidification or alkalinization of the medium. Nitrogen (N) supply may also alter solution pH, if N is added only in the form of NO3 − (alkalinization) or NH4 + (acidification) (Asher and Edwards, 1983). Yet, both N forms are usually added to hydroponic solutions. In general, saturation conditions for a certain nutrient could be reached if its concentration is increased due to water evaporation from the hydroponic system (owing to high temperatures or plant evapotranspiration). However, it has been recently observed that water losses by 20% (or even more) do not significantly influence precipitation equilibria (Tomasi et al., 2015a).
Strictly connected with precipitation are co-precipitation phenomena. These latter refer to the entrapment of an element (usually trace metals) within the structure of an insoluble compound (normally not containing that element) during its precipitation from solution. Co-precipitation may strongly reduce the solubility of nutrients added at trace concentrations like Cu, Zn, MnII, and Ni, when insoluble species like Fe (hydr)oxides, Ca-carbonates, or Ca-phosphates are formed (McBride, 1994). Co-precipitation is a process capable of strongly reducing the solubility of an element, well below that of the least soluble pure mineral phases of the element likely to form under environmental conditions (Martínez and McBride, 2000).
Another important process to consider in hydroponic solutions is complexation, i.e., the formation of a chemical compound where a metal nutrient is bound by one or more neutral molecules or anions (ligands), either of organic or inorganic nature. The resulting complex can be a neutral compound, a cation, or an anion, depending on whether positive or negative charges prevail. Complexation reactions diminish the concentration of the free ions in the nutrient solution, changing elemental bioavailability. In general, the formation of complexes increases metal solubility even if very often, for some nutrients, they are less available for plant uptake than their free ions (De Rijck and Schrevens, 1998a). The addition, organic ligands such as EDTA, DTPA, EDDHA, citrate, etc. can increase the stability of certain elements in solution, especially Fe, Cu, Zn (Lucena, 2003). However, as discussed later in this review, the different complexes may differently affect plant nutrient uptake and allocation (e.g., the case of Fe acquisition). In addition, some forms of soluble hydrogen carbonates and phosphates, as well as chlorides, can reduce the concentration of actual free metal cations in solution through complexation. The stability of complexes is another parameter that must be taken into account when preparing a nutrient solution: while on one side, efficient complexing agents can facilitate the solubilization of a nutrient in water, on the other side, strong complexes are usually more difficultly usable by plants (Kraemer et al., 2006).
Considering all these aspects, it seems thus likely that the composition of a real hydroponic solution could reasonably be different from the planned one; being an altered availability of the nutrients in the growing medium a known risk for the quality of the vegetables (Tomasi et al., 2015a), the availability of tools for a realtime analysis of the nutrient solution composition is undoubtedly of particular usefulness and interest.
For a more accurate calculation of chemical equilibria in solution, ion activity should be considered instead of concentration. In fact, ion concentrations can be used only in calculations regarding ideal solutions, i.e., diluted solutions where no interactions among solutes occur. In real solutions, like in hydroponic solutions, interactions among solutes cannot be neglected and therefore ion activity should be used in calculations instead of concentrations. An ion activity is determined by multiplying the concentration of that ion in solution for an activity coefficient (≤1), which depends also on the concentration of all other ions in solution and their charge, i.e., on the ionic strength of the solution. Ion active concentration diminishes as the concentration of electrolytes in solution increases. For highly diluted (ideal) solutions, the activity coefficient for the chemical species in solution can be approximated to 1, and therefore ion concentrations are not influenced by phenomena such as ion-pair formation or ion conductivity reduction. But, when dealing with more concentrated solutions of strong electrolytes, like in hydroponic solutions, these phenomena become relevant and may reduce the ion active concentration. For example, for a sole KNO3 solution at 6 mM concentration (as normally present in Hoagland nutrient solution), the activity coefficient for KNO3 is about 0.92 and therefore its active concentration is 8% lower than the concentration actually added to solution. However, the activity coefficient of a single electrolyte depends on the ion strength of the whole solution and decreases with increasing concentration of all the electrolytes dissolved and of their charge. Usually, the concentrations of electrolytes in nutrient solutions for soilless cultivation are quite high, in the range 6.4–37.8 meq L−1 (corresponding to EC in the range 0.8–4.0 dS m−1) (Savvas, 2003), and therefore the ion activity can be significantly reduced (e.g., the activity coefficient for KNO3 is about 0.85, with an overall reduction of 15% active concentration).
All the abovementioned processes can be simulated and foreseen by using dedicated software to manage chemical equilibria calculations. Among these, Visual MINTEQ (Gustafsson, 2013), MINEQL+ (Environmental Research Software, USA), CHEMEQL (Müller, 2015), CHEAQS (Verweij, 2017) are some of the most used both in water and soil chemistry. All the mathematical models employed by these software tools are based on thermodynamic data and usually kinetic parameters are not taken into account. However, it is known that kinetic constraints can prevent a process from occurring or limit its representativeness (Terzano et al., 2015). For instance, with respect to the precipitation processes, oversaturation or under-saturation phenomena can change nutrient solubility, respectively, above or below the concentration allowed by thermodynamics.
In a context of a more sustainable agriculture, a specific comment must be dedicated to the exhausted hydroponic solutions at the end of a productive cycle. In this regard, it is clear that they represent an interesting resource in terms of water and fertilizer savings, which is becoming increasingly relevant especially in those countries where there is shortage of rain or good-quality water and farmers cannot afford the expenses to buy large amounts of fertilizers. Moreover, recycling exhausted solutions may also represent an efficient strategy to prevent groundwater and environmental pollution, especially from intensive agricultural productions. However, the main problem with the reuse of exhausted nutrient solutions is the shortage of some key macro and micronutrients (da Silva Cuba Carvalho et al., 2018) and their increased salinity (Grattan and Grieve, 1998; Pardossi et al., 2005; Bar-Yosef, 2008) causing, in turn, problems for crops (Carmassi et al., 2005; Parida and Das, 2005) even if to different extents from one plant species to another (Bar-Yosef, 2008). Thus, a research challenge in this context is surely to develop management practices/tools that reduce salinity in recycled solutions and/or minimize the physiological impact of salinity on plants (Neocleous et al., 2017). In addition, considering the composition of a typical Hoagland solution and according to chemical equilibrium models (Visual Minteq 2.61), at pH 5.5, according to thermodynamic data, few other nutrients could easily reach oversaturating conditions if certain ions accumulate in exhaust Hoagland solutions, mostly phosphates (precipitating as Ca, Mg, or Fe phosphates) and molybdate (as Ca molybdate). Also, this aspect needs a careful monitoring when a reuse of the hydroponic solution for another cycle is planned.
Salinity increase could be contrasted by treating the recycled water with appropriate osmotic systems, including forward and reverse osmosis. In the last years, particular attention has been paid to forward osmosis (FO) technologies, also with the purpose to reuse wastewater for fertigation purposes (Van der Bruggen and Luis, 2015). In this sense, Phuntsho et al. (2012) proposed the use of concentrated fertilizer solutions as draw solutions in FO systems. This application maybe of interest for hydroponic cultures particularly when the feed water is of a low quality, like an exhausted nutrient solution or wastewater. The concentrated fertilizer solution is used to withdraw pure water from this source (see the scheme in Figure 1). In this way, water is recycled and is also enriched in those nutrients that have been lost by plant uptake or other chemical processes (e.g., precipitation, complexation, sorption). This technology, called fertilizer drawn forward osmosis (FDFO), has been recently applied to grow hydroponic lettuce using a commercial nutrient solution (Chekli et al., 2017). In a pilot-scale study, these authors demonstrated that the FDFO process is able to produce the required nutrient concentration and final water quality (i.e., pH and conductivity) suitable for hydroponic applications and the hydroponic lettuce showed similar growth patterns as the control without any sign of nutrient deficiency.
Figure 1. Scheme of a fertilizer drawn forward osmosis desalination process for nutrient solution preparation to be used in hydroponic cultivation systems (modified from Phuntsho et al., 2012).
Another relevant issue when dealing with closed-loop hydroponic cropping systems is the accumulation of potentially toxic organic compounds released by the roots of cultivated plants. This phenomenon is known as allelopathy/autotoxicity (Kitazawa et al., 2005; Asaduzzaman and Asao, 2012) and occurs when a plant species releases chemical substances which inhibit or delay germination and/or growth of the same plant species (Singh et al., 1999; Mondal et al., 2015). Among these substances, benzoic acids were mainly found to inhibit the growth and yield of several crops (Hosseinzadeh et al., 2019). To overcome this issue, a number of treatment techniques have been proposed for root exudates degradation or removal, either physical (e.g., activated carbon adsorption, ion exchange resins, membrane filtration, sand filtration); chemical (e.g., TiO2 photocatalysis, UV treatments, ozonation, electrodegradation); or biological (e.g., use of degrading microbial strains) (Asaduzzaman et al., 2012; Mondal et al., 2013; Hosseinzadeh et al., 2017; Talukder et al., 2018, 2019). All these treatments may have advantages and drawbacks as reviewed by Hosseinzadeh et al. (2017). In general, to be effective, the treatment technique applied should be able to remove root exudates without interfering with the inorganic mineral nutrients in solution. One of the main problems in this sense is the degradation/adsorption of Fe chelates (Ehret et al., 2001).
With respect to the chemical forms of a nutrient, N represents one of the most explicative examples. In fact, it is well demonstrated that plants can use a wide variety of N forms, ranging from the inorganic, namely NH4 + and NO3 −, to the organic ones, as for instance urea and amino acids (Nacry et al., 2013). Due to environmental conditions and plant species, the relative contribution of each N form to plant N supply can be quite variable (Haynes and Goh, 1978; Schimel and Chapin, 1996; Marschner, 2012). From a metabolic point of view, the assimilation of NH4 + is less expensive as compared to NO3 −, NH4 + being directly usable to generate glutamine (Marschner, 2012). However, a pure NH4 + nutrition has been shown to cause the development of toxicity symptoms in many herbaceous plants, as well as to inhibit NO3 − uptake (Kronzucker et al., 1999). Therefore, a balanced N diet (NO3 − and NH4 +) is clearly beneficial for several plant species as compared to that based exclusively on NO3 − (Forde and Clarkson, 1999). Accordingly, it has been observed in tomato plants that the root growth was optimal when NO3 − and NH4 + were supplied in a 3:1 ratio; on the contrary, when NH4 + concentration was too high, a strong inhibition in the root development was observed (Bloom et al., 1993). A more recent study in watermelon plants showed that with decreasing NO3 −/NH4 + ratio, the leaf number, leaf area, shoot height, net photosynthesis, biomass, and root growth significantly decreased, as well as the concentration of several macronutrients (Na et al., 2014). Nevertheless, it has also been observed that the optimal NO3 −/ NH4 + ratio to be applied for cultivation can be dependent on both the plant species and the environmental conditions (e.g., salinity) (Errebhi and Wilcox, 1990). For instance, in canola plants grown in salinity conditions, a NO3 −/NH4 + ratio of 1:1 is suitable to minimize the negative effects produced by the abiotic stress, thus maximizing the yield and quality (Bybordi et al., 2012). Overall, these results clearly indicate that not only the forms of N but also the concurrently presence of more than one form and in specific ratios among these forms are decisive for specific productive objectives.
Also in the case of Fe nutrition, the nature/forms of the Fe sources utilized can play an important role not only at the level of its uptake but also in the Fe allocation within the plant. In natural environments (soil grown cultures), Fe is present in inorganic mineral forms (e.g., goethite, hematite, and ferrihydrite), which are poorly bioavailable (Colombo et al., 2013; Mimmo et al., 2014). Therefore, plants need to rely on a series of natural ligands for the mobilization of the micronutrient from the insoluble sources and to guarantee appropriate levels of Fe availability (Cesco et al., 2000; Tomasi et al., 2013). However, often this pool of Fe sources cannot satisfactorily sustain an equilibrate growth of plants triggering the onset of Fe deficiency symptoms. The Fe supply (in terms of quantities and usable forms) is an issue also for soilless crops. Several pieces of research have compared in hydroponic solutions the efficiency of different organic ligands complexed with Fe as micronutrient source for plants (Pinton et al., 1999; Tomasi et al., 2009, 2013, 2014). These reports clearly demonstrated that Fe complexed by water-extractable humic substance (WEHS) fractions can be used with higher efficiency than other natural or synthetic sources (Pinton et al., 1999; Tomasi et al., 2009; Zanin et al., 2019), favoring a faster recovery from the deficiency symptoms (Tomasi et al., 2014). The effect appears to be not exclusively ascribable to phenomena occurring at the root level (Tomasi et al., 2013; Zamboni et al., 2016), but also to those happening in the shoot/leaves (Tomasi et al., 2009, 2014). Therefore, from these experimental evidences, it is clear that different Fe sources can be used with different efficiencies, which are the result of a complex response, including biochemical-physiological-molecular aspects, involving the whole plant. However, despite all this knowledge, little still exists in the literature in relation to the application of these findings in the soilless cultures at the production scale. This aspect is even more critical if the increasing demand for Fe-enriched vegetable products is considered.
