- 1Departamento de Microbiología del Suelo y Sistemas Simbióticos, Estación Experimental del Zaidín, Consejo Superior de Investigaciones Científicas, Granada, Spain
- 2Genomic Medicine Department, GENYO, Centre for Genomics and Oncological Research, Pfizer-University of Granada-Andalusian Regional Government, Granada, Spain
- 3Biochimie et Physiologie Moléculaire des Plantes, Institut National de la Recherche Agronomique, Centre National de la Recherche Scientifique, Université de Montpellier, Montpellier SupAgro, Montpellier, France
Arbuscular mycorrhizal fungi increase fitness of their host plants under Cu deficient and toxic conditions. In this study, we have characterized two Cu transporters of the CTR family (RiCTR1 and RiCTR2) and a CTR-like protein (RiCTR3A) of Rhizophagus irregularis. Functional analyses in yeast revealed that RiCTR1 encodes a plasma membrane Cu transporter, RiCTR2 a vacuolar Cu transporter and RiCTR3A a plasma membrane protein involved in Cu tolerance. RiCTR1 was more highly expressed in the extraradical mycelia (ERM) and RiCTR2 in the intraradical mycelia (IRM). In the ERM, RiCTR1 expression was up-regulated by Cu deficiency and down-regulated by Cu toxicity. RiCTR2 expression increased only in the ERM grown under severe Cu-deficient conditions. These data suggest that RiCTR1 is involved in Cu uptake by the ERM and RiCTR2 in mobilization of vacuolar Cu stores. Cu deficiency decreased mycorrhizal colonization and arbuscule frequency, but increased RiCTR1 and RiCTR2 expression in the IRM, which suggest that the IRM has a high Cu demand. The two alternatively spliced products of RiCTR3, RiCTR3A and RiCTR3B, were more highly expressed in the ERM. Up-regulation of RiCTR3A by Cu toxicity and the yeast complementation assays suggest that RiCTR3A might function as a Cu receptor involved in Cu tolerance.
Introduction
The transition metal copper (Cu) is a micronutrient acting as a redox active cofactor of key enzymes involved in a wide array of biochemical processes essential for life, such as respiration, superoxide scavenging and iron mobilization (Linder, 1991; Festa and Thiele, 2011). However, when in excess, it becomes toxic due to its ability to displace other metal ions in structural or catalytic protein motifs (Macomber and Imlay, 2009) and through the generation of hydroxyl radicals by Fenton-like reactions (Halliwell and Gutteridge, 1984). Due to the dual nature of Cu, organisms have developed sophisticated homeostatic networks to tightly regulate its intracellular levels, in which membrane transporters mediating Cu uptake and efflux and specific chaperones that handle and deliver Cu to its specific target enzymes play a key role (Puig and Thiele, 2002; Kim et al., 2008; Smith et al., 2017).
In eukaryotes, the major entrance of Cu into the cells occurs through members of the Cu transporter (CTR) family, small integral membrane proteins of variable length (from 150 to 500 amino acid residues) that have three transmembrane (TM) domains and the characteristic signature MetXXXMet-X12-GlyXXXGly embedded within TM2 and TM3 (Dumay et al., 2006; De Feo et al., 2007). CTRs are present in the membranes as a homo-oligomer or hetero-oligomer complex, being the cooperation between the different subunits crucial for Cu transport (Puig et al., 2002; Beaudoin et al., 2011). The MetXXXMet motif is located in TM2 and together with a cluster of Met residues in the N terminal domain is involved in Cu sensing and uptake (Puig et al., 2002; Guo et al., 2004), while TM3 harbors the GlyXXXGly motif that is critical for protein folding and oligomerization (Aller et al., 2004). The carboxy terminal domain usually contains Cys and/or His motifs that bind and transfer Cu to cytosolic chaperones for its final targeted distribution (Dancis et al., 1994b; Xiao et al., 2004; Puig, 2014). Additionally, under Cu toxicity this domain allows protein inactivation through conformational structural changes (Wu et al., 2009). This family of transporters has been widely studied in Saccharomyces cerevisiae, which encodes three members (Ctr1, Ctr2, and Ctr3). Ctr1 and Ctr3 are functionally redundant plasma membrane transporters that mediate Cu acquisition from the environment (Dancis et al., 1994a; Peña et al., 2000), while Ctr2 is located in the vacuolar membrane and pumps vacuolar Cu stores to the cytosol (Portnoy et al., 2001; Rees et al., 2004). Ctr1 and Ctr3 expression is highly induced under Cu deficiency in order to facilitate high-affinity Cu acquisition and Ctr2 mobilizes Cu vacuolar stores when Cu levels are extremely low. Apart from other yeasts (Bellemare et al., 2002; Marvin et al., 2003; Beaudoin et al., 2011), CTRs have been characterized in the basidiomycetes Pleurotus ostreatus (Penas et al., 2005), Coprinopsis cinerea (Nakagawa et al., 2010) and Amanita strobiliformis (Beneš et al., 2016), as well as in the filamentous ascomycetes Podospora anserina (Borghouts et al., 2002), Colletotrichum gloeosporioides (Barhoom et al., 2008) and Neurospora crassa (Korripally et al., 2010). Fungal Ctr proteins have been shown to be involved in different processes. For example, the vacuolar Cu transporter Ctr2 of the plant pathogen C. gloeosporioides is essential for optimal spore germination and pathogenesis (Barhoom et al., 2008) and the high-affinity Cu transporter TCU-1 of N. crassa is essential for saprophytic conidical germination and vegetative growth under Cu limiting conditions (Korripally et al., 2010). However, very little is known about the mechanisms of Cu uptake in arbuscular mycorrhizal (AM) fungi, the most ancient and widespread fungal plant symbionts.
Arbuscular mycorrhizal fungi are soil-borne microorganisms of the subphylum Glomeromycotina within the Mucoromycota (Spatafora et al., 2016) that establish a mutualistic symbiosis with the majority of land plants. In this mutualistic relationship the fungal partner receives carbon compounds from the plant in exchange of low mobility mineral nutrients in soil, mainly phosphorus and some micronutrients, such as Zn and Cu (Smith and Read, 2008; Lanfranco et al., 2018). Besides improving plant mineral nutrition, AM fungi increase plant ability to overcome biotic and abiotic stress conditions, such as salinity, drought and metal toxicity (Ruiz-Lozano, 2003; Pozo et al., 2013; Ferrol et al., 2016). It is noteworthy the ability of AM fungi to increase plant fitness under deficient and excess Cu availability (Lehmann and Rillig, 2015; Ferrol et al., 2016). As revealed by isotopic labeling experiments, improvements in Cu nutrition by AM fungi are due to the capability of the extraradical mycelia (ERM) to absorb the micronutrient beyond the depletion zone that develops around the roots (Li et al., 1991; Lee and George, 2005). On the other hand, increased plant performance in Cu-polluted soils is mainly due to the ability of the fungus to act as a barrier for Cu entry into the plant tissues (Ferrol et al., 2016; Merlos et al., 2016). Despite the central role Cu transporters play in all organisms to cope with a range of Cu availability, from scarcity to excess, the mechanisms of Cu import in AM fungi have not been characterized yet. In a previous genome-wide analysis of metal transporters in the AM fungus Rhizophagus irregularis, we identified three genes putatively encoding Cu transporters of the CTR family that mediate Cu transport into the cytosol (Tamayo et al., 2014). With the aim to get further insights into the mechanisms of Cu homeostasis in AM fungi, in this work we carried out the first characterization of the R. irregularis CTR transporters.
Materials and Methods
Biological Materials and Growth Conditions
The AM fungal isolate used in this study was Rhizophagus irregularis (Blaszk., Wubet, Renker & Buscot) C. Walker & A. Schüßler DAOM 197198. The fungal inoculum used for the root organ cultures and for the seedlings was obtained in monoxenic cultures. AM monoxenic cultures were established according to St-Arnaud et al. (1996), with some modifications. Briefly, Ri T-DNA transformed carrot (Daucus carota L. clone DC2) roots were cultured with R. irregularis in solid M medium (Chabot et al., 1992) in two-compartment Petri dishes. Cultures were started in one compartment by placing the fungal inoculum (ERM, spores and mycorrhizal roots fragments) and some pieces of carrot roots. Plates were incubated in the dark at 24°C for 6–8 weeks until the other compartment of the Petri dish was profusely colonized by the fungus and roots (root compartment). The older compartment was removed and filled with liquid M medium without sucrose (M-C medium) and the fungal mycelium was allowed to colonize this compartment (hyphal compartment) during the two subsequent weeks (Control plates).
For the Cu deficiency treatments, monoxenic cultures were established in media without Cu and started with roots and inoculum previously grown either in M media, which contains 0.5 μM CuSO4, (moderate Cu deficiency treatment) or in M media without Cu (severe Cu deficiency treatment), and grown in the same conditions than the control plates but in media lacking Cu. ERM and mycorrhizal roots grown, respectively, in the hyphal and root compartment of each plate were collected, rapidly dried on filter paper, immediately frozen in liquid N and stored at -80°C until used. An aliquot of the roots from each treatment was separated to estimate mycorrhizal colonization.
For the Cu toxicity and H2O2 treatments, the M-C medium of the hyphal compartment was removed and replaced with fresh liquid M-C medium (Control, 0.5 μM CuSO4) or with M-C medium supplemented with 250 μM CuSO4, 500 μM CuSO4 or 1 mM H2O2 and incubated at 24°C. The time of medium exchange was referred as time 0. Mycelia were collected 1, 2, and 7 days after Cu addition and 1 h after H2O2 supplementation.
For gene expression comparison between ERM and IRM, several non-mycorrhizal carrot roots pieces were placed on the top of a densely fungal colonized compartment and grown for 15 days at 24°C. Roots were carefully collected with tweezers under a bionocular microscope trying to remove the attached extraradical hyphae, frozen in liquid N and stored at -80°C until used. An aliquot of root fragments was separated to estimate mycorrhizal colonization.
Rhizophagus irregularis ERM was also collected from mycorrhizal plants grown in the in vivo whole plant bidimensional experimental system described by Pepe et al. (2017) with some modifications (Supplementary Figure S1). Briefly, chicory (Cichorium intybus L.) seeds were surface-sterilized and germinated for 10–15 days in sterilized sand. Seedlings were transplanted into 50 mL pots filled with sterilized sand and inoculated with spores, ERM and colonized roots obtained from monoxenic cultures. Pots were placed in sun-transparent bags (Sigma-Aldrich, B7026) and maintained during 1 month in a growth chamber at 24°C/21°C day/night and 16 h light photoperiod. The root system of each plant was cleaned, wrapped in a nylon net (41 μM mesh, Millipore NY4100010) and placed between two 13 cm membranes of mixed cellulose esters (0.45 μm pore diameter size, MF-Millipore HAWP14250) in 14 cm diameter Petri dishes having a hole on the edge to allow plant shoot growth and containing sterilized sand. Petri plates containing plants were sealed with parafilm, wrapped with aluminum foil, placed into sun-transparent bags and maintained in a growth chamber. Plants were watered weekly with a 0.5X modified Hoagland nutrient solution containing 125 μM KH2PO4 and 0.16 μM CuSO4 (control treatment) or without Cu (Cu deficiency treatment). Each treatment consisted of five replicates. Petri dishes were opened 2 weeks after preparing the root sandwiches and ERM spreading from the nylon net onto the membranes was collected with tweezers, frozen in liquid N and stored at -80°C until used. Roots wrapped in the nylon net were also frozen and stored at -80°C. An aliquot of the roots was separated to estimate mycorrhizal colonization.
The Saccharomyces cerevisiae strains used in this study were MPY17 (ctr1Δctr3Δ), a double-mutant lacking the plasma membrane transporters Ctr1 and Ctr3 (Peña et al., 1998) and MPY17 ctr2Δ (ctr1Δctr2Δctr3Δ), a triple mutant lacking also the vacuolar transporter ScCtr2 (Rees et al., 2004) and WYT (yap1Δ) a strain lacking the transcription factor yap1 (Kuge and Jones, 1994). Detailed characteristics of yeast strains are listed in Supplementary Table S1. Yeast cells were maintained on YPD or minimal synthetic dextrose (SD) medium, supplemented with appropriate amino acids.
Mycorrhizal Colonization
Histochemical quantification of mycorrhizal colonization was performed according to Trouvelot et al. (1986) using the Mycocalc program1 in root samples previously cleared with 10% KOH and stained with 0.05% trypan blue (Phillips and Hayman, 1970). The abundance of the AM fungus was also assessed by determining the expression level of the R. irregularis elongation factor 1α (RiEF1α; GenBank Accession No. DQ282611), using as internal control the elongation factor 1α of the corresponding host plant (Daucus carota L. DcEF1α, GenBank Accession No. XM_017391845; Cichorium intybus L. CiEF1α, GenBank Accession No. KP752079).
Nucleic Acids Extraction and cDNA Synthesis
Rhizophagus irregularis genomic DNA was isolated from ERM developed in the hyphal compartment of control plates using the DNeasy Plant Mini Kit (Qiagen), according to the manufacturer’s instructions.
Total RNA extraction from fungal ERM and mycorrhizal carrot roots developed in monoxenic cultures was performed using the Plant RNeasy Kit (Qiagen) following manufacturer’s instructions. Total RNA from mycorrhizal chicory roots was isolated using the phenol/SDS method followed by LiCl precipitation (Kay et al., 1987). RNAs were DNase treated with the RNA-free DNase set (Qiagen) according to manufacturer’s instructions and quantified using the NanoDrop 1000 Spectrophotometer (Thermo Scientific). cDNAs were synthetized from 1 μg of total DNase-treated RNAs in a 20 μL reaction containing 200 U of SuperScript III Reverse Transcriptase (Invitrogen) and 2.5 μM oligo (dT)20 primer (Invitrogen), following the manufacturer’s instructions.
