- 1College of Resources and Environment, Shandong Agricultural University, Tai’an, China
- 2Shandong Provincial Engineering and Technology Research Center for Phyto-Microremediation in Saline-Alkali Land, Shandong, China
- 3Shandong Provincial Animal Husbandry General Station, Shandong Province Grass Products Quality Inspection Center, Jinan, China
Photosynthesis plays an essential role in plant growth and crop yield, and the mechanisms of the effects of cadmium (Cd) on photosynthetic performance require more attention. The acute toxicity of Cd in soil to the photosynthetic capacity of Hybrid Pennisetum was evaluated using gas exchange parameters, A/Ci curves, light response curves, and chlorophyll a fluorescence transients after exposure to elevated Cd concentrations (0, 10, 20, 50, 70, and 100 mg kg−1) for a 3-month period. The results indicated that leaf Cd concentration in Hybrid Pennisetum increased with the strength of soil Cd stress and ranged from 4.9 to 15.8 μg g−1 DW. The accumulation of leaf Cd severely restricted photosynthesis and its non-stomatal limitation in regulating the photosynthetic performance of Hybrid Pennisetum. The leaf chloroplasts at 10 and 20 mg kg−1 Cd concentrations showed no noticeable change, but the chlorophyll content significantly decreased by 9.0–20.4% at 50–100 mg kg−1 Cd concentrations. The Cd treatments also decreased plant ribulose-1,5-bisphosphate (RuBP) activity (Vcmax) and regeneration capacity (Jmax), triose phosphate utilization (TPU), light-saturated photosynthesis (Amax), apparent quantum yield (AQY), light saturation point (LSP), and dark respiration (Rday), but Cd treatment increased the light compensation point (LCP). The shape of chlorophyll a fluorescence transients in leaves was altered under different Cd treatments. The increased OJ phase and the decreased IP phase in fluorescence induction curves suggested that Cd toxicity inhibited both light use efficiency and photodamage avoidance ability. These results suggested that the decrease in photosynthesis through exposure to Cd may be a result of the decrease in leaf chlorophyll content, Rubisco activity, and RuBP regeneration, inhibition of triose phosphate utilization, reduction of the ability to use light and provide energy, and restrictions on electron transport in PSII.
Introduction
With the increasing anthropogenic activities of phosphate fertilizer abuse and sewage sludge, herbicide and pesticide application, excessive industrial and aquaculture wastewater for being used for irrigation, as well as a high frequency of mining and smelting, contaminated soil polluted by heavy metals is attracting increasing attention worldwide (Mcintyre, 2003; Rizwan et al., 2017). Cadmium (Cd), as one of the most harmful heavy metals in soils, has been widely accepted to be an extremely dangerous pollutant due to its acute toxicity, high water solubility, non-degradability, and persistence inside most live organisms (Ghosh and Singh, 2005; Groppa et al., 2012; Parmar et al., 2013). Studies have found that Cd can be more easily taken up by many plant species than most other heavy metals (Gallego et al., 2012; Yu et al., 2017). Excessive accumulation of Cd2+ in plant tissues could cause serious phytotoxicity (Groppa et al., 2007) and numerous morphological, physiological, and biochemical toxic effects on plant growth and development (Lutts and Lefèvre, 2015; Wali et al., 2015), such as destroying leaf chlorophyll structure (Santos et al., 2018), depressing photosynthesis and respiration (Hendrik et al., 2010; Lee et al., 2010; Zhang et al., 2015), disturbing the uptake and translocation of mineral nutrients (Erdal and Turk, 2016), accumulating reactive oxygen species (ROS) (Gallego et al., 2012), restraining protein synthesis and enzymatic activity (Fornazier, 2002; Wu et al., 2015), dwarfing plants (Rascio et al., 2008), inhibiting the growth of roots (Vartika et al., 2005), decreasing biomass (Rascio et al., 2008), and even leading to plant death if Cd exceeds their resistance values (Clemens et al., 2013; Shanying et al., 2017).
Among all the physiological and biochemical processes in plants, photosynthesis, as a central carbon anabolic pathway providing energy-rich organic compounds, plays an essential role in maintaining the equilibrium between the light energy absorbed by photosystems and the chemical energy consumed by metabolic sinks (Brestic et al., 2015). The efficiency of photosynthesis has a direct relationship with plant growth and development (Kalaji et al., 2017). Photosynthesis is also a process that is highly sensitive to any changes in environmental conditions. Actually, it has been proven to be a primary target of inhibition caused by metal-induced stress (Joshi and Mohanty, 2004). Any structural and functional alterations in the photosynthetic apparatus will adversely affect physiological activities and likely affect plant growth and survival (Baek et al., 2004). Therefore, a deep understanding of the responses of plant photosynthetic performance to heavy metal stress is necessary to scientifically address the problems of soil contamination.
The deleterious effects of Cd stress on photosynthetic attributes occur in various ways. Cd stress inhibits the biosynthesis of chlorophylls (Stephen et al., 2007; Shukla et al., 2008) and their stable binding to proteins (Horváth et al., 1996), causing stomatal opening (Sandalio et al., 2001), damaging light-harvesting complex II and photosystems (Hendrik et al., 2010), decreasing the transcription of photosynthesis-related genes such as psbA, psaB, and rbcL (Qian et al., 2009), thereby inducing a considerable reduction in the quantum yields of both photosystem I (PSI) and photosystem II (PSII) and the electron transport rate and slowing down the net photosynthetic rate (Goussi et al., 2018). PSII of photosynthesis is the primary target of Cd toxicity (Alexander et al., 2008; Popova et al., 2009). Cadmium may arrest the photosynthetic electron flow (Voigt and Nagel, 2002), inhibit the water-splitting complex of the oxidizing site of PS II (Nirupama and Mohn, 2003), or competitively bind to the essential Ca2+ site in PSII during photoactivation (Faller et al., 2005). Furthermore, Cd has also been shown to inhibit energy utilization and carbon sequestration by slowing down the activity of various enzymes, such as ribulose-1,5-bisphosphate (RuBP) carboxylase oxygenase (Mobin and Khan, 2007), phosphoenolpyruvate carboxylase (Alexander et al., 2008), aldolase (Sheoran et al., 1990), fructose-6-phosphate kinase (Malik et al., 1992), fructose-1,6-bisphosphatase (Sheoran et al., 1990), NADP+-glyceraldehyde-3-phosphate dehydrogenase (Sheoran et al., 1990), and carbonic anhydrase (Mobin and Khan, 2007).
Although great progress has been made in understanding the Cd hazard to plant photosynthetic performance (Li et al., 2013), the effects of Cd ions on photosynthetic performance varied with different Cd concentrations, plant species, and stress tolerance degrees (Ghnaya et al., 2005; Mobin and Khan, 2007; Wali et al., 2016, 2017). Therefore, the disturbance mechanisms of Cd on photosynthesis still need to be studied further. Hybrid Pennisetum is a high-stalk perennial grass and is widely planted in south China for the remarkable advantages in fast growth, high biomass, and high ability in endure adverse stress conditions. However, the Cd risk to Hybrid Pennisetum has seldom been explored. The aims of this work were to explore the changes in photosystem efficiency and photosynthetic activity in the leaves of Hybrid Pennisetum treated with different amounts of Cd. In the present study, leaf gas exchange parameters, light response curves, CO2 response curves, and chlorophyll a fluorescence transients were determined to examine the photosynthetic responses of Hybrid Pennisetum to soil Cd stress.
Materials and Methods
Plant Material and Growth Conditions
The pot experiment was conducted in a greenhouse at the Experimental Station of Shandong Agricultural University, Tai’an, China (36°09′N, 117°09′E). Growing conditions in the greenhouse were maintained as follows: 27/22°C temperature (day/night), a 14 h photoperiod per day, 65–75% relative humidity, and 1000 mol⋅m−2 day−1 of average daily photosynthetically active radiation (PAR).
The seeds of Hybrid Pennisetum (cv. Ningza NO. 4), kindly provided by Shandong Provincial Animal Husbandry General Station, were used in this study. Healthy seeds were surface-sterilized in 10% H2O2 for 10 min and cleaned with sterile deionized water three times. Seeds were then sown in plastic pots (8 cm in height, 10 cm in diameter) containing soil composed of peat and vermiculite (4:1, w/w) mixed with sand (3:1, w/w) for germination.
Experimental Designs
Six treatments (0, 10, 20, 50, 70, and 100 mg⋅kg−1 Cd) with four replicates were set up and designated as Cd0, Cd10, Cd20, Cd50, Cd70, and Cd100, respectively. Each plastic pot (40 cm in height and 48 cm in diameter) was filled with 20.0 kg of air-dried soil (sieved through 2 cm mesh) that was retrieved from the local surface soil (0–20 cm) and then saturated with a heavy metal solution containing the required amount of CdCl2⋅2.5H2O. The soil basic physical and chemical properties were as follows: soil texture of 73.8% sand, 12.1% silt, and 14.1% clay, soil pH of 6.67, organic matter of 13.52 g kg−1, available Cd concentration of 0.08 mg kg−1, total nitrogen of 75.62 mg kg−1, available phosphorus of 67.38 mg kg−1, and available potassium of 80.12 mg kg−1. After the third leaf emerged (approximately 2 weeks after sowing), five uniform seedlings were transferred to Cd-treated plastic pots. All pots were watered each day to keep the soil moisture at 75–85% with deionized water. To avoid nutrient deficiency, the soil was supplied with urea (0.20 g N kg−1), diammonium phosphate (0.15 g P2O5 kg−1) and potassium sulfate (0.20 g K2O kg−1) as basal fertilizers in each pot. All the following measurements were conducted after three months of plant growth with Cd treatments. Each plant was tested starting from the treatment of Cd0–Cd100 and again starting from the treatment of Cd0 to reduce the differences caused by the determination time.
Chlorophyll Content Measurements
The SPAD values of chlorophyll were determined with a portable chlorophyll meter (SPAD-502, Osaka 590-8551, Japan). Leaf samples were obtained from new and fully expanded leaves from the tip, with 15 plant replicates for each treatment.
Gas Exchange Measurements
The second fully expanded young leaves from the tops of the plants were used to estimate the net photosynthetic rate (Pn), stomatal conductance (Gs), intercellular CO2 concentration (Ci), transpiration rate (Tr), and water use efficiency (WUE = Pn/Tr) using a portable photosynthesis system (CIRAS-3, PP-system, Hitchin, United Kingdom). The experiment was performed on sunny days from 9:00 to 11:00 am and 14:00 to 16:00 pm. The illumination was supplied to the leaves from a red-blue LED light source. Leaf chamber temperature was maintained at room temperature with a CO2 concentration of 390 ppm and a photosynthetic photon flux density (PPFD) of 1000 mol photon⋅m−2⋅s−1. These measurements were performed on three plants in each Cd treatment.
Light Response Curves
Light response curves were measured in the same leaf that was chosen for gas exchange parameter measurement with a portable photosynthesis system (CIRAS-3, PP-system, Hitchin, United Kingdom) under constant leaf temperature (25°C) and CO2 concentration (390 mol⋅mol−1). The leaves were placed under 300 mol photon⋅m−2⋅s−1 for 15 min to fully activate the photosynthesis systems. The light series of photosynthetic light flux density were set as follows: 1600, 1200, 800, 600, 400, 200, 150, 100, 50, and 0 mol photon⋅m−2⋅s−1. All of the measurements were carried out from 9:00 to 11:00 am and 14:00 to 16:00 pm on sunny days. Three plants were measured each time.
The responses of net photosynthetic rate to different light levels were modeled using the non-rectangular hyperbola in the Farquhar model (Prioul and Chartier, 1977):
where Pn is the net photosynthetic rate (μmol⋅CO2 m−2⋅s−1), Amax is the light-saturated rate of CO2 accumulation (μmol⋅CO2 m−2⋅s−1), I is the photosynthetic photon flux density (μmol⋅m−2⋅s−1), AQY is the leaf maximum apparent quantum yield of CO2 uptake, θ is the convexity of the light response curve, and Rd is the dark respiration (μmol⋅m−2⋅s−1). The quantum yield could be estimated from the initial slope by fitting linear regression to the low-photon flux data (less than 200 μmol⋅m−2⋅s−1) of the light response curve. The intersection point of the straight line with the X-axis corresponded to LCP (the light compensation point, μmol⋅m−2⋅s−1). The projection of Amax to the X-axis corresponded to LSP (the light saturation point, μmol⋅m−2⋅s−1).
A/Ci Curves
In the same leaves, the response of light-saturated CO2 assimilation to variable internal CO2 concentrations (A/Ci curves) was measured as described in Guidi et al. (2008). Pn was measured at CO2 concentrations of 50, 100, 200, 300, 360, 400, 600, 800, 1000, 1300, 1600, and 2000 mol⋅mol−1 at photosynthesis saturating irradiance 400 mol⋅photon⋅m−2⋅s−1. From the A/Ci curves, the following photosynthetic parameters were calculated according to Long and Bernacchi (2003): maximum carboxylation (Vcmax), maximum rate of RuBP regeneration (Jmax), and triose phosphate utilization (TPU). A buffer bottle was used to control the concentrations of CO2.
Measurements of Leaf OJIP Transients
The fast Chl fluorescence induction kinetics curve was measured using a Handy-PEA chlorophyll fluorometer (Hansatech, United Kingdom). The leaves used to measure the net photosynthetic rate were dark-adapted for 30 min before determination of the minimal (F0) and maximal (Fm) fluorescence yields. The fluorescence intensity was measured from 20 μs (initial fluorescence F0) to 1 s. Biolyzer HP3 software (Bioenergetics Lab., Geneva, Switzerland) was used to analyze the energy pipeline models and specific energy fluxes.
