- 1Research Institute for Bioresources and Biotechnology, Ishikawa Prefectural University, Ishikawa, Japan
- 2Department of Biological Production, Faculty of Bioresource Sciences, Akita Prefectural University, Akita, Japan
- 3Center for Liberal Arts, Meiji Gakuin University, Kanagawa, Japan
- 4Department of Global Agricultural Sciences, The University of Tokyo, Tokyo, Japan
- 5Crop Biotech Institute and Graduate School of Biotechnology, Kyung Hee University, Yongin, South Korea
Iron (Fe) toxicity in plants causes tissue damage and cellular homeostasis disorders, thereby affecting plant growth and development. Nicotianamine (NA) is a ubiquitous chelator of metal cations and is responsible for metal homeostasis. Rice has three NA synthase (NAS) genes, of which the expression of OsNAS1 and OsNAS2 but not of OsNAS3 is strongly induced in response to Fe deficiency. Recently, we found that OsNAS3 expression is strongly induced with excess Fe in most rice tissues, particularly old leaves, suggesting that it may play a vital role under excess Fe conditions. However, the mechanism by which OsNAS3 responds to excess Fe in rice remains poorly understood. In this study, we clarified the physiological response of OsNAS3 expression to excess Fe and the role of NA synthesis in this condition. Promoter GUS analyses revealed that OsNAS3 was widely expressed in roots, especially in vascular bundle, epidermis, exodermis, stem, and old leaf tissues under Fe excess compared to control plants. Nicotianamine and deoxymugineic acid (DMA; a type of phytosiderophore synthesized by Strategy II species) were present in roots and shoots under Fe excess likewise under control conditions. In addition, OsNAS3 knockout plants were sensitive to excess Fe, exhibiting inferior growth, reduced dry weight, severer leaf bronzing, and greater Fe accumulation in their leaves than non-transformants with excess Fe. We also observed that NA-overproducing rice was tolerant of excess Fe. These results show that NA synthesized by OsNAS3 under Fe excess condition is to mitigate excess Fe whereas NA synthesized by OsNAS1 and OsNAS2 under normal Fe condition is to enhance Fe translocation, suggesting the different roles and functions of the NA existence between these two conditions. Overall, these findings suggest that rice synthesizes NA with OsNAS3 under Fe excess in roots and shoots, and that NA and DMA within the plant body are important for mitigating excess Fe stress and alleviating other metal deficiencies in rice. This report will be important for the development of tolerant rice adapted to Fe-contaminated soils.
Introduction
Iron (Fe) is an essential nutrient for most living organisms and is a key determinant of crop production, yield, and quality; however, it can be toxic when hyperaccumulated within cells. Iron toxicity is one of the most important stressors of rice in many lowland environments worldwide. Iron is reduced from ferric ion [Fe(III)] to more soluble ferrous ion [Fe(II)] when submerged in water. Ferrous ion is more easily dissolved in water (Ksp = 8 × 10-16) compared to ferric ion (Ksp = ∼1 × 10-36) at 25°C (Stumm and Lee, 1961). Thus, Fe toxicity occurs more readily in submerged environments with acid soils. Acid soils occupy approximately 30% (or 3950 million hectares) of land worldwide, and approximately 50% of the world’s arable land is estimated to be acidic (von Uexküll and Mutert, 1995). Iron toxicity is a serious constraint on rice growth and yield in regions where rice production is high such as China and Southeast Asia, and the regions mainly characterized by acid soils such as ultisols (NRCS, 2005). Iron overload in plants leads to leaf bronzing, tissue damage, and cellular homeostasis disorders. To mitigate this problem and maintain Fe homeostasis within the plant body, plants use strict and sophisticated Fe regulation mechanisms.
Nicotianamine (NA) is a ubiquitous plant-derived chelator of various divalent cations such as Fe2+ and Zn2+, and is biosynthesized from S-adenosylmethionine by NA synthase (NAS; Higuchi et al., 1994). All higher plants synthesize NA and utilize it for chelation as well as internal transport of Fe2+ and other metal cations to maintain metal homeostasis (Hell and Stephan, 2003; Takahashi et al., 2003). Numerous studies have reported disrupted internal metal transport in NA-defective plants. For example, NA-defective tomato mutant exhibits an iron-deficient phenotype (Pich and Scholz, 1996; Stephan et al., 1996), NA-deficient transgenic tobacco plants have serious chlorosis in young leaves and decreased Fe and zinc (Zn) concentrations in leaves and flowers (Takahashi et al., 2003), and the Arabidopsis AtNAS quadruple mutant has a decreased Fe concentration in its seeds (Klatte et al., 2009). Introducing overexpression of a barley NAS gene, HvNAS1, to tobacco plants leads to increased Fe and Zn concentrations in the leaves, flowers, and seeds (Takahashi et al., 2003). These reports suggest that NA plays an important role in internal Fe transport in higher plants.
In rice, NA is biosynthesized by three NAS enzymes: OsNAS1, OsNAS2, and OsNAS3 (Higuchi et al., 2001), all of which exhibit NA synthase activity in vitro (Inoue et al., 2003). Among three encoding genes, expression of OsNAS1 and OsNAS2 is strongly induced in rice roots and yellow leaves under Fe deficiency, whereas OsNAS3 expression is mildly induced in roots but is suppressed in leaves under Fe deficiency (Inoue et al., 2003). The sequences of OsNAS1 and OsNAS2 are located very close to each other on rice chromosome 3, whereas OsNAS3 is located on chromosome 7 (Higuchi et al., 2001; Inoue et al., 2003). Many other genes involved in Fe acquisition under Fe deficiency also show Fe deficiency-induced expression changes similar to those of the OsNAS1 and OsNAS2 genes, but not that of OsNAS3 (Kobayashi et al., 2014). Thus, OsNAS3 has a unique expression pattern compared to OsNAS1 or OsNAS2, and as such, likely plays a different role than OsNAS1 and OsNAS2.
Nicotianamine is not only involved in long-distance Fe transport in rice, but also serves as a substrate for production of deoxymugineic acid (DMA) via a 3″–oxo intermediate by NA aminotransferase (NAAT) and DMA synthase (DMAS) (Mori and Nishizawa, 1987; Shojima et al., 1989). In rice, one NAAT gene (OsNAAT1) and one DMAS gene (OsDMAS1) have been isolated (Bashir et al., 2006; Inoue et al., 2008). For DMA, it serves as a type of mugineic acid family phytosiderophore synthesized and secreted from graminaceous plants under Fe-deficient conditions to acquire Fe from the soil (Takagi, 1976; Marschner et al., 1986; Marschner and Romheld, 1994). Iron is thought to chelate with NA and DMA in the rice plant body and is translocated to various tissues including seeds. A correlation has been found between seed NA or DMA concentration and seed Fe concentration in transgenic rice with higher NAS expression (Masuda et al., 2009; Johnson et al., 2011).
Nicotianamine also plays a vital role in enhancing the nutritional quality of rice and is a candidate for biofortification of rice. For example, overexpression of the NAS gene increases Fe and Zn concentrations in rice seeds (Lee et al., 2009; Masuda et al., 2009; Johnson et al., 2011) and rice glutelin B1 promoter-driven OsNAS1 increases Fe concentrations in leaves and polished seeds (Zheng et al., 2010). Nicotianamine works synergistically with other genes to achieve high Fe in rice grains. For example, HvNAS1, GmFER, and OsYSL2 (Masuda et al., 2012; Aung et al., 2013), OsNAS2 and GmFER (Trijatmiko et al., 2016), and AtNAS1, AtIRT1, and PvFER (Boonyaves et al., 2017) together contribute to grain Fe accumulation. The increased Fe content in rice grains caused by enhanced NAS expression is also bioavailable (Lee et al., 2009). These reports suggest that NA synthesized by NAS enhances Fe translocation within rice plants to the seeds.
Nicotianamine is thought to play vital roles not only in Fe deficiency tolerance and seed Fe accumulation but also in detoxification of excess intracellular Fe (von Wirén et al., 1999). Former studies have shown the importance of NA in heavy metal metabolism in dicot plants (Pich et al., 2001; Takahashi et al., 2003; Douchkov et al., 2005; Kim et al., 2005; Han et al., 2018) and monocot plants (Lee et al., 2009; Aung et al., 2018). For example, among dicot plants, Pich et al. (2001) suggested a possible role of NA in vacuolar sequestration for detoxification of excess Fe in pea and tomato. In other dicots, transgenic Arabidopsis and tobacco plants overexpressing HvNAS1 tolerated excess metal toxicity, particularly Ni (Kim et al., 2005). In addition, overexpression of apple MxNAS1, MxNAS2 and MxNAS3 genes led to enhanced tolerance to low and high levels of Fe stress in transgenic tomato (Han et al., 2013; Yang et al., 2015) and transgenic Arabidopsis by influencing NA synthesis (Han et al., 2018), respectively.
In dicot plants, NA functions exclusively as a metal chelator, as they do not produce MAs. In graminaceous plants, NA plays two roles, functioning not only as a metal chelator for internal transport but also as a precursor of MAs. In the monocot rice, overexpression of OsNAS3 increased Fe and Zn concentrations and NA levels in grains as well as tolerance to Fe and Zn deficiencies and Zn, copper (Cu), and nickel (Ni) toxicities (Lee et al., 2009). Recently, we reported the transcriptomic analyses of various rice tissues in response to ferrous Fe toxicity (Aung et al., 2018). The results showed that Fe homeostasis-related genes were suppressed under excess Fe, principally in the roots. In particular, the NA synthase genes OsNAS1 and OsNAS2 were clearly suppressed in the roots and junction nodes between the root and shoot (discrimination center, DC). By contrast, OsNAS3 expression was increased in all tissues in response to excess Fe, by 2- to 10-fold in the roots, 2- to 9-fold in the DC, 2- to 8-fold in stems, 10- to 120-fold in old leaves and 3- to 22-fold in the newest leaves. Under Fe excess, OsNAS3 expression was as high as that of other important Fe excess-responsive genes, such as those of the Fe storage protein ferritin and vacuolar Fe transporter OsVIT2 (Aung et al., 2018). These results suggest that NA synthesized by induced OsNAS3 may play an important role in rice under Fe excess conditions. However, further in-depth analyses of OsNAS3 are required to elucidate its physiological function in Fe excess and its role in Fe detoxification in rice.
In this study, we clarified the roles of NA and OsNAS3 in rice under Fe excess conditions. To this end, we analyzed spatial expression patterns in various tissues of OsNAS3 promoter-GUS plants (roots, DC, stems, old leaves, and newest leaves) in response to Fe excess. In addition, the amounts of NA and DMA in roots and shoots were investigated. An OsNAS3 knockout line was cultivated under control and Fe excess conditions for observation of growth, leaf bronzing, metal concentrations, and Fe histochemical localization. Moreover, tolerance to Fe excess was observed in a NA-overproducing rice line. These results suggest a novel role for NA synthesized by OsNAS3 in the Fe detoxification process.
Materials and Methods
Plant Materials and Growth Conditions in Hydroponic Culture
Plant Cultivation by Hydroponic Culture
Rice seeds were germinated on Murashige and Skoog (MS) medium (Murashige and Skoog, 1962) with and without hygromycin B (50 mg L-1) for transformant and non-transformant (NT) plants, respectively. The seedlings were grown in modified Kasugai’s hydroponic culture solution (× 1 Fe; 35.7 μM FeCl2) at pH 5.5 for 1 week, as previously described by Aung et al. (2018), after which the plants were cultivated under conditions of control Fe (× 1 Fe) or ferrous Fe excess (× 70 Fe; 2.50 mM FeCl2) at pH 4.0. The pH of the solution was adjusted to 4.0 every 2 days, and the culture solution was renewed every week. One plant per hill and three or four biological replicates were used for each Fe condition. All experiments were conducted in a greenhouse at 30°C during the 14 h day and 25°C during the 10 h night with natural light.
Growth Analyses of OsNAS3 Knockout Rice Plants
Rice seeds (Oryza sativa L. cv. Hwayoung) of NT and the OsNAS3 knockout mutant line 2D30228, osnas3–1 (Lee et al., 2009) were obtained from the Rice T-DNA Insertion Sequence Database (POSTECH; Pohang University of Science and Technology, Pohang, Korea; Jeong et al., 2002). The T-DNA insertion position was described by Lee et al. (2009).
The 18-day-old seedlings (first experiment) or 14-day-old seedlings (second experiment) of knockout and NT plants were cultured hydroponically and exposed to excess Fe in hydroponic culture as described above for 39 days (first experiment) or 23 days (second experiment). Four biological replicates were used for each Fe condition. In both experiments, shoot and root lengths were measured throughout cultivation. The severity of Fe toxicity in leaves was measured after 11 or 17 days of exposure to excess Fe by determining the bronzing score of the fully expanded newest leaf (NL) and older leaves, as previously described by Aung et al. (2018).
Growth Analyses of NAS-Overexpressing Rice Plants
Rice seeds (O. sativa L. cv. Tsukinohikari) of NT plants and the T4 generation of NAS-overexpressing (Actin promoter-HvNAS1) plants (Masuda et al., 2009) were used for growth analyses. Eighteen-day-old seedlings were cultured hydroponically and exposed to excess Fe in hydroponic culture for 39 days. Plant growth and bronzing scores were measured as described above.
