- Hawkesbury Institute for the Environment, Western Sydney University, Penrith, NSW, Australia
Elevated CO2 affects C cycling processes which in turn can influence the nitrogen (N) and phosphorus (P) concentrations of plant tissues. Given differences in how N and P are used by plants, we asked if their stoichiometry in leaves and wood was maintained or altered in a long-term elevated CO2 experiment in a mature Eucalyptus forest on a low P soil (EucFACE). We measured N and P concentrations in green leaves at different ages at the top of mature trees across 6 years including 5 years in elevated CO2. N and P concentrations in green and senesced leaves and wood were determined to evaluate both spatial and temporal variation of leaf N and P concentrations, including the N and P retranslocation in leaves and wood. Leaf P concentrations were 32% lower in old mature leaves compared to newly flushed leaves with no effect of elevated CO2 on leaf P. By contrast, elevated CO2 significantly decreased leaf N concentrations in newly flushed leaves but this effect disappeared as leaves matured. As such, newly flushed leaves had 9% lower N:P ratios in elevated CO2 and N:P ratios were not different in mature green leaves (CO2 by Age effect, P = 0.02). Over time, leaf N and P concentrations in the upper canopy slightly declined in both CO2 treatments compared to before the start of the experiment. P retranslocation in leaves was 50%, almost double that of N retranslocation (29%), indicating that this site was P-limited and that P retranslocation was an important mechanism in this ecosystem to retain P in plants. As P-limited trees tend to store relatively more N than P, we found an increased N:P ratio in sapwood in response to elevated CO2 (P < 0.01), implying N accumulation in live wood. The flexible stoichiometric ratios we observed can have important implications for how plants adjust to variable environmental conditions including climate change. Hence, variable nutrient stoichiometry should be accounted for in large-scale Earth Systems models invoking biogeochemical processes.
Introduction
Nitrogen (N) and phosphorus (P) are arguably the two most essential macronutrients to regulate plant growth and productivity in terrestrial ecosystems (Elser et al., 2007; Harpole et al., 2011) because of their key role in many cellular functions of plant metabolism. Nitrogen has been related to plant carbon assimilation via the many photosynthetic proteins (Field and Mooney, 1986; Evans, 1989; Reich et al., 2008), whilst P is a central component of energy metabolism (ATP and Pi) and takes part in the formation of the key macromolecules such as nucleic acids, phospholipids, ribosomes and sugar phosphates (Bieleski, 1973; Jacob and Lawlor, 1991; Rao and Terry, 1995). However, both of these essential nutrients are often in short supply relative to a plant’s growth requirements, especially in forest ecosystems on nutrient-poor soils (Vitousek and Farrington, 1997; Vitousek et al., 2010). Scarcity of N and P can affect the metabolic performance of plants (Sterner and Elser, 2002; Reich and Oleksyn, 2004; Ågren et al., 2012). Most native ecosystems exhibit strong N- or P-limitation, or a co-limitation of both as N and P tend to co-vary in nature (Sterner and Elser, 2002). However, N-limitation can occur more frequently than P-limitation in geologically young soils (LeBauer and Treseder, 2008); whereas P-limitation tends to be more prevalent in old, highly weathered soils (Walker and Syers, 1976; Vitousek et al., 2004; Turner et al., 2013). In green leaves, N and P concentrations are important not only because of their functional relationships to photosynthetic assimilation rates and growth (Aerts and Chapin, 2000; Wright et al., 2004; Ågren, 2008), but also because they may regulate carbon (C) and nutrient cycles and food webs in terrestrial ecosystems (Chapin et al., 2011).
Approximately one-third of the world’s soils are estimated to be P-limited based on the geographical extent of soil types (Yang and Post, 2011), and the high N:P ratios in leaves of plants growing on such soils (Reich and Oleksyn, 2004; Cleveland et al., 2011; Goll et al., 2012). The Australian continent is well-known for its geologically old landscapes with low P availability in soils (Beadle, 1966; Lambers et al., 2010; Kooyman et al., 2017), related to the parental materials that lack renewal from glaciation and tectonic action (Hopper, 2009; Augusto et al., 2017). Hence, many but not all landscapes on this continent may be particularly low in P (Kooyman et al., 2017).
Retranslocation of nutrients from leaves during senescence is a strategy to efficiently retain P (Escudero et al., 1992; Aerts, 1996; Killingbeck, 1996). This retranslocation by resorption serves to withdraw nutrients from leaves prior to abscission for later redeployment in developing tissues. While resorption can occur throughout the lifespan of the leaf (Ackerly and Bazzaz, 1995; Wright and Westoby, 2003), it is especially an intrinsic process of leaf senescence that occurs before leaf abscission. The nutrient resorption efficiency or retranslocation rate is the ratio determined by comparing the nutrient concentration in senesced leaves with the nutrient concentrations in fully expanded, mature green leaves (Killingbeck, 1996). The extent to which N and P are retranslocated and re-used depends on the plant nutrient status (Aerts and Chapin, 2000). Generally, with decreasing nutrient availability in an ecosystem, the amount of resorption of both N and P tend to increase (Reed et al., 2012; Vergutz et al., 2012) and this ratio could be an indicator of which nutrient is most limiting in a given ecosystem (de Campos et al., 2013).
Given that N and P availability control the global carbon-cycle response to environmental changes (van Groenigen et al., 2006; Matear et al., 2010; Wassen et al., 2013), it is important to investigate both elements and their stoichiometric balance. N and P concentrations in green leaves are typically correlated both within and across plant species and ecosystems (McGroddy et al., 2004; Hessen et al., 2007), leading to a narrow stoichiometric range for N:P (Elser et al., 2007). As low P availability in the soil tends to decrease leaf P concentrations (Cleveland et al., 2011; Hidaka and Kitayama, 2011; Marklein and Houlton, 2012), foliar N:P ratios increase. Moreover, N-use efficiency tends to decrease as plants had higher N:P ratios (Crous et al., 2017). However, plant species differ in their ability to maintain a constant N:P ratio under variable supply of both nutrients (Sterner and Elser, 2002; Güsewell, 2004) and it is unclear how flexible this stoichiometry can be, especially in response to climate change factors such as elevated atmospheric CO2. Several studies have reported a decrease in leaf N:P ratios in response to elevated CO2 (Dijkstra et al., 2012; Liu et al., 2013; Deng et al., 2015; Yue et al., 2017) although some species did not show such a change (Milla et al., 2006; Liu et al., 2013). The decrease in N:P in response to elevated CO2 has been explained by the observation that N concentrations may be more sensitive to elevated CO2 than P-concentrations, something that has primarily been observed in N-limiting ecosystems (Feng et al., 2015; Huang et al., 2015). However, the importance of P dynamics in elevated CO2 is less clear, and the assumption of a homeostatic N:P ratio in elevated CO2 due to similar proportional N and P responses needs to be tested (Ågren, 2008; Goll et al., 2012; Zhang et al., 2014). Given the potential for elevated CO2 to change stoichiometric ratios (Sardans et al., 2012; Deng et al., 2015; Yuan and Chen, 2015), it is critical to understand how elevated CO2 affects plant N:P ratios to evaluate nutrient limitations in terrestrial ecosystems in the future.
In this study, we hypothesized that (1) P-retranslocation would be higher than N-retranslocation in a mature woodland on the Sydney Cumberland Plain, given that there is evidence it is a P-limited ecosystem (Crous et al., 2015; Ellsworth et al., 2017) and (2) that elevated CO2 would reduce N:P ratios in leaves and/or wood due to the greater mobility of N in leaves and the large body of evidence showing strong N reductions under elevated CO2 (Feng et al., 2015). To test these, we determined nutrient concentrations and their retranslocation of Eucalyptus tereticornis leaves and wood from a mature woodland exposed to long-term elevated CO2 at Eucalyptus Free-Air CO2 Enrichment experiment (EucFACE). We investigated both spatial and temporal variations in leaf P and N concentrations in this ecosystem and tested whether long-term elevated CO2 affected P concentrations and N:P ratios in green leaves, senesced leaves and wood.
Materials and Methods
Site Description for EucFACE
The Eucalyptus Free-Air CO2 Enrichment (EucFACE) experiment is located in a remnant patch of native Cumberland Plain woodland on an ancient alluvial floodplain in western Sydney (Australia, 33° 37′S, 150° 44′E, 30 m a.s.l.) and has been unmanaged for over 70 years. The soil is a loamy sand (Podosol in the Australian Soil Classification system) with >75% sand content changing into sandy clay loam with >30% silt and clay between 0.5 – 2 m depth, transitioning to sandy clay beyond 2 m depth down to the water table at 13 m. The surface soil pH is slightly acidic (pHwater ∼5.2 ± 0.1, lower than reported in Crous et al., 2015). Total P concentration in the soil done by Aqua Regia assay was 76 mg kg-1 (0–10 cm soil depth, Drake et al., 2016), with available P much lower than this at <10 mg kg-1 (Bray extraction method used in Hasegawa et al., 2016). The soil is nutrient poor, and a strong limitation of P on tree growth at the site has been demonstrated (Crous et al., 2015). The open woodland (600–1000 trees ha-1) has a mean annual temperature of 17°C and mean annual precipitation of ∼800 mm (1881 – 2018, Bureau of Meteorology, station 067105 in Richmond, NSW Australia1). The vegetation is dominated by Eucalyptus tereticornis Sm. in the overstory with a few scattered individuals of E. amplifolia Naudin, while the understory is dominated by Microlaena stipoides (Labill.) R.Br. (a C3 grass) and some shrubs (see Pathare et al., 2017).
The Free-Air CO2 enrichment site consists of six 25 m diameter circular plots (hereafter referred to as ‘rings’) from which 32 vertical pipes are extended above the forest canopy, as described previously in Gimeno et al. (2016) and Duursma et al. (2016). Three rings were exposed to an elevated [CO2] treatment (ambient +150 μmol mol-1) while the other three rings were used as control plots representing the ambient [CO2] treatment (∼400 μmol mol-1), with identical infrastructure and instrumentation as the treatment plots. Pre-diluted CO2 is released into the treatment rings in a computer controlled system aiming for a CO2 mole fraction of 150 μmol mol-1 above ambient since February 2013.
