- 1Department of Botany, Rajiv Gandhi University, Itanagar, India
- 2Department of Botany, University of Delhi, New Delhi, India
Modern agriculture is facing twin challenge of ensuring global food security and executing it in a sustainable manner. However, the rapidly expanding salinity stress in cultivable areas poses a major peril to crop yield. Among various biotechnological techniques being used to reduce the negative effects of salinity, the use of arbuscular mycorrhizal fungi (AMF) is considered to be an efficient approach for bio-amelioration of salinity stress. AMF deploy an array of biochemical and physiological mechanisms that act in a concerted manner to provide more salinity tolerance to the host plant. Some of the well-known mechanisms include improved nutrient uptake and maintenance of ionic homeostasis, superior water use efficiency and osmoprotection, enhanced photosynthetic efficiency, preservation of cell ultrastructure, and reinforced antioxidant metabolism. Molecular studies in past one decade have further elucidated the processes involved in amelioration of salt stress in mycorrhizal plants. The participating AMF induce expression of genes involved in Na+ extrusion to the soil solution, K+ acquisition (by phloem loading and unloading) and release into the xylem, therefore maintaining favorable Na+:K+ ratio. Colonization by AMF differentially affects expression of plasma membrane and tonoplast aquaporins (PIPs and TIPs), which consequently improves water status of the plant. Formation of AM (arbuscular mycorrhiza) surges the capacity of plant to mend photosystem-II (PSII) and boosts quantum efficiency of PSII under salt stress conditions by mounting the transcript levels of chloroplast genes encoding antenna proteins involved in transfer of excitation energy. Furthermore, AM-induced interplay of phytohormones, including strigolactones, abscisic acid, gibberellic acid, salicylic acid, and jasmonic acid have also been associated with the salt tolerance mechanism. This review comprehensively covers major research advances on physiological, biochemical, and molecular mechanisms implicated in AM-induced salt stress tolerance in plants. The review identifies the challenges involved in the application of AM in alleviation of salt stress in plants in order to improve crop productivity.
Introduction
Worldwide, soil salinity is becoming a significant problem as it is encountered in all climates. Amongst the various salts present in the soil, NaCl is the most prevalent one. Soils are rendered saline due to deposition of salt either by natural (primary) or anthropogenic (secondary) processes. Primary processes include weathering of parent rocks, deposition from sea water and atmospheric deposition. Secondary processes include poor drainage facilities, irrigation with brackish groundwater, continuous irrigation for long durations, improper management of water, and cultural methods in irrigated agriculture. In addition, growing annual plants with shallow root-systems in place of deep-rooted perennial flora raises the water table leading to rise of saline groundwater (Food and Agriculture Organization [FAO], 2015). It is projected that around one billion hectares (ha) traversing more than 100 countries encounter salinity problems (Food and Agriculture Organization [FAO], 2015). Soil salinity is rapidly increasing with an estimated yearly addition of 0.3–1.5 million ha of farmland thereby decreasing crop production by more than 20% (Porcel et al., 2012; Food and Agriculture Organization [FAO], 2015). It also renders another 20–46 million ha with decreased capacity for production. On the other hand, the earth is home to 7.7 billion people with addition of 83 million people every year at the rate of 1.09% (United Nations [UN], 2018). Therefore, achieving food security for the growing population amidst the declining farmland is one of the most important missions for modern agriculture.
High salt deposition in the soil results in osmotic as well as specific ion effects, which further lead to secondary oxidative stress in plants. Thus, salinity shows adverse effects on germination, growth, and reproduction of plants that subsequently diminish crop yield (Chinnusamy et al., 2005).
Plants have evolved to be a highly flexible system which can adjust its morphological, physiological, biochemical, and molecular mechanisms to survive and sustain the changing environment. To counter the problems of salinity, plants exhibit growth plasticity (morphological and developmental pattern change), accumulation of compatible osmolytes to maintain turgor as well as prevent ultrastructural damage, ion-homeostasis, regulation of water uptake and enhanced water use efficiency, enhanced photosynthesis, detoxification of ROS through antioxidant enzymes and molecules, and induction of phytohormones (Munns and Tester, 2008; Ruiz-Lozano et al., 2012; Augé et al., 2014). However, these adaptive strategies become inefficient to cope with the rapidly increasing salinity.
Arbuscular mycorrhizal fungi establish a symbiotic union with roots of 80% land plants (Smith and Read, 2008). This symbiosis constitutes a distinctive system with more efficiency than roots alone for uptake and transfer of mineral nutrients from the soil (Ruiz-Lozano et al., 2012). The plant associated extramatrical hyphae of AMF can extent up to 100 m g-1 of soil, and enhance the plant’s ability to explore soil. These hyphae are leaner than roots, hence facilitate mining of water-filled pores, which otherwise are inaccessible to roots (Smith et al., 2010). Owing to this, AM can boost several mechanisms in plant to manage salt stress (Evelin et al., 2009; Ruiz-Lozano et al., 2012). Formation of AM has been reported to – (i) improve nutrient acquisition and maintain ionic homeostasis; (ii) improve water uptake and maintain osmotic equilibrium in plants; (iii) induce antioxidant system to prevent damage by ROS; (iv) protect photosynthetic apparatus and enhance photosynthetic efficiency; and (v) modulate phytohormone profile to minimize salt effects on growth and development (Figure 1) (Evelin et al., 2009, 2012; Ruiz-Lozano et al., 2012; Augé et al., 2014; Khalloufi et al., 2017). These effects act in coordination to improve plant’s resilience to salinity stress. These ameliorative effects can be evaluated in terms of improved plant growth exhibited by mycorrhizal (M) in comparison to NM plants. In the past decade, significant progress has been made to understand these mechanisms. This review comprehensively covers all biochemical and physiological changes that occur in plants that are inoculated with AM fungi and exposed to salt stress. It essentially takes stock of the advances made in the last decade toward better understanding of the mechanisms that contribute to salt stress alleviation in M plants. These include molecular bases for higher K+:Na+ ratio and higher concentration of N in M plants via regulation of transporters; maintenance of efficient water status via differential regulation of aquaporin genes (PIPs and TIPs); and elucidation of better photosynthetic efficiency via upregulation of RuBisCo gene as well as protection of PSII by upregulation of genes encoding D1 and D2 proteins of PSII. It updates the role of AM in influencing interplay of phytohormones in plants subjected to salt stress. Finally, it identifies the gaps in the understanding of the mechanisms, and presents the research challenges to be met in the future studies.