Histochemical Analyses of Promoter-GUS Rice Lines
The seeds of the OsNAS3 promoter-GUS lines (T3 and T4 seeds; O. sativa L. cv. Tsukinohikari) described by Inoue et al. (2003) were used for histochemical analyses. Fifteen-day-old seedlings were transferred to hydroponic culture and exposed to excess Fe for 14 days. Roots, leaf blades, stems, DCs were cut with a scalpel into approximately 1 cm sections. Then, the sections were embedded into 5% agar and cut into 100 μm transverse, or longitudinal sections by a DTK-1000N MicroSlicer (Dosaka EM Co., Ltd., Kyoto, Japan) as described by Kobayashi et al. (2010). GUS reaction buffer was prepared with 1 mM 5-bromo-4-chloro-3-indolyl-ß-D-glucuronide Cyclohexyl ammonium salt (X-Gluc; Wako, Japan) in staining buffer (100 mM Na2HPO4, 1 mM K3Fe(CN)6, 1 mM K4Fe(CN)6, and 20% methanol). The samples were subjected to histochemical assays for GUS activity, with vacuum infiltration on ice for 30 min and then incubated at 37°C dark for 7 min for roots, 1 min for DCs, 9 min for stems, 2 h 30 min for old leaves and 1 h for the newest leaves. Once the staining appeared, the reactions of both control and Fe excess sections were stopped at the same time by washing 70% ethanol and then reserved in 70% ethanol. Histochemical localization was observed in both the T3 and T4 generations of at least two independent lines under a Zeiss Axion Vision Image 4.2 microscope and a Zeiss Axioskop 2 Plus fluorescence microscope (Carl Zeiss Microscopy, Jena, Germany).
NA and DMA Concentration Analyses
Rice seeds (O. sativa L. cv. Tsukinohikari) were used for NA and DMA concentration analyses. Sixteen-day-old rice seedlings were transferred to hydroponic culture, and half were exposed to excess Fe at pH 4.0 for 14 days. Four biological replicates were used in control (× 1 Fe) and Fe excess (× 70 Fe) hydroponic cultures. Leaf and root samples were ground to powder in liquid nitrogen using a mortar and pestle, and then the endogenous NA and DMA concentrations of the samples were determined through high-performance liquid chromatography according to the method described by Nozoye et al. (2014).
Metal Concentration Analyses
Samples of roots and shoots (newest leaves and third newest leaves) from control and Fe-treated plants (from the second experiment; 23 days of excess Fe exposure) were collected for metal concentration analyses. The roots were washed with distilled water or Milli-Q water containing 50 mM sodium ethylenediaminetetraacetic acid (Na-EDTA) for control or Fe excess plants, respectively. Root and leaf samples were dried for 3 days at 60°C, and 50–200 mg samples were digested with 2 mL HNO3 and 2 mL H2O2 at 220°C for 20 min using the MARS XPRESS oven (CEM Japan, Tokyo, Japan), and then messed up and filtered as described by Masuda et al. (2009). The concentrations of Fe, Zn, manganese (Mn), and Cu were measured with an inductively coupled plasma atomic emission spectrometer (ICPS-8100; Shimadzu, Kyoto, Japan). Three biological replicates were performed.
Iron Histochemical Localization Analyses
To detect the presence of Fe in the leaf tissues of OsNAS3 knockout plants, leaf sections from control and Fe excess cultures were obtained (from the second experiment) and placed in ethanol for 24 h to remove the chlorophyll. Then the sections were exposed to a solution containing 2% potassium ferricyanide (Wako Co., Ltd., Tokyo, Japan) and 2% hydrochloric acid (Wako) for 24 h. After rinsing with distilled water, the sections were mounted in distilled water and localization was observed under the Zeiss Axion Vision Image 4.2 microscope (Carl Zeiss Microscopy).
Quantitative Real-Time RT-PCR Analyses
Total RNA from rice prepared for the microarray analyses as described by Aung et al. (2018) was used to confirm the expression patterns of the genes OsDMAS1 and OsNAAT1 under Fe excess conditions by quantitative real-time polymerase chain reaction (RT-PCR) analyses. For the expression analyses of OsNAS3 knockout and NT plants from ×1 Fe and ×70 Fe cultures, rice RNA was extracted from hydroponically grown leaves. For all samples, the first-strand cDNA was synthesized using the ReverTra Ace reverse transcriptase kit (Toyobo, Osaka, Japan) and oligo-d(T)30 primers. Then, qPCR was performed in a StepOnePlusTM Real-Time PCR System (Life Technology, Tokyo, Japan) with SYBR Premix Ex Taq II reagent (Takara, Shiga, Japan). The transcript abundance was normalized against the rice alpha-tubulin transcript level. The primer sequences used for gene expression analyses were as follows: 5′GCC GGC ATC CCG GCA GCG GAA GAT CA 3′ for OsDMAS1 FW and 5′ CTC TCT CTC TCG GGC ACG TGC TAG CGT 3′ for OsDMAS1 RV; 5′-TAAGAGGATAATTGATTTGCTTAC-3′ for OsNAAT1 FW and 5′-CTGATCATTCCAATCCTAGTACAAT-3′ for OsNAAT1 RV; 5′ CGA TGA CTG CTT CCA TCG CTT G 3′ for OsNAS3 FW and 5′ GGC A TG CAT TCA TGC ATG ACT GC 3′ for OsNAS3 RV; 5′ TCT TCC ACC CTG AGC AGC TC 3′ for alpha-tubulin FW and 5′ AAC CTT GGA GAC CAG TGC AG 3′ for alpha-tubulin RV.
Statistical Analyses
Statistical analyses were conducted using Microsoft Excel software. Comparisons were made between ×1 Fe and each level of Fe excess in each tissue. For each set of comparisons, a two-sample Student’s t-test for an equal or unequal variance was performed based on the F-test for equal variance.
Results
Expression of OsNAS3 Was Observed in All Tissues Investigated Under Fe Excess
To uncover the physiological role of OsNAS3 in Fe excess, the tissue-specific expression of OsNAS3 was investigated based on histochemical localization in transgenic rice plants with the introduced OsNAS3 promoter–GUS described by Inoue et al. (2003). Expression of OsNAS3 was observed in every tissue investigated. In the transverse section of roots under control Fe conditions, OsNAS3 expression was mainly restricted to phloem cells and lateral roots (Figures 1A,C,F). Under excess Fe, strong expression was observed throughout the entire root, particularly in the vascular bundles, exodermis, and epidermis of the roots observed in transverse sections (Figures 1B,D,E,G). Enlarged transverse sections of the vascular bundle exhibited extremely deep staining in the phloem cells, phloem companion cells, protoxylem, xylem parenchyma cells, and epidermal cells (Figures 1D,E). In the longitudinal sections, OsNAS3 expression in roots was very clear under Fe excess compared to control conditions (Figures 1H,I). Expression of OsNAS3 was observed in lateral roots in rice under both control and Fe excess conditions (Figure 1A and Supplementary Figure S1A).
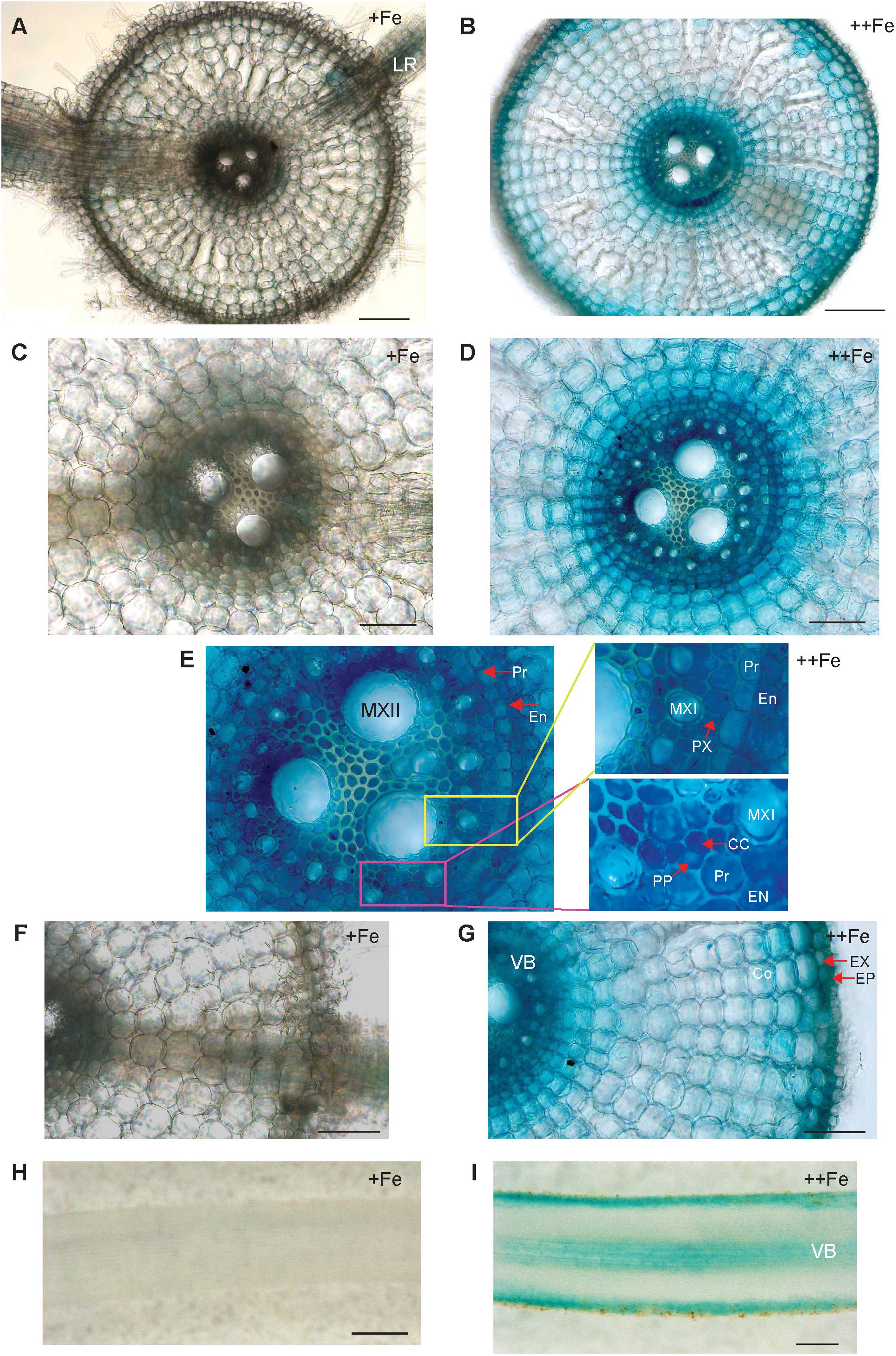
Figure 1. Cellular localization of OsNAS3 promoter-GUS expression in roots. (A) Transverse section of control root. (B) Transverse section of Fe-excess root. (C,D) Enlarged views of vascular tissues shown in (A,B), respectively. (E) An enlarged view of xylem and phloem shown in (D). (F,G) Enlarged views of outer root layers shown in (A,B), respectively. (H) Longitudinal section of control root. (I) Longitudinal section of Fe-excess root. LR, lateral root; MXI, metaxylem I; MXII, metaxylem II; PP, protophloem; CC, companion cells; EN, endodermis; Pr, pericycle; PX, protoxylem; EX, exodermis; EP, epidermis; Co, cortex; VB, vascular bundle. Scale bars: 20 μm for (A–D,F,G); 100 μm for (H,I).
In old leaf blades, weak expression of OsNAS3 under Fe sufficiency was present only in the small vascular bundles and phloem cells of large vascular bundles (Figures 2A,C). Under Fe excess, leaves showed stronger expression of OsNAS3 in the small and large vascular bundles (Figure 2B) and xylem parenchyma and phloem cells (Figure 2D). In addition, weak expression was observed in extracellular veins and chloroplasts within mesophyll cells in leaves with excess Fe (Figure 2D and Supplementary Figure S1B). In the midrib of the control Fe leaf, expression was very weak (Figure 2E), whereas the Fe excess leaf midrib showed dominant expression of OsNAS3 in the vascular bundles, bundle sheath cells and adjacent cells, the lower and upper epidermis (Figures 2F,G), trichomes (Figure 2H), some parts of the bulliform (motor) cells present on the adaxial side of the leaf (Figure 2I), and also in the veins connecting small vascular bundles (Figure 2J). The outer layer of the stem showed dominant expression under excess Fe than under control (Figures 3A,B). In the inner part of the stem, expression of OsNAS3 in the prospective new leaves that would soon emerge, but were folded inside stem tissue at the time, was more pronounced under excess Fe compared to control Fe condition (Figures 3C–F).