Leaf and Wood Sampling
Green fully expanded mature leaves were collected from three (co-)dominant trees in each plot using multiple 36 m tall canopy-access cranes (Jaso crane J-4010, Jaso S.L., Idiazabal, Spain). Leaves were collected from the top of the tree crowns at 17–23 m height, depending on the height of dominant trees within the plots. Measurements of leaf N and leaf P on fully expanded mature green leaves started in May 2012 before CO2 fumigation at EucFACE was turned on. Additional leaves were sampled several times per year from 2013–2018, viz. Jan./Feb., April/May, and Sept./Oct., totaling 473 green leaf samples. This roughly corresponded to 1 or 2 subsample leaves per tree and three trees × 6 plots ×3–4 times of year ×5 ½ years. In a randomized design for the overall experiment, degrees of freedom were allocated for true replicates (plots) and subsamples within plots were averaged. Leaf life span in E. tereticornis was on average 13 months (Duursma et al., 2016) with newly flushed leaves here defined as less than 90 days old and mature leaves defined as more than 90 days old, consistent with Wujeska-Klause et al. (2019) and based on phenological observations of timing of leaf flushing (Ellsworth, unpublished). For most of the year, leaves were mature and older than 90 days, but during summer when canopy turnover occurred, two leaf age classes were collected (when available) including newly flushed fully expanded leaves and old mature leaves. Upon collection, leaves were placed on ice and measured for leaf area, after which they were freeze-dried for at least 72 h to determine leaf mass per area (LMA, g m-2), and then prepared for chemical analyses when ground to a fine powder. All green leaves used to compute retranslocation were live, mature green leaves collected from the upper canopy toward the end of their lifespan (∼360–410 days old).
Leaf litter was collected monthly in ∼0.2 m2 circular fine-mesh traps at eight random locations per ring (Duursma et al., 2016). Litter was sorted into leaf, twigs, bark, and other material, then oven-dried at 40°C and weighed. Given that canopy turnover occurred in summer, a subsample of senesced leaves was analyzed from 2–3 traps per ring for total N and total P concentrations for the summer months of 2013–2018, totaling 105 samples. Some senesced leaves were also collected from individual trees during canopy turnover in the summers of January 2014 and February 2015, in which case the values were averaged per ring with the litter trap nutrient values. Senesced leaf nutrient concentrations were also used to compute retranslocation, calculated as the fraction of nutrients removed from its senescing leaves compared with how much is in its adult leaves before leaf abscission:
Wood was sampled in November 2015 at the base of the stem (about breast height) in three (co-) dominant trees per ring. Sapwood was defined as the outer 20 mm of wood beneath the bark, and was sampled for a 4 cm × 2 cm patch using a chisel. Gimeno et al. (2018) determined that the outer 20 mm comprised most of the sapwood in dominant and co-dominant trees in the stand. Heartwood was sampled at one timepoint outside one ring in the middle of the EucFACE site. Heartwood was judged on the basis of wood color and density which distinguishes it from sapwood in this species. Heartwood could not be collected from within the experimental rings due to the long-term nature of the experiment.
Elemental Analyses of Leaf Nitrogen and Phosphorus
Mass-based leaf N (Nm) and C concentrations were analyzed with the Dumas combustion technique using elemental analyzers (TruSpec micro, LECO Corp., St. Joseph, MI, United States; and FLASH EA 1122 Series CHN analyzer, Thermo-Finnigan, Waltham, MA, United States). Total P concentrations of leaves were determined via a standard Kjeldahl digestion procedure using 3 mL of sulphuric acid (pure H2SO4) and 2 mL of hydrogen peroxide (H2O2, 30%). The amounts of dried material digested varied depending on how much total P was expected in the sample. For example, we used 55 mg for green leaves, 250 mg for senesced leaves and 450 mg for sapwood. The total P concentrations of the Kjeldahl digests were colorimetrically analyzed at 880 nm after a molybdate reaction in a discrete analyzer (AQ2, SEAL Analytical, Ltd., Milwaukee, WI, United States and EPA135 method). Elemental analyses of other macronutrients are reported in Crous et al. (2015). Sapwood was analyzed by inductively coupled plasma optical emission spectroscopy (ICP-OES, Perkin-Elmer, Waltham, MA, United States) for total P and via combustion for total N (FLASH EA 1122 Series CHN analyzer, Thermo-Finnigan, Waltham, MA, United States) for 2–3 trees per ring. Heartwood was also analyzed for total P concentration with the same method and used to calculate P retranslocation in wood across CO2 treatments. For all chemical analyses we ran internal standards, using NIST Standard Reference Material 1515 (United States National Institute of Standards and Technology, Bethesda, MD, United States) for quality control purposes. Area-based N (Na; g m-2) and P (Pa; g m-2) content were calculated based on Pm and Nm concentrations and multiplied by the leaf per mass area (LMA; g m-2).
Statistical Analyses
All statistical analyses were performed in R (R Core Team, 2018, v. 3.5.1). Variables were tested for normality to conform with linearity assumptions before further statistical analyses. Transformations were applied, where necessary to achieve a normal distribution: Pm and C:N were both log-transformed in green leaves. Over this 6-year dataset, we only used campaigns, where both N and P concentrations had been measured, and as such this dataset is slightly different from the dataset used in Wujeska-Klause et al. (2019). Most findings regarding N are consistent with this previous paper, and our analyses here additionally focus on P, including evaluating annual variation.
We conducted a set of mixed model analyses on green leaves with CO2, Year and Age as fixed factors and tree nested within ring as a random factor using the ‘lmer’ function from lme4 package (Bates et al., 2015). We also analyzed leaf P concentrations using Year as a continuous variable and obtained similar results. Differences between means were tested using Tukey HSD post hoc test [‘glht’ from multcomp package, Hothorn et al. (2008)]. All analyses used tree-specific data for each measurement campaign. Means and standard error bars of CO2 treatment effects were computed based on ring averages to reflect the correct number of replicates (n = 3). Mixed model analyses of senesced leaves included CO2 and Year as fixed factors with Ring as a random factor, sampled each year in February. February was chosen because most leaf litter falls in the summer months as the canopy is turning over. Given the destructive nature, we sampled sapwood from trees at the site at a single time. Thus, we performed 2-sample t-tests to evaluate the CO2 effect on sapwood C, N and P concentrations along with their ratios. Data are stored on Western Sydney’s data archive (https://hiev.uws.edu.au/) and are publicly available at https://doi.org/10.6084/m9.figshare.7898048.v1.
Results
Effects of Leaf Age and Its Interaction With Elevated CO2
There was no elevated CO2 effect on mass-based leaf P (Pm) concentrations. There was a strong decline in green leaf Pm as leaves aged (P < 0.0001, Tables 1, 2), with Pm in old green leaves being 32% lower compared to newly flushed leaves across years (<90 days old) (Table 2). Over the total leaf lifespan, there was a fairly steady decline in mean Pm concentrations (i.e., on a continuous leaf age basis expressed as days since flushing across years) from recently flushed leaves until leaves over 400 days old, shortly before abscission (Figure 1). Across years, related to the Age effect on leaf P, both N:P and C:P ratios also exhibited significant leaf age effects. N:P ratios increased by 42% in older leaves compared to newly flushed leaves (P < 0.0001), where C:P ratio was 45% higher in older leaves than new leaves (P < 0.0001, Table 2).
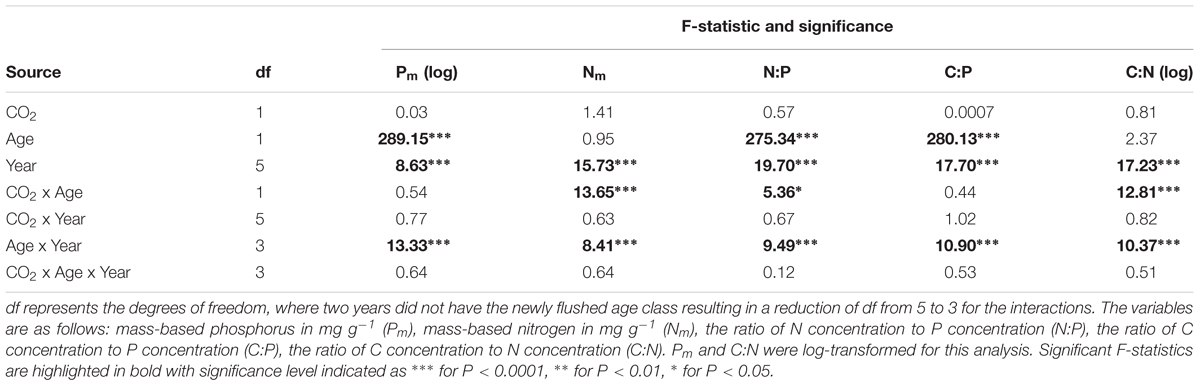
Table 1. Mixed model Anova results with F-statistic of green upper canopy leaves over 5 years with CO2, Age and Year as fixed factors and tree nested within ring as random factors.
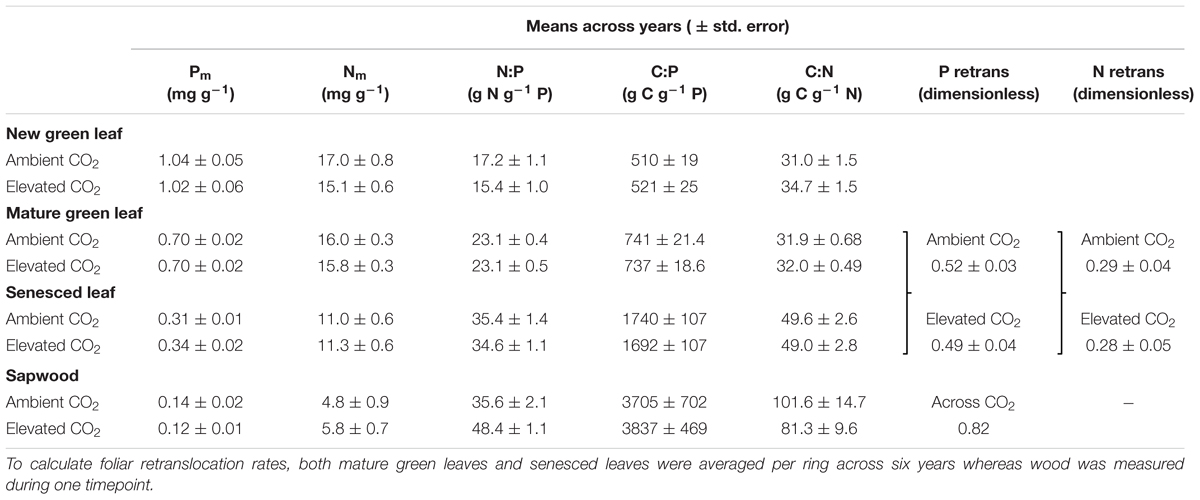
Table 2. Means ± std. errors across years for mass-based phosphorus (Pm), mass-based nitrogen (Nm), the ratio between N and P (N:P), the ratio between C and P (C:P), the ratio between C and N (C:N) and the retranslocation fraction for P (P retrans) and N (N retrans) in ambient and elevated CO2 in newly flushed and mature green leaves (>90 days since flushing), senesced leaves and sapwood.