When nutrient ions are analyzed in hydroponic solutions, one aspect to consider is also the nature of the counter-ion forming the ion pair in solution. In fact, it is well demonstrated that anion which is taken up relatively slowly can also reduce the uptake speed of its counter-ion, as observed for SO4 2− on potassium (K+) uptake (Marschner, 2012). Also, the root capability to extrude protons in the external medium, generating the electrochemical gradient across the plasma membrane at the base of the active nutrient uptake, is consistently influenced by this phenomenon (Pinton et al., 1997). With respect to the hydroponic solutions of soilless cultures, the simultaneous presence of different cations and anions (also at different concentrations) unfortunately makes the phenomenon even more complex and difficult to understand and to manage. However, although the impact of this phenomenon on the overall process of radical nutrient acquisition has long been clear, up to now, there are no specific studies applied to the hydroponic system.
It is well known that, at the level of the nutrient acquisition mechanisms of roots, competitive or antagonistic phenomena among elements can occur (Marschner, 2012), leading, in some contexts, also to relevant nutritional disorders affecting the whole plant. A clear example is represented by the interaction between NH4 + and K+. The acquisition of NH4 + involves an active transport which is very specific for the cation (von Wirén and Merrick, 2004). Differently, K+ acquisition is described as a biphasic transmembrane transport of high and low affinity transporters (Gupta et al., 2008; Zhang et al., 2012; Shin, 2014). However, the selectivity of these K+ transporters is definitely lower than that of others, including the NH4 + ones (Gierth and Mäser, 2007). In this regard, it is interesting to note that an inhibition of K+ uptake by NH4 + has been already described, whereas the vice versa does not happen (Mengel et al., 1976; Rufty et al., 1982; Shaviv et al., 1987). This phenomenon has been ascribed to the capability of K+ transporters to catalyze also the transmembrane transport also of NH4 + (ten Hoopen et al., 2010). Certainly, the similarity of the two cations (NH4 + and K+) in terms of valence and ion diameter is, at least in part, at the base of the phenomenon. From the agronomical point of view, this aspect could be crucial for NH4 +-fed plants when exposed to a sub-optimal/unbalanced availability of K+ because the competition could induce/exacerbate K+ deficiency (Zhang et al., 2010). This aspect could be of particular relevance when the additional application of NH4 + is of pivotal role to achieve specific qualitative objectives of the edible fruits (Valentinuzzi et al., 2018).
It is also worth mentioning that the interactions K+/Na+ and Cl−/NO3 − could represent a limiting factor for soilless cultivation of crop plants, especially in semiarid environment characterized by saline water. It is well known that NaCl interferes with the uptake processes of both K+ and NO3 −, since K+ is sensitive to sodium (Na+) in the external environment, while the uptake of NO3 − is inhibited by chloride (Cl−) (Silberbush and Ben-Asher, 1989). This phenomenon could be even more pronounced in hydroponic solutions particularly when used for more than one cycle (closed system) (i.e., exhausted hydroponic solutions).
A further example of competitive interaction between nutrients is that occurring among Ca2+, Mg2+, and K+ (Marschner, 2012), although observed up to now only in soil-grown crops. It is well known that a high availability of K+ and Ca2+, most often after an unbalanced fertilization practice, can induce Mg2+ deficiency in crop plants. At the moment, the mechanisms underlying such interactions have not been clarified. However, as suggested by Schimansky (1981), the excessive availability of the other two cations (K+ and Ca2+) could reasonably inhibit Mg2+ uptake by roots. In this context, and in particular with respect to hydroponic cultures, monitoring the ratio between these three cations in the solutions is without any doubt advisable in order to avoid K+/Ca2+-induced Mg2+ deficiency, particularly when closed systems are considered.
Also Fe acquisition mechanism offers the possibility to describe several examples of interactions among elements/nutrients. In dicots and non-graminaceous monocots, Fe is taken up through the IRT1 transporter (Connolly et al., 2003), which was shown to transport also other divalent cations such as Mn2+, Zn2+, and Cu2+ (Korshunova et al., 1999). In sub-optimal availability of Fe (Zamboni et al., 2016), a marked accumulation of these elements in plant tissue has been observed, with also a different spatial allocation of each element (Tomasi et al., 2014). On the other hand, a rather worrying aspect affecting the quality of the edible plant tissue is that the IRT1 transporter can also mediate the uptake of toxic elements like cadmium (Cd) (Astolfi et al., 2012, 2014; He et al., 2017). This last evidence clearly highlights how crucial is the quality of the nutrient sources adopted as well as that of the water used to prepare the hydroponic solution (in terms of trace elements), to avoid unexpected contamination of crops, the worsening of the edible parts’ quality, and threatening the food safety (Zhao et al., 2010).
Another interesting example of nutrient interaction has been described by Prosser et al. (2001) using S-starved spinach plants (Prosser et al., 2001). In this experience, the authors recorded as a consequence of S starvation a consistent accumulation of NO3 − in leaves of plants, one of the greatest issues concerning hydroponic productions (Santamaria, 2006). It is interesting to note that this phenomenon seems to be not specific for S, since it has also been observed for Fe-starved cucumber plants (Nikolic et al., 2007). In both these cases, although the uptake of NO3 − was hampered by the two nutrient shortages (S or Fe), the effect on the assimilation process seems to play a dominant role in determining the NO3 − accumulation at the leaf level. However, such nutrient interactions (S vs. N and Fe vs. N) seem to be pretty complex and not so easy to simply explain in terms of competition/antagonism phenomena for their transmembrane transporters. In fact, it has also been demonstrated that Fe uptake and the mechanism underlying the process (i.e., the Fe3+ reduction step mediated by the plasma membrane reductase FRO) are strongly influenced by the availability of N (Nikolic et al., 2007). Interestingly, Zuchi et al. (2009) have demonstrated that the same Fe acquisition mechanism is severely affected also by S availability. Although the overall impact in terms of nutrient acquisition appears to be essentially the same, the components of the transport machinery seem to be differentially affected by the starvation of the two nutrients; in fact, while FRO is inhibited by both N and S starvation, the transport of Fe2+ through IRT1 seems to be hindered only by limited availability of S. These data highlight that the uptake of each single element (e.g., N, S, or Fe) is not exclusively dependent on its availability in the hydroponic solution but also on the presence (and availability levels) of other elements. Thus, it appears evident that an optimized and well-balanced supply of nutrients is a prerequisite for an efficient use of the resources by hydroponically grown vegetables, not only to ensure a high yield but also to guarantee the quality of the edible tissues. Interestingly, it has been demonstrated that a higher Fe accumulation in plant tissues (Fe fortification) can be reached by means of a sulfate over-fertilization (Zuchi et al., 2012).
In the context of nutrient interactions, it is interesting to highlight that these phenomena have been observed also in the case of biofortification programs like with Se. Generally, the biofortification of vegetables is obtained by the application of Se, in the form of selenate, in the nutrient solution, this form being the most available for plants (Schiavon and Pilon-Smits, 2017). Due to the similarities in the chemical and physical features (Shibagaki et al., 2002; El Kassis et al., 2007), the acquisition of selenate follows the same pathway exploited by SO4 2−, based on the sulfate transporters SULTR (Malagoli et al., 2015). For this reason, it is clear that, competing for the same transporter, a sort of antagonism between sulfate and selenate can occur. As demonstrated by (Hopper and Parker, 1999), a high availability of sulfate might prevent the acquisition of selenate, thus vanishing the potential biofortification of the edible parts.
Differently, the experiences aimed at Si biofortification in agricultural crops, both ready to eat and to be transformed (Gottardi et al., 2012; Montesano et al., 2016; D’Imperio et al., 2016a), did not reveal interaction phenomena with other nutrients, at least with negative outcome on the plants’ whole nutrient balance. Several authors have reported a mitigation effect of Si in P-starved crop plants in soilless cultivation systems (Ma and Takahashi, 1989; Ma, 2004); similarly, it has been observed that the supplementation of Si in the nutrient solution of Fe-deficient dicots can alleviate Fe chlorosis (Gonzalo et al., 2013; Pavlovic et al., 2013). Indeed, Gottardi et al. (2012) showed that the Fe reduction and uptake rate at root level were up-regulated by Si supplementation, although Fe translocation to leaves was not influenced. On the other hand, the effect of Si on Fe uptake/allocation in dicots is still debated (Liang et al., 2015). In addition, the Si fertilization might not be only important for Si biofortification approaches, but also to improve plant fitness. The application of silicate fertilizers to paddy fields worldwide has resulted in an increased yield in rice production, with a higher photosynthesis rate and a better light interception due to an improved leaf blade position (Tamai and Ma, 2008). These last aspects, even if observed in soil-grown crops, should also be taken into consideration for soilless cultures for the undoubted advantages that they could guarantee.
The issues described earlier clearly highlight how unexpected physicochemical phenomena happening in the hydroponic solution can easily modify its composition as well as a series of nutrient interactions can seriously alter the efficiency of the nutrient acquisition process of crops. These phenomena considered together are able, unfortunately, to considerably affect the production of the soilless system based on hydroponics, both from a quantitative and qualitative point of view. For this reason, the availability of new forms of nutrients (nanoparticles, section “Nanoparticles”) and/or of bioeffectors able to enhance the functionality of the root nutrient acquisition mechanisms (PGPRs, section “Use of Plant Growth-Promoting Rhizobacteria in Hydroponic Solutions”) may be of particular relevance. Similarly, the possibility of exploiting tools to monitor the composition of a nutrient solution in realtime (sensors, section “Realtime Monitoring of Hydroponic Solutions via Sensors”) and to analyze the data (interpretation algorithms, section “Interpretation Algorithms and Smart Agriculture”) for a prompt correction may clearly facilitate a more efficient use of the hydroponic solutions.
In the agricultural context, the use of nanoparticles (NPs) is mainly aimed at reducing nutrient losses in the environment as well as at increasing yields through an optimal management of nutrients and water. In fact, thanks to their high specific surface and relevant reactivity (having a particle size lower than 100 nm), nanoparticles might supply the plant with more soluble and available forms of nutrients limiting precipitation and insolubilization processes often described for several fertilizers (e.g., phosphate ones) (Liu and Lal, 2015). For this reason, in comparison to the traditional fertilizers, nanoparticles are considered much more efficient carriers of nutrients for plants (Nair et al., 2010; Campos et al., 2014; Roosta et al., 2017). These advantageous aspects are valid not only for the soil system but even more for the soilless systems (considering also what described for chemical equilibria in hydroponic solution, section “Chemical Management of Nutrient Availability in the Hydroponic Solution”). Therefore, nanoparticles surely represent a promising tool in general, specifically for the soilless growing systems. The use of nanoparticles in soilless cultivation has also been evaluated in the ability to control potential pathogens during cultivation through the use of NP of silver (Ag), Cu, Si, titanium (Ti), and Zn improving the plant defense (Amooaghaie, 2011). Elmer and White (2016) tested the use of metallic oxide nanoparticle to enhance tomatoes’ and eggplants’ growth. Their results showed that NP of CuO increased fresh weights by 64%, reducing the Verticillium wilt fungus by 69%, having 32% more Cu in the roots. Within metallic NP, growth enhancement was recorded also for hydroponic spinach treated with iron oxide (Fe2O3) NP (Jeyasubramanian et al., 2016). The mechanism of Fe uptake by the spinach plant from the Fe2O3 NP can be explained as follows: in general, the uptake of Fe3+ is found to be pH sensitive and naturally Fe3+ is insoluble which will be slowly converted into Fe2+ under acidic environment. This determined an increase in root and shoot length, biomass, and Fe content in a dose-dependent manner. NP can also be used to recover waste nutrient solution from soilless cultivation systems. An example is given by the photocatalytic treatment of waste nutrient solution coming from tomato cultivation in rice hull substrate (Miyama et al., 2009). In this experiment, the substrate was treated with TiO2-coated porous alumina filter, that when irradiated with ultraviolet light, exhibits a strong oxidation effect, decomposing organic compounds. By this process, the phytotoxic compounds could be decomposed and detoxified and the nutrient solution recycled. Moreover, it was observed that tomato growth in the photocatalytically treated system was significantly higher than control in six experiments over 3 years and yields were comparable to those in a currently used open cultivation system using stonewool substrate. In addition to these interesting aspects, it has been clearly ascertained that nanoparticles are able also to affect key processes of plants including germination, seedling vigor, root growth, photosynthesis, and even flowering (Lin and Xing, 2007; Tripathi et al., 2016). Moreover, a protective action of nanoparticles against oxidative stress in plants has been recently demonstrated; the phenomenon was ascribed to the capability of these particles to mimic the role played by antioxidant enzymes (e.g., superoxide dismutase, catalase, and peroxidase) (Burman et al., 2013). Khan et al., (2017) have shown that other abiotic stresses (such as temperature, salinity, drought stress) can be alleviated by nanoparticles as well as drought-stress resistance in plants can be enhanced by applying nanoparticles (Tripathi et al., 2017). In particular, the application of NPs of analcite (Zaimenko et al., 2014) significantly alleviated the drought stress in wheat and corn by increasing the photosynthetic pigments and the accumulation of protective antioxidants in these plant species. Recently, nanomaterials are used as an important tool for increasing the growth and yield of crops under salinity condition (Khan et al., 2017). The application of nano-Si for example significantly reduced salt stress, increasing seed germination and antioxidative enzyme activity, photosynthetic rate, and leaf water content (Haghighi and Pessarakli, 2013; Qados, 2015). In addition, these mitigating effects have been also noticed in other plant species such Ocimum basilicum (Kalteh et al., 2018) Cucurbita pepo (Siddiqui et al., 2014), and Vicia faba (Qados and Moftah, 2014).