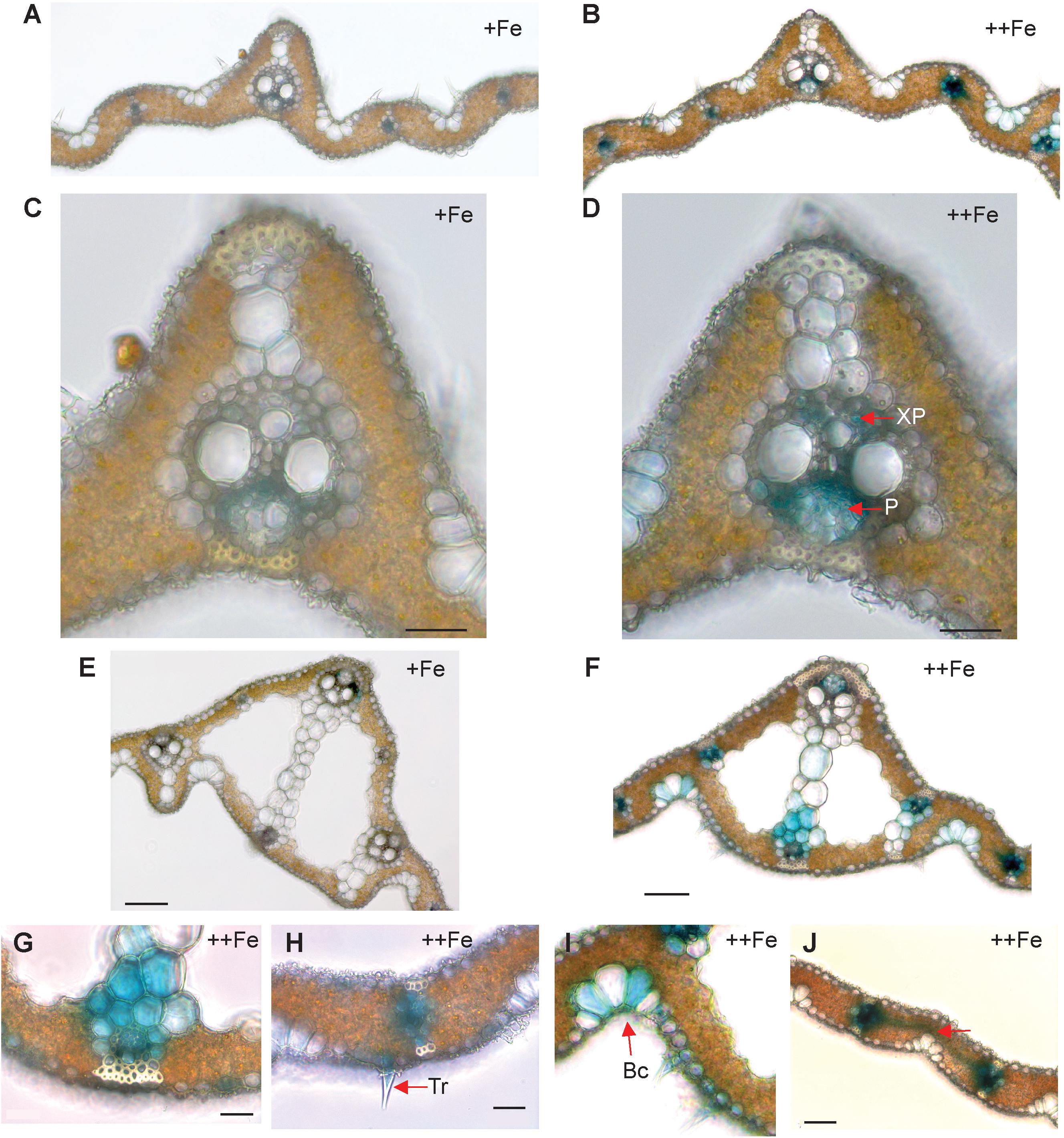
Figure 2. Cellular localization of OsNAS3 promoter-GUS expression in old leaves. (A) Transverse sections of control old leaf. (B) Fe-excess old leaf. (C) Enlarged view of control leaf. (D) Enlarged view of Fe-excess leaf. (E) Midrib of control leaf. (F) Midrib of Fe-excess leaf. (G) An enlarged view of (F). (H) Fe excess leaf showing trichome. (I) Fe excess leaf showing bulliform cells. (J) Vascular vein connecting vascular bundles of Fe-excess leaf shown by arrow. XP, xylem parenchyma; P, phloem; Tr, trichome; Bc, bulliform cells. Scale bars: 20 μm for (C–J).
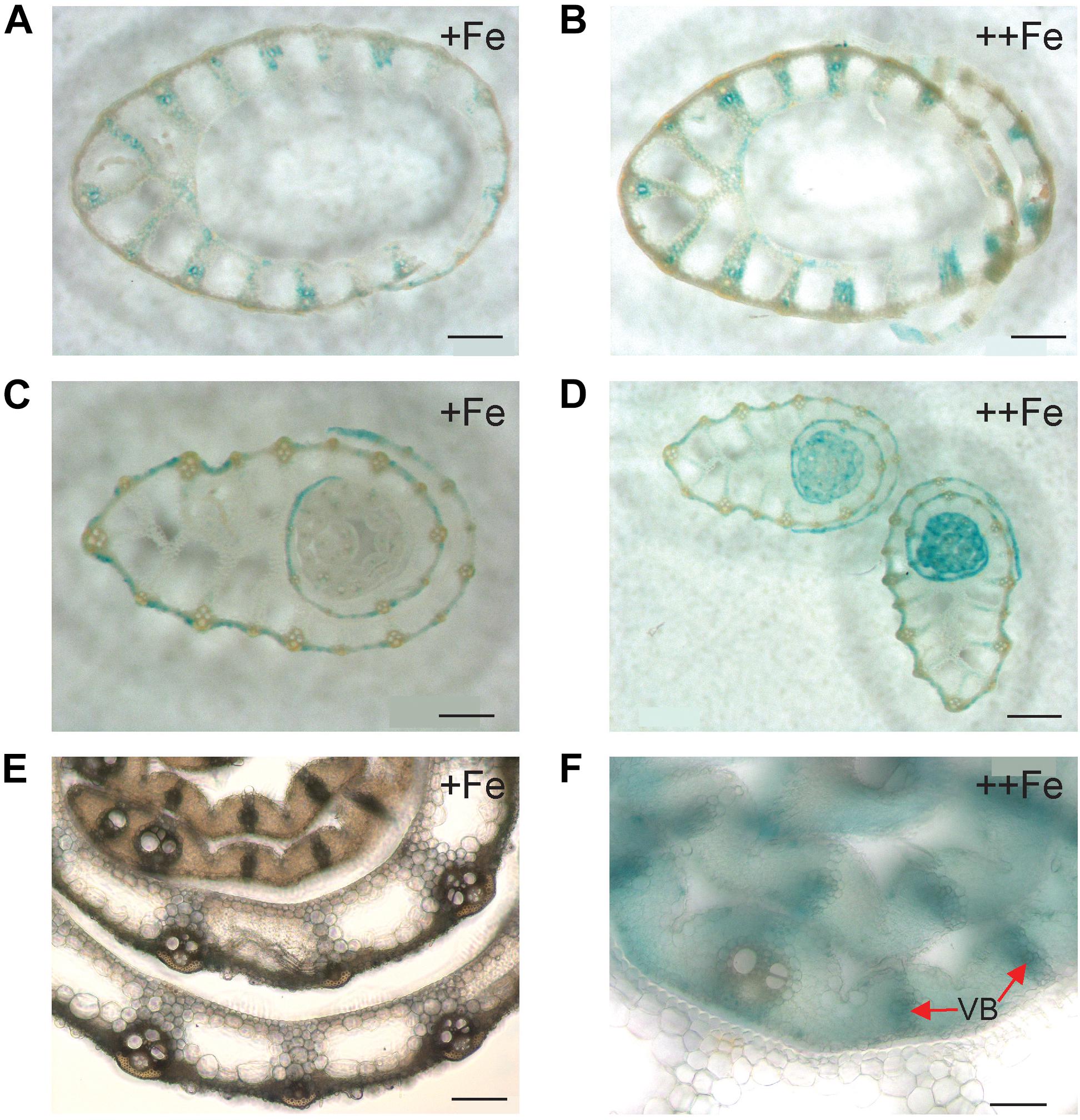
Figure 3. Cellular localization of OsNAS3 promoter-GUS expression in the stem. (A) The outer part of the control stem. (B) The outer part of the Fe-excess stem. (C) The inner part of the control stem. (D) The inner part of the Fe-excess stem. (E,F) Enlarged views of (C,D), respectively. VB, vascular bundle. Scale bars: 500 μm for (A–D); 20 μm for (E–F).
In the newest leaves, OsNAS3 expression patterns were not affected by Fe status (Figures 4A–D). Expression was localized to the small and large vascular bundles, bundle sheath cells, some parts of mesophyll cells and collenchyma fibers, and bulliform and epidermis cells of both the abaxial and adaxial surfaces (Figures 4A–D and Supplementary Figures S1C,D). More dense and dominant GUS activity was observed inside the large vascular bundles of leaves particularly in phloem companion cells under Fe excess than in control leaves. Bulliform cells, which only expressed OsNAS3 under Fe excess conditions in old leaves, expressed OsNAS3 in the newest leaves under both control and Fe excess conditions (Figures 4A,B). Bundle sheath cells, which did not express OsNAS3 in old leaves, expressed this gene dominantly in the newest leaves (Figures 4C,D). DCs constitutively exhibited strong OsNAS3 expression, particularly in the thick cortex, medullary cavity, and leaf sheath of the second leaf, whether Fe was sufficient or excessive (Figures 4E,F and Supplementary Figures S1E,F). Expression patterns were similar for DCs in the control and Fe excess conditions.
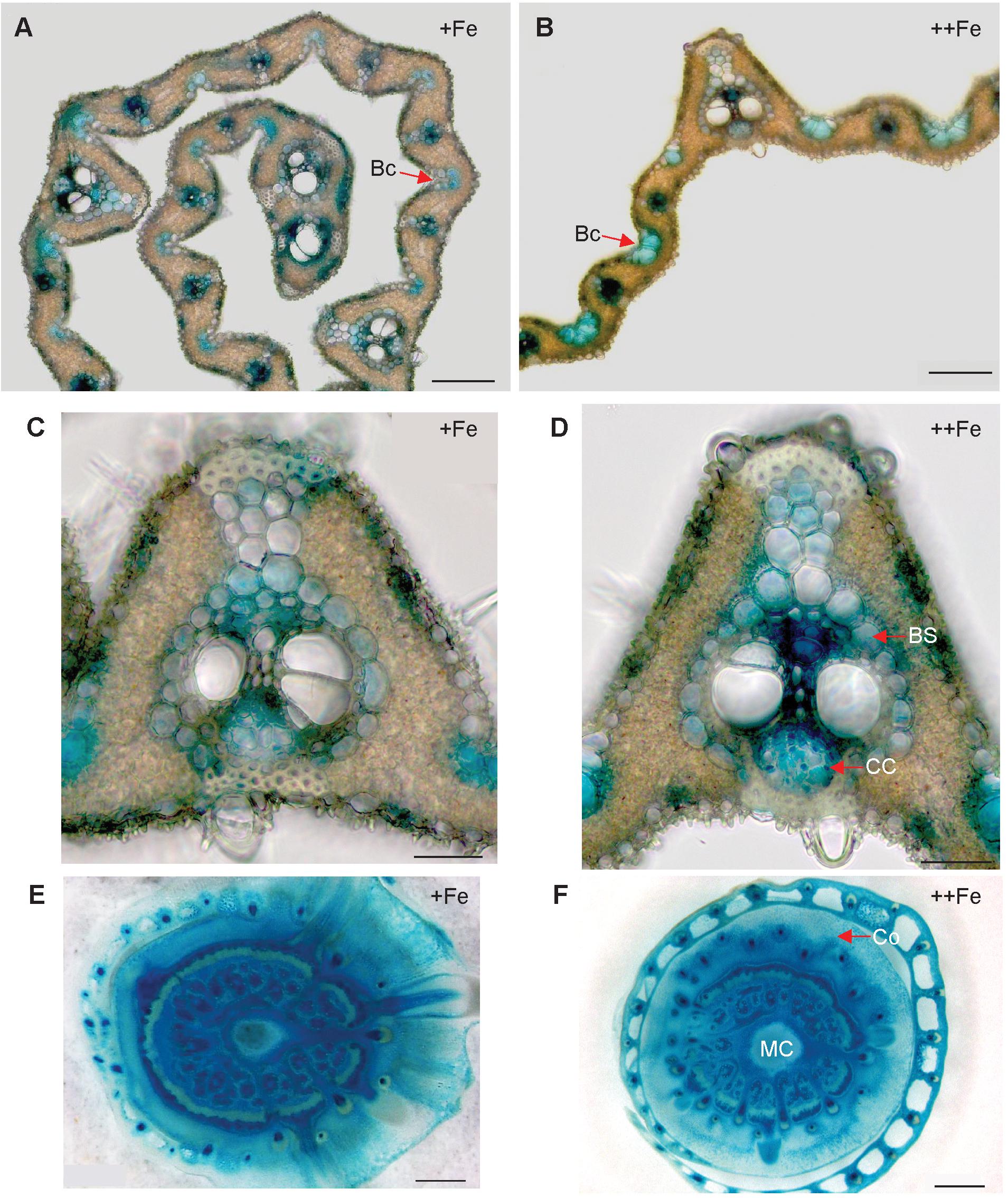
Figure 4. Cellular localization of OsNAS3 promoter-GUS expression in the newest leaves and DCs. (A) Transverse sections of the control newest leaf. (B) Fe-excess newest leaf. (C,D) Enlarged views of (A,B), respectively. (E) Control DC. (F) Fe-excess DC. Bc, bulliform cells; BS, bundle sheath cells; CC, companion cells; Co, cortex; MC, medullary cavity. Scale bars: 20 μm for (A–D); 500 μm for (E,F).
