- 1Department of Biological and Environmental Science, Graduate School of Agricultural Science, Kobe University, Kobe, Japan
- 2Core Research for Environmental Science and Technology, Japan Science and Technology Agency, Tokyo, Japan
Light is an important factor for determining photosynthetic performance in land plants. At high light intensity, land plants develop photosynthetic activity by increasing electron sinks, such as the Calvin cycle and photorespiration and photoprotective mechanisms in photosystem II (PSII), to effectively utilize light and protect them from photoinhibition. In addition to PSII, photosystem I (PSI) has a risk of undergoing photoinhibition under high light intensity because of the reactive oxygen species (ROS) produced within PSI. However, the acclimation response has hardly been evaluated in the relationship of PSI photoprotection to growth light. In this study, we studied the effect of growth light intensity on the photoprotective mechanisms in PSI using six wheat cultivars. To evaluate the susceptibility of PSI to its photoinhibition, we used the repetitive short-pulse (rSP) illumination method to cause O2-dependent PSI photoinhibition. We found that PSI photoinhibition induced by rSP illumination was much more alleviated in wheat cultivars grown under high-light conditions compared to those grown under low-light conditions. Here, we observed that wheat plant grown under high-light conditions lowered the susceptibility of PSI to its photoinhibition compared to those grown under low-light conditions. Furthermore, the acclimation response toward PSI photoinhibition was significantly different among the studied wheat cultivars, although the quantum yields both of PSII and PSI were increased by high-light acclimation in all wheat cultivars as reported previously. Interestingly, we observed that total chlorophyll content in leaves correlated with the susceptibility of PSI to its photoinhibition. On the basis of these results, we suggest that high-light acclimation induces protection mechanisms against PSI photoinhibition in land plants, and the increase in the leaf chlorophyll content relates to the susceptibility of PSI photoinhibition in wheat plants.
Introduction
Land plants grow by photosynthesizing at the sites where they germinate and perform their life cycles even under an unfavorable growth environment. The growth environment greatly affects photosynthetic activity in land plants. For example, a change in temperature affects the carbon fixation rate by influencing the activities or activation states of enzymes involved in the Calvin cycle and other related metabolic processes in the mitochondria and cytoplasm (Atkin and Tjoelker, 2003; Makino and Sage, 2007; Yamori et al., 2014; Noguchi et al., 2015). Moreover, a balance between the rates of carbon reduction in the Calvin cycle and carbon oxidation in photorespiration fluctuates depending on the temperature (Sage et al., 2008). The water status in the soil culture is also important; drought induces stomatal closure and suppresses photosynthesis by limiting the CO2 supply in leaves (Zivcak et al., 2013). Furthermore, a fluctuating light environment disturbs the redox state in thylakoid membranes and stimulates the production of reactive oxygen species (ROS) during photosynthesis (Tikkanen et al., 2010, 2012; Alter et al., 2012; Suorsa et al., 2012; Kono et al., 2014).
To accomplish their life-cycles, land plants possess an ability to maximize photosynthesis under their growth conditions. They can acclimate to fluctuating environmental conditions by optimizing their photosynthetic ability (Walters, 2005; Ensminger et al., 2006; Yamori et al., 2014; Schumann et al., 2017). Acclimation in land plants is comprised of many macro-responses, such as a change in leaf morphology, and micro-responses, such as a change in gene expression or protein composition (De la Torre and Burkey, 1990; Hüner et al., 1998, 2014; Bailey et al., 2001; Savitch et al., 2001; Oguchi et al., 2003; Walters et al., 2003; Dahal et al., 2012; Dumlao et al., 2012; Cohu et al., 2014). Light intensity and quality are two of the most important factors for determining leaf protein composition and morphology (Suorsa et al., 2015; Bielczynski et al., 2016; Schumann et al., 2017). In high-light (HL)-acclimated plants, an increase in leaf thickness, leaf nitrogen content, and photosynthetic protein [Rubisco, Photosystem II (PSII), Cytochrome b6f, etc.] is observed compared to the plants grown under low-light (LL) conditions (De la Torre and Burkey, 1990; Bailey et al., 2001; Oguchi et al., 2003; Walters et al., 2003; Dietz, 2015; Bielczynski et al., 2016; Schumann et al., 2017). Because of these acclimation responses, the photosynthetic rate on leaf area basis increases in acclimated plants compared to non-acclimated plants (Oguchi et al., 2003; Dahal et al., 2012; Schumann et al., 2017). In addition to the photosynthetic rate, the photoprotective mechanisms in PSII are also strengthened by HL acclimation. For protecting PSII from photoinhibition, various photoprotective mechanisms, such as non-photochemical quenching (NPQ), state-transition, and PSII repair-cycle, have been proposed (Müller et al., 2001; Nishiyama et al., 2011; Goldschmidt-Clermont and Roberto, 2015; Liu and Last, 2017). These mechanisms respond to the growth light environment, allowing the land plants to modulate their photoprotective capacity (Miyata et al., 2015; Ware et al., 2015; Schumann et al., 2017). As a result, the land plants grown under HL conditions showed higher tolerance against PSII photoinhibition compared to the plants grown under LL conditions (Gray et al., 1996, 1997; Pocock et al., 2001; Dietz, 2015; Miyata et al., 2015).
As described above, several reports have focused on differences in photosynthetic activity, particularly the differences in electron sink capacity and PSII photoprotective mechanisms of plants. However, the effect of the photoprotective mechanisms of PSI during growth light acclimation has rarely been discussed (Sonoike et al., 1995a,b; Sonoike, 1996a, 1998; Ivanov et al., 1998). PSI causes photoinhibition by the ROS produced within the thylakoid membranes when the photosynthetic electron transport chain is highly reduced (Sonoike and Terashima, 1994; Terashima et al., 1994; Sonoike, 1995, 1996a, 1996b, 1999, 2011; Sonoike et al., 1995a,b; Sejima et al., 2014; Takagi et al., 2016a). For protecting PSI from its photoinhibition, the P700 reaction center chlorophyll (P700) oxidation system is primarily important (Sejima et al., 2014; Shimakawa et al., 2016, 2017, 2019; Takagi et al., 2016b, 2017a,b). The P700 oxidation system consists of the donor side and the acceptor side photosynthetic electron transport regulation mechanisms. The donor side-dependent P700 oxidation system is further separated into the ΔpH-dependent and ΔpH-independent reactions termed as the reduction-induced suppression of photosynthetic electron flow (RISE) reactions (Shaku et al., 2015; Shimakawa et al., 2017; Takagi et al., 2017a,b). At the acceptor side of PSI, photorespiration and flavodiiron proteins contribute to P700 oxidation by stimulating electron outflow from PSI (Shimakawa et al., 2016, 2017; Takagi et al., 2016b, 2017a). The increase in oxidized P700 reduces the risk of over-reduction state within PSI due to the relative decrease in the ground state of P700 and is capable of reducing ROS production within the thylakoid membranes (Sejima et al., 2014; Takagi et al., 2016a, 2017a). Importantly, P700 oxidation is greatly stimulated at the sites where CO2 fixation is limited (Miyake et al., 2005; Takagi et al., 2016b). Several studies have revealed that the P700 oxidation systems contribute to P700 oxidation to prevent the ROS-induced PSI photoinhibition (Shaku et al., 2015; Shimakawa et al., 2016, 2017; Takagi et al., 2016b, 2017b). Therefore, the P700 oxidation systems are fundamental protective mechanisms to escape PSI photoinhibition in photosynthetic organisms.
In addition to the P700 oxidation system, PSI photoinhibition can be modulated independently of the photosynthetic electron transport regulation. Takagi et al. (2017a) reported that angiosperms show a different susceptibility of PSI to photoinhibition, which is independent of P700 oxidation. However, the molecular mechanisms of this difference have remained unknown. Because the difference in the susceptibility of PSI to photoinhibition was observed among the angiosperms growing at different sites in the field, it is ambiguous to predict whether this difference among angiosperms is physiologically controllable within the same plant species.
In the present study, we characterized the effect of growth light conditions on the susceptibility of PSI to its photoinhibition by using six wheat cultivars. To determine the effect of the growth light conditions on the susceptibility of PSI to its photoinhibition in intact leaves, we used repetitive short-pulse (rSP) illumination for wheat cultivars grown under LL (50–60 μmol photons m−2 s−1) and HL (700–800 μmol photons m−2 s−1) conditions. Subsequently, we found that the HL-acclimated wheat plants showed lower susceptibility to PSI photoinhibition compared to the LL-acclimated wheat cultivars. Furthermore, we observed cultivar differences in the susceptibility of PSI photoinhibition to growth light conditions, even though the quantum yield of photosystem (PS) II and PSI showed acclimation responses to growth light conditions among all wheat cultivars. Here, we discussed a new insight for protecting PSI from its photoinhibition.
Materials And Methods
Plant Materials and Growth Conditions
In this study, we used six cultivars of wheat (Triticum aestivum L.), including three spring-type cultivars—“Chinese Spring” (CS), “Bobwhite” (Bob), and “Haruyokoi” (Haru)—and three winter-type cultivars—“Mironovskaya 808” (M808), “Norin 61” (N61), and “Akadaruma” (Aka). Seeds were imbibed with wet cotton at 4°C for 3 days to promote synchronized germination. The imbibed seeds were grown in a mixture of soil (Metro-Mix 350; Sun Gro Horticulture, Bellevue, WA, USA) and vermiculite (Konan, Osaka, Japan) in pots (7.5 cm × 7.5 cm in width and 6 cm in depth). The plants were placed in an environmentally controlled chamber with a photoperiod of 14 h light (25°C) and 10 h dark (20°C). The light intensity was 700–800 μmol photons m−2 s−1 (high-light condition) or 50–60 μmol photons m−2 s−1 (low-light condition). The seedlings were watered every second day with 0.1% Hyponex solution (N:P:K = 5:10:5, Hyponex, Osaka, Japan). For the analysis, we used the fully expanded mature leaves of the plants grown for at least 6 weeks.
Measurement of Chlorophyll Content in Wheat Leaves
The leaf chlorophyll content and chlorophyll a/b ratio were determined as reported previously (Takagi et al., 2017b). In brief, leaf segments were incubated in N, N-dimethylformamide at 4°C overnight, followed by the extraction of chlorophylls from leaves. The absorbances of the aliquots were measured at 750, 663.8, and 646.8 nm to calculate the chlorophyll content (Porra et al., 1989).
Measurement of Chlorophyll Fluorescence and P700+
Chlorophyll fluorescence and P700+ were simultaneously measured with the Dual-PAM-100 system (Heintz Walz GmbH, Effeltrich, Germany) equipped with a 3010 Dual gas exchange chamber (Heintz Walz GmbH, Effeltrich, Germany). The atmospheric gas (40 Pa CO2/21 kPa O2) and the gas with the indicated mixture of pure O2 and CO2, as prepared by mixing 20.1% (v/v) O2 in 79.9% (v/v) N2, 1% (v/v) CO2 in 99% N2, and pure N2 gas using a mass-flow controller (Kofloc model 1203; Kojima Instrument Co.), were used in this study. The gases were saturated with water vapor at 13.5 ± 0.1°C, and the leaf temperature was controlled at 25°C. The chlorophyll fluorescence parameters were calculated as follows (Baker, 2008): maximum quantum efficiency of PSII photochemistry, Fv/Fm = (Fm − Fo)/Fm; quantum yield of photochemical energy conversion in PSII, Y(II) = (Fm′ − Fs)/Fm′, where Fo is the minimum fluorescence yield, Fm is the maximum fluorescence yield, and Fs is the steady-state fluorescence yield; non-photochemical quenching (NPQ) = (Fm – Fm′)/Fm′. To determine Fo and Fm, measuring light (0.1 μmol photons m−2 s−1) and saturating pulse (20,000 μmol photons m−2 s−1, 300 ms) were applied. The oxidation-reduction state of P700+ was determined according to the methods of Klughammer and Schreiber (1994) as follows: quantum yield of photochemical energy in PSI, Y(I) = (Pm′ − P)/Pm; quantum yield of non-photochemical quenching due to acceptor side limitation, Y(NA) = (Pm − Pm′)/Pm; quantum yield of non-photochemical quenching due to donor side limitation, Y(ND) = P/Pm. The maximum oxidation level of P700 (Pm) was obtained from a saturating pulse under far-red light, reflecting the maximum amount of photooxidized P700. The parameter P reflects the steady-state oxidation level of P700, and Pm′ was obtained from the saturating pulse at a steady state. To obtain the maximized Pm′ value, Pm′ value was determined by extrapolation of the slow signal decline of 700+ (from 5 to 35 ms after the beginning of pulse illumination) to time zero according to Klughammer and Schreiber (1994). The zero level of P700+ after one pulse illumination was determined at the steady-state level of P700 in the dark for 1 s. Actinic light (AL), a mixture of red and blue lights, was used to measure the photosynthetic parameters. Light curve analysis was conducted at light intensities 48, 186, 289, 447, 788, 1,134, 1,625, and 2,439 μmol photons m−2 s−1 and the photosynthetic parameters were obtained at the steady-state condition.
Repetitive Short-Pulse Illumination
rSP illumination was applied to the dark-adapted wheat leaves, as described previously (Sejima et al., 2014). Before this measurement, wheat plants were adapted in the dark at least for 1 h. A saturating pulse (20,000 μmol photons m−2 s−1, 300 ms) was used to illuminate the plants every 10 s in the absence of AL for 1 h under the ambient conditions (40 Pa CO2, 21 kPa O2) at 25°C. After rSP illumination, the change in Fv/Fm and Pm was determined as described in the figure legends. The kinetics of the oxidized P700 was determined at the beginning of rSP illumination, averaging all pulse kinetics in the first 1 min of rSP illumination (Takagi et al., 2017a). The P700+ signal was normalized using the Pm value before rSP illumination, and the relative changes in P700+ during one pulse illumination were shown.
Statistical Analysis
All measurement data were expressed as mean value ± SD of at least three independent analyses. For the detection of differences among the studied wheat cultivars, we used the one-way analysis of variance (ANOVA), Student’s t-test, Tukey-Kramer honestly significant difference (HSD) test, and linear and nonlinear regression analysis. All statistical analyses were performed using Microsoft Excel 2010 (Microsoft) and Origin Pro 2019 (LightStone Corp.).
Results
High-Light Acclimation Increased the Quantum Yield of Both Photosystem II and Photosystem I in All Wheat Cultivars
First, we studied the light response of the quantum yield of both PSII and PSI in six wheat cultivars grown under the HL and LL conditions (see Materials and Methods). In this study, we used three spring-type (CS, Bob, and Haru) and three winter-type (M808, N61, Aka) wheat cultivars. The maximum quantum yield of PSII (Fv/Fm) showed a similar value between the HL- and LL-grown wheat cultivars. Furthermore, the Fv/Fm was similar among the wheat cultivars grown under each growth light condition (Figure 1A). The total chlorophyll content was similar among the wheat cultivars grown under LL conditions (Figure 1B). In contrast, the HL-grown wheat cultivars showed significant differences, with N61 showing the highest chlorophyll content on the leaf area basis. In addition, compared to the LL-grown wheat cultivars, the HL-grown wheat cultivars showed an increase in the total chlorophyll content on the leaf area basis. This is a major HL acclimation response reported previously (De la Torre and Burkey, 1990; Hüner et al., 1998; Bailey et al., 2001; Savitch et al., 2001; Oguchi et al., 2003; Walters et al., 2003; Dahal et al., 2012; Schumann et al., 2017). The chlorophyll a/b ratio was not significantly different among the wheat cultivars grown under LL conditions (Figure 1C), whereas the HL-grown wheat cultivars showed differences in their chlorophyll a/b ratios (Figure 1C).