RiCTRs Identification and Sequences Analyses
Candidate gene sequences putatively encoding RiCTRs were previously identified by Tamayo et al. (2014). Additional Blastp searches were performed in the filtered model datasets of the R. irregularis isolates DAOM197198 v2.0 and A1, A4, A5, B3, and C2 v1.0 (Chen et al., 2018) recently deposited at the JGI website2, using as a query the previously identified RiCTR candidates. These sequences were also used to identify CTR homologs via Blastp in the sequence datasets of other Glomeromycotina species deposited on the JGI (Gigaspora rosea v1.0, Rhizophagus cerebriforme DAOM 227022 v1.0 and Rhizophagus diaphanus v1.0 (Morin et al., 2019) and NCBI [Diversispora epigaea (Sun et al., 2019) and Rhizophagus clarus (Kobayashi et al., 2018)] websites.
Sequence analyses were performed using the DNAstar Lasergene software package (DNAstar), BLAST tool of NCBI3 and Clustal Omega for sequence alignments4. Gene promoter sequences were screened for the presence of regulatory cis elements employing the tools included in the Promoter Database of Saccharomyces cerevisiae SCPD5. Specific Cu responsive elements (CuREs) and AP-1 sites were further screened through DNA pattern matching analyses in the fungal RSAT server6. Identity and Similarity percentages between proteins were calculated using Ident and Sim tool from Sequence Manipulation Suite7. Conserved domains of proteins were identified using the Conserved Domain Database at NCBI8, predictions of putative TM domains via the TMHMM Server v.2.09, the SOSUI engine v. 1.1110 and the TOPCONS web server11, Structural models of the RiCTRs were generated using MyDomains tool of Prosite12. Phylogenetic analyses were performed via the Neighbor-Joining method implemented in the Molecular Evolutionary Genetics Analysis software v. 6. (MEGA), with 1,000 bootstrap replicates, using Poisson model and pairwise deletion of gaps options for distance computation.
Gene Isolation
The cDNA sequences of the 5′ and 3′ends of RiCTR1-3 were confirmed and completed, when necessary, by RACE using the SMARTer® RACE 5′/3′ kit (Clontech) according to the manufacturer’s protocol. The primers used for RACE reactions are listed in Supplementary Table S2. Genomic clones and full length cDNAs were obtained by PCR amplification of R. irregularis genomic DNA and cDNA, respectively, from ERM grown under control conditions in monoxenic cultures, using a set of primers flanking the complete open reading frames (Supplementary Table S2). PCR products were cloned into the pGEM-T Easy vector (Promega), following manufacturer’s instructions. Plasmids were amplified by transformation of chemically Escherichia coli DH5α competent cells according to standard procedures and purified using the GenEluteTM Plasmid Miniprep Kit (Sigma-Aldrich). All plasmids were checked by sequencing (ABI PRISM 3130xl Genetic Analyzer, Applied Biosystems, Carlsbad, CA, United States).
Functional Complementation Analyses in Yeast
RiCTR open reading frames were sub-cloned between SmaI and XhoI sites (RiCTR1) or PstI and SalI sites (RiCTR2 and RiCTR3) of the yeast expression vector pDR196. For this purpose, the respective full-length cDNA sequences were flanked with the sequences recognized by the corresponding restriction enzymes by PCR using the primers described in Supplementary Table S2. PCR products were cloned into the pGEM-T Easy vector (Promega), following manufacturer’s instructions. The full-length cDNAs were then isolated from the pGEM-T Easy vector by digestion with the corresponding restriction enzymes and ligated into the digested pDR196 vector. All constructs were verified by sequencing. The S. cerevisiae strains ctr1Δctr3Δ, ctr1Δctr2Δctr3Δ and yap-1Δ were transformed with the corresponding pDR196-RiCTR constructs or with the empty vector using a lithium acetate-based method (Gietz and Schiestl, 2007). Transformants were selected in SD medium by autotrophy to uracil. For drop tests, transformants were grown to exponential phase in SD medium without uracil. Cells were harvested by centrifugation, washed twice and adjusted to a final OD600 of 1. Then, 5 μL of serial 1:10 dilutions were spotted on the corresponding selective medium. The transformed ctr1Δctr3Δ and ctr1Δctr2Δctr3Δ strains were spotted onto a non-fermentable carbon source ethanol-glycerol medium (YPEG: 1% yeast extract, 2% bacto-peptone, 2% ethanol, 3% glycerol, 1.5% bacto-agar) supplemented with 0, 10, or 20μM CuSO4. The transformed yap-1Δ cells were spotted onto SD without uracil supplemented either with 1.5 mM CuSO4 or 0.5 mM H2O2.
Protein Localization
Subcellular localization of RiCTR1-3 was assessed with N or C terminal fusions of these genes to the enhanced green fluorescent protein (eGFP) in the S. cerevisiae triple mutant ctr1Δctr2Δctr3Δ or in yap-1Δ. The coding sequences of RiCTR1, RiCTR2 and RiCTR3A were cloned with or without their stop codon into pENTR/D-TOPO (Invitrogen) via TOPO reactions and then into the destination vectors pFGWDR196 or pGWFDR196 by using the Gateway LR Clonase recombination system (Invitrogen) for eGFP-tagging at the amino- or carboxy-terminus, respectively. Primers pairs used are listed in Supplementary Table S2. The corresponding yeast mutants were transformed with the resulting pFGWDR/pGWFDR196-RiCTR constructs or with the empty vector (negative control). Functionality of the GFP-tagged versions of RiCTRT1, RiCTR2, and RiCTR3A was tested in complementation assays, as previously described. For the protein localization assays, yeast cells were grown to exponential phase in liquid SD without uracil and visualized using a Leica TCS SP8 laser scanning microscope with a 63× oil N.A. 1.4 immersion objective. Emission fluorescence of GFP was excited at 488 nm and the emitted signal was collected between 500 and 540 nm. To reduce overexpression artifacts, yeast cells were treated just before visualization with the protein synthesis inhibitor cycloheximide (100 μM) for 45 min. Images were processed using ImageJ software.
Gene Expression Analyses
Gene expressions were analyzed by real-time quantitative RT-PCR using an iQTM 5 Multicolor Real-Time PCR Detection System (Bio-Rad). Each 20 μl reaction contained 1 μl of a 1:10 dilution of the cDNA, 200 nM each primer and 10 μl iQTM SYBR Green Supermix (Bio-Rad). The primer sets used are listed in Supplementary Table S2. The program consisted in an initial incubation at 95°C for 3 min, followed by 38 cycles of 95°C for 30 s, 58°C for 30 s and 72°C for 30 s, where the fluorescence signal was measured. The specificity of the PCR amplification procedure was checked with a heat-dissociation protocol (from 58 to 95°C) after the final PCR cycle. Since RNA extracted from mycorrhizal roots contains plant and fungal RNAs, specificity of the primers pairs was also analyzed by PCR amplification of carrot and chicory genomic DNA and cDNA from non-mycorrhizal carrot and chicory roots. Specificity of the RiCTR3A and RiCTR3B primer pairs was analyzed by PCR amplification of the RiCTR3A and RiCTR3B plasmid DNAs. RT-PCR determinations were performed in three independent biological samples with the threshold cycle (Ct) determined in duplicate in at least two independent PCR experiments. The relative abundance of the transcripts was calculated by using 2-ΔΔCT method (Livak and Schmittgen, 2001) and normalized according to the expression of the R. irregularis elongation factor 1α (RiEF1α; GenBank Accession No. DQ282611).
Statistical Analyses
IBM SPSS Statistic software v.23 was used for the statistical analysis of the means and standard error determinations. Data were subjected to the Student’s t-test when two means were compared or by one-way ANOVA followed by a Fisher’s LSD to find out differences among groups of means (P < 0.05). All the analyses are based on at least three biological replicates per each treatment (n ≥ 3).
Results
Features of the R. irregularis CTR Proteins
The R. irregularis CTR1, CTR2, and CTR3 full-length cDNA sequences were obtained by RACE using gene-specific primers based on the sequences described by Tamayo et al. (2014) [GenBank Accession No. /JGI IDs: PKC06371/1491164 (RiCTR1), EXX67481/1726366 (RiCTR2) and PKC14368/495436 (RiCTR3)]. Interestingly, two types of CTR3 transcripts were identified in the R. irregularis ERM, RiCTR3A of 531 bp and RiCTR3B of 606 bp. Comparisons of the full-length cDNAs with the genomic sequences revealed the presence of three introns in RiCTR1 and two in RiCTR2 and RiCTR3, all of them flanked by the canonical splicing sequences GT and AG at the 5′ and 3′ ends, respectively (Figure 1A). Alignment of the RiCTR3A and RiCTR3B transcripts with the RiCTR3 gene sequence indicates that both transcripts are alternatively spliced products of the same gene, as the RiCTR3A and RiCTR3B sequences are contained within the genomic sequence. RiCTR3B, the longest RiCTR3 variant, contains the first intron after the RiCTR3A start codon generating a premature termination codon-containing mRNA. However, an additional start codon located within the second exon becomes available to produce a protein that contains the last 97 amino acids of RiCTR3A.
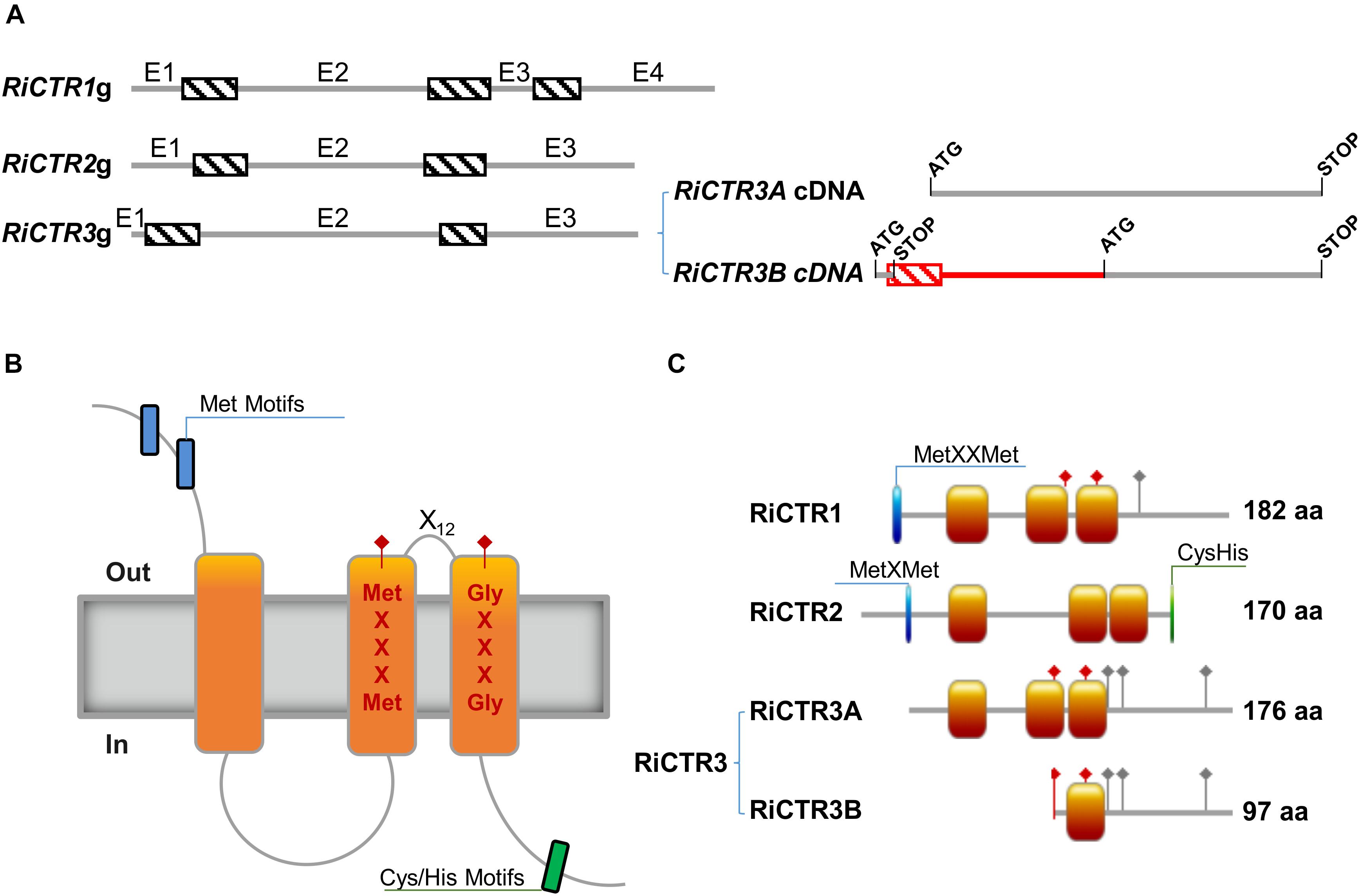
Figure 1. Structure of the R. irregularis CTR proteins. (A) Exon/intron organization of the R. irregularis CTR genes. Exons (E) and introns are represented by gray lines and dashed boxes, respectively. The two transcript variants of RiCTR3 are illustrated at the right. The coding regions of the two spliced variants of RiCTR3 are shown in gray and the non-coding in red. The start and stop codons are indicated. (B) Typical topological model of a CTR transporter. (C) Schematic representation of the structure of the R. irregularis CTR transporters. Orange boxes illustrate transmembrane domains, blue boxes Met motifs and green boxes Cys and His motifs. Red diamonds show the positions of the MetXXXMet and the GlyXXXGly motives of the CTRs master signature and gray diamonds the positions of the C-terminal His/Cys residues. Amino acid lengths are also indicated.