Determination of Leaf Cd Content
Leaf samples were washed with deionized water and oven-dried at 105°C for 30 min and then 75°C until a constant weight was achieved and maintained. A ground sample of 0.250 g was microwave-digested (CEM, MARS5, United States) with 8 mL of HNO3 at 180°C for 30 min. Then, the leaf Cd concentration was determined by ICP-MS (Agilent ICP-MS 7700ce, Agilent Technologies, Santa Clara, CA, United States).
Statistics Analysis
All of the parameters described above were measured 90 days after the plants were subjected to soil Cd treatments. SPSS 19.0 statistical software package (SPSS, Chicago, IL, United States) were used in the study to perform the statistical analyses. For each treatment, the mean values with standard deviation (±SD) are shown in the figures and tables. The parameters were analyzed by one-way analysis of variance (ANOVA, p < 0.05), followed by Duncan’s multiple range tests. The graphs were created using Origin 9.0 software (Origin Lab, United States).
Results
Cd Contents in Leaves
The Cd concentration in leaves of Hybrid Pennisetum exhibited remarkable differences among various Cd soil treatments (Figure 1). In the low soil Cd treatments (Cd10 and Cd20), the Cd concentration in leaves ranged from 4.9 to 15.8 μg g−1. With the increase in Cd added into the soil, the Cd concentration in leaves showed a sharp increase. At Cd100, the Cd concentration in leaves reaches 86.4 μg g−1, which is approximately 20 times higher than the Cd concentration in leaves from the Cd10 treatment.
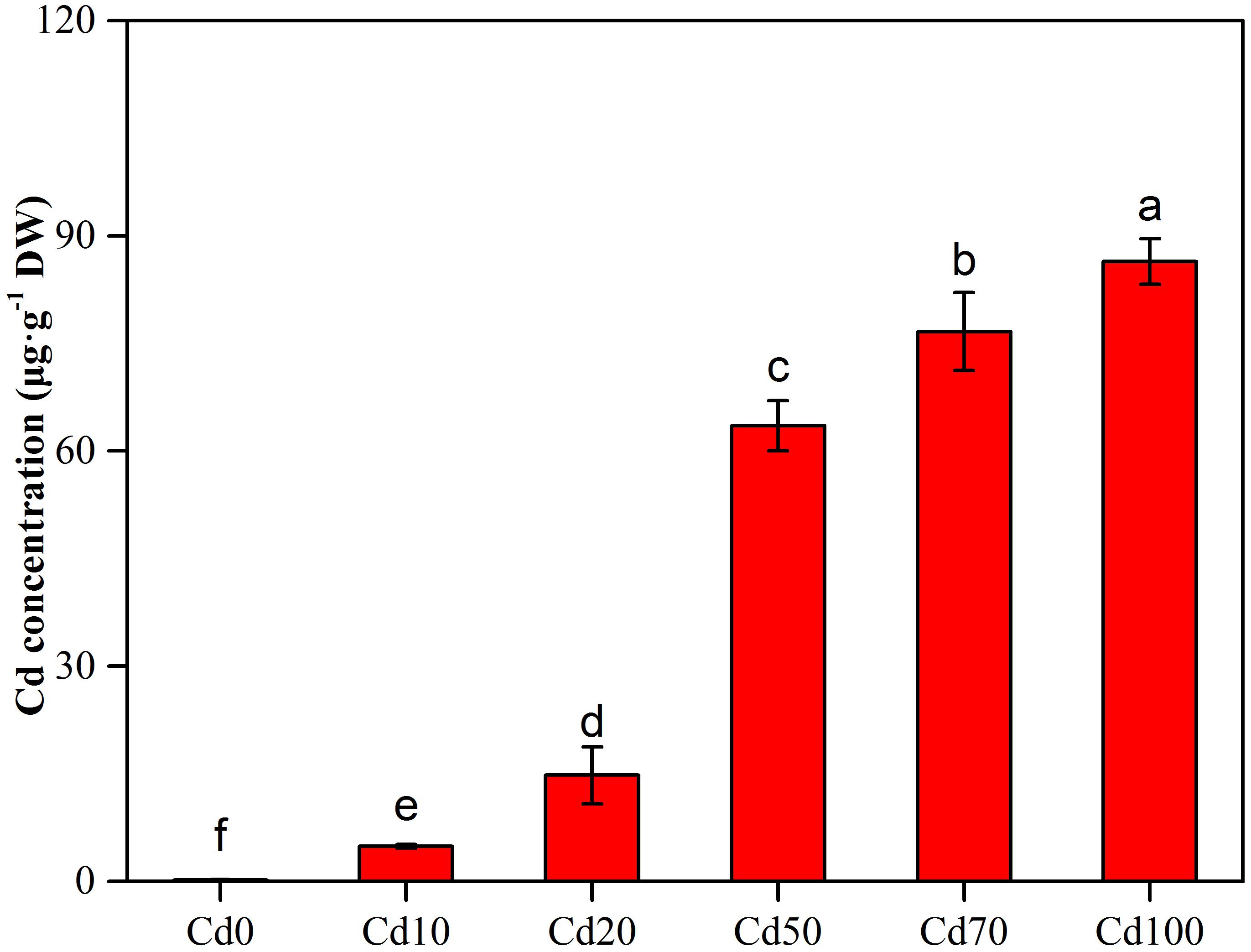
Figure 1. Effect of different concentrations of Cd concentration in leaves of Hybrid Pennisetum. Vertical bars represent ± SD of the mean (n = 3); different letters on the SD bars indicate significant differences among the Cd treatments (p < 0.05).
SPAD Values in Leaves
The SPAD chlorophyll values of Hybrid Pennisetum are shown in Figure 2. Compared to Cd0, the chlorophyll values of the leaves in Hybrid Pennisetum were not influenced by 10 to 20 mg kg−1 of Cd2+ pollution. With the increase in Cd added to the soil, the SPAD chlorophyll values significantly decreased. Compared to Cd0 plants, the SPAD values of Cd50, Cd70, and Cd100 plants decreased by 9.0, 9.6, and 20.4%, respectively.
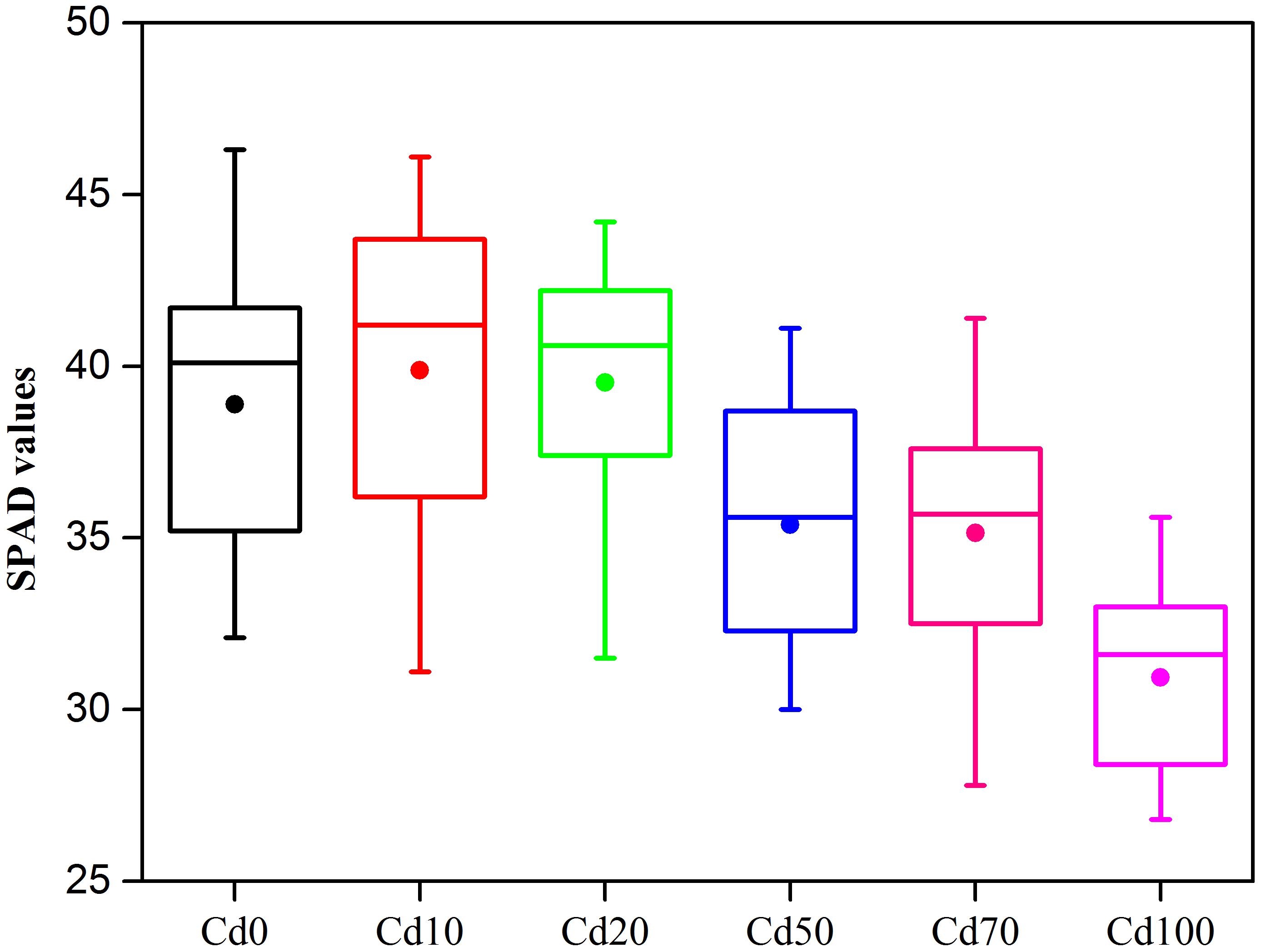
Figure 2. Boxplots of SPAD under different Cd stress. The solid circle in the box plots represents the mean values, n = 15.
Gas Exchange Parameters
Instantaneous gas exchange parameters corresponding to plants subjected to different soil Cd treatments are presented in Figures 3A–E. The maximum values of net CO2 assimilation rate (Pn, Figure 3A), stomatal conductance (Gs, Figure 3B), and water use efficiency (WUE, Figure 3E) found in Cd0 were 13.4 μmol⋅CO2 m−2⋅s−1, 92 mol⋅H2O m−2⋅s−1, and 6.7 μmol CO2 mmol⋅H2O−1, respectively. With the increase in soil Cd content, Pn, Gs, and WUE in leaves decreased significantly. The values of Pn, Gs, and WUE in Cd10, Cd20, Cd50, Cd70, and Cd100 were decreased from 10.7 to 0.7 μmol⋅CO2 m−2⋅s−1, 71.0 to 35.3 mol⋅H2O m−2⋅s−1, and 4.9 to 0.5 μmol⋅CO2 mmol⋅H2O−1, respectively. The intercellular CO2 concentration (Ci, Figure 3C) in leaves showed the opposite trend as Pn, Gs, and WUE. Ci in Cd0 and Cd10 had the lowest values: 118.3 and 126.3 μmol⋅mol−1, respectively. With the increase of soil Cd content, Ci in the Cd20, Cd50, Cd70, and Cd100 treatments ranged from 191.0 to 254.3 μmol⋅mol−1. However, based on the one-way ANOVA, Ci in high soil Cd content (Cd50, Cd70, and Cd100) plants showed no significant differences (p > 0.05, Figure 3C). The leaf transpiration rate (Tr, Figure 3D) in Cd0 plants was 2.0 mmol⋅m−1⋅s−1. Although compared to Cd0, Ci in Cd10, Cd20, and Cd50 plants increased 0.22, 0.17, and 0.07 mmol⋅m−1⋅s−1, respectively, there were no remarkable differences between them. With the increase in soil Cd content, Ci in Cd70 and Cd100 plants showed a significant decrease, and the lowest value was 1.3 mmol⋅m−1⋅s−1 in Cd100 plants.