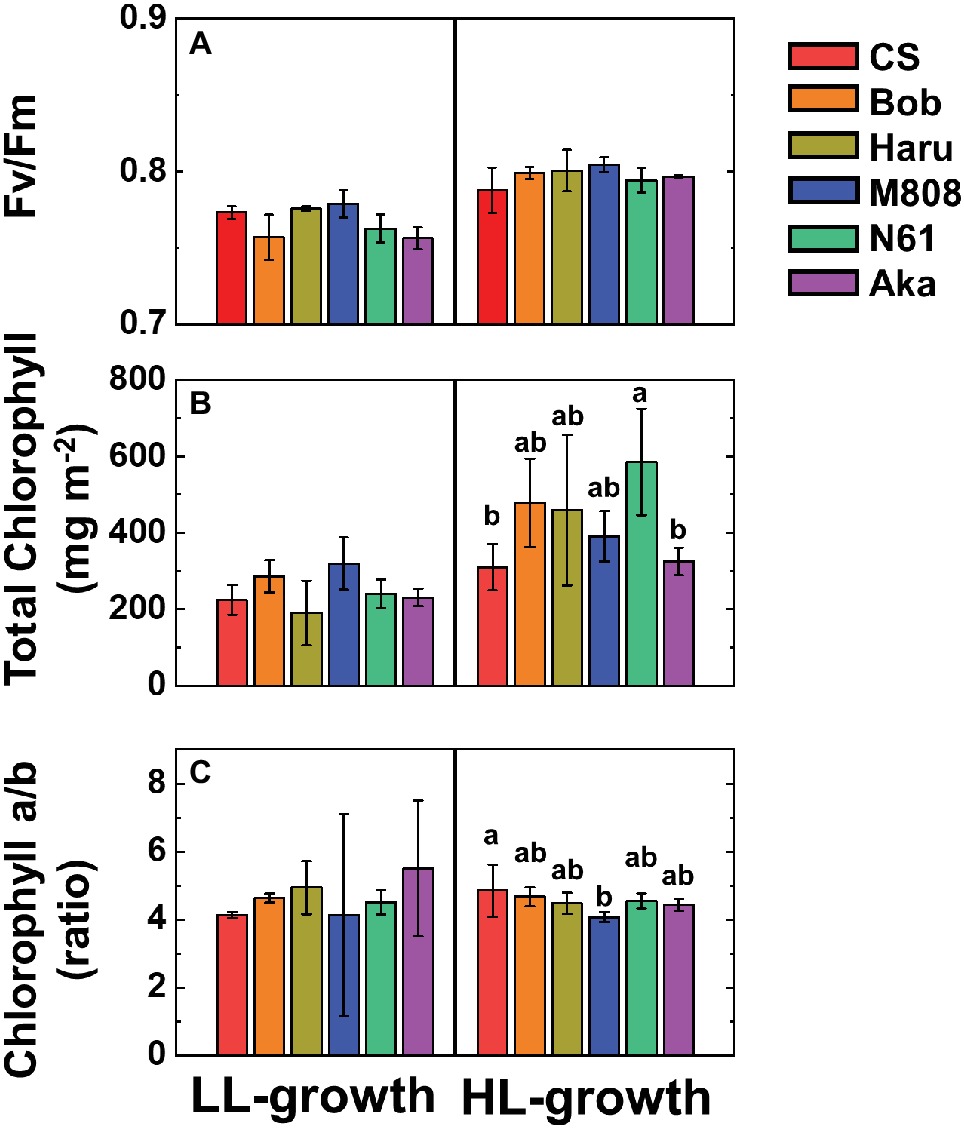
Figure 1. The effect of growth light conditions on the maximum quantum yield of PSII (Fv/Fm) (A), total chlorophyll content on leaf area basis (B), and chlorophyll a/b ratio (C). Data are expressed as mean ± SD of three to five independent experiments. Different letters above the bars indicate significant differences among wheat cultivars (ANOVA and Tukey-Kramer HSD test, p < 0.05). Red bars indicate the results of CS, orange bars indicate the results of Bob, dark yellow bars indicate the results of Haru, blue bars indicate the results of M808, green bars indicate the results of N61, and purple bars indicate the results of Aka.
The quantum yield of PSII [Y(II)] was compared among the six wheat cultivars grown under both LL and HL conditions (Figure 2). Under LL growth conditions, Y(II) was decreased in accordance with the increase in illuminated light intensity in all wheat cultivars, showing no significant differences among them (Figure 2, Supplementary Figure S1A). Compared to LL conditions, HL conditions increased Y(II) toward the illuminated light intensity in all wheat cultivars (Figure 2). However, no significant difference was observed among the wheat cultivars grown under HL conditions (Supplementary Figure S1B).
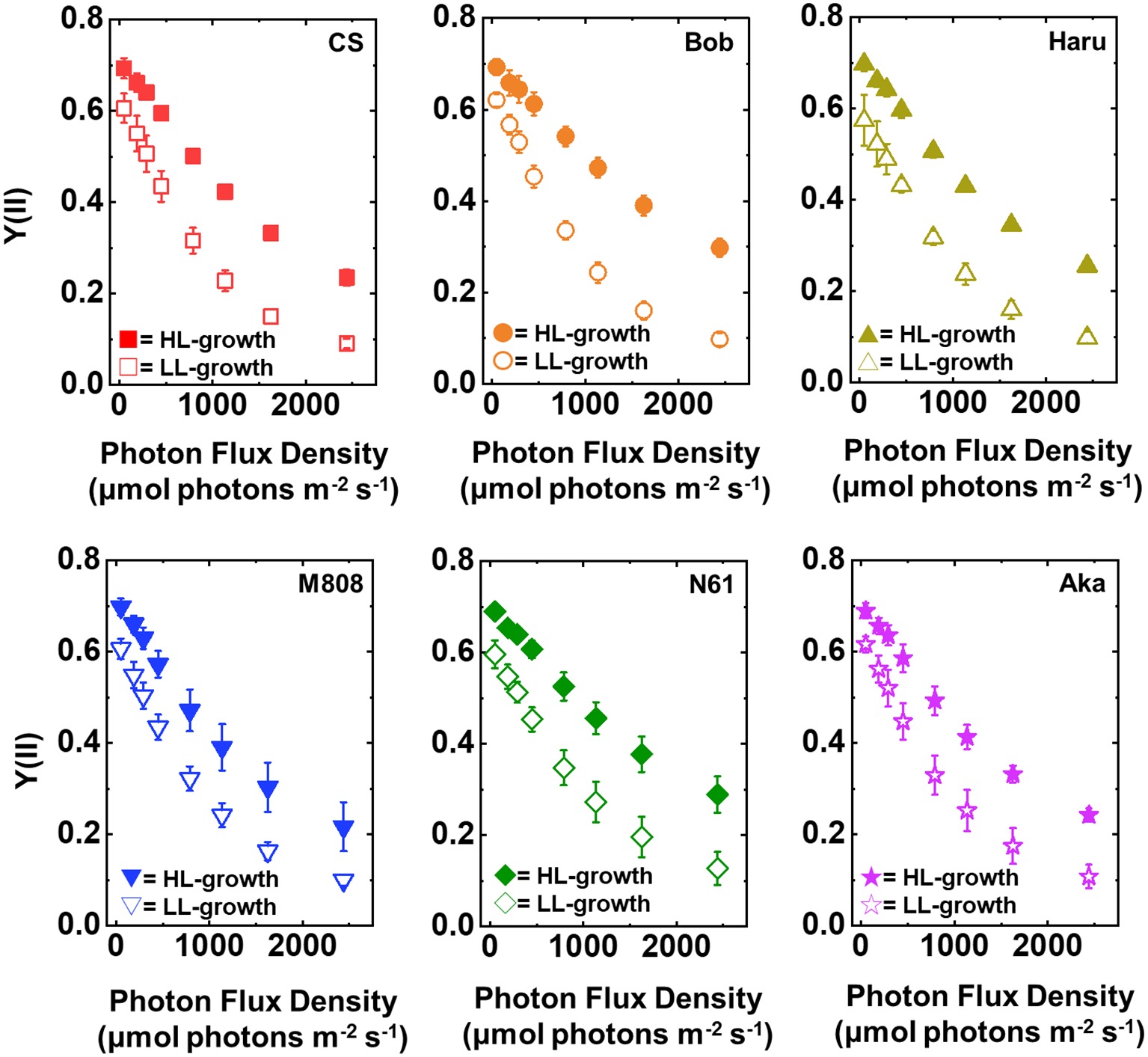
Figure 2. The response of Y(II) to light intensity in six wheat cultivars grown under LL (open symbol) and HL (closed symbol) conditions. The measurement was conducted under atmospheric conditions (21 kPa O2, 40 Pa CO2) at 25°C. Data were expressed as mean ± SD of three to five independent experiments. Red squares indicate the results of CS, orange circles indicate the results of Bob, dark yellow triangles indicate the results of Haru, blue inverted-triangles indicate the results of M808, green diamonds indicate the results of N61, and purple stars indicate the results of Aka.
In concert with the result of Y(II), Y(I) toward the increase in illuminated light intensity was higher in the HL-grown wheat cultivars than that in the LL-grown wheat cultivars (Figure 3). Similar to Y(II), no significant cultivar difference of Y(I) was detected among the wheat cultivars grown under both growth light conditions (Supplementary Figures S1C,D). These acclimation responses of the quantum yield of both PSII and PSI were similar to that of the previous report (Miyake et al., 2005). These results indicated that all wheat cultivars possess a capacity to change the quantum yield of both photosystems under HL conditions and increased the quantum yield of both PSII and PSI by acclimating the HL growth conditions compared to the LL growth conditions.
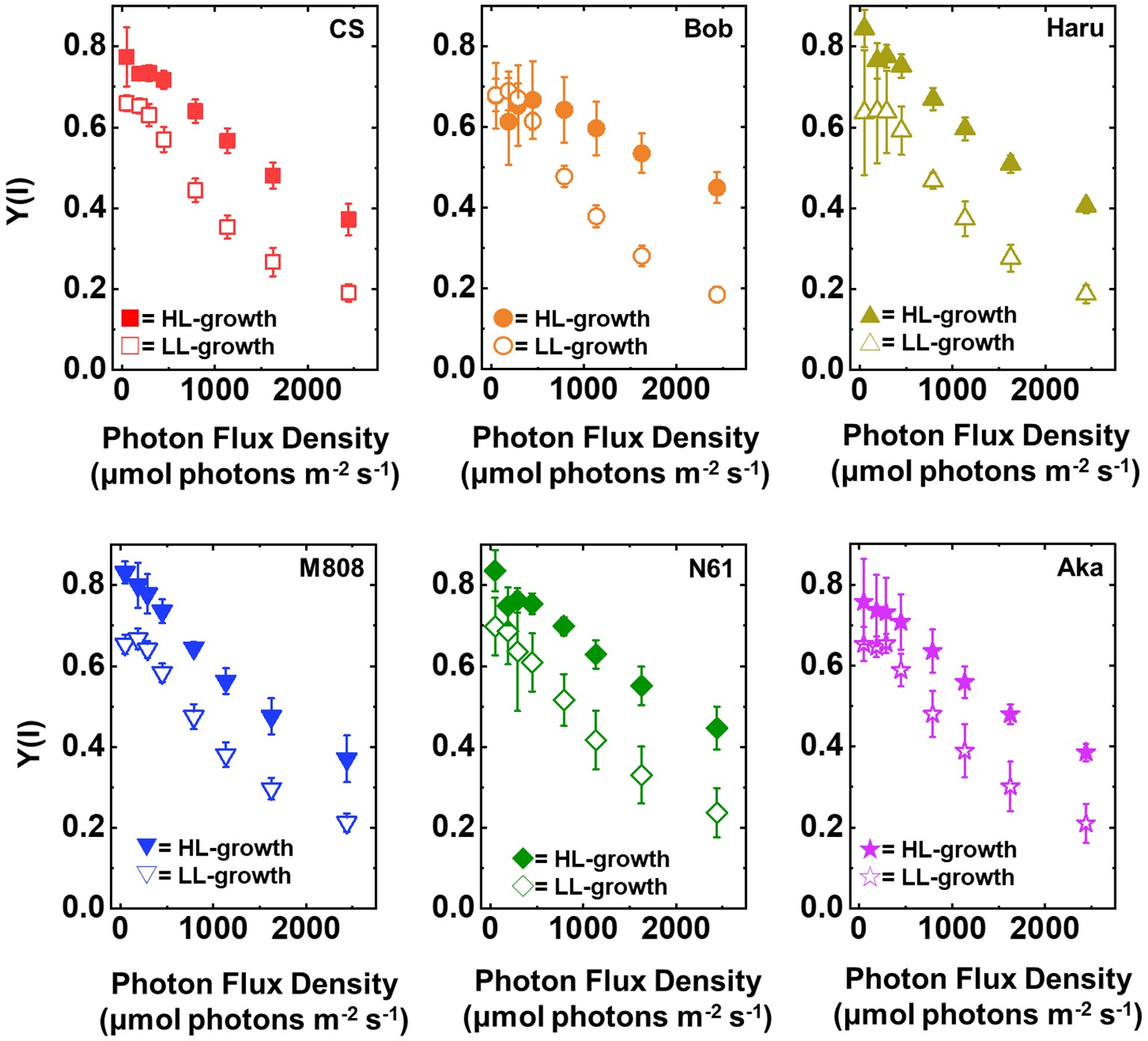
Figure 3. The response of Y(I) to light intensity in six wheat cultivars grown under LL (open symbol) and HL (closed symbol) conditions. The measurement was conducted under atmospheric conditions (21 kPa O2, 40 Pa CO2) at 25°C. Data were expressed as mean ± SD of three to five independent experiments. Red squares indicate the results of CS, orange circles indicate the results of Bob, dark yellow triangles indicate the results of Haru, blue inverted-triangles indicate the results of M808, green diamonds indicate the results of N61, and purple stars indicate the results of Aka.
High-Light-Grown Wheat Plants Showed Lower P700+ Under Illumination Than Low-Light-Grown Wheat Plants Although the Acceptor Side of Electron Transport Limitation in Photosystem I Was Similar Between Low-Light- and High-Light-Grown Wheat Plants
Y(ND) indicates the photosynthetic electron transport limitation at the donor side of PSI, and the increase in Y(ND) indicates the accumulation of oxidized P700 (P700+) in PSI (Klughammer and Schreiber, 1994). In LL-grown wheat cultivars, Y(ND) increased from approximately 100 μmol photons m−2 s−1 of light intensity and showed a saturation of approximately 0.6 at the maximum light intensity (Figure 4). On the other hand, Y(ND) in the HL-grown wheat cultivars hardly increased until the light intensity reached 250 μmol photons m−2 s−1 (Figure 4). Furthermore, Y(ND) was linearly increased with the rise of illuminated light intensity in the HL-grown wheat cultivars. At the maximum light intensity, the HL-grown wheat plants showed lower Y(ND) than the LL-grown wheat cultivars (Figure 4). A similar effect of growth light conditions on Y(ND) was reported by Schumann et al. (2017). As with the response of Y(I), the significant cultivar differences in Y(ND) in each wheat plant grown under LL and HL conditions were not observed (Supplementary Figures S1E,F).