RiCTR1, RiCTR2, and RiCTR3A encode proteins of 182, 170, and 176 amino acids, respectively, that have three TM domains with the MetXXXMet-X12-GlyXXXGly signature embedded within TM2 and TM3, an intracellular loop connecting TM1 and TM2, the N terminus toward the extracellular space and the C terminus facing the cytosol (Figures 1B,C). RiCTR1 and RiCTR2 present a Met motif, MetXXMet in RiCTR1 and MetXMet in RiCTR2, in the N terminal extracytosolic region 29 and 23 amino acids before TM1, respectively. This methionine motif, which is essential for CTR function (Puig et al., 2002), is absent in RiCTR3A. RiCTR2 harbors a Cys/His motif in the carboxy-terminal region facing the cytoplasm, while RiCTR1 has a single His residue and RiCTR3A a Cys and two His residues (Figure 1C and Supplementary Figure S2). Despite the similar structure and sequence amino acid length of RiCTR1, RiCTR2, and RiCTR3A, similarity between their deduced amino acid sequences is lower than 53%, displaying RiCTR1 and RiCTR3A the highest similarity (Supplementary Table S3). RiCTR3B encodes a protein of 97 amino acids that harbors the MetXXXMet-X12-GlyXXXGly signature of CTR proteins, but has a single TM domain, the MetXXXMet is mislocalized in the N terminal domain and the GlyXXXGly motif is embedded in its only TM domain.
A phylogenetic analysis of fungal CTR transporters revealed that RiCTR1 and RiCTR3 clustered with the S. cerevisiae plasma membrane Ctr1/Ctr3-like Cu transporters and RiCTR2 with the S. cerevisiae vacuolar Ctr2-like transporters. Within each clade, all Glomeromycotina sequences were grouped together (Supplementary Figure S3).
In silico searches for putative regulatory elements in their promoter sequences resulted in the identification of several core elements identical to the Cu response cis-element (CuRE) GTAC present in the promoters of Cu-responsive genes (Jamison McDaniels et al., 1999; Kropat et al., 2005) and the consensus sequence of the AP-1 cis-acting element (TTATTAA/TTAGTAA) recognized as a conserved motif in the oxidative stress-responsive genes (Toone and Jones, 1999) (Supplementary Figure S4). Interestingly, the 5′-flanking region of RiCTR3 is especially rich in AP-1 motifs and contains the preferred DNA binding site (TTACTAA) of the transcription factor YAP1 (Toone and Jones, 1999), which is essential for the oxidative stress response in S. cerevisiae (Kuge and Jones, 1994).
RiCTR1 and RiCTR2 Encode Functional Cu Transporters
Since AM fungi cannot be genetically manipulated, functionality of the RiCTRs was assessed in yeast by testing their ability to revert the inability of the double (ctr1Δctr3Δ) and triple (ctr1Δctr2Δctr3Δ) S. cerevisiae CTR mutants, which lack the plasma membrane Ctr1 and Ctr3 Cu transporters and the plasma membrane Ctr1/Ctr3 and the vacuolar Ctr2 transporters, to grow on a non-fermentable carbon source at low Cu concentrations. This growth defect is due to the inability of the cytochrome c oxidase to obtain its Cu cofactor, resulting in a defective mitochondrial respiratory chain (Dancis et al., 1994a; Glerum et al., 1996; Rees et al., 2004). To perform the yeast complementation assays, the full-length cDNA coding sequences of RiCTR1, RiCTR2, RiCTR3A, or RiCTR3B were expressed under the control of the yeast PMA1 promoter in both yeast CTR mutants and plated on ethanol-glycerol (YPEG) medium supplemented with different Cu concentrations. The empty vector-expressing cells were unable to grow on YPEG medium containing <20 μM Cu (Figure 2A). Expression of RiCTR1 restored the inability of the ctr1Δctr3Δ and ctr1Δctr2Δctr3Δ yeast strains to grow on YPEG medium supplemented with 10 μM Cu, indicating that RiCTR1 is a functional homolog of the yeast plasma membrane Cu transporters Ctr1/Ctr3. RiCTR2 complemented the inability of the ctr1Δctr2Δctr3Δ mutant strain to grow on YPEG medium supplemented with 10 μM Cu but not of the double mutant lacking the two plasma membrane transporters, which suggests that RiCTR2 is a functional homolog of the S. cerevisiae vacuolar transporter Ctr2. However, none of the RiCTR3 variants rescued the phenotype of either the double or the triple CTR mutants (Figure 2A and Supplementary Figure S5), which was expected since their encoded proteins did not contain the required features for CTR function.
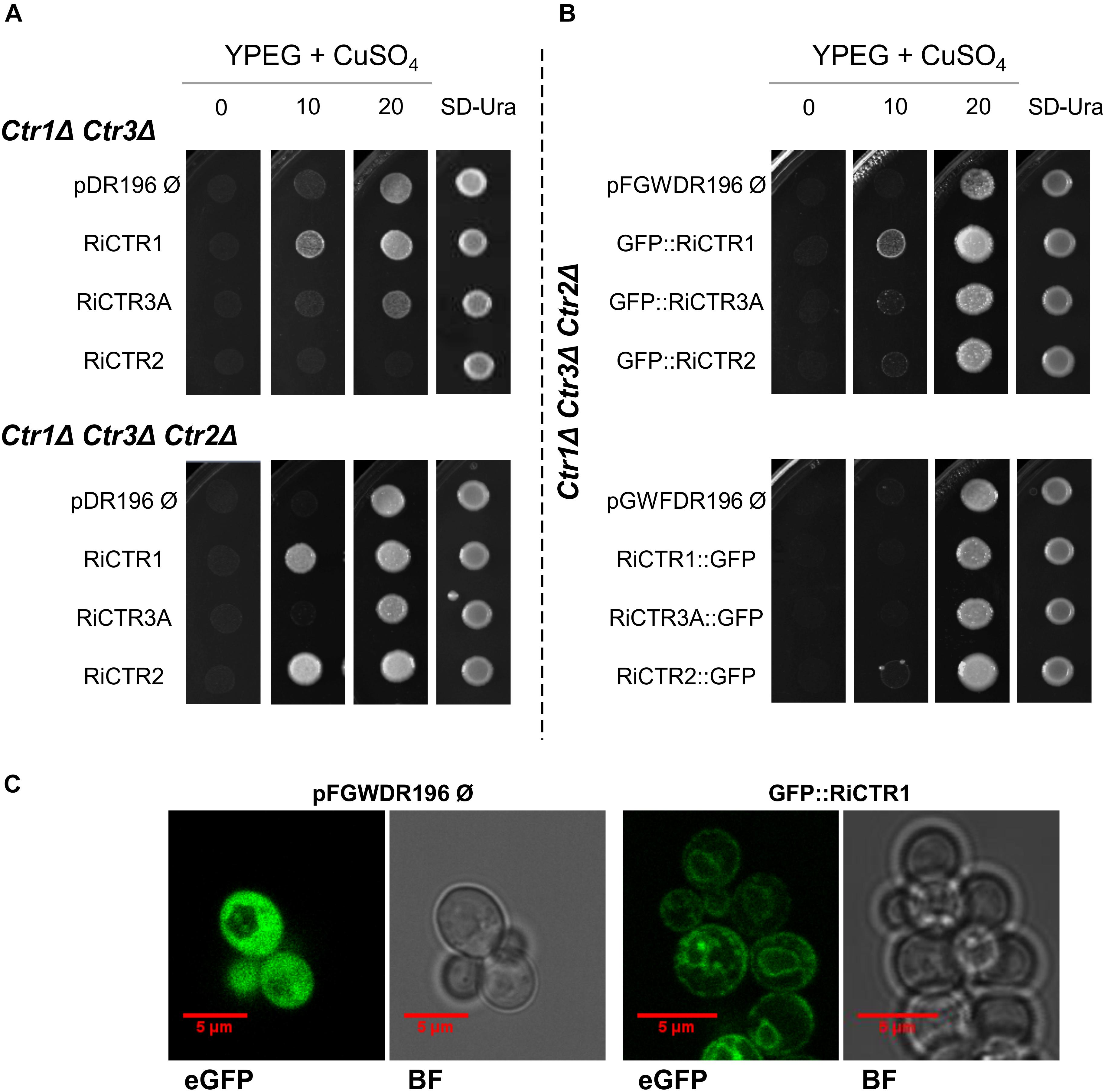
Figure 2. Analysis of the Cu transport activity and subcellular localization of the R. irregularis CTRs in yeast. (A) ctr1Δctr3Δ and ctr1Δctr2Δctr3Δ yeast cells transformed with the empty vector or expressing RiCTR1, RiCTR2, RiCTR3A or RiCTR3B were plated on YPEG media supplemented with Cu (0, 10, or 20 μM CuSO4) or on SD medium without uracil. ctr1Δctr3Δ and ctr1Δctr2Δctr3Δ plated cells were incubated at 30°C for 4 and 7 days, respectively. RiCTR3A and RiCTR3B had the same effect (see Supplementary Figure S5), only the result with RiCTR3A is illustrated. (B) ctr1Δctr2Δctr3Δ yeast cells expressing GFP (empty vector), N-terminal (upper panel) or C-terminal (lower panel) GFP-tagged versions of RiCTR1, RiCTR2 and RiCTR3A were plated on YPEG media supplemented with Cu (0, 10, or 20 μM CuSO4) or on SD medium without uracil. Plates were incubated at 30°C for 7 days. (C) ctr1Δctr2Δctr3Δ cells expressing GFP (the empty vector pFGWDR196) and GFP::RiCTR1 were visualized with a confocal microscope. eGFP, enhanced GFP fluorescence; BF, bright field.
Subcellular localization of RiCTR1 and RiCTR2 was assessed in the heterologous system by expressing N- and C-terminal GFP-tagged versions of these proteins in the ctr1Δctr2Δctr3Δ strain and visualizing the fusion proteins with a confocal fluorescence microscope. S. cerevisiae cells transformed with the empty vector and expressing GFP under the control of the PMA1 promoter were used as a negative control; and functionality of the RiCTR1-2 fusion proteins was assessed before their visualization (Figure 2B). The control cells expressing the soluble GFP showed a general cytosolic fluorescence (Figure 2C). The RiCTR1-GFP, RiCTR2-GFP and GFP-RiCTR2 fusion proteins were unable to revert the mutant phenotype of the ctr1Δctr2Δctr3Δ strain and were expressed within the perinuclear endoplasmic reticulum region (data not shown), indicating that the fusion proteins failed to exit the endoplasmic reticulum. As expected from the complementation assays, the GFP-RiCTR1-expressing cells showed a clear fluorescent signal at the cell periphery indicative of a plasma membrane localization. GFP-RiCTR1 was also localized within the perinuclear endoplasmic reticulum membrane, a phenomenon commonly found in yeast membrane protein overexpression assays (Figure 2C).
RiCTR Genes Are Differentially Expressed in the IRM and ERM
To gain information about the expression of RiCTR1-3 during symbiosis and about their relative abundance in the intraradical mycelia (IRM) and ERM, their expression levels were determined by real time quantitative RT-PCR (RT-qPCR) in ERM grown in liquid monoxenic cultures and in the in vivo sandwich system, and in the IRM developed in carrot roots grown in vitro for 2 weeks on a densely colonized hyphal compartment and devoid of ERM and in mycorrhizal chicory roots collected from the in vivo sandwich system. Mycorrhizal colonization levels of the carrot and chicory roots were 10 and 78%, respectively. As a reference for fungal activity, we measured transcript levels of the R. irregularis high-affinity monosaccharide transporter RiMST2, which is highly expressed in the IRM during AM symbiosis (Helber et al., 2011). In both experimental systems, RiCTR1 was the isoform more highly expressed in the ERM and the expression levels of RiCTR2 were higher in the IRM than in the ERM. Expression levels of the two spliced-variants of RiCTR3 were very low in both fungal structures and more highly expressed in the ERM (Figure 3).
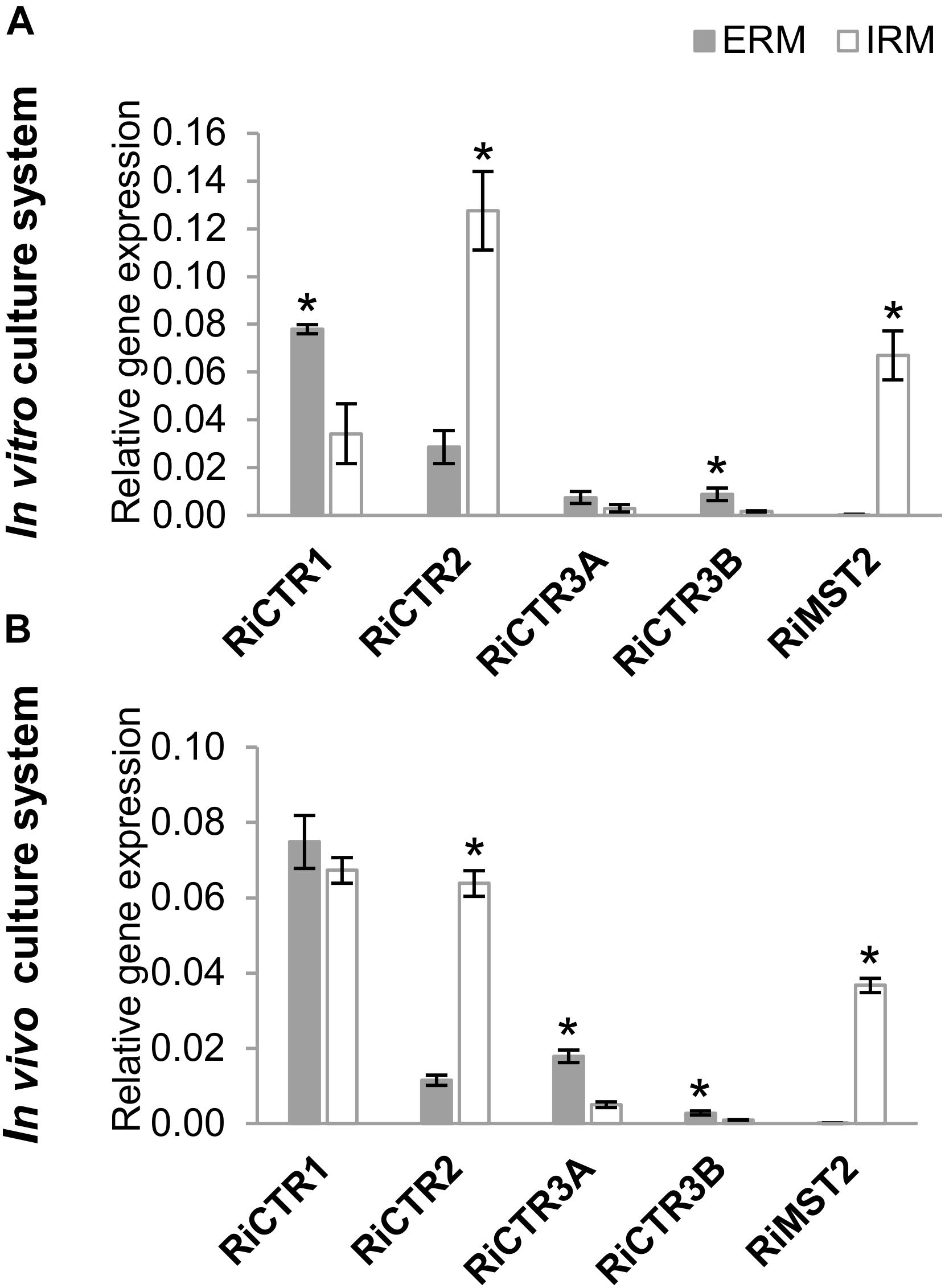
Figure 3. RiCTRs expression levels in the R. irregularis ERM and IRM. (A) R. irregularis ERM and mycorrhizal carrot roots (IRM) were grown in compartmented monoxenic cultures (in vitro culture system). (B) R. irregularis ERM and mycorrhizal chicory roots (IRM) were grown in the whole plant bidimensional experimental system (in vivo culture system). Relative gene expression levels were calculated by the 2-ΔCT method using RiEF1α as a normalizer. Bars represent standard error. Asterisks show statistically significant differences (P < 0.05; n = 4) between ERM and IRM.