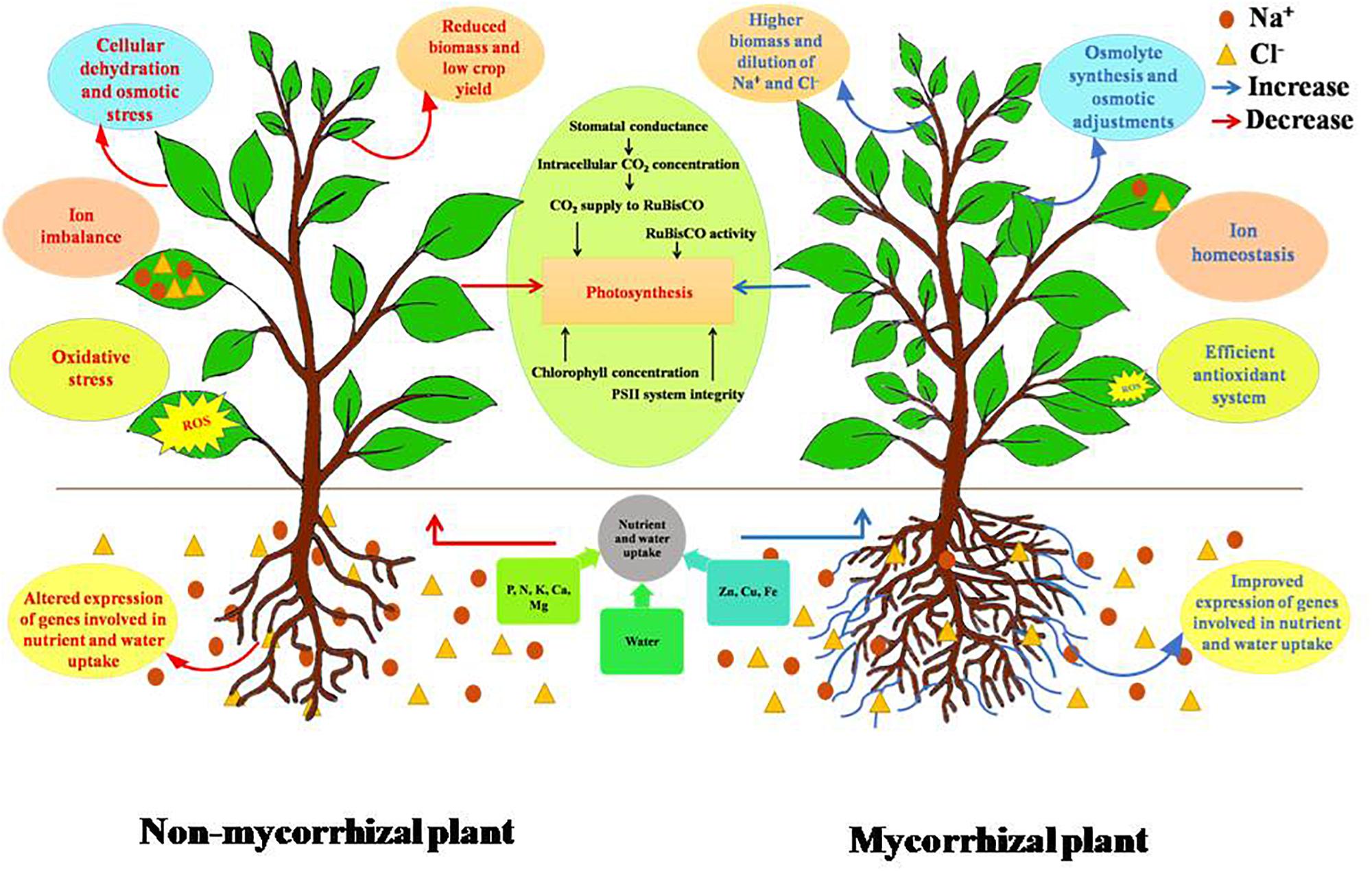
Figure 1. Differential response of non-mycorrhizal and mycorrhizal plants under salt stress. Accumulation of salt in soil creates competition for nutrient uptake and transport. This leads to imbalance of the ionic composition of plant, thereby affecting plant’s physiological traits. AMF increase the volume of soil explored by plant roots, upregulate several cation transporters, leading to improved nutrient uptake, and also maintains ionic homeostasis. Salinity lowers soil water potential causing cellular dehydration due to decrease in water uptake. AM negates this effect by mediating accumulation of osmolytes and also improves plant’s water status by improving root hydraulic conductivity. Salinity induces oxidative stress due to imbalance in ROS (reactive oxygen species) generation and the quenching activities of antioxidants. AMF are known to improve both enzymatic and non-enzymatic antioxidant systems of plants. Photosynthesis is also negatively affected by salinity. AM has a positive effect on photosynthesis under salt stress. Overall, AMF improve the performance of plant under salt stress.
Mechanisms of Salt Tolerance in M Plants
The ability of plants to tolerate salinity stress is usually evaluated in terms of biomass produced (da Silva et al., 2008; Ruiz-Lozano et al., 2012). Several studies have highlighted that AMF imparts salinity tolerance in host plants by virtue of higher biomass as compared to NM plants. AMF colonization enhanced biomass in Trigonella foenum-graecum (Evelin et al., 2012), Oryza sativa (Porcel et al., 2015), Medicago sativa (Campanelli et al., 2013), Gossypium hirsutum (Liu et al., 2016), Elaeagnus angustifolia (Chang et al., 2018), and Chrysanthemum morifolium (Wang et al., 2018). Higher biomass subsequently leads to dilution of Na+ and Cl-, and manifests as better crop yield (Talaat and Shawky, 2011).
Alteration in Root Architecture
Root system, being the plant structure responsible for uptake of water and nutrients, is crucial for enhancing plant resistance to salt stress. It is also the organ that regulates salt acquisition and translocation (Jung and McCouch, 2013). In the presence of salt in the rhizosphere, plants experience a decline in primary root growth due to salt-induced inhibition of cell division and elongation of root epidermal cells while lateral root development gets initiated (Rahnama et al., 2011; Jung and McCouch, 2013). In fact, it is well established that AMF colonization can improve plant’s adaptive ability by modifying the structure of root according to the requirements of time and space (Kapoor et al., 2008). Wu et al. (2010) observed that the length, surface area, and projected area of the root were more in M than the NM Citrus plants. Kumar et al. (2010) reported greater root length and biomass in M than NM Jatropha curcas plants. Similar observations have been reported in Trigonella foenum-graecum (Evelin et al., 2012), Medicago sativa (Campanelli et al., 2013), Ephedra aphylla (Alqarawi et al., 2014), and Cucurbita pepo var. pepo (Harris-Valle et al., 2018). Better root system enables the plant to track non-saline areas for water and minerals until exploitation of salt areas become indispensable (Wu et al., 2010; Campanelli et al., 2013; Alqarawi et al., 2014).
Nutrient Acquisition and Ionic Homeostasis
Excess salt (Na+ and Cl-) in the soil affects availability of nutrients by imposing competition during uptake, translocation or apportioning within the plant (Rabie, 2005). Therefore, high Na+ and Cl- concentrations in the soil solution may suppress nutrient associated activities and result in undesired ratios of Na+:Ca2+, Na+:K+, and Ca2+:Mg2+ (Abdel-Fattah and Asrar, 2012). Such a phenomenon can lead to imbalance in ionic composition of the plant, thereby affecting plant’s physiological traits (Hasegawa et al., 2000; Munns et al., 2006). However, AMF colonization has been shown to improve nutrient uptake and maintain ionic homeostasis in host plants grown in saline soils (Table 1). In fact, it has been assessed that the extramatrical hyphae of AMF can supply up to 80, 25, 10, 25, and 60% of plant’s P (phosphorus), N (nitrogen), K (potassium), Zn (zinc), and Cu (copper), respectively (Marschner and Dell, 1994). AMF colonization also influences the concentration and profile of organic acids and polyamines in plants (Sheng et al., 2011; Evelin et al., 2013; Talaat and Shawky, 2013). Organic acids play important role in lowering soil electrical conductivity and increasing the availability of N, P, and K in soil (Sheng et al., 2011). Polyamines help in retaining ion homeostasis in plant cells by enhancing the uptake of nutrients and water (Pang et al., 2007).