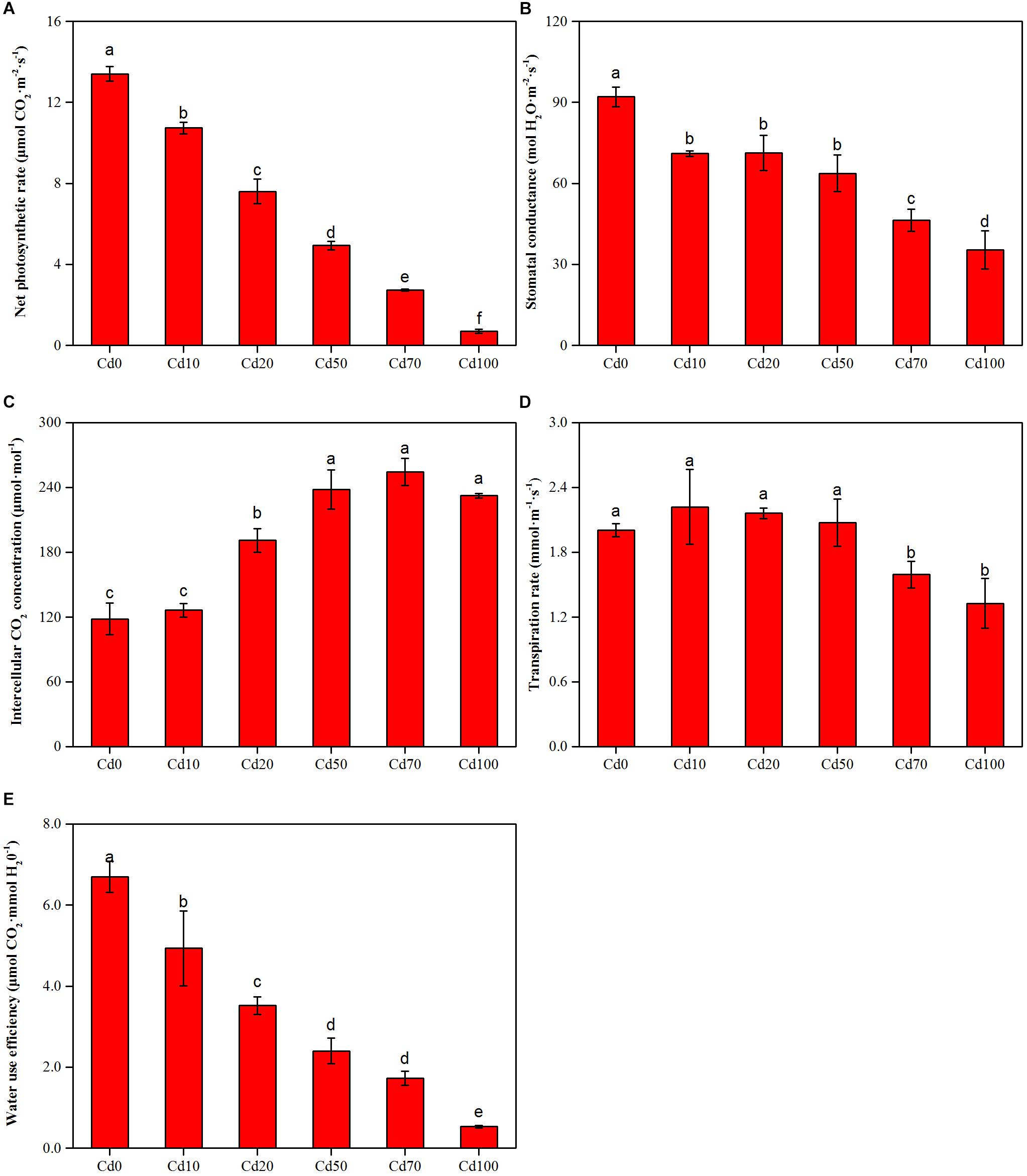
Figure 3. Net photosynthetic rate (A), Stomatal conductance (B), Intercellular CO2 concentration (C), Transpiration rate (D), and Water use efficiency (E) of Hybrid Pennisetum leaves treated with different doses of Cd concentration in soil. Vertical bars represent ± SD of the mean (n = 3); different letters on the SD bars indicate significant differences among the Cd treatments (p < 0.05).
Leaf Photosynthetic CO2 Response Parameters
The values for the maximum rate of Rubisco carboxylation (Vcmax), the maximum rate of RuBP regeneration (Jmax), and the triose phosphate utilization (TPU) fitted from A/Ci curves are shown in Figures 4A–C. The changes in Vcmax, Jmax, and TPU showed similar trends, decreasing with the enhancement of soil Cd content, and the highest values of Vcmax, Jmax, and TPU were found in Cd0 plants (113.5, 138.9, and 8.2 μmol⋅m−2⋅s−1, respectively). Compared to Cd0 plants, Vcmax, Jmax, and TPU in Cd10, Cd20, Cd50, Cd70, and Cd100 plants decreased by 10.8–52.1, 15.1–64.6, and 2.4–5.7 μmol⋅m−2⋅s−1, respectively.
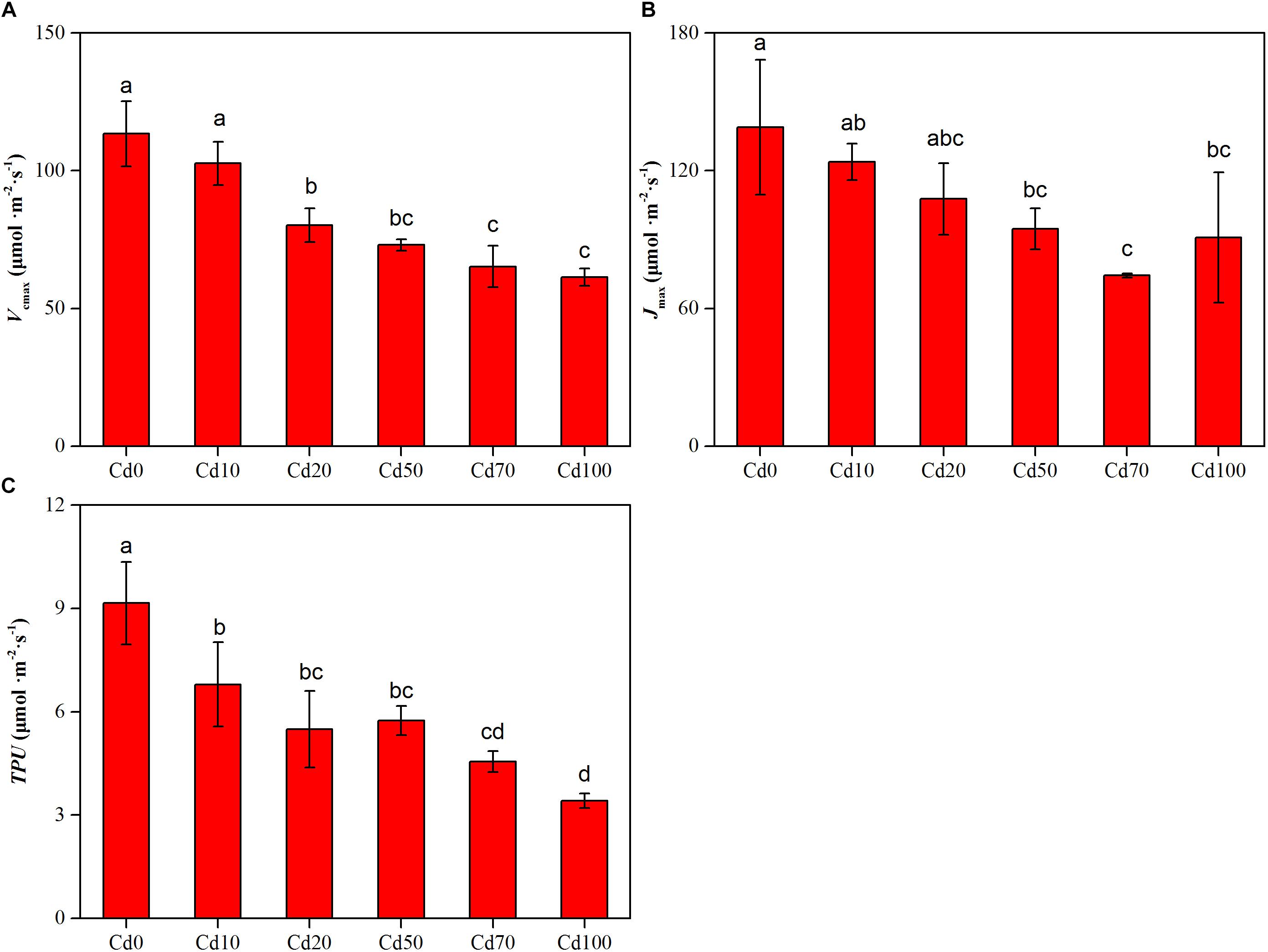
Figure 4. Effects of Cd treatment on the maximum velocity of Rubisco carboxylation (A), the maximum velocity of RuBP regeneration (B), and the triose phosphate utilization (C) in leaves of Hybrid Pennisetum. Vertical bars represent ± SD of the mean (n = 3); different letters on the SD bars indicate significant differences among the Cd treatments (p < 0.05).
Light Response Curves
The light response curves of Hybrid Pennisetum in various soil Cd treatments are illustrated in Figure 5. As shown in the light response curves, the response of Pn to the light intensity is significantly different during different soil Cd treatments. The Pn in leaves decreased with increasing Cd, and the differences between treatments were more obvious with increasing irradiance. When the level of PAR was higher than that of LCP, at the same PAR, the levels of Pn were Cd0 > Cd10 > Cd20 > Cd50 > Cd70 > Cd100. According to the simulated analysis of the light response curves (Table 1), Amax, Rday, AQY, LCP, and LSP in the leaves of Hybrid Pennisetum under Cd0 treatment were 20.7 μmol⋅CO2 m−2⋅s−1, 3.7 μmol⋅m−2⋅s−1, 0.064 mol⋅mol−1, 64.6 μmol⋅m−2⋅s−1, and 473.7 μmol⋅m−2⋅s−1, respectively. When plants suffered soil Cd stress, Amax, Rday, AQY, and LSP in the leaves of Hybrid Pennisetum decreased with the increase in soil Cd, but LCP in leaves that grew with high soil Cd was higher than the LCP of leaves that grew with low soil Cd. Compared with the Cd0 treatment, Amax, Rday, and AQY in Cd10, Cd20, Cd50, Cd70, and Cd100 leaves decreased by 30.0–81.6, 5.4–35.1, and 29.7–62.5%, respectively, whereas LCP increased by 38.1–130.0%. Although compared to Cd0, the value of LSP increased by 17.7 μmol⋅m−2⋅s−1 in Cd10 leaves and decreased by 4.8 μmol⋅m−2⋅s−1 in Cd20 leaves, there were no significant differences among those three treatments (p < 0.05). When the soil Cd content reached 50 mg kg−1, LSP showed a remarkable decrease and had the lowest value (409 μmol⋅m−2⋅s−1) in the leaves of the Cd100 treatment.
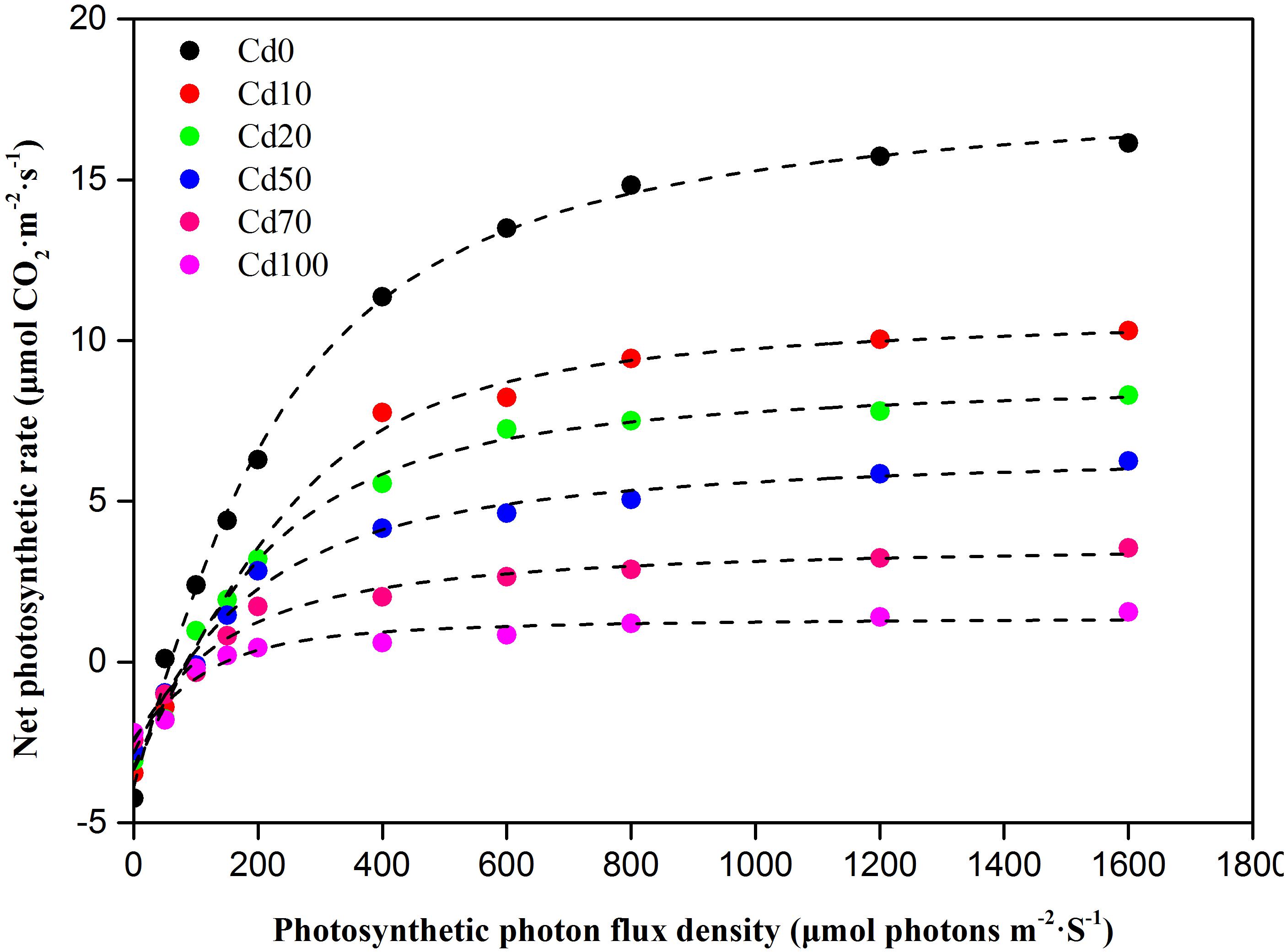
Figure 5. Photosynthetic light response curves in leaves of Hybrid Pennisetum under different soil Cd stress conditions.