Cu Deficiency Inhibits AM Colonization and Regulates RiCTR Expression in the IRM
To further understand the role of RiCTR1-3 in the intraradical phase of the fungus, their expression levels were analyzed in mycorrhizal carrot roots grown in vitro in monoxenic cultures and in mycorrhizal chicory roots grown in vivo in the sandwich system under Cu-optimal and -deficient conditions. Interestingly, irrespective of the culture method, development of the roots under Cu-deficient conditions decreased mycorrhizal intensity and arbuscule frequency (Figure 4). These results were confirmed by determining the transcript levels of the R. irregularis elongation factor RiEF1α by qRT-PCR (Figure 4).
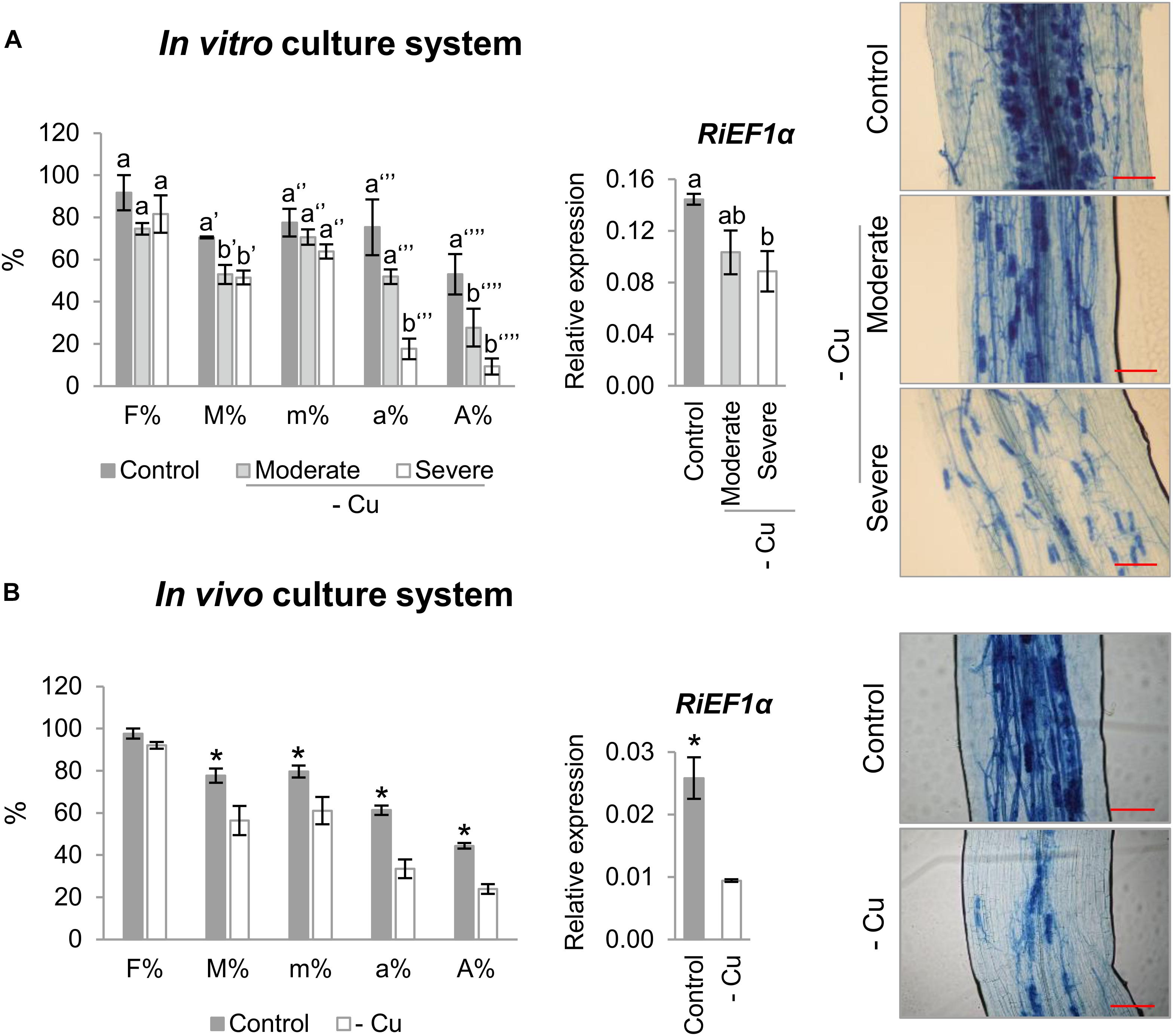
Figure 4. Effect of Cu deficiency on mycorrhizal colonization. (A) Mycorrhizal colonization of carrot roots grown in monoxenic cultures in M media (control, 0.5 μM Cu) or in M media lacking Cu in plates started either with roots and inoculum previously grown in M media containing 0.5 μM CuSO4 (moderate Cu deficiency) or without Cu (severe Cu deficiency). (B) Mycorrhizal colonization of chicory roots grown in the whole plant bidimensional experimental system fertilized with half-strength Hoagland solution (control, 0.16 μM Cu) or with a modified nutrient solution without Cu. Colonization rates were determined by using the Trouvelot method after histochemical staining and by determining the expression level of the R. irregularis elongation factor 1α (RiEF1α). The relative expression of RiEF1α was calculated using the 2-ΔCT method with EF1α of the corresponding host plant as internal control. Bars represent standard error. Different letters indicate significant differences (P < 0.05; n = 4) between treatments and asterisks statistically significant differences (P < 0.05; n = 4) in comparison with the control. F%, frequency of mycorrhiza in the root system; M%, intensity of the mycorrhizal colonization in the root system; m%, intensity of the mycorrhizal colonization in the root fragments; a%, arbuscule abundance in mycorrhizal parts of root fragments; A%, arbuscule abundance in the root system. Scale bar of the left panels: 100 μm.
Cu deficiency increased RiCTR1 expression in the IRM developed in the carrot root organ cultures and in the chicory roots grown in the sandwich system (Figure 5A). However, RiCTR2 expression was only up-regulated in the IRM of the carrot roots grown under the severe Cu deficiency treatment and of the chicory roots fed with a nutrient solution without Cu (Figure 5B). None of the RiCTR3 splicing variants were detected in the mycorrhizal roots grown under Cu-limiting conditions, probably because their low expression levels in the IRM and the decrease in mycorrhizal colonization.
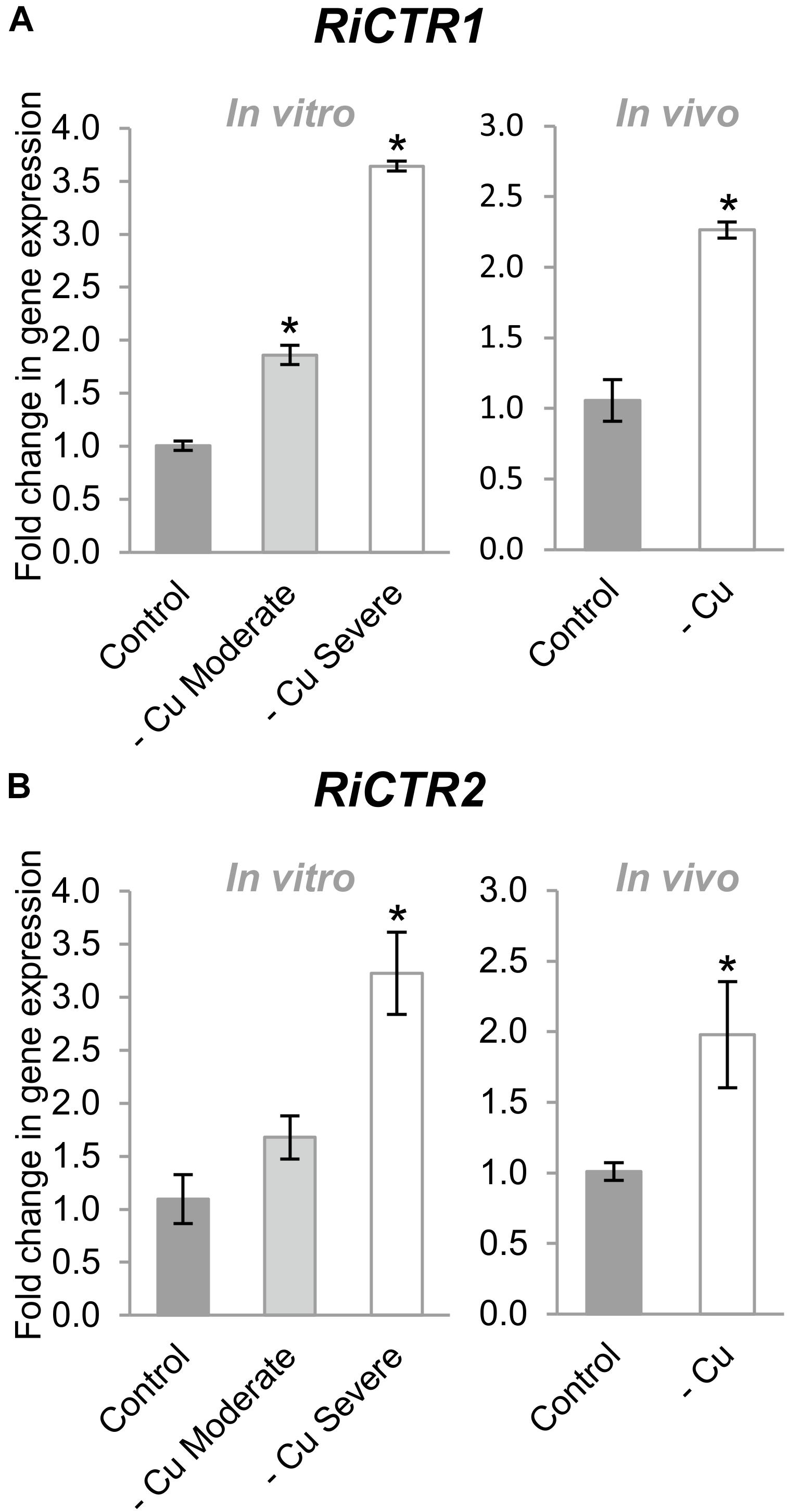
Figure 5. Effect of Cu deficiency on RiCTR1 and RiCTR2 IRM expression. (A) RiCTR1 and (B) RiCTR2 expression in mycorrhizal carrot roots developed in monoxenic cultures in M media (control, 0.5 μM Cu) or in M media lacking Cu in plates started either with roots and inoculum previously grown in M media containing 0.5 μM CuSO4 (moderate Cu deficiency) or without Cu (severe Cu deficiency) (left panel) and in mycorrhizal chicory roots grown in the whole plant bidimensional experimental system and fertilized with half-strength Hoagland solution (control, 0.16 μM Cu) or with a modified nutrient solution without Cu (right panel). Relative gene expression levels were calculated by the 2-ΔΔCT method using RiEF1α as a normalizer. Bars represent standard error. Asterisks show statistically significant differences (P < 0.05; n = 4) in comparison to the corresponding control value.
RiCTRs Expression in the ERM Is Regulated by Cu Availability
To get further insights into the role of the R. irregularis CTR family members on fungal Cu homeostasis, their gene expression patterns were assessed in ERM grown monoxenically under Cu deficient and toxic conditions. Given that development of the ERM was seriously inhibited when the hyphal compartment of the split Petri dishes was supplied with high Cu levels (data not shown), the Cu toxicity treatments were applied by exposing the ERM grown in M media to 250 μM CuSO4 for 1, 2, and 7 days or to 500 μM CuSO4 for 1 and 2 days.
RiCTR1 expression was up-regulated by Cu deficiency and down-regulated by Cu toxicity. A twofold induction was observed in the ERM grown both under moderate and severe Cu limiting conditions (Figure 6A). In contrast, RiCTR2 transcript levels were significantly increased (twofold) only in the ERM grown under the severe Cu-limiting treatment. RiCTR2 expression was not affected by any of the toxic Cu conditions considered in our study (Figure 6B).