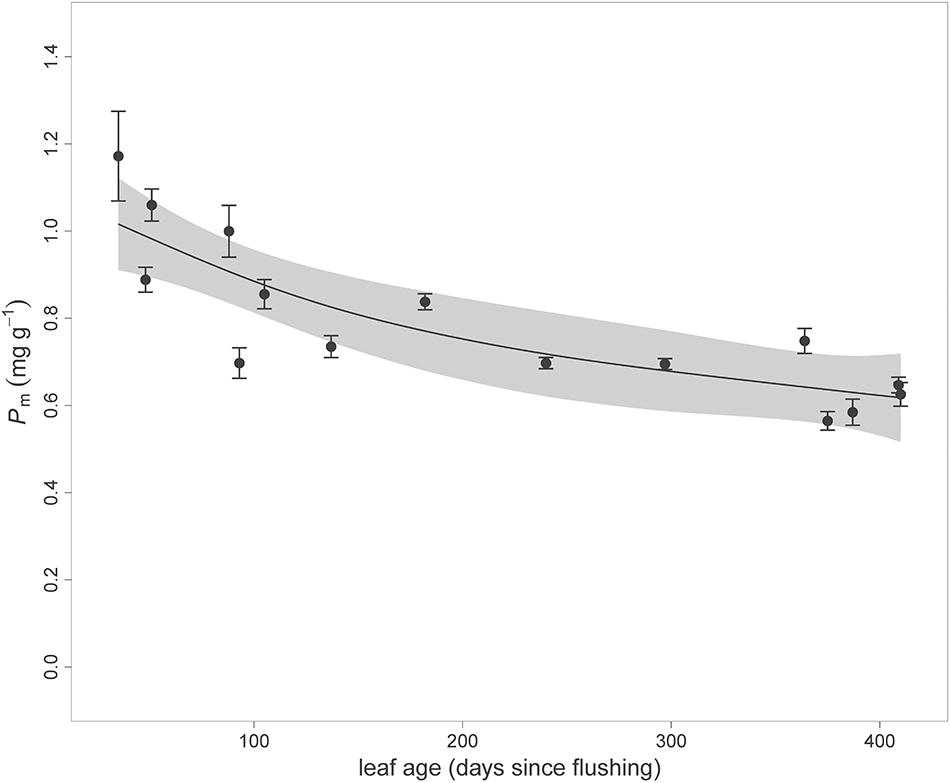
Figure 1. Means and standard errors of mass-based leaf phosphorus concentrations (Pm) since leaf flushing for each campaign across years, showing a decline in P concentrations over the lifetime of leaves (expressed as days since flushing where leaves are ‘new’ when <90 days). The line shows the trend fit using a generalized additive model (GAM), and the gray area shows the 95% confidence interval around the mean decline in Pm. Mass-based N as a function of leaf age can be found in Wujeska-Klause et al. (2019).
Leaf Nm did not differ between CO2 treatments or across leaf age classes, but there was a significant CO2 by Age effect in which newly flushed leaves reduced leaf N by 11% in elevated CO2; whereas old leaves had similar leaf N concentrations in both CO2 treatments, consistent with Wujeska-Klause et al. (2019). Related to this CO2 by Age interaction in leaf N, both N:P and C:N ratios also exhibited CO2 by Age interactions. The ratio N:P declined by 9% (P = 0.02), while C:N increased by 12% (P = 0.0004) in newly flushed leaves in elevated CO2 whereas older leaves did not show a CO2 treatment difference for either variable (Tables 1, 2).
Area-based P (Pa) and N (Na) did not differ between CO2 treatments, although Na showed a marginal CO2 by Age effect (P = 0.07, Supplementary Table S1) where new leaves were 5% lower in elevated CO2 compared to ambient CO2. This pattern is in the same direction as Nm concentrations and suggests that there was no dilution effect due to elevated CO2, as reported in Wujeska-Klause et al. (2019). Both Pa and Na exhibited significant differences between leaf age classes where Na was 23% lower in new leaves (P < 0.0001) due to a 23% lower LMA when compared with old leaves (P < 0.0001), while Pa was about 12% higher in new compared to old leaves (P = 0.0001). Leaf mass per area ratio (LMA) was not significantly different between CO2 treatments but was trending toward a 5% higher LMA in elevated CO2 (P = 0.08, Supplementary Table S1).
Year-Effects on P and N in Green Leaves
All variables showed highly significant differences among years (P < 0.0001) and significant interactions of Year ×Age (Table 1). These Year by Age interactions generally showed that newly flushed leaves exhibited more variable responses across years, likely due to the time of measurement after they flushed, which differed between 35 and 88 days. By contrast, older mature leaves were more steady in their response over the years, regardless of sampling time. Therefore, we focused on mature leaves only to evaluate the year effects (Figure 2).
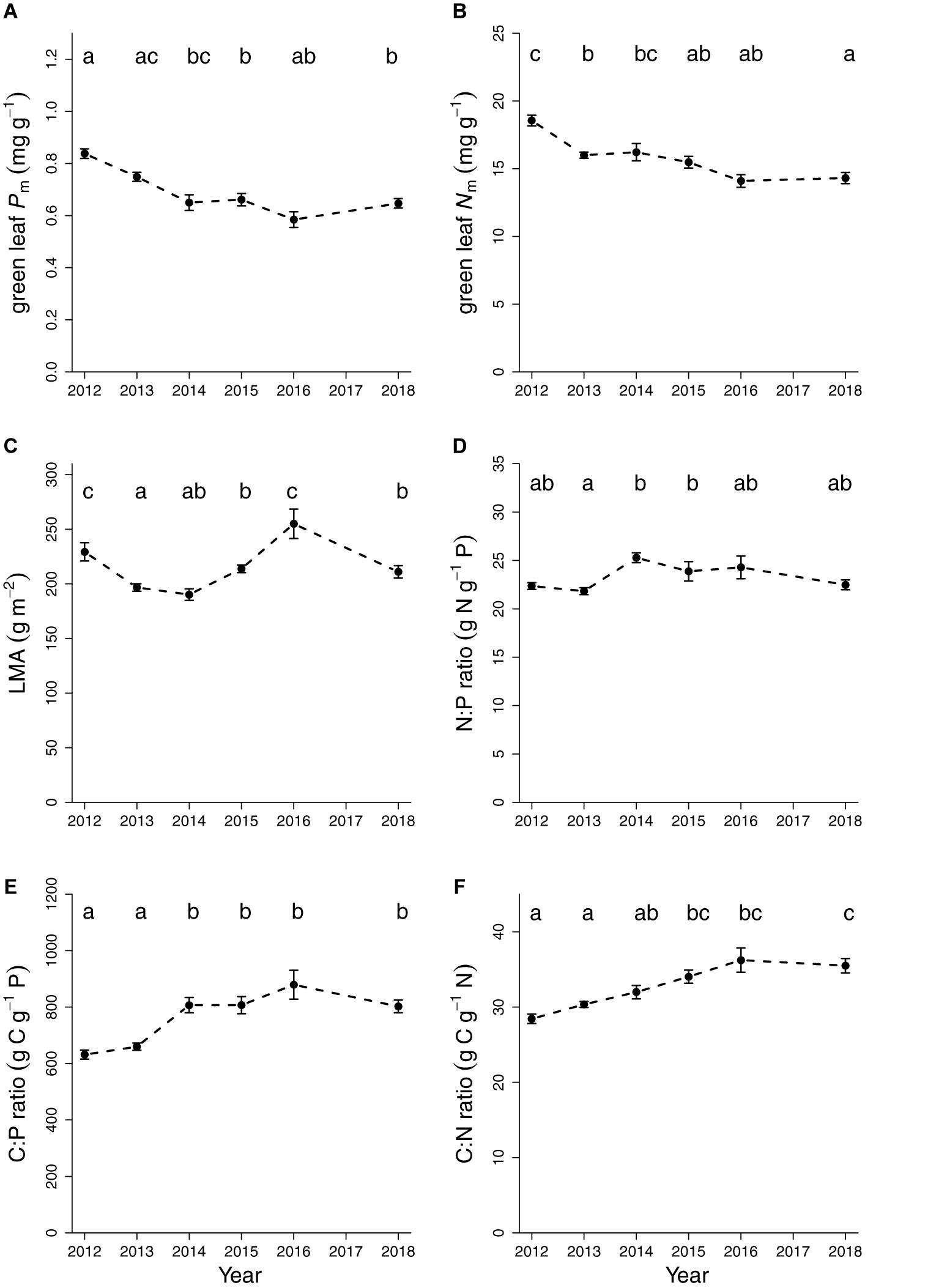
Figure 2. Annual variation in chemistry and structure for mature green leaves averaged across measurement campaigns for (A) mass-based phosphorus in mg g-1, (B) mass-based nitrogen in mg g-1, (C) LMA in g m-2, (D) N:P ratio, (E) C:P ratio and (F) C:N ratio. Data are means and standard errors. There were no mature green leaves in February 2017 due to early flushing.
Post hoc Tukey tests indicated that Pm concentrations in mature leaves in the first 2 years (2012 and 2013) were higher compared to the latter years. As there was no effect of elevated CO2, this decline was similar in both CO2 treatments. Hence, across CO2 treatments Pm concentrations in green mature leaves declined from 0.79 ± 0.03 mg g-1 (2012 and 2013 together) to 0.64 ± 0.02 mg g-1 in later years (2014, 2015, 2016, 2018) (Figure 2A). This pattern across years was similar regardless of sampling time (Supplementary Figure S1).
Leaf Nm concentrations also declined across years but the trend was less clear compared to leaf Pm. While post hoc tests indicated significant differences between 2012, 2013 and 2018, there were no differences between 2013 and 2016 for Nm in mature green leaves. Across CO2 treatments, leaf Nm declined from 18.5 ± 0.4 mg g-1 in 2012 to 15.4 ± 0.4 mg g-1 in 2013–2016, and then to 14.3 ± 0.4 mg g-1 in 2018 (Figure 2B).