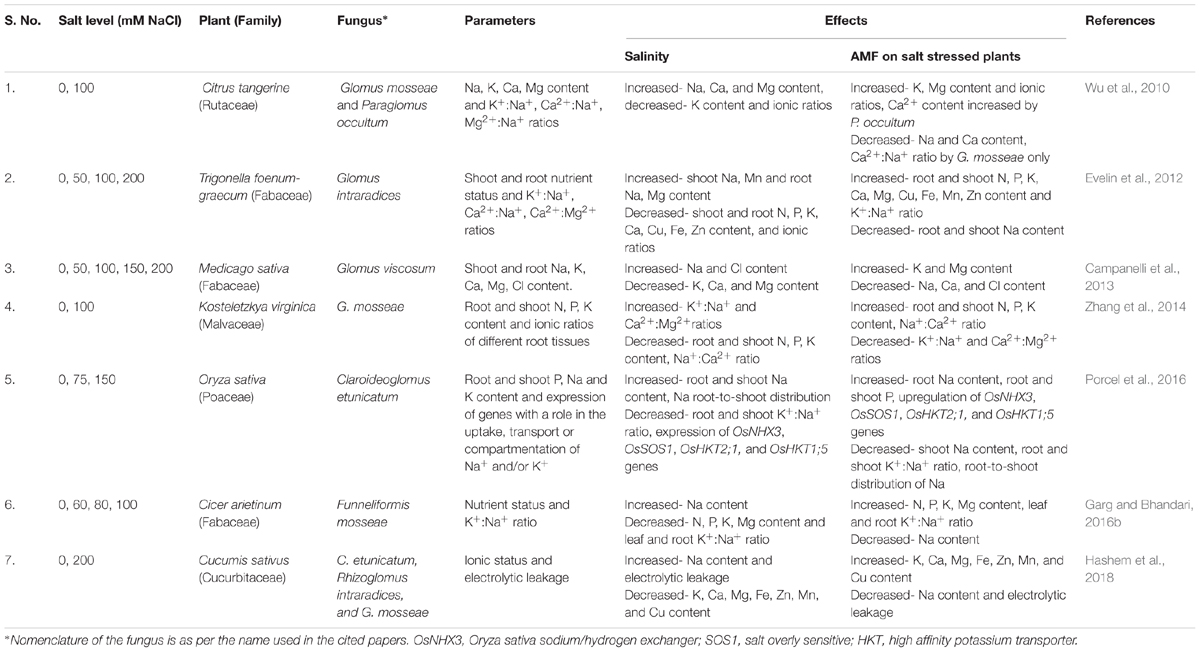
Table 1. Some of the studies on effect of salinity and AMF on nutrient concentration and ionic ratios in plants.
Phosphorus
Salinization renders P unavailable to plants due to its precipitation with other cations, such as Ca2+, Mg2+, and Zn2+ depending upon the pH of the soil environment (Azcon-G de Aguilar et al., 1979), thereby creating salt-induced P deficiency in plants. This results in stunted growth of the plant and the older leaves die prematurely (Taiz and Zeiger, 2006). However, AMF can significantly improve P acquisition for better growth and development of host plant (Table 1). Enhanced P acquisition in M plants is attributed to – (i) increased availability of P in the soil due to secretion of acid and alkaline phosphatases by hyphae that liberates P from its bound form; (ii) maintenance of intrinsic phosphate concentration (Pi) by forming polyphosphates inside the hyphae; (iii) ability of AMF to take up P at lower threshold owing to the expression of high affinity phosphate transporter genes (GvPT, GiPT, and GmosPT); and (iv) sustained movement of P into the roots as AMF are capable of accumulating vast amounts of absorbed P than roots (Bolan, 1991; Marschner and Dell, 1994; Selvaraj and Chellappan, 2006; Abdel-Fattah and Asrar, 2012). Thus in M plants, effective P uptake aids in – (i) preserving the integrity of cell membrane; (ii) reducing leakage of ions; (iii) compartmentalization of toxic ions in vacuoles and; (iv) selective uptake of ions (Rinaldelli and Mancuso, 1996; Evelin et al., 2012), consequently reducing the adverse effects of salinity.
K+:Na+ Ratio
Sodium and potassium ions, due to their similar physico-chemical nature, compete at the transport sites for entry into the symplast. Therefore, in saline soils where concentration of Na+ in rhizosphere is very high, K+ uptake faces a stiff competition from Na+, eventually decreasing K+:Na+ ratio in the cytosol. Low K+:Na+ ratio in the cell subsequently disrupts protein synthesis, enzyme activity, photosynthesis, turgor maintenance, and stomatal movement (Maathuis and Amtmann, 1999). The integrity and selectivity of root membrane are also altered by Na+ (Grattan and Grieve, 1999).
High Na+:K+ ratio in plants indicates a higher level of stress. Therefore, plants must consistently maintain low Na+:K+ to be able to resist the deleterious effects of salinity (Evelin et al., 2012). One of the significant advantages of favorable K+:Na+ ratio is the protection of photosynthetic tissues by inhibition of Na+ entry into them, a pivotal trait involved in overcoming salinity stress in glycophytes (Colmer et al., 2006; Munns and Tester, 2008; Cuin et al., 2011). In fact, photosynthetic organs constitute a major site for maintaining desirable K+:Na+ ratio. This, in turn, determines the photosynthetic ability, and hence development and productivity of the plant under saline conditions (Wu et al., 2013).
Mycorrhizal plants have unfailingly shown higher K+:Na+ ratio than their NM counterparts under salt stress conditions atleast in reported studies (Table 1). M plants can control Na+ translocation to aboveground parts as well as regulate internal concentrations of Na+. This is attributed to M plant’s ability to sequester Na+ into the vacuoles or exclude it from the cytosol. The toxic effects of Na+ in apoplast are lesser with respect to cytoplasm. Furthermore, AM facilitates host plant to retract Na+ from xylem, and divert it away from photosynthetic tissues to roots (Evelin et al., 2012; Maathuis, 2013). In this regard, it is interesting to note that, M plants have an added advantage over their NM counterparts as salinity induces accumulation of glomalin in participating AMF (Hammer and Rillig, 2011). Glomalin, a glycoprotein has been described as a heat shock protein 60 (HSP60) homolog (Gadkar and Rillig, 2006) and is hypothesized to participate in lowering cytosolic damages due to Na+-mediated protein misfolding (Maathuis and Amtmann, 1999). The strong correlation between salinity and production of glomalin only strengthens our understanding of AM protection of plants under salt stress (Hammer and Rillig, 2011).
Recent studies have explained the molecular bases of high K+:Na+ ratio in M plants (Asins et al., 2013; Porcel et al., 2016; Chen et al., 2017). Mycorrhizal Oryza sativa plants were able to compartmentalize Na+ into the vacuole via up regulation of OsNHX3 (sodium/hydrogen exchanger), and mediate efflux of Na+ from cytosol to apoplastic spaces via higher expression of OsSOS1 (salt overly sensitive) and OsHKT2;1 (high affinity potassium transporter) (Porcel et al., 2016). NHXs are vacuolar Na+/H+ antiporters present in roots and leaves that help in sequestering Na+ in the vacuole (Munns, 2005). SOS1 are plasma membrane Na+/H+ antiporters responsible for secretion of Na+ from the cytosol beyond plasma membrane, their reallocation in roots and shoots, and restraining them from getting into the photosynthetic organs (Munns, 2005; Olías et al., 2009). HKT are Na+/K+ transporters responsible for diverting Na+ from photosynthetic tissues to roots, and confiscating Na+ from the xylem (Figure 2) (Davenport et al., 2007). It is proposed that the functions of SOS1 and HKT are synchronized to bring Na+ homeostasis and apportioning among the plant organs (Pardo et al., 2006; Olías et al., 2009). Moreover, the level of stress is crucial in regulating the expression of a HKT gene (Porcel et al., 2016). For instance, OsHKT1;5 gene expression increased seven-fold in M rice plants at 75 mM NaCl; however, its expression remained more or less same in NM plants at higher salinity level (Porcel et al., 2016).