With respect to temperature stress, this can either be due to too high or too low temperature. Loss of fluidity of membranes and leakage of solutes are typical symptoms of cold stress. In this regard, nanomaterials such as TiO2 may help in alleviating the dangerous effects of cold stress by limiting the membrane damages and electrolyte leakage (Mohammadi et al., 2013). On the other hand, heat stress accelerates the overproduction of reactive oxygen species and increases oxidative stress leading to disintegration of membrane lipids, leakage of electrolytes, and denaturation of biomolecules (Karuppanapandian et al., 2011). It has been shown that, among nanomaterials, low concentration of selenium (Se) can alleviate the effects of heat stress for its antioxidative properties (Haghighi et al., 2014).
However, despite these pieces of evidence, there are still several open questions about the molecular mechanisms underlying the aforementioned phenomena. In addition, the phytotoxic effects of nanoparticles (presumably via an enhanced production of reactive oxygen species) described by Khan et al. (2017) make the issue even more complex and less clear. Moreover, little is yet known about their capacity to enter, through the edible tissues, in the food chain and, even more critical, about what effects they may have on human health and the environment. For these reasons, right now, the exploitation at the field scale of these particles appears to be quite premature; in fact, a cautionary principle reasonably prevails. In summary, despite nanoparticles representing a promising tool, further studies are needed to evaluate the impacts on crop growth, quality, and safety (Gardea-Torresdey et al., 2014) before their massive use in the agricultural production system.
As widely addressed in literature, the molecular machinery used by plants for the acquisition of mineral elements is characterized by an extreme plasticity in order to guarantee the adaptability to the nutrient fluctuations in the growth medium. Such variations, particularly in the bioavailable nutrient fraction, can be ascribed to several factors, as for instance the concentration and type of nutrient source, the pH, and the redox potential (Tomasi et al., 2009; Marschner, 2012; Mimmo et al., 2014). In addition, a recent body of evidence has drawn the attention to the role played by the plant growth-promoting rhizobacteria (PGPRs) in contributing to the mineral nutrition of plants (Pii et al., 2015). The different mechanisms brought about by PGPRs aimed at increasing the bioavailability of mineral nutrients in the rhizosphere (i.e., atmospheric N2 fixation, P solubilization, siderophores production for Fe3+ chelation) have been extensively investigated and reviewed (Lugtenberg and Kamilova, 2009; Glick, 2012; Pii et al., 2015, 2019; Alegria Terrazas et al., 2016). Nevertheless, recent pieces of research have highlighted that the PGPRs can themselves induce alteration in the functionality of the molecular machinery devoted to nutrient acquisition (Pii et al., 2015). In this sense, PGPRs were shown to alter the release of protons from wheat roots and other model plants, thus supporting the hypothesis that they could have a direct effect on plasma membrane (PM) H+-ATPases (Bashan et al., 1989; Bashan, 1990; Bertrand et al., 2000; Canellas et al., 2002, 2013). Considering that the H+ electrochemical gradient at level of the PM is necessary for the absorption of several mineral nutrients, like H2PO4 −, SO4 2−, and NO3 − (White, 2003), the increased PGPR-induced H+ release could indeed be reflected in a higher ability of plants to take up nutrients. Similarly, the bacteria Achromobacter were shown to induce an increase in the concentration of NO3 − in plant tissues, most likely for its action on the constitutive high-affinity transport system (cHATS) for NO3 − (Bertrand et al., 2000; Nacry et al., 2013). Furthermore, the influence of PGPRs was also demonstrated on the molecular mechanisms underpinning the acquisition of Fe (Fe3+ reduction – FRO, Fe2+ transport – IRT1, and rhizosphere acidification – PM H+-ATPase) in dicots plants. The fungus Trichoderma asperellum could stimulate Fe uptake in Fe-sufficient cucumber and Lupinus albus by enhancing the activity of the root Fe-chelate reductase (de Santiago et al., 2013; Zhao et al., 2014), while Bacillus subtilis GB03 could induce the expression of genes coding Fe-chelate reductase and the PM H+-ATPase in Arabidopsis thaliana (Zhang et al., 2009). Recently, Pii et al. (2016) demonstrated that the PGPR Azospirillum brasilense can affect the Fe acquisition machinery in cucumber plants independently from the Fe nutritional status, thus suggesting that the different actors (Fe-chelate reductase, Fe2+ transporter, PM H + -ATPase) of the mechanism underlying Fe acquisition in roots can undergo a different regulation following PGPR inoculation. The majority of these studies have been carried out in soil conditions, while very little is known about the real performance of PGPRs in hydroponic systems, where their actions also depend on the ability of thriving and proliferating in specific environments, as well as, on colonizing the plant roots (Lee and Lee, 2015). However, several bacterial strains have been already successfully tested in hydroponically grown fruits and vegetables, obtaining positive effects on the yield and the quality of the agricultural products. The PGPRs belonging to Bacillus spp. have been tested for their effect on the growth and productivity of both tomato and pepper plants cultivated in hydroponic conditions (García et al., 2004; Gül et al., 2008). In particular, B. licheniformis was shown to significantly enhance the height of plants and the leaf area in both pepper and tomato plants, the effect being species-dependent (García et al., 2004). Nevertheless, the inoculation induced an increase in the number and in the diameter of tomato fruits (García et al., 2004). Similarly, the inoculation of tomato plants with the commercially available PGPR B. amyloliquefaciens (FZB24 and FZB42) resulted in an increase in the fruit yield of about 8–9% (Gül et al., 2008). Further studies have demonstrated that the application of specific PGPRs, such as B. sphaericus UPMB10 and A. brasilense Sp7, which are able to carry out the biological nitrogen (N2) fixation, might contribute to reducing the external input of nitrogen sources in the hydroponic solution used for the soilless cultivation of banana plants, still guaranteeing an adequate plant production (Baset Mia et al., 2010). Interestingly, A. brasilense was also demonstrated to increase the size of strawberries delivered by inoculated plants, to enhance the nutraceutical qualities (i.e., flavonoids, flavonols, and micronutrients concentration) as well as the sweetness index of fruits (Pii et al., 2018). Nonetheless, hydroponically grown plants in indoor systems might be threatened by pathogen attack; therefore, disinfection practices, either physical (e.g., ultraviolet light, gamma radiations) or chemical (e.g., the use of using carbendazim, hymexazol, imidazole, prochloraz triazole), could be required (Lee and Lee, 2015). Indeed, these control methods can result also in a decrease of PGPR population in the hydroponic systems, thus representing a limitation to the application of beneficial microorganisms to the hydroponic cultivation systems (Hibar et al., 2006). On the other hand, the use of PGPRs that feature also biocontrol traits might represent a valuable alternative to the abovementioned disinfection procedures.
Overall, these observations prefigure a very interesting scenario, in which the potential application of beneficial microorganisms (PGPRs) in the hydroponic cultivation of plants could lead to a further improvement in the productivity and in the nutraceutical properties of crops (Lee and Lee, 2015). Indeed, this practice would also impact on the sustainability of the agricultural systems, allowing a rationalization in the use of water resources and in the external inputs of agrochemicals (e.g., fertilizers and pesticides).
The hydroponic cultivation of small or medium-size fruiting crops, nowadays gaining ever more importance for the high productivity per area of cultivation (Bradley and Marulanda, 2001), often is based on closed-loop systems on field scale. In this case, the hydroponic solutions are often utilized for more than one single culture cycle; therefore, they need to feature high concentrations of mineral elements in order to guarantee an adequate nutrient supply for plants’ growth in the repeated cycles (Tomasi et al., 2015a). However, the selective removal of nutrients due to plant growth, as well as the evapotranspiration process, could in any case change the concentration of nutrients in the hydroponic solution as well as the accumulation of undesired counter-ions (i.e., Na+, Cl−), and have also an impact on the electrical conductivity (EC) of the solution itself (Wild et al., 1987; Zekki et al., 2019). Since such alterations in the qualitative and quantitative composition of the substrate solution can adversely impact on crop yield and quality, the need of timely tuning the nutrient solutions is of paramount importance to guarantee an adequate production (Cho et al., 2017). A possible tool for the on-line monitoring of hydroponic solutions can be represented by the use of ion-selective electrodes (ISEs) that are also applied for the assessment of drinking water quality (Melzer et al., 2016). Several authors have already applied this technology to measure the multiple components of a nutrient solution in order to ensure the optimal composition required for plant growth (Chen et al., 2011; Cho et al., 2017). However, at present, many studies have been carried out by measuring one or few plant macronutrients at a time (Bailey et al., 1988; Bamsey et al., 2012; Kim et al., 2013; Rius-Ruiz et al., 2014; Vardar et al., 2015), and this prevented obtaining a complete and realistic picture of the elements’ availability in the nutrient solution. In addition, to the best of our knowledge, selective electrodes for the determination of plant micronutrients, as for instance Fe, Cu, and Zn, have not been developed and/or applied yet. In addition, the use of ISEs as on-line monitor systems may present issues related to the signal drift and the reduction in sensitivity over time, due to a continuous exposure to the nutrient solutions, without appropriate calibration procedures (Gutiérrez et al., 2007, 2008; Bamsey et al., 2012; Kim et al., 2013). The development of a new generation of ISEs through the overcoming of the aforementioned technical limitations (i.e., simultaneous macro and microelements measurements, longer signal stability) might represent a useful tool for the on-line and realtime monitoring of nutrient solutions, with the aim of satisfying the nutritional requirements of crop plants for optimal growth.
The sensors and the other new technologies described in section “Realtime Monitoring of Hydroponic Solutions via Sensors” create massive flows of data that should then be analyzed in order to be adequately exploited. Machine learning algorithms (such as neural networks and genetic algorithms) might be applied for self-calibrating and managing the parameters of hydroponic solutions based on sensors data (Morimoto and Hashimoto, 1996; Suhardiyanto et al., 2009). The constant control of the composition and concentrations could in fact allow the recirculation and reuse of nutrient solutions within closed growing systems, thus reducing the economic costs and minimizing the environmental impact of soilless cultivation systems (Jung et al., 2015). Similarly, advanced Big Data analytics and simulation techniques might allow to forecast the quality and quantity of vegetable or fruit production under various conditions – for instance by creating a realtime “digital twin” of the real/physical hydroponic system – and in turn to determine the optimal parameters, such as the composition and concentration of the hydroponic nutrient solution; the temperature, humidity, and CO2 levels (in case of greenhouses); and the lighting (in case of greenhouses with artificial light). Finally, Internet of Things and cloud computing might be employed to share the data among different farmers and make the data analysis more efficient and effective.
The use of sensors and data analysis tools is fully included in the concept of precision agriculture (PA) – or smart agriculture (SA) as it has been recently labeled – a new paradigm based on the use of information and communication technologies in the cyber-physical farm management cycle. More formally, PA has been defined as a “management strategy that uses information & communication technologies (ICT) to collect data from multiple sources in view of their later use in decisions concerning production activities” (National Research Council, 1997). Originally, this definition was intended to refer to field processes, being mainly focused on the highly automated site-specific approaches, aiming at overcoming the management limits imposed by the relevant spatial variability in field properties. Later, it was extended to many other types of farming systems, such as livestock, viticulture, and orchards. SA is based on the so-called Knowledge Management approach (also renamed as Knowledge Management 4.0 or KM4.0; Calcante and Mazzetto, 2014; Mazzetto et al., 2017; Meško et al., 2017; Neumann, 2018), which includes the following aspects: (1) ensuring an approach highly oriented to the Internet of Things (IoT) knowledge processes; (2) managing and treating Big Data acquired directly from things (= elements of processes and products) and customers (people acting in the system); (3) sharing information between people or things without any limitation; (4) storing all data and information directly in clouds through Internet of Services (IoS); (5) ensuring that all contents are always available online, also to implement any realtime automation process; (6) providing information sharing (C2C, C2M) via wireless solutions (hyper-connectivity); (7) fostering of predictive analysis in the main maintenance and control tasks.