Given the differences between years in N and P concentrations of mature green leaves, the N:P, C:P and C:N ratios were also different across years (P < 0.0001) and no CO2 effects were observed (P > 0.1, Table 1). While N:P was highest in 2014 and 2015 (24.6 ± 0.8) and different from the N:P in 2013 (21.8 ± 0.4), differences in other years were subtle and non-significant. Hence, the overall N:P ratio across years was 23.1 ± 0.5 in mature leaves (Figure 2C and Table 2). The C:P ratio was higher in later years compared to earlier years (2012, 2013), being 824 ± 32 versus 645 ± 14, respectively, likely reflecting the reduced leaf P concentrations in the later years (Figure 2D). Across CO2 treatments, C:N increased over the years reflecting declining leaf N concentrations with very clear trends contrasting earlier (2012–2013: 29.4 ± 0.5) to later years (2015–2018: 35.3 ± 1.1).
On an area-basis, Na and Pa declined in similar fashion as their mass-based variables. Both Pa and Na had higher concentrations in 2012 compared to all other years. Pa also showed a CO2 by year interaction (P = 0.05, Supplementary Table S1) because in some years Pa was higher in elevated CO2 while in other years it was lower compared to ambient CO2 within a given year. These small variations did not result in any overall CO2 effect on Pa. LMA varied over the years averaging at 206 ± 3 g m-2 in old leaves with the lowest value in 2014 (175 ± 6 g m-2) and the highest in 2016 (240 ± 3 g m-2) (Figure 2C). These values are consistent with Wujeska-Klause et al. (2019).
P and N Concentrations in Senesced Leaves and Their Retranslocation Rate
Across years and CO2 treatments, the concentrations of P and N in senesced leaves were, respectively, 0.32 ± 0.04 mg g-1 and 11.1 ± 1.5 mg g-1 on average. Mixed model analyses indicated no effects of elevated CO2 on P or N concentrations in senesced leaves, but as with green leaves, there were significant year effects in which leaf P and N concentrations varied between years (Figure 3 and Table 3). Both N and P concentrations in senesced leaves were lower in 2014, 2015, 2016 and 2018 compared to 2013 and 2017 (P < 0.05, Tukey test). Senesced leaf C was on average 51%. All ratios also varied across years but without a clear directional trend. Although senesced leaf N:P ratio has a weak year effect (P = 0.046), post hoc comparison did not indicate significant differences. Across years, the N:P ratio was 35.0 ± 2.9 (Figure 3 and Table 2). Similarly for C:P in senesced leaves, there were no significant post hoc comparisons with the C:P ratio being 1716 ± 259 across years. C:N mirrored the pattern in senesced leaf N with 2013 and 2017 being similar whereas the ratio increased in the middle years.
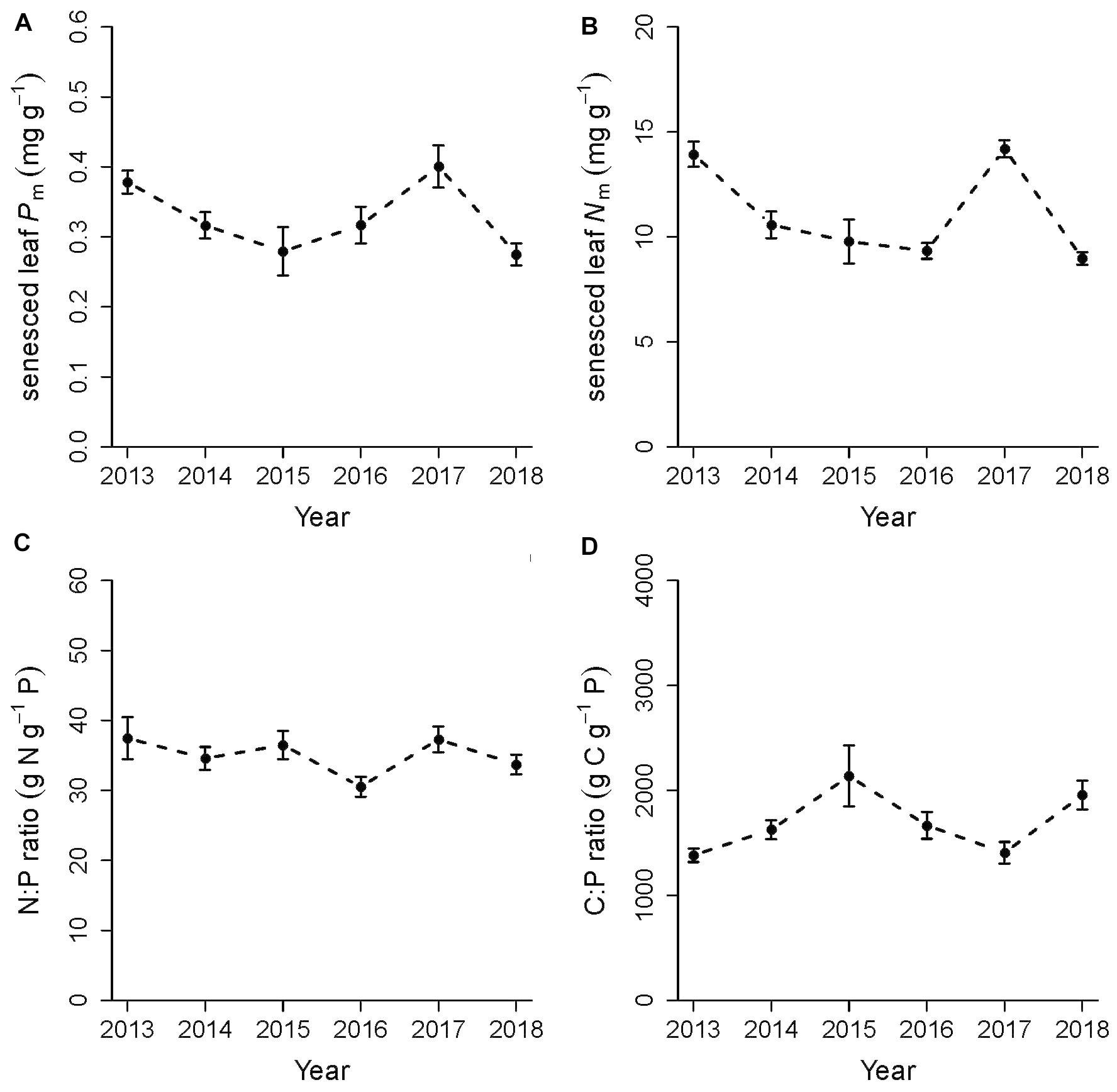
Figure 3. Annual variation in chemical composition of senesced leaves collected from litter traps in February of each year (2013–2018) for (A) mass-based phosphorus in mg g-1, (B) mass-based nitrogen in mg g-1, (C) N:P ratio and (D) C:P ratio. Data are means and standard errors.
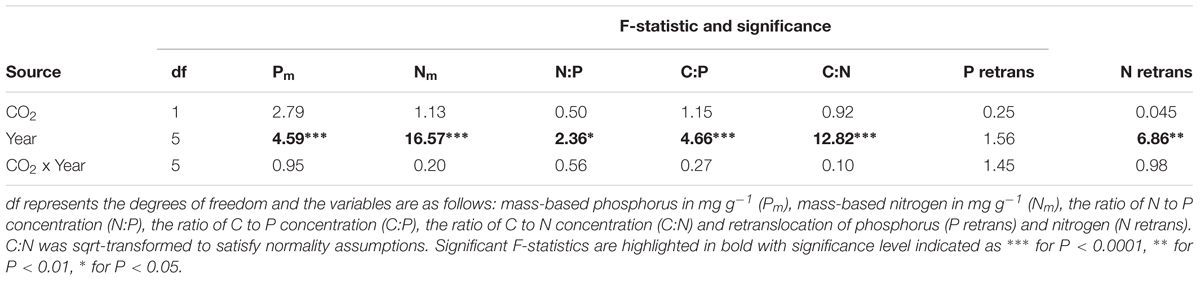
Table 3. Mixed model Anova table with F-statistic of senesced leaves over five years with CO2 and Year as fixed factors and ring as random factor.
The retranslocation rate or resorption efficiency was calculated using N and P concentrations in the oldest green leaves and comparing these with the respective concentrations in senesced leaves (leaf age > 360 days, last 4 ticks in Figure 1). Both green leaves and senesced leaves were collected in the January-February period of each year (except for January 2017 where no old leaves were available). On average, leaves re-translocated 50.1 ± 2.4% P and 28.7 ± 3.0% N across years (means ± s.e. across years; Table 2). While there was no year effect on P retranslocation, N retranslocation was different across years because N retranslocation in 2013 was much lower compared to the other years. Overall, there was a strong positive relationship (R2 = 0.66) between N and P retranslocation across the years at the site with no differences regarding CO2 treatment (Figure 4).