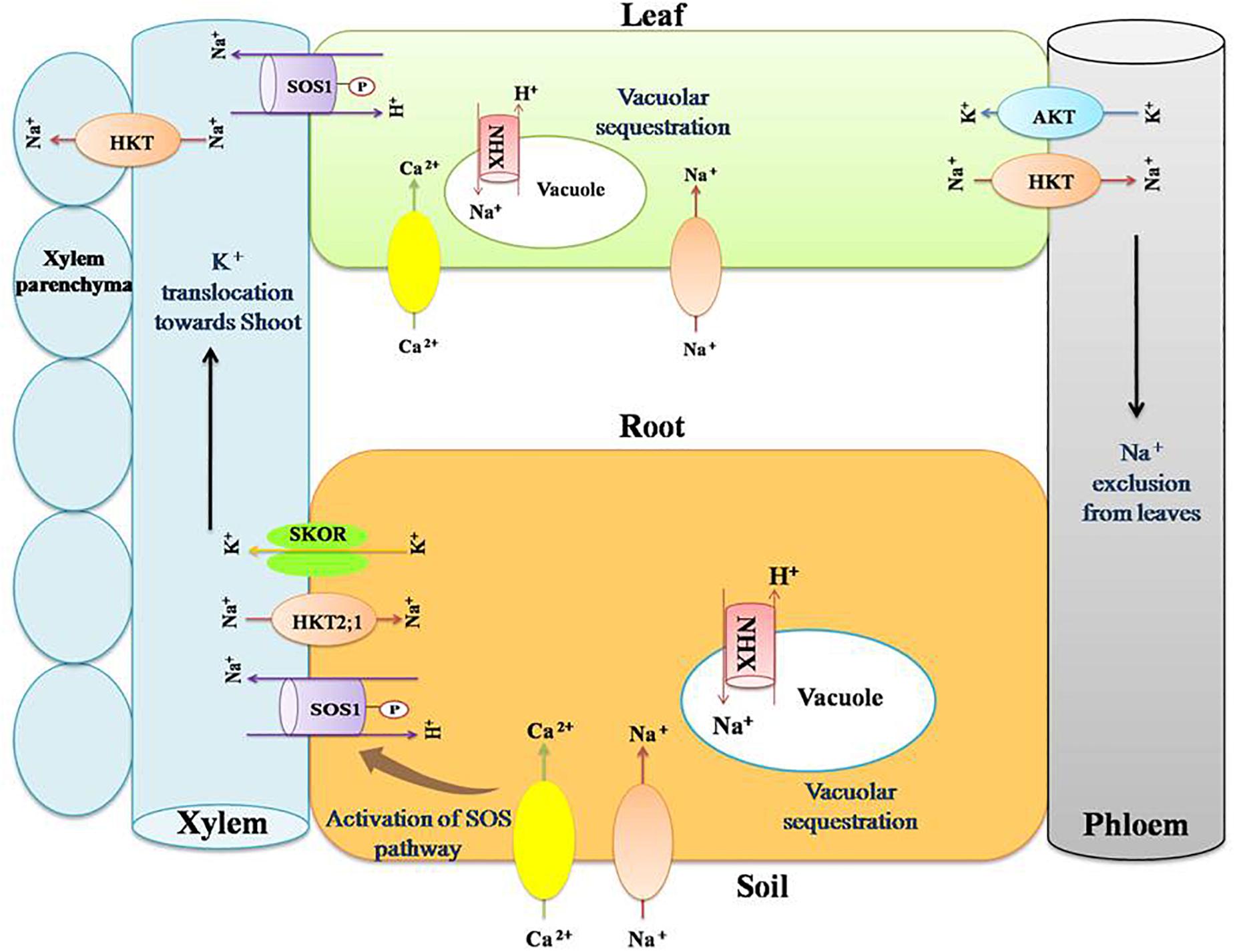
Figure 2. Role of transporter proteins in maintaining favorable K+:Na+ ratio in salt stressed plants. Salinity renders more concentration of Na+ and Cl- in the soil. This results in imbalance in the ion uptake by plants. Na+ and K+ have similar physico-chemical nature and hence compete at their transport sites for entry into root symplast. Salt stress results in higher Na+ uptake and thus its cellular composition increases, leading to disruption of enzyme activity, protein synthesis, turgor maintenance, and so on. Plants counteract such negative effects by maintaining lower cellular Na+ content by activating certain cation transporters such as NHXs (sodium/hydrogen exchanger), SOS (salt overly sensitive), SKOR (outward rectifying K+ channel), HKT (high affinity potassium transporters), and AKT (inward rectifying K+ channel). NHXs are vacuolar Na+/H+antiporters. They help in maintaining lower cellular Na+ content by sequestering Na+ inside the vacuole. SOS1 is a plasma membrane Na+/H+ antiporter that extrude Na+ from the cytosol. It helps in reallocation of Na+ in roots and shoots. HKTs are Na+/K+ transporters that act in removing Na+ from the xylem stream and translocating it into the xylem parenchyma. SKOR mediates translocation of K+ toward shoot through xylem. AKT is a K+ channel present in phloem and mediate influx of K+ to shoots.
In Robinia pseudoacacia roots, under salinity stress, AM symbiosis enabled exclusion of Na+ from root cells, unloading of Na+ from xylem, and translocation of K+ to the shoots. These effects were associated with the up regulation of root RpSOS1, RpSKOR, and RpHKT1 (Chen et al., 2017). SKOR (outwardly rectifying K+ channel) is involved in the translocation of K+ toward shoot through xylem (Figure 2) (Gaymard et al., 1998).
Nitrogen
Plants absorb N as nitrate (NO32-) and ammonium (NH4+) ions (Frechilla et al., 2001). However, salinity conditions interfere with their uptake by immobilizing them (Hodge and Fitter, 2010; Miransari, 2010). While NO32- uptake is challenged by Cl-, NH4+ absorption faces competition from Na+ at the membrane. Salt-induced disruption of membrane proteins that change plasma membrane integrity also affects the uptake of NO32- and NH4+ (Köhler and Raschke, 2000). This competition results in a low flux of NO32- from soil to roots leading to reduced activity of NR, as it is a substrate-inducible enzyme (Hoff et al., 1992). Several studies have reported that AMF colonization helps in increasing N uptake under stress conditions (Table 1). In fact, AMF hyphae have been reported to supply up to 25% of plant’s N (Marschner and Dell, 1994). Improved nitrate uptake in M plants is attributed to AMF-facilitated maintenance of membrane stability and increased NR activity (Talaat and Shawky, 2014). Higher NR activity in M plants can be explained by – (i) the sustained phosphate supply to the enzyme (Ruiz-Lozano and Azcón, 1996); (ii) a higher flux of nitrate (substrate), mediated by extramatrical mycelium of AMF; and (iii) regulation of NR activity (Kaldorf et al., 1998). Recently, Felicia et al. (2017) demonstrated that higher nitrogen uptake in M plants under salt stress is due to higher expression of nitrate (NRT1.1, NAR2.2) and ammonium transporters (AMT1.1 and AMT1.2) in M durum Triticum aestivum plants (colonized with a mixture of Rhizophagus irregularis and Funneliformis mosseae). NRT1.1 is a dual affinity transporter protein that is responsible for both low and high affinity nitrate uptake in roots (Crawford and Glass, 1998; Wang et al., 1998; Liu et al., 1999) while NAR2.2 is a protein that takes part in HATs (high affinity transporter systems) by actively interacting with the genes involved in effective NO32- transport (Filleur et al., 2001). However, in a prior study, it was observed that AMF symbiosis significantly up regulated the expression of NRT1.1, NRT2, NAR2.2, AMT1.2, and AMT2.1 in durum Triticum aestivum only when grown under N-limiting conditions (Saia et al., 2015).