Hydroponic systems represent an ideal application context for SA (Dinesh et al., 2018) since they are closer to the industrial context: production processes are carried out in contained and more controllable spaces (greenhouses or tunnels); they are more repeatable (lighting, climatic parameters, and nutrient supply can be controlled); and they can be more easily automated. In the hydroponic practices, there are already some technological examples moving just toward such a direction. Fujitsu, ORIX Corporation, and Masuda Seed launched in 2016 the Iwata Smart Agriculture Project, which aims at collecting a wide set of data concerning the optimal parameters (temperature, humidity, CO2 levels, and nutrient concentration of hydroponic solutions) for hydroponic production of various seed varieties and sharing them through Internet. The (Big) Data are collected by Fujitsu in a plant factory located in the city of Iwata (Japan) and consisting of several sensorized and fully controlled greenhouses and then analyzed through the Microsoft’s Azure cloud, also relying on machine learning algorithms. They are then made available to farmers (both professional and amateur farmers) through the IoT and cloud computing platform Akisai Food and Agriculture. Ray (2017) provides an overview of various commercial IoT-based agriculture sensor systems, some of which are suitable for hydroponics and aquaponics farming (e.g., Bitponics, Open garden, and Niwa). Other studies (De Silva and De Silva, 2016; Yolanda et al., 2016; Charumathi et al., 2017) propose instead “new” IoT architectures for hydroponics and aquaponics farming, based on sensors, data loggers, actuators, and software tools (e.g., Arduino).
This overview of some issues affecting nutrient solutions in soilless cultivation systems clearly highlights the main working topics in which the research world is involved in the field. The huge potential offered by this cultivation approach is indisputable and ranges from productive and qualitative advantages to environmental benefits due to higher efficiency in the use of water and nutritional resources. Currently, there are well-studied and tested research areas whose results are commonly exploited in the soilless cultivation such as the NO3 − management or the crop quality increase by managing the electric conductivity of the solution. On the other side, besides these positive aspects, there are others, more difficult to manage, related to the interactions among nutrients in their acquisition processes and the nutrient dynamics (bio-geochemical cycles) in the hydroponic solution. These aspects represent a significant discriminant for the soilless management and, in some cases, may limit its diffusion, since the growers must possess specific knowledge and detailed skills to cope with and specifically per each crop species. It is interesting to note that parallel to the research areas dealing with these aspects, there are others newly emerging, technologically advanced but still not widely studied, even if they seem to provide promising tools (nanoparticles, PGPRs) for a more efficient use of this hydroponic-based cultivation approach. Nonetheless, a better knowledge concerning the processes underpinning the acquisition of nutrients and their allocation in the different tissues, also in the presence of these promising tools, is of fundamental importance. Moreover, in a context of smart agriculture strategies application, a consistent and pertinent design of the features of the Information System with the related hardware (sensors) and software (algorithms) components is crucial. Perhaps, borrowing these tools from the industrial environment, where the new paradigm of Industry 4.0 is already applied, could be strategic. Moreover, from the practical point of view, the application of this smart approach in the hydroponic production system will unavoidably require a decoupling of the hardware component management (sensors and data loggers, connections, actuators – in charge to the farmer) from that of all the software components (maintenance of database structures/persistency, interpretation algorithms, controlling data consistency, reporting updates). In fact, the complexity of this latter task requires – at least until when a massive employment of native digitals in the agriculture context is achieved – the presence of a service center specialized in smart agriculture.
Overall, the qualitative management of the crop through the nutrient solution is therefore a concrete strategy, already applicable and characterized by completely new perspectives that will help overcoming the current limits.
All the authors have equally contributed to the preparation of the manuscript.
This work has been financially supported by the Free University of Bozen – Bolzano (TN2053, TN2071, and TN2081).
The authors declare that the research was conducted in the absence of any commercial or financial relationships that could be construed as a potential conflict of interest.
Ahmed, A. F., Yu, H., Yang, X., and Jiang, W. (2014). Deficit irrigation affects growth, yield, vitamin C content, and irrigation water use efficiency of hot pepper grown in soilless culture. HortScience 49, 722–728. doi: 10.21273/HORTSCI.49.6.722
Alegria Terrazas, R., Giles, C., Paterson, E., Robertson-Albertyn, S., Cesco, S., Mimmo, T., et al. (2016). Plant-microbiota interactions as a driver of the mineral turnover in the rhizosphere. Adv. Appl. Microbiol. 95, 1–67. doi: 10.1016/bs.aambs.2016.03.001
Amalfitano, C., Del Vacchio, L., Somma, S., Cuciniello, A., and Caruso, G. (2017). Effects of cultural cycle and nutrient solution electrical conductivity on plant growth, yield and fruit quality of “Friariello” pepper grown in hydroponics. Hortic. Sci. 44, 91–98. doi: 10.17221/172/2015-HORTSCI
Amooaghaie, R. (2011). Fungal disinfection by nanofiltration in tomato soilless culture. World Acad. Sci. Eng. Technol. 5, 454–456. doi: 10.5281/zenodo.1078324
Asaduzzaman, M., and Asao, T. (2012). Autotoxicity in beans and their allelochemicals. Sci. Hortic. 134, 26–31. doi: 10.1016/j.scienta.2011.11.035
Asaduzzaman, M., Kobayashi, Y., Isogami, K., Tokura, M., Tokumasa, K., and Asao, T. (2012). Growth and yield recovery in strawberry plants under autotoxicity through electrodegradation. Eur. J. Hortic. Sci. 77, 58–67. ISSN: 1611-4426.
Asher, C. J., and Edwards, D. G. (1983). “Modern solution culture techniques” in Inorganic plant nutrition. eds. A. Pirson and M. H. Zimmermann (Berlin, Heidelberg: Springer), 94–119.
Astolfi, S., Ortolani, M. R., Catarcione, G., Paolacci, A. R., Cesco, S., Pinton, R., et al. (2014). Cadmium exposure affects iron acquisition in barley (Hordeum vulgare) seedlings. Physiol. Plant. 152, 646–659. doi: 10.1111/ppl.12207
Astolfi, S., Zuchi, S., Neumann, G., Cesco, S., di Toppi, L. S., and Pinton, R. (2012). Response of barley plants to Fe deficiency and Cd contamination as affected by S starvation. J. Exp. Bot. 63, 1241–1250. doi: 10.1093/jxb/err344
Ávila, F. W., Yang, Y., Faquin, V., Ramos, S. J., Guilherme, L. R. G., Thannhauser, T. W., et al. (2014). Impact of selenium supply on Se-methylselenocysteine and glucosinolate accumulation in selenium-biofortified Brassica sprouts. Food Chem. 165, 578–586. doi: 10.1016/j.foodchem.2014.05.134
Bailey, B. J., Haggett, B. G. D., Hunter, A., Albery, W. J., and Svanberg, L. R. (1988). Monitoring nutrient film solutions using ion-selective electrodes. J. Agric. Eng. Res. 40, 129–142. doi: 10.1016/0021-8634(88)90110-2
Bamsey, M., Graham, T., Thompson, C., Berinstain, A., Scott, A., and Dixon, M. (2012). Ion-specific nutrient management in closed systems: the necessity for ion-selective sensors in terrestrial and space-based agriculture and water management systems. Sensors 12, 13349–13392. doi: 10.3390/s121013349
Barbosa, G. L., Almeida Gadelha, F. D., Kublik, N., Proctor, A., Reichelm, L., Weissinger, E., et al. (2015). Comparison of land, water, and energy requirements of lettuce grown using hydroponic vs. conventional agricultural methods. Int. J. Environ. Res. Public Health 12, 6879–6891. doi: 10.3390/ijerph120606879
Bar-Yosef, B. (2008). “Fertigation management and crops response to solution recycling in semi-closed greenhouses” in Soilless culture: Theory and practice. eds. M. Raviv and H. J. Lieth (Amsterdam, The Netherlands: Elsevier), 341–424.
Baset Mia, M. A., Shamsuddin, Z. H., Wahab, Z., and Marziah, M. (2010). Effect of plant growth promoting rhizobacterial (PGPR) inoculation on growth and nitrogen incorporation of tissue-cultured Musa plantlets under nitrogen-free hydroponics condition. Aust. J. Crop. Sci. 4, 85–90. Available at: http://www.cropj.com/mia_4_2_2010_85_90.pdf
Bashan, Y. (1990). Short exposure to Azospirillum brasilense Cd inoculation enhanced proton efflux of intact wheat roots. Can. J. Microbiol. 36, 419–425. doi: 10.1139/m90-073
Bashan, Y., Levanony, H., and Mitiku, G. (1989). Changes in proton efflux of intact wheat roots induced by Azospirillum brasilense Cd. Can. J. Microbiol. 35, 691–697. doi: 10.1139/m89-113
Benke, K., and Tomkins, B. (2017). Future food-production systems: vertical farming and controlled-environment agriculture. Sustain. Sci. Pract. Policy 13, 13–26. doi: 10.1080/15487733.2017.1394054
Bertrand, H., Plassard, C., Pinochet, X., Touraine, B., Normand, P., and Cleyet-Marel, J. C. (2000). Stimulation of the ionic transport system in Brassica napus by a plant growth-promoting rhizobacterium (Achromobacter sp.). Can. J. Microbiol. 46, 229–236. doi: 10.1139/w99-137
Bloom, A. J., Jackson, L. E., and Smart, D. R. (1993). Root growth as a function of ammonium and nitrate in the root zone. Plant Cell Environ. 16, 199–206. doi: 10.1111/j.1365-3040.1993.tb00861.x
Bradley, P., and Marulanda, C. (2001). Simplified hydroponics to reduce global hunger. Acta Hortic. 554, 289–295. Available at: https://www.scopus.com/inward/record.uri?eid=2-s2.0-60849112730&partnerID=40&md5=acf7509279425410e4536d6658aab38b
Burman, U., Saini, M., and Kumar, P. (2013). Effect of zinc oxide nanoparticles on growth and antioxidant system of chickpea seedlings. Toxicol. Environ. Chem. 95, 605–612. doi: 10.1080/02772248.2013.803796
Bybordi, A., Tabatabaei, S. J., and Ahmadov, A. (2012). Influence of salinity and ammonium:nitrate ratio on growth, photosynthesis, fatty acid and the activity of antioxidative enzymes in canola. J. Plant Nutr. 35, 2089–2106. doi: 10.1080/01904167.2012.723772
Calcante, A., and Mazzetto, F. (2014). Design, development and evaluation of a wireless system for the automatic identification of implements. Comput. Electron. Agric. 101, 118–127. doi: 10.1016/j.compag.2013.12.010
Campos, E. V. R., de Oliveira, J. L., and Fraceto, L. F. (2014). Applications of controlled release systems for fungicides, herbicides, acaricides, nutrients, and plant growth hormones: a review. Adv. Sci. Eng. Med. 6, 373–387. doi: 10.1166/asem.2014.1538
Canellas, L., Balmori, D., Médici, L., Aguiar, N., Campostrini, E., Rosa, R. C., et al. (2013). A combination of humic substances and Herbaspirillum seropedicae inoculation enhances the growth of maize (Zea mays L.). Plant Soil 366, 119–132. doi: 10.1007/s11104-012-1382-5
Canellas, L. P., Olivares, F. L., and Okorokova-fac, A. L. (2002). Humic acids isolated from earthworm compost enhance root elongation, lateral root emergence, and plasma membrane H+-ATPase activity in maize roots. Plant Physiol. 130, 1951–1957. doi: 10.1104/pp.007088
Carmassi, G., Incrocci, L., Maggini, R., Malorgio, F., Tognoni, F., and Pardossi, A. (2005). Modeling salinity build-up in recirculating nutrient solution culture. J. Plant Nutr. 28, 431–445. doi: 10.1081/PLN-200049163
Cesco, S., Römheld, V., Varanini, Z., and Pinton, R. (2000). Solubilization of iron by water-extractable humic substances. J. Plant Nutr. Soil Sci. 163, 285–290. doi: 10.1002/1522-2624(200006)163:3<285::AID-JPLN285>3.0.CO;2-Z
Charumathi, S., Kaviya, R. M., Kumariyarasi, J., Manisha, R., and Dhivya, P. (2017). Optimization and control of hydroponics agriculture using IOT. Asian J. Appl. Sci. Technol. 1, 96–98.