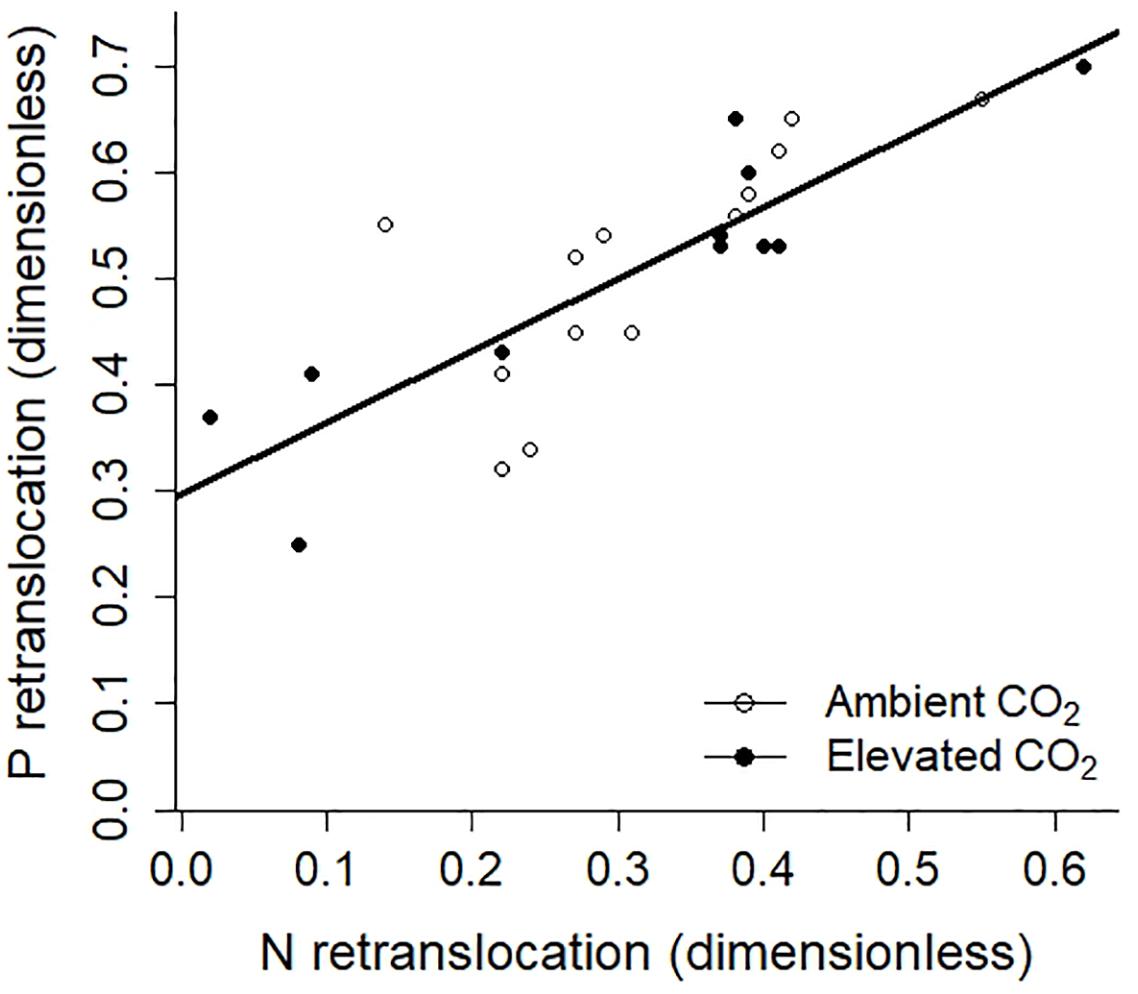
Figure 4. Linear relationship between P retranslocation and N retranslocation across 5 years in ambient (open symbols) and elevated (closed symbols) CO2 treatments, expressed as a fraction (dimensionless). The relationship was described as Y = 0.68 ∗ N retranslocation fraction + 0.30, R2 = 0.66 and P < 0.0001.
Leaf P, N and C Concentrations in Wood
Concentrations of P and N in sapwood were 50–60% lower than concentrations in senesced leaves. Neither wood N nor P concentrations were statistically different between CO2 treatments, with averages of 5.3 ± 0.8 mg g-1 (N) and 0.13 ± 0.01 mg g-1 (P) (Table 2). In sapwood, the C concentration was 45%. The total P concentration in heartwood was 0.023 ± 0.005 mg g-1, so assuming heartwood was representative across treatments this resulted in a 82% P retranslocation rate from sapwood (Table 2). Whereas C:N and C:P in sapwood were not significantly different between CO2 treatments (P > 0.3), there was a significant increase in sapwood N:P ratios in elevated CO2 compared to ambient CO2 (P = 0.006). Here, sapwood N:P ratios increased to 48 ± 1 in elevated CO2 compared to 36 ± 2 in ambient CO2 (Figure 5 and Table 2). Although both wood N and P concentrations were not significantly different between CO2 treatments (P > 0.2), the CO2 effect on N:P appears to be underpinned by a 20% increase in mean N concentrations in sapwood in elevated CO2, and a smaller decrease in wood P concentration in elevated CO2 (Figure 5). These together led to the observed CO2 effect on wood N:P ratio.
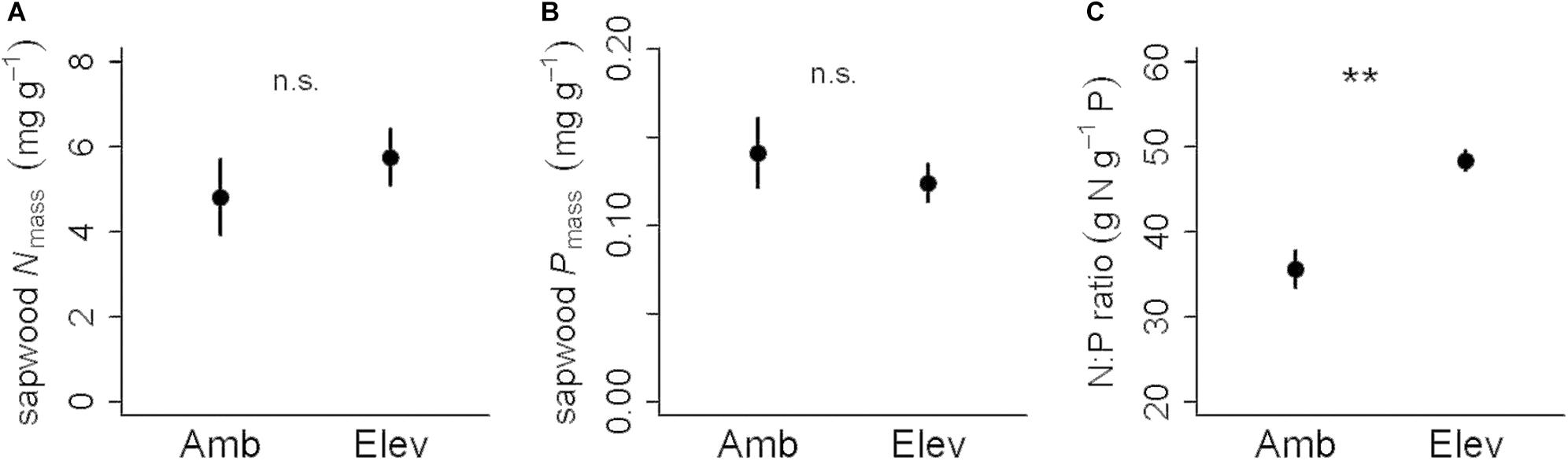
Figure 5. Means and standard errors for chemical composition of sapwood in ambient (Amb) and elevated (Elev) CO2 treatments for (A) mass-based nitrogen concentration in mg g-1, (B) mass-based P concentration in mg g-1 and (C) N:P ratio. While N and P concentrations did not differ between CO2 treatments (n.s. in panels A and B), the N:P ratio in wood was significantly increased in elevated CO2 compared to ambient CO2 at P < 0.01 (∗∗ in panel C).
Discussion
In this mature Eucalyptus forest, long-term elevated CO2 exposure did not significantly change N or P concentrations in mature green leaves (P > 0.10, Table 1), though such changes have been observed by others (Sardans et al., 2012) and were initially hypothesized. In contrast to our study, several studies reported decreased N:P ratio in elevated CO2, in part as a consequence of observed lower leaf N in elevated CO2 relative to leaf P (Sardans et al., 2012; Liu et al., 2013; Deng et al., 2015; Huang et al., 2015). However, severely nutrient-limited bog sites also did not show a CO2 effect on foliar N and P concentrations (Milla et al., 2006). When N is limiting in an ecosystem, plants can reduce leaf N in elevated CO2 as has been frequently observed (Ainsworth and Rogers, 2007; Liu et al., 2013; Deng et al., 2015). We observed a transient elevated CO2 effect on leaf N in newly flushed leaves but not in older, mature leaves. This is shown by the significant interaction between elevated CO2 and Age for Nm, Na, N:P and C:N ratios in our study (Table 1), but not for Pm. Hence, elevated CO2 reduced N:P in newly flushed leaves but N:P was similar in both CO2 treatments in mature green leaves because leaf N concentrations declined more strongly with age x CO2 (Wujeska-Klause et al., 2019) compared to leaf P concentrations across the lifetime of leaves in this evergreen eucalypt forest (Figure 1). Wujeska-Klause et al. (2019) reported non-significant differences in carbohydrates between CO2 treatments and no strong evidence of structural dilution in elevated CO2. Therefore, these processes unlikely influenced Nm and Pm. Hence, any elevated CO2 effects detected for leaf N in newly flushed leaves diminished as leaves matured beyond three months since flushing, noting that the Eucalyptus leaf longevity at our site was about a year (Duursma et al., 2016).
The EucFACE mature woodland is P-limited, indicated by high N:P ratio both for green and senesced leaves (Table 2). These N:P ratios suggest a clear P-limitation at the site (Koerselman and Meuleman, 1996), consistent with interpretations from Crous et al. (2015). Moreover, P- retranslocation was about 50%, almost twice as high as N-retranslocation (28%), so greater retranslocation of a more scarce nutrient is further evidence of P-limitation (Wright and Westoby, 2003). There was proportionally more P in green leaves being removed prior to senescence than N, shown by the higher N:P ratio (N:P of 35) in senesced leaves compared to green leaves. The higher resorption efficiency of P than N represents a key process that can generate differences between foliar N and P concentrations as well as different N:P ratios in leaves and wood (Sardans and Peñuelas, 2013).
The resorption proficiency, defined as the level of nutrient concentrations in senesced leaves (Wright and Westoby, 2003), was 0.32 ± 0.04 mg g-1 in our study. Killingbeck (1996) reported a biochemically complete resorption of P when the P concentration of senesced leaves in evergreen species is less than 0.4 mg g-1. Therefore, P resorption in E. tereticornis leaves can be considered ‘complete,’ suggesting that leaves did not only resorb all soluble P but also hydrolyzed P in lipids and nucleic acids before senescence (Hidaka and Kitayama, 2011). Hydrolyzing enzymes as phospholipase and ribonucleases have a certain cost in terms of N requirements in synthesis (Fischer, 2007). However, when P availability is low, the balance between N cost for these enzymes and the benefit of P gain by resorption (Hidaka and Kitayama, 2011) is apparently in favor of P resorption in this ecosystem.
When a particular nutrient is limiting plant growth, there are several mechanisms to mitigate this nutrient limitation, two of which are increased uptake or increased nutrient-use efficiency. In N-limited pine plantation in Duke Forest, increased N demand from faster growth in elevated CO2 was largely accommodated via increased N uptake from soils (Phillips et al., 2012), while N retranslocation only played a minor role in this pine ecosystem (Finzi et al., 2002). In contrast, a small increase in P mineralization was observed in the elevated CO2 soil after the first 3 months of CO2 exposure (in 2012) in this Eucalyptus forest ecosystem after which no significant CO2 treatment effects were found on P mineralization rates (Hasegawa et al., 2016). There is no evidence that this transient effect influenced canopy P concentrations (Figure 2), arguing against increased P uptake in the trees in elevated CO2. A more definitive analysis would require assembling a full P budget for this ecosystem. However, high retention of foliar P across CO2 treatments was evident, given the almost doubled retranslocation rate of P compared to N. Hence, we conclude that this ecosystem has a high P retention rate, which can contribute to maintain canopy P-stocks over the long term. We suggest that P retranslocation played an important role in this woodland to retain P and enhance its residence time in plants.