Ca2+:Na+ and Ca2+:Mg2+ Ratio
One of the parameters to measure salt stress in plants is to determine Ca2+:Na+ ratio. Under salt stress, an elevated Na+ concentration in the rhizosphere hampers the absorption of Ca2+ by replacing them in cell wall and plasma membrane. This results in translocation of less Ca2+, thereby reducing Ca2+:Na+ ratio in salt stressed plants (Grattan and Grieve, 1999). Low Ca2+:Na+ ratio decreases hydraulic conductivity and cell turgor, and disturbs Ca2+ signaling (Läuchli and Lüttge, 2002). Ca2+ uptake is also challenged by the presence of Mg2+. Magnesium is a macronutrient and is the central ion of the chlorophyll molecule. It is responsible for harvesting light for photosynthesis. It is also required for proper functioning of many enzymes, such as RNA polymerases, phosphatases, carboxylases, ATPases, protein kinases, and glutathione synthase (Shaul, 2002). Several studies have reported that Mg2+ concentration in plants decreases under salt stress conditions (Table 1). However, M plants possess higher concentration of Mg2+ than NM plants. It is interesting to note that M plants, despite accumulating more Mg2+ than NM plants, are also shown to have desirable Ca2+:Na+ ratio by improving Ca2+ uptake under salt stress conditions (Evelin et al., 2012). This AM effect is yet to be understood and requires further studies to unravel the mechanisms involved.
Micronutrients
The acquisition of micronutrients (Zn, Cu, Fe) by plants is exceedingly affected by salinity (Grattan and Grieve, 1992). Salinity decreases the solubility and mobility of micronutrients, such as Cu and Fe (Grattan and Grieve, 1999), thereby creating a depletion zone around the root. The depletion zone around the root results in decrease in uptake of the micronutrients by plants. However, M plants showed higher concentration of these micronutrients than NM plants (Table 1). This may be credited to – (i) widespread root-hyphal system that shortens the path of nutrients’ entry into plant (Subramanian et al., 2009); (ii) fungal mycelium serving as a substrate for nutrients to bind; (iii) AMF-induced changes in the pH of the rhizosphere, which modulates nutrient solubility and thus their availability (Li and Christie, 2001); (iv) increase in sink size of Cu and Zn due to higher shoot P, which subsequently instigate nutrient uptake and translocation to shoots (Liu et al., 2000); (v) up regulation of the expression of transporter gene of these nutrients, for example, a plasma membrane Zn transporter gene, MtZIP2 is up regulated upon colonization by AMF (Burleigh et al., 2003).
The role of AMF in maintaining ionic balance is being elucidated at molecular level. However, much remains to be investigated on AMF-influenced higher Ca2+:Na+, Ca2+:Mg2+ ratios as well as uptake of micronutrients.
Osmoregulation
Salt stress causes cellular dehydration by lowering turgor pressure in plant cells. In order to negate this effect, plants employ osmoregulation as a mechanism to tolerate salt stress (Munns, 1993; Zou et al., 2013). The buildup of Na+ and Cl- in soil decreases the water potential of the soil. In such a condition, plants must retort to lower water potential in order to maintain a favorable gradient for water flow from soil into roots, and prevent dehydration of cells. To accomplish this, plants start to accumulate osmolytes, such as proline, betaine, polyamines, sugars, organic acids, amino acids, and trehalose. Osmolytes are small organic solutes that are water soluble and non-toxic at high concentrations and are also known as compatible solutes (Chen and Murata, 2011). Under salt stress, M plants have been shown to possess higher osmotic potential than their NM counterparts (Navarro et al., 2014) due to accumulation of more osmolytes (Figure 3) (Evelin et al., 2013; Garg and Baher, 2013; Alqarawi et al., 2014). The fundamental role of these osmolytes is osmotic adjustment (Hasegawa et al., 2000) In addition, they are also involved in ROS quenching, maintenance of membrane integrity, and enzyme as well as protein stabilization, hence are also known as osmoprotectants (Ashraf and Foolad, 2007). The osmolytes and their role in improving salt tolerance in M plants are discussed subsequently.
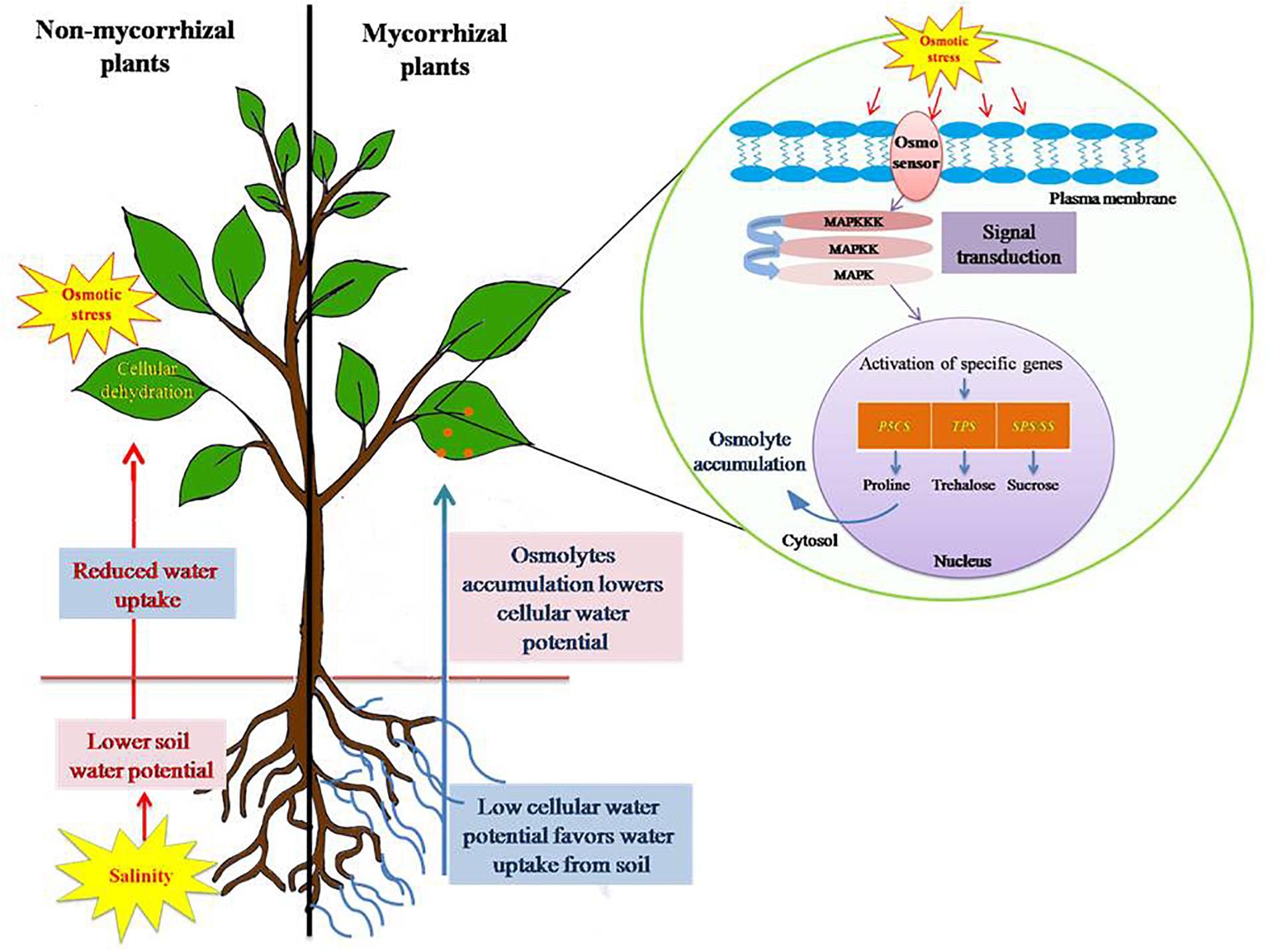
Figure 3. Salinity stress induced osmotic stress tolerance mechanism in plants. Salinity leads to build up of Na+ and Cl- in soil, consequently lowering the soil water potential as compared to water potential of plant cells. This leads to reduced water uptake by plants and eventually causes cellular dehydration. Plants, in order to avoid such consequences, accumulate osmolytes, such as proline, trehalose, polyamines, and sucrose in higher concentration. Osmolytes accumulation results in lowering of cellular water potential and thereby maintains a favorable gradient for water uptake from soil to root. Thus, it prevents cellular dehydration and subside osmotic stress caused by salinity. AM symbiosis alleviates osmotic stress by influencing the expression of specific genes (P5CS, pyrroline-5-carboxylate synthase; TPS, trehalose-6-phosphate synthase; SPS, sucrose phosphate synthase; SS, sucrose synthase) involved in the biosynthesis of osmolytes.