Chekli, L., Kim, J. E., El Saliby, I., Kim, Y., Phuntsho, S., Li, S., et al. (2017). Fertilizer drawn forward osmosis process for sustainable water reuse to grow hydroponic lettuce using commercial nutrient solution. Sep. Purif. Technol. 181, 18–28. doi: 10.1016/j.seppur.2017.03.008
Chen, J. (2007). Rapid urbanization in China: a real challenge to soil protection and food security. Catena 69, 1–15. doi: 10.1016/j.catena.2006.04.019
Chen, F., Wei, D., and Tang, Y. (2011). Virtual ion selective electrode for online measurement of nutrient solution components. IEEE Sensors J. 11, 462–468. doi: 10.1109/JSEN.2010.2060479
Cho, W. J., Kim, H.-J., Jung, D. H., Kang, C. I., Choi, G.-L., and Son, J.-E. (2017). An embedded system for automated hydroponic nutrient solution management. Trans. ASABE 60, 1083–1096. doi: 10.13031/trans.12163
Colombo, C., Palumbo, G., He, J.-Z., Pinton, R., and Cesco, S. (2013). Review on iron availability in soil: interaction of Fe minerals, plants, and microbes. J. Soils Sediments 14, 538–548. doi: 10.1007/s11368-013-0814-z
Connolly, E. L., Campbell, N. H., Grotz, N., Prichard, C. L., and Guerinot, M. L. (2003). Overexpression of the FRO2 Ferric Chelate Reductase confers tolerance to growth on low iron and uncovers posttranscriptional control. Plant Physiol. 133, 1102–1110. doi: 10.1104/pp.103.025122
D’Imperio, M., Renna, M., Cardinali, A., Buttaro, D., Santamaria, P., and Serio, F. (2016a). Silicon biofortification of leafy vegetables and its bioaccessibility in the edible parts. J. Sci. Food Agric. 96, 751–756. doi: 10.1002/jsfa.7142
D’Imperio, M., Renna, M., Cardinali, A., Buttaro, D., Serio, F., and Santamaria, P. (2016b). Calcium biofortification and bioaccessibility in soilless “baby leaf” vegetable production. Food Chem. 213, 149–156. doi: 10.1016/j.foodchem.2016.06.071
da Silva Cuba Carvalho, R., Bastos, R. G., and Souza, C. F. (2018). Influence of the use of wastewater on nutrient absorption and production of lettuce grown in a hydroponic system. Agric. Water Manag. 203, 311–321. doi: 10.1016/j.agwat.2018.03.028
De Rijck, G., and Schrevens, E. (1998a). Elemental bioavailability in nutrient solutions in relation to complexation reactions. J. Plant Nutr. 21, 849–859. doi: 10.1080/01904169809365448
De Rijck, G., and Schrevens, E. (1998b). Elemental bioavailability in nutrient solutions in relation to precipitation reactions. J. Plant Nutr. 21, 2103–2113. doi: 10.1080/01904169809365547
de Santiago, A., García-López, A. M., Quintero, J. M., Avilés, M., and Delgado, A. (2013). Effect of Trichoderma asperellum strain T34 and glucose addition on iron nutrition in cucumber grown on calcareous soils. Soil Biol. Biochem. 57, 598–605. doi: 10.1016/j.soilbio.2012.06.020
De Silva, P. C. P., and De Silva, P. C. A. (2016). “Ipanera: an industry 4.0 based architecture for distributed soil-less food production systems” in 2016 Manufacturing & Industrial Engineering Symposium (MIES), (Colombo, Sri Lanka: IEEE), 1–5.
Dinesh, K., Gobinath, M., and Subathra, M. (2018). “A survey on intelligent internet of things-technology and its application” in International Conference on Inventive Research in Computing Applications (ICIRCA), (Coimbatore, India), 81–84.
Dukes, M. D., Zotarelli, L., Liu, G. D., and Simonne, E. H. (2012). Principles and practices of irrigation management for vegetables.
Ehret, D. L., Alsanius, B., Wohanka, W., Menzies, J. G., and Utkhede, R. (2001). Disinfestation of recirculating nutrient solutions in greenhouse horticulture. Agronomie 21, 323–339. doi: 10.1051/agro:2001127
El Kassis, E., Cathala, N., Rouached, H., Fourcroy, P., Berthomieu, P., Terry, N., et al. (2007). Characterization of a selenate-resistant Arabidopsis mutant. Root growth as a potential target for selenate toxicity. Plant Physiol. 143, 1231–1241. doi: 10.1104/pp.106.091462
Elmer, W. H., and White, J. C. (2016). The use of metallic oxide nanoparticles to enhance growth of tomatoes and eggplants in disease infested soil or soilless medium. Environ. Sci. Nano 3, 1072–1079. doi: 10.1039/C6EN00146G
El-Sayed, S. F., Hassan, H. A., and Mahmoud, S. O. (2015). Effect of some soilless culture techniques on sweet pepper growth, production, leaves chemical contents and water consumption under greenhouse conditions. Middle East J. Agric. Res., 682–691. ISSN: 2077-4605.
Errebhi, M., and Wilcox, G. E. (1990). Plant species response to ammonium-nitrate concentration ratios. J. Plant Nutr. 13, 1017–1029. doi: 10.1080/01904169009364132
Fageria, N. K. (2010). The use of nutrients in crop plants. Broken Sound Parakway NW, Suite, Boca Raton: CRC Press.
Fallovo, C., Rouphael, Y., Rea, E., Battistelli, A., and Colla, G. (2009). Nutrient solution concentration and growing season affect yield and quality of Lactuca sativa L. var. acephala in floating raft culture. J. Sci. Food Agric. 89, 1682–1689. doi: 10.1002/jsfa.3641
Fazlil Ilahi, W. F., Ahmad, D., and Husain, M. C. (2017). Effects of root zone cooling on butterhead lettuce grown in tropical conditions in a coir-perlite mixture. Hortic. Environ. Biotechnol. 58, 1–4. doi: 10.1007/s13580-017-0123-3
Ferrarese, M., Sourestani, M., Quattrini, E., Schiavi, M., and Ferrante, A. (2012). Biofortification of spinach plants applying selenium in the nutrient solution of floating system. Veg. Crop. Res. Bull. 76, 127–136. doi: 10.2478/v10032-012-0009-y
Forde, B. G., and Clarkson, D. T. (1999). Nitrate and ammonium nutrition of plants: physiological and molecular perspectives. Adv. Bot. Res. 30, 1–90. doi: 10.1016/S0065-2296(08)60226-8
García, J. A. L., Probanza, A., Ramos, B., Palomino, M. R., and Gutiérrez Mañero, F. J. (2004). Effect of inoculation of Bacillus licheniformis on tomato and pepper. Agronomie 24, 169–176. doi: 10.1051/agro:2004020
Gardea-Torresdey, J. L., Rico, C. M., and White, J. C. (2014). Trophic transfer, transformation, and impact of engineered nanomaterials in terrestrial environments. Environ. Sci. Technol. 48, 2526–2540. doi: 10.1021/es4050665
Garibaldi, A., Gilardi, G., Cogliati, E. E., and Gullino, M. L. (2012). Silicon and increased electrical conductivity reduce downy mildew of soilless grown lettuce. Eur. J. Plant Pathol. 132, 123–132. doi: 10.1007/s10658-011-9855-6
Gierth, M., and Mäser, P. (2007). Potassium transporters in plants – involvement in K+ acquisition, redistribution and homeostasis. FEBS Letters 581, 2348–2356. doi: 10.1016/j.febslet.2007.03.035
Giro, A., and Ferrante, A. (2017). Postharvest physiology of Corchorus olitorius baby leaf growing with different nutrient solutions. J. Hortic. Sci. Biotechnol. 93, 1–9. doi: 10.1080/14620316.2017.1382313
Giuffrida, F., Cassaniti, C., Malvuccio, A., and Leonardi, C. (2017). Effects of salt stress imposed during two growth phases on cauliflower production and quality. J. Sci. Food Agric. 97, 1552–1560. doi: 10.1002/jsfa.7900
Giuffrida, F., Graziani, G., Fogliano, V., Scuderi, D., Romano, D., and Leonardi, C. (2014). Effects of nutrient and NaCl salinity on growth, yield, quality and composition of pepper grown in soilless closed system. J. Plant Nutr. 37, 1455–1474. doi: 10.1080/01904167.2014.881874
Glick, B. R. (2012). Plant growth-promoting bacteria: mechanisms and applications. Scientifica 2012, 1–15. doi: 10.6064/2012/963401
Gonzalo, M. J., Lucena, J. J., and Hernández-Apaolaza, L. (2013). Effect of silicon addition on soybean (Glycine max) and cucumber (Cucumis sativus) plants grown under iron deficiency. Plant Physiol. Biochem. 70, 455–461. doi: 10.1016/j.plaphy.2013.06.007
Gottardi, S., Iacuzzo, F., Tomasi, N., Cortella, G., Manzocco, L., Pinton, R., et al. (2012). Beneficial effects of silicon on hydroponically grown corn salad (Valerianella locusta (L.) Laterr) plants. Plant Physiol. Biochem. 56, 14–23. doi: 10.1016/j.plaphy.2012.04.002
Grattan, S., and Grieve, C. (1998). Salinity-mineral nutrient relations in horticultural crops. Sci. Hortic. 78, 127–157. doi: 10.1016/S0304-4238(98)00192-7
Gruda, N. (2009). Do soilless culture systems have an influence on product quality of vegetables? J. Appl. Bot. Food Qual. 82, 141–147.
Gül, A., Kidoglu, F., and Tüzel, Y. (2008). Effects of nutrition and Bacillus amyloliquefaciens on tomato (Solanum lycopersicum L.) growing in perlite. Span. J. Agric. Res. 6, 422–429. doi: 10.5424/sjar/2008063-335
Gupta, M., Qiu, X., Wang, L., Xie, W., Zhang, C., Xiong, L., et al. (2008). KT/HAK/KUP potassium transporters gene family and their whole-life cycle expression profile in rice (Oryza sativa). Mol. Gen. Genomics. 280, 437–452. doi: 10.1007/s00438-008-0377-7
Gutiérrez, M., Alegret, S., Cáceres, R., Casadesús, J., Marfà, O., and del Valle, M. (2007). Application of a potentiometric electronic tongue to fertigation strategy in greenhouse cultivation. Comput. Electron. Agric. 57, 12–22. doi: 10.1016/j.compag.2007.01.012
Gutiérrez, M., Alegret, S., Cáceres, R., Casadesús, J., Marfà, O., and del Valle, M. (2008). Nutrient solution monitoring in greenhouse cultivation employing a potentiometric electronic tongue. J. Agric. Food Chem. 56, 1810–1817. doi: 10.1021/jf073438s
Haghighi, M., Abolghasemi, R., and Teixeira da Silva, J. A. (2014). Low and high temperature stress affect the growth characteristics of tomato in hydroponic culture with Se and nano-Se amendment. Sci. Hortic. 178, 231–240. doi: 10.1016/J.SCIENTA.2014.09.006
Haghighi, M., and Pessarakli, M. (2013). Influence of silicon and nano-silicon on salinity tolerance of cherry tomatoes (Solanum lycopersicum L.) at early growth stage. Sci. Hortic. 161, 111–117. doi: 10.1016/j.scienta.2013.06.034
Hamdy, A., Ahmed, F. T., and Choukr-Allah, R. (2002). Muskmelon production in soilless culture under saline irrigation practices and soil conditioner application. Acta Hortic., 321–330. doi: 10.17660/actahortic.2002.573.37
Haynes, R. J., and Goh, K. M. (1978). Ammonium and nitrate nutrition of plants. Biol. Rev. 53, 465–510. doi: 10.1111/j.1469-185X.1978.tb00862.x
He, X. L., Fan, S. K., Zhu, J., Guan, M. Y., Liu, X. X., Zhang, Y. S., et al. (2017). Iron supply prevents Cd uptake in Arabidopsis by inhibiting IRT1 expression and favoring competition between Fe and Cd uptake. Plant Soil 416, 453–462. doi: 10.1007/s11104-017-3232-y
Hibar, K., Daami-Remadi, M., Hamada, W., and El-Mahjoub, M. (2006). Bio-fungicides as an alternative for tomato Fusarium crown and root rot control. Tunis. J. Plant Prot. 1, 19–29.
Hickman, G. (2016). International greenhouse vegetable production – Statistics. Mariposa, CA, USA: Cuesta Roble Greenhouse Vegetable Consulting.