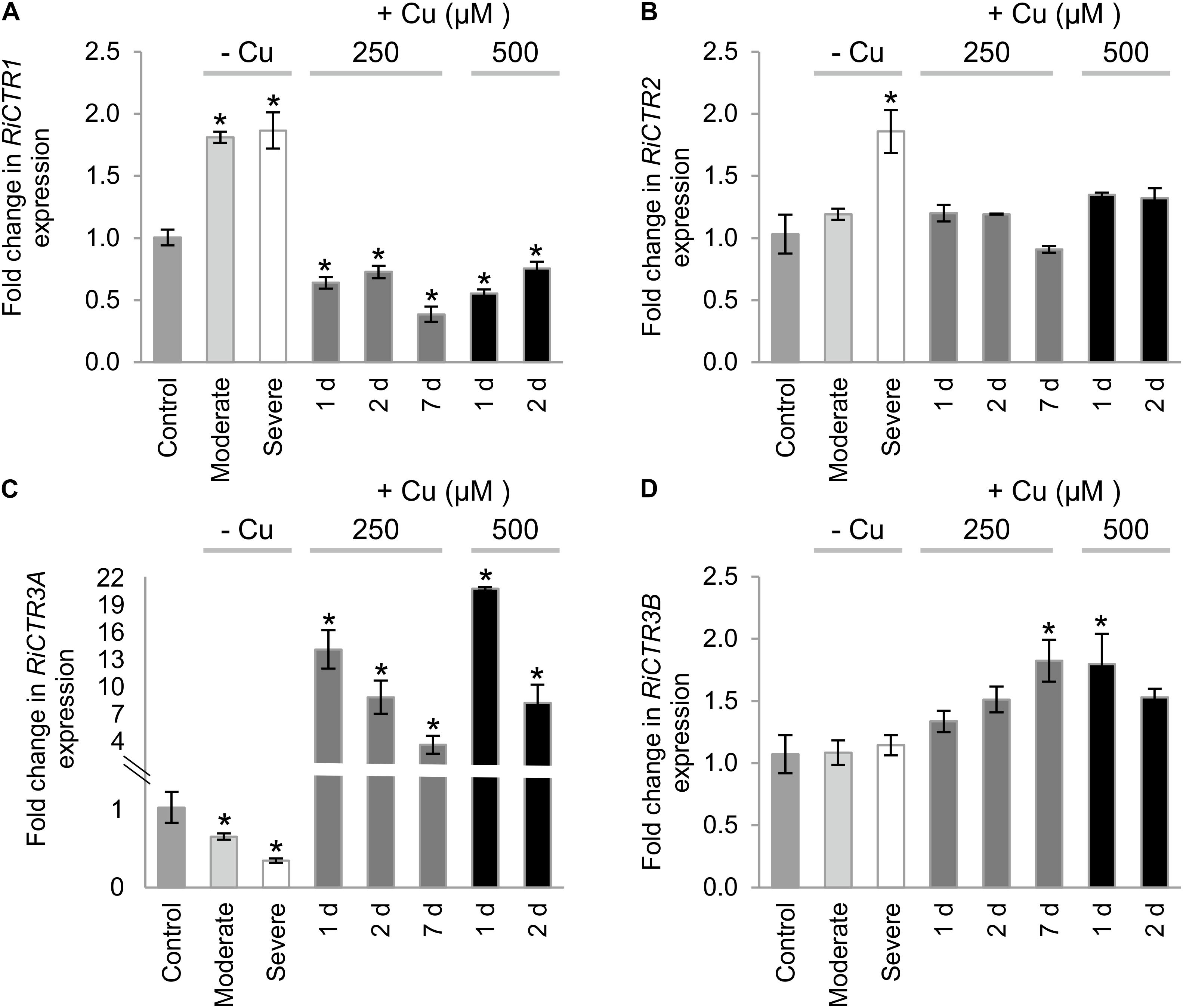
Figure 6. Regulation of RiCTRs expression by Cu availability. R. irregularis ERM was grown in monoxenic cultures in M media containing 0.5 μM CuSO4 (control) or in M media lacking Cu in plates started with roots and inoculum previously grown either in M media containing 0.5 μM CuSO4 (moderate Cu deficiency) or in M media without Cu (severe Cu deficiency). For the Cu toxicity treatments, the ERM grown in optimal M media was exposed for 1, 2, and 7 days to 250 μM CuSO4 or for 1 and 2 days to 500 μM CuSO4. (A) RiCTR1, (B) RiCTR2, (C) RiCTR3A and (D) RiCTR3B gene expression. Relative expression levels were calculated by the 2-ΔΔCT method using RiEF1α as a normalizer. Bars represent standard error. Asterisks show statistically significant differences (P < 0.05; n = 3) in comparison to the corresponding control value.
The expression levels of RiCTR3A and RiCTR3B were similar in the control untreated ERM. Interestingly, RiCTR3A expression was highly up-regulated in ERM subjected to the Cu toxicity treatments and down-regulated in the ERM grown under Cu limiting conditions. RiCTR3A induction by Cu toxicity seemed to be transient, reaching a maximum expression level (>20-fold induction) in the ERM exposed to 500 μM CuSO4 for 1 day (Figure 6C). This expression pattern was unexpected for a gene encoding a protein that transports Cu into the cytosol and suggests a role for RiCTR3A in Cu tolerance. RiCTR3B expression was just slightly up-regulated in the ERM grown for 7 d at 250 μM Cu and for 1 d at 500 μM Cu (Figure 6D). Differential regulation of the two RiCTR3 splicing variants by Cu leads to higher transcript levels of RiCTR3A than of RiCTR3B under Cu toxic levels and to higher transcript levels of RiCTR3B under the severe Cu deficient treatment (Supplementary Figure S6).
RiCTRs Expression Is Regulated by Oxidative Stress
Taking into account that several oxidative-stress response elements were identified in the promoter sequences of the R. irregularis CTR genes and that toxic Cu levels induce an oxidative stress to the ERM (Benabdellah et al., 2009), in an attempt to further understand the role of the R. irregularis CTRs, their gene expression patterns were analyzed in the ERM exposed to H2O2. As a marker of the oxidative stress treatment, the expression of the R. irregularis Cu, Zn superoxide dismutase gene RiSOD1 (González-Guerrero et al., 2010) was determined. Exposure of the ERM to 1 mM H2O2 for 1 h up-regulated RiCTR1, RiCTR2, RiCTR3B and RiSOD1 expression (Figures 7A,B,D,E). However, RiCTR3A expression was not significantly affected by H2O2, which indicates that its activation by Cu was independent of the Cu-induced oxidative stress (Figure 7C). Differential regulation of the two RiCTR3 splicing variants by H2O2 leads to higher RiCTR3B transcript levels in the H2O2-treated ERM (Supplementary Figure S7).
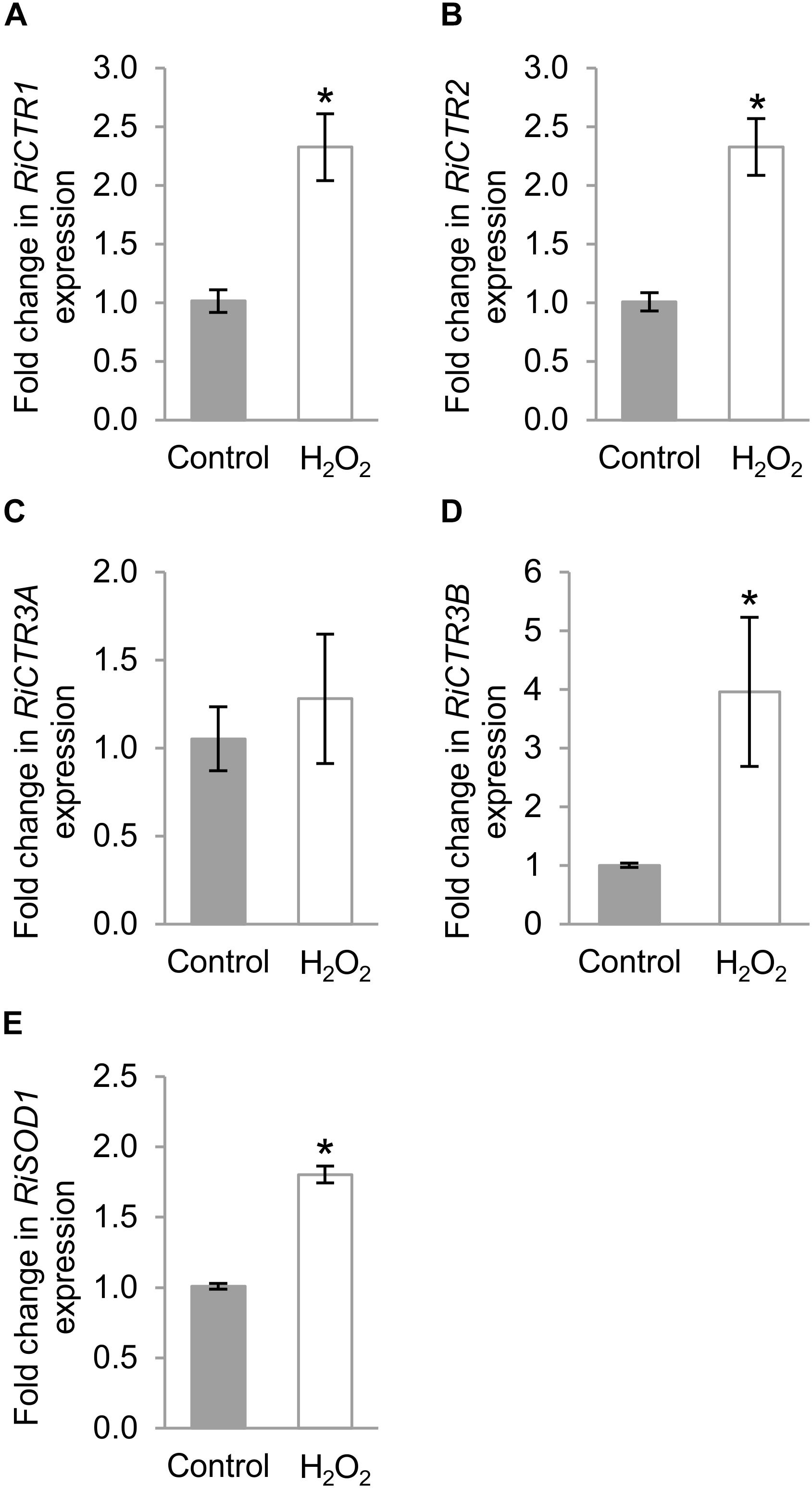
Figure 7. Regulation of RiCTRs expression by oxidative stress. R. irregularis ERM grown in monoxenic cultures in M-C medium was exposed or not (Control) for 1 h to 1 mM H2O2. (A) RiCTR1, (B) RiCTR2, (C) RiCTR3A (D) RiCTR3B, and (E) RiSOD1 gene expression. Relative expression levels were calculated by the 2-ΔΔCT method using RiEF1α as a normalizer. Bars represent standard error. Asterisks show statistically significant differences (P < 0.05; n = 3) in comparison to the control value.
RiCTR3A Enhances Metal Tolerance of the yap1Δ Yeast Strain
As a step forward to understand RiCTR3A and RiCTR3B function and taking into account that their transcript levels were regulated by Cu toxicity or H2O2, we assessed their capability to rescue metal and H2O2 sensitivity of the yap1Δ S. cerevisiae strain lacking the transcriptional regulator Yap1 that mediates cell’s response to oxidants and metals. Neither the empty vector-transformed cells nor the RiCTR3B-expressing cells were able to grow on SD media supplemented with Cu or H2O2 (Figure 8A). However, RiCTR3A rescued the growth defect of the mutant yeast in media supplemented with 1.5 mM CuSO4 but not the inability of the yap1Δ cells to grow in the presence of 0.5 mM H2O2 (Figure 8A). These data indicate that RiCTR3A plays, at least in the heterologous system, a role in Cu tolerance. To determine RiCTR3A subcellular location in yap1Δ, N- and C-terminal GFP-tagged versions of RiCTR3A were expressed in the mutant yeast cells. However, only GFP-RiCTR3A remained functional (Figure 8B). This fusion protein was localized to the yeast plasma membrane. Additionally, as usually occurs when transport proteins are overexpressed in yeast, a perinuclear fluorescence pattern indicative of endoplasmic reticulum localization was observed (Figure 8C).