Change in Leaf N and P Concentrations Over Years
Both N and P concentrations varied over the years in this study, for annual values of Nm and Pm there was some decline in the first 2 years of the experiment (2012–2013), leveling in the last 2–3 years (Figures 1A,B,E,F). This trend was similar or stronger when a consistent leaf age class was selected (i.e., it was not a sampling effect). Some early transients in the N cycle due to elevated CO2 have been identified in past studies (Luo et al., 2004), but as indicated in Table 1, the temporal decline we observed in this forest was unrelated to elevated CO2, as there was a similar trend in ambient and elevated CO2 (Table 1 and Figure 2). Moreover, the elevated leaf N and P concentrations actually preceded the start of the CO2 experiment in September 2012. Area-based nutrients (Na and Pa) also showed the same pattern of cross-year decline, though to a lesser extent (Supplementary Table S1). It is unclear what was responsible for this trend in leaf N and P concentrations given that the trees were mature and the soil at the site remained undisturbed for several decades prior to this study, even during construction of the experimental facility. A possible factor involved in this time trend could have been rainfall, as the elevated CO2 experiment was established and begun during a La Niña period, which typically brings rainy conditions to eastern Australia (Chiew et al., 1998). While speculative, higher N and P availability or uptake from the soil may have arisen from more readily available nutrients in the wetter soils during the La Niña period compared to less mobility or reduced uptake during drier periods. This possibility is suggested by the correlations found between leaf Pm and Nm concentrations and the preceding 6-months rainfall (Supplementary Figure S2). If this speculation is supported by further work, it would indicate that the stand experienced transient higher-nutrient conditions with a rainy period in 2011–2012 but subsequently stabilized leaf N and P concentrations. However, this is still an area for active research.
Regardless of origin, the temporal trend toward a reduction in green leaf N and P concentrations in this mature Eucalyptus stand is notable for two reasons. First, it establishes a backdrop to the start of the elevated CO2 experiment. Second, it demonstrates that E. tereticornis shows flexible N:P rather than maintaining a more conservative stoichiometric homeostasis (Sardans and Peñuelas, 2012). Maintaining stoichiometric homeostasis in environments in the face of variable nutrient supply is energetically demanding for plants (Sterner and Elser, 2002; Dijkstra et al., 2012). However, N:P and C:P ratios in native Australian woodlands seem to have a greater range of values than the C:N ratio, due to smaller variation in N concentrations than P concentrations (see Judd et al., 1996). For instance, Eucalyptus incrassata showed a 2-fold variation in N:P across sites with barely any change in C:N (Specht and Rundel, 1990). While the overall response of C:N:P stoichiometry to elevated CO2 can significantly vary depending on ecosystem type, climate factors and experimental conditions (Sterner and George, 2000; Castle and Neff, 2009; Yue et al., 2017), the yearly variation in N:P ratios was relatively small in our study. This variation allowed plants to adjust to changing annual conditions while still maintaining their stoichiometry within a fairly narrow range (Elser et al., 2005; Feller et al., 2007; Fitter and Hillebrand, 2009). How flexible leaf and whole-plant stoichiometry are in elevated CO2 relative to ambient CO2 has important implications for how process-based biogeochemical models represent nutrient limitations that may be manifest in elevated CO2 conditions (Zaehle et al., 2014). Thus, the information from this Eucalyptus stand establishes a range of flexible foliar N:P values that may be indicative of stoichiometric flexibility in P-limited stands.
Wood N:P Stoichiometry in Mature Trees
In spite of the lack of CO2 treatment-induced changes in leaf stoichiometry over the 5 years, the N:P ratio in sapwood was 36% higher in elevated CO2 compared to ambient CO2. This was surprising given the advanced age of the mature trees at the site, which were more than 100 years old according to available records. This finding has important implications because wood represents a massive C pool in forest ecosystems, and flexibility in the elemental composition of sapwood can mean greater biomass in elevated CO2 without requiring more nutrients. This was not the case in the present study as there was neither a change in biomass accumulation (from Ellsworth et al., 2017) nor a change in sapwood C:P ratio with elevated CO2 (Figure 5B).
While this dataset was limited given the destructive nature of the sampling and the need to maintain the long-term integrity of the trees, a higher N:P ratio in wood may indicate an increase in the N supply toward wood relative to P, suggesting storage or accumulation of structural proteins localized in cell walls, or proteins associated with metabolically active parenchyma cells, or both. While not significant, the average wood N concentrations were 20% higher in elevated CO2 compared to ambient CO2 (Figure 5A) suggesting that the increased N:P ratio of wood in elevated CO2 is mainly driven by higher wood N concentrations. Although plants growing in P-limited conditions tend to store a considerable amount of N (Ågren, 2004), the underpinning mechanisms may need to be further investigated. With woody biomass constituting a small P pool (compared to leaves), but a very large carbon pool owing to high C:P ratios (nearly 4000), there may be important implications of stoichiometric flexibility within the sapwood. This magnitude of variable stoichiometry should be considered in large-scale Earth Systems models involving biogeochemistry, as few models currently allow this sort of flexibility (Zaehle et al., 2014).
Conclusion
This study provided strong evidence of P-limitation in a mature E. tereticornis woodland via high foliar N:P ratios and a higher retranslocation of P compared to N on average, supporting our first hypothesis. This is also consistent with our previous findings at the site where P was added to mature trees and enhanced growth relative to unamended trees (Crous et al., 2015). We expected lower leaf N and P concentrations in elevated CO2, but for mature leaves there was no significant CO2 effect, suggesting these pools were unaltered in the Eucalyptus canopy in elevated CO2. By contrast, sapwood N:P ratio in elevated CO2 increased, suggesting increased N storage in wood, possibly releasing extra CO2 as a result of having greater respiratory proteins. Nutrient concentrations and their ratios varied dynamically from year to year depending on varying environmental conditions, with a subtle decline in leaf N and P in both ambient and elevated CO2 treatments from the start of the experiment to its fifth year. Thus, the yearly dynamics of nutrient concentration in leaves allowed plants to vary their stoichiometric ratio of N:P to a certain degree. The time-related change in leaf N:P and the CO2-treatment induced change in sapwood N:P together suggest that variable nutrient stoichiometry should be accounted for in large-scale Earth Systems models involving biogeochemistry.
Author Contributions
KC and DE designed the study. KC, DE, AW-K and BM collected the samples with KC performing the P digests and all P analyses as well as N analyses in the first 3 years. AW-K handled the N analyses in the last 3 years. KC wrote the first draft with input from DE, and led the analyses with significant contributions from MJ and DE. All authors commented on this manuscript.
Funding
This research was supported by the Australian Research Council, Discovery grants DP110105102 and DP160102452 to DE and DE160101484 to KC.
Conflict of Interest Statement
The authors declare that the research was conducted in the absence of any commercial or financial relationships that could be construed as a potential conflict of interest.
Acknowledgments
EucFACE was built as part of the Nation-building Economic Stimulus Package as an initiative of the Australian government. We are grateful to Dr. Vinod Kumar and Mr. Craig McNamara for technical support and expert crane operation during the study, and Drs. Craig Barton and Sebastian Pfautsch for wood sampling. Dr. Paul Milham is acknowledged for expert assistance regarding P chemistry during the development of the elemental analyses method for total P concentrations.
Supplementary Material
The Supplementary Material for this article can be found online at: https://www.frontiersin.org/articles/10.3389/fpls.2019.00664/full#supplementary-material
Footnotes
References
Ackerly, D. D., and Bazzaz, F. A. (1995). Leaf dynamics, self-shading and carbon gain in seedlings of a tropical pioneer tree. Oecologia 101, 289–298. doi: 10.1007/BF00328814
Aerts, R. (1996). Nutrient resorption from senescing leaves of perennials: are there general patterns? J. Ecol. 84, 597–608.
Aerts, R., and Chapin, F. S. (2000). The Mineral Nutrition of Wild Plants Revisited: A Re-evaluation of Processes and Patterns, Vol. 30. San Diego, CA: Academic Press Inc, 1–67.
Ågren, G. I. (2004). The C : N : P stoichiometry of autotrophs - theory and observations. Ecol. Lett. 7, 185–191. doi: 10.1111/j.1461-0248.2004.00567.x
Ågren, G. I. (2008). Stoichiometry and nutrition of pllant growth in natural communities. Annu. Rev. Ecol. Evol. Syst. 39, 153–170. doi: 10.1111/1365-2656.12613
Ågren, G. I., Wetterstedt, J. A. M., and Billberger, M. F. K. (2012). Nutrient limitation on terrestrial plant growth - modeling the interaction between nitrogen and phosphorus. New Phytol. 194, 953–960. doi: 10.1111/j.1469-8137.2012.04116.x
Ainsworth, E. A., and Rogers, A. (2007). The response of photosynthesis and stomatal conductance to rising [CO2]: mechanisms and environmental interactions. Plant Cell Environ. 30, 258–270. doi: 10.1111/j.1365-3040.2007.01641.x
Augusto, L., Achat, D. L., Jonard, M., Vidal, D., and Ringeval, B. (2017). Soil parent material-A major driver of plant nutrient limitations in terrestrial ecosystems. Glob. Change Biol. 23, 3808–3824. doi: 10.1111/gcb.13691
Bates, D., Maechler, M., Bolker, B., and Walker, S. (2015). Fitting linear mixed-effects models using lme4. J. Stat. Softw. 67, 1–48.
Beadle, N. C. W. (1966). Soil phosphate and its role in molding segments of australian flor and vegetation with special reference to xeromorphy and sclerophylly. Ecology 47, 992–1007. doi: 10.2307/1935647
Bieleski, R. L. (1973). Phosphate pools, phosphate transport, and phosphate availability. Annu. Rev. Plant Physiol. Plant Mol. Biol. 24, 225–252. doi: 10.1146/annurev.pp.24.060173.001301
Castle, S. C., and Neff, J. C. (2009). Plant response to nutrient availability across variable bedrock geologies. Ecosystems 12, 101–113. doi: 10.1007/s10021-008-9210-8
Chapin, F. S., Power, M. E., Pickett, S. T. A., Freitag, A., Reynolds, J. A., Jackson, R. B., et al. (2011). Earth stewardship: science for action to sustain the human-earth system. Ecosphere 2, 1–20.