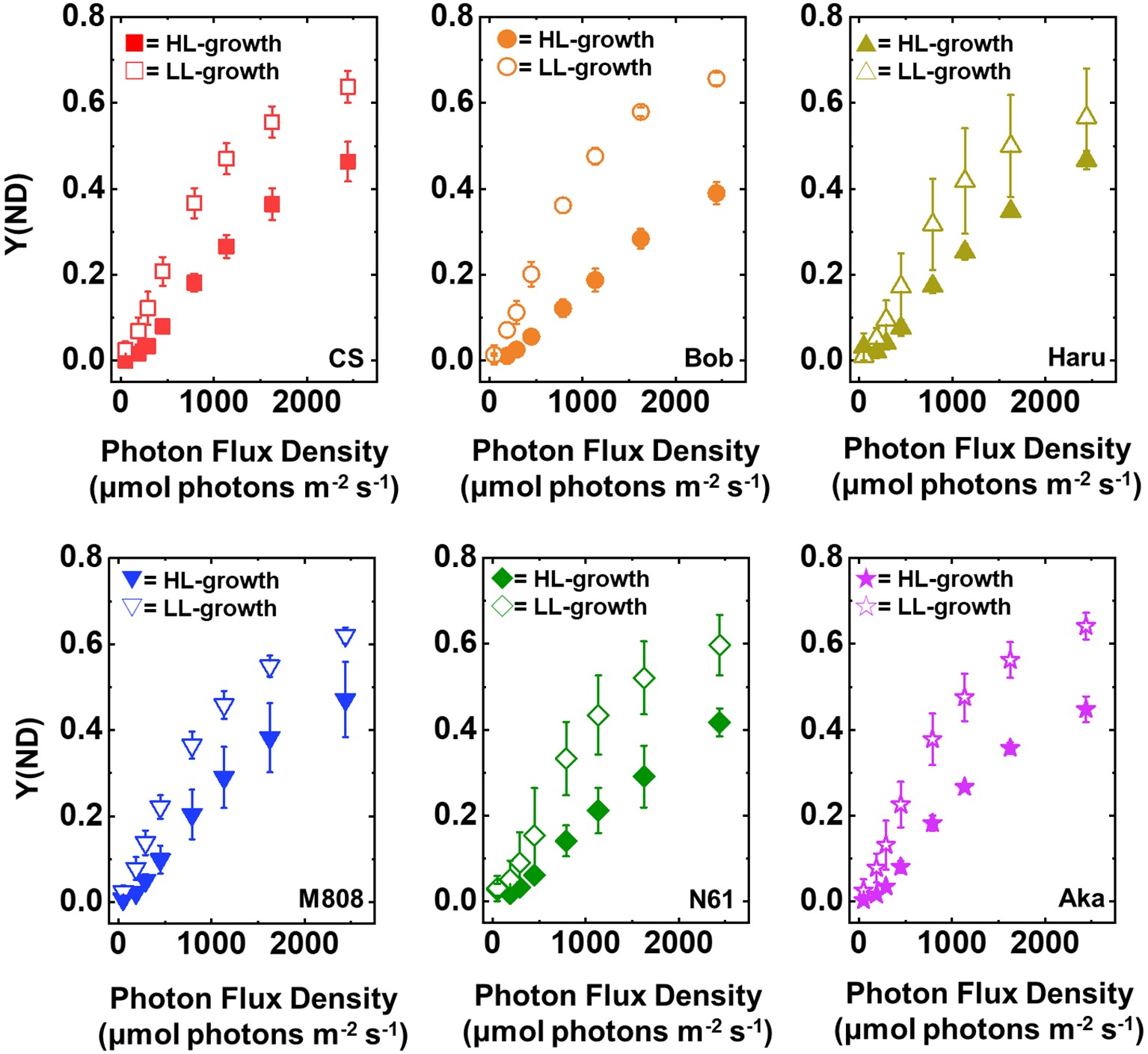
Figure 4. The response of Y(ND) to light intensity in six wheat cultivars grown under LL (open symbol) and HL (closed symbol) conditions. The measurement was conducted under atmospheric conditions (21 kPa O2, 40 Pa CO2) at 25°C. Data were expressed as mean ± SD of three to five independent experiments. Red squares indicate the results of CS, orange circles indicate the results of Bob, dark yellow triangles indicate the results of Haru, blue inverted-triangles indicate the results of M808, green diamonds indicate the results of N61, and purple stars indicate the results of Aka.
Y(NA) indicates the photosynthetic electron transport limitation at the acceptor side of PSI (Klughammer and Schreiber, 1994). The increase in Y(NA) indicates the risk of ROS production within PSI (Munekage et al., 2002; DalCorso et al., 2008; Suorsa et al., 2012; Shimakawa et al., 2017; Takagi et al., 2017b; Takagi and Miyake, 2018). The light response of Y(NA) was similar among the studied wheat cultivars and growth light conditions even under maximum light intensity, and Y(NA) did not show the cultivar difference in each wheat plant grown under LL and HL conditions (Figure 5, Supplementary Figures S1G,H). These results indicated that the wheat cultivars were capable of maintaining the reduction state of PSI even under different growth light conditions and illuminated light intensities.
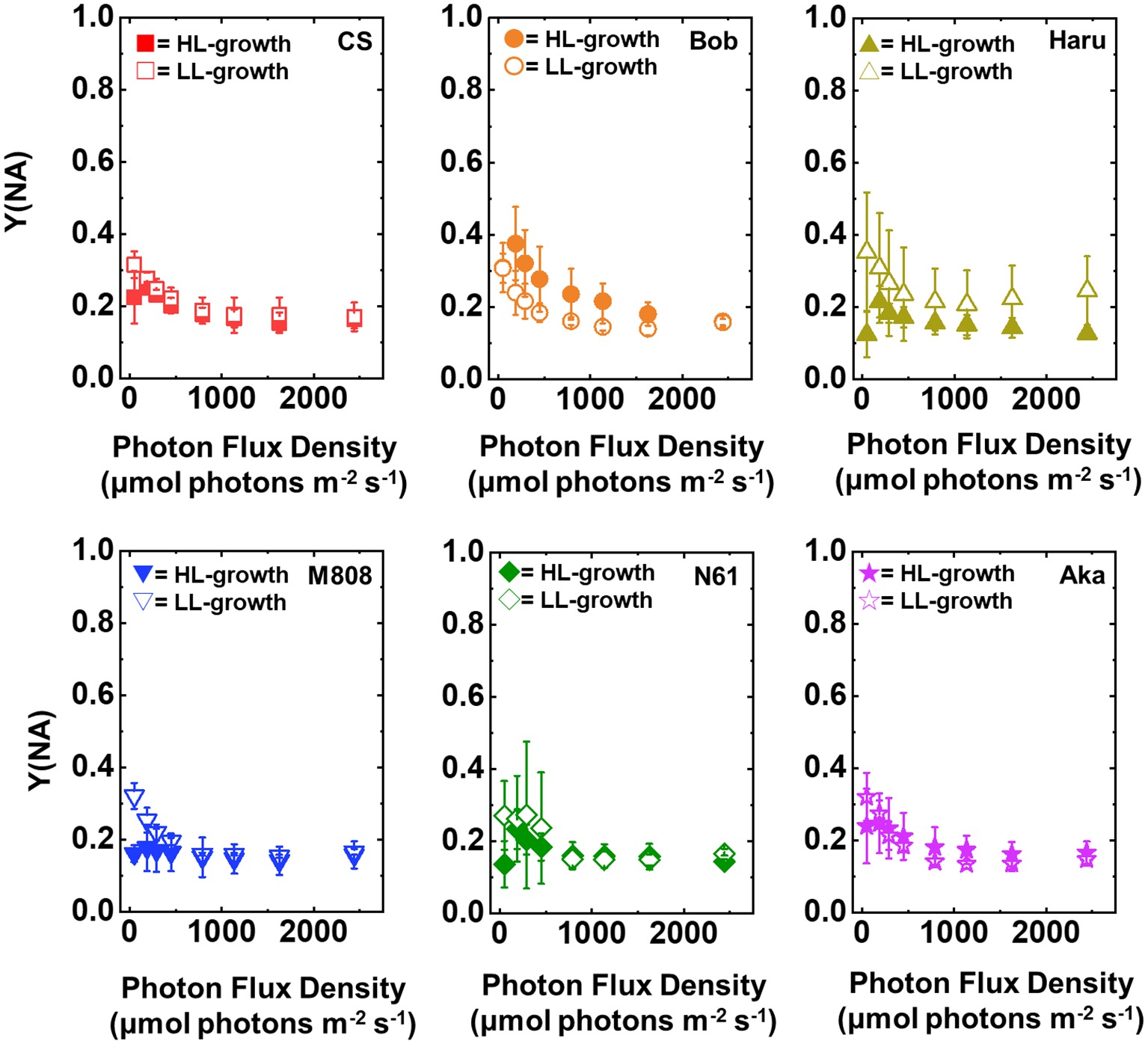
Figure 5. The response of Y(NA) to light intensity in six wheat cultivars grown under LL (open symbol) and HL (closed symbol) conditions. The measurement was conducted under atmospheric conditions (21 kPa O2, 40 Pa CO2) at 25°C. Data were expressed as mean ± SD of three to five independent experiments. Red squares indicate the results of CS, orange circles indicate the results of Bob, dark yellow triangles indicate the results of Haru, blue inverted-triangles indicate the results of M808, green diamonds indicate the results of N61, and purple stars indicate the results of Aka.
High-Light Acclimation Provides Tolerance to Photosystem I Photoinhibition Depending on Wheat Cultivars and Independently of P700 Oxidation
Next, we studied the effect of growth light conditions on the susceptibility of PSI to its photoinhibition using rSP illumination in the dark under ambient air conditions at 25°C (Sejima et al., 2014; Takagi et al., 2017a). The rSP illumination in the dark stimulates electron accumulation within the thylakoid membranes and causes O2-dependent PSI photoinhibition (Sejima et al., 2014; Takagi et al., 2016a, 2017a,b). The relative photoinhibitory effect in PSI during rSP illumination was shown by the relative decrease in Y(I), and the averaged Y(I) for first 1 min was set as “1” according to our previous study (Takagi et al., 2017a). Figure 6A shows the relative change in Y(I) in the dark under ambient air conditions in both LL- and HL-grown wheat cultivars. Interestingly, at the beginning of rSP illumination, we observed the rapid change in relative Y(I) in all wheat cultivars (initial spike; Figure 6A). This phenomenon was also observed in land plants previously (Takagi et al., 2017a). To evaluate whether this initial change in Y(I) indicates the PSI photoinhibition in wheat cultivars, we studied the recovery of this initial change in Y(I) by using CS and M808 grown under LL and HL conditions (Supplementary Figure S2). We confirmed that the rapid change in Y(I) at the beginning of rSP illumination was similar between two wheat cultivars and between LL and HL growth conditions, and the decrease in Y(I) was rapidly recoverable in both LL- and HL-grown wheat cultivars when pulse illumination intervals were prolonged (Supplementary Figure S2). Therefore, the initial change in Y(I) does not show PSI photoinhibition because the recovery of PSI photoinhibition takes several days (Sonoike, 2011; Zivcak et al., 2015). This could indicate the electron accumulation in electron acceptors following P700+ by illuminating pulse illumination. It is noteworthy that the decrease in Y(I) during rSP illumination was more suppressed in the HL-grown wheat cultivars compared to the LL-grown ones; however, the effect of HL acclimation varied between the wheat cultivars (Figure 6A). For example, CS showed a similar decrease in Y(I) during rSP illumination in both HL- and LL-grown wheat plants (Figure 6A). On the other hand, the N61 plants grown under HL conditions showed a large alleviation of the decrease in Y(I), compared to those grown under LL conditions (Figure 6A). The relative change in Y(II) during rSP illumination was also studied (Supplementary Figure S3). Similar to the results of Y(I), the decrease in Y(II) was also suppressed in the HL-grown wheat cultivars, compared to that in the LL-grown wheat cultivars (Supplementary Figure S3). The effect of HL acclimation on the change in Y(II) was also cultivar-dependent, but was smaller than that on the change in Y(I). After rSP illumination for 1 h, we evaluated the change in Pm and Fv/Fm in the wheat cultivars grown under HL and LL conditions to study the extent of photoinhibition in both PSI and PSII (Sejima et al., 2014; Takagi et al., 2016a, 2017a). Compared to the Pm before rSP illumination, the steady-state value of oxidized P700 induced by far-red light (FR) was suppressed in all wheat cultivars after rSP illumination for 1 h, and Pm obtained by FR and pulse illumination was also decreased after rSP illumination (Supplementary Figure S4). Among the LL-grown wheat cultivars, the decrease in Fv/Fm and Pm was not different between the wheat cultivars (Figure 6B, Supplementary Figure S4). On the contrary, significant differences were observed among the HL-grown wheat cultivars. N61 showed the smallest decrease in Pm after rSP illumination, and CS showed the largest decrease in Pm after rSP illumination (Figure 6B, Supplementary Figure S4). Furthermore, the HL-grown wheat cultivars, except for CS and Aka, suppressed the decrease in Pm before and after rSP illumination compared to the LL-grown wheat cultivars (Figure 6B). The change in Fv/Fm was also significantly different among the wheat cultivars grown under HL conditions. However, the effect of growth light conditions on the change in Fv/Fm after rSP illumination was smaller than that on the change in Pm after rSP illumination. This is because the rSP illumination mainly targets PSI to induce photoinhibition; therefore, the lesser photoinhibitory effect was observed on PSII in intact leaves (Sejima et al., 2014; Zivcak et al., 2015; Takagi et al., 2016a, 2017b).
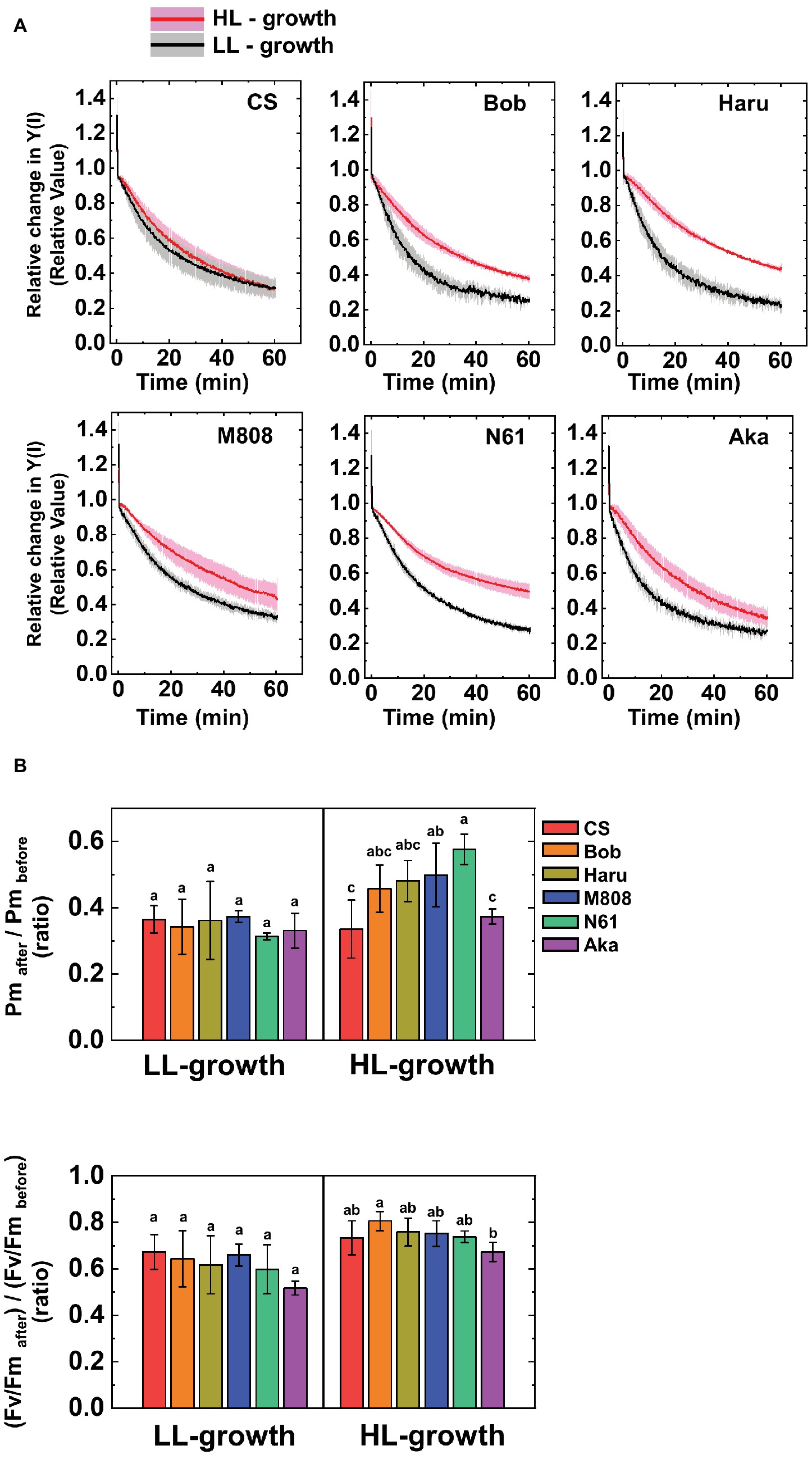
Figure 6. The susceptibility of PSI photoinhibition to repetitive short-pulse (rSP) illumination in wheat cultivars grown under LL and HL conditions. (A) Time-course analysis of the relative change in Y(I) during rSP illumination under atmospheric conditions (21 kPa O2, 40 Pa CO2) at 25°C. Before rSP illumination, wheat plants were adapted in the dark for at least 1 h. Wheat leaves were illuminated every 10 s with a saturating pulse (300 ms, 20,000 μE m−2 s−1) for 1 h in the dark. Data were expressed as mean ± SD of three to five independent experiments. Black line indicates the mean result of LL-grown wheat cultivars and gray shadow indicates the SD of the results of the LL-grown wheat cultivars. Red line indicates the mean results of the LL-grown wheat cultivars and pink shadow indicates the SD of the results of the HL-grown wheat cultivars. The change in Fv/Fm and Pm between before and after rSP illumination are shown in (B). After rSP illumination, wheat cultivars were kept in the dark for 30 min, and Fv/Fm and Pm were measured. Data were normalized to the Fv/Fm and Pm before rSP illumination. Data were expressed as mean ± SD of three to five independent experiments. Different letters above the bars indicate a significant difference between those wheat cultivars (ANOVA and Tukey-Kramer HSD test, p < 0.05). Red bars indicate the results of CS, orange bars indicate the results of Bob, dark yellow bars indicate the results of Haru, blue bars indicate the results of M808, green bars indicate the results of N61, and purple bars indicate the results of Aka.