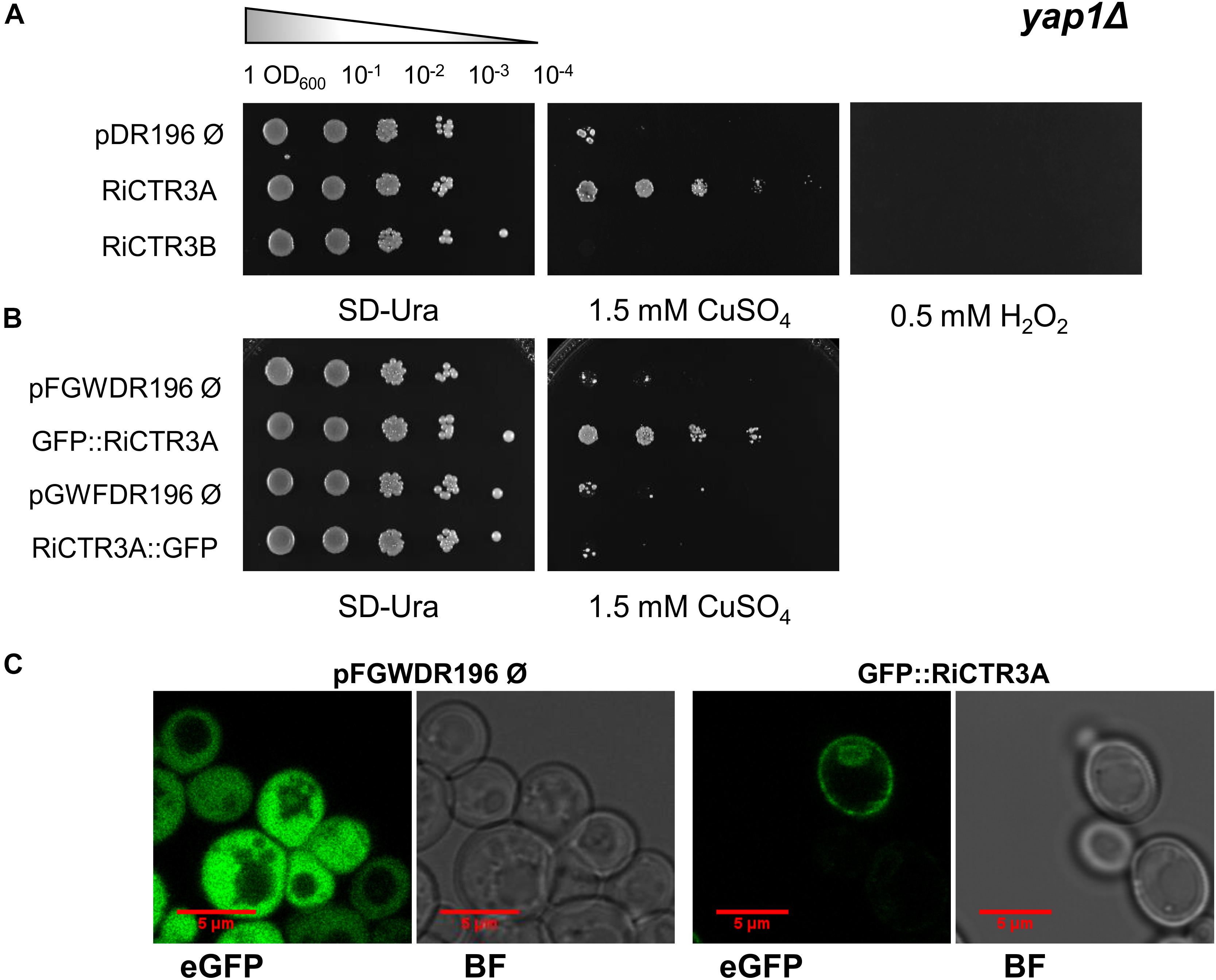
Figure 8. Functional analysis of RiCTR3A and RiCTR3B in the Δ-yap1 S. cerevisiae mutant. (A) Δ-yap1 cells transformed with the empty vector or expressing RiCTR3A or RiCTR3B were plated on SD media supplemented or not with 1.5 mM CuSO4 or with 0.5 mM H2O2. (B) Δ-yap1 cells transformed with the corresponding empty vector expressing GFP or N-terminal or C-terminal GFP-tagged versions of RiCTR3A were plated on SD media supplemented or not with 1.5 mM CuSO4. Plates were incubated 4 days at 30°C. (C) Δ-yap1 cells expressing GFP (the empty vector pFGWDR196) and GFP::RiCTR3A were visualized with a confocal microscope. eGFP, enhanced GFP fluorescence; BF, bright field.
Discussion
The ability of AM fungi to acquire Cu from the soil and to transfer it to their host plants has been shown in several physiological studies. Whereas much progress has been made in understanding the mechanisms of phosphorus and nitrogen transport in the AM symbiosis, very little is known about the mechanisms of Cu acquisition by AM fungi. Here, we characterize for the first time the Cu transporters of the CTR family in an AM fungus. Our data strongly suggest that R. irregularis acquires Cu (I) from the soil through the activity of RiCTR1, a plasma membrane Cu transporter that is highly expressed in the ERM, and that RiCTR2 and RiCTR3A play a role in Cu homeostasis in R. irregularis.
A previous genome-wide analysis of Cu transporters in R. irregularis revealed the presence of three candidate gene sequences, RiCTR1, RiCTR2, and RiCTR3, encoding transporters belonging to the CTR family (Tamayo et al., 2014). Interestingly, our RACE approach identified two RiCTR3 transcripts, which result from an alternative splicing event through the retention of the first intron in its coding sequence, the most common alternative splicing type described in fungi (Grutzmann et al., 2014; Gonzalez-Hilarion et al., 2016). Alternative splicing is a common mechanism used to produce multiple proteins from a single gene, thereby increasing the proteome size of an organism (Black, 2003; Benabdellah et al., 2007; Kornblihtt et al., 2013; Mockenhaupt and Makeyev, 2015). In addition, it can influence gene expression through its impact on different stages of mRNA metabolism including transcription, polyadenylation, nuclear mRNA export, translation efficiency and the rate of mRNA decay (Le Hir et al., 2003; Jacob and Smith, 2017). Although functionality of alternative splicing is poorly understood in fungi, it seems that usually leads to non-functional isoforms, providing an additional mechanism to regulate the overall expression of a gene (Goebels et al., 2013; Grutzmann et al., 2014; Gonzalez-Hilarion et al., 2016; Jin et al., 2017; Sieber et al., 2018). However, the extent and biological significance of this process in AM fungi is currently unknown.
CTR proteins contain three TM regions, with a characteristic MetXXXMet motif located in the second TM domain that is absolutely necessary for Cu transport, and an amino-terminal region rich in Met motifs (De Feo et al., 2007). Although most of these methionine motifs are dispensable for Cu transport, a Met/Cys-X-Met motif near the first TM domain is essential for function (Puig et al., 2002). Our in silico analyses revealed that out of the four identified RiCTR open reading frames, only RiCTR1 and RiCTR2 present all the structural features of CTR proteins. In fact, these two proteins were the only R. irregularis CTRs displaying Cu transport activity in the heterologous system. The finding that RiCTR1 reverts the mutant phenotype of the ctr1Δctr3Δ strain lacking the high affinity plasma membrane Cu transporters Ctr1 and Ctr3 indicates that RiCTR1 encodes a plasma membrane Cu transporter that transports Cu (I). Localization of RiCTR1 in the yeast plasma membrane and RCTR1 expression patterns in the ERM in response to external Cu, that is up-regulation by Cu deficiency and down-regulation by Cu toxicity, supports this hypothesis. Although RiCTR2 subcellular localization could not be demonstrated in the heterologous system, it seems to be the functional ortholog of the S. cerevisiae vacuolar Cu transporter Ctr2, as it complemented the growth defect of the triple CTR mutant yeast ctr1Δctr2Δctr3Δ lacking both the vacuolar and plasma membrane transporters but not of the double mutant lacking only the plasma membrane transporters. These data strongly suggest a role for RiCTR2 in mobilization of vacuolar Cu stores, which is supported by up-regulation of RiCTR2 expression when the ERM was grown under the severe Cu deficient conditions. Therefore, as it has been shown for the S. cerevisiae plasma membrane transporters Ctr1 and Ctr3 (Dancis et al., 1994a; Peña et al., 2000) and the vacuolar transporter Ctr2 (Rees et al., 2004), RiCTR1 is required to facilitate Cu acquisition under Cu deficient conditions and RiCTR2 to mobilize Cu vacuolar stores when Cu levels are extremely low.
Interestingly, RiCTR1 and RiCTR2 transcript levels raised in the ERM in response to H2O2. A potential explanation could be that under these conditions RiCTR1 and RiCTR2 are needed to increase Cu availability for Cu/Zn-superoxide dismutase, one of the cofactors needed for its reactive oxygen species scavenging activity. In fact, yeast cells lacking CTR transporters show oxidative stress sensitive phenotypes linked with an insufficient delivery of Cu, either from the external environment or from the vacuolar reserves, to the Cu/Zn superoxide dismutase (Dancis et al., 1994a; Knight et al., 1996). These data suggest, therefore, a role for Cu in oxidative stress protection in R. irregularis.
As reported for the R. irregularis genes RiPT (Fiorilli et al., 2013) RiAMT1-3 (Pérez-Tienda et al., 2011; Calabrese et al., 2016) and RiFTR1 (Tamayo et al., 2018) encoding, respectively, plasma membrane phosphate, ammonium and iron transporters, RiCTR1 and RiCTR2 mRNAs were detected in the IRM. Expression of RiCTR1 in the IRM suggests, as it has been proposed for the other fungal transporters, that there might exist a competition between the plant and the fungus for the Cu present in the apoplast of the symbiotic interface (Balestrini et al., 2007; Kiers et al., 2011). It is tempting to hypothesize that during its in planta phase, the fungus needs to take up Cu from the interfacial apoplast to meet its Cu demands for growth and activity. This hypothesis is supported by the observed increase of the RiCTR1 transcript levels in the IRM when the symbiosis was developed under Cu-limiting conditions. Under these conditions, Cu released by the fungus into the apoplast of the arbuscular interface should be perceived not only by the plant but also by the fungus. Further experiments are needed to understand how Cu homeostasis is regulated at the symbiotic interface, a process that will require fine-tuning between the plant and the fungus and that will depend on the Cu status of both symbionts. The high expression levels of RiCTR2 in the IRM together with its up-regulation when the symbiosis was developed under Cu-deficient conditions suggest that the fungus needs to mobilize its vacuolar Cu reserves to support its growth and metabolism. Overall, these data indicate that the fungus has a high Cu demand for growth and activity within the roots, which is supported by the observation that root colonization and arbuscule development are inhibited when the symbiosis was developed under Cu-deficient conditions. The requirement of Cu for AM fungal colonization it is not surprising given that this transition metal is an essential micronutrient that acts as cofactor of key enzymes involved in a wide array of biochemical processes essential for growth (Pena et al., 1999; Festa and Thiele, 2011).
Unlike RiCTR1 and RiCTR2, none of the RiCTR3 gene products seem to have a role in Cu transport. RiCTR3A presents the typical topology of CTR proteins but lacks the conserved Met/Cys-X-Met motif near the first TM domain that is strictly required for Cu transport; and RiCTR3B has a single TM domain. As expected, neither RiCTR3A nor RiCTR3B restored the respiratory defect of the CTR mutant yeasts. Furthermore, their gene expression patterns in response to external Cu presented the opposite trend of a protein that mediates Cu transport into the cytosol, as both were transiently up-regulated when the ERM was exposed to high Cu levels. The strong up-regulation of RiCTR3A expression in the Cu-treated ERM together with the capability of its gene product to revert metal sensitivity of the Δyap-1 yeast cells suggest that RiCTR3A is involved in metal tolerance in the ERM. Given that RiCTR3A was unable to complement oxidative stress sensitivity of the Δyap-1 mutant and that a functional GFP-RiCTR3A fusion protein was localized to the Δyap-1 plasma membrane, it is temping to hypothesize that RiCTR3A might function as a Cu sensor that activates downstream signal transduction pathways involved in Cu tolerance. Nutrient sensing in fungi is mediated by different classes of plasma membrane proteins that activate downstream signaling pathways, such as non-transporting receptors, transceptors and G-proteins-coupled receptors (Van Dijck et al., 2017). Non-transporting receptors are structural homologs to nutrient transporters that have lost their transport capacity while gaining a receptor function (Conrad et al., 2014). It is believed that these transporter-like proteins are used as sensors for the nutrient they likely once transported previously in evolution. This is the case of the S. cerevisiae glucose receptors Snf3 and Rgt2, structural homologs glucose transporters that sense availability of external glucose but cannot transport glucose (Ozcan et al., 1998), and of the nitrogen receptor SSy1, a member of the amino acid permease family that does not transport amino acids but senses them at the plasma membrane (Klasson et al., 1999). Despite failure of RiCTR3A to complement the mutant phenotype of the yeast CTR mutants could be an artifact of the heterologous system, the absence in its N-terminal end of the Met/Cys-X-Met motif that is strictly required for CTR function supports the hypothesis that RiCTR3A does not have Cu transport activity. Although micronutrient receptors have been not reported yet, the yeast iron transporter Ftr1 and the zinc transporter Ztr1 have recently been identified as the first micronutrient transceptors, since they present both transport and receptor functions (Schothorst et al., 2017). RiCTR3A might be the first described micronutrient receptor. However, further studies are required to confirm this hypothesis.
Unfortunately, we could not assign a role to RiCTR3B, the intron-retaining transcript of RiCTR3. Alternative splicing variants of CTR genes have been previously described in other fungi, such as C. gloeosporioides (Barhoom et al., 2008) and N. crassa (Korripally et al., 2010). However, in contrast with what happens with the protein encoded by RiCTR3B, the predicted proteins of the two spliced variants of the C. gloeosporioides CTR2 gene and of the N. crassa TCU-2 present all the characteristic features of CTR proteins and their gene products are fully functional in the yeast Ctr triple mutant. Since intron retention in RiCTR3B produces a frame shift that generates a premature termination codon, it is possible that the alternative RiCTR3 protein RiCTR3B is not produced. If that were the case, as it has been described for other fungi (Gonzalez-Hilarion et al., 2016), intron retention might be a post-transcriptional mechanism to regulate RiCTR3 gene expression. A systemic genome-wide comparative analysis of alternative splicing in 23 fungal species has revealed that most of the alternative splicing-affected genes encode proteins that mediate the stress response (Grutzmann et al., 2014). Interestingly, RiCTR3A seems to be involved in the ERM response to Cu toxicity. The finding that both RiCTR3 splicing variants were differentially expressed during Cu and oxidative stress agrees with previous observations in several human pathogenic fungi that the expression of a certain isoform is not exclusive to a certain condition and that the ratio between expressed isoforms changes (Sieber et al., 2018). These authors suggested that alternative splicing is important in fungi for adaptation and stress tolerance via the generation of suitable splice variants. The higher expression levels of the RiCTR3A, the transcript lacking the first intron, under Cu toxicity suggests that alternative splicing may be a mechanism to control the activation of the RiCTR3A protein during Cu stress. The finding that RiCTR3B expression increased in the H2O2-exposed ERM suggests that it might play a role in oxidative stress tolerance. However, further studies are needed to determine whether RiCTR3B encodes a functional protein and the significance of the alternative splicing of RiCTR3.