Hopper, J. L., and Parker, D. R. (1999). Plant availability of selenite and selenate as influenced by the competing ions phosphate and sulfate. Plant Soil 210, 199–207. doi: 10.1023/A:1004639906245
Hosseinzadeh, S., Liu, Z., De Graeve, J., Kheet, M. B., Libbrecht, W., De Clercq, J., et al. (2019). Recirculating water treatment in closed hydroponic systems: assessment of granular activated carbon and soft templated mesoporous carbon for adsorptive removal of root exudates. Environ. Processes 6, 1–23. doi: 10.1007/s40710-019-00347-0
Hosseinzadeh, S., Verheust, Y., Bonarrigo, G., and Van Hulle, S. (2017). Closed hydroponic systems: operational parameters, root exudates occurrence and related water treatment. Rev. Environ. Sci. Biotechnol. 16, 59–79. doi: 10.1007/s11157-016-9418-6
Islam, M. Z., Mele, M. A., Baek, J. P., and Kang, H. (2018). Iron, iodine and selenium effects on quality, shelf life and microbial activity of cherry tomatoes. Notulae Botanicae Horti Agrobotanici Cluj-Napoca 46, 388–392. doi: 10.1186/s41182-018-0085-x
Jeyasubramanian, K., Gopalakrishnan Thoppey, U. U., Hikku, G. S., Selvakumar, N., Subramania, A., and Krishnamoorthy, K. (2016). Enhancement in growth rate and productivity of spinach grown in hydroponics with iron oxide nanoparticles. RSC Adv. 6, 15451–15459. doi: 10.1039/C5RA23425E
Jones, J. B., Zitter, T. A., Momol, T. M., and Miller, S. A. (1991). Compendium of tomato diseases and pests. The American Phytopathological Society.
Jung, D. H., Kim, H.-J., Choi, G. L., Ahn, T.-I., Son, J.-E., and Sudduth, K. A. (2015). Automated lettuce nutrient solution management using an array of ion-selective electrodes. Trans. ASABE 58, 1309–1319. doi: 10.13031/trans.58.11228
Kagermann, H., Wahlster, W., and Helbig, J. (2013). Umsetzungsempfehlungen für das Zukunftsprojekt Industrie 4.0. Abschlussbericht des Arbeitskreises Industrie 4.0. Frankfurt: Promotorengruppe Kommunikation der Forschungsunion Wirtschaft – Wissenschaft, acatech.
Kalteh, M., Alipour, Z. T., Ashraf, S., Marashi Aliabadi, M., and Falah Nosratabadi, A. (2018). Effect of silica nanoparticles on basil (Ocimum basilicum) under salinity stress. J. Chem. Health Risks 4, 49–55. doi: 10.22034/JCHR.2018.544075
Karuppanapandian, T., Wang, H. W., Prabakaran, N., Jeyalakshmi, K., Kwon, M., Manoharan, K., et al. (2011). 2,4-dichlorophenoxyacetic acid-induced leaf senescence in mung bean (Vigna radiata L. Wilczek) and senescence inhibition by co-treatment with silver nanoparticles. Plant Physiol. Biochem. 49, 168–177. doi: 10.1016/j.plaphy.2010.11.007
Khan, M. N., Mobin, M., Abbas, Z. K., AlMutairi, K. A., and Siddiqui, Z. H. (2017). Role of nanomaterials in plants under challenging environments. Plant Physiol. Biochem. 110, 194–209. doi: 10.1016/j.plaphy.2016.05.038
Kim, H.-J., Kim, W.-K., Roh, M.-Y., Kang, C.-I., Park, J.-M., and Sudduth, K. A. (2013). Automated sensing of hydroponic macronutrients using a computer-controlled system with an array of ion-selective electrodes. Comput. Electron. Agric. 93, 46–54. doi: 10.1016/j.compag.2013.01.011
Kinoshita, T., Yamazaki, H., Inamoto, K., and Yamazaki, H. (2016). Analysis of yield components and dry matter production in a simplified soilless tomato culture system by using controlled-release fertilizers during summer-winter greenhouse production. Sci. Hortic. 202, 17–24. doi: 10.1016/j.scienta.2016.02.019
Kitazawa, H., Asao, T., Ban, T., Pramanik, M. H. R., and Hosoki, T. (2005). Autotoxicity of root exudates from strawberry in hydroponic culture. J. Hortic. Sci. Biotechnol. 80, 677–680. doi: 10.1080/14620316.2005.11511997
Korshunova, Y., Eide, D., Gregg Clark, W., Lou Guerinot, M., and Pakrasi, H. (1999). The IRT1 protein from Arabidopsis thaliana is a metal transporter with a broad substrate range. Plant Mol. Biol. 40, 37–44. doi: 10.1023/A:1026438615520
Kraemer, S. M., Crowley, D. E., and Kretzschmar, R. (2006). Geochemical aspects of phytosiderophore-promoted iron acquisition by plants. Adv. Agron. 91, 1–46. doi: 10.1016/S0065-2113(06)91001-3
Kronzucker, H. J., Glass, A. D. M., and Siddiqi, M. Y. (1999). Inhibition of nitrate uptake by ammonium in barley. Analysis of component fluxes. Plant Physiol. 120, 283–292. doi: 10.1104/pp.120.1.283
Lee, S., and Lee, J. (2015). Beneficial bacteria and fungi in hydroponic systems: types and characteristics of hydroponic food production methods. Sci. Hortic. 195, 206–215. doi: 10.1016/j.scienta.2015.09.011
Lee, J. Y., Rahman, A., Azam, H., Kim, H. S., and Kwon, M. J. (2017). Characterizing nutrient uptake kinetics for efficient crop production during Solanum lycopersicum var. cerasiforme Alef. growth in a closed indoor hydroponic system. PLoS One 12:e0177041. doi: 10.1371/journal.pone.0177041
Lee, Y. D., and Takakura, T. (1995). Root cooling for spinach in deep hydroponic culture under high air temperature conditions. Acta Hortic. 399, 121–126. doi: 10.17660/ActaHortic.1995.399.12
Li, R., Li, D.-W., Yan, A.-L., Hong, C.-L., Liu, H.-P., Pan, L.-H., et al. (2017). The bioaccessibility of iodine in the biofortified vegetables throughout cooking and simulated digestion. J. Food Sci. Technol. 55, 366–375. doi: 10.1007/s13197-017-2946-4
Liang, Y., Nikolic, M., Bélanger, R., Gong, H., and Song, A. (2015). Silicon in agriculture: From theory to practice. Netherlands: Springer.
Lin, D., and Xing, B. (2007). Phytotoxicity of nanoparticles: inhibition of seed germination and root growth. Environ. Pollut. 150, 243–250. doi: 10.1016/j.envpol.2007.01.016
Liu, R., and Lal, R. (2015). Potentials of engineered nanoparticles as fertilizers for increasing agronomic productions. Sci. Total Environ. 514, 131–139. doi: 10.1016/j.scitotenv.2015.01.104
Lucena, J. J. (2003). Fe chelates for remediation of Fe chlorosis in strategy I plants. J. Plant Nutr. 26, 1969–1984. doi: 10.1081/PLN-120024257
Lugtenberg, B., and Kamilova, F. (2009). Plant-growth-promoting rhizobacteria. Annu. Rev. Microbiol. 63, 541–556. doi: 10.1146/annurev.micro.62.081307.162918
Ma, J. F. (2004). Role of silicon in enhancing the resistance of plants to biotic and abiotic stresses. Soil Sci. Plant Nutr. 50, 11–18. doi: 10.1080/00380768.2004.10408447
Ma, J., and Takahashi, E. (1989). Effect of silicic acid on phosphorus uptake by rice plant. Soil Sci. Plant Nutr. 35, 227–234. doi: 10.1080/00380768.1989.10434755
Malagoli, M., Schiavon, M., Dall’Acqua, S., and Pilon-Smits, E. A. H. (2015). Effects of selenium biofortification on crop nutritional quality. Front. Plant Sci. 6:280. doi: 10.3389/fpls.2015.00280
Marschner, P. (2012). Marschner’s mineral nutrition of higher plants. 3rd Edn. London: Academic Press.
Martínez, C. E., and McBride, M. B. (2000). Aging of coprecipitated Cu in alumina: changes in structural location, chemical form, and solubility. Geochim. Cosmochim. Acta 64, 1729–1736. doi: 10.1016/S0016-7037(00)00344-6
Massa, D., Incrocci, L., Maggini, R., Carmassi, G., Campiotti, C. A., and Pardossi, A. (2010). Strategies to decrease water drainage and nitrate emission from soilless cultures of greenhouse tomato. Agric. Water Manage. 97, 971–980. doi: 10.1016/j.agwat.2010.01.029
Maucieri, C., Nicoletto, C., Junge, R., Schmautz, Z., Sambo, P., and Borin, M. (2017). Hydroponic systems and water management in aquaponics: a review. Ital. J. Agron. 11, 1–31. doi: 10.4081/ija.2017.1012
Mazzetto, F., Gallo, R., Importuni, P., Petrera, S., and Sacco, P. (2017). Automatic filling of field activities register, from challenge into reality. Chem. Eng. Trans. 58, 667–672. doi: 10.3303/CET1758112
Melzer, K., Bhatt, V. D., Schuster, T., Jaworska, E., Maksymiuk, K., Michalska, A., et al. (2016). “Multi ion-sensor arrays: towards an electronic tongue” in 16th International Conference on Nanotechnology – IEEE NANO 2016, 475–478.
Mengel, K., Viro, M., and Hehl, G. (1976). Effect of potassium on uptake and incorporation of ammonium-nitrogen of rice plants. Plant Soil 44, 547–558. doi: 10.1007/BF00011374
Meško, M., Suklan, J., and Roblek, V. (2017). “The impact of the Internet of Things to value added in knowledge-intensive organizations” in Knowledge management strategies and applications (InTech).
Mimmo, T., Del Buono, D., Terzano, R., Tomasi, N., Vigani, G., Crecchio, C., et al. (2014). Rhizospheric organic compounds in the soil-microorganism-plant system: their role in iron availability. Eur. J. Soil Sci. 65, 629–642. doi: 10.1111/ejss.12158
Mimmo, T., Tiziani, R., Valentinuzzi, F., Lucini, L., Nicoletto, C., Sambo, P., et al. (2017). Selenium biofortification in Fragaria × ananassa: implications on strawberry fruits quality, content of bioactive health beneficial compounds and metabolomic profile. Front. Plant Sci. 8:1887. Available at: https://www.frontiersin.org/article/10.3389/fpls.2017.01887
Miyama, Y., Sunada, K., Fujiwara, S., and Hashimoto, K. (2009). Photocatalytic treatment of waste nutrient solution from soil-less cultivation of tomatoes planted in rice hull substrate. Plant Soil 318, 275–283. doi: 10.1007/s11104-008-9837-4
Mohammadi, R., Maali-Amiri, R., and Abbasi, A. (2013). Effect of TiO2 nanoparticles on chickpea response to cold stress. Biol. Trace Elem. Res. 152, 403–410. doi: 10.1007/s12011-013-9631-x
Mondal, M. F., Asaduzzaman, M., Kobayashi, Y., Ban, T., and Asao, T. (2013). Recovery from autotoxicity in strawberry by supplementation of amino acids. Sci. Hortic. 164, 137–144. doi: 10.1016/j.scienta.2013.09.019
Mondal, M. F., Asaduzzaman, M., Tanaka, H., and Asao, T. (2015). Effects of amino acids on the growth and flowering of Eustoma grandiflorum under autotoxicity in closed hydroponic culture. Sci. Hortic. 192, 453–459. doi: 10.1016/j.scienta.2015.05.024
Montesano, F. F., D’Imperio, M., Parente, A., Cardinali, A., Renna, M., and Serio, F. (2016). Green bean biofortification for Si through soilless cultivation: plant response and Si bioaccessibility in pods. 6. doi: 10.1038/srep31662
Morimoto, T., and Hashimoto, Y. (1996). Optimal control of plant growth in hydroponics using neural network and genetic algorithms. Acta Hortic., 433–440. doi: 10.17660/ActaHortic.1996.406.43
Müller, C., Elliott, J., Chryssanthacopoulos, J., Arneth, A., Balkovic, J., and Ciais, P. (2017). Global gridded crop model evaluation: benchmarking, skills, deficiencies and implications. Geosci. Model Dev. Discuss. 10, 1403–1422. doi: 10.5194/gmd-2016-207
Na, L., Li, Z., Xiangxiang, M., Ara, N., Jinghua, Y., and Mingfang, Z. (2014). Effect of nitrate/ammonium ratios on growth, root morphology and nutrient elements uptake of watermelon (Citrullus lanatus) seedlings. J. Plant Nutr. 37, 1859–1872. doi: 10.1080/01904167.2014.911321
Nacry, P., Bouguyon, E., and Gojon, A. (2013). Nitrogen acquisition by roots: physiological and developmental mechanisms ensuring plant adaptation to a fluctuating resource. Plant Soil 270, 1–29. doi: 10.1007/s11104-013-1645-9
Nair, R., Varghese, S. H., Nair, B. G., Maekawa, T., Yoshida, Y., and Kumar, D. S. (2010). Nanoparticulate material delivery to plants. Plant Sci. 179, 154–163. doi: 10.1016/J.PLANTSCI.2010.04.012
Nancy, D., and Arulselvi, P. I. (2014). Effect of Selenium fortification on biochemical activities of tomato (Solanum lycopersicum) plants. Indo Am. J. Pharm. Res. 4, 3997–4005. Available at: www.iajpr.comwww.iajpr.com (Accessed April 10, 2019).