Expression of OsDMAS1 in Various Rice Tissues
To determine whether OsDMAS1 and OsNAAT1 are involved in DMA synthesis in rice exposed to excess Fe, we analyzed the expression patterns of these genes in various tissues (Figure 5). In roots, OsDMAS1 expression was reduced but not completely suppressed under Fe excess compared to control conditions (Figure 5A). In aboveground tissues (i.e., DCs, stems, old leaves and newest leaves), the expression of OsDMAS1 was similar under both control and Fe excess conditions, or higher under excess Fe conditions (Figures 5B–E). We also measured OsNAAT1 expression, which was reduced in roots, but not completely suppressed. Its levels remained similar or increased with Fe excess in DC, old leaf and newest leaf tissues (Supplementary Figure S2).
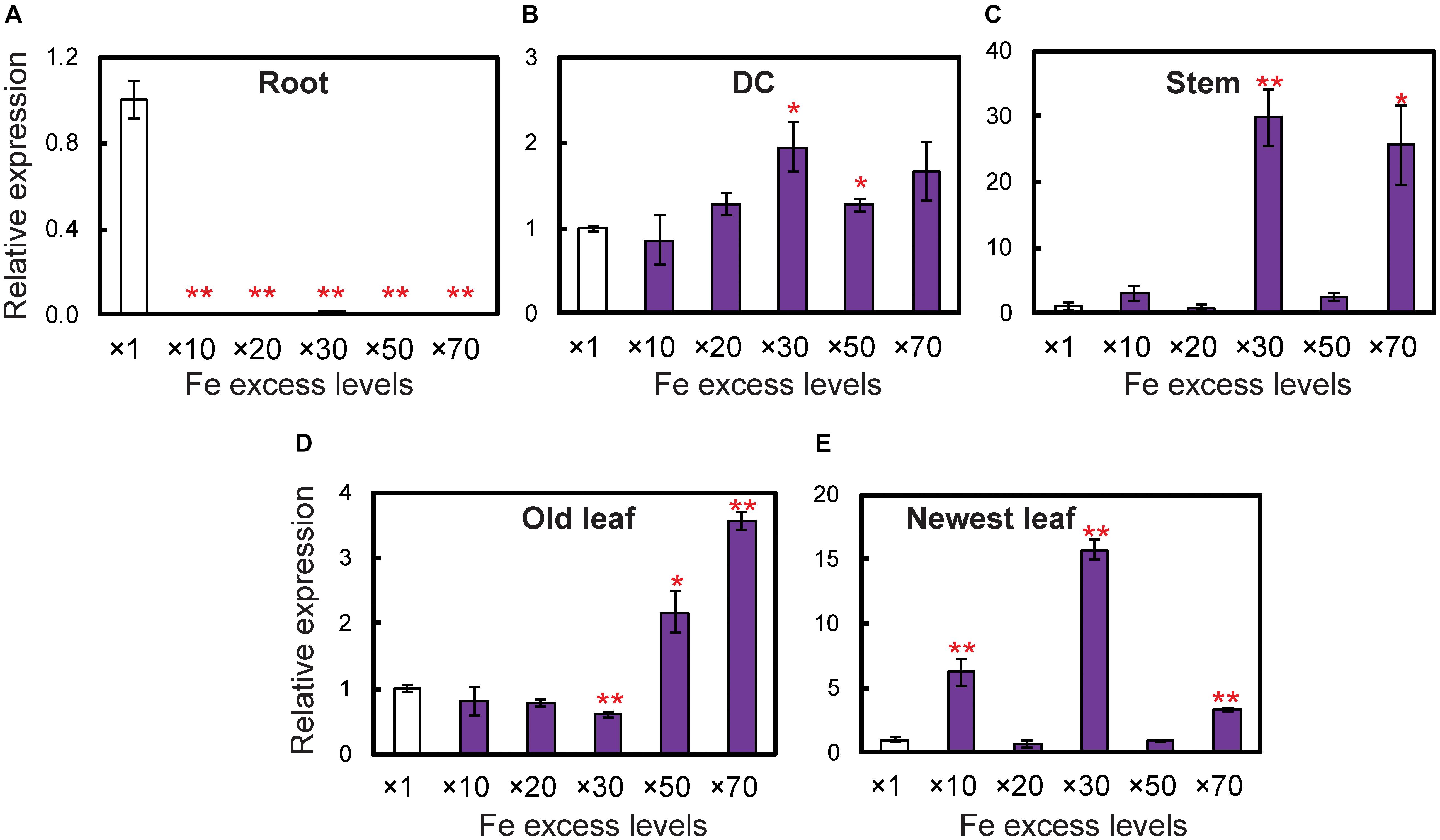
Figure 5. Expression levels of the OsDMAS1 gene in rice tissues under control and various Fe excess conditions. (A) Roots. (B) DCs. (C) Stems. (D) Old leaves. (E) Newest leaves. DC, discrimination center. This figure shows confirmation of the microarray results listed in Table 1 of Aung et al. (2018) from qPCR analyses. Error bars represent ± 1 standard error (SE) of the technical variation, n = 3. Data were normalized to the observed expression levels of alpha-tubulin and presented as relative gene expression in each tissue (×1 Fe = 1). Asterisks above the bars indicate significant differences (∗P < 0.05; ∗∗P < 0.01) compared to the control (×1 Fe).
Endogenous NA and DMA Are Present in Both Roots and Shoots With Excess Fe
Endogenous NA and DMA concentrations were analyzed in roots and shoots of NT rice grown under control and Fe excess conditions, and were present in both roots and shoots under excess Fe and control Fe conditions (Figure 6). Shoots had higher concentrations of NA and DMA than roots, and these concentrations did not significantly differ between control and Fe excess plants.
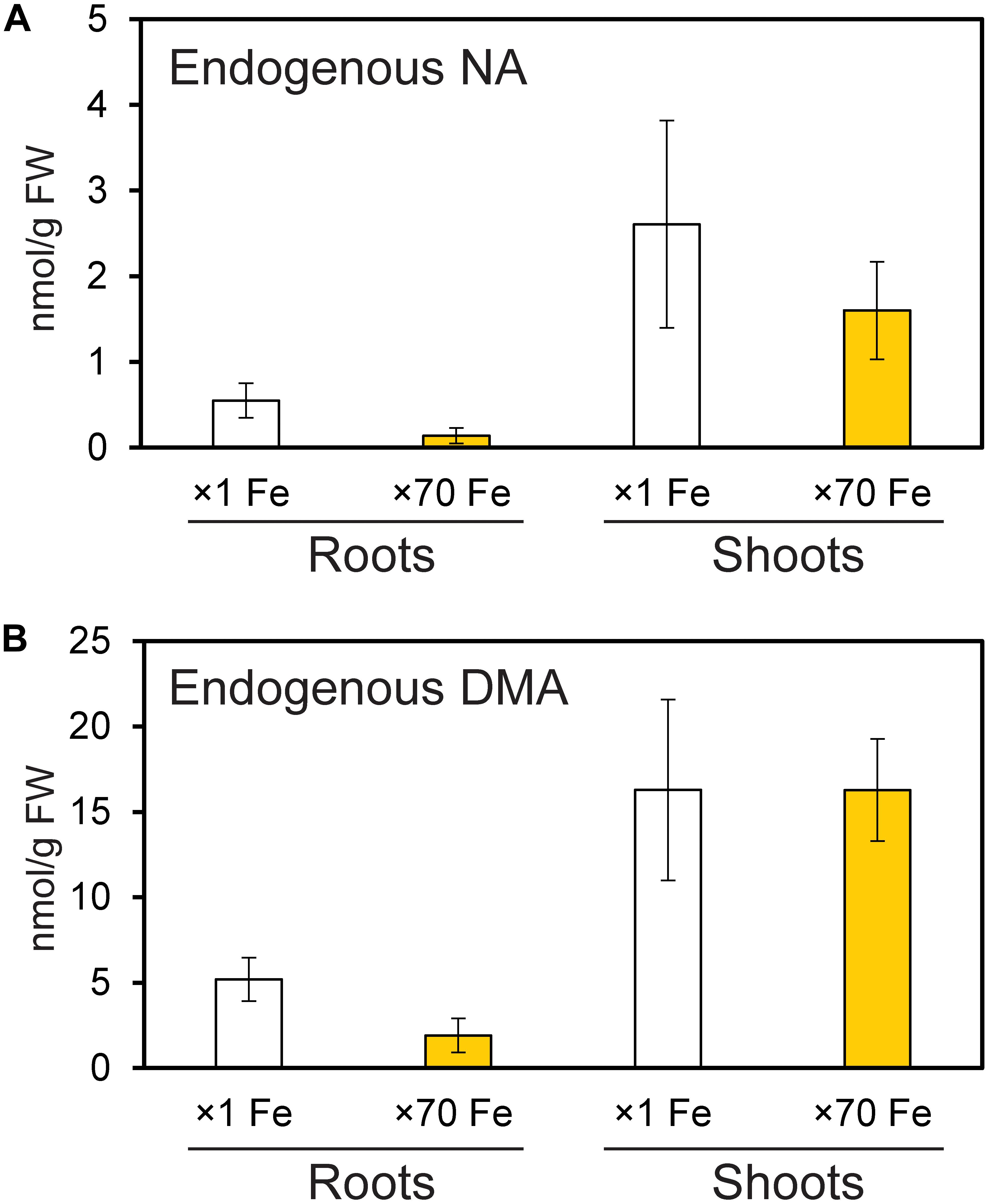
Figure 6. Amounts of endogenous nicotianamine (NA) and deoxymugineic acid (DMA) in the roots and shoots of control and Fe-excess rice. (A) The amount of endogenous NA and (B) the amount of endogenous DMA in roots and shoots of non-transformant rice under control and Fe excess conditions. Plants were grown hydroponically under control (×1 Fe) and excess ferrous Fe (×70 Fe) conditions at pH 4.0 for 14 days. Error bars represent ± 1 SE of three biological replicates. There were no significant differences between × 1 Fe and × 70 Fe in roots or shoots in (A,B), as determined using a t-test (∗P < 0.05; ∗∗P < 0.01).
The OsNAS3 Knockout Plants Are Weaker Under Excess Fe
The OsNAS3 knockout mutant line described by Lee et al. (2009) was used to investigate the response to Fe excess. The knockout plants and NT were grown in hydroponic cultures with control and excess Fe conditions. We also measured OsNAS3 expression in knockout plants under control and excess Fe conditions (Supplementary Figure S3). Under excess Fe supply, all knockout plants showed growth defects compared to NT plants (Figures 7A,B and Supplementary Figure S4). The NT plants under Fe-excess set panicles likewise under control Fe conditions but the knockout plants under Fe excess did not (Figures 7A,B). Shoot and root growth were significantly suppressed in knockout plants compared to NT under both control and excess Fe conditions (Figures 7C,D). Continuous growth was observed in both shoots and roots of all plants under control conditions (Figures 7C,E). Inferior shoot growth became pronounced in knockout plants with excess Fe after 7 days, and growth was stunted after 27 days, while root growth retardation occurred earlier, at 5 days after excess Fe exposure (Figures 7D,F). In the second experiment, the dry weight of roots and shoots were also measured. The shoot dry weights of the knockout plants were similar with NT under control condition, but reduced 36% in excess Fe compared with NT (Figure 7G). The root dry weights of the knockout plants reduced 20% in control condition and 46% in Fe excess condition compared to those of NT (Figure 7H). In this experiment, rather than the growth defect, the decrease in dry weight and the leaf bronzing caused by Fe excess damage in knockout plants were more pronounced. Bronzing symptoms on leaves were observed and bronzing scores were recorded for control and Fe-excess NT and OsNAS3 knockout plants. All leaves were healthy in both NT and knockout plants under control Fe conditions (Figure 8A). By contrast, OsNAS3 knockout plants showed serious leaf bronzing, particularly in older leaves, with excess Fe compared to NT plants (Figure 8A). Higher bronzing scores in all leaves of knockout plants (first to sixth newest leaves) were observed compared to those of NT plants exposed to excess Fe (Figure 8B).