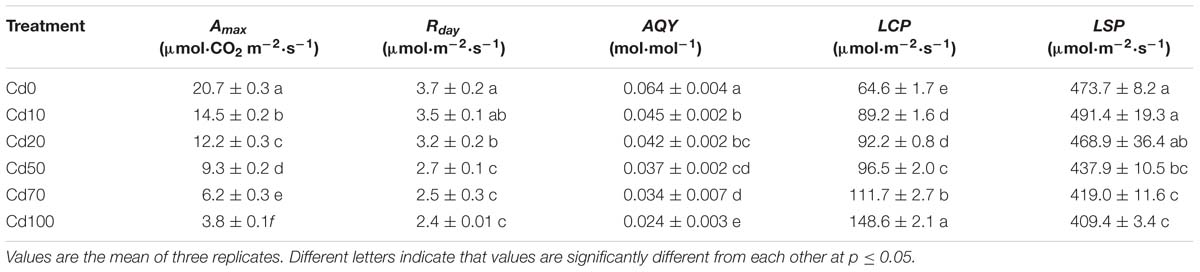
Table 1. Cd stress effect on light-saturated photosynthesis, apparent quantum yield, light compensation point, light saturation point, and dark respiration in leaves of Hybrid Pennisetum.
Chlorophyll a Fluorescence Transient Analysis
Chlorophyll a fluorescence induction kinetics was measured in order to evaluate the inhibitory effects of Cd on photochemical efficiency of PSII in Hybrid Pennisetum plants (Figure 6). The Chl a induction curves (O-J-I-P) obtained from different Cd treatments revealed that Cd stress caused a prominent change in the shape of fluorescence induction curve compared to control. At the O-J phase, a faster fluorescence increase was found in Cd-treated samples. With the reaction time go on, the curves from the I to P phase showed a significant decreased under Cd treatment.
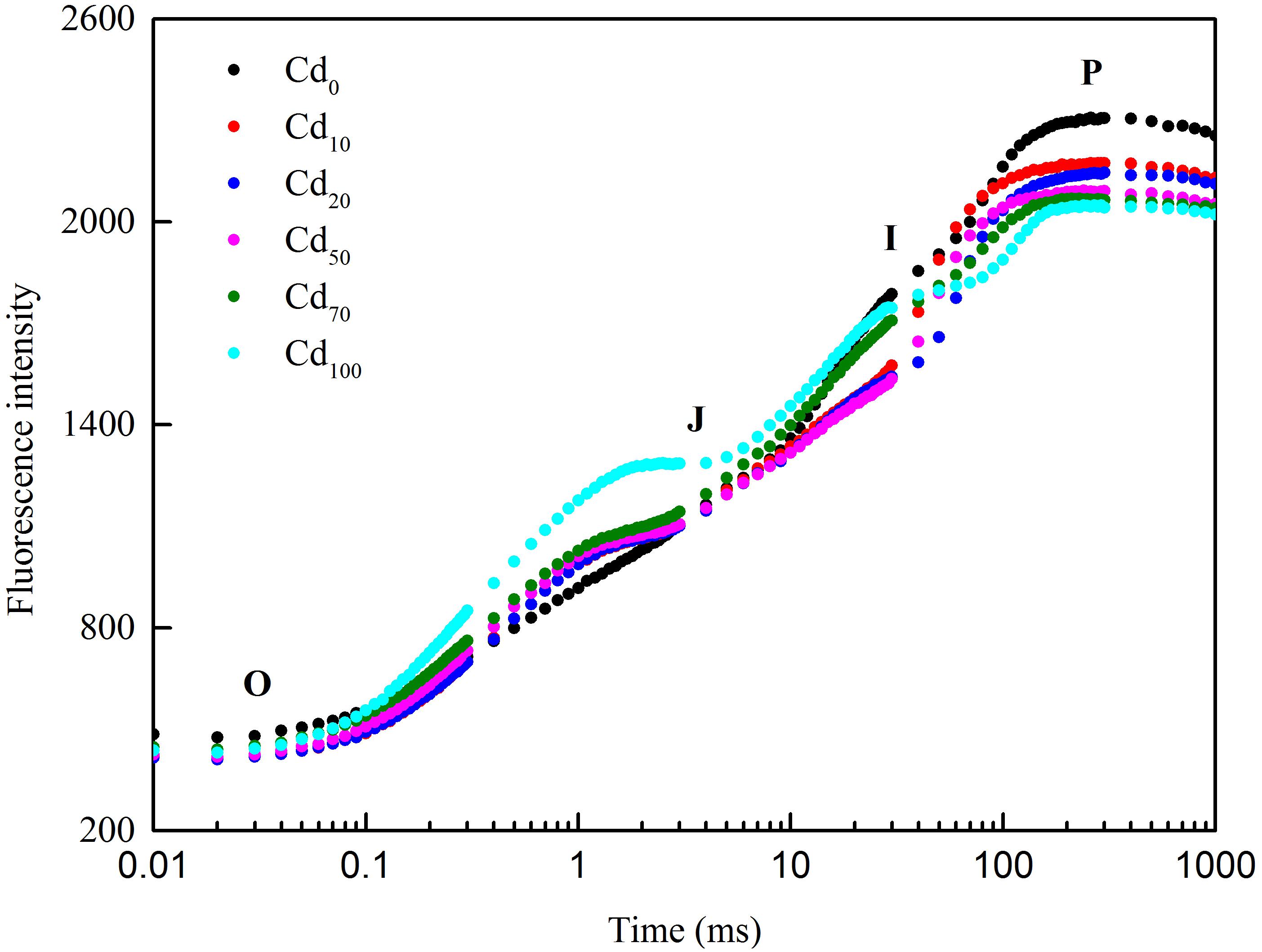
Figure 6. The fluorescence intensity of the original fluorescence kinetic curve of Hybrid Pennisetum leaves under different Cd treatments.
A leaf model of phenomenological energy fluxes (per cross-section) was used in the study to present the alteration of PSII energy fluxes in response to Cd stress (Figure 7). In the dynamic model, the energy fluxes such as ABS/CSm, TRo/CSm, ETo/CSm, and DIo/CSm, which were expressed by the width of corresponding arrows, indicate the efficiency of light absorption, trapping and electron transport, and dissipation per cross-section of PS II in different Cd treatments. In the present study, there was no significant difference in energy absorbed per excited cross-section (ABS/CSm), trapped energy flux per CS (TRO/CSm), and non-photochemical quenching (DIO/CSm) under all Cd treatments. In contrast, the reoxidation of reduced QA via electron transport over a cross-section of active and inactive RCs (ETo/CSm, indicated by a significantly smaller blue arrow) was reduced significantly in Hybrid Pennisetum leaves under Cd stress and gradually decreased with increasing soil Cd content. Furthermore, the decreased density of the active RCs (indicated as open circles) and the increased density of the inactive RCs (expressed by open closed circles) in the PS II cross-section under Cd stress conditions suggest that electron transport was inhibited because 18–23% of active RCs were converted into inactive RCs under Cd stress conditions.
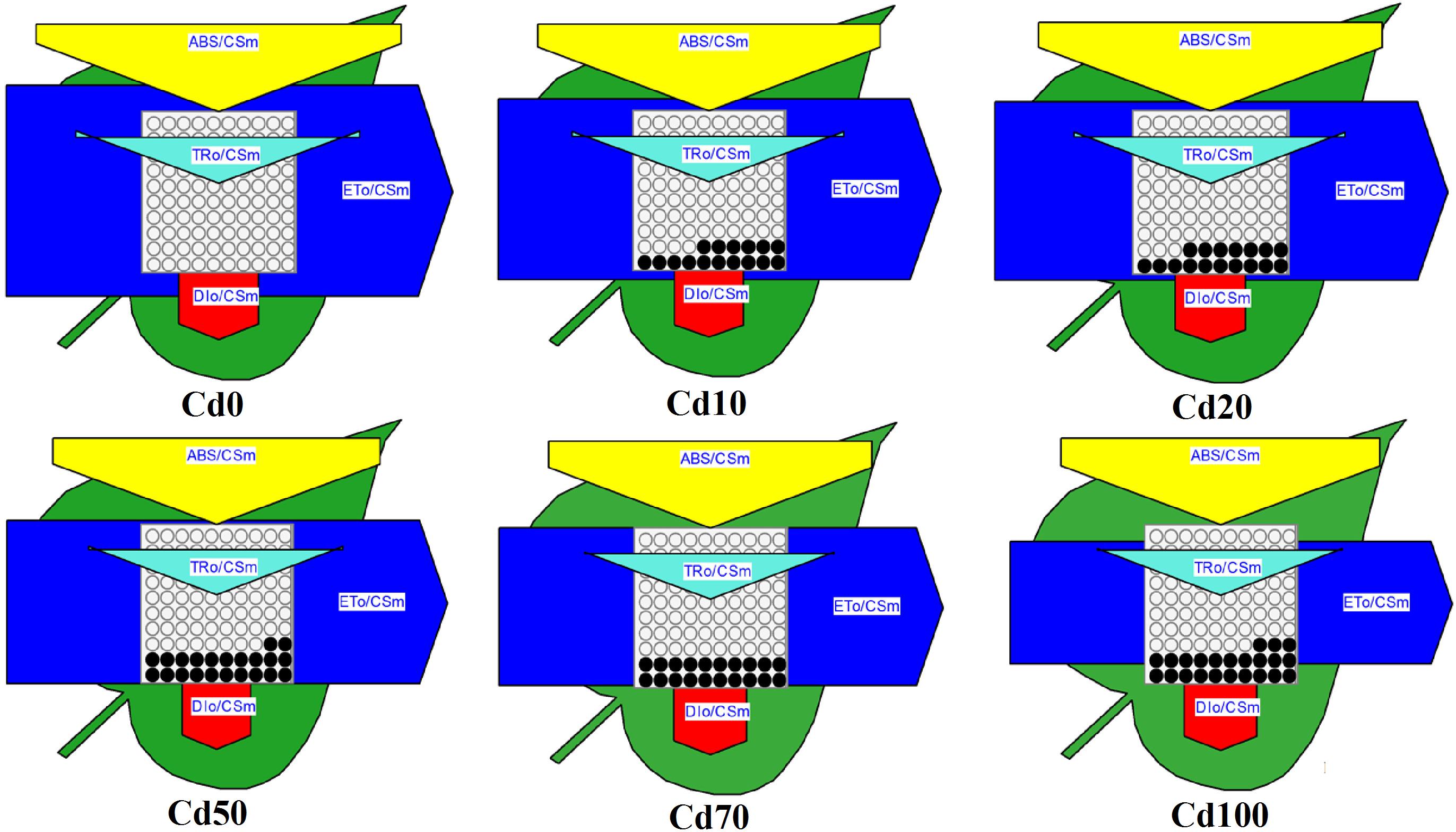
Figure 7. Energy pipeline leaf model of phenomenological fluxes (per cross-section, CS) in last fully expanded leaves as affected by Cd stress. Data are means ± standard errors (n = 3). Each relative value is drawn by the width of the corresponding arrow, standing for a parameter. Empty and full black circles indicate, respectively, the percentage of active (QA reducing) and non-active (non-QA reducing) reaction centers of PS II. TRO/CSm – trapped energy flux per CS; ETO/CSm – electron transport flux per CS; ABS/CSm – absorption flux per CS; DIO/CSm – non-photochemical quenching per CS.
Discussion
Phytoextraction is an important technique that has been widely used to remediate heavy metal contaminated soils by using accumulators to extract contaminants from soil into plants. An ideal phytoextraction plant should have a high metal bioaccumulation factor and a high metal tolerance (Zhao et al., 2003). In our study, there was significant Cd accumulation in the leaves of Hybrid Pennisetum, and the leaf Cd concentration exhibited a remarkable increase with the strength of soil Cd stress. The results indicated that Hybrid Pennisetum has a high ability to uptake Cd metal and quickly transfer Cd2+ from roots to leaves. According to the study of Lux et al. (2011), leaf Cd concentrations exceeding 5–10 μg g−1 are toxic to most plants. After 3 months of Cd treatments on Hybrid Pennisetum, the leaf Cd concentrations reached 63.5 μg g−1 at Cd50, 76.4 μg g−1 at Cd70, and 86.4 μg g−1 at Cd100 treatment. Although photosynthesis was extremely suppressed under high Cd treatments, Hybrid Pennisetum still had a low carbon assimilation ability (Pn in Cd50, Cd70, and Cd100 were 4.9, 2.7, and 0.7 μmol CO2 m−2⋅s−1, respectively).