After rSP illumination to induce PSI photoinhibition, we measured the photosynthetic activities of the wheat cultivars grown under LL and HL conditions. In both LL- and HL-grown wheat cultivars, the values of Y(II) after rSP illumination were lower than those before rSP illumination (Figure 7). The LL-grown wheat cultivars showed similar values of Y(II) among the studied wheat cultivars; however, significant differences were observed among the HL-grown wheat cultivars, with N61 showing the highest Y(II) and CS showing the lowest Y(II) after rSP illumination (Supplementary Figures S5A,B).
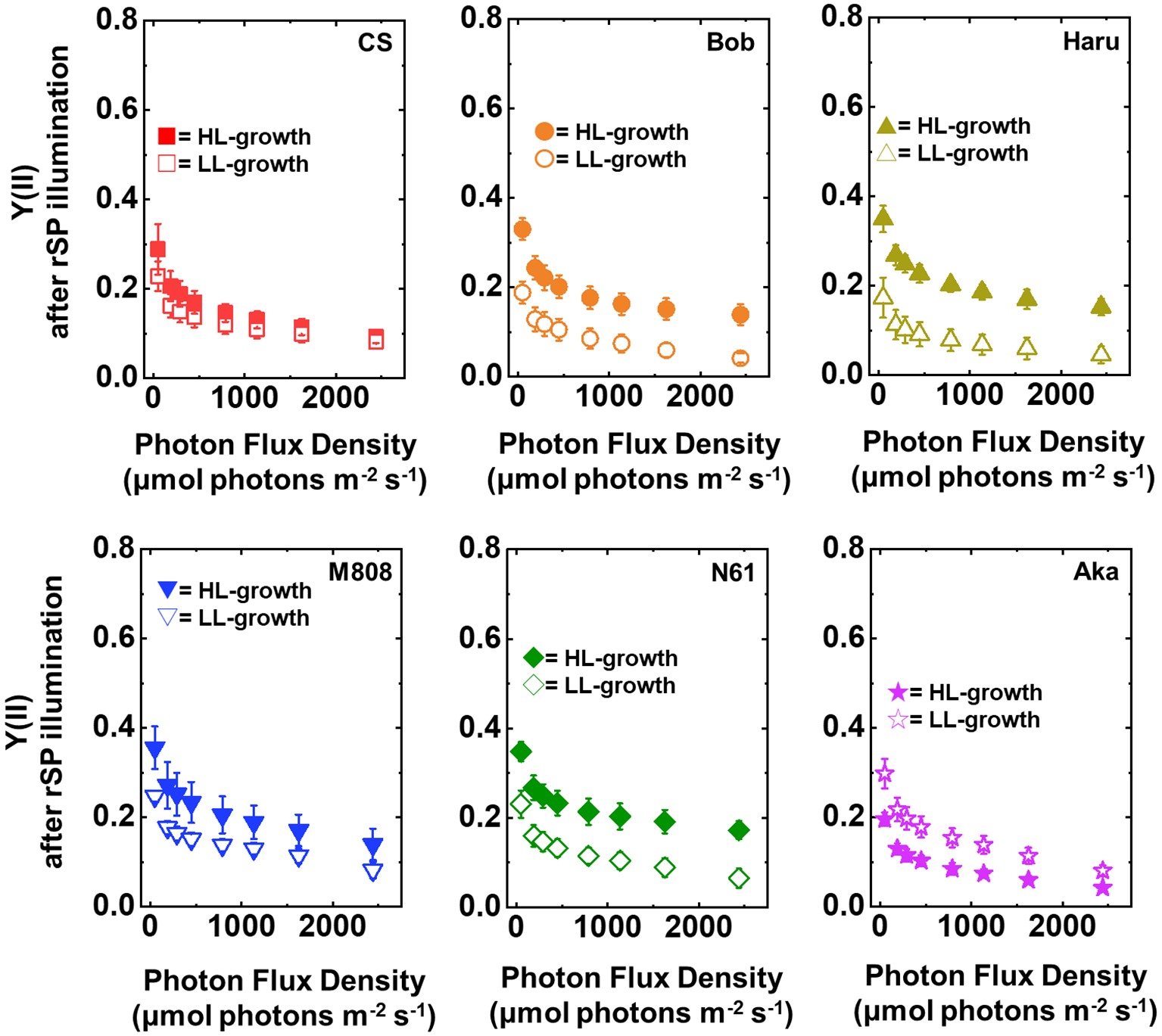
Figure 7. The response of Y(II) to light intensity in six wheat cultivars grown under LL (open symbol) and HL (closed symbol) conditions after rSP illumination for 1 h. The measurement was conducted under atmospheric conditions (21 kPa O2, 40 Pa CO2) at 25°C. Data are expressed as mean ± SD of three to four independent experiments. Red squares indicate the results of CS, orange circles indicate the results of Bob, dark yellow triangles indicate the results of Haru, blue inverted-triangles indicate the results of M808, green diamonds indicate the results of N61, and purple stars indicate the results of Aka.
Furthermore, the Y(I) value after rSP illumination was significantly lower than that before rSP illumination in all wheat cultivars, hardly showing any change toward the illuminated light intensity (Figure 8). These results agreed with the previous report (Zivcak et al., 2015). Similar to the result of Y(II), the LL-grown wheat cultivars showed similar Y(I) (Figure 8, Supplementary Figures S5C,D). However, among the wheat plants grown under HL conditions, N61 showed the highest Y(I) and CS showed the lowest Y(I) after rSP illumination (Supplementary Figures S5C,D). The results of Y(I) were consistent with those of Y(II) (Figure 7).
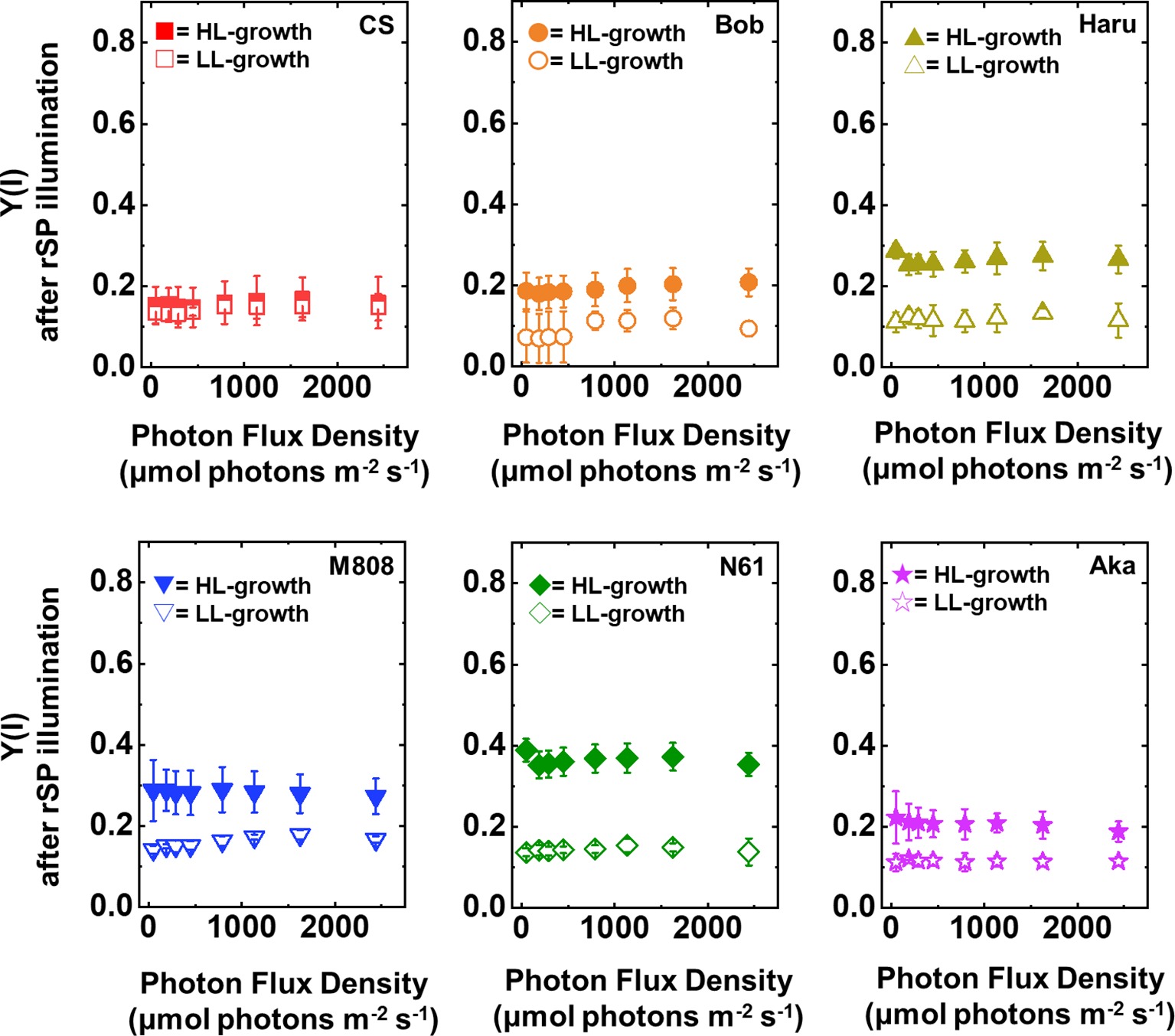
Figure 8. The response of Y(I) to light intensity in six wheat cultivars grown under LL (open symbol) and HL (closed symbol) conditions after rSP illumination for 1 h. The measurement was conducted under atmospheric conditions (21 kPa O2, 40 Pa CO2) at 25°C. Data are expressed as mean ± SD of three to four independent experiments. Red squares indicate the results of CS, orange circles indicate the results of Bob, dark yellow triangles indicate the results of Haru, blue inverted-triangles indicate the results of M808, green diamonds indicate the results of N61, and purple stars indicate the results of Aka.
Next, we compared Y(ND) and Y(NA) after rSP illumination in both LL- and HL-grown wheat cultivars (Figures 9, 10). Y(ND) hardly responded to the illuminated light intensity, and the increase in Y(ND) was greatly inhibited (Figure 9). Furthermore, the LL- and HL-grown wheat cultivars showed similar Y(ND) values under illumination (Figure 9, Supplementary Figures S5E,F). Y(NA) in LL-grown wheat cultivars showed similar values after rSP illumination, and the values after rSP illumination were higher than those before rSP illumination (Figure 10, Supplementary Figures S5G,H). These results indicated that rSP illumination triggered the photoinhibition at the acceptor side of PSI and suppressed the photosynthetic electron transport reaction at that site as reported previously (Sejima et al., 2014; Zivcak et al., 2015; Takagi et al., 2016a). Compared to the results of LL-grown wheat cultivars, HL-grown wheat cultivars showed lower Y(NA) and the cultivar differences in Y(NA) were observed among the wheat cultivars after rSP illumination (Figure 10, Supplementary Figures S5G,H). N61 showed the lowest Y(NA) value and CS showed the highest Y(NA) value after rSP illumination (Supplementary Figures S5G,H). These results indicated that N61 showed lower susceptibility of PSI to its photoinhibition compared to CS. To understand the detailed mechanism that rSP illumination caused cultivar difference in Y(I) and Y(NA) among wheat cultivars, we studied the P700+ kinetics, from which the Y(I), Y(ND), and Y(NA) were determined (Supplementary Figure S6). As shown in Figure 4, the steady-state level of P700+ was higher in LL-grown wheat plants compared to that in HL-grown wheat plants before rSP illumination (Supplementary Figure S6). However, after rSP illumination, the steady-state level of P700+ was greatly lowered in both LL- and HL-grown wheat plants, which corresponded to the results of Y(ND) (Figure 9). The cultivar difference in Y(I) and Y(NA) was simply estimated by the difference from the pulse-induced P700+ to determine Y(I) and Y(NA) (Supplementary Figure S6).