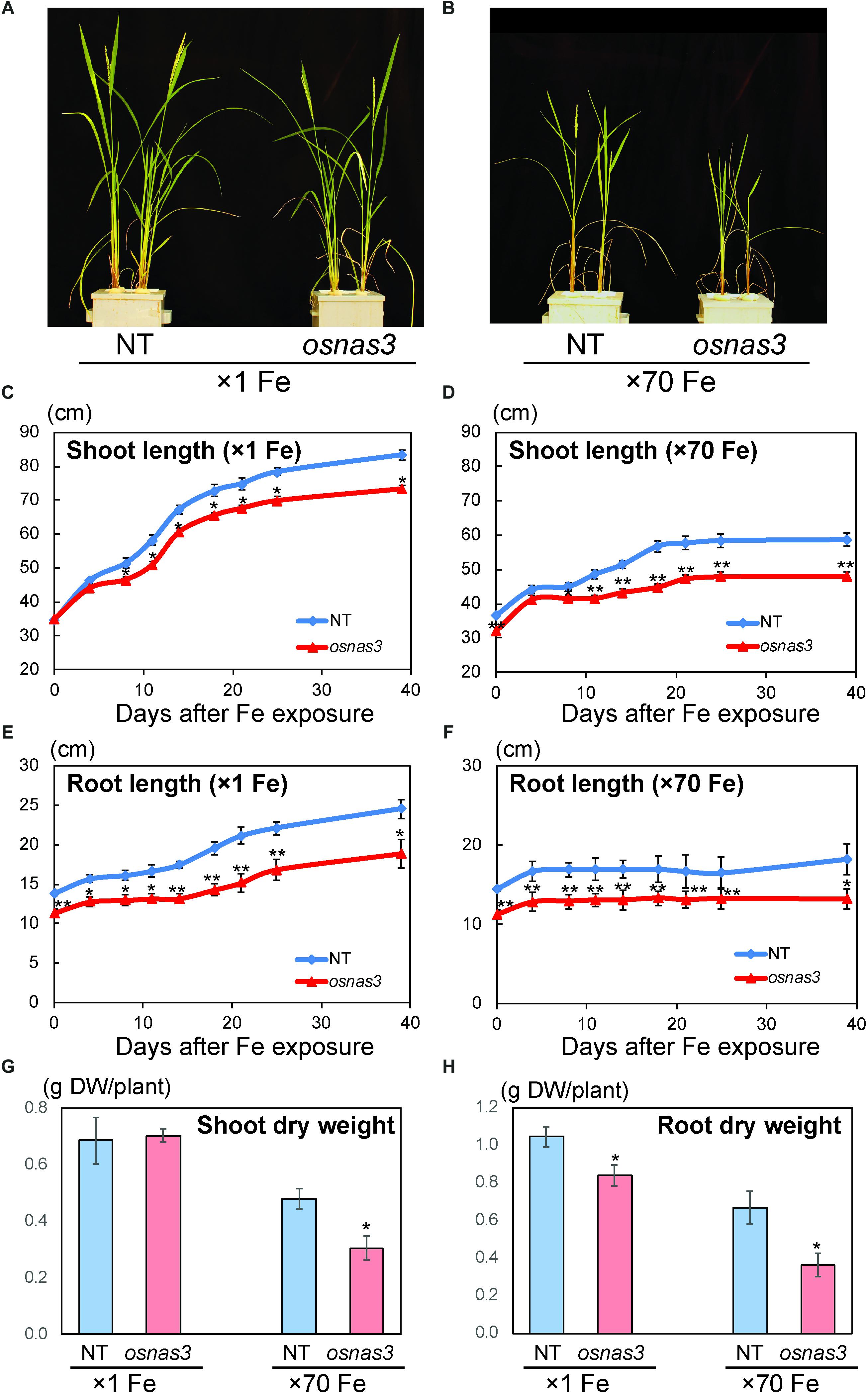
Figure 7. Plant appearance and growth of NT and OsNAS3 knockout rice. (A,B) Appearance of OsNAS3 knockout plants and non-transformants (NT) under control (A) or Fe excess (B) conditions after 39-day treatment. (C,D) Shoot length under control (C) or Fe excess (D) conditions. (E,F) Root length under control (E) or Fe excess (F) conditions. (G,H) Dry weights of shoots (G) and roots (H) under control and Fe excess condition at 23 days after transplanting (from 2nd experiment). Error bars represent the standard error (SE) of biological replicates, n = 4 for NT and n = 3 for knockout plants. Plants were grown hydroponically under control (×1 Fe) or excess ferrous Fe (×70 Fe) conditions at pH 4.0. Asterisks indicate significant differences compared to the NT at each time point or each Fe condition (∗P < 0.05, ∗∗P < 0.01).
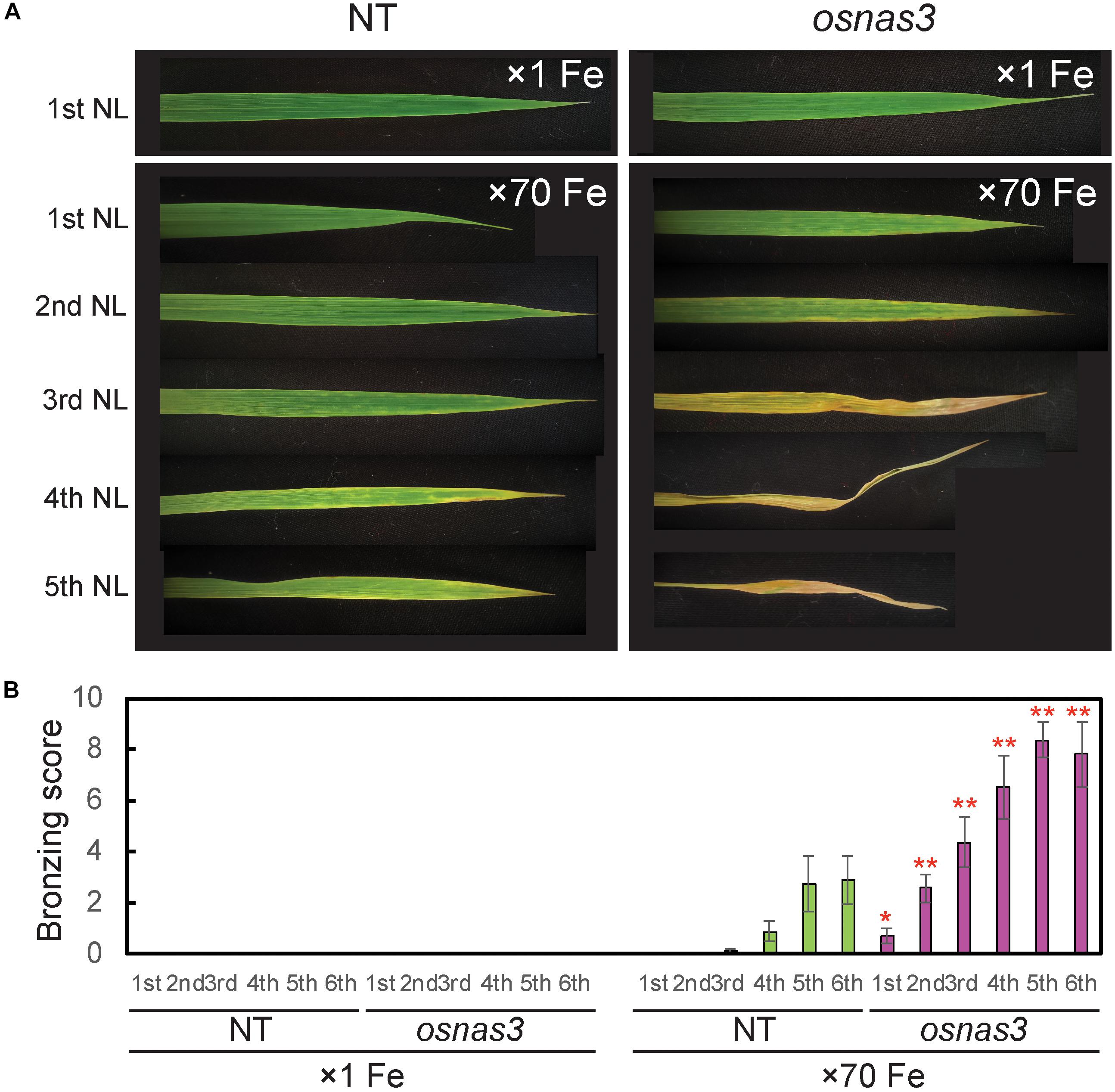
Figure 8. Leaf appearance and leaf bronzing scores of NT and OsNAS3 knockout rice. (A) Bronzing symptoms on representative leaves after 16 days of control or excess Fe stress treatment. The first, second, third, fourth, and fifth newest leaves (NL) are shown. (B) Bronzing scores after 17 days of Fe exposure. The first, second, third, fourth, fifth and six newest leaves are indicated on the horizontal axis as 1st, 2nd, 3rd, 4th, 5th, and 6th, respectively. Error bars represent the SE of biological replicates, n = 8. Plants were grown hydroponically under control (×1 Fe) and excess ferrous Fe (×70 Fe) conditions at pH 4.0. Asterisks indicate significant differences compared to the NT for each Fe condition and plant part (∗P < 0.05, ∗∗P < 0.01).
We also measured metal concentrations in the newest leaves, third newest leaves (older leaves), and whole roots of OsNAS3 knockout mutants and NT plants grown for 23 days under either control or Fe excess conditions (Figure 9). With excess Fe, both NT and knockout plants showed higher Fe accumulation in the newest leaves, which was even higher in older leaves, compared to the control condition (Figure 9A). In particular, OsNAS3 knockout plants had significantly higher Fe accumulation in both the newest (3 times higher) and older leaves (2 times higher) compared to the NT under excess Fe, while there were no differences from the NT in the control Fe treatment (Figure 9A). The Zn and Cu concentration trends did not differ between knockouts and NT under control conditions, but these trends were altered with excess Fe (Figures 9B,C). Both NT and knockout plants had higher Zn and lower Cu concentrations in the newest leaves than in older leaves. The concentrations of these metals were similar between the newest and older leaves of knockout plants with excess Fe (Figures 9B,C). Under excess Fe, knockout plants tended to have lower Zn in the newest leaves and lower Cu in older leaves compared to NT (Figures 9B,C). Mn accumulation was greater in older leaves than the newest leaves in the control condition, and its accumulation decreased with excess Fe in both NT and knockout plants (Figure 9D). Under excess Fe conditions, knockout plants tended to have higher Mn accumulation than NT plants, particularly in older leaves. The concentrations of Fe in roots were elevated in both knockouts and NT plants grown under excess Fe compared to control treatments, but there were no significant differences between knockout mutants and NT plants (Figure 9E). In roots, plants treated with excess Fe had lower concentrations of Zn, Cu, and Mn (Figures 9F–H). These metal accumulations were unaltered between NT and knockout plants, regardless of Fe status (Figures 9F–H). To evaluate how Fe is localized in leaf tissues of knockout plants, we observed Fe histochemical localization as deep blue staining using the Prussian blue staining method. Under control Fe conditions, both NT and knockout plants showed low Fe contents in leaves (Figures 10A,B). Iron staining was observed with Fe excess in both first and third newest leaves of NT plants (Figures 10C,E). Iron staining was more prominent in the newest leaves and in older leaves of Fe-excess knockout plants that exhibited serious leaf bronzing than in NT leaves (Figures 10D,F).
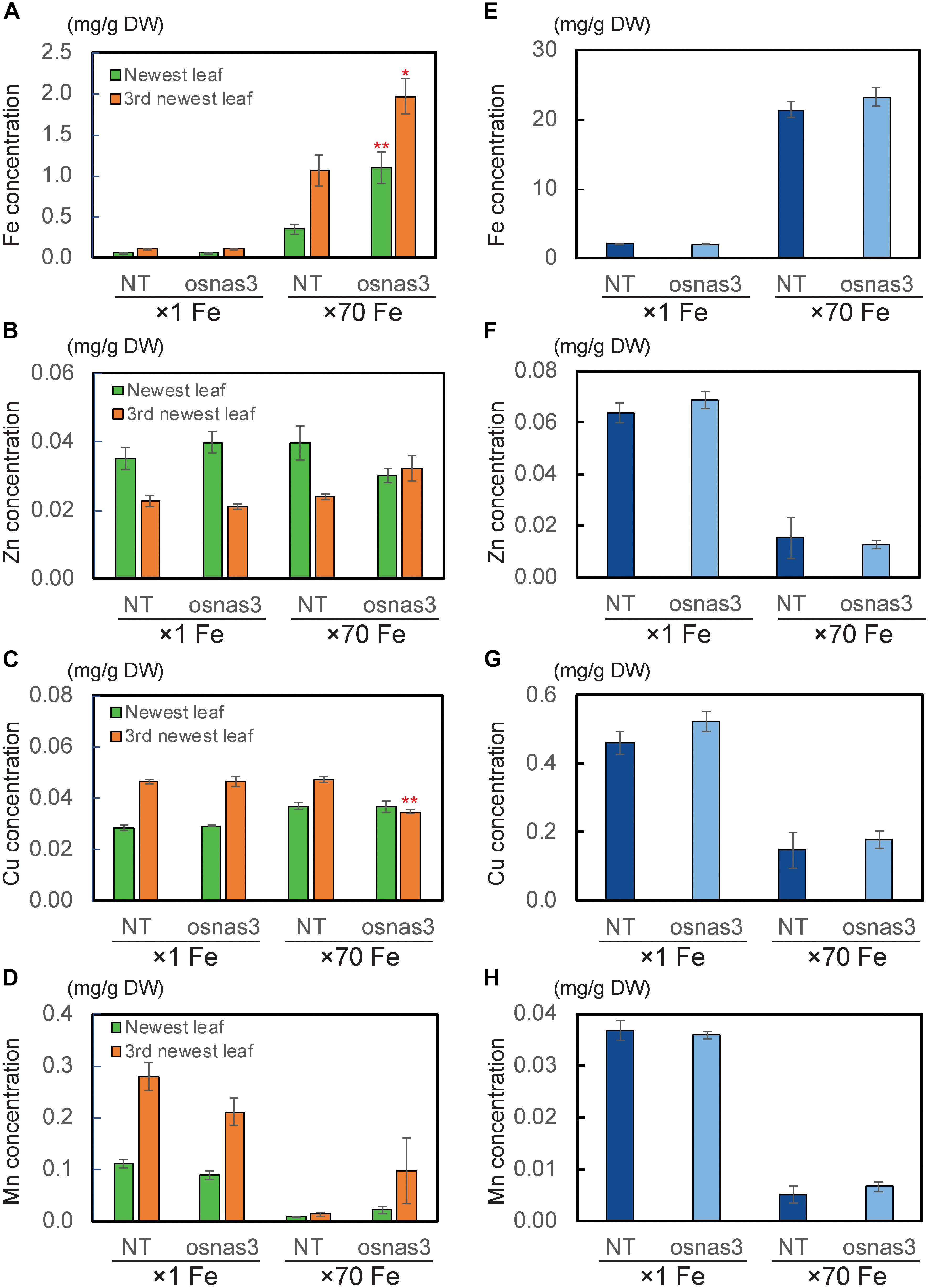
Figure 9. The metal concentrations in NT and OsNAS3 knockout plants under control and Fe excess conditions. (A) Fe, (B) Zn, (C) Cu and (D) Mn concentrations in leaves. (E) Fe, (F) Zn, (G) Cu and (H) Mn concentrations in roots. Plants were grown hydroponically under control (×1 Fe) and excess ferrous Fe (×70 Fe) conditions at pH 4.0 for 23 days. Green and orange bars indicate concentrations in the newest and third newest leaves, respectively. Error bars represent ± 1 SE from 3 biological replicates. Asterisks above the bars indicate significant differences compared to NT for each condition and plant part (∗P < 0.05; ∗∗P < 0.01).