Conclusion
Here, we show for the first time that the AM fungus R. irregularis expresses two genes encoding Cu transporters of the CTR family, RiCTR1 and RiCTR2, and two alternative spliced variants of a third gene, RiCTR3. RiCTR3A, the shortest spliced variant of RiCTR3, encodes a protein that is likely involved in Cu tolerance while RiCTR3B might contribute to oxidative stress protection. Our data also show for the first time the requirement of Cu for AM fungal colonization.
Data Availability
The raw data supporting the conclusions of this manuscript will be made available by the authors, without undue reservation, to any qualified researcher.
Author Contributions
TG-G performed the majority of the experimental work. KB, MM, and AJ-J contributed to RiCTR2 characterization. CA and PB contributed to the protein localization assays. NF defined the research theme, supervised all the experiments, and coordinated the research project. TG and NF contributed to data interpretations and wrote the manuscript. All authors have revised and approved the manuscript.
Funding
This research was supported by the Spanish Ministry of Science, Innovation and Universities (MINECO) through projects AGL2012-35611, AGL2015-67098-R, and RTI2018-098756-B-I00, and by the Consejería de Salud (CS) of the Junta de Andalucía through the research grant PI-0014-2016. TG-G was supported by a Ph.D. contract (FPI) from the MINECO and KB by a Nicolás Monardes (0088/2018) contract. CSIC Library covered part of the open access publication fees.
Conflict of Interest Statement
The authors declare that the research was conducted in the absence of any commercial or financial relationships that could be construed as a potential conflict of interest.
Acknowledgments
We are grateful to Ascensión Valderas for excellent technical assistance.
Supplementary Material
The Supplementary Material for this article can be found online at: https://www.frontiersin.org/articles/10.3389/fpls.2019.00604/full#supplementary-material
Footnotes
- ^ https://www2.dijon.inra.fr/mychintec/Mycocalc-prg/download.html
- ^ https://genome.jgi.doe.gov/portal/
- ^ https://blast.ncbi.nlm.nih.gov/Blast.cgi
- ^ https://www.ebi.ac.uk/Tools/msa/clustalo/
- ^ http://rulai.cshl.edu/SCPD/
- ^ http://rsat-tagc.univ-mrs.fr/rsat/
- ^ http://www.bioinformatics.org/sms2/ident_sim.html
- ^ https://www.ncbi.nlm.nih.gov/Structure/cdd/wrpsb.cgi
- ^ http://www.cbs.dtu.dk/services/TMHMM/
- ^ http://harrier.nagahama-i-bio.ac.jp/sosui/sosui_submit.html
- ^ http://topcons.cbr.su.se/
- ^ https://prosite.expasy.org/mydomains/
References
Aller, S. G., Eng, E. T., De Feo, C. J., and Unger, V. M. (2004). Eukaryotic CTR copper uptake transporters require two faces of the third transmembrane domain for helix packing, oligomerization, and function. J. Biol. Chem. 279, 53435–53441. doi: 10.1074/jbc.M409421200
Balestrini, R., Gómez-Ariza, J., Lanfranco, L., and Bonfante, P. (2007). Laser microdissection reveals that transcripts for five plant and one fungal phosphate transporter genes are contemporaneously present in arbusculated cells. Mol. Plant Microb. Interact. 20, 1055–1062. doi: 10.1094/mpmi-20-9-1055
Barhoom, S., Kupiec, M., Zhao, X., Xu, J.-R., and Sharon, A. (2008). Functional characterization of CgCTR2, a putative vacuole copper transporter that is involved in germination and pathogenicity in Colletotrichum gloeosporioides. Eukaryot. Cell 7, 1098–1108. doi: 10.1128/ec.00109-07
Beaudoin, J., Thiele, D. J., Labbé, S., and Puig, S. (2011). Dissection of the relative contribution of the Schizosaccharomyces pombe Ctr4 and Ctr5 proteins to the copper transport and cell surface delivery functions. Microbiology 157, 1021–1031. doi: 10.1099/mic.0.046854-0
Bellemare, D. R., Shaner, L., Morano, K. A., Beaudoin, J., Langlois, R., and Labbe, S. (2002). Ctr6, a vacuolar membrane copper transporter in Schizosaccharomyces pombe. J. Biol. Chem. 277, 46676–46686. doi: 10.1074/jbc.M206444200
Benabdellah, K., Gonzalez-Rey, E., and Gonzalez, A. (2007). Alternative trans-splicing of the Trypanosoma cruzi LYT1 gene transcript results in compartmental and functional switch for the encoded protein. Mol. Microbiol. 65, 1559–1567. doi: 10.1111/j.1365-2958.2007.05892.x
Benabdellah, K., Merlos, M. A., Azcón-Aguilar, C., and Ferrol, N. (2009). GintGRX1, the first characterized glomeromycotan glutaredoxin, is a multifunctional enzyme that responds to oxidative stress. Fungal Genet. Biol. 46, 94–103. doi: 10.1016/j.fgb.2008.09.013
Beneš, V., Hložková, K., Matěnová, M., Borovička, J., and Kotrba, P. (2016). Accumulation of Ag and Cu in Amanita strobiliformis and characterization of its Cu and Ag uptake transporter genes AsCTR2 and AsCTR3. BioMetals 29, 249–264. doi: 10.1007/s10534-016-9912-x
Black, D. L. (2003). Mechanisms of alternative pre-messenger RNA splicing. Annu. Rev. Biochem. 72, 291–336. doi: 10.1146/annurev.biochem.72.121801.161720
Borghouts, C., Scheckhuber, C. Q., Stephan, O., and Osiewacz, H. D. (2002). Copper homeostasis and aging in the fungal model system Podospora anserina: differential expression of PaCtr3 encoding a copper transporter. Int. J. Biochem. Cell Biol. 34, 1355–1371. doi: 10.1016/S1357-2725(02)00078-X
Calabrese, S., Pérez-Tienda, J., Ellerbeck, M., Arnould, C., Chatagnier, O., Boller, T., et al. (2016). GintAMT3 – a low-affinity ammonium transporter of the arbuscular mycorrhizal Rhizophagus irregularis. Front. Plant Sci. 7:679. doi: 10.3389/fpls.2016.00679
Chabot, S., Bécard, G., and Piché, Y. (1992). Life cycle of Glomus intraradix in root organ culture. Mycologia 84, 315–321. doi: 10.2307/3760183
Chen, E. C. H., Morin, E., Beaudet, D., Noel, J., Yildirir, G., Ndikumana, S., et al. (2018). High intraspecific genome diversity in the model arbuscular mycorrhizal symbiont Rhizophagus irregularis. New Phytol. 220, 1161–1171. doi: 10.1111/nph.14989
Conrad, M., Schothorst, J., Kankipati, H. N., Van Zeebroeck, G., Rubio-Texeira, M., and Thevelein, J. M. (2014). Nutrient sensing and signaling in the yeast Saccharomyces cerevisiae. FEMS Microbiol. Rev. 38, 254–299. doi: 10.1111/1574-6976.12065
Dancis, A., Haile, D., Yuan, D. S., and Klausner, R. D. (1994a). The Saccharomyces cerevisiae copper transport protein (Ctr1p). Biochemical characterization, regulation by copper, and physiologic role in copper uptake. J. Biol. Chem. 269, 25660–25667.
Dancis, A., Yuan, D. S., Haile, D., Askwith, C., Eide, D., Moehle, C., et al. (1994b). Molecular characterization of a copper transport protein in S. cerevisiae: an unexpected role for copper in iron transport. Cell 76, 393–402. doi: 10.1016/0092-8674(94)90345-X
De Feo, C. J., Aller, S. G., and Unger, V. M. (2007). A structural perspective on copper uptake in eukaryotes. BioMetals 20, 705–716. doi: 10.1007/s10534-006-9054-7
Dumay, Q. C., Debut, A. J., Mansour, N. M., and Saier, M. H. Jr. (2006). The copper transporter (Ctr) family of Cu+ uptake systems. J. Mol. Microbiol. Biotechnol. 11, 10–19. doi: 10.1159/000092815
Ferrol, N., Tamayo, E., and Vargas, P. (2016). The heavy metal paradox in arbuscular mycorrhizas: from mechanisms to biotechnological applications. J. Exp. Bot. 67, 6253–6265. doi: 10.1093/jxb/erw403
Festa, R. A., and Thiele, D. J. (2011). Copper: an essential metal in biology. Curr. Biol. 21, R877–R883. doi: 10.1016/j.cub.2011.09.040
Fiorilli, V., Lanfranco, L., and Bonfante, P. (2013). The expression of GintPT, the phosphate transporter of Rhizophagus irregularis, depends on the symbiotic status and phosphate availability. Planta 237, 1267–1277. doi: 10.1007/s00425-013-1842-z
Gietz, R. D., and Schiestl, R. H. (2007). High-efficiency yeast transformation using the LiAc/SS carrier DNA/PEG method. Nat. Protoc. 2, 31–34. doi: 10.1038/nprot.2007.13
Glerum, D. M., Shtanko, A., and Tzagoloff, A. (1996). Characterization of COX17, a yeast gene involved in copper metabolism and assembly of cytochrome oxidase. J. Biol. Chem. 271, 14504–14509. doi: 10.1074/jbc.271.24.14504
Goebels, C., Thonn, A., Gonzalez-Hilarion, S., Rolland, O., Moyrand, F., Beilharz, T. H., et al. (2013). Introns regulate gene expression in Cryptococcus neoformans in a Pab2p dependent pathway. PLoS Genet. 9:e1003686. doi: 10.1371/journal.pgen.1003686
González-Guerrero, M., Oger, E., Benabdellah, K., Azcon-Aguilar, C., Lanfranco, L., and Ferrol, N. (2010). Characterization of a CuZn superoxide dismutase gene in the arbuscular mycorrhizal fungus Glomus intraradices. Curr. Genet. 56, 265–274. doi: 10.1007/s00294-010-0298-y
Gonzalez-Hilarion, S., Paulet, D., Lee, K. T., Hon, C. C., Lechat, P., Mogensen, E., et al. (2016). Intron retention-dependent gene regulation in Cryptococcus neoformans. Sci. Rep. 6:32252. doi: 10.1038/srep32252
Grutzmann, K., Szafranski, K., Pohl, M., Voigt, K., Petzold, A., and Schuster, S. (2014). Fungal alternative splicing is associated with multicellular complexity and virulence: a genome-wide multi-species study. DNA Res. 21, 27–39. doi: 10.1093/dnares/dst038
Guo, Y., Smith, K., Lee, J., Thiele, D. J., and Petris, M. J. (2004). Identification of methionine-rich clusters that regulate copper-stimulated endocytosis of the human Ctr1 copper transporter. J. Biol. Chem. 279, 17428–17433. doi: 10.1074/jbc.M401493200
Halliwell, B., and Gutteridge, J. M. (1984). Oxygen toxicity, oxygen radicals, transition metals and disease. Biochem. J. 219, 1–14.
Helber, N., Wippel, K., Sauer, N., Schaarschmidt, S., Hause, B., and Requena, N. (2011). A versatile monosaccharide transporter that operates in the arbuscular mycorrhizal fungus Glomus sp is crucial for the symbiotic relationship with plants. Plant Cell 23, 3812–3823. doi: 10.1105/tpc.111.089813
Jacob, A. G., and Smith, C. W. J. (2017). Intron retention as a component of regulated gene expression programs. Hum. Genet. 136, 1043–1057. doi: 10.1007/s00439-017-1791-x
Jamison McDaniels, C. P., Jensen, L. T., Srinivasan, C., Winge, D. R., and Tullius, T. D. (1999). The yeast transcription factor Mac1 binds to DNA in a modular fashion. J. Biol. Chem. 274, 26962–26967. doi: 10.1074/jbc.274.38.26962
Jin, L., Li, G., Yu, D., Huang, W., Cheng, C., Liao, S., et al. (2017). Transcriptome analysis reveals the complexity of alternative splicing regulation in the fungus Verticillium dahliae. BMC Genomics 18:130. doi: 10.1186/s12864-017-3507-y
Kay, R., Chan, A., Daly, M., and McPherson, J. (1987). Duplication of CaMV 35S promoter sequences creates a strong enhancer for plant genes. Science 236, 1299–1302. doi: 10.1126/science.236.4806.1299
Kiers, E. T., Duhamel, M., Beesetty, Y., Mensah, J. A., Franken, O., Verbruggen, E., et al. (2011). Reciprocal rewards stabilize cooperation in the mycorrhizal symbiosis. Science 333, 880–882. doi: 10.1126/science.1208473
Kim, B.-E., Nevitt, T., and Thiele, D. J. (2008). Mechanisms for copper acquisition, distribution and regulation. Nat. Chem. Biol. 4, 176–185. doi: 10.1038/nchembio.72
Klasson, H., Fink, G. R., and Ljungdahl, P. O. (1999). Ssy1p and Ptr3p are plasma membrane components of a yeast system that senses extracellular amino acids. Mol. Cell Biol. 19, 5405–5416. doi: 10.1128/MCB.19.8.5405
Knight, S. A., Labbé, S., Kwon, L. F., Kosman, D. J., and Thiele, D. J. (1996). A widespread transposable element masks expression of a yeast copper transport gene. Genes Dev. 10, 1917–1929. doi: 10.1101/gad.10.15.1917
Kobayashi, Y., Maeda, T., Yamaguchi, K., Kameoka, H., Tanaka, S., Ezawa, T., et al. (2018). The genome of Rhizophagus clarus HR1 reveals a common genetic basis for auxotrophy among arbuscular mycorrhizal fungi. BMC Genomics 19:465. doi: 10.1186/s12864-018-4853-0
Kornblihtt, A. R., Schor, I. E., Allo, M., Dujardin, G., Petrillo, E., and Munoz, M. J. (2013). Alternative splicing: a pivotal step between eukaryotic transcription and translation. Nat. Rev. Mol. Cell Biol. 14, 153–165. doi: 10.1038/nrm3525
Korripally, P., Tiwari, A., Haritha, A., Kiranmayi, P., and Bhanoori, M. (2010). Characterization of Ctr family genes and the elucidation of their role in the life cycle of Neurospora crassa. Fungal Genet. Biol. 47, 237–245. doi: 10.1016/j.fgb.2009.12.006
Kropat, J., Tottey, S., Birkenbihl, R. P., Depege, N., Huijser, P., and Merchant, S. (2005). A regulator of nutritional copper signaling in Chlamydomonas is an SBP domain protein that recognizes the GTAC core of copper response element. Proc. Natl. Acad. Sci. U.S.A. 102, 18730–18735. doi: 10.1073/pnas.0507693102
Kuge, S., and Jones, N. (1994). YAP1 dependent activation of TRX2 is essential for the response of Saccharomyces cerevisiae to oxidative stress by hydroperoxides. EMBO J. 13, 655–664.