National Research Council (1997). Precision agriculture in the 21st century: Geospatial and information technologies in crop management. Washington, D.C. National Academies Press.
Neocleous, D., Ntatsi, G., and Savvas, D. (2017). Physiological, nutritional and growth responses of melon (Cucumis melo L.) to a gradual salinity built-up in recirculating nutrient solution. J. Plant Nutr. 40, 2168–2180. doi: 10.1080/01904167.2017.1346673
Neumann, G. (2018). Knowledge management 4.0 – implications of the fourth industrial revolution on knowledge management in supply chains. Theory Appl. Knowl. Econ. 452.
Nikolic, M., Cesco, S., Römheld, V., Varanini, Z., and Pinton, R. (2007). Short-term interactions between nitrate and iron nutrition in cucumber. Funct. Plant Biol. 34, 402–408. doi: 10.1071/FP07022
Packter, A. (1974). The precipitation of calcium sulphate dihydrate from aqueous solution: induction periods, crystal numbers and final size. J. Cryst. Growth 21, 191–194. doi: 10.1016/0022-0248(74)90004-9
Pardossi, A., Falossi, F., Malorgio, F., Incrocci, L., and Bellocchi, G. (2005). Empirical models of macronutrient uptake in melon plants grown in recirculating nutrient solution culture. J. Plant Nutr. 27, 1261–1280. doi: 10.1081/PLN-120038547
Parida, A. K., and Das, A. B. (2005). Salt tolerance and salinity effects on plants: a review. Ecotoxicol. Environ. Saf. 60, 324–349. doi: 10.1016/j.ecoenv.2004.06.010
Park, S., Elless, M. P., Park, J., Jenkins, A., Lim, W., Chambers, E. IV, et al. (2009). Sensory analysis of calcium-biofortified lettuce. Plant Biotechnol. J. 7, 106–117. doi: 10.1111/j.1467-7652.2008.00379.x
Pavlovic, J., Samardzic, J., Maksimovi, V., Timotijevic, G., Stevic, N., Laursen, K. H., et al. (2013). Silicon alleviates iron deficiency in cucumber by promoting mobilization of iron in the root apoplast. New Phytol 198, 1096–1107. doi: 10.1111/nph.12213
Phuntsho, S., Shon, H. K., Hong, S., Lee, S., Vigneswaran, S., and Kandasamy, J. (2012). Fertiliser drawn forward osmosis desalination: the concept, performance and limitations for fertigation. Rev. Environ. Sci. Biotechnol. 11, 147–168. doi: 10.1007/s11157-011-9259-2
Pignata, G., Casale, M., and Nicola, S. (2017). “Water and nutrient supply in horticultural crops grown in soilless culture: resource efficiency in dynamic and intensive systems” in Advances in research on fertilization management of vegetable crops eds. F. Tei, S. Nicola, and P. Benincasa (Cham: Springer), 183–219.
Pii, Y., Aldrighetti, A., Valentinuzzi, F., Mimmo, T., and Cesco, S. (2019). Azospirillum brasilense inoculation counteracts the induction of nitrate uptake in maize plants. J. Exp. Bot. 70, 1313–1324. doi: 10.1093/jxb/ery433
Pii, Y., Graf, H., Valentinuzzi, F., Cesco, S., and Mimmo, T. (2018). The effects of plant growth-promoting rhizobacteria (PGPR) on the growth and quality of strawberries. Acta Hortic. 1217, 231–238. doi: 10.17660/ActaHortic.2018.1217.29
Pii, Y., Marastoni, L., Springeth, C., Fontanella, M. C., Beone, G. M., Cesco, S., et al. (2016). Modulation of Fe acquisition process by Azospirillum brasilense in cucumber plants. Environ. Exp. Bot. 130, 216–225. doi: 10.1016/j.envexpbot.2016.06.011
Pii, Y., Mimmo, T., Tomasi, N., Terzano, R., Cesco, S., and Crecchio, C. (2015). Microbial interactions in the rhizosphere: beneficial influences of plant growth-promoting rhizobacteria on nutrient acquisition process. A review. Biol. Fertil. Soils 51, 403–415. doi: 10.1007/s00374-015-0996-1
Pinton, R., Cesco, S., Santi, S., Agnolon, F., and Varanini, Z. (1999). Water-extractable humic substances enhance iron deficiency responses by Fe-deficient cucumber plants. Plant and Soil 210, 145–157. doi: 10.1023/A:1004329513498
Pinton, R., Cesco, S., Santi, S., and Varanini, Z. (1997). Soil humic substances stimulate proton release by intact oat seedling roots. J. Plant Nutr. 20, 857–869. doi: 10.1080/01904169709365301
Prosser, I. M., Purves, J. V., Saker, L. R., and Clarkson, D. T. (2001). Rapid disruption of nitrogen metabolism and nitrate transport in spinach plants deprived of sulphate. J. Exp. Bot. 52, 113–121. doi: 10.1093/jxb/52.354.113
Putra, P. A., and Yuliando, H. (2015). Soilless culture system to support water use efficiency and product quality: a review. Agric. Agric. Sci. Procedia 3, 283–288. doi: 10.1016/j.aaspro.2015.01.054
Qados, A. M. S. A. (2015). Mechanism of nanosilicon-mediated alleviation of salinity stress in faba bean (Vicia faba L.) plants. Am. J. Exp. Agric. 7, 78–95. Available at: https://www.cabdirect.org/cabdirect/abstract/20153160126 (Accessed May 15, 2019).
Qados, A., and Moftah, A. (2014). Influence of silicon and nano-silicon on germination, growth and yield of faba bean (Vicia faba L.) under salt stress conditions. Am. J. Exp. Agric. 5, 509–524. doi: 10.9734/ajea/2015/14109
Ray, P. P. (2017). Internet of things for smart agriculture: technologies, practices and future direction. J. Ambient Intell. Smart Environ. 9, 395–420. doi: 10.3233/AIS-170440
Rius-Ruiz, F. X., Andrade, F. J., Riu, J., and Rius, F. X. (2014). Computer-operated analytical platform for the determination of nutrients in hydroponic systems. Food Chem. 147, 92–97. doi: 10.1016/j.foodchem.2013.09.114
Rodriguez-Ortega, W. M., Martinez, V., Rivero, R. M., Camara-Zapata, J. M., Mestre, T., and Garcia-Sanchez, F. (2017). Use of a smart irrigation system to study the effects of irrigation management on the agronomic and physiological responses of tomato plants grown under different temperatures regimes. Agric. Water Manag. 183, 158–168. doi: 10.1016/j.agwat.2016.07.014
Roosta, H. R., Safarizadeh, M., and Hamidpour, M. (2017). Effect of humic acid contained nano-fertile fertilizer spray on concentration of some nutrient elements in two lettuce cultivars in hydroponic system. J. Sci. Technol. Greenhouse Cult. 7. doi: 10.18869/acadpub.ejgcst.7.4.51
Rouphael, Y., Colla, G., Cardarelli, M., Fanasca, S., Salerno, A., Rivera, C. M., et al. (2005). Water use efficiency of greenhouse summer squash in relation to the method of culture: soil vs. soilless. Acta Hortic. 81–86. doi: 10.17660/ActaHortic.2005.697.8
Rufty, T. W., Jackson, W. A., and Raper, C. D. (1982). Inhibition of nitrate assimilation in roots in the presence of ammonium: the moderating influence of potassium. J. Exp. Bot. 33, 1122–1137. doi: 10.1093/jxb/33.6.1122
Santamaria, P. (2006). Nitrate in vegetables: toxicity, content, intake and EC regulation. J. Sci. Food Agric. 86, 10–17. doi: 10.1002/jsfa.2351
Savvas, D. (2003). Hydroponics: A modern technology supporting the application of integrated crop management in greenhouse. Available at: www.world-food.net (Accessed April 9, 2019).
Schiavon, M., Dall’acqua, S., Mietto, A., Pilon-Smits, E. A. H., Sambo, P., Masi, A., et al. (2013). Selenium fertilization alters the chemical composition and antioxidant constituents of tomato (Solanum lycopersicon L.). J. Agric. Food Chem. 61, 10542–10554. doi: 10.1021/jf4031822
Schiavon, M., and Pilon-Smits, E. A. H. (2017). The fascinating facets of plant selenium accumulation – biochemistry, physiology, evolution and ecology. New Phytol. 213, 1582–1596. doi: 10.1111/nph.14378
Schimansky, C. (1981). Der Einfluß einiger Versuchsparameter auf das Fluxverhalten von 28Mg bei Gerstenkeimpflanzen in Hydrokulturversuchen. Landwirtsch. Forsch. 34, 154–165.
Schimel, J. P., and Chapin, F. S. (1996). Tundra plant uptake of amino acid and NH4+ nitrogen in situ: plants complete well for amino acid N. Ecology 77, 2142–2147. doi: 10.2307/2265708
Shafique, H. A., Sultana, V., Ehteshamul-Haque, S., and Athar, M. (2016). Management of soil-borne diseases of organic vegetables. J. Plant Prot. Res. 56, 221–230. doi: 10.1515/jppr-2016-0043
Shaviv, A., Hagin, J., and Neumann, P. M. (1987). Effects of a nitrification inhibitor on efficiency of nitrogen utilization by wheat and millet. Commun. Soil Sci. Plant Anal. 18, 815–833. doi: 10.1080/00103628709367865
Shibagaki, N., Rose, A., McDermott, J. P., Fujiwara, T., Hayashi, H., Yoneyama, T., et al. (2002). Selenate-resistant mutants of Arabidopsis thaliana identify Sultr1;2, a sulfate transporter required for efficient transport of sulfate into roots. Plant J. 29, 475–486. doi: 10.1046/j.0960-7412.2001.01232.x
Shin, R. (2014). Strategies for improving potassium use efficiency in plants. Mol. Cells 37, 575. doi: 10.14348/molcells.2014.0141
Siddiqui, M. H., Al-Whaibi, M. H., Faisal, M., and Al Sahli, A. A. (2014). Nano-silicon dioxide mitigates the adverse effects of salt stress on Cucurbita pepo L. Environ. Toxicol. Chem. 33, 2429–2437. doi: 10.1002/etc.2697
Silberbush, M., and Ben-Asher, J. (1989). The effect of NaCl concentration on NO3−, K+ and orthophosphate-P influx to peanut roots. Sci. Hortic. 39, 279–287. doi: 10.1016/0304-4238(89)90121-0
Singh, H., Batish, D. R., and Kohli, R. (1999). Autotoxicity: concept, organisms, and ecological significance. Crit. Rev. Plant Sci. 18, 757–772. doi: 10.1080/07352689991309478
Smoleń, S., Kowalska, I., Czernicka, M., Halka, M., Kęska, K., and Sady, W. (2016). Iodine and Selenium biofortification with additional application of salicylic acid affects yield, selected molecular parameters and chemical composition of lettuce plants (Lactuca sativa L. var. capitata). Front. Plant Sci. 7, 1–16. doi: 10.3389/fpls.2016.01553
Smoleń, S., Kowalska, I., and Sady, W. (2014). Assessment of biofortification with iodine and selenium of lettuce cultivated in the NFT hydroponic system. Sci. Hortic. 166, 9–16. doi: 10.1016/j.scienta.2013.11.011
Stamatakis, A., Papadantonakis, N., Lydakis-Simantiris, N., Kefalas, P., and Savvas, D. (2003). Effects of silicon and salinity on fruit yield and quality of tomato grown hydroponically. Acta Hortic. 609, 141–147. doi: 10.17660/ActaHortic.2003.609.18
Suhardiyanto, H., Arif, C., and Setiawan, I. B. (2009). Optimization of EC values of nutrient solution for tomato fruits quality in hydroponics system using artificial neural network and genetic algorithms. ITB J. Sci. 41, 38–49. doi: 10.5614/itbj.sci.2009.41.1.3
Tajudeen, A. L., and Taiwo, O. S. (2018). Soilless farming – a key player in the realisation of “zero hunger” of the sustainable development goals in Nigeria. Int. J. Ecol. Sci. Environ. Eng. 5, 1–7. ISSN: 2375-3854.
Takeno, N. (2005). Atlas of Eh-pH diagrams. Intercomparison of thermodynamic databases : National Institute of Advanced Industrial Science and Technology, 285.