Chloroplasts are pigments that play an important role in light energy absorption, transmission, and conversion to chemical energy during photosynthesis. The reduction of chlorophyll content and the inhibition of chlorophyll synthesis were proved to be the primary causes of diminished photosynthetic activity (Yasemin et al., 2008). In the present study, the progressive decreased photosynthetic activity in Hybrid Pennisetum was partially attributable to the remarkably reduced chloroplast contents due to exposure to Cd (Figure 2). Similar results were observed in a previous study in which chlorophyll and carotenoid concentrations in leaves decreased as a result of Cd stress (Su and Wang, 2004; Pagliano et al., 2006; Ahammed et al., 2012; Xu et al., 2014; Per et al., 2016). The chloroplast reduction could be explained by Cd replacing the magnesium atom within chlorophyll to form a chlorophyll-Cd complex. This replacement results in damage to the photosynthetic apparatus (Siedlecka et al., 1998) and inhibits the production of chlorophyll by obstructing the formation of the protochlorophyllide reductase ternary complex and the synthesis of 5-aminolaevulinic acid (Huang et al., 1997) and by reducing chlorophyll synthesis (Gonçalves et al., 2009) or enhancing enzymatic degradation (Somashekaraiah et al., 1992).
Cadmium toxicity caused a notable reduction in the photosynthetic rate in different plant species (Alexander et al., 2008). However, in other studies, no direct Cd effects on the photosystem were found in either Brassica juncea (Haag et al., 1999) or Arabidopsis thaliana (Laetitia et al., 2010) plants. These contrasting results could be explained by a threshold of phytotoxic concentration of Cd toxicity in plants that depends on plant species, ecotypes, cultivars, and even plant tissues (He et al., 2015). In our study, Cd caused a significant reduction in Pn (Figure 3A), indicating that plant carbon assimilation ability was suppressed due to Cd toxicity. Accompanied by the significant reduction in Pn, lower Gs (Figure 3B) and higher Ci (Figure 3C) were simultaneously observed in Hybrid Pennisetum leaves. The results indicated that the decrease in Pn did not result from a low Gs or low CO2 concentration in chloroplasts because the Ci levels were even higher in leaves of those plants treated with Cd than in the control, indicating that Cd reduced Pn by reducing CO2 fixation by Rubisco (Wahid et al., 2010). Therefore, the inhibition of photosynthetic processes by Cd in Hybrid Pennisetum was due to non-stomatal restriction but not stomatal limitation. These findings agree with the effect of Cd shown on CO2 fixation in cucumber (Feng et al., 2010), lettuce (Monteiro et al., 2009), and Ceratopteris pteridoides (Deng et al., 2014). Although the stomata were closed by Cd stress, the Tr of Hybrid Pennisetum in the Cd10, Cd20, and Cd50 treatments maintained a stable value (Figure 3D), indicating that the transpiration of Hybrid Pennisetum was not affected by the addition of 50 mg kg−1 Cd to the soil. On the contrary, Tr in Cd70 and Cd100 treatment decreased significantly due to the remarkable stomata closure. Cd stress also produced disturbances in water balance, and a noticeable reduction of WUE was observed in all Cd treatments (Figure 3E). This could be explained by the inhibition of absorption and translocation of water, as previously observed by Barceló and Poschenrieder (1990) and Singh et al. (2008). Figure 8 exhibits the non-stomatal limitation in regulating the photosynthetic performance of Hybrid Pennisetum under Cd stress conditions.
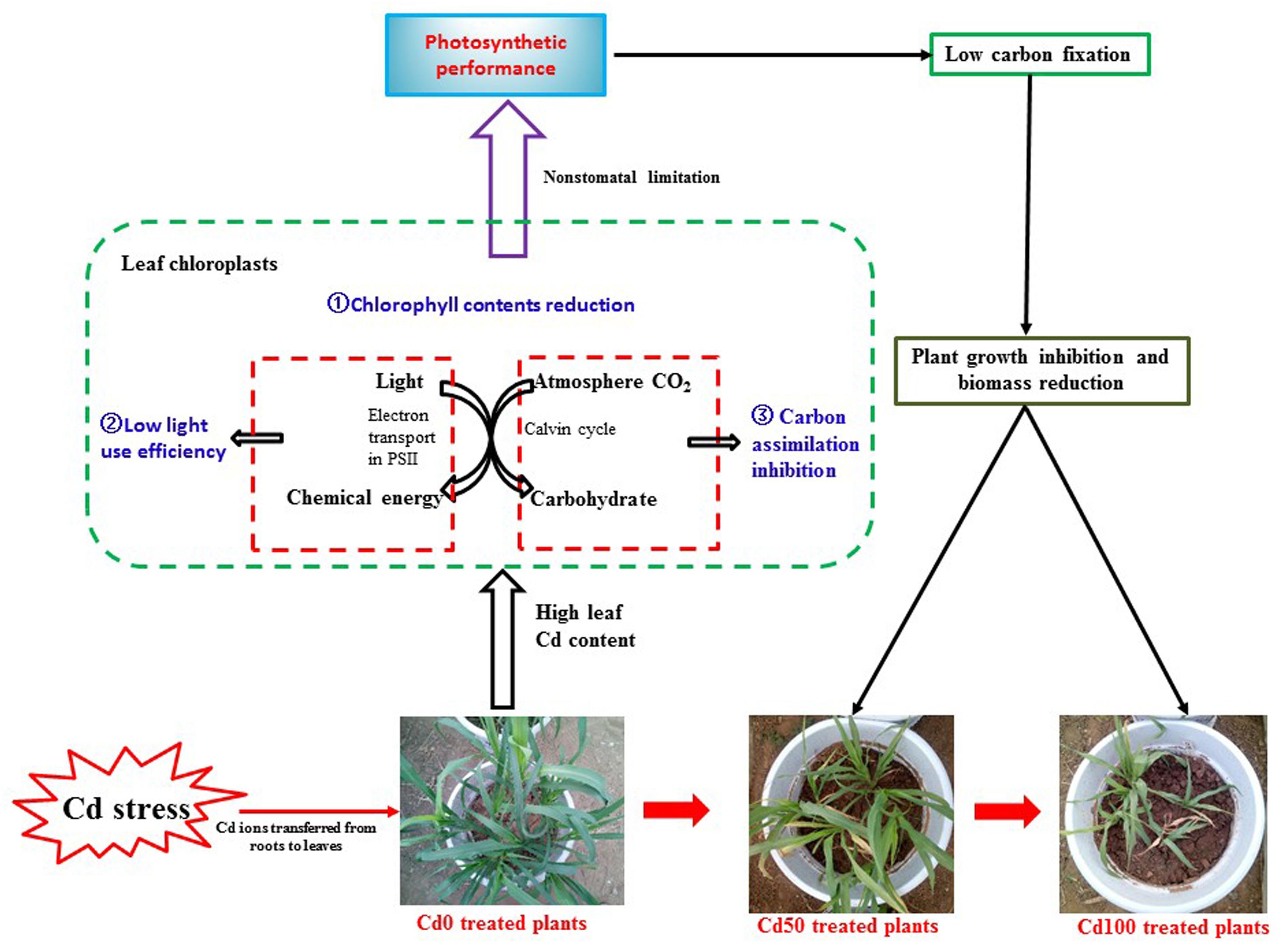
Figure 8. Schematic representation of the non-stomatal limitation in regulating the photosynthetic performance of Hybrid Pennisetum under Cd stress conditions. The figure shows that the decrease in leaf chlorophyll content, reduction of the ability to use light, and restrictions on carbon assimilation caused by high Cd content in leaves limit the capacity of Hybrid Pennisetum in carbon fixation.
The non-stomatal limitation of photosynthesis could be expressed by the change in photosynthetic capacity. The Vcmax at low CO2 concentrations and Jmax at high CO2 concentrations are often used to reflect the photosynthetic capacity of plants in response to environmental stress (Farquhar et al., 1980; Sharkey et al., 2007; Song et al., 2016). In our study, Cd stress caused a decrease in Vcmax and Jmax of Hybrid Pennisetum (Figure 4). The decline in the Vcmax might be due to the reduced amount of active Rubisco (Peña-Rojas et al., 2004), and the reduction in the Jmax might be due to the limited activity of photosynthetic enzyme (such as sedoheptulose-1,7-bisphosphatase and fructose-1,6-bisphosphatase) and the insufficient supply of NADPH or ATP (Lawlor and Cornic, 2010). The relationship between Vcmax and Jmax represents resource allocation between the two photosynthetic cycles-electron transport and the Calvin–Benson cycle (Walker et al., 2015). In this study, Jmax was strongly related to Vcmax, and neither leaf nitrogen, phosphorus, nor specific leaf area had a substantial impact on the relationship. Similar results were supported by the analysis of empirical data from 109 species (Wullschleger, 1993). As shown in Figure 9, although the changes in the two parameters were different among the various Cd treatments, there was a significant positive linear relationship between Jmax and Vcmax (p < 0.01). The result suggested the viewpoint of (Walker et al., 2015), that tight coupling of Jmax with Vcmax is a fundamental feature of plant photosynthetic trait relationships regardless of whether Hybrid Pennisetum suffered environmental stress. Triose phosphate utilization occurs when the chloroplast reactions have a higher rate than the capacity of the leaf to use the products of the chloroplasts (Sharkey et al., 2007). TPU is the rate of use of triose phosphates and it has been proposed to at least provide an indication of the feedback between growth and CO2 assimilation (Wullschleger, 1993). The drop in TPU in the Cd-treated plants (Figure 4) could be related to the decrease in the sink strength of the roots and leaves (Long and Bernacchi, 2003; Sharkey et al., 2007).
The light response curve has been widely used for reflecting the photochemical efficiency of plant photosynthesis in response to environmental factors such as drought (Song et al., 2016), elevated CO2 concentration (Herrick and Thomas, 1999), heat (Lewis et al., 1999), salt (Li and Ong, 1998), and metal stress (Zhang et al., 2014). The parameter Amax, which reflects the maximum photosynthetic capacity of the leaf (Zhou et al., 2010), is the maximum absolute value of photosynthesis under optimal environmental conditions (Tartachnyk and Blanke, 2004). In our study, Amax was significantly reduced by Cd stress. As shown in Table 1, compared to Cd0, Amax decreased by 6.2 μmol⋅CO2 m−2⋅s−1 even at a low Cd concentration (10 mg kg−1 in Cd10). AQY could be used to reflect the plant’s ability in absorbing, converting, and using light energy at low light intensities, and high AQY indicates that plants have a high light energy transfer efficiency (Marshall and Biscoe, 1980). In the present study, the change in AQY showed a similar trend as Amax and decreased with increasing Cd concentration. This decline in the light use efficiency of Hybrid Pennisetum shows plants’ self-photoprotection mechanism in avoiding damage (Zhu et al., 2004). Under light saturation conditions, Cd stress inhibited the photochemical reactions, resulting the excitation energy could not be used in photochemical reactions totally. To protect the photosynthetic apparatus like chloroplast and cell membrane from being damaged by the excess excitation energy, the plant activates the self-photoprotection mechanism by dissipating the excess excitation energy as harmless heat through the xanthophyll cycle (Omasa and Takayama, 2003). With more heat dissipation, the light use efficiency was lower and the decrease in the photosynthetic rate was higher (Song et al., 2016).
Light saturation point and LCP reflect the plant ability in using high and low light intensities. They are very useful primary indicators for measuring the relationship between light use and photosynthesis (Zhou et al., 2010). When the environmental conditions are not suitable, plants typically decrease the LSP or increase the LCP to ensure the normal operation of photosynthesis. In our study, compared to Cd0, LSP decreased and LCP increased with increasing soil Cd concentration (Figure 5 and Table 1), suggesting that soil Cd stress decreased the light use efficiency both at high light levels and at low light levels. The decreased light use efficiency at LSP showed the inhibition of CO2 assimilation ability in Hybrid Pennisetum. Furthermore, LCP is the light value at which the rate of CO2 release by respiration and photorespiration is equal to the rate of CO2 fixation by photosynthesis. High LCP reflects plants suffers higher respiration and lower Rubisco carboxylase activity or higher Rubisco oxygenase activity (Nunes et al., 2010). The obvious increase in LCP in Cd-treated plants observed in the present study implies that Cd could inhibit both photosynthesis and organic compounds accumulation in Hybrid Pennisetum (Zhang et al., 2014). Rd, which is the index of the plant respiration rate in the dark, provides the power for a plant’s metabolic activity (Arnold et al., 2002). The decrease of Rd under various Cd stress conditions, as shown in Table 1, reflects the reduction in the need for energy and intermediate metabolites, resulting in an extremely inhibited plant physiological activity (Atkin and Macherel, 2009). The Cd stress caused by the accumulation of Cd in leaves was the first and main reason for the reduction in both Amax and Rd. We hypothesized that there was a significant relationship between Amax and Rd and leaf Cd concentration (Lcd). To test this hypothesis, linear curves were fitted to determine the relation between Amax and Lcd as well as the relation between Rday and Lcd in Figure 10. The fitted linear curves showed that Amax and Rday had a noticeable negative relationship with Lcd (p < 0.01), indicating that the maximum photosynthetic capacity and respiration rate of Hybrid Pennisetum were significantly suppressed with the increase of Cd concentration in Hybrid Pennisetum leaves. It is important to note that we used Amax but not Pn to determine the relationship with Lcd. The reason is that Pn as an instantaneous measure parameter is very affected by the change in PPFD at different measurement times. Relatively, Amax was more stable than Pn because it was measured under optimal environmental conditions.