Lanfranco, L., Fiorilli, V., and Gutjahr, C. (2018). Partner communication and role of nutrients in the arbuscular mycorrhizal symbiosis. New Phytol. 220, 1031–1046. doi: 10.1111/nph.15230
Le Hir, H., Nott, A., and Moore, M. J. (2003). How introns influence and enhance eukaryotic gene expression. Trends Biochem. Sci. 28, 215–220. doi: 10.1016/s0968-0004(03)00052-5
Lee, Y.-J., and George, E. (2005). Contribution of mycorrhizal hyphae to the uptake of metal cations by cucumber plants at two levels of phosphorus supply. Plant Soil 278, 361–370. doi: 10.1007/s11104-005-0373-1
Lehmann, A., and Rillig, M. C. (2015). Arbuscular mycorrhizal contribution to copper, manganese and iron nutrient concentrations in crops – a meta-analysis. Soil Biol. Biochem. 81, 147–158. doi: 10.1016/j.soilbio.2014.11.013
Li, X.-L., Marschner, H., and George, E. (1991). Acquisition of phosphorus and copper by VA-mycorrhizal hyphae and root-to-shoot transport in white clover. Plant Soil 136, 49–57. doi: 10.1007/bf02465219
Livak, K. J., and Schmittgen, T. D. (2001). Analysis of relative gene expression data using real-time quantitative PCR and the 2(-Delta Delta C(T)) method. Methods 25, 402–408. doi: 10.1006/meth.2001.1262
Macomber, L., and Imlay, J. A. (2009). The iron-sulfur clusters of dehydratases are primary intracellular targets of copper toxicity. Proc. Natl. Acad. Sci. U.S.A. 106, 8344–8349. doi: 10.1073/pnas.0812808106
Marvin, M. E., Williams, P. H., and Cashmore, A. M. (2003). The Candida albicans CTR1 gene encodes a functional copper transporter. Microbiology 149, 1461–1474. doi: 10.1099/mic.0.26172-0
Merlos, M. A., Zitka, O., Vojtech, A., Azcón-Aguilar, C., and Ferrol, N. (2016). The arbuscular mycorrhizal fungus Rhizophagus irregularis differentially regulates the copper response of two maize cultivars differing in copper tolerance. Plant Sci. 253, 68–76. doi: 10.1016/j.plantsci.2016.09.010
Mockenhaupt, S., and Makeyev, E. V. (2015). Non-coding functions of alternative pre-mRNA splicing in development. Semin. Cell Dev. Biol. 4, 32–39. doi: 10.1016/j.semcdb.2015.10.018
Morin, E., Miyauchi, S., San Clemente, H., Chen, E. C., Pelin, A., de la Providencia, I., et al. (2019). Comparative genomics of Rhizophagus irregularis, R. cerebriforme, R. diaphanus and Gigaspora rosea highlights specific genetic features in Glomeromycotina. New Phytol. 222, 1584–1598. doi: 10.1111/nph.15687
Nakagawa, Y., Kikuchi, S., Sakamoto, Y., and Yano, A. (2010). Identification and characterization of CcCTR1, a copper uptake transporter-like gene, in Coprinopsis cinerea. Microbiol. Res. 165, 276–287. doi: 10.1016/j.micres.2009.05.004
Ozcan, S., Dover, J., and Johnston, M. (1998). Glucose sensing and signaling by two glucose receptors in the yeast Saccharomyces cerevisiae. EMBO J. 17, 2566–2573. doi: 10.1093/emboj/17.9.2566
Pena, M. M., Lee, J., and Thiele, D. J. (1999). A delicate balance: homeostatic control of copper uptake and distribution. J. Nutr. 129, 1251–1260. doi: 10.1093/jn/129.7.1251
Peña, M. M. O., Koch, K. A., and Thiele, D. J. (1998). Dynamic regulation of copper uptake and detoxification genes in Saccharomyces cerevisiae. Mol. Cell. Biol. 18, 2514–2523.
Peña, M. M. O., Puig, S., and Thiele, D. J. (2000). Characterization of the Saccharomyces cerevisiae high affinity copper transporter Ctr3. J. Biol. Chem. 275, 33244–33251. doi: 10.1074/jbc.M005392200
Penas, M. M., Azparren, G., Dominguez, A., Sommer, H., Ramirez, L., and Pisabarro, A. G. (2005). Identification and functional characterisation of ctr1, a Pleurotus ostreatus gene coding for a copper transporter. Mol. Genet. Genomics 274, 402–409. doi: 10.1007/s00438-005-0033-4
Pepe, A., Sbrana, C., Ferrol, N., and Giovannetti, M. (2017). An in vivo whole-plant experimental system for the analysis of gene expression in extraradical mycorrhizal mycelium. Mycorrhiza 27, 659–668. doi: 10.1007/s00572-017-0779-7
Pérez-Tienda, J., Testillano, P. S., Balestrini, R., Fiorilli, V., Azcón-Aguilar, C., and Ferrol, N. (2011). GintAMT2, a new member of the ammonium transporter family in the arbuscular mycorrhizal fungus Glomus intraradices. Fungal Genet. Biol. 48, 1044–1055. doi: 10.1016/j.fgb.2011.08.003
Phillips, J. M., and Hayman, D. S. (1970). Improved procedures for clearing roots and staining parasitic and vesicular-arbuscular mycorrhizal fungi for rapid assessment of infection. Trans. Br. Mycol. Soc. 55, 158–161. doi: 10.1016/S0007-1536(70)80110-3
Portnoy, M. E., Schmidt, P. J., Rogers, R. S., and Culotta, V. C. (2001). Metal transporters that contribute copper to metallochaperones in Saccharomyces cerevisiae. Mol. Genet. Genomics 265, 873–882. doi: 10.1007/s004380100482
Pozo, M. J., Jung, S. C., Martínez-Medina, A., López-Ráez, J. A., Azcón-Aguilar, C., and Barea, J.-M. (2013). “Root allies: arbuscular mycorrhizal fungi help plants to cope with biotic stresses,” in Symbiotic Endophytes, ed. R. Aroca (Berlin: Springer), 289–307.
Puig, S. (2014). Function and regulation of the plant COPT family of high-affinity copper transport proteins. Adv. Bot. 2014:476917. doi: 10.1155/2014/476917
Puig, S., Lee, J., Lau, M., and Thiele, D. J. (2002). Biochemical and genetic analyses of yeast and human high affinity copper transporters suggest a conserved mechanism for copper uptake. J. Biol. Chem. 277, 26021–26030. doi: 10.1074/jbc.M202547200
Puig, S., and Thiele, D. J. (2002). Molecular mechanisms of copper uptake and distribution. Curr. Opin. Chem. Biol. 6, 171–180. doi: 10.1016/S1367-5931(02)00298-3
Rees, E. M., Lee, J., and Thiele, D. J. (2004). Mobilization of intracellular copper stores by the Ctr2 vacuolar copper transporter. J. Biol. Chem. 279, 54221–54229. doi: 10.1074/jbc.M411669200
Ruiz-Lozano, J. M. (2003). Arbuscular mycorrhizal symbiosis and alleviation of osmotic stress. New perspectives for molecular studies. Mycorrhiza 13, 309–317. doi: 10.1007/s00572-003-0237-6
Schothorst, J., Zeebroeck, G. V., and Thevelein, J. M. (2017). Identification of Ftr1 and Zrt1 as iron and zinc micronutrient transceptors for activation of the PKA pathway in Saccharomyces cerevisiae. Microb. Cell 4, 74–89. doi: 10.15698/mic2017.03.561
Sieber, P., Voigt, K., Kämmer, P., Brunke, S., Schuster, S., and Linde, J. (2018). Comparative study on alternative splicing in human fungal pathogens suggests its involvement during host invasion. Front. Microbiol. 9:2313. doi: 10.3389/fmicb.2018.02313
Smith, A. D., Logeman, B. L., and Thiele, D. J. (2017). Copper acquisition and utilization in fungi. Annu. Rev. Microbiol. 71, 597–623. doi: 10.1146/annurev-micro-030117-020444
Spatafora, J. W., Chang, Y., Benny, G. L., Lazarus, K., Smith, M. E., Berbee, M. L., et al. (2016). A phylum-level phylogenetic classification of zygomycete fungi based on genome-scale data. Mycologia 108, 1028–1046. doi: 10.3852/16-042
St-Arnaud, M., Hamel, C., Vimard, B., Caron, M., and Fortin, J. A. (1996). Enhanced hyphal growth and spore production of the arbuscular mycorrhizal fungus Glomus intraradices in an in vitro system in the absence of host roots. Mycol. Res. 100, 328–332. doi: 10.1016/S0953-7562(96)80164-X
Sun, X., Chen, W., Ivanov, S., MacLean, A. M., Wight, H., Ramaraj, T., et al. (2019). Genome and evolution of the arbuscular mycorrhizal fungus Diversispora epigaea (formerly Glomus versiforme) and its bacterial endosymbionts. New Phytol. 221, 1556–1573. doi: 10.1111/nph.15472
Tamayo, E., Gómez-Gallego, T., Azcón-Aguilar, C., and Ferrol, N. (2014). Genome-wide analysis of copper, iron and zinc transporters in the arbuscular mycorrhizal fungus Rhizophagus irregularis. Front. Plant Sci. 5:547. doi: 10.3389/fpls.2014.00547
Tamayo, E., Knight, S. A. B., Valderas, A., Dancis, A., and Ferrol, N. (2018). The arbuscular mycorrhizal fungus Rhizophagus irregularis uses a reductive iron assimilation pathway for high-affinity iron uptake. Environ. Microbiol. 20, 1857–1872. doi: 10.1111/1462-2920.14121
Toone, W. M., and Jones, N. (1999). AP-1 transcription factors in yeast. Curr. Opin. Genet. Dev. 9, 55–61. doi: 10.1016/S0959-437X(99)80008-2
Trouvelot, A., Kough, J., and Gianinazzi-Pearson, V. (1986). “Evaluation of VA infection levels in root systems. Research for estimation methods having a functional significance,” in Physiological and Genetical Aspects of Mycorrhizae, eds V. Gianinazzi-Pearsonand and S. Gianinazzi (Paris: INRA), 217–221.
Van Dijck, P., Brown, N. A., Goldman, G. H., Rutherford, J., Xue, C., and Van Zeebroeck, G. (2017). Nutrient sensing at the plasma membrane of fungal cells. Microbiol. Spectr. 5, 419–439. doi: 10.1128/microbiolspec.FUNK-0031-2016
Wu, X., Sinani, D., Kim, H., and Lee, J. (2009). Copper transport activity of yeast Ctr1 is down-regulated via its C terminus in response to excess copper. J. Biol. Chem. 284, 4112–4122. doi: 10.1074/jbc.M807909200
Xiao, Z., Loughlin, F., George, G. N., Howlett, G. J., and Wedd, A. G. (2004). C-terminal domain of the membrane copper transporter Ctr1 from Saccharomyces cerevisiae binds four Cu(I) ions as a cuprous-thiolate polynuclear cluster: sub-femtomolar Cu(I) affinity of three proteins involved in copper trafficking. J. Am. Chem. Soc. 126, 3081–3090. doi: 10.1021/ja0390350
Keywords: arbuscular mycorrhizal fungi, copper homeostasis, copper transporters, CTR family, Rhizophagus irregularis, symbiosis
Citation: Gómez-Gallego T, Benabdellah K, Merlos MA, Jiménez-Jiménez AM, Alcon C, Berthomieu P and Ferrol N (2019) The Rhizophagus irregularis Genome Encodes Two CTR Copper Transporters That Mediate Cu Import Into the Cytosol and a CTR-Like Protein Likely Involved in Copper Tolerance. Front. Plant Sci. 10:604. doi: 10.3389/fpls.2019.00604
Received: 26 February 2019; Accepted: 24 April 2019;
Published: 16 May 2019.
Edited by:
Alessandra Salvioli, University of Turin, ItalyReviewed by:
Philipp Franken, University of Applied Sciences Erfurt, GermanyIlaria Colzi, University of Florence, Italy
Copyright © 2019 Gómez-Gallego, Benabdellah, Merlos, Jiménez-Jiménez, Alcon, Berthomieu and Ferrol. This is an open-access article distributed under the terms of the Creative Commons Attribution License (CC BY). The use, distribution or reproduction in other forums is permitted, provided the original author(s) and the copyright owner(s) are credited and that the original publication in this journal is cited, in accordance with accepted academic practice. No use, distribution or reproduction is permitted which does not comply with these terms.
*Correspondence: Nuria Ferrol, bnVyaWEuZmVycm9sQGVlei5jc2ljLmVz
†Present address: Miguel A. Merlos, Department of Chemistry and Biochemistry, Mendel University in Brno, Brno, Czechia
Ana M. Jiménez-Jiménez, Central European Institute of Technology, Brno University of Technology, Brno, Czechia