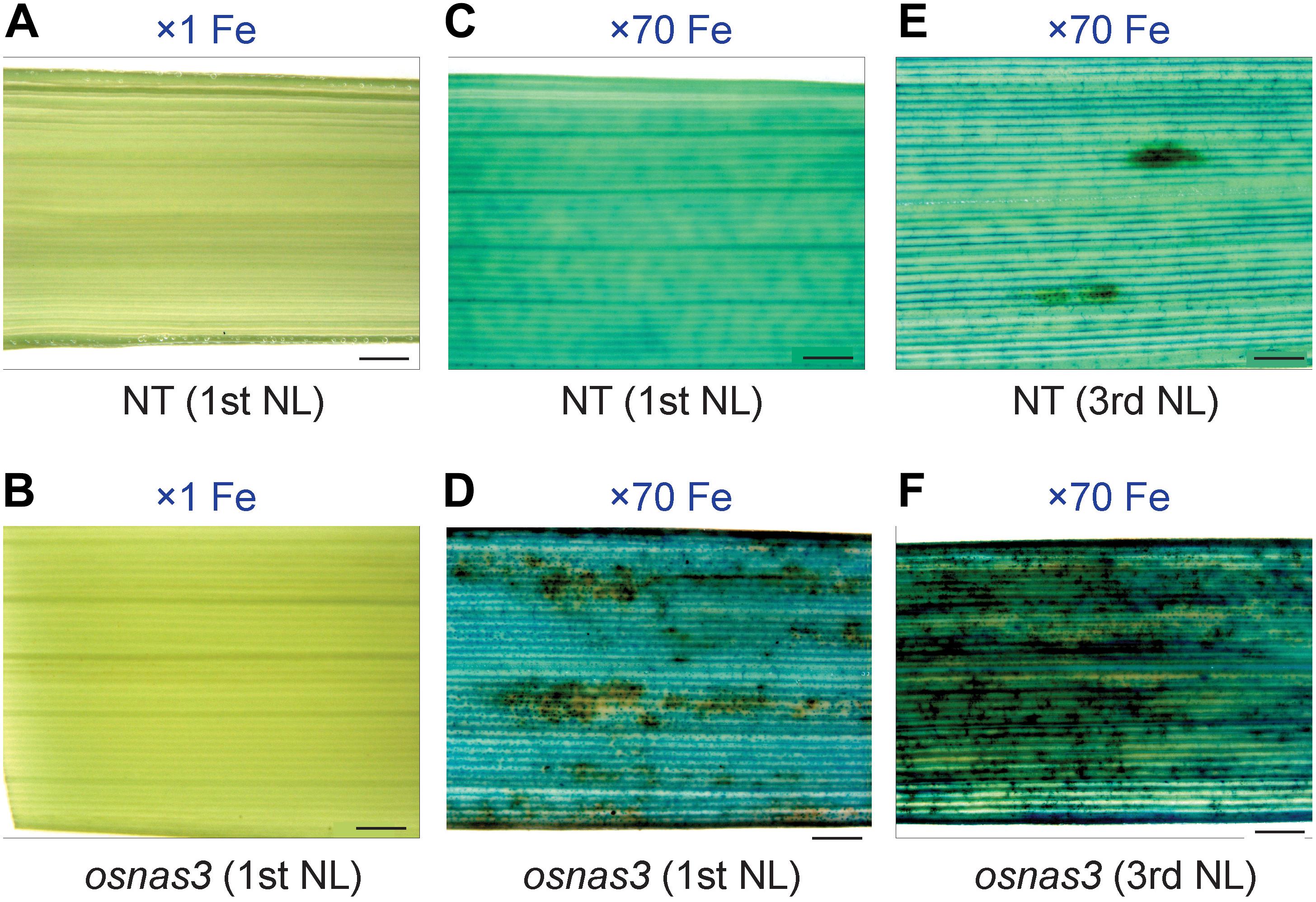
Figure 10. Histochemical Fe localization in leaves of NT and OsNAS3 knockout rice determined through Prussian blue staining. (A) First newest leaf of NT and (B) first newest leaf of knockout plant under control Fe conditions. (C) First newest leaf of NT, (D) first newest leaf of knockout plant, (E) third newest leaf of NT and (F) third newest leaf of knockout plant under Fe excess conditions. NL, newest leaf. Plants were grown hydroponically under control (×1 Fe) and excess ferrous Fe (× 70 Fe) conditions at pH 4.0 for 23 days. Scale bars: 500 μm.
Transgenic Line With Elevated NA Production Was More Tolerant to Excess Fe
To further examine the role of NA in the response to excess Fe in rice, a transgenic rice line with 15-fold elevated NA production in shoot tissues via induced expression of barley HvNAS1 (Masuda et al., 2009) was cultivated under both control and Fe excess conditions, and its growth and morphological characteristics were observed. Under excess Fe, the high-NA line maintained its growth and remained healthy throughout cultivation for 39 days, showing increased tolerance to excess Fe compared to NT plants, and similar growth to NT plants under control conditions (Supplementary Figure S5).
Discussion
Expression of OsNAS3 Is Investigated in Various Tissues Under Fe Excess Conditions
The localization of OsNAS3 expression was investigated using promoter-GUS analyses to determine the physiological role of this gene under Fe excess conditions. The strong expression of OsNAS3 was observed throughout Fe-excess root cells compared to control roots, suggesting that OsNAS3 functions deep within roots in the presence of excess Fe. Inoue et al. (2003) showed that OsNAS3 expression was slightly induced in roots under Fe deficiency. Thus, it is conceivable that OsNAS3 preferentially works under stress conditions: it may function in roots weakly under Fe deficiency and strongly under excess Fe conditions.
Under excess Fe, OsNAS3 expression was particularly dominant in exodermis, epidermis and vascular bundles (Figure 1), especially, extremely strong activity was observed in phloem cells, phloem companion cells, protoxylem, xylem parenchyma cells, and epidermal cells (Figures 1D,E). These results suggest that NA synthesis due to OsNAS3 may be involved in the radial movement of Fe to vascular bundle cells, as well as chelating excess Fe in roots and enhancing xylem and phloem loading.
Inoue et al. (2003) reported that under Fe deficiency, OsNAS3 expression was restricted to the central cylinder cells and did not extend to all root cells, suggesting that unlike OsNAS1 and OsNAS2, OsNAS3 does not contribute to the enhanced secretion of DMA from Fe-deficient roots. However, DMA accumulation was detected in the xylem sap of Fe-sufficient rice and under control Fe conditions (Kawai et al., 2001; Kakei et al., 2009). Our results also showed DMA accumulation in both control and Fe excess roots (Figures 5, 6). Expression of OsNAAT1 and OsDMS1 was observed in both control and excess Fe roots (Figure 5A and Supplementary Figure S2A), suggesting that NA is further converted into DMA in these cells under control and excess Fe conditions and that not only NA but also DMA may participate in Fe detoxification.
Stronger expression of OsNAS3 was observed in Fe-excess old leaves compared to control Fe leaves (Figure 2). Under control Fe conditions, OsNAS3 expression was restricted to phloem cells inside the large and small vascular bundles of old leaves (Figures 2A,C,E). Strong induction of OsNAS3 expression in old leaves was also observed through microarray and qPCR analyses (Aung et al., 2018). The GUS analyses showed dominant expression in vascular bundles, but only weak expression throughout the leaves, particularly in mesophyll cells. This result might be due to Fe stress causing old leaves to turn bronze in color, associated with cell death due to Fe overload (Figures 2D,G–J and Supplementary Figure S1B). In this study, we applied strong Fe excess stress to plants (70 times the control level). Thus, an experiment using milder Fe excess stress may be more suitable for identifying differences in old leaves.
Expression of OsNAS3 was observed in the trichomes of old leaves under excess Fe (Figure 2H). The Fe toxicity induces oxidative stress, which in turn causes limitation of photosynthesis. A rice variety that is sensitive to Fe excess showed impairment in light energy partitioning and oxidative damage before the onset of visual symptoms (Pinto et al., 2016). Some plant species accumulate excess metals in trichomes, cuticles or epidermal common cells to avoid greater damage to photosynthetic machinery (Küpper et al., 2000; Robinson et al., 2003; Freeman et al., 2006). Presumably, NA synthesis or allocation in trichomes and epidermal cells may be responsible for protecting leaf photosynthesis during Fe excess stress. Trichomes and epidermal cells showed high levels of GUS activity in the newest leaves irrespective of Fe status in this study (data not shown). OsNAS3 expression was also frequently seen in bulliform (motor) cells in old leaves treated with excess Fe (Figure 2I). Bulliform cells act as an entrance for light into the mesophyll cells (Clayton and Renvoize, 1986) and participate in the folding of mature leaves to minimize water loss during drought as well as the expansion of young leaves rolled in the apex (Shields, 1951; Jane and Chiang, 1991). Similar OsNAS3 expression in bulliform cells was observed in Fe-deficient leaves, suggesting a possible role of metals bound to NA in the regulation of water volume in bulliform cells (Inoue et al., 2003). Our result provides further support for OsNAS3 expression in these cells being for young leaf expansion and protection against water loss induced by excess Fe stress.
Expression of OsNAS3 was observed in both vascular bundles and mesophyll cells in the newest green leaves of both Fe sufficient and excess conditions (Figures 4C,D and Supplementary Figures S1C,D). Inoue et al. (2003) showed GUS expression in Fe sufficient green leaf tissue. Nicotianamine produced by OsNAS3 in the newest leaves may chelate excess Fe to mitigate Fe excess damage to the newest leaves. Iron excess causes a deficiency of other metals, such as Zn (Aung et al., 2018). Thus, NA is thought to support to the transport of other minerals, including Zn, in these leaves. In the inner part of the stem, prospective new leaves folded inside stem tissues showed denser GUS staining under Fe excess conditions compared to the control (Figures 3C–F). These very young new leaves inside the inner part of the stem are prone to Fe exposure transported via phloem pathway, but less Fe exposure than the previously extended leaves where Fe is transported via xylem stream from Fe excess roots. Still, protection from Fe excess is thought to be very important for new leaves inside the stem. OsNAS3 is thought to work in the next set of leaves to emerge when they are folded inside the stem. Discrimination center (DC) is a region that includes the shoot meristem, node, and internode (Mori, 1998; Itoh et al., 2005) and plays an important role in mineral and metabolite transport in graminaceous plants. Iron and other minerals absorbed by the roots first accumulate in the DC and then are distributed to shoots (Tsukamoto et al., 2009). OsNAS3 is strongly induced in DC under Fe excess (Aung et al., 2018). In this study, the strong expression of OsNAS3 was localized in DC, regardless of Fe status (Figures 4E,F and Supplementary Figures S1E,F). Iron chelated with NA controlled by OsNAS3 in DC might be transported to the shoots through YSL transporters such as OsYSL17 and OsYSL18, which are induced under Fe excess in the roots and DC (Aung et al., 2018).