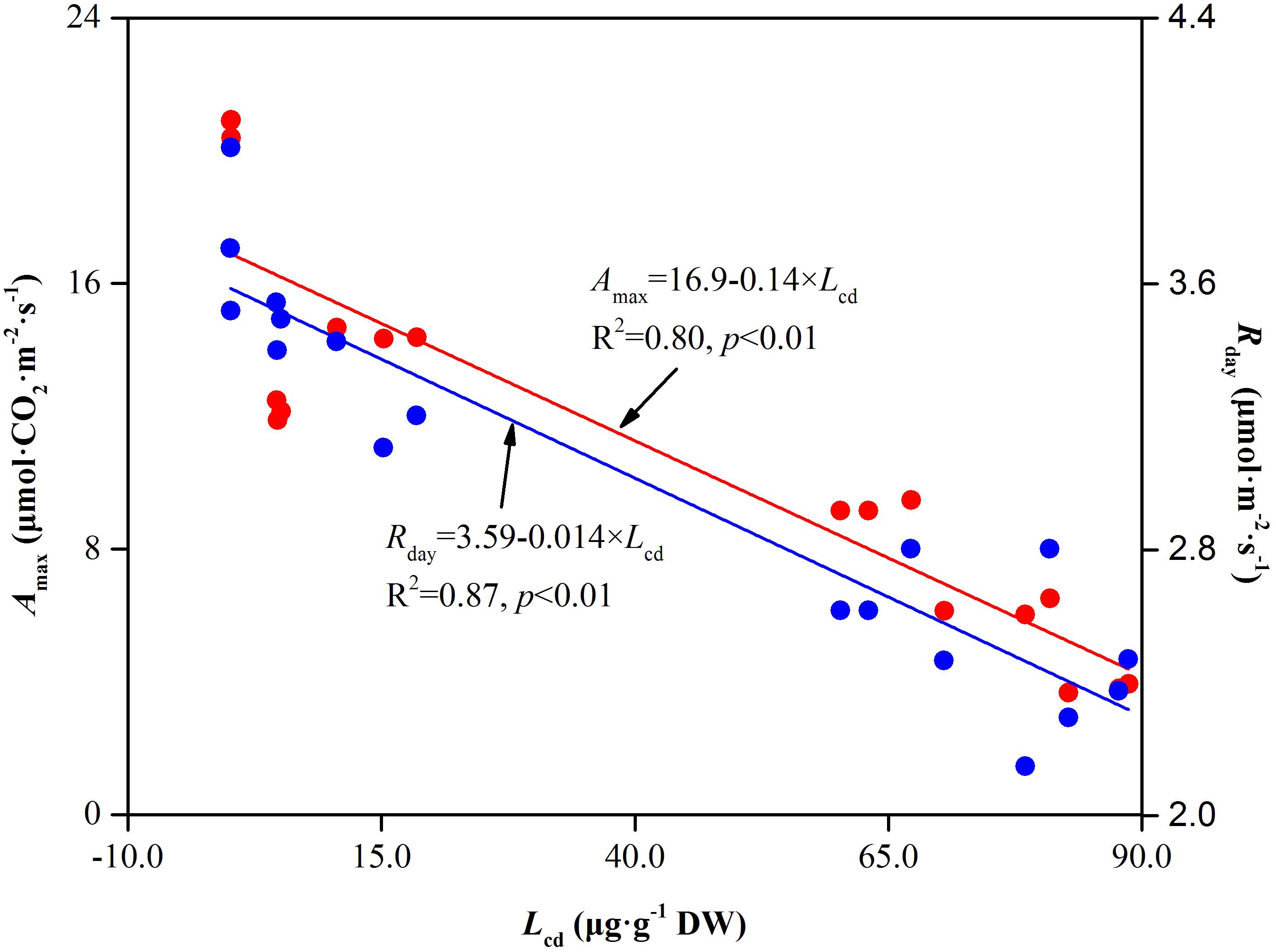
Figure 10. The relationship between leaf Cd concentration and respiration rate in leaves of Hybrid Pennisetum.
Chl fluorescence has a close relationship with plant photosynthesis, and Chl fluorescence analysis has been proven to be a useful tool in monitoring the influence of various stress conditions, such as salt (Mehta et al., 2010; Sun et al., 2016), high temperature (Mathur et al., 2015), pH (Tongra et al., 2011; Long et al., 2017), drought (Oukarroum et al., 2007), fluoranthene (Rupal and Anjana, 2013), photooxidation (Jing et al., 2017), and heavy metals (Hendrik et al., 2010; Goussi et al., 2018), on the photosynthetic activity of plants or microalgae/cyanobacteria. Although Chl fluorescence emissions only account for 2–5% of the energy that is not used in photosynthesis (Zhao et al., 2018), it can supply extensive information related to the components,structure, and function of plant photosynthetic performance (Strasser et al., 2004). Changes in Chl fluorescence indicate a decline in the ability of plants to cope with excess light intensity both in protective regulatory mechanisms and in the regulation of PSII (Logan, 2007). Therefore, any small change in Chl fluorescence in plants can be used to identify the response of plants to environmental stress. PSII of photosynthesis has been proven to be the primary target of Cd toxicity (Alexander et al., 2008; Popova et al., 2009). To characterize the PSII activities in Cd-treated plants, the OJIP transients that reflect the successive reduction in the electron pools (Pheo, QA and QB) of PSII (Strasser et al., 2004) were used in our study. The OJ phase in the OJIP transients is strongly light dependent (Neubauer and Schreiber, 1987; Schansker et al., 2015) and contains information on antenna size and connectivity between PSII reaction centers. The J to P rise in the OJIP transients is called the thermal phase and reflects a reduction in the rest of the electron transport chain (Schansker et al., 2005). As shown in Figure 6, a faster fluorescence increase in samples treated with different Cd stress was found at the OJ phase, indicating that the reoxidation of QA− is inhibited and that there is a larger accumulation of QA− due to the curtailment efficiency of electron transport beyond QA (Strasser and Govindjee, 1992; Strauss et al., 2006; Chen et al., 2011; Kalaji et al., 2017). With the increase in reaction times, the curves from the I phase to the P phase under Cd treatment decreased, indicating a slowdown in electron donation from the PSII donor side (Oukarroum et al., 2015). The different shapes of the fluorescence induction curves under different Cd treatments compared to the Cd0 treatment indicated that Cd toxicity not only inhibited the light use efficiency but also the ability to avoid photodamage.
The dynamic energy pipeline leaf model of the photosynthetic apparatus has been shown to be a direct and visual way to present the alteration of PSII energy fluxes (Li et al., 2014; Sun et al., 2016). In the present study, except for ETo/CSm, the other parameters, such as ABS/CSm, TR0/CSm, and DI0/CSm, showed no significant reduction during all Cd treatments compared with Cd0 plants (Figure 7). The unchanging ABS/CSm suggested that the energy trapped per excited CS was not suppressed by Cd. Electron transport (ETo/CSm) decreased with Cd treatment, indicating the inactivation of reaction center complexes. The ratio TR0/CSm and DI0/CSm was not significantly affected by Cd, indicating that the trapped light in the reaction centers was not affected significantly and that the excess energy was not dissipated in the form of heat but was utilized in some other form. In addition, the decreased ETo/CSm and relatively stable DIO/CSm contributed to the imbalance between light energy absorbed by photosystems and energy consumed by metabolic sinks in the plant.
Conclusion
In this study, we concluded that the high Cd concentration in the leaves of Hybrid Pennisetum caused acute Cd toxicity by impairing photosynthetic performance after 3 months of soil Cd treatments. According to the changes in gas exchange parameters under Cd stress conditions, non-stomatal limitation, rather than stomatal limitation, regulates the photosynthetic processes of Hybrid Pennisetum. The mechanism by which Cd stress affected photosynthesis in Hybrid Pennisetum was through decreasing the leaf chlorophyll content, inhibiting Rubisco activity, RuBP regeneration, and triose phosphate utilization, reducing both light use and energy-providing ability, and preventing electron transport in PSII. The inhibition of photosynthetic processes caused a significant decrease in photosynthetic capacity.
Data Availability
All datasets generated for this study are included in the manuscript.
Author Contributions
WC and HJ conceived the experiment. XY, YH, XL, and XS conducted the experiment. XS analyzed the results and wrote the manuscript. All authors reviewed and approved the manuscript.
Funding
This work was supported by the Shandong Provincial Agricultural Seed-Breeding Improvement Program (Grant No. 2017LZN009).
Conflict of Interest Statement
The authors declare that the research was conducted in the absence of any commercial or financial relationships that could be construed as a potential conflict of interest.
Acknowledgments
The authors thank Man Chen and Derui Wang for her help during the experiment.
References
Ahammed, G. J., Yuan, H. L., Ogweno, J. O., Zhou, Y. H., Xia, X. J., Mao, W. H., et al. (2012). Brassinosteroid alleviates phenanthrene and pyrene phytotoxicity by increasing detoxification activity and photosynthesis in tomato. Chemosphere 86, 546–555. doi: 10.1016/j.chemosphere.2011.10.038
Alexander, K., Rusina, Y., Tibor, J., Gabriella, S., and Losanka, P. (2008). Treatment with salicylic acid decreases the effect of cadmium on photosynthesis in maize plants. J. Plant Physiol. 165, 920–931. doi: 10.1016/j.jplph.2006.11.014
Arnold, S. V., Sabala, I., Bozhkov, P., Dyachok, J., and Filonova, L. (2002). Developmental pathways of somatic embryogenesis. Plant Cell Tissue Organ Cult. 69, 233–249. doi: 10.1023/a:1015673200621
Atkin, O. K., and Macherel, D. (2009). The crucial role of plant mitochondria in orchestrating drought tolerance. Ann. Bot. 103, 581–597. doi: 10.1093/aob/mcn094
Baek, K. H., Kim, H. S., Oh, H. M., Yoon, B. D., Kim, J., and Lee, I. S. (2004). Effects of crude oil, oil components, and bioremediation on plant growth. J. Environ. Sci. Health Part A 39, 2465–2472. doi: 10.1081/ESE-200026309
Barceló, J., and Poschenrieder, C. (1990). Plant water relations as affected by heavy metal stress: a review. J. Plant Nutr. 13, 1–37. doi: 10.1080/01904169009364057
Brestic, M., Zivcak, M., Kunderlikova, K., Sytar, O., Shao, H., Kalaji, H. M., et al. (2015). Low PSI content limits the photoprotection of PSI and PSII in early growth stages of chlorophyll b-deficient wheat mutant lines. Photosynthesis Research 125, 151–166. doi: 10.1007/s11120-015-0093-1
Chen, S., Zhou, F., Yin, C., Strasser, R. J., Yang, C., and Sheng, Q. (2011). Application of fast chlorophyll a fluorescence kinetics to probe action target of 3-acetyl-5-isopropyltetramic acid. Environ. Exp. Bot. 71, 269–279. doi: 10.1016/j.envexpbot.2011.08.005
Clemens, S., Aarts, M. G. M., Thomine, S., and Verbruggen, N. (2013). Plant science: the key to preventing slow cadmium poisoning. Trends Plant Sci. 18, 92–99. doi: 10.1016/j.tplants.2012.08.003
Deng, G., Ming, L. I., Hong, L. I., Yin, L., and Wei, L. I. (2014). Exposure to cadmium causes declines in growth and photosynthesis in the endangered aquatic fern (Ceratopteris pteridoides). Aquat. Bot. 112, 23–32. doi: 10.1016/j.aquabot.2013.07.003
Erdal, S., and Turk, H. (2016). Cysteine-induced upregulation of nitrogen metabolism-related genes and enzyme activities enhance tolerance of maize seedlings to cadmium stress. Environ. Exp. Bot. 132, 92–99. doi: 10.1016/j.envexpbot.2016.08.014
Faller, P., Kienzler, K., and Krieger-Liszkay, A. (2005). Mechanism of Cd2+ toxicity: Cd2+ inhibits photoactivation of Photosystem II by competitive binding to the essential Ca2+ site. Biochim. Biophys. Acta 1706, 158–164. doi: 10.1016/j.bbabio.2004.10.005
Farquhar, G. D., Von, C. S., and Berry, J. A. (1980). A biochemical model of photosynthetic CO2 assimilation in leaves of C3 species. Planta 149, 78–90. doi: 10.1007/BF00386231
Feng, J., Shi, Q., Wang, X., Wei, M., Yang, F., and Xu, H. (2010). Silicon supplementation ameliorated the inhibition of photosynthesis and nitrate metabolism by cadmium (Cd) toxicity in Cucumis sativus L. Sci. Hortic. 123, 521–530. doi: 10.1016/j.scienta.2009.10.013
Fornazier, R. F. (2002). Changes in antioxidant enzyme activities in soybean under cadmium stress. J. Plant Nutr. 25, 327–342. doi: 10.1081/PLN-100108839
Gallego, S. M., Pena, L. B., Barcia, R. A., Azpilicueta, C. E., Iannone, M. F., Rosales, E. P., et al. (2012). Unravelling cadmium toxicity and tolerance in plants: insight into regulatory mechanisms. Environ. Exp. Bot. 83, 33–46. doi: 10.1016/j.envexpbot.2012.04.006
Ghnaya, T., Nouairi, I., Slama, I., Messedi, D., Grignon, C., Abdelly, C., et al. (2005). Cadmium effects on growth and mineral nutrition of two halophytes: Sesuvium portulacastrum and Mesembryanthemum crystallinum. J. Plant Physiol. 162, 1133–1140. doi: 10.1016/j.jplph.2004.11.011
Ghosh, M., and Singh, S. P. (2005). A comparative study of cadmium phytoextraction by accumulator and weed species. Environ. Pollut. 133,365–371. doi: 10.1016/j.envpol.2004.05.015
Gonçalves, J. F., Nicoloso, F. T., Becker, A. G., Pereira, L. B., Tabaldi, L. A., Cargnelutti, D., et al. (2009). Photosynthetic pigments content, δ -aminolevulinic acid dehydratase and acid phosphatase activities and mineral nutrients concentration in cadmium-exposed Cucumis sativus L. Biologia 64, 310–318. doi: 10.2478/s11756-009-0034-6
Goussi, R., Manaa, A., Derbali, W., Ghnaya, T., Abdelly, C., and Barbato, R. (2018). Combined effects of NaCl and Cd2+ stress on the photosynthetic apparatus of Thellungiella salsuginea. Biochim. Biophys. Acta 1859, 1274–1287. doi: 10.1016/j.bbabio.2018.10.001
Groppa, M. D., Ianuzzo, M. P., Rosales, E. P., Vázquez, S. C., and Benavides, M. P. (2012). Cadmium modulates NADPH oxidase activity and expression in sunflower leaves. Biol. Plant. 56, 167–171. doi: 10.1007/s10535-012-0036-z
Groppa, M. D., Tomaro, M. L., and Benavides, M. P. (2007). Polyamines and heavy metal stress: the antioxidant behavior of spermine in cadmium- and copper-treated wheat leaves. Biometals 20, 185–195. doi: 10.1007/s10534-006-9026-y