Talukder, M. R., Asaduzzaman, M., Tanaka, H., and Asao, T. (2018). Light-emitting diodes and exogenous amino acids application improve growth and yield of strawberry plants cultivated in recycled hydroponics. Sci. Hortic. 239, 93–103. doi: 10.1016/j.scienta.2018.05.033
Talukder, M. R., Asaduzzaman, M., Tanaka, H., and Asao, T. (2019). Electro-degradation of culture solution improves growth, yield and quality of strawberry plants grown in closed hydroponics. Sci. Hortic. 243, 243–251. doi: 10.1016/j.scienta.2018.08.024
Tamai, K., and Ma, J. F. (2008). Reexamination of silicon effects on rice growth and production under field conditions using a low silicon mutant. Plant Soil 307, 21–27. doi: 10.1007/s11104-008-9571-y
ten Hoopen, F., Cuin, T. A., Pedas, P., Hegelund, J. N., Shabala, S., Schjoerring, J. K., et al. (2010). Competition between uptake of ammonium and potassium in barley and Arabidopsis roots: molecular mechanisms and physiological consequences. J. Exp. Bot. 61, 2303–2315. doi: 10.1093/jxb/erq057
Terzano, R., Cesco, S., and Mimmo, T. (2015). Dynamics, thermodynamics and kinetics of exudates: crucial issues in understanding rhizosphere processes. Plant Soil 386, 399–406. doi: 10.1007/s11104-014-2308-1
Tilman, D., Cassman, K. G., Matson, P. A., Naylor, R., and Polasky, S. (2002). Agriculture sustainability and intensive production practices. Nature 418, 671–677. doi: 10.1038/nature01014
Tittarelli, F., Båth, B., Ceglie, F. G., Garcia, C., Möller, K., Reents, H. J., et al. (2016). Soil fertility management in organic greenhouses in Europe. BioGreenhouse COST Action FA 1105. doi: 10.18174/373583
Tomasi, N., De Nobili, M., Gottardi, S., Zanin, L., Mimmo, T., Varanini, Z., et al. (2013). Physiological and molecular characterization of Fe acquisition by tomato plants from natural Fe complexes. Biol. Fertil. Soils 49, 187–200. doi: 10.1007/s00374-012-0706-1
Tomasi, N., Mimmo, T., Terzano, R., Alfeld, M., Janssens, K., Zanin, L., et al. (2014). Nutrient accumulation in leaves of Fe-deficient cucumber plants treated with natural Fe complexes. Biol. Fertil. Soils 50, 973–982. doi: 10.1007/s00374-014-0919-6
Tomasi, N., Pinton, R., Dalla Costa, L., Cortella, G., Terzano, R., Mimmo, T., et al. (2015a). New “solutions” for floating cultivation system of ready-to-eat salad: a review. Trends Food Sci. Technol. 46, 267–276. doi: 10.1016/j.tifs.2015.08.004
Tomasi, N., Pinton, R., Gottardi, S., Mimmo, T., Scampicchio, M., and Cesco, S. (2015b). Selenium fortification of hydroponically grown corn salad (Valerianella locusta). Crop Pasture Sci. 66, 1128–1136. doi: 10.1071/CP14218
Tomasi, N., Rizzardo, C., Monte, R., Gottardi, S., Jelali, N., Terzano, R., et al. (2009). Micro-analytical, physiological and molecular aspects of Fe acquisition in leaves of Fe-deficient tomato plants re-supplied with natural Fe-complexes in nutrient solution. Plant Soil 325, 25–38. doi: 10.1007/s11104-009-0069-z
Tripathi, D. K., Shweta,, Singh, S., Singh, S., Pandey, R., Singh, V. P., et al. (2017). An overview on manufactured nanoparticles in plants: uptake, translocation, accumulation and phytotoxicity. Plant Physiol. Biochem. 110, 2–12. doi: 10.1016/J.PLAPHY.2016.07.030
Tripathi, D. K., Singh, S., Singh, S., Dubey, N. K., and Chauhan, D. K. (2016). Impact of nanoparticles on photosynthesis: challenges and opportunities. Mater. Focus 5, 405–411. doi: 10.1166/mat.2016.1327
Valentinuzzi, F., Pii, Y., Mimmo, T., Savini, G., Curzel, S., and Cesco, S. (2018). Fertilization strategies as a tool to modify the organoleptic properties of raspberry (Rubus idaeus L.) fruits. Sci. Hortic. 240, 205–212. doi: 10.1016/j.scienta.2018.06.024
Valentinuzzi, F., Pii, Y., Vigani, G., Lehmann, M., Cesco, S., and Mimmo, T. (2015). Phosphorus and iron defciencies induce a metabolic reprogramming and affect the exudation traits of the woody plant Fragaria×ananassa. J. Exp. Bot. 66, 6483–6495. doi: 10.1093/jxb/erv364
Van der Bruggen, B., and Luis, P. (2015). Forward osmosis: understanding the hype. Rev. Chem. Eng. 31, 1–12. doi: 10.1515/revce-2014-0033
Van Ginkel, S. W., Igou, T., and Chen, Y. (2017). Energy, water and nutrient impacts of California-grown vegetables compared to controlled environmental agriculture systems in Atlanta, GA. Resour. Conserv. Recycl. 122, 319–325. doi: 10.1016/j.resconrec.2017.03.003
Vardar, G., Altıkatoğlu, M., Ortaç, D., Cemek, M., and Işıldak, İ. (2015). Measuring calcium, potassium, and nitrate in plant nutrient solutions using ion-selective electrodes in hydroponic greenhouse of some vegetables. Biotechnol. Appl. Biochem. 62, 663–668. doi: 10.1002/bab.1317
Verones, F., Pfister, S., van Zelm, R., and Hellweg, S. (2017). Biodiversity impacts from water consumption on a global scale for use in life cycle assessment. Int. J. Life Cycle Assess. 22, 1247–1256. doi: 10.1007/s11367-016-1236-0
von Wirén, N., and Merrick, M. (2004). “Regulation and function of ammonium carriers in bacteria, fungi, and plants” in Molecular mechanisms controlling transmembrane transport SE – 3 topics in current genetics. eds. E. Boles and R. Krämer (Berlin/Heidelberg: Springer), 95–120.
Weng, H. X., Hong, C. L., Yan, A. L., Pan, L. H., Qin, Y. C., Bao, L. T., et al. (2008). Mechanism of iodine uptake by cabbage: effects of iodine species and where it is stored. Biol. Trace Elem. Res. 125, 59–71. doi: 10.1007/s12011-008-8155-2
White, P. J. (2003). “Ion transport” in Encyclopaedia of applied plant sciences. eds. B. Thomas, D. J. Murphy, and B. G. Murray (London: Academic Press), 625–634.
Wiesner-Reinhold, M., Schreiner, M., Baldermann, S., Schwarz, D., Hanschen, F. S., Kipp, A. P., et al. (2017). Mechanisms of selenium enrichment and measurement in brassicaceous vegetables, and their application to human health. Front. Plant Sci. 8:1365. doi: 10.3389/fpls.2017.01365
Wild, A., Jones, L. H. P., and Macduff, J. H. (1987). Uptake of mineral nutrients and crop growth: the use of flowing nutrient solutions. Adv. Agron. 41, 171–219. doi: 10.1016/S0065-2113(08)60805-6
Yasuor, H., Tamir, G., Stein, A., Cohen, S., Bar-Tal, A., Ben-Gal, A., et al. (2017). Does water salinity affect pepper plant response to nitrogen fertigation? Agric. Water Manag. 191, 57–66. doi: 10.1016/j.agwat.2017.05.012
Yolanda, D., Hindersah, H., Hadiatna, F., and Triawan, M. (2016). “Implementation of real-time fuzzy logic control for NFT-based hydroponic system on Internet of Things environment” in 6th International Conference on System Engineering and Technology (ICSET). (Bandung, Indonesia), 153–159.
Zaimenko, N. V., Didyk, N. P., Dzyuba, O. I., Zakrasov, O. V., Rositska, N. V., and Viter, A. V. (2014). Enhancement of drought resistance in wheat and corn by nanoparticles of natural mineral analcite. Ecol. Balk. 6, 1–10. Available at: http://eb.bio.uni-plovdiv.bg (Accessed May 15, 2019).
Zamboni, A., Zanin, L., Tomasi, N., Avesani, L., Pinton, R., Varanini, Z., et al. (2016). Early transcriptomic response to Fe supply in Fe-deficient tomato plants is strongly influenced by the nature of the chelating agent. BMC Genomics 17:35. doi: 10.1186/s12864-015-2331-5
Zanin, L., Tomasi, N., Cesco, S., Varanini, Z., and Pinton, R. (2019). Humic substances contribute to plant iron nutrition acting as chelators and biostimulants. Front. Plant Sci. 10:675. doi: 10.3389/fpls.2019.00675
Zekki, H., Gauthier, L., and Gosselin, A. (2019). Growth, productivity, and mineral composition of hydroponically cultivated greenhouse tomatoes, with or without nutrient solution recycling. J. Am. Soc. Hortic. Sci. 121, 1082–1088. doi: 10.21273/jashs.121.6.1082
Zhang, F., Niu, J., Zhang, W., Chen, X., Li, C., Yuan, L., et al. (2010). Potassium nutrition of crops under varied regimes of nitrogen supply. Plant Soil 335, 21–34. doi: 10.1007/s11104-010-0323-4
Zhang, H., Sun, Y., Xie, X., Kim, M.-S., Dowd, S. E., and Paré, P. W. (2009). A soil bacterium regulates plant acquisition of iron via deficiency-inducible mechanisms. Plant J. 58, 568–577. doi: 10.1111/j.1365-313X.2009.03803.x
Zhang, Z., Zhang, J., Chen, Y., Li, R., Wang, H., and Wei, J. (2012). Genome-wide analysis and identification of HAK potassium transporter gene family in maize (Zea mays L.). Mol. Biol. Rep. 39, 8465–8473. doi: 10.1007/s11033-012-1700-2
Zhao, F.-J., McGrath, S. P., and Meharg, A. A. (2010). Arsenic as a food chain contaminant: mechanisms of plant uptake and metabolism and mitigation strategies. Annu. Rev. Plant Biol. 61, 535–559. doi: 10.1146/annurev-arplant-042809-112152
Zhao, L., Wang, F., Zhang, Y., and Zhang, J. (2014). Involvement of Trichoderma asperellum strain T6 in regulating iron acquisition in plants. J. Basic Microbiol. 54, S115–S124. doi: 10.1002/jobm.201400148
Zhu, Y. G., Huang, Y. Z., Hu, Y., and Liu, Y. X. (2003). Iodine uptake by spinach (Spinacia oleracea L.) plants grown in solution culture: effects of iodine species and solution concentrations. Environ. Int. 29, 33–37. doi: 10.1016/S0160-4120(02)00129-0
Zuchi, S., Cesco, S., and Astolfi, S. (2012). High S supply improves Fe accumulation in durum wheat plants grown under Fe limitation. Environ. Exp. Bot. 77, 25–32. doi: 10.1016/j.envexpbot.2011.11.001
Keywords: nutrient acquisition, biofortification, nutrient interaction, plant growth-promoting rhizobacteria, nanoparticles, sensors, smart agriculture
Citation: Sambo P, Nicoletto C, Giro A, Pii Y, Valentinuzzi F, Mimmo T, Lugli P, Orzes G, Mazzetto F, Astolfi S, Terzano R and Cesco S (2019) Hydroponic Solutions for Soilless Production Systems: Issues and Opportunities in a Smart Agriculture Perspective. Front. Plant Sci. 10:923. doi: 10.3389/fpls.2019.00923
Received: 10 April 2019; Accepted: 01 July 2019;
Published: 24 July 2019.
Edited by:
Victoria Fernandez, Polytechnic University of Madrid, SpainReviewed by:
Md Asaduzzaman, Bangladesh Agricultural Research Institute, BangladeshCopyright © 2019 Sambo, Nicoletto, Giro, Pii, Valentinuzzi, Mimmo, Lugli, Orzes, Mazzetto, Astolfi, Terzano and Cesco. This is an open-access article distributed under the terms of the Creative Commons Attribution License (CC BY). The use, distribution or reproduction in other forums is permitted, provided the original author(s) and the copyright owner(s) are credited and that the original publication in this journal is cited, in accordance with accepted academic practice. No use, distribution or reproduction is permitted which does not comply with these terms.
*Correspondence: Stefano Cesco, c3RlZmFuby5jZXNjb0B1bmliei5pdA==
Disclaimer: All claims expressed in this article are solely those of the authors and do not necessarily represent those of their affiliated organizations, or those of the publisher, the editors and the reviewers. Any product that may be evaluated in this article or claim that may be made by its manufacturer is not guaranteed or endorsed by the publisher.
Research integrity at Frontiers
Learn more about the work of our research integrity team to safeguard the quality of each article we publish.