Endogenous NA and DMA Are Present in Both Roots and Shoots Under Excess Fe
Under both normal and limited Fe conditions, graminaceous plants produce NA, which is further converted into DMA to acquire Fe from the rhizosphere (Takagi, 1976; Marschner et al., 1986). Nicotianamine enhances Fe translocation within plants under both limited and normal Fe conditions (Takahashi et al., 2003). Our results confirmed the presence of NA in control roots (Figure 6). This NA was produced by OsNAS1 and OsNAS2 to enhance Fe translocation under normal condition. On the other hand, under Fe excess condition, plants might not need to produce further NA within the plant to take up more Fe. However, NA and DMA were present in roots and shoots under Fe excess (Figure 6). OsNAS3 was strongly induced in most rice tissues under Fe excess compared to the control (Aung et al., 2018). Thus, this NA might be produced by OsNAS3. Under normal Fe condition (x 1 Fe), rice plants should have efforts to take up and translocate Fe within the plant body. Thus, the OsNAS1 and OsNAS2 expression was high in roots under x1 Fe condition for the transport of Fe and other metals within the plant body, whereas their expression in roots was extremely low under ×70 Fe condition (Aung et al., 2018). Moreover, the produced NA in roots will be transported to shoot under normal Fe condition together with other metal transportation. Because of this reason, although NA productivity increased by OsNAS3 under Fe excess, total NA concentrations was the same or slightly reduced under Fe excess condition (Figure 6). These results clearly show that the roles and the functions of the NA between these two conditions are different. It is well understood in plant nutrition that NA is used for Fe translocation or a precursor of DMA for Fe uptake. Our finding is that NA and DMA still exist under Fe excess and there is a role of them under Fe excess. The expression of OsNAAT1 and OsDMAS1 in roots was reduced but not completely suppressed with excess Fe (Figure 5A and Supplementary Figure S2). Their expression levels were even higher under Fe excess in the DC, stem, old leaves, and newest leaves (Figures 5B–E and Supplementary Figure S2; Aung et al., 2018). Thus, DMA may also participate in Fe detoxification processes under Fe excess conditions. Free Fe causes cellular Fe toxicity because it serves as a catalyst in the formation of free radicals from reactive oxygen species via the Fenton reaction. But Fe chelated with NA does not undergo the Fenton reaction and can be safely sequestered and stored in ferritins or vacuoles within plant tissues. The Fe(II)-NA complexes are poor Fenton reagents, based on their ability to mediate H2O2-dependent oxidation of deoxyribose, suggesting that NA has a vital role in scavenging Fe and protecting the cell from Fe-induced oxidative damage (von Wirén et al., 1999). Thus, NA and DMA production by OsNAS3 under Fe excess conditions is to chelate free excess Fe and thus mitigate Fe excess stress.
Among NAS genes in rice, OsNAS3 belongs to Clade II, (Mizuno et al., 2003; Bonneau et al., 2016). Bonneau et al. (2016) reported that maize ZmNAS3, ZmNAS4, ZmNAS5, barley NASHOR2, wheat TaNAS9-A, TaNAS9-B, and TaNAS9-D are included in Clade II, and among these, wheat TaNAS9-A and TaNAS9-D have greater relative expression in roots under Fe sufficiency than Fe deficiency. These genes are closely related to OsNAS3 and they might operate under Fe excess in the same manner as OsNAS3. Expression of Clade II NAS genes decreased under Fe deficiency and was induced in roots and shoots under normal Fe condition. Thus, Clade II NAS proteins may not contribute to MA biosynthesis under Fe deficiency, but may be involved in NA biosynthesis to support Fe loading of vascular tissues and maintenance of cellular Fe homeostasis (Bonneau et al., 2016).
The OsNAS3 Knockout Plants Are Sensitive to Fe Excess
The OsNAS3 knockout plants resulted in impaired shoot and root growth than NT under both control and Fe excess conditions (Figure 7). The NT plants under Fe-excess set panicles likewise under control Fe condition but the knockout plants under Fe-excess did not set panicles (Figures 7A,B). Dry weights of the knockout plants reduced in both shoots and roots under excess Fe (Figures 7G,H). In this study, the leaf bronzing caused by Fe excess damage in knockout plants was more pronounced than the growth defect. Our results consistently indicated that the knockout line was more susceptible to Fe excess than NT plants in terms of leaf bronzing levels and Fe accumulation in the newest leaves and old leaves (third newest leaves) compared to NT plants (Figures 8, 9, 10). Disruption of OsNAS3 clearly enhanced Fe accumulation in leaves in response to excess Fe, but only slightly higher Fe accumulation occurred in the roots than in NT roots (Figures 9A,E). These results suggest that enhanced Fe toxicity in OsNAS3 knockout lines might be due to enhanced Fe translocation from roots to shoots. In fact, NA chelates excess Fe and supports its efficient translocation and sequestration, and hence the plant can reduce Fe translocation to shoots. However, high Fe accumulation was observed in Fe-excess shoots, showing that this knockout rice was unable to translocate excess Fe to the suitable tissues in roots. Iron uptake-related transporters such as OsIRT1, OsIRT2, OsNRAMP1, OsYSL2, and OsYSL15 were highly suppressed under Fe excess (Aung et al., 2018). Thus, this high level of Fe accumulation in shoots may be due to Fe uptake by other chelators or other mineral transporters, for example, Zn transporters, which can take up Fe as well as Zn. These results indicate that OsNAS3 plays an important role in mitigating Fe excess in rice.
In this study, the treatment applied was severe excess ferrous Fe stress (× 70 Fe; 2520 μM Fe2+) compared to the control in the hydroponic culture at low pH (pH 4.0). We also showed that the transgenic line with higher NA production showed tolerance to this severe level of Fe excess stress (Supplementary Figure S5). This line accumulated 15 times more NA and 3 times more DMA in its shoots (Masuda et al., 2009). These results suggest that increased NA and DMA biosynthesis might mitigate the damage from excess Fe in the plant.
Role of NA in Fe Excess and Zn Deficiency
Iron excess leads to Zn deficiency. The Zn concentration was decreased under Fe excess in roots of both NT plants and the OsNAS3 knockout line (Figure 9F). The concentrations of Zn decrease proportionally with the increase in Fe excess levels in roots (Aung et al., 2018). Preventing Fe uptake in response to Fe excess may reduce Zn uptake capacity. At the same time, expression of some putative Zn transporter genes such as OsZIP4, OsZIP5, OsZIP7, and OsZIP9 is strongly induced in Fe excess roots, suggesting that these putative Zn transporters may participate in enhanced Zn transport under Fe excess (Aung et al., 2018). Nicotianamine plays a vital role in intercellular and long-distance transport of Zn to maintain Zn homeostasis in plants (Clemens et al., 2013). The Zn concentration tended to decrease in new leaves and increased in old leaves in knockouts compared to NT plants under Fe excess conditions (Figure 9B). The knockout plant was also impaired in Zn translocation and distribution, suggesting that NA produced by OsNAS3 may be important to maintaining Zn levels in the newest leaves with excess Fe. Interestingly, expression of OsNAS3 increased in Zn-deficient roots and shoots, but not that of OsNAS1 or OsNAS2 (Suzuki et al., 2008). Under Zn deficiency, OsNAS3 expression is strongly induced in almost all tissues, by about 3 to 8 times (unpublished data). By contrast, under excess Zn, OsNAS3 was highly repressed in both roots and shoots, while OsNAS1 and OsNAS2 were highly induced (Ishimaru et al., 2008). In this study, in addition to NA, DMA was present in roots and shoots under Fe excess (Figure 6B). DMA increases Zn translocation in Zn-deficient rice (Suzuki et al., 2008). It indicates another important role of NA synthesized by OsNAS3 under excess Fe conditions, alleviating Zn deficiency in rice.
Conclusion
In this study, we provide evidence that OsNAS3 is functional and physiologically crucial under excess Fe, acting as an iron-excess induced gene. Our results suggest that NA and DMA synthesized by OsNAS3 under excess Fe conditions contribute to Fe detoxification in rice. Nicotianamine plays multiple important roles in Fe nutrition in plants, which can be applied to Fe detoxification as well as Fe deficiency tolerance and Fe biofortification. This finding will contribute to developing Fe toxicity tolerant rice for growth in acidic paddy fields, which has the potential for improving rice yield to feed the increasing global population.
Author Contributions
MSA, HM, and NKN designed, led, and coordinated the overall study. MSA performed most of the experiments, analyzed the results, and wrote the manuscript with assistance from HM. TN analyzed the NA and DMA concentrations. GA and J-SJ provided OsNAS3 knockout seeds. HM, TK, and NKN discussed the results and improved the manuscript.
Funding
This research was supported by the Japan Society for the Promotion of Sciences (JSPS) Fellowship Program for Overseas Researchers, MSA (ID No. P04079) and (JSPS KAKENHI Grant No. 14F04079) host researcher NKN, Grant-in-Aid for Young Scientists (JSPS KAKENHI Grant No. 18K14367) to MSA, and the Advanced Low Carbon Technology Research and Development Program (ALCA) of the Japan Science and Technology Agency (Grant No. JPMJAL1107) to NKN.
Conflict of Interest Statement
The authors declare that the research was conducted in the absence of any commercial or financial relationships that could be construed as a potential conflict of interest.
Acknowledgments
We thank Dr. Haruhiro Inoue from the National Agriculture and Food Research Organization (NARO) and Dr. Reiko Nakanishi Itai from The University of Tokyo for providing OsNAS3 promoter-GUS seeds. We thank Dr. Sichul Lee for providing seeds of the OsNAS3 knockout mutant. We also thank the following individuals from Ishikawa Prefectural University: Dr. Tatsuro Hamada for support of research facilities, and Ms. Yukiko Sato, Ms. Reiko Omote, and Ms. Emiko Nakanishi for research assistance.
Supplementary Material
The Supplementary Material for this article can be found online at: https://www.frontiersin.org/articles/10.3389/fpls.2019.00660/full#supplementary-material
References
Aung, M. S., Masuda, H., Kobayashi, T., Nakanishi, H., Yamakawa, T., and Nishizawa, N. K. (2013). Iron biofortification of myanmar rice. Front. Plant Sci. 4:158. doi: 10.3389/fpls.2013.00158
Aung, M. S., Masuda, H., Kobayashi, T., and Nishizawa, N. K. (2018). Physiological and transcriptomic analysis of responses to different levels of iron excess stress in various rice tissues. Soil Sci. Plant Nutr. 64, 370–385. doi: 10.1080/00380768.2018.1443754
Bashir, K., Inoue, H., Nagasaka, S., Takahashi, M., Nakanishi, H., Mori, S., et al. (2006). Cloning and characterization of deoxymugineic acid synthase genes from graminaceous plants. J. Biol. Chem. 281, 32395–32402. doi: 10.1074/jbc.M604133200
Bonneau, J., Baumann, U., Beasley, J., Li, Y., and Johnson, A. A. T. (2016). Identification and molecular characterization of the nicotianamine synthase gene family in bread wheat plant. Biotech. J. 14, 2228–2239. doi: 10.1111/pbi.12577
Boonyaves, K., Wu, T.-Y., Gruissem, W., and Bhullar, N. K. (2017). Enhanced grain iron levels in rice expressing an iron-regulated metal transporter, nicotianamine synthase, and ferritin gene cassette. Front. Plant Sci. 8:130. doi: 10.3389/fpls.2017.00130
Clayton, W. D., and Renvoize, S. A. (1986). Genera Graminum – Grasses of the World. Kew Bulletin Additional Series XIII. London: Her Majestic Stationary Office.
Clemens, S., Deinlein, U., Ahmadi, H., Horeth, S., and Uraguchi, S. (2013). Nicotianamine is a major player in plant Zn homeostasis. Biometals 26, 623–632. doi: 10.1007/s10534-013-9643-1
Douchkov, D., Gryczka, C., Stephan, U. W., Hell, R., and Baumlein, H. (2005). Ectopic expression of nicotianamine synthase genes results in improved iron accumulation and increased nickel tolerance in transgenic tobacco. Plant Cell Environ. 28, 365–374. doi: 10.1111/j.1365-3040.2005.01273.x
Freeman, J. L., Zhang, L. H., Marcus, M. A., Fakra, S., McGrath, S. P., and Pilon-Smits, E. A. H. (2006). Spatial imaging, speciation, and quantification of selenium in the hyperaccumulator plants Astragalus bisulcatus and Stanleya pinnata. Plant Physiol. 142, 124–134. doi: 10.1104/pp.106.081158
Han, D., Zhang, Z., Ni, B., Ding, H., Liu, W., Li, W., et al. (2018). Isolation and functional analysis of MxNAS3 involved in enhanced iron stress tolerance and abnormal flower in transgenic Arabidopsis. J Plant Int. 13, 433–441. doi: 10.1080/17429145.2018.1499145
Han, D. G., Yang, G. H., Xu, K. D., Shao, Q., Yu, Z. Y., Wang, B., et al. (2013). Overexpression of a malus xiaojinensis Nas1 gene influences flower development and tolerance to iron stress in transgenic tobacco. Plant Mol. Biol. Rep. 31, 802–809. doi: 10.1007/s11105-012-0551-2
Hell, R., and Stephan, U. W. (2003). Iron uptake, trafficking and homeostasis in plants. Planta 216, 541–551.