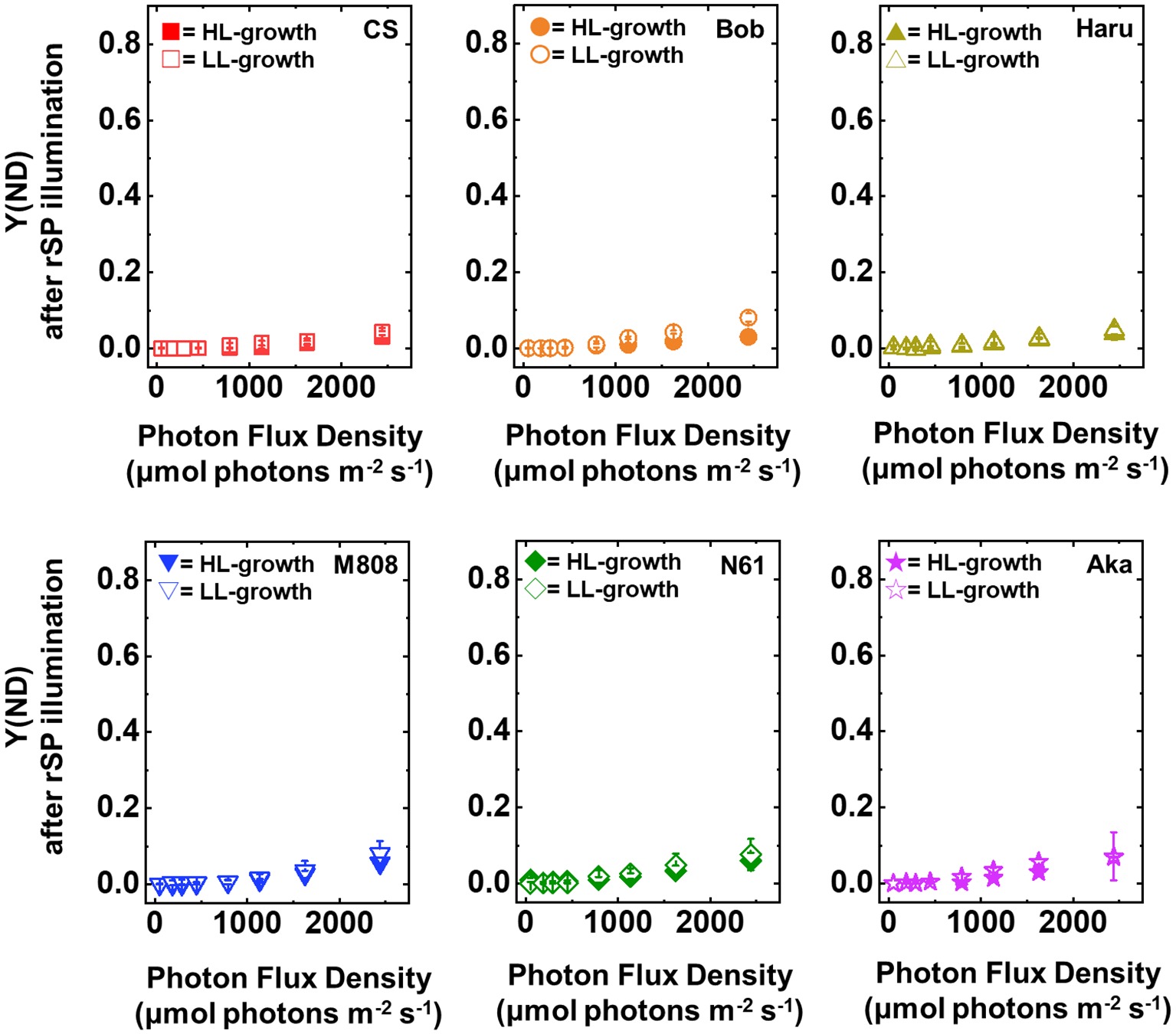
Figure 9. The response of Y(ND) to light intensity in six wheat cultivars grown under LL (open symbol) and HL (closed symbol) conditions after rSP illumination for 1 h. The measurement was conducted under atmospheric conditions (21 kPa O2, 40 Pa CO2) at 25°C. Data are expressed as mean ± SD of three to four independent experiments. Red squares indicate the results of CS, orange circles indicate the results of Bob, dark yellow triangles indicate the results of Haru, blue inverted-triangles indicate the results of M808, green diamonds indicate the results of N61, and purple stars indicate the results of Aka.
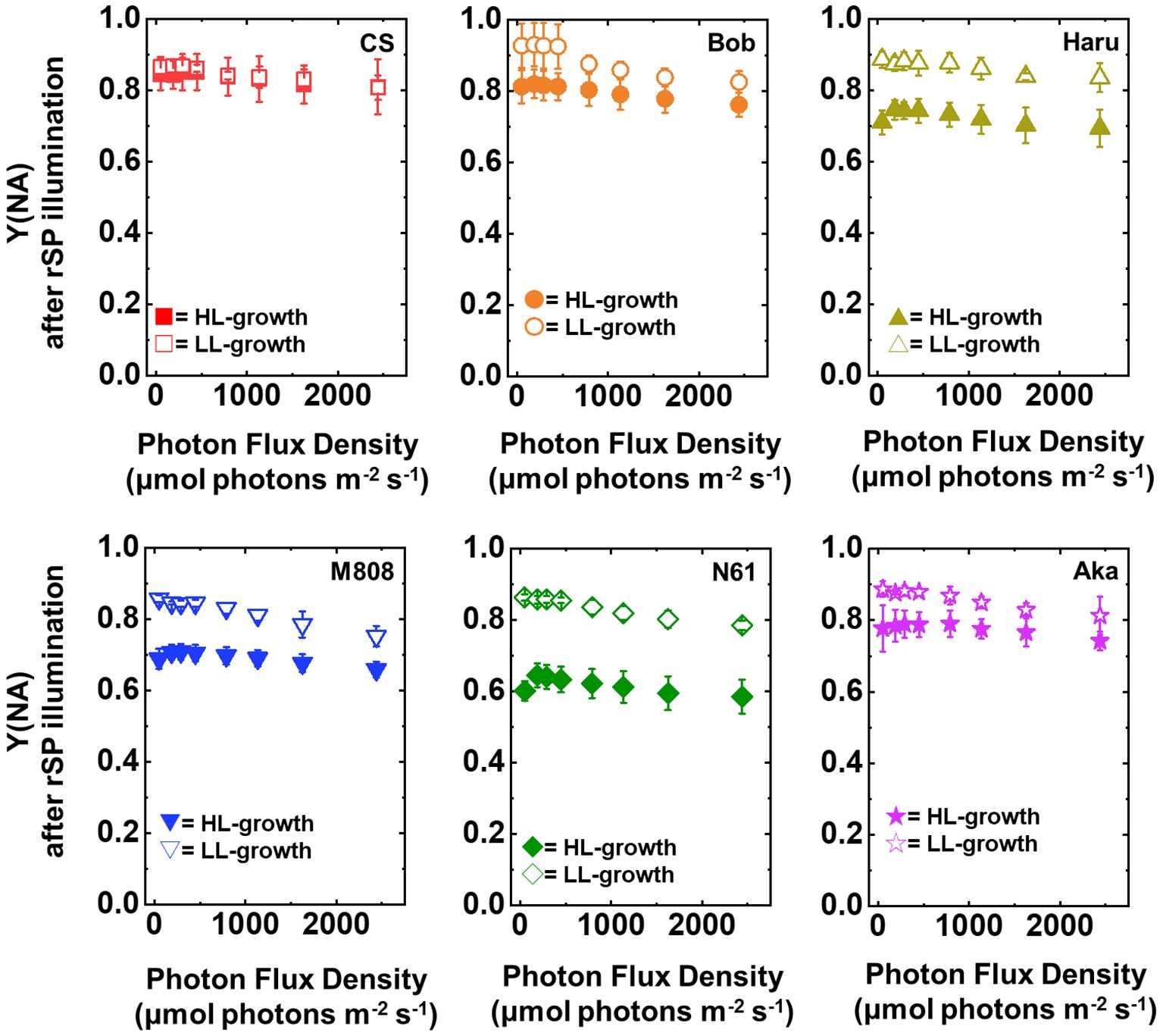
Figure 10. The response of Y(NA) to light intensity in six wheat cultivars grown under LL (open symbol) and HL (closed symbol) conditions after rSP illumination for 1 h. The measurement was conducted under atmospheric conditions (21 kPa O2, 40 Pa CO2) at 25°C. Data are expressed as mean ± SD of three to four independent experiments. Red squares indicate the results of CS, orange circles indicate the results of Bob, dark yellow triangles indicate the results of Haru, blue inverted-triangles indicate the results of M808, green diamonds indicate the results of N61, and purple stars indicate the results of Aka.
To address whether the susceptibility of PSI to its photoinhibition is determined by P700 oxidation during pulse illumination, we analyzed the P700 oxidation kinetics during one pulse illumination (Takagi et al., 2017a). As with the previous study, we studied the P700 oxidation kinetics at the beginning of rSP illumination to evaluate the effect of P700 oxidation kinetics on PSI photoinhibition (Takagi et al., 2017a). In LL-grown wheat cultivars, the rapid P700 oxidation was observed at the onset of pulse illumination (Figure 11). Subsequently, P700+ was reduced even under strong illumination. These results were similar to the results of our previous study on angiosperms (Takagi et al., 2017a). In the HL-grown wheat cultivars, P700 was also rapidly oxidized at the onset of pulse illumination; however, P700+ was not maintained in the oxidized state similar to that in the LL-grown wheat cultivars (Figure 11). Importantly, the LL-grown wheat cultivars showed partially higher P700+ at the end of pulse illumination (Figure 11). To check the statistical difference in the extent of P700+ during pulse illumination, we compared the P700+ value at the initial peak and at 100, 200, and 300 ms during the pulse illumination between LL- and HL-grown wheat cultivars. We found that LL-grown wheat plants showed higher P700+ especially at the end of pulse illumination (Figure 11). At the same time, we compared the extent of P700+ among wheat cultivars grown under LL and HL conditions respectively. Subsequently, we confirmed that P700+ kinetics during one pulse illumination did not show cultivar differences among LL- and HL-grown wheat cultivars respectively (ANOVA, p > 0.05). Therefore, the HL acclimation response and cultivar differences in susceptibility to PSI photoinhibition cannot be attributed to the P700 oxidation during pulse illumination.
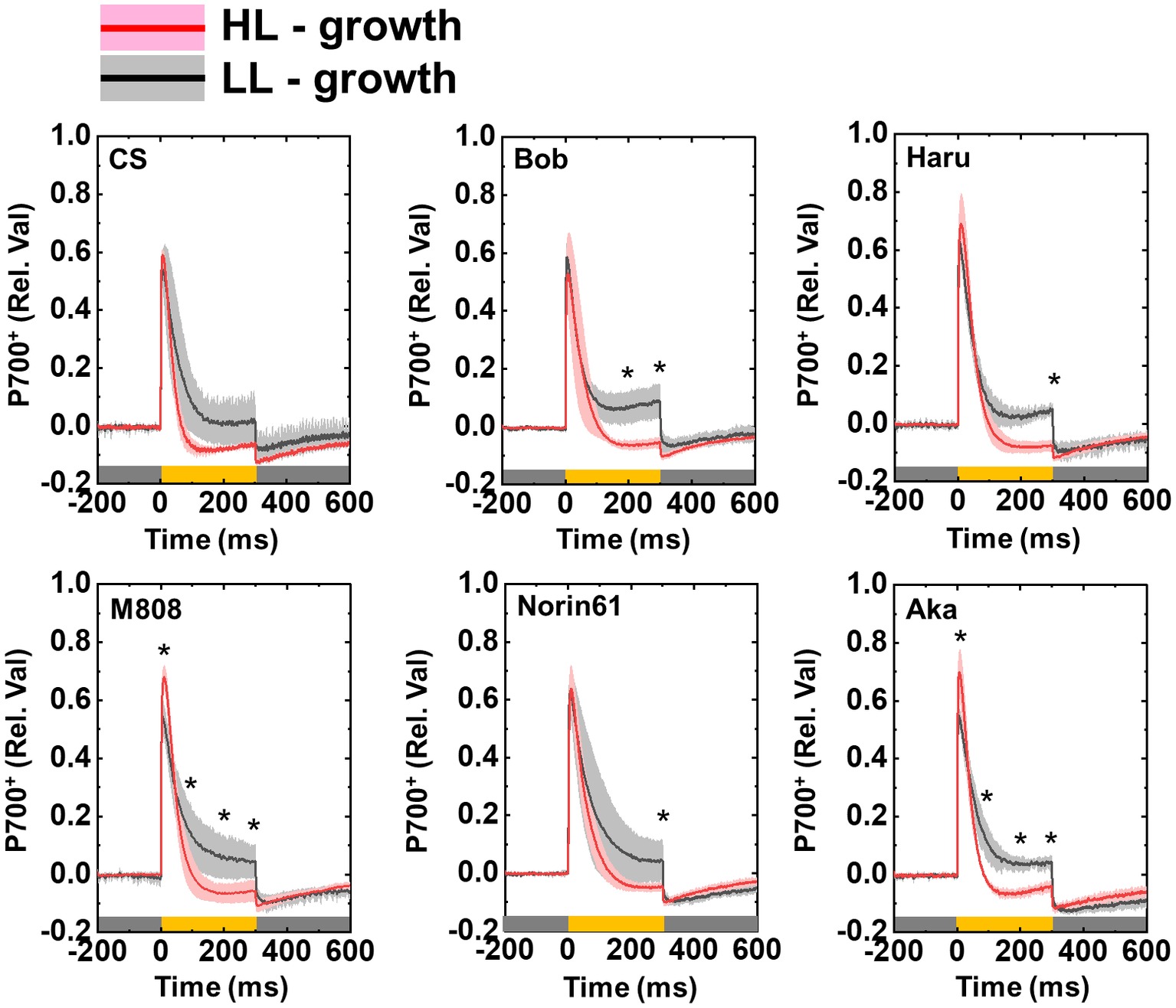
Figure 11. Effect of growth light intensity on the P700+ kinetics of wheat cultivars during the one-pulse illumination in rSP illumination. Black lines and gray shadows show the results of wheat cultivars grown under LL conditions, and red lines and pink shadows show the results of wheat cultivars grown under HL conditions. Experiments were performed three times independently, and the data were expressed as mean (solid line) ± SD (shade area). Before measurement, the leaves were kept in the dark for at least 1 h. Pulse illumination (20,000 μmol photons m−2 s−1, 300 ms) was applied to the leaves every 10 s in the dark at 25°C. Pulse was illuminated from the time “0” in this figure. Dark gray bars indicate the dark conditions and yellow bars indicate the pulse illumination. The difference in the extent of P700+ between LL- and HL-grown wheat plants was statistically analyzed at the times 100, 200, and 300 ms during pulse illumination, and peak value (Student’s t-test). Asterisks indicate the significant difference in the P700+ induction between LL- and HL-grown wheat plants (p < 0.05). The cultivar difference in P700+ kinetics was also analyzed among LL- and HL-grown wheat cultivars by Tukey-Kramer HSD test; however, no significant difference was detected.
Discussion
When land plants grow under LL intensity, they develop light-harvesting mechanisms for effective light absorption (De la Torre and Burkey, 1990; Bailey et al., 2001; Oguchi et al., 2003; Walters et al., 2003; Dietz, 2015; Bielczynski et al., 2016; Schumann et al., 2017). This is because photosynthesis is limited by light absorption under LL conditions (Roach and Krieger-Liszkay, 2014). On the other hand, for surviving under the strong light environment, land plants improved their electron sink capacities and photoprotective mechanisms in PSII (Miyata et al., 2015; Ware et al., 2015; Schumann et al., 2017). These responses contribute to escaping excessive electron accumulation in the photosynthetic electron transport chain and reducing the risk of ROS production and oxidative damage in chloroplasts. In this study, we characterized the effect of HL acclimation on the susceptibility of PSI to its photoinhibition by using rSP illumination (Sejima et al., 2014; Takagi et al., 2016a). Subsequently, we found that HL acclimation lowered the susceptibility of PSI to PSI photoinhibition depending on wheat cultivars (Figure 6). In general, the photosynthetic electron transport activity exceeds the electron sink capacity under HL illumination (Roach and Krieger-Liszkay, 2014). The excessive accumulation of electrons on the thylakoid membranes stimulates ROS production within PSI and causes oxidative damage to the photosynthetic apparatus in order to inhibit CO2 fixation (Zivcak et al., 2015). A decrease in the susceptibility of PSI to its photoinhibition would be a physiological mechanism to escape the risk of ROS-dependent oxidative damage. Until now, the effects of growth light conditions on PSI photoprotective mechanisms have been rarely studied (Sonoike et al., 1995a,b; Sonoike, 1996a, 1998; Ivanov et al., 1998), although the effects of HL acclimation on the photoprotective mechanisms in PSII have been greatly focused on. This would be due to the difficulty in evaluating the susceptibility to PSI photoinhibition compared to that of PSII. As discussed later, the effects of rSP illumination on PSI photochemistry and the chilling treatment on PSI photochemistry certainly resembled. Based on these facts, we suggest the possibility that rSP illumination would reproduce the effect of chilling stress on PSI photoinhibition independent of chilling temperature. Therefore, the results of this study would provide the opportunity to analyze the relationship between the photoprotective mechanisms of PSI and growth light conditions in the future.