Proline
Salt stress tolerance mechanisms often include accumulation of proline. Proline can scavenge ROS and stabilize DNA, proteins and membranes, and reduce NaCl-induced enzyme denaturation (Kaur and Asthir, 2015). In addition, it also delivers carbon, nitrogen, and energy. This, in turn, supports plant during stress and aids in its recovery from stress. The reports on impact of AMF on proline concentration in salt stressed plants have been inconsistent. Many studies reported higher proline content in M plants compared to NM plants, while others have reported lower proline content in M plants (Table 2). Higher proline content in M plants has been attributed to – (i) increase in the expression of gene encoding P5CS involved in proline biosynthesis; (ii) higher activity of the enzyme P5CS; (iii) higher activity of the enzyme, glutamate dehydrogenase, that is responsible for synthesizing glutamate, the precursor of proline; and (iv) inactivation of proline dehydrogenase, an enzyme that catalyze the degradation of proline (Abo-Doma et al., 2016). Besides its role as an osmoprotectant, proline is also considered as a stress marker. Therefore, M plants may accumulate less proline as they experience reduced stress (Rabie and Almadini, 2005; Sannazzaro et al., 2007).
Trehalose (Tre)
Trehalose (α-D-glucopyranosyl-1,1-α-D-glucopyranoside) is a non-reducing storage disaccharide that regulates carbohydrate metabolism (Lunn et al., 2014). It acts as a stress protection metabolite by maintaining K+:Na+ ratio, scavenging ROS, and increasing the concentration of soluble sugars in plants (Garg et al., 2002; Redillas et al., 2012; Chang et al., 2014). Salinity enhances the buildup of trehalose and AM symbiosis can further boost the accumulation of this osmolyte. Garg and Pandey (2016) reported that M plants under salt stress accumulate more trehalose than their NM counterparts. Higher trehalose concentration in M plants can be attributed to AMF-facilitated increased activities of TPS and TPP and lower activity of TRE (Garg and Pandey, 2016). TPS and TPP are enzymes responsible for the biosynthesis of trehalose while TRE is a trehalose degrading enzyme.
Organic Acids
Organic acids are important osmolytes in plant vacuoles, and the regulation of their metabolism plays an important role in providing tolerance to salt stress (Guo et al., 2010). AMF colonization can also influence the concentration and profile of organic acids in plants. Under salt stress, M Zea mays plants accumulated more of acetic, citric, fumaric, malic, and oxalic acids, whereas concentration of formic acid and succinic acid decreased, while there was no difference in lactic acid concentration as compared to NM plants (Sheng et al., 2011). However, the mechanisms underlying changes in organic acids in M plants are not known and calls for investigation. It is speculated that AMF impart protection to the enzymes involved in organic acid biosynthesis (Sheng et al., 2011).
Polyamines
Polyamines are aliphatic, low molecular weight polycations that have been indicated to participate in cellular osmoregulation in plants under salt stress (Duan et al., 2008; Kuznetsov and Shevyakova, 2010). Under salt stress, polyamines accumulate in plants as compatible solutes (Minocha et al., 2014; Singh et al., 2015; Yang and Guo, 2017; Zapata et al., 2017). Major polyamines that accumulate under salt stress are putrescine (Put, diamine), spermidine (Spd, triamine), and spermine (Spm, tetraamine) (Pang et al., 2007; Kusano et al., 2008; Minocha et al., 2014). However, it remains unclear as to which polyamine is responsible more in imparting salt tolerance. AMF has also been found to modulate polyamine pool to help plants tolerate salt stress (Sannazzaro et al., 2007; Evelin et al., 2013; Talaat and Shawky, 2013). Mycorrhizal plants had higher Spd+Spm:Put ratio than NM Trigonella foenum-graecum plants (Evelin et al., 2013). The effect of AM on individual polyamine varies with genotype and intensity of stress (Sannazzaro et al., 2007). However, the mechanism underlying AMF-facilitated modulation of polyamines for salt tolerance in plants is yet to be deciphered, and further investigations should focus polyamines metabolism.
Sugars
Accumulation of TSSs such as, glucose, sucrose, dextrins, and maltose in salt stressed plants is another way of osmotic adjustment. They play a vital role in osmoprotection and as carbon storage (Parvaiz and Satyawati, 2008). Plants direct the synthesis of TSS from starch and sucrose by upregulating the activities of sucrose anabolizing enzymes. Starch is converted into dextrins and maltose by α- and β-amylases, respectively (Schrader and Sauter, 2002; Ballicora et al., 2004). SPS and SS catalyze the synthesis of sucrose while AI catalyzes the breakdown of sucrose to glucose (Van den Ende et al., 2002; Peng et al., 2016). During salt stress, sucrose undergoes decomposition in order to meet the requirement of glucose (Geigenberger and Stitt, 1993). Salt stress induced TSS accumulation is further enhanced by AM symbiosis (Talaat and Shawky, 2011; Liu et al., 2016; Garg and Bharti, 2018; Zhu et al., 2018). The higher accumulation of TSS in M plants has been credited to – (i) higher photosynthetic efficiency; (ii) higher activities of α- and β-amylases, SS, and AI; (iii) higher organic acid content; and (iv) carbon requirement of AMF (Garg and Baher, 2013; Yu et al., 2015; Zhu et al., 2016, 2018). Garg and Bharti (2018) reported that salt tolerant M Cicer arietinum cultivar (PBG 5) accumulated more sugars than its salt sensitive M counterparts (BG256) indicating that accumulation of TSS can enhance tolerance to salt stress. This is accompanied by higher activities of α- and β-amylases in M plants, indicating rapid hydrolysis of starch to glucose in salt tolerant M Cicer arietinum (Garg and Bharti, 2018). The activity of SPS as well as SS and AI increased simultaneously indicating that sucrose is synthesized upon salt stress, and concomitantly converted to glucose leading to increase in TSS concentration (Garg and Bharti, 2018).
The role of AM symbiosis in reinforcing osmotic adjustment in plants under salt stress via enhanced accumulation of osmolyte is now well-understood. However, these mechanisms are yet to be explicated at the molecular level. Therefore, directing future research in unraveling the molecular bases of osmolyte accumulation in M plants will help in all-inclusive understanding of the mechanisms.