Guidi, L., Degl’Innocenti, E., Remorini, D., Massai, R., and Tattini, M. (2008). Interactions of water stress and solar irradiance on the physiology and biochemistry of Ligustrum vulgare. Tree Physiol. 28, 873–883. doi: 10.1093/treephys/28.6.873
Haag, K. A., Schafer, H., Heiss, S., Walter, C., and Rausch, T. (1999). Cadmium stress in Brassica juncea causes a decline in transpiration rate and leaf expansion without effect on photosynthesis. J. Exp. Bot. 50, 1827–1835. doi: 10.1093/jexbot/50.341.1827
He, S., He, Z., Yang, X., Stoffella, P. J., and Baligar, V. C. (2015). Soil biogeochemistry, plant physiology, and phytoremediation of cadmium-contaminated soils. Adv. Agron. 134, 135–225. doi: 10.1016/bs.agron.2015.06.005
Hendrik, K., Aravind, P., Barbara, L., Martin, T., and Ivan, S. (2010). Cadmium-induced inhibition of photosynthesis and long-term acclimation to cadmium stress in the hyperaccumulator Thlaspi caerulescens. New Phytol. 175, 655–674. doi: 10.1111/j.1469-8137.2007.02139.x
Herrick, J. D., and Thomas, R. B. (1999). Effects of CO2 enrichment on the photosynthetic light response of sun and shade leaves of canopy sweetgum (Liquidambar styraciflua) in a forest ecosystem. Tree Physiology 19, 779–786. doi: 10.1093/treephys/19.12.779
Horváth, G., Droppa, M., Oravecz, Á., Raskin, V. I., and Marder, J. B. (1996). Formation of the photosynthetic apparatus during greening of cadmium-poisoned barley leaves. Planta 199, 238–243. doi: 10.2307/23384301
Huang, X. D., Mcconkey, B. J., Babu, T. S., and Greenberg, B. M. (1997). Mechanisms of photoinduced toxicity of photomodified anthracene to plants: inhibition of photosynthesis in the aquatic higher plant Lemna gibba (duckweed). Environ. Toxicol. Chem. 16, 1707–1715. doi: 10.1002/etc.5620160819
Jing, M., Lv, C., Xu, M., Hao, P., Wang, Y., Shen, W., et al. (2017). Analysis of chlorophyll a fluorescence and proteomic differences of rice leaves in response to photooxidation. Acta Physiol. Plant. 39:46. doi: 10.1007/s11738-016-2342-0
Joshi, M. K., and Mohanty, P. (2004). “Chlorophyll a fluorescence as a probe of heavy metal ion toxicity in plants,” in Chlorophyll a Fluorescence. Advances in Photosynthesis and Respiration, Vol. 19, eds G. C. Papageorgiou and Govindjee (Dordrecht: Springer). doi: 10.1007/978-1-4020-3218-9_25
Kalaji, H. M., Račková, L., Paganová, V., Swoczyna, T., Rusinowski, S., and Sitko, K. (2017). Can chlorophyll- a fluorescence parameters be used as bio-indicators to distinguish between drought and salinity stress in Tilia cordata Mill? Environ. Exp. Bot. 152, 149–157. doi: 10.1016/j.envexpbot.2017.11.001
Laetitia, P. B., Nathalie, L., Alain, V., and Cyrille, F. (2010). Heavy metal toxicity: cadmium permeates through calcium channels and disturbs the plant water status. Plant J. 32, 539–548. doi: 10.1046/j.1365-313X.2002.01442.x
Lawlor, D. W., and Cornic, G. (2010). Photosynthetic carbon assimilation and associated metabolism in relation to water deficits in higher plants [Review]. Plant Cell Environ. 25, 275–294. doi: 10.1046/j.0016-8025.2001.00814.x
Lee, K. C., Cunningham, B. A., Paulsen, G. M., Liang, G. H., and Moore, R. B. (2010). Effects of Cadmium on respiration rate and activities of several enzymes in soybean seedlings. Physiol. Plant. 36, 4–6. doi: 10.1111/j.1399-3054.1976.tb05017.x
Lewis, J. D., Olszyk, D., and Tingey, D. T. (1999). Seasonal patterns of photosynthetic light response in Douglas-fir seedlings subjected to elevated atmospheric CO2 and temperature. Tree Physiol. 19, 243–252. doi: 10.1093/treephys/19.4-5.243
Li, M. P., and Ong, B. L. (1998). Responses of photosynthesis to NaCl in gametophytes of Acrostichum aureum. Physiol. Plant. 102, 119–127. doi: 10.1034/j.1399-3054.1998.1020116.x
Li, Q., Lu, Y., Shi, Y., Wang, T., Ni, K., Xu, L., et al. (2013). Combined effects of cadmium and fluoranthene on germination, growth and photosynthesis of soybean seedlings. J. Environ. Sci. 25, 1936–1946. doi: 10.1016/S1001-0742(12)60264-2
Li, X., Jian, C., Liu, F., Dai, T., Cao, W., and Dong, J. (2014). Cold priming drives the sub-cellular antioxidant systems to protect photosynthetic electron transport against subsequent low temperature stress in winter wheat. Plant Physiol. Biochem. 82, 34–43. doi: 10.1016/j.plaphy.2014.05.005
Logan, B. A. (2007). Chlorophyll a fluorescence: a signature of photosynthesis. J. Torrey Bot. Soc. 132, 650–650.
Long, A., Zhang, J., Yang, L. T., Ye, X., Lai, N. W., Tan, L. L., et al. (2017). Effects of low pH on photosynthesis, related physiological parameters, and nutrient profiles of citrus. Front. Plant Sci. 8:185. doi: 10.3389/fpls.2017.00185
Long, S. P., and Bernacchi, C. J. (2003). Gas exchange measurements, what can they tell us about the underlying limitations to photosynthesis? Procedures and sources of error. J. Exp. Bot. 54, 2393–2401. doi: 10.1093/jxb/erg262
Lutts, S., and Lefèvre, I. (2015). How can we take advantage of halophyte properties to cope with heavy metal toxicity in salt-affected areas? Ann. Bot. 115, 509–528. doi: 10.1093/aob/mcu264
Lux, A., Martinka, M., Vaculík, M., and White, P. J. (2011). Root responses to cadmium in the rhizosphere: a review. J. Exp. Bot. 62, 21–37. doi: 10.1093/jxb/erq281
Malik, D., Sheoran, I. S., and Singh, R. (1992). Carbon metabolism in leaves of cadmium treated wheat seedlings. Plant Physiol. Biochem. 30, 223–229. doi: 10.1104/pp.98.3.1214
Marshall, B., and Biscoe, P. V. (1980). A model for C3 leaves describing the dependence of net photosynthesis on irradiance. 2. Application to the analysis of flag leaf photosynthesis. J. Exp. Bot. 31, 41–48. doi: 10.1093/jxb/31.1.41
Mathur, S., Jajoo, A., Mehta, P., and Bharti, S. (2015). Analysis of elevated temperature-induced inhibition of photosystem II using chlorophyll a fluorescence induction kinetics in wheat leaves (Triticum aestivum). Plant Biol. 13, 1–6. doi: 10.1111/j.1438-8677.2009.00319.x
Mcintyre, T. (2003). Phytoremediation of heavy metals from soils. Adv. Biochem. Eng. Biotechnol. 78, 97–123. doi: 10.1007/3-540-45991-X_4
Mehta, P., Jajoo, A., Mathur, S., and Bharti, S. (2010). Chlorophyll a fluorescence study revealing effects of high salt stress on Photosystem II in wheat leaves. Plant Physiol. Biochem. 48, 16–20. doi: 10.1016/j.plaphy.2009.10.006
Mobin, M., and Khan, N. A. (2007). Photosynthetic activity, pigment composition and antioxidative response of two mustard (Brassica juncea) cultivars differing in photosynthetic capacity subjected to cadmium stress. J. Plant Physiol. 164, 601–610. doi: 10.1016/j.jplph.2006.03.003
Monteiro, M. S., Santos, C., Soares, A. M. V. M., and Mann, R. M. (2009). Assessment of biomarkers of cadmium stress in lettuce. Ecotoxicol. Environ. Saf. 72, 811–818. doi: 10.1016/j.ecoenv.2008.08.002
Neubauer, C., and Schreiber, U. (1987). The polyphasic rise of chlorophyll fluorescence upon onset of strong continuous illumination: I. Saturation characteristics and partial control by the photosystem II acceptor side. Z. Naturforsch. C 42, 1246–1254. doi: 10.1515/znc-1987-11-1217
Nirupama, M., and Mohn, F. H. (2003). Use of chlorophyll fluorescence in metal-stress research: a case study with the green microalga Scenedesmus. Ecotoxicol. Environ. Saf. 55, 64–69. doi: 10.1016/S0147-6513(02)00122-7
Nunes, C., Araújo, S. S., Silva, J. M., Fevereiro, P., and Silva, A. B. (2010). Photosynthesis light curves: a method for screening water deficit resistance in the model legume Medicago truncatula. Ann. Appl. Biol. 155, 321–332. doi: 10.1111/j.1744-7348.2009.00341.x
Omasa, K., and Takayama, K. (2003). Simultaneous measurement of stomatal conductance, non-photochemical quenching, and photochemical yield of photosystem II in intact leaves by thermal and chlorophyll fluorescence imaging. Plant Cell Physiol. 44, 1290–1300. doi: 10.1093/pcp/pcg165
Oukarroum, A., Bussotti, F., Goltsev, V., and Kalaji, H. M. (2015). Correlation between reactive oxygen species production and photochemistry of photosystems I and II in Lemna gibba L. plants under salt stress. Environ. Exp. Bot. 109, 80–88. doi: 10.1016/j.envexpbot.2014.08.005
Oukarroum, A., Madidi, S. E., Schansker, G., and Strasser, R. J. (2007). Probing the responses of barley cultivars (Hordeum vulgare L.) by chlorophyll a fluorescence OLKJIP under drought stress and re-watering. Environ. Exp. Bot. 60, 438–446. doi: 10.1016/j.envexpbot.2007.01.002
Pagliano, C., Raviolo, M., Vecchia, F. D., Gabbrielli, R., Gonnelli, C., Rascio, N., et al. (2006). Evidence for PSII donor-side damage and photoinhibition induced by cadmium treatment on rice (Oryza sativa L.). J. Photochem. Photobiol. B Biol. 84, 70–78. doi: 10.1016/j.jphotobiol.2006.01.012
Parmar, P., Kumari, N., and Sharma, V. (2013). Structural and functional alterations in photosynthetic apparatus of plants under cadmium stress. Bot. Stud. 54:45. doi: 10.1186/1999-3110-54-45
Peña-Rojas, K., Aranda, X., and Fleck, I. (2004). Stomatal limitation to CO2 assimilation and down-regulation of photosynthesis in Quercus ilex resprouts in response to slowly imposed drought. Tree Physiol. 24, 813–822. doi: 10.1093/treephys/24.7.813
Per, T. S., Masood, A., and Khan, N. A. (2016). Nitric oxide improves S-assimilation and GSH production to prevent inhibitory effects of cadmium stress on photosynthesis in mustard (Brassica juncea L.). Nitric Oxide 68, 111–124. doi: 10.1016/j.niox.2016.12.012
Popova, L. P., Maslenkova, L. T., Yordanova, R. Y., Ivanova, A. P., Krantev, A. P., Szalai, G., et al. (2009). Exogenous treatment with salicylic acid attenuates cadmium toxicity in pea seedlings. Plant Physiol. Biochem. 47, 224–231. doi: 10.1016/j.plaphy.2008.11.007
Prioul, J. L., and Chartier, P. (1977). Partitioning of transfer and carboxylation components of intracellular resistance to photosynthetic CO2 fixation: a critical analysis of the methods Used. Ann. Bot. 41, 789–800. doi: 10.1093/oxfordjournals.aob.a085354
Qian, H., Li, J., Sun, L., Chen, W., Sheng, G. D., and Liu, W. F. (2009). Combined effect of copper and cadmium on Chlorella vulgaris growth and photosynthesis-related gene transcription. Aquat. Toxicol. 94, 56–61. doi: 10.1016/j.aquatox.2009.05.014
Rascio, N., Vecchia, F. D., Rocca, N. L., Barbato, R., Pagliano, C., Raviolo, M., et al. (2008). Metal accumulation and damage in rice (cv. Vialone nano) seedlings exposed to cadmium. Environ. Exp. Bot. 62, 267–278. doi: 10.1016/j.envexpbot.2007.09.002
Rizwan, M., Ali, S., Adrees, M., Ibrahim, M., Tsang, D. C. W., Zia-Ur-Rehman, M., et al. (2017). A critical review on effects, tolerance mechanisms and management of cadmium in vegetables. Chemosphere 182, 90–105. doi: 10.1016/j.chemosphere.2017.05.013