The susceptibility of PSI to its photoinhibition was not differentiated between the spring-type and winter-type wheat plants. Several studies reported that the winter-type wheat plants showed an increase in photoprotective mechanisms in PSII by acclimating to HL or cold temperature (Hurry et al., 1995; Gray et al., 1996, 1997; Savitch et al., 2000; Pocock et al., 2001). However, we found no clear difference in the HL acclimation response to the susceptibility of PSI to its photoinhibition between the spring-type and winter-type wheat cultivars (Figure 6). In general, a difference of the two growth habits, spring-type and winter-type, is determined by vernalization requirement for a transition from the vegetative phase to the reproductive phase in wheat, and this phenotypic difference is mainly controlled by Vrn1 on the long arms of group 5 chromosomes (Fu et al., 2005). Genetic factors controlling frost and freezing tolerance might be present in the Vrn-1 chromosomal regions (Galiba et al., 2009), and the winter-type wheat cultivars should provide frost and freezing tolerance. It is still under discussion whether Vrn-1 itself could be a regulator of frost and freezing tolerance in wheat. The results of this study indicated that the Vrn-1 allelic differences between the spring-type and winter-type wheat cultivars are irrelevant to the acclimation response of PSI photoprotective mechanisms.
The photoinhibitory effects of rSP illumination would reproduce the PSI photoinhibition in chilling-sensitive plants under chilling treatment. Havaux and Davaud (1994) reported that chilling-stressed potato leaves decrease the P700 oxidation efficiency by illuminating FR light in addition to the maximum oxidizable P700, and the Emerson enhancement of photosynthetic O2 evolution is stimulated compared to non-chilling stressed leaves. Sonoike (1999) also showed a similar result by using cucumber leaves in vivo, and P700 oxidation was much slower under FR light illumination compared to non-stressed cucumber leaves. These results indicated that the quantum efficiency in PSI is lowered after PSI photoinhibition in chilling-sensitive plants by chilling treatment besides the decrease in P700 content. Here, we also observed the decrease in the light responsiveness of Y(I) after rSP illumination in all wheat leaves (Figure 8; Zivcak et al., 2015). These results correspond to the lower quantum efficiency in PSI (Terao and Katoh, 1996). Moreover, the quantum efficiency evaluated from dichlorophenolindophenol (DCIP) and methyl-viologen (MV)-dependent O2 absorption by PSI is also lowered in isolated chloroplasts causing PSI photoinhibition by rSP illumination (Takagi et al., 2016a). Based on these observations, the effects of chilling stress on PSI in chilling-sensitive plants and the effect of rSP illumination on PSI in land plants could be similar. In the previous reports, the decrease in the quantum efficiency in PSI has been explained as the stimulation of charge recombination reaction due to the higher acceptor side electron transport limitation (Havaux and Davaud, 1994) and the stimulation of CEF-PSI (Sonoike, 1999). In addition to those, the possibility that the antenna system of PSI is injured during PSI photoinhibition cannot be discarded (Scheller and Haldrup, 2005; Benson et al., 2015; Yamatani et al., 2018). Kok et al. (1965) showed that 77 K fluorescence emission corresponding to PSI-light harvesting complex (LHC) I was decreased after PSI photoinhibition. Furthermore, Rajagopal et al. (2005) reported that LHCI is target by ROS produced in PSI, and Tiwari et al. (2016) reported that the direct light absorption by PSI-core but not by LHCI is increased during the progress of PSI photoinhibition by using PROTON GRADIENT REGULATION 5 mutant (pgr5) in Arabidopsis thaliana which is susceptible to PSI photoinhibition (Munekage et al., 2002). Here we should note that we only evaluated the PSI photoinhibition by absorbance change in P700+ based on non-stressed leaves, and we calculated the redox parameters of PSI after rSP illumination based on the Pm before rSP illumination (see Materials and Methods). In this case, we cannot distinguish the cause of the change in PSI redox parameters by a qualitative change in electron transport within PSI (Sonoike et al., 1995a,b; Tiwari et al., 2016) or a quantitative change in PSI-LHCI complex and its subunits (Sonoike, 1996b; Tjus et al., 1999). In this study, we cannot conclude the cause of lowering the quantum yield of PSI after PSI photoinhibition. However, the general aspect of PSI photoinhibition to PSI photochemistry now can be addressed by using rSP illumination.
We proposed that two independent mechanisms operate for protecting PSI from its ROS-triggered photoinhibition. Recently, we suggested that the P700 oxidation system is important for reducing the risk of PSI photoinhibition in various photosynthetic organisms (Shimakawa et al., 2016, 2017, 2019; Takagi et al., 2016b, 2017a; Takagi and Miyake, 2018). The stimulation of P700 oxidation by P700 oxidation systems is achieved by limiting electron transport from PSII to PSI or stimulating an outflow of electrons from PSI (Shimakawa et al., 2016, 2017, 2019; Takagi et al., 2016b, 2017a). In this study, we analyzed the P700 oxidation kinetics during pulse illumination (Figure 11). However, compared to HL-grown wheat cultivars, LL-grown wheat cultivars showed higher P700+ during one pulse illumination, although the change in Pm after rSP illumination was greater in LL-grown wheat plants than HL-grown wheat plants (Figure 6). Moreover, we cannot detect the significant difference in the extent of P700+ during one pulse illumination between wheat cultivars grown under HL conditions (Figure 11). Therefore, the susceptibility of PSI to its photoinhibition cannot be explained by P700+ oxidation. The effect of HL acclimation on the susceptibility of PSI photoinhibition evaluated by rSP illumination agrees with the previous reports which showed the acclimation response of the susceptibility of PSI to its photoinhibition by using chilling stress and chilling-sensitive plants (Sonoike et al., 1995a,b; Sonoike, 1996a; Ivanov et al., 1998). Ivanov et al. (1998) proposed that the susceptibility of PSI to its photoinhibition depends on the down-regulation of PSII activity. This is a novel PSI protection mechanism to avoid the electron accumulation within PSI (Sonoike, 1996b; Tikkanen et al., 2014). However, during rSP illumination, wheat plants which maintained higher Pm after rSP illumination also maintained higher Y(II) during rSP illumination, and the change in Fv/Fm was also minor (Figure 6B, Supplementary Figure S3). Therefore, although the acclimation response of the susceptibility of PSI to its photoinhibition identified in this study is similar to previous reports (Ivanov et al., 1998), the protection mechanism of PSI which can be addressed by rSP illumination would be different from those addressed by chilling treatment. On the basis of P700+ kinetics during pulse illumination, the electron accumulation within PSI would be higher in HL-grown wheat cultivars than that in LL-grown wheat cultivars during rSP illumination (Figure 11). When we focused on the cause of PSI photoinhibition attributing to , the difference in the susceptibility of PSI to photoinhibition should be caused by production efficiency or the scavenging activity within the thylakoid membranes. On the other hand, the production of 1O2 within PSI cannot be monitored from the P700 oxidation kinetics. In fact, a 1O2 scavenger suppresses PSI photoinhibition induced by rSP illumination without affecting the P700+ kinetics during pulse illumination in isolated chloroplasts (Takagi et al., 2016a). The suppression of the charge separation from P700 to P700+ during pulse illumination increases the risk that excited P700 (*P700) within PSI triggers 1O2 production through 3P700 (Takagi et al., 2016a). From this view, we additionally suggest that the difference in the susceptibility of PSI to its photoinhibition might be determined by the 1O2-scavenging or quenching mechanisms of *P700 or 3P700 to suppress the production of 1O2 (Telfer et al., 1994; Rajagopal et al., 2005; Cazzaniga et al., 2012; Dall’Osto et al., 2012; Ballottari et al., 2014). In summary, we propose a new photoprotective mechanism in PSI whose capacity is modulated by growth light environment, and this PSI photoprotective mechanism would cooperate with P700 oxidation system to reduce the risk of PSI photoinhibition caused by ROS.
We suggest that leaf chlorophyll content would be one important determinant of the susceptibility of PSI to its photoinhibition. Unfortunately, we cannot distinguish the susceptibility of PSI to its photoinhibition from the acclimation response of Y(II), Y(I), Y(ND), and Y(NA). This is because all wheat cultivars showed a similar change in these photosynthetic parameters toward the LL and HL growth conditions (Figures 2–5, Supplementary Figure S1). We also examined the non-photochemical quenching (NPQ) in both LL- and HL-grown wheat cultivars (Supplementary Figure S7). In LL-grown wheat plants, all wheat cultivars showed similar NPQ in the light curve analysis (Supplementary Figure S7). HL-grown wheat cultivars showed lower NPQ in the light curve analysis compared to LL-grown wheat cultivars, and also showed cultivar differences in the light curve analysis (Supplementary Figure S7). Generally, electron sink capacity is increased when land plants grow under HL conditions as observed in Figures 2, 3 (Bailey et al., 2001; Oguchi et al., 2003; Dahal et al., 2012; Schumann et al., 2017). Therefore, the induction of NPQ would be lowered in HL-grown wheat cultivars compared to LL-grown wheat cultivars under strong illumination (Ballottari et al., 2007). In addition to the change in electron sink capacity during HL acclimation, PSII:PSI ratio, PSII:LHCII ratio, and the protein contents which are involved in NPQ like PsbS, violaxanthin de-epoxidase (VDE), and zeaxanthin epoxidase (ZE) are also changed depending on the growth light (Bailey et al., 2001; Ballottari et al., 2007; Kouřil et al., 2013). These changes might be caused by the cultivar differences grown under HL conditions. In spite of the cultivar difference in NPQ in the light curve analysis, NPQ induction was not different between wheat cultivars during rSP illumination (Supplementary Figure S8). During rSP illumination, NPQ was slowly induced (Supplementary Figure S8). This would be due to the progress of PSII photoinhibition as observed in the decrease in Y(II) during rSP illumination (Supplementary Figure S3). From these observations, we suggest that the susceptibility of PSI to its photoinhibition cannot be determined by the NPQ kinetics in the light curve analysis and during rSP illumination. With the responses of photosynthetic parameters to the growth light conditions, the total chlorophyll contents and chlorophyll a/b ratio showed significant differences among the wheat cultivars grown under HL conditions (Figure 1). Based on this observation, we plotted the relationship between the total chlorophyll contents, chlorophyll a/b ratio, and the susceptibility of PSI to photoinhibition. Subsequently, we observed a positive linear relationship between the total chlorophyll content and the residual activity of PSI after rSP illumination, although the chlorophyll a/b ratio showed no significant relationship to the residual activity of PSI after rSP illumination (Figure 12). Therefore, the light acclimation response of the susceptibility of PSI to photoinhibition would be changed with the change in chlorophyll content in leaves in wheat cultivars. Such correlation has not been observed before (Sonoike et al., 1995a,b). This would be a hint for elucidating the molecular mechanism to determine the susceptibility of PSI to photoinhibition for future research. Alboresi et al. (2009) reported that the susceptibility of PSI to its photoinhibition depends on the PSI-LHCI complex formation. The binding of carotenoids in LHCI is also an important factor to determine the ROS production (Cazzaniga et al., 2012; Dall’Osto et al., 2012; Ballottari et al., 2014). As discussed above, LHCI is a possible target of PSI photoinhibition. Therefore, LHCI is likely involved in PSI photoinhibition. Furthermore, because the chlorophyll a/b ratio is higher in LHCI (1.8–5.5) than in LHCII (1.15–1.5) (Hemelrijk et al., 1992; Morosinotto et al., 2003), the quantity of LHCI hardly affects the leaf chlorophyll a/b ratio (Wientjes et al., 2009; Yamatani et al., 2018). If our hypothesis mentioned above is correct, exploration of the interaction between susceptibility to PSI photoinhibition, LHCI content and energy transfer from LHCI to PSI core should certainly lead to the elucidation of the detailed mechanisms of the PSI photoinhibition in planta.
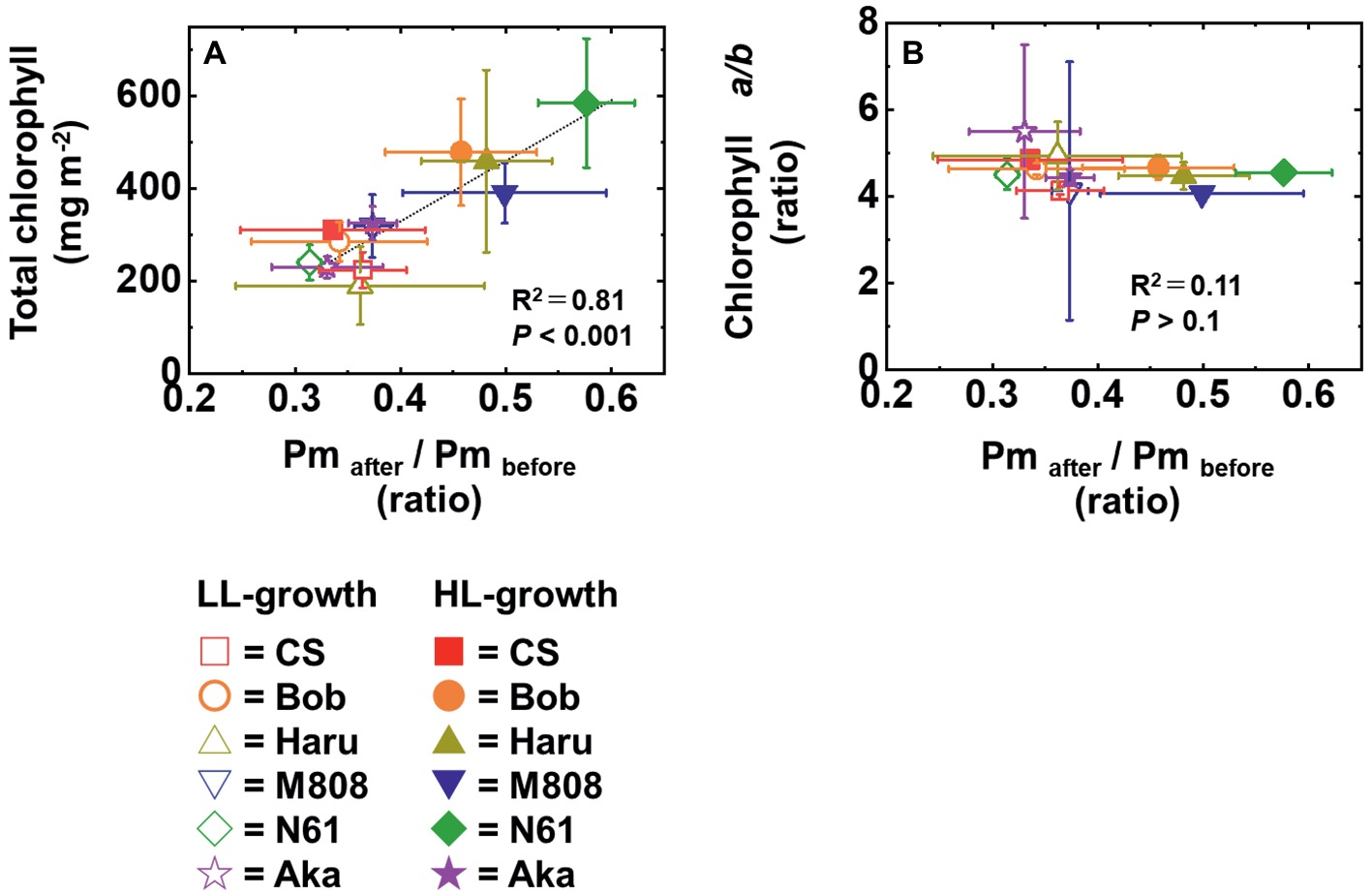
Figure 12. The relationship between the change in Pm by rSP illumination for 1 h and total chlorophyll content in leaves (A) and between the change in Pm by rSP illumination for 1 h and chlorophyll a/b ratio (B). The data of the change in Pm after rSP illumination were used from Figure 6B, the data of the total chlorophyll content were used from Figure 1B, and the data of the total chlorophyll a/b ratio were used from Figure 1C. Data were expressed as mean ± SD of three to five independent experiments. R2 represents the determination coefficient of the linear regression analysis. R2 and p were determined by fitting a linear regression model (see section Materials and methods). The linear regression line determined by the statistical analysis is shown as the black broken line.