Oxidative Stress
Salinity mediated hyperosmotic and hyperionic stress induce another secondary stress in plants, called oxidative stress. It results due to the disturbance of equilibrium between ROS generation and its diminution by several antioxidants (Gill and Tuteja, 2010). ROS consists of a group of chemically reactive oxygen molecules such as hydroxyl radical (OH-), H2O2, .O2-, and O2-. ROS are generated as an after-effect of disengaged pathways in plant metabolism that causes the transfer of high energy electrons to molecular oxygen (Gill and Tuteja, 2010). Excessive generation of ROS disturbs various cell functions by attacking several biomolecules such as nucleic acid, protein, and membrane lipid (Foyer and Noctor, 2005). Salinity increases the level of lipid peroxidation (Evelin et al., 2012; Pedranzani et al., 2016; Zhang et al., 2018) resulting in higher membrane permeability and loss of ions from the cells (Estrada et al., 2013; Felicia et al., 2017).
Plants employ a two-pronged system to counteract the adverse consequences of ROS; enzymatic and non-enzymatic antioxidative systems. The enzymatic system consists of SOD, POX, CAT, APX, and GR. Non-enzymatic antioxidant molecules such as AsA, glutathione (GSH), carotenoids, and α-tocopherol also take part in quenching of toxic by-products of ROS (Gill and Tuteja, 2010). In fact, salinity tolerance in plants has been associated with the induction of antioxidative pathways and diminution of oxidative damage (Figure 4) (Porcel et al., 2012).
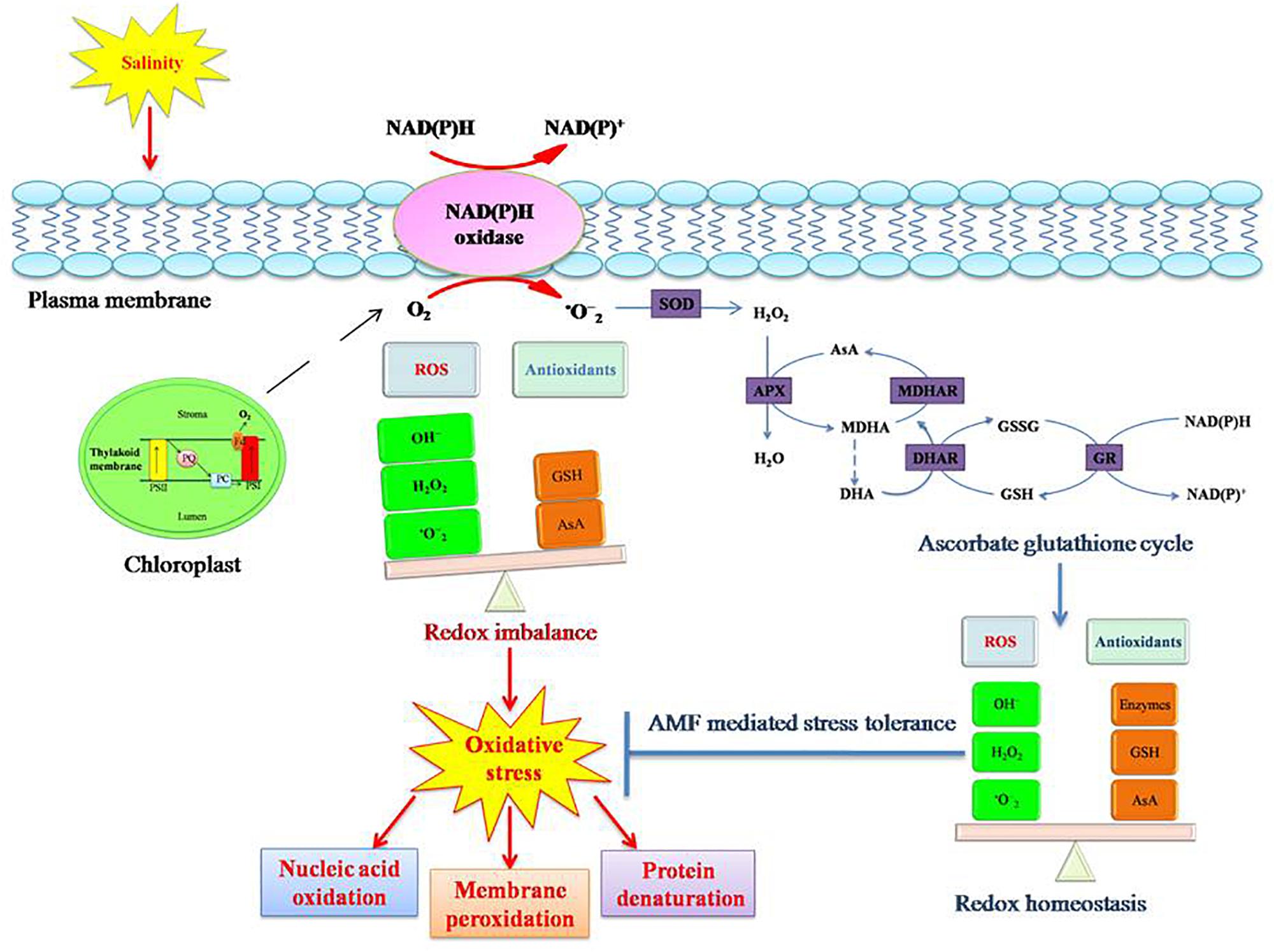
Figure 4. Salinity stress induced oxidative stress tolerance mechanism in plants. Salinity causes oxidative stress in plants due to redox imbalance resulting from disturbance in equilibrium between ROS (reactive oxygen species) and antioxidants. Increased ROS concentration in the cell results in protein denaturation, membrane peroxidation, and nucleic acid denaturation. This consequently disturbs the normal functioning of the cell. To counteract the adverse consequences, plants induce antioxidative pathways (enzymatic and non-enzymatic). Enzymatic antioxidants include superoxide dismutase (SOD), peroxidase (POX), catalase (CAT), ascorbate peroxidase (APX), monodehydroascorbate reductase (MDHAR), dehydroascorbate reductase (DHAR), and glutathione reductase (GR). Non-enzymatic antioxidants include ascorbate (AsA), glutathione (GSH), carotenoids, and α-tocopherol. An efficient antioxidative system abates the oxidative damage in plants. AM symbiosis reinforces the tolerance mechanism of plants to salinity induced oxidative stress.
In the last few years, several studies have shown that one of the mechanisms AMF employ to alleviate salinity stress is an efficient ROS scavenging system. AMF augment the activities of antioxidant enzymes as well as increase the production of antioxidant molecules, ensuring a better ROS scavenging system. AMF colonization increases the concentrations of antioxidant molecules such as, α-tocopherols, AsA, GSH, and carotenoids in plants (Evelin and Kapoor, 2014). High α-tocopherol content limits auto-oxidation of lipids, thus preventing M plants from lipid peroxidation (Serbinova and Packer, 1994). High α-tocopherol content in M plants can be attributed to improved production of tocopheroxyl radicals, facilitated by the high AsA content (Thomas et al., 1992; Noctor and Foyer, 1998). Higher GSH levels and GR activity result in higher AsA levels in plants. GSH enables plants to directly scavenge more .O2- and H2O2 as well as other ROS (Smirnoff, 1993; Briviba et al., 1998; Noctor and Foyer, 1998), and recycle more AsA via the AsA-glutathione cycle (Foyer and Halliwell, 1976). Carotenoids also prevent .O2-production (Viljanen et al., 2002; Ramel et al., 2012).