Chiew, F. H. S., Piechota, T. C., Dracup, J. A., and McMahon, T. A. (1998). El nino southern oscillation and Australian rainfall, streamflow and drought: links and potential for forecasting. J. Hydrol. 204, 138–149. doi: 10.1016/s0022-1694(97)00121-2
Cleveland, C. C., Townsend, A. R., Taylor, P., Alvarez-Clare, S., Bustamante, M. M. C., Chuyong, G., et al. (2011). Relationships among net primary productivity, nutrients and climate in tropical rain forest: a pan-tropical analysis. Ecol. Lett. 14, 1313–1317. doi: 10.1111/j.1461-0248.2011.01658.x
Crous, K. Y., O’Sullivan, O. S., Zaragoza-Castells, J., Bloomfield, K. J., Negrini, A. C. A., Meir, P., et al. (2017). Nitrogen and phosphorus availabilities interact to modulate leaf trait scaling relationships across six plant functional types in a controlled-environment study. New Phytol. 215, 992–1008. doi: 10.1111/nph.14591
Crous, K. Y., Ósvaldsson, A., and Ellsworth, D. S. (2015). Is phosphorus limiting in a mature Eucalyptus woodland? Phosphorus fertilisation stimulates stem growth. Plant Soil 391, 293–305. doi: 10.1007/s11104-015-2426-4
de Campos, M. C. R., Pearse, S. J., Oliveira, R. S., and Lambers, H. (2013). Downregulation of net phosphorus-uptake capacity is inversely related to leaf phosphorus-resorption proficiency in four species from a phosphorus-impoverished environment. Ann. Bot. 111, 445–454. doi: 10.1093/aob/mcs299
Deng, Q., Hui, D. F., Luo, Y. Q., Elser, J., Wang, Y. P., Loladze, I., et al. (2015). Down-regulation of tissue N:P ratios in terrestrial plants by elevated CO2. Ecology 96, 3354–3362. doi: 10.1890/15-0217.1
Dijkstra, F. A., Pendall, E., Morgan, J. A., Blumenthal, D. M., Carrillo, Y., LeCain, D. R., et al. (2012). Climate change alters stoichiometry of phosphorus and nitrogen in a semiarid grassland. New Phytol. 196, 807–815. doi: 10.1111/j.1469-8137.2012.04349.x
Drake, J. E., Macdonald, C. A., Tjoelker, M. G., Crous, K. Y., Gimeno, T. E., Singh, B. K., et al. (2016). Short-term carbon cycling responses of a mature eucalypt woodland to gradual stepwise enrichment of atmospheric CO2 concentration. Glob. Change Biol. 22, 380–390. doi: 10.1111/gcb.13109
Duursma, R. A., Gimeno, T. E., Boer, M. M., Crous, K. Y., Tjoelker, M. G., and Ellsworth, D. S. (2016). Canopy leaf area of a mature evergreen Eucalyptus woodland does not respond to elevated atmospheric CO2 but tracks water availability. Glob. Change Biol. 22, 1666–1676. doi: 10.1111/gcb.13151
Ellsworth, D. S., Anderson, I. C., Crous, K. Y., Cooke, J., Drake, J. E., Gherlenda, A. N., et al. (2017). Elevated CO2 does not increase eucalypt forest productivity on a low-phosphorus soil. Nat. Clim. Chang. 7, 279–280.
Elser, J. J., Bracken, M. E. S., Cleland, E. E., Gruner, D. S., Harpole, W. S., Hillebrand, H., et al. (2007). Global analysis of nitrogen and phosphorus limitation of primary producers in freshwater, marine and terrestrial ecosystems. Ecol. Lett. 10, 1135–1142. doi: 10.1111/j.1461-0248.2007.01113.x
Elser, J. J., Schampel, J. H., Garcia-Pichel, F., Wade, B. D., Souza, V., Eguiarte, L., et al. (2005). Effects of phosphorus enrichment and grazing snails on modern stromatolitic microbial communities. Freshw. Biol. 50, 1808–1825. doi: 10.1111/j.1365-2427.2005.01451.x
Escudero, A., Delarco, J. M., Sanz, I. C., and Ayala, J. (1992). Effects of leaf longevity and retranslocation efficiency on the retention time of nutrients in the leaf biomass of different woody species. Oecologia 90, 80–87. doi: 10.1007/BF00317812
Evans, J. R. (1989). Photosynthesis and nitrogen relationships in leaves of C3 plants. Oecologia 78, 9–19. doi: 10.1007/BF00377192
Feller, I. C., Lovelock, C. E., and McKee, K. L. (2007). Nutrient addition differentially affects ecological processes of Avicennia germinans in nitrogen versus phosphorus limited mangrove ecosystems. Ecosystems 10, 347–359. doi: 10.1007/s10021-007-9025-z
Feng, Z. Z., Rutting, T., Pleijel, H., Wallin, G., Reich, P. B., Kammann, C. I., et al. (2015). Constraints to nitrogen acquisition of terrestrial plants under elevated CO2. Glob. Change Biol. 21, 3152–3168. doi: 10.1111/gcb.12938
Field, C. B., and Mooney, H. A. (1986). “The photosynthesis-nitrogen relationship in wild plants,” in On the Economy of Plant Form and Function, ed. T. J. Givnish (Cambridge, MA: Cambridge University Press), 25–55.
Finzi, A. C., DeLucia, E. H., Hamilton, J. G., Richter, D. D., and Schlesinger, W. H. (2002). The nitrogen budget of a pine forest under free air CO2 enrichment. Oecologia 132, 567–578. doi: 10.1007/s00442-002-0996-3
Fischer, A. M. (2007). “Nutrient remobilization during leaf senescence,” in Senescence Processes in Plants, ed. S. Gan (Oxford: Blackwell Publishing Ltd), 87–107. doi: 10.1002/9780470988855.ch5
Fitter, A., and Hillebrand, H. (2009). Microbial food web structure affects bottom-up effects and elemental stoichiometry in periphyton assemblages. Limnol. Oceanogr. 54, 2183–2200. doi: 10.4319/lo.2009.54.6.2183
Gimeno, T. E., Crous, K. Y., Cooke, J., O’Grady, A. P., Osvaldsson, A., Medlyn, B. E., et al. (2016). Conserved stomatal behaviour under elevated CO2 and varying water availability in a mature woodland. Funct. Ecol. 30, 700–709. doi: 10.1111/1365-2435.12532
Gimeno, T. E., McVicar, T. R., O’Grady, A. P., Tissue, D. T., and Ellsworth, D. S. (2018). Elevated CO2 did not affect the hydrological balance of a mature native Eucalyptus woodland. Glob. Change Biol. 24, 3010–3024. doi: 10.1111/gcb.14139
Goll, D. S., Brovkin, V., Parida, B. R., Reick, C. H., Kattge, J., Reich, P. B., et al. (2012). Nutrient limitation reduces land carbon uptake in simulations with a model of combined carbon, nitrogen and phosphorus cycling. Biogeosciences 9, 3547–3569. doi: 10.5194/bg-9-3547-2012
Güsewell, S. (2004). N : P ratios in terrestrial plants: variation and functional significance. New Phytol. 164, 243–266. doi: 10.1002/ece3.2587
Harpole, W. S., Ngai, J. T., Cleland, E. E., Seabloom, E. W., Borer, E. T., Bracken, M. E. S., et al. (2011). Nutrient co-limitation of primary producer communities. Ecol. Lett. 14, 852–862. doi: 10.1111/j.1461-0248.2011.01651.x
Hasegawa, S., Macdonald, C. A., and Power, S. A. (2016). Elevated carbon dioxide increases soil nitrogen and phosphorus availability in a phosphorus-limited Eucalyptus woodland. Glob. Change Biol. 22, 1628–1643. doi: 10.1111/gcb.13147
Hessen, D. O., Jensen, T. C., Kyle, M., and Elser, J. J. (2007). RNA responses to N- and P-limitation; reciprocal regulation of stoichiometry and growth rate in Brachionus. Funct. Ecol. 21, 956–962.
Hidaka, A., and Kitayama, K. (2011). Allocation of foliar phosphorus fractions and leaf traits of tropical tree species in response to decreased soil phosphorus availability on mount kinabalu. Borneo. J. Ecol. 99, 849–857. doi: 10.1111/j.1365-2745.2011.01805.x
Hopper, S. D. (2009). OCBIL theory: towards an integrated understanding of the evolution, ecology and conservation of biodiversity on old, climatically buffered, infertile landscapes. Plant Soil 322, 49–86. doi: 10.1007/s11104-009-0068-0
Hothorn, T., Bretz, F., and Westfall, P. (2008). Simultaneous inference in general parametric models. Biom. J. 50, 346–363. doi: 10.1002/bimj.200810425
Huang, W. J., Houlton, B. Z., Marklein, A. R., Liu, J. X., and Zhou, G. Y. (2015). Plant stoichiometric responses to elevated CO2 vary with nitrogen and phosphorus inputs: evidence from a global-scale meta-analysis. Sci. Rep. 5:18225. doi: 10.1038/srep18225
Jacob, J., and Lawlor, D. W. (1991). Stomatal and mesphyll limitations of photosynthesis in phosphate deficient sunflower, maize and wheat plants. J. Exp. Bot. 42, 1003–1011. doi: 10.1093/jxb/42.8.1003
Judd, T. S., Attiwill, P. M., and Adams, M. A. (1996). “Nutrient concentrations in Eucalyptus: a synthesis in relation to differences between taxa, sites and components,” in The Nutrition of Eucalypts, eds P. M. Attiwill and M. A. Adams (Melbourne, VIC: CSIRO Publishing), 123–153.
Killingbeck, K. T. (1996). Nutrients in senesced leaves: keys to the search for potential resorption and resorption proficiency. Ecology 77, 1716–1727. doi: 10.2307/2265777
Koerselman, W., and Meuleman, A. F. M. (1996). The vegetation N:P ratio: a new tool to detect the nature of nutrient limitation. J. Appl. Ecol. 33, 1441–1450.