In addition to the change in the susceptibility of PSI to its photoinhibition in leaves, we found that the wheat plants grown under both LL and HL conditions keep Y(NA) low and maintain similar values under the illumination (Figure 5). The value of Y(NA) is determined by the balance of electron inflow and outflow within PSI (Klughammer and Schreiber, 1994; Shimakawa et al., 2016, 2017, 2019; Takagi et al., 2016b, 2017a; Takagi and Miyake, 2018). In other words, when wheat plants possess electron sink capacities lower than photosynthetic electron transport reactions, they build up Y(ND) to decrease Y(NA) (Figures 4, 5; Takagi et al., 2016b, 2017b; Wada et al., 2018). On the other hand, when wheat plants possess large electron sink capacities, they relax Y(ND) and stimulate energy production for driving photosynthesis as can be judged from the change in Y(I) (Figure 3; Takagi et al., 2016b, 2017b; Wada et al., 2018). This would be modulated by P700 oxidation systems (Shimakawa et al., 2016, 2017, 2019; Takagi et al., 2016b, 2017a; Takagi and Miyake, 2018). We propose that a P700 oxidation system could respond to growth light intensity and flexibly control the redox state of PSI at the steady state photosynthesis to reduce the risk of ROS.
Here, we proposed that rSP illumination is a useful method to elucidate the mechanism of PSI photoinhibition in vivo. In the present study, rSP illumination showed an HL acclimation effect on PSI photoinhibition and cultivar differences in PSI photoinhibition after HL acclimation among the studied wheat cultivars. To our knowledge, this is the first study that showed the growth light acclimation response of the susceptibility of PSI to its photoinhibition by using rSP illumination. Therefore, rSP illumination enables the researchers to explore the new world of PSI photoprotection mechanisms by applying rSP illumination to various plant species more easily. At this stage, we have not yet identified the key factor underlying the difference in PSI photoinhibition among the studied wheat cultivars. However, it would be possible to isolate the causative gene by the forward genetic experiments like the quantitative trait locus (QTL) analysis combined with rSP illumination because the HL acclimation response of the susceptibility of PSI showed cultivar differences. We also suggest the combined analysis of rSP illumination and chilling treatment would contribute to elucidating the general protection mechanisms of PSI to its photoinhibition. Under chilling treatment, PSI photoinhibition can be easily caused by low light illumination in intact leaves (Sonoike and Terashima, 1994; Terashima et al., 1994; Sonoike, 1999). At that time, various changes in the temperature-dependent biochemical reaction would cause the susceptibility of PSI to its photoinhibition (ex. Lipid fluidity or enzyme stabilities/activities) (Sonoike, 1996a, 1998). On the other hand, rSP illumination can cause PSI photoinhibition under room temperature. The two PSI photoinhibitory treatments could distinguish photoprotective mechanism of PSI, which is involved in temperature-dependent components or temperature-independent components. For example, under chilling treatment, the uncoupling of chloroplastic ATP synthase stimulates the PSI photoinhibition through the over-reduction of PSI by suppressing ΔpH-dependent photosynthetic electron transport regulation (Terashima et al., 1991a,b). In contrast, PSI photoinhibition induced by rSP illumination in the dark does not affect the ΔpH formation ability because short pulse cannot build up enough ΔpH during rSP illumination under the dark conditions (Takagi et al., 2017b). We hope that our present results trigger a wide range of interest in PSI photoinhibition as well as PSII photoinhibition and the key factor to be identified in the future.
Author Contributions
DT, ST, and CM contributed to the conceptualization of this study. DT and HI carried out investigations. DT and ST prepared the original draft. DT, HI, ST, and CM wrote, reviewed, and edited the manuscript. CM contributed to funding acquisition.
Funding
This work was supported by the Core Research for Environmental Science and Technology (Scientific Research Grant No. AL65D21010 to CM).
Conflict of Interest Statement
The authors declare that the research was conducted in the absence of any commercial or financial relationships that could be construed as a potential conflict of interest.
Acknowledgments
The authors thank the HOKUREN Federation of Agricultural Cooperatives for providing them with the seeds of Haruyokoi. The authors also thank the Editage (Cactus Communications Inc., https://www.editage.jp/) for editing the present manuscript.
Supplementary Material
The Supplementary Material for this article can be found online at: https://www.frontiersin.org/articles/10.3389/fpls.2019.00686/full#supplementary-material
References
Alboresi, A., Ballottari, M., Hienerwadel, R., Giacometti, G. M., and Morosinotto, T. (2009). Antenna complexes protect photosystem I from photoinhibition. BMC Plant Biol. 9:71. doi: 10.1186/1471-2229-9-71
Alter, P., Dreissen, A., Luo, F. L., and Matsubara, S. (2012). Acclimatory responses of Arabidopsis to fluctuating light environment: comparison of different sunfleck regimes and accessions. Photosynth. Res. 113, 221–237. doi: 10.1007/s11120-012-9757-2
Atkin, O. K., and Tjoelker, M. G. (2003). Thermal acclimation and the dynamic response of plant respiration to temperature. Trends Plant Sci. 8, 343–351. doi: 10.1016/S1360-1385(03)00136-5
Bailey, S., Walters, R. G., Jansson, S., and Horton, P. (2001). Acclimation of Arabidopsis thaliana to the light environment: the existence of separate low light and high light responses. Planta 213, 794–801. doi: 10.1007/s004250100556
Baker, N. R. (2008). Chlorophyll fluorescence: a probe of photosynthesis in vivo. Annu. Rev. Plant Biol. 59, 89–113. doi: 10.1146/annurev.arplant.59.032607.092759
Ballottari, M., Alcocer, M. J., D’Andrea, C., Viola, D., Ahn, T. K., Petrozza, A., et al. (2014). Regulation of photosystem I light harvesting by zeaxanthin. Proc. Natl. Acad. Sci. USA 111, E2431–E2438. doi: 10.1073/pnas.1404377111
Ballottari, M., Dall’Osto, L., Morosinotto, T., and Bassi, R. (2007). Contrasting behavior of higher plant photosystem I and II antenna systems during acclimation. J. Biol. Chem. 282, 8947–8958. doi: 10.1074/jbc.M606417200
Benson, S. L., Maheswaran, P., Ware, M. A., Hunter, C. N., Horton, P., Jansson, S., et al. (2015). An intact light harvesting complex I antenna system is required for complete state transitions in Arabidopsis. Nat. Plants 1:15176. doi: 10.1038/nplants.2015.176
Bielczynski, L. W., Schansker, G., and Croce, R. (2016). Effect of light acclimation on the organization of photosystem II super- and sub-complexes in Arabidopsis thaliana. Front. Plant Sci. 7:105. doi: 10.3389/fpls.2016.00105
Cazzaniga, S., Li, Z., Niyogi, K. K., Bassi, R., and Dall’Osto, L. (2012). The Arabidopsis szl1 mutant reveals a critical role of β-carotene in photosystem I photoprotection. Plant Physiol. 159, 1745–1758. doi: 10.1104/pp.112.201137
Cohu, C. M., Muller, O., Adams, W. W., and Demmig-Adams, B. (2014). Leaf anatomical and photosynthetic acclimation to cool temperature and high light in two winter versus two summer annuals. Physiol. Plant. 152, 164–173. doi: 10.1111/ppl.12154
Dahal, K., Kane, K., Gadapati, W., Webb, E., Savitch, L. V., Singh, J., et al. (2012). The effects of phenotypic plasticity on photosynthetic performance in winter rye, winter wheat and Brassica napus. Physiol. Plant. 144, 169–188. doi: 10.1111/j.1399-3054.2011.01513.x
DalCorso, G., Pesaresi, P., Masiero, S., Aseeva, E., Schünemann, D., Finazzi, G., et al. (2008). A complex containing PGRL1 and PGR5 is involved in the switch between linear and cyclic electron flow in Arabidopsis. Cell 132, 273–285. doi: 10.1016/j.cell.2007.12.028
Dall’Osto, L., Holt, N. E., Kaligotla, S., Fuciman, M., Cazzaniga, S., Carbonera, D., et al. (2012). Zeaxanthin protects plant photosynthesis by modulating chlorophyll triplet yield in specific light-harvesting antenna subunits. J. Biol. Chem. 287, 41820–41834. doi: 10.1074/jbc.M112.405498
De la Torre, W. R., and Burkey, K. O. (1990). Acclimation of barley to changes in light intensity: photosynthetic electron transport activity and components. Photosynth. Res. 24, 127–136. doi: 10.1007/BF00032593
Dietz, K. J. (2015). Efficient high light acclimation involves rapid processes at multiple mechanistic levels. J. Exp. Bot. 66, 2401–2414. doi: 10.1093/jxb/eru505
Dumlao, M. R., Darehshouri, A., Cohu, C. M., Muller, O., Mathias, J., Adams, W. W. III, et al. (2012). Low temperature acclimation of photosynthetic capacity and leaf morphology in the context of phloem loading type. Photosynth. Res. 113, 181–189. doi: 10.1007/s11120-012-9762-5
Ensminger, I., Busch, F., and Huner, N. (2006). Photostasis and cold acclimation: sensing low temperature through photosynthesis. Physiol. Plant. 126, 28–44. doi: 10.1111/j.1399-3054.2006.00627.x
Fu, D., Szűcs, P., Yan, L., Helguera, M., Skinner, J. S., Von Zitzewitz, J., et al. (2005). Large deletions within the first intron in VRN-1 are associated with spring growth habit in barley and wheat. Mol. Gen. Genomics. 273, 54–65. doi: 10.1007/s00438-004-1095-4
Galiba, G., Vágújfalvi, A., Li, C., Soltész, A., and Dubcovsky, J. (2009). Regulatory genes involved in the determination of frost tolerance in temperate cereals. Plant Sci. 176, 12–19. doi: 10.1016/j.plantsci.2008.09.016
Goldschmidt-Clermont, M., and Roberto, B. (2015). Sharing light between two photosystems: mechanism of state transitions. Curr. Opin. Plant Biol. 25, 71–78. doi: 10.1016/j.pbi.2015.04.009
Gray, G. R., Chauvin, L. P., Sarhan, F., and Huner, N. P. (1997). Cold acclimation and freezing tolerance (a complex interaction of light and temperature). Plant Physiol. 114, 467–474. doi: 10.1104/pp.114.2.467
Gray, G. R., Savitch, L. V., Ivanov, A. G., and Huner, N. P. (1996). Photosystem II excitation pressure and development of resistance to photoinhibition (II. Adjustment of photosynthetic capacity in winter wheat and winter rye). Plant Physiol. 110, 61–71. doi: 10.1104/pp.110.1.61
Havaux, M., and Davaud, A. (1994). Photoinhibition of photosynthesis in chilled potato leaves is not correlated with a loss of photosystem-II activity. Photosynth. Res. 40, 75–92. doi: 10.1007/BF00019047
Hemelrijk, P. W., Kwa, S. L., van Grondelle, R., and Dekker, J. P. (1992). Spectroscopic properties of LHC-II, the main light-harvesting chlorophyll a/b protein complex from chloroplast membranes. Biochim. Biophys. Acta Bioenerg. 1098, 159–166. doi: 10.1016/S0005-2728(05)80331-7
Hüner, N. P., Dahal, K., Kurepin, L. V., Savitch, L., Singh, J., Ivanov, A. G., et al. (2014). Potential for increased photosynthetic performance and crop productivity in response to climate change: role of CBFs and gibberellic acid. Front. Chem. 2:18. doi: 10.3389/fchem.2014.00018
Hüner, N., Öquist, G., and Sarhan, F. (1998). Energy balance and acclimation to light and cold. Trends Plant Sci. 3, 224–230. doi: 10.1016/S1360-1385(98)01248-5
Hurry, V. M., Strand, A., Tobiaeson, M., Gardestrom, P., and Oquist, G. (1995). Cold hardening of spring and winter wheat and rape results in differential effects on growth, carbon metabolism, and carbohydrate content. Plant Physiol. 109, 697–706. doi: 10.1104/pp.109.2.697
Ivanov, A. G., Morgan, R. M., Gray, G. R., Velitchkova, M. Y., and Huner, N. P. A. (1998). Temperature/light dependent development of selective resistance to photoinhibition of photosystem I. FEBS Lett. 430, 288–292. doi: 10.1016/S0014-5793(98)00681-4
Klughammer, C., and Schreiber, U. (1994). An improved method, using saturating light pulses, for the determination of photosystem I quantum yield via P700+-absorbance changes at 830 nm. Planta 192, 261–268. doi: 10.1007/BF00194461
Kok, B., Gassner, E. S., and Rurainski, H. J. (1965). Photoinhibition of chloroplast reactions. Photochem. Photobiol. 4, 215–227. doi: 10.1111/j.1751-1097.1965.tb05739.x
Kono, M., Noguchi, K., and Terashima, I. (2014). Roles of the cyclic electron flow around PSI (CEF-PSI) and O2-dependent alternative pathways in regulation of the photosynthetic electron flow in short-term fluctuating light in Arabidopsis thaliana. Plant Cell Physiol. 55, 990–1004. doi: 10.1093/pcp/pcu033
Kouřil, R., Wientjes, E., Bultema, J. B., Croce, R., and Boekema, E. J. (2013). High-light vs. low-light: effect of light acclimation on photosystem II composition and organization in Arabidopsis thaliana. Biochim. Biophys. Acta Bioenerg. 1827, 411–419. doi: 10.1016/j.bbabio.2012.12.003
Liu, J., and Last, R. L. (2017). A chloroplast thylakoid lumen protein is required for proper photosynthetic acclimation of plants under fluctuating light environments. Proc. Natl. Acad. Sci. USA 114, E8110–E8117. doi: 10.1073/pnas.1712206114
Makino, A., and Sage, R. F. (2007). Temperature response of photosynthesis in transgenic rice transformed with ‘sense’ or ‘antisense’ rbcS. Plant Cell Physiol. 48, 1472–1483. doi: 10.1093/pcp/pcm118
Miyake, C., Horiguchi, S., Makino, A., Shinzaki, Y., Yamamoto, H., and Tomizawa, K. I. (2005). Effects of light intensity on cyclic electron flow around PSI and its relationship to non-photochemical quenching of Chl fluorescence in tobacco leaves. Plant Cell Physiol. 46, 1819–1830. doi: 10.1093/pcp/pci197
Miyata, K., Ikeda, H., Nakaji, M., Kanel, D. R., and Terashima, I. (2015). Rate constants of PSII photoinhibition and its repair, and PSII fluorescence parameters in field plants in relation to their growth light environments. Plant Cell Physiol. 56, 1841–1854. doi: 10.1093/pcp/pcv107
Morosinotto, T., Breton, J., Bassi, R., and Croce, R. (2003). The nature of a chlorophyll ligand in Lhca proteins determines the far red fluorescence emission typical of photosystem I. J. Biol. Chem. 278, 49223–49229. doi: 10.1074/jbc.M309203200