Higuchi, K., Kanazawa, K., Nishizawa, N. K., Chino, M., and Mori, S. (1994). Purification and characterization of nicotianamine synthase from Fe- deficient barley roots. Plant Soil 165, 173–179. doi: 10.1007/bf00008059
Higuchi, K., Watanabe, S., Takahashi, M., Kawasaki, S., Nakanishi, H., Nishizawa, N. K., et al. (2001). Nicotianamine synthase gene expression differs in barley and rice under Fe-deficient conditions. Plant J. 25, 159–167. doi: 10.1111/j.1365-313x.2001.00951.x
Inoue, H., Higuchi, K., Takahashi, M., Nakanishi, H., Mori, S., and Nishizawa, N. K. (2003). Three rice nicotianamine synthase genes, OsNAS1, OsNAS2, and OsNAS3 are expressed in cells involved in long-distance transport of iron and differentially regulated by iron. Plant J. 36, 366–381. doi: 10.1046/j.1365-313X.2003.01878.x
Inoue, H., Takahashi, M., Kobayashi, T., Suzuki, M., Nakanishi, H., Mori, S., et al. (2008). Identification and localization of the rice nicotianamine aminotransferase gene OsNAAT1 expression suggests the site of phytosiderophore synthesis in rice. Plant Mol. Biol. 66, 193–203. doi: 10.1007/s11103-007-9262-8
Ishimaru, Y., Suzuki, M., Ogo, Y., Takahashi, M., Nakanishi, H., Mori, S., et al. (2008). Synthesis of nicotianamine and deoxymugineic acid is regulated by OsIRO2 in Zn excess rice plants. Soil Sci. Plant Nutr. 54, 417–423. doi: 10.1111/j.1747-0765.2008.00259.x
Itoh, J., Nonomura, K., Ikeda, K., Yamaki, S., Inukai, Y., Yamagishi, H., et al. (2005). Rice plant development: from zygote to spikelet. Plant Cell Physiol. 46, 23–47. doi: 10.1093/pcp/pci501
Jane, W. N., and Chiang, S. H. T. (1991). Morphology and development of bulliform cells in arundo formosana hack. Taiwania 36, 85–97.
Jeong, D. H., An, S., Kang, H. G., Moon, S., Han, J. J., Park, S., et al. (2002). T-DNA insertional mutagenesis for activation tagging in rice. Plant Physiol. 130, 1636–1644. doi: 10.1104/pp.014357
Johnson, A. A. T., Kyriacou, B., Callahan, D. L., Carruthers, L., Stangoulis, J., Lombi, E., et al. (2011). Constitutive overexpression of the OsNAS gene family reveals single gene strategies for effective iron- and zinc-biofortification of rice endosperm. PLoS One 6:e24476. doi: 10.1371/journal.pone.0024476
Kakei, Y., Yamaguchi, I., Kobayashi, T., Takahashi, M., Nakanishi, H., Yamakawa, T., et al. (2009). A highly sensitive, quick and simple quantification method for nicotianamine and 2′-deoxymugineic acid from minimum samples using LC/ESI-TOF-MS achieves functional analysis of these components in plants. Plant Cell Physiol. 50, 1988–1993. doi: 10.1093/pcp/pcp141
Kawai, S., Kamei, S., Matsuda, Y., Ando, R., Kondo, S., Ishizawa, A., et al. (2001). Concentrations of iron and phytosiderophores in xylem sap of iron-deficient barley plants. Soil Sci. Plant Nutr. 47, 265–272. doi: 10.1080/00380768.2001.10408390
Kim, S., Takahashi, M., Higuchi, K., Tsunoda, K., Nakanishi, H., Yoshimura, E., et al. (2005). Increased nicotianamine biosynthesis confers enhanced tolerance of high levels of metals, in particular nickel, to plants. Plant Cell Physiol. 46, 1809–1818. doi: 10.1093/pcp/pci196
Klatte, M., Schuler, M., Wirtz, M., Fink-Straube, C., Hell, R., and Bauer, P. (2009). The analysis of arabidopsis nicotianamine synthase mutants reveals functions for nicotianamine in seed iron loading and iron deficiency responses. Plant Physiol. 150, 257–271. doi: 10.1104/pp.109.136374
Kobayashi, T., Itai, R. N., and Nishizawa, N. K. (2014). Iron deficiency responses in rice roots. Rice 7:27. doi: 10.1186/s12284-014-0027-0
Kobayashi, T., Ogo, Y., Aung, M. S., Nozoye, T., Itai, R. N., Nakanishi, H., et al. (2010). The spatial expression and regulation of transcription factors IDEF1 and IDEF2. Ann. Bot. 105, 1109–1117. doi: 10.1093/aob/mcq002
Küpper, H., Lombi, E., Zhao, F. J., and McGrath, S. P. (2000). Cellular compartmentation of cadmium and zinc in relation to other elements in the hyperaccumulator Arabidopsis halleri. Planta 212, 75–84. doi: 10.1007/s004250000366
Lee, S., Jeon, U. S., Lee, S. J., Kim, Y. K., Persson, D. P., Husted, S., et al. (2009). Iron fortification of rice seeds through activation of the nicotianamine synthase gene. Proc. Natl. Acad. Sci. U.S.A. 106, 22014–22019. doi: 10.1073/pnas.0910950106
Marschner, H., and Romheld, V. (1994). Strategies of plants for acquisition of iron. Plant Soil 165, 261–274. doi: 10.1007/bf00008069
Marschner, H., Romheld, V., and Kissel, M. (1986). Different strategies in higherplants in mobilization and uptake of iron. J. Plant Nutr. 9, 695–713. doi: 10.1080/01904168609363475
Masuda, H., Ishimaru, Y., Aung, M. S., Kobayashi, T., Kakei, Y., Takahashi, M., et al. (2012). Iron biofortification in rice by the introduction of multiple genes involved in iron nutrition. Sci. Rep. 2:543. doi: 10.1038/srep00543
Masuda, H., Usuda, K., Kobayashi, T., Ishimaru, Y., Kakei, Y., Takahashi, M., et al. (2009). Overexpression of the barley nicotianamine synthase gene HvNAS1 increase iron and zinc concentrations in rice grains. Rice 2, 155–166. doi: 10.1007/s12284-009-9031-1
Mizuno, D., Higuchi, K., Sakamoto, T., Nakanishi, H., Mori, S., and Nishizawa, N. K. (2003). Three nicotianamine synthase genes isolated from maize are differentially regulated by iron nutritional status. Plant Physiol. 132, 1989–1997. doi: 10.1104/pp.102.019869
Mori, S. (1998). “Iron transport in graminaceous plants,” in Iron Transport and Storage in Microorganisms, Plants and Animals. Vol. 35, Metal Ions in Biological Systems, eds A. Sigel and H. Sigel (New York, NY: Marcel Dekker), 215–237.
Mori, S., and Nishizawa, N. (1987). Methionine as a dominant precursor of phytosiderophores in graminaceae plants. Plant Cell Physiol. 28, 1081–1092.
Murashige, T., and Skoog, F. (1962). A revised medium for rapid growth and bioassay with tobacco tissue cultures. Physiol Plant. 15, 473–497. doi: 10.1111/j.1399-3054.1962.tb08052.x
Nozoye, T., Nagasaka, S., Bashir, K., Takahashi, M., Kobayashi, T., Nakanishi, H., et al. (2014). Nicotianamine synthase 2 localizes to the vesicles of iron-deficient rice roots, and its mutation in (the) YXXphi or LL motif causes the disruption of vesicle formation or movement in rice. Plant J. 77, 246–260. doi: 10.1111/tpj.12383
Pich, A., Manteuffel, R., Hillmer, S., Scholz, G., and Schmidt, W. (2001). Fe homeostasis in plant cells: does nicotianamine play multiple roles in the regulation of cytoplasmic Fe concentration? Planta 213, 967–976. doi: 10.1007/s004250100573
Pich, A., and Scholz, G. (1996). Translocation of copper and other micronutrients in tomato plants (Lycopersicon esculentum Mill.): nicotianamine-stimulated copper transport in the xylem. J. Exp. Bot. 47, 41–47. doi: 10.1093/jxb/47.1.41
Pinto, S. D. S., de Souza, A. E., Oliva, M. A., and Pereira, E. G. (2016). Oxidative damage and photosynthetic impairment in tropical rice cultivars upon exposure to excess iron. Sci. Agricola 73, 217–226. doi: 10.1590/0103-9016-2015-0288
Robinson, B. H., Lombi, E., Zhao, F. J., and McGrath, S. P. (2003). Uptake and distribution of nickel and other metals in the hyperaccumulator Berkheya coddii. New Phytol. 158, 279–285. doi: 10.1046/j.1469-8137.2003.00743.x
Shields, L. M. (1951). The involution mechanism in leaves of certain xeric grasses. Phytomorphology 1, 225–241.
Shojima, S., Nishizawa, N. K., Fushiya, S., Nozoe, S., Kumashiro, T., Nagata, T., et al. (1989). Biosynthesis of nicotianamine in the suspension-cultured cells of tobacco (Nicotiana megalosiphon). Biol. Metals 2, 142–145. doi: 10.1007/BF01142552
Stephan, U. W., Schmidke, I., Stephan, V. W., and Scholz, G. (1996). The nicotianamine molecule is made-to-measure for complexation of metal micronutrients in plants. Biometals 9, 84–90.
Stumm, W., and Lee, G. F. (1961). Oxygenation of ferrous iron. Ind. Eng. Chem. 53, 143–146. doi: 10.1021/ie50614a030
Suzuki, M., Tsukamoto, T., Inoue, H., Watanabe, S., Matsuhashi, S., Takahashi, M., et al. (2008). Deoxymugineic acid increases Zn translocation in Zn-deficient rice plants. Plant Mol. Biol. 66, 609. doi: 10.1007/s11103-008-9292-x
Takagi, S. (1976). Naturally occurring iron-chelating compounds in oat- and rice-root washing. I: activity measurement and preliminary characterization. Soil Sci. Plant Nutr. 22, 423–433. doi: 10.1080/00380768.1976.10433004
Takahashi, M., Terada, Y., Nakai, I., Nakanishi, H., Yoshimura, E., Mori, S., et al. (2003). The role of nicotianamine in the intracellular delivery of metals and plant reproductive development. Plant Cell 15, 1263–1280.
Trijatmiko, K. R., Duenas, C., Tsakirpaloglou, N., Torrizo, L., Arines, F. M., Adeva, C., et al. (2016). Biofortified indica rice attains iron and zinc nutrition dietary targets in the field. Sci. Rep. 6:19792. doi: 10.1038/srep19792
Tsukamoto, T., Nakanishi, H., Uchida, H., Watanabe, S., Matsuhashi, S., Mori, S., et al. (2009). 52Fe translocation in barley as monitored by a positron emitting tracer imaging system (PETIS): evidence for the direct translocation of Fe from roots to young leaves via phloem. Plant Cell Physiol. 50, 48–57. doi: 10.1093/pcp/pcn192
von Uexküll, H. R., and Mutert, E. (1995). “Global extent, development and economic impact of acid soils,” in Plant–Soil Interactions at Low pH: Principles and Management, eds R. A. Date, N. J. Grundon, G. E. Raymet, and M. E. Probert (Dordrecht: Kluwer Academic Publishers), 5–19. doi: 10.1007/978-94-011-0221-6_1
von Wirén, N., Klair, S., Bansal, S., Briat, J. F., Khodr, H., Shioiri, T., et al. (1999). Nicotianamine chelates both Fe-III and Fe-II. Implications for metal transport in plants. Plant Physiol. 119, 1107–1114. doi: 10.1104/pp.119.3.1107
Yang, G. H., Li, J., Liu, W., Yu, Z. Y., Shi, Y., Lv, B. Y., et al. (2015). Molecular cloning and characterization of MxNAS2, a gene encoding nicotianamine synthase in Malus xiaojinensis, with functions in tolerance to iron stress and misshapen flower in transgenic tobacco. Sci. Hortic. 183, 77–86. doi: 10.1016/j.scienta.2014.12.014
Keywords: iron excess, rice, OsNAS3, nicotianamine, deoxymugineic acid, detoxification
Citation: Aung MS, Masuda H, Nozoye T, Kobayashi T, Jeon J-S, An G and Nishizawa NK (2019) Nicotianamine Synthesis by OsNAS3 Is Important for Mitigating Iron Excess Stress in Rice. Front. Plant Sci. 10:660. doi: 10.3389/fpls.2019.00660
Received: 31 January 2019; Accepted: 02 May 2019;
Published: 04 June 2019.
Edited by:
Wolfgang Schmidt, Academia Sinica, TaiwanReviewed by:
Elsbeth L. Walker, University of Massachusetts Amherst, United StatesAlexander Arthur Theodore Johnson, The University of Melbourne, Australia
Copyright © 2019 Aung, Masuda, Nozoye, Kobayashi, Jeon, An and Nishizawa. This is an open-access article distributed under the terms of the Creative Commons Attribution License (CC BY). The use, distribution or reproduction in other forums is permitted, provided the original author(s) and the copyright owner(s) are credited and that the original publication in this journal is cited, in accordance with accepted academic practice. No use, distribution or reproduction is permitted which does not comply with these terms.
*Correspondence: Naoko K. Nishizawa, YW5uYW9rb0BtYWlsLmVjYy51LXRva3lvLmFjLmpw