Kooyman, R. M., Laffan, S. W., and Westoby, M. (2017). The incidence of low phosphorus soils in Australia. Plant Soil 412, 143–150. doi: 10.1007/s11104-016-3057-0
Lambers, H., Brundrett, M. C., Raven, J. A., and Hopper, S. D. (2010). Plant mineral nutrition in ancient landscapes: high plant species diversity on infertile soils is linked to functional diversity for nutritional strategies. Plant Soil 334, 11–31. doi: 10.1007/s11104-010-0444-9
LeBauer, D. S., and Treseder, K. K. (2008). Nitrogen limitation of net primary productivity in terrestrial ecosystems is globally distributed. Ecology 89, 371–379. doi: 10.1890/06-2057.1
Liu, J. X., Huang, W. J., Zhou, G. Y., Zhang, D. Q., Liu, S. Z., and Li, Y. Y. (2013). Nitrogen to phosphorus ratios of tree species in response to elevated carbon dioxide and nitrogen addition in subtropical forests. Glob. Change Biol. 19, 208–216. doi: 10.1111/gcb.12022
Luo, Y., Su, B., Currie, W. S., Dukes, J. S., Finzi, A. C., Hartwig, U., et al. (2004). Progressive nitrogen limitation of ecosystem responses to rising atmospheric carbon dioxide. Bioscience 54, 731–739.
Marklein, A. R., and Houlton, B. Z. (2012). Nitrogen inputs accelerate phosphorus cycling rates across a wide variety of terrestrial ecosystems. New Phytol. 193, 696–704. doi: 10.1111/j.1469-8137.2011.03967.x
Matear, R. J., Wang, Y. P., and Lenton, A. (2010). Land and ocean nutrient and carbon cycle interactions. Curr. Opin. Environ. Sustain. 2, 258–263. doi: 10.1016/j.cosust.2010.05.009
McGroddy, M. E., Daufresne, T., and Hedin, L. O. (2004). Scaling of C : N : P stoichiometry in forests worldwide: implications of terrestrial redfield-type ratios. Ecology 85, 2390–2401. doi: 10.1890/03-0351
Milla, R., Palacio-Blasco, S., Maestro-Martinez, M., and Montserrat-Marti, G. (2006). Phosphorus accretion in old leaves of a mediterranean shrub growing at a phosphorus-rich site. Plant Soil 280, 369–372. doi: 10.1007/s11104-005-3529-0
Pathare, V. S., Crous, K. Y., Cooke, J., Creek, D., Ghannoum, O., and Ellsworth, D. S. (2017). Water availability affects seasonal CO2-induced photosynthetic enhancement in herbaceous species in a periodically dry woodland. Glob. Change Biol. 23, 5164–5178. doi: 10.1111/gcb.13778
Phillips, R. P., Meier, I. C., Bernhardt, E. S., Grandy, A. S., Wickings, K., and Finzi, A. C. (2012). Roots and fungi accelerate carbon and nitrogen cycling in forests exposed to elevated CO2. Ecol. Lett. 15, 1042–1049. doi: 10.1111/j.1461-0248.2012.01827.x
R Core Team (2018). R: A Language and Environment for Statistical Computing. Vienna: R Foundation for Statistical Computing.
Rao, I. M., and Terry, N. (1995). Leaf phosphate status, photosynthesis and carbon partitioning in sugar beet. 4. changes with time following increased supply of phosphate to low-phosphate plants. Plant Physiol. 107, 1313–1321. doi: 10.1104/pp.107.4.1313
Reed, S. C., Townsend, A. R., Davidson, E. A., and Cleveland, C. C. (2012). Stoichiometric patterns in foliar nutrient resorption across multiple scales. New Phytol. 196, 173–180. doi: 10.1111/j.1469-8137.2012.04249.x
Reich, P. B., and Oleksyn, J. (2004). Global patterns of plant leaf N and P in relation to temperature and latitude. Proc. Natl. Acad. Sci. U.S.A. 101, 11001–11006. doi: 10.1073/pnas.0403588101
Reich, P. B., Tjoelker, M. G., Pregitzer, K. S., Wright, I. J., Oleksyn, J., and Machado, J.-L. (2008). Scaling of respiration to nitrogen in leaves, stems and roots of higher land plants. Ecol. Lett. 11, 793–801. doi: 10.1111/j.1461-0248.2008.01185.x
Sardans, J., and Peñuelas, J. (2012). The role of plants in the effects of global change on nutrient availability and stoichiometry in the plant-soil system. Plant Physiol. 160, 1741–1761. doi: 10.1104/pp.112.208785
Sardans, J., and Peñuelas, J. (2013). Tree growth changes with climate and forest type are associated with relative allocation of nutrients, especially phosphorus, to leaves and wood. Glob. Ecol. Biogeogr. 22, 494–507. doi: 10.1111/geb.12015
Sardans, J., Rivas-Ubach, A., and Peñuelas, J. (2012). The C:N:P stoichiometry of organisms and ecosystems in a changing world: a review and perspectives. Perspect. Plant Ecol. Evol. Syst. 14, 33–47. doi: 10.1016/j.ppees.2011.08.002
Specht, R. L., and Rundel, P. W. (1990). Sclerophylly and foliar nutrient status of mediterranean-climate plant communities in Southern Australia. Aust. J. Bot. 38, 459–474.
Sterner, R. W., and Elser, J. J. (2002). Ecological Stoichiometry. Princeton, N.J: Princeton University Press.
Sterner, R. W., and George, N. B. (2000). Carbon, nitrogen, and phosphorus stoichiometry of cyprinid fishes. Ecology 81, 127–140. doi: 10.1890/0012-9658(2000)081
Turner, B. L., Yavitt, J. B., Harms, K. E., Garcia, M. N., Romero, T. E., and Wright, S. J. (2013). Seasonal changes and treatment effects on soil inorganic nutrients following a decade of fertilizer addition in a lowland tropical forest. Soil Sci. Soc. Am. J. 77, 1357–1369.
van Groenigen, K. J., de Graaff, M. A., Six, J., Harris, D., Kuikman, P., and van Kessel, C. (2006). The impact of elevated atmospheric [CO2] on soil C and N dynamics: a meta-analysis. Manag. Ecosyst. CO2 187, 373–391. doi: 10.1007/3-540-31237-4_21
Vergutz, L., Manzoni, S., Porporato, A., Novais, R. F., and Jackson, R. B. (2012). Global resorption efficiencies and concentrations of carbon and nutrients in leaves of terrestrial plants. Ecol. Monogr. 82, 205–220. doi: 10.1890/11-0416.1
Vitousek, P. M., and Farrington, H. (1997). Nutrient limitation and soil development: experimental test of a biogeochemical theory. Biogeochemistry 37, 63–75.
Vitousek, P. M., Ladefoged, T. N., Kirch, P. V., Hartshorn, A. S., Graves, M. W., Hotchkiss, S. C., et al. (2004). Soils, agriculture, and society in precontact Hawaii. Science 304, 1665–1669. doi: 10.1126/science.1099619
Vitousek, P. M., Porder, S., Houlton, B. Z., and Chadwick, O. A. (2010). Terrestrial phosphorus limitation: mechanisms, implications, and nitrogen-phosphorus interactions. Ecol. Appl. 20, 5–15. doi: 10.1890/08-0127.1
Walker, T. W., and Syers, J. K. (1976). Fate of phosphorus during pedogenesis. Geoderma 15, 1–19. doi: 10.3390/ijerph14121475
Wassen, M. J., de Boer, H. J., Fleischer, K., Rebel, K. T., and Dekker, S. C. (2013). Vegetation-mediated feedback in water, carbon, nitrogen and phosphorus cycles. Landsc. Ecol. 28, 599–614. doi: 10.1007/s10980-012-9843-z
Wright, I. J., Reich, P. B., Westoby, M., Ackerly, D. D., Baruch, Z., Bongers, F., et al. (2004). The worldwide leaf economics spectrum. Nature 428, 821–827.
Wright, I. J., and Westoby, M. (2003). Nutrient concentration, resorption and lifespan: leaf traits of Australian sclerophyll species. Funct. Ecol. 17, 10–19. doi: 10.1046/j.1365-2435.2003.00694.x
Wujeska-Klause, A., Crous, K. Y., Ghannoum, O., and Ellsworth, D. S. (2019). Lower photorespiration in elevated CO2 reduces leaf N concentrations in mature Eucalyptus trees in the field. Glob. Change Biol. 25, 1282–1295. doi: 10.1111/gcb.14555
Yang, X., and Post, W. M. (2011). Phosphorus transformations as a function of pedogenesis: a synthesis of soil phosphorus data using hedley fractionation method. Biogeosciences 8, 2907–2916. doi: 10.5194/bg-8-2907-2011
Yuan, Z. Y., and Chen, H. Y. H. (2015). Decoupling of nitrogen and phosphorus in terrestrial plants associated with global changes. Nat. Clim. Chang. 5, 465–469. doi: 10.1038/nclimate2549
Yue, K., Fornara, D. A., Yang, W. Q., Peng, Y., Li, Z. J., Wu, F. Z., et al. (2017). Effects of three global change drivers on terrestrial C:N:P stoichiometry: a global synthesis. Glob. Change Biol. 23, 2450–2463. doi: 10.1111/gcb.13569
Zaehle, S., Medlyn, B. E., De Kauwe, M. G., Walker, A. P., Dietze, M. C., Hickler, T., et al. (2014). Evaluation of 11 terrestrial carbon-nitrogen cycle models against observations from two temperate free-air CO2 enrichment studies. New Phytol. 202, 803–822. doi: 10.1111/nph.12697
Keywords: elevated CO2 concentration, FACE, N:P ratio, stoichiometry, phosphorus limitation, senesced leaves, resorption
Citation: Crous KY, Wujeska-Klause A, Jiang M, Medlyn BE and Ellsworth DS (2019) Nitrogen and Phosphorus Retranslocation of Leaves and Stemwood in a Mature Eucalyptus Forest Exposed to 5 Years of Elevated CO2. Front. Plant Sci. 10:664. doi: 10.3389/fpls.2019.00664
Received: 29 November 2018; Accepted: 02 May 2019;
Published: 31 May 2019.
Edited by:
Scott Alan Heckathorn, The University of Toledo, United StatesReviewed by:
Mitsutoshi Kitao, Forestry and Forest Products Research Institute, JapanJean-Paul Laclau, Centre de Coopération Internationale en Recherche Ggronomique pour le Développement (CIRAD), France
Copyright © 2019 Crous, Wujeska-Klause, Jiang, Medlyn and Ellsworth. This is an open-access article distributed under the terms of the Creative Commons Attribution License (CC BY). The use, distribution or reproduction in other forums is permitted, provided the original author(s) and the copyright owner(s) are credited and that the original publication in this journal is cited, in accordance with accepted academic practice. No use, distribution or reproduction is permitted which does not comply with these terms.
*Correspondence: Kristine Y. Crous, ay5jcm91c0B3ZXN0ZXJuc3lkbmV5LmVkdS5hdQ==