Rupal, S. T., and Anjana, J. (2013). A quick investigation of the detrimental effects of environmental pollutant polycyclic aromatic hydrocarbon fluoranthene on the photosynthetic efficiency of wheat (Triticum aestivum). Ecotoxicology 22, 1313–1318. doi: 10.1007/s10646-013-1118-1
Sandalio, L. M., Dalurzo, H. C., Gomez, M., Romeropuertas, M. C., and Del, R. L. A. (2001). Cadmium-induced changes in the growth and oxidative metabolism of pea plants. J. Exp. Bot. 52, 2115–2126. doi: 10.1093/jexbot/52.364.2115
Santos, L. R., Batista, B. L., and Lobato, A. K. S. (2018). Brassinosteroids mitigate cadmium toxicity in cowpea plants. Photosynthetica 56, 591–605. doi: 10.1007/s11099-017-0700-9
Schansker, G., Tóth, S. Z., and Strasser, R. J. (2005). Methylviologen and dibromothymoquinone treatments of pea leaves reveal the role of photosystem I in Chl a fluorescene rise OJIP. Biochim. Biophys. Acta 1706, 250–261. doi: 10.1016/j.bbabio.2004.11.006
Schansker, G., Tóth, S. Z., and Strasser, R. J. (2015). Dark recovery of the Chl a fluorescence transient (OJIP) after light adaptation: the qT-component of non-photochemical quenching is related to an activated photosystem I acceptor side. Biochim. Biophys. Acta 1757, 787–797. doi: 10.1016/j.bbabio.2006.04.019
Shanying, H. E., Yang, X., Zhenli, H. E., and Baligar, V. C. (2017). Morphological and physiological responses of plants to cadmium toxicity: a review. Pedosphere 27, 421–438. doi: 10.1016/s1002-0160(17)60339-4
Sharkey, T. D., Bernacchi, C. J., Farquhar, G. D., and Singsaas, E. L. (2007). Fitting photosynthetic carbon dioxide response curves for C3 leaves. Plant Cell Environ. 30, 1035–1040. doi: 10.1111/j.1365-3040.2007.01710.x
Sheoran, I. S., Singal, H. R., and Singh, R. (1990). Effect of cadmium and nickel on photosynthesis and the enzymes of the photosynthetic carbon reduction cycle in pigeonpea (Cajanus cajan L.). Photosynth. Res. 23, 345–351. doi: 10.1007/bf00034865
Shukla, U. C., Murthy, R. C., and Kakkar, P. (2008). Combined effect of ultraviolet-B radiation and cadmium contamination on nutrient uptake and photosynthetic pigments in Brassica campestris L. seedlings. Environ. Toxicol. 23, 712–719. doi: 10.1002/tox.20378
Siedlecka, A., Samuelsson, G., Gardeström, P., Kleczkowslci, L. A., and Krupa, Z. (1998). “The “Activatory Model” of plant response to moderate cadmiumstress - relationship between carbonic anhydrase and rubisco,” in Photosynthesis: Mechanisms and Effects, ed. G. Garab (Dordrecht: Springer). doi: 10.1007/978-94-011-3953-3_630
Singh, S., Khan, N. A., Nazar, R., and Anjum, N. A. (2008). Photosynthetic traits and activities of antioxidant enzymes in blackgram (Vigna mungo L. Hepper) under cadmium stress. Am. J. Plant Physiol. 3, 25–32. doi: 10.3923/ajpp.2008.25.32
Somashekaraiah, B. V., Padmaja, K., and Prasad, A. R. K. (1992). Phytotoxicity of cadmium ions on germinating seedlings of mung bean (Phaseolus vulgaris): involvement of lipid peroxides in chlorphyll degradation. Physiol. Plant. 85, 85–89. doi: 10.1111/j.1399-3054.1992.tb05267.x
Song, X., Zhou, G., Xu, Z., Lv, X., and Wang, Y. (2016). A self-photoprotection mechanism helps Stipa baicalensis adapt to future climate change. Sci. Rep. 6:25839. doi: 10.1038/srep25839
Stephen, E., Mottram, D. S., Nira, M., Dodson, A. T., Parry, M. A. J., and Halford, N. G. (2007). Changes in free amino acids and sugars in potatoes due to sulfate fertilization and the effect on acrylamide formation. J. Agric. Food Chem. 55, 5363–5366. doi: 10.1021/jf070447s
Strasser, R. J., and Govindjee (1992). The FO and the O-J-I-P Fluorescence Rise in Higher Plants and Algae. Heidelberg: Springer. doi: 10.1007/978-1-4615-3366-5_60
Strasser, R. J., Tsimilli-Michael, M., and Srivastava, A. (2004). Analysis of the Chlorophyll a Fluorescence Transient. Dordrecht: Springer. doi: 10.1007/978-1-4020-3218-9_12
Strauss, A. J., Krüger, G. H. J., Strasser, R. J., and Heerden, P. D. R. V. (2006). Ranking of dark chilling tolerance in soybean genotypes probed by the chlorophyll a fluorescence transient O-J-I-P. Environ. Exp. Bot. 56, 147–157. doi: 10.1016/j.envexpbot.2005.01.011
Su, J. W., and Wang, X. P. (2004). Effect of Cadmium ions on photosynthetic structure and its functions of tea leaves. J. Tea Sci. 24, 65–69. doi: 10.13305/j.cnki.jts.2004.01.013
Sun, Z. W., Ren, L. K., Fan, J. W., Li, Q., Wang, K. J., Guo, M. M., et al. (2016). Salt response of photosynthetic electron transport system in wheat cultivars with contrasting tolerance. Plant Soil Environ. 62, 515–521. doi: 10.17221/529/2016-PSE
Tartachnyk, I. I., and Blanke, M. M. (2004). Effect of delayed fruit harvest on photosynthesis, transpiration and nutrient remobilization of apple leaves. New Phytol. 164, 441–450. doi: 10.1111/j.1469-8137.2004.01197.x
Tongra, T., Mehta, P., Mathur, S., Agrawal, D., Bharti, S., Los, D. A., et al. (2011). Computational analysis of fluorescence induction curves in intact spinach leaves treated at different pH. Biosystems 103, 158–163. doi: 10.1016/j.biosystems.2010.07.019
Vartika, R., Sayyada, K., Bisht, S. S., and Shanta, M. (2005). Effect of cadmium on growth, ultramorphology of leaf and secondary metabolites of Phyllanthus amarus Schum. and Thonn. Chemosphere 61, 1644–1650. doi: 10.1016/j.chemosphere.2005.04.052
Voigt, J., and Nagel, K. (2002). The donor side of photosystem II is impaired in a Cd2+-tolerant mutant strain of the unicellular green alga Chlamydomonas reinhardtii. J. Plant Physiol. 159, 941–950. doi: 10.1078/0176-1617-00705
Wahid, A., Ghani, A., Ali, I., and Ashraf, M. Y. (2010). Effects of Cadmium on carbon and nitrogen assimilation in shoots of mungbean [Vigna radiata (L.) Wilczek] seedlings. J. Agron. Crop Sci. 193, 357–365. doi: 10.1111/j.1439-037X.2007.00270.x
Wali, M., Fourati, E., Hmaeid, N., Ghabriche, R., Poschenrieder, C., Abdelly, C., et al. (2015). NaCl alleviates Cd toxicity by changing its chemical forms of accumulation in the halophyte Sesuvium portulacastrum. Environ. Sci. Pollut. Res. Int. 22, 10769–10777. doi: 10.1007/s11356-015-4298-9
Wali, M., Gunsè, B., Llugany, M., Corrales, I., Abdelly, C., Poschenrieder, C., et al. (2016). High salinity helps the halophyte Sesuvium portulacastrum in defense against Cd toxicity by maintaining redox balance and photosynthesis. Planta 244, 1–14. doi: 10.1007/s00425-016-2515-5
Wali, M., Martos, S., Pérez-Martín, L., Abdelly, C., Ghnaya, T., Poschenrieder, C., et al. (2017). Cadmium hampers salt tolerance of Sesuvium portulacastrum. Plant Physiol. Biochem. 115, 390–399. doi: 10.1016/j.plaphy.2017.04.014
Walker, A. P., Beckerman, A. P., Gu, L., Kattge, J., Cernusak, L. A., Domingues, T. F., et al. (2015). The relationship of leaf photosynthetic traits - Vcmax and Jmax - to leaf nitrogen, leaf phosphorus, and specific leaf area: a meta-analysis and modeling study. Ecol. Evol. 4, 3218–3235. doi: 10.1002/ece3.1173
Wu, Z., Zhao, X., Sun, X., Tan, Q., Tang, Y., Nie, Z., et al. (2015). Antioxidant enzyme systems and the ascorbate–glutathione cycle as contributing factors to cadmium accumulation and tolerance in two oilseed rape cultivars (Brassica napus L.) under moderate cadmium stress. Chemosphere 138, 526–536. doi: 10.1016/j.chemosphere.2015.06.080
Wullschleger, S. D. (1993). Biochemical limitations to carbon assimilation in C3 plants—A retrospective analysis of the A/Ci curves from 109 Species. J. Exp. Bot. 44, 907–920. doi: 10.1093/jxb/44.5.907
Xu, L. L., Dong, Y. J., Kong, J., and Liu, S. (2014). Effects of root and foliar applications of exogenous NO on alleviating cadmium toxicity in lettuce seedlings. Plant Growth Regul. 72, 39–50. doi: 10.1007/s10725-013-9834-3
Yasemin, E. I., Deniz, T., and Beycan, A. (2008). Effects of cadmium on antioxidant enzyme and photosynthetic activities in leaves of two maize cultivars. J. Plant Physiol. 165, 600–611. doi: 10.1016/j.jplph.2007.01.017
Yu, Y., Wan, Y., Wang, Q., and Li, H. (2017). Effect of humic acid-based amendments with foliar application of Zn and Se on Cd accumulation in tobacco. Ecotoxicol. Environ. Saf. 138, 286–291. doi: 10.1016/j.ecoenv.2017.01.011
Zhang, X., Zhang, X., Gao, B., Li, Z., Xia, H., Li, H., et al. (2014). Effect of cadmium on growth, photosynthesis, mineral nutrition and metal accumulation of anenergy crop, king grass (Pennisetum americanum × P. purpureum). Biomass Bioenergy 67, 179–187. doi: 10.1016/j.biombioe.2014.04.030
Zhang, Y., Xu, S., Yang, S., and Chen, Y. (2015). Salicylic acid alleviates cadmium-induced inhibition of growth and photosynthesis through upregulating antioxidant defense system in two melon cultivars (Cucumis melo L.). Protoplasma 252, 911–924. doi: 10.1007/s00709-014-0732-y
Zhao, F. J., Lombi, E., and Mcgrath, S. P. (2003). Assessing the potential for zinc and cadmium phytoremediation with the hyperaccumulator Thlaspi caerulescens. Plant Soil 249, 37–43. doi: 10.1023/a:1022530217289
Zhao, L. J., Xie, J. F., Zhang, H., Wang, Z. T., Jiang, H. J., and Gao, S. L. (2018). Enzymatic activity and chlorophyll fluorescence imaging of maize seedlings (Zea mays L.) after exposure to low doses of chlorsulfuron and cadmium. J. Integr. Agric. 17, 826–836. doi: 10.1016/S2095-3119(17)61717-9
Zhou, H. H., Chen, Y. N., Li, W. H., and Chen, Y. P. (2010). Photosynthesis of Populus euphratica in relation to groundwater depths and high temperature in arid environment, northwest China. Photosynthetica 48, 257–268. doi: 10.1007/s11099-010-0032-5
Keywords: cadmium stress, photosynthetic performance, electron transport, non-stomatal limitation, Hybrid Pennisetum
Citation: Song X, Yue X, Chen W, Jiang H, Han Y and Li X (2019) Detection of Cadmium Risk to the Photosynthetic Performance of Hybrid Pennisetum. Front. Plant Sci. 10:798. doi: 10.3389/fpls.2019.00798
Received: 22 March 2019; Accepted: 03 June 2019;
Published: 20 June 2019.
Edited by:
Benoit Schoefs, Le Mans Université, FranceReviewed by:
Tasir Sharief Per, Aligarh Muslim University, IndiaMohd Asgher, Baba Ghulam Shah Badshah University, India
Copyright © 2019 Song, Yue, Chen, Jiang, Han and Li. This is an open-access article distributed under the terms of the Creative Commons Attribution License (CC BY). The use, distribution or reproduction in other forums is permitted, provided the original author(s) and the copyright owner(s) are credited and that the original publication in this journal is cited, in accordance with accepted academic practice. No use, distribution or reproduction is permitted which does not comply with these terms.
*Correspondence: Weifeng Chen, Y2h3ZkBzZGF1LmVkdS5jbg==