Müller, P., Li, X. P., and Niyogi, K. K. (2001). Non-photochemical quenching. A response to excess light energy. Plant Physiol. 125, 1558–1566. doi: 10.1104/pp.125.4.1558
Munekage, Y., Hojo, M., Meurer, J., Endo, T., Tasaka, M., and Shikanai, T. (2002). PGR5 is involved in cyclic electron flow around photosystem I and is essential for photoprotection in Arabidopsis. Cell 110, 361–371. doi: 10.1016/S0092-8674(02)00867-X
Nishiyama, Y., Allakhverdiev, S. I., and Murata, N. (2011). Protein synthesis is the primary target of reactive oxygen species in the photoinhibition of photosystem II. Physiol. Plant. 142, 35–46. doi: 10.1111/j.1399-3054.2011.01457.x
Noguchi, K., Yamori, W., Hikosaka, K., and Terashima, I. (2015). Homeostasis of the temperature sensitivity of respiration over a range of growth temperatures indicated by a modified Arrhenius model. New Phytol. 207, 34–42. doi: 10.1111/nph.13339
Oguchi, R., Hikosaka, K., and Hirose, T. (2003). Does the photosynthetic light-acclimation need change in leaf anatomy? Plant Cell Environ. 26, 505–512. doi: 10.1046/j.1365-3040.2003.00981.x
Pocock, T. H., Hurry, V., Savitch, L. V., and Huner, N. (2001). Susceptibility to low-temperature photoinhibition and the acquisition of freezing tolerance in winter and spring wheat: the role of growth temperature and irradiance. Physiol. Plant. 113, 499–506. doi: 10.1034/j.1399-3054.2001.1130408.x
Porra, R. J., Thompson, W. A., and Kriedemann, P. E. (1989). Determination of accurate extinction coefficients and simultaneous equations for assaying chlorophylls a and b extracted with four different solvents: verification of the concentration of chlorophyll standards by atomic absorption spectroscopy. Biochim. Biophys. Acta Bioenerg. 975, 384–394. doi: 10.1016/S0005-2728(89)80347-0
Rajagopal, S., Joly, D., Gauthier, A., Beauregard, M., and Carpentier, R. (2005). Protective effect of active oxygen scavengers on protein degradation and photochemical function in photosystem I submembrane fractions during light stress. FEBS J. 272, 892–902. doi: 10.1111/j.1742-4658.2004.04512.x
Roach, T., and Krieger-Liszkay, A. (2014). Regulation of photosynthetic electron transport and photoinhibition. Curr. Protein Pept. Sci. 15, 351–362. doi: 10.2174/1389203715666140327105143
Sage, R. F., Way, D. A., and Kubien, D. S. (2008). Rubisco, Rubisco activase, and global climate change. J. Exp. Bot. 59, 1581–1595. doi: 10.1093/jxb/ern053
Savitch, L. V., Barker-Åstrom, J., Ivanov, A. G., Hurry, V., Öquist, G., Huner, N. P., et al. (2001). Cold acclimation of Arabidopsis thaliana results in incomplete recovery of photosynthetic capacity, associated with an increased reduction of the chloroplast stroma. Planta 214, 295–303. doi: 10.1007/s004250100622
Savitch, L. V., Harney, T., and Huner, N. (2000). Sucrose metabolism in spring and winter wheat in response to high irradiance, cold stress and cold acclimation. Physiol. Plant. 108, 270–278. doi: 10.1034/j.1399-3054.2000.108003270.x
Scheller, H. V., and Haldrup, A. (2005). Photoinhibition of photosystem I. Planta 221, 5–8. doi: 10.1007/s00425-005-1507-7
Schumann, T., Paul, S., Melzer, M., Dörmann, P., and Jahns, P. (2017). Plant growth under natural light conditions provides highly flexible short-term acclimation properties toward high light stress. Front. Plant Sci. 8:681. doi: 10.3389/fpls.2017.00681
Sejima, T., Takagi, D., Fukayama, H., Makino, A., and Miyake, C. (2014). Repetitive short-pulse light mainly inactivates photosystem I in sunflower leaves. Plant Cell Physiol. 55, 1184–1193. doi: 10.1093/pcp/pcu061
Shaku, K., Shimakawa, G., Hashiguchi, M., and Miyake, C. (2015). Reduction-induced suppression of electron flow (RISE) in the photosynthetic electron transport system of Synechococcus elongatus PCC 7942. Plant Cell Physiol. 57, 1443–1453. doi: 10.1093/pcp/pcv198
Shimakawa, G., Ishizaki, K., Tsukamoto, S., Tanaka, M., Sejima, T., and Miyake, C. (2017). The liverwort, Marchantia, drives alternative electron flow using a flavodiiron protein to protect PSI. Plant Physiol. 173, 1636–1647. doi: 10.1104/pp.16.01038
Shimakawa, G., Murakami, A., Niwa, K., Matsuda, Y., Wada, A., and Miyake, C. (2019). Comparative analysis of strategies to prepare electron sinks in aquatic photoautotrophs. Photosynth. Res. 139, 401–411. doi: 10.1007/s11120-018-0522-z
Shimakawa, G., Shaku, K., and Miyake, C. (2016). Oxidation of P700 in photosystem I is essential for the growth of cyanobacteria. Plant Physiol. 172, 1443–1450. doi: 10.1104/pp.16.01227
Sonoike, K. (1995). Selective photoinhibition of photosystem I in isolated thylakoid membranes from cucumber and spinach. Plant Cell Physiol. 36, 825–830. doi: 10.1093/oxfordjournals.pcp.a078827
Sonoike, K. (1996a). Photoinhibition of photosystem I: its physiological significance in the chilling sensitivity of plants. Plant Cell Physiol. 37, 239–247. doi: 10.1093/oxfordjournals.pcp.a028938
Sonoike, K. (1996b). Degradation of psaB gene product, the reaction center subunit of photosystem I, is caused during photoinhibition of photosystem I: possible involvement of active oxygen species. Plant Sci. 115, 157–164. doi: 10.1016/0168-9452(96)04341-5
Sonoike, K. (1998). Various aspects of inhibition of photosynthesis under light/chilling stress: “photoinhibition at chilling temperatures” versus “chilling damage in the light”. J. Plant Res. 111, 121–129. doi: 10.1007/BF02507158
Sonoike, K. (1999). The different roles of chilling temperatures in the photoinhibition of photosystem I and photosystem II. J. Photochem. Photobiol. B Biol. 48, 136–141. doi: 10.1016/S1011-1344(99)00030-5
Sonoike, K. (2011). Photoinhibition of photosystem I. Physiol. Plant. 142, 56–64. doi: 10.1111/j.1399-3054.2010.01437.x
Sonoike, K., Ishibashi, M., and Watanabe, A. (1995a). “Chilling sensitive steps in leaves of Phaseolus vulgaris L. examination of the effects of growth irradiances on PSI photoinhibition” in Photosynthesis: From light to biosphere. ed. P. Matis, vol. IV (The Hague: Kluwer Academic Publishers), 853–856.
Sonoike, K., and Terashima, I. (1994). Mechanism of photosystem-I photoinhibition in leaves of Cucumis sativus L. Planta 194, 287–293. doi: 10.1007/BF01101690
Sonoike, K., Terashima, I., Iwaki, M., and Itoh, S. (1995b). Destruction of photosystem I iron-sulfur centers in leaves of Cucumis sativus L. by weak illumination at chilling temperatures. FEBS Lett. 362, 235–238. doi: 10.1016/0014-5793(95)00254-7
Suorsa, M., Järvi, S., Grieco, M., Nurmi, M., Pietrzykowska, M., Rantala, M., et al. (2012). PROTON GRADIENT REGULATION5 is essential for proper acclimation of Arabidopsis photosystem I to naturally and artificially fluctuating light conditions. Plant Cell 24, 2934–2948. doi: 10.1105/tpc.112.097162
Suorsa, M., Rantala, M., Mamedov, F., Lespinasse, M., Trotta, A., Grieco, M., et al. (2015). Light acclimation involves dynamic re-organization of the pigment-protein megacomplexes in non-appressed thylakoid domains. Plant J. 84, 360–373. doi: 10.1111/tpj.13004
Takagi, D., Amako, K., Hashiguchi, M., Fukaki, H., Ishizaki, K., Goh, T., et al. (2017b). Chloroplastic ATP synthase builds up a proton motive force preventing production of reactive oxygen species in photosystem I. Plant J. 91, 306–324. doi: 10.1111/tpj.13566
Takagi, D., Hashiguchi, M., Sejima, T., Makino, A., and Miyake, C. (2016b). Photorespiration provides the chance of cyclic electron flow to operate for the redox-regulation of P700 in photosynthetic electron transport system of sunflower leaves. Photosynth. Res. 129, 279–290. doi: 10.1007/s11120-016-0267-5
Takagi, D., Ishizaki, K., Hanawa, H., Mabuchi, T., Shimakawa, G., Yamamoto, H., et al. (2017a). Diversity of strategies for escaping reactive oxygen species production within photosystem I among land plants: P700 oxidation system is prerequisite for alleviating photoinhibition in photosystem I. Physiol. Plant. 161, 56–74. doi: 10.1111/ppl.12562
Takagi, D., and Miyake, C. (2018). Proton gradient regulation 5 supports linear electron flow to oxidize photosystem I. Physiol. Plant. 164, 337–348. doi: 10.1111/ppl.12723
Takagi, D., Takumi, S., Hashiguchi, M., Sejima, T., and Miyake, C. (2016a). Superoxide and singlet oxygen produced within the thylakoid membranes both cause photosystem I photoinhibition. Plant Physiol. 171, 1626–1634. doi: 10.1104/pp.16.00246
Telfer, A., Dhami, S., Bishop, S. M., Phillips, D., and Barber, J. (1994). β-carotene quenches singlet oxygen formed by isolated photosystem II reaction centers. Biochemistry 33, 14469–14474. doi: 10.1021/bi00252a013
Terao, T., and Katoh, S. (1996). Antenna sizes of photosystem I and photosystem II in chlorophyll b-deficient mutants of rice. Evidence for an antenna function of photosystem II centers that are inactive in electron transport. Plant Cell Physiol. 37, 307–312. doi: 10.1093/oxfordjournals.pcp.a028947
Terashima, I., Funayama, S., and Sonoike, K. (1994). The site of photoinhibition in leaves of Cucumis sativus L. at low temperatures is photosystem I, not photosystem II. Planta 193, 300–306. doi: 10.1007/BF00192544
Terashima, I., Kashino, Y., and Katoh, S. (1991a). Exposure of leaves of Cucumis sativus L. to low temperatures in the light causes uncoupling of thylakoids I. Studies with isolated thylakoids. Plant Cell Physiol. 32, 1267–1274. doi: 10.1093/oxfordjournals.pcp.a078205
Terashima, I., Sonoike, K., Kawazu, T., and Katoh, S. (1991b). Exposure of leaves of Cucumis sativus L. to low temperatures in the light causes uncoupling of thylakoids II. Non-destructive measurements with intact leaves. Plant Cell Physiol. 32, 1275–1283. doi: 10.1093/oxfordjournals.pcp.a078206
Tikkanen, M., Grieco, M., Kangasjärvi, S., and Aro, E. M. (2010). Thylakoid protein phosphorylation in higher plant chloroplasts optimizes electron transfer under fluctuating light. Plant Physiol. 152, 723–735. doi: 10.1104/pp.109.150250
Tikkanen, M., Grieco, M., Nurmi, M., Rantala, M., Suorsa, M., and Aro, E. M. (2012). Regulation of the photosynthetic apparatus under fluctuating growth light. Philos. Trans. R. Soc. Lond. B Biol. Sci. 367, 3486–3493. doi: 10.1098/rstb.2012.0067
Tikkanen, M., Mekala, N. R., and Aro, E. M. (2014). Photosystem II photoinhibition-repair cycle protects Photosystem I from irreversible damage. Biochim. Biophys. Acta Bioenerg. 1837, 210–215. doi: 10.1016/j.bbabio.2013.10.001
Tiwari, A., Mamedov, F., Grieco, M., Suorsa, M., Jajoo, A., Styring, S., et al. (2016). Photodamage of iron–sulphur clusters in photosystem I induces non-photochemical energy dissipation. Nat. Plants 2:16035. doi: 10.1038/nplants.2016.35
Tjus, S. E., Møller, B. L., and Scheller, H. V. (1999). Photoinhibition of photosystem I damages both reaction centre proteins PSI-A and PSI-B and acceptor-side located small photosystem I polypeptides. Photosynth. Res. 60, 75–86. doi: 10.1023/A:1006283618695
Wada, S., Suzuki, Y., Takagi, D., Miyake, C., and Makino, A. (2018). Effects of genetic manipulation of the activity of photorespiration on the redox state of photosystem I and its robustness against excess light stress under CO2-limited conditions in rice. Photosynth. Res. 137, 431–441. doi: 10.1007/s11120-018-0515-y
Walters, R. G. (2005). Towards an understanding of photosynthetic acclimation. J. Exp. Bot. 56, 435–447. doi: 10.1093/jxb/eri060
Walters, R. G., Shephard, F., Rogers, J. J., Rolfe, S. A., and Horton, P. (2003). Identification of mutants of Arabidopsis defective in acclimation of photosynthesis to the light environment. Plant Physiol. 131, 472–481. doi: 10.1104/pp.015479
Ware, M. A., Belgio, E., and Ruban, A. V. (2015). Photoprotective capacity of non-photochemical quenching in plants acclimated to different light intensities. Photosynth. Res. 126, 261–274. doi: 10.1007/s11120-015-0102-4
Wientjes, E., Oostergetel, G. T., Jansson, S., Boekema, E. J., and Croce, R. (2009). The role of Lhca complexes in the supramolecular organization of higher plant photosystem I. J. Biol. Chem. 284, 7803–7810. doi: 10.1074/jbc.M808395200
Yamatani, H., Kohzuma, K., Nakano, M., Takami, T., Kato, Y., Hayashi, Y., et al. (2018). Impairment of Lhca4, a subunit of LHCI, causes high accumulation of chlorophyll and the stay-green phenotype in rice. J. Exp. Bot. 69, 1027–1035. doi: 10.1093/jxb/erx468
Yamori, W., Hikosaka, K., and Way, D. A. (2014). Temperature response of photosynthesis in C3, C4, and CAM plants: temperature acclimation and temperature adaptation. Photosynth. Res. 119, 101–117. doi: 10.1007/s11120-013-9874-6
Zivcak, M., Brestic, M., Balatova, Z., Drevenakova, P., Olsovska, K., Kalaji, H. M., et al. (2013). Photosynthetic electron transport and specific photoprotective responses in wheat leaves under drought stress. Photosynth. Res. 117, 529–546. doi: 10.1007/s11120-013-9885-3
Keywords: photosystem I, photoinhibition, reactive oxygen species, light acclimation, wheat (Triticum aestivum L.)
Citation: Takagi D, Ihara H, Takumi S and Miyake C (2019) Growth Light Environment Changes the Sensitivity of Photosystem I Photoinhibition Depending on Common Wheat Cultivars. Front. Plant Sci. 10:686. doi: 10.3389/fpls.2019.00686
Edited by:
Stefano Santabarbara, Italian National Research Council (CNR), ItalyReviewed by:
Kintake Sonoike, Waseda University, JapanWojciech Jacek Nawrocki, VU University Amsterdam, Netherlands
Copyright © 2019 Takagi, Ihara, Takumi and Miyake. This is an open-access article distributed under the terms of the Creative Commons Attribution License (CC BY). The use, distribution or reproduction in other forums is permitted, provided the original author(s) and the copyright owner(s) are credited and that the original publication in this journal is cited, in accordance with accepted academic practice. No use, distribution or reproduction is permitted which does not comply with these terms.
*Correspondence: Chikahiro Miyake, Y21peWFrZUBoYXdrLmtvYmUtdS5hYy5qcA==