Mycorrhizal plants are reported to possess higher activities of enzymatic antioxidants under both saline and non-saline conditions. Elevated SOD activity was reported in M plants in comparison to their NM counterparts (Table 3). Higher SOD activity is associated with higher tolerance to salinity (Benavídes et al., 2000). Higher SOD enables detoxification of more O2- to H2O2, that is further detoxified to H2O. The conversion of H2O2 to H2O can be catalyzed by enzymes, such as CAT, APX, and POX. Mycorrhizal plants are reported to have higher activities of H2O2 scavenging enzymes. Higher AsA concentration in M plants induces higher activity of APX, that is consecutively reliant on enhanced GR activity in M plants. This results in higher GSH pool, which ultimately depletes DHA concentration and recycles AsA (Foyer and Halliwell, 1976). Improved efficiency of GR maintains higher GSH:GSSG and NADP+:NADPH ratios and lowers superoxide radical production by sustaining the photosynthetic electron transport (Noctor and Foyer, 1998; Mittler, 2002). Higher activities of these enzymes in M plants are partially explained by better nutritional status. Mycorrhizal plants have been shown to have higher concentrations of micronutrients, such as Cu, Zn, Mn, and Fe. On the other hand, these enzymes are metalloenzymes whose activities are also governed by the availability of these micronutrients. However, the activities of these enzymes are dependent on various factors such as plant species, plant tissues, AMF species, level of salinity, and duration of stress.
Additionally, lower oxidative stress in M plants is also due to improved concentrations of osmolytes, such as proline, polyamine, and glycinebetaine (Pang et al., 2007; Evelin et al., 2012; Frosi et al., 2017). They bring about stabilization of sub-cellular components of cell membranes such as lipids and proteins, quenching of free radicals, and buffer cellular redox potential under salinity stress (Kishor et al., 1995; Yang et al., 2008).
Water Status
High salt concentration in the rhizosphere also imposes physiological drought in plants. Salt immobilizes water and renders it unavailable for the plants (Füzy et al., 2008). Yang et al. (2014) observed that M Malus hupehensis seedlings maintained relatively higher leaf turgidity and lower leaf osmotic potential compared to NM plants, when subjected to salt stress. Chen et al. (2017) reported higher relative water content in M as compared to NM Robinia pseudoacacia plants under salt stress. This observation is explained by improved hydraulic conductivity of M plants attributed to AMF-induced altered root morphology, and the ability of M plants to explore macroelements well beyond the depletion zone facilitated by the extensive extramatrical mycelium of AMF (Schnepf et al., 2011). In addition, M plants also accumulate more compatible solutes to adjust the osmotic potential and enable efficient water usage by host plants (Graham and Syvertsen, 1984). Better water status in M plants may be explicated by the AMF regulated expression of aquaporin genes present in leaves and roots of salt stressed plants. However, each aquaporin gene in roots of M plants may respond differently to salt stress. For instance, in Lycopersicon esculentum, AMF colonization downregulated LePIP1 gene (Ouziad et al., 2006) while the same gene was upregulated in Lactuca sativa (Jahromi et al., 2008). Recently, Chen et al. (2017) described the expression profile of aquaporin genes (RpPIP1;1, RpPIP1;3, RpPIP2;1, RpTIP1;1, RpTIP1;3, RpTIP2;1) in leaves and roots of M and NM Robinia pseudoacacia subjected to saline stress. Hence, the effect of AM symbiosis on transcription of aquaporin genes varies with the plant species, type of plant tissue they are expressed, and level of salinity. Furthermore, potential of different aquaporin genes to transport water and other solutes may differ and depend on their location in the cell (Ruiz-Lozano et al., 2012).
Photosynthesis
Salt stress impedes photosynthesis and brings an enormous decline in crop productivity (Pitman and Läuchli, 2002; Sheng et al., 2008). Salt stress affects photosynthesis in several ways (Table 4). Salt-induced osmotic stress leads to lowering of leaf area, coupled with a decrease in stomatal and mesophyll conductance, which limits CO2 availability and assimilation (Chaves et al., 2009). This consequently decreases the supply of CO2 to RuBisCO. In addition to this, RuBisCO being sensitive to Cl-, loses its activity under salt stress (Seemann and Critchley, 1985). The decline in CO2 assimilation also leads to accumulation of excess energy that leads to increased accumulation of electrons in the thylakoid membranes. To dissipate this energy, PSII loses massive amount of electrons and causes injury of the photosynthetic tissues, which subsequently affects the net photosynthetic rate (Redondo-Gómez et al., 2010). Furthermore, salt stress has also been shown to degrade D1 and D2 proteins of PSII reaction center. These proteins are structural components of the PSII reaction center and play fundamental roles in phosphorylation of proteins coupled with flow of electrons (Jansen et al., 1996). A decline in the activity of photosynthetic pigment synthesizing enzymes is another way by which salt stress affects photosynthesis resulting in decreased concentration of photosynthetic pigments (Giri and Mukerji, 2004; Murkute et al., 2006; Sheng et al., 2008). The low concentration of chlorophyll may also be attributed to salt-induced low uptake of Mg2+ (Giri and Mukerji, 2004; Ibrahim et al., 2011), destruction of pigment-protein complexes due to salt-induced augmentation of chlorophyllase enzyme activity, and reduction of de novo protein synthesis (Levitt, 1980; Sultana et al., 1999; El-Tayeb, 2005).
Plants can safeguard the photosystems from light induced inhibition and damage in many ways. These include minimizing harvesting of light and dispersion of excess energy by non-photochemical quenching (NPQ) or cyclic electron flow (Lima Neto et al., 2015). AM symbiosis is known to bolster up these mechanisms and alleviate the negative effects of salinity on plant photosynthetic capacity (Talaat and Shawky, 2014; Porcel et al., 2015; Wu et al., 2015; Hidri et al., 2016; Chen et al., 2017). As compared to NM plants, M plants have higher net photosynthetic rate, more quantum yield (Fv/Fm; Fv = Fm − F0; Fm = maximal fluorescence; F0 = minimal fluorescence), and actual quantum yield of PSII (ΦPSII) photochemistry under salt stress (Table 4) (Talaat and Shawky, 2014; Porcel et al., 2015; Wu et al., 2015; Hidri et al., 2016; Chen et al., 2017). AM symbiosis combats the negative effects of salt stress on photosynthesis in following ways – (i) improved water status in M plants results in maintaining larger leaf area and higher stomatal conductance, and consequently better assimilation of CO2 (Wu et al., 2015; Chen et al., 2017). Furthermore, aquaporins besides transporting water, can also promote diffusion of CO2 across plasma membrane of the mesophyll cells (Yang and Cui, 2009); (ii) ability of M plants to abate intercellular CO2 concentration (Ci) ensures protection of the photosynthetic apparatus (Sheng et al., 2008; Chen et al., 2017). This is possible due to enhanced RuBisCO activity in M plants (Talaat and Shawky, 2014; Porcel et al., 2015; Garg and Bhandari, 2016b; Chen et al., 2017) owing to higher expression of the large subunit of RuBisCO, RprbcL gene (Chen et al., 2017). However, up regulation of gene expression may not always translate to increased RuBisCO activity due to delay in protein translation after gene transcription (Porcel et al., 2015); (iii) AM symbiosis empowers host plants to maintain the integrity of PSII by its prompt action to repair salt-induced degradation of D1 and D2 proteins. The genes encoding D1 (RppsbA) and D2 (RppsbD) were found to be up regulated by AM symbiosis under salinity conditions (Chen et al., 2017). This may also be due to higher concentration of polyamines and glycinebetaine in M plants (Pang et al., 2007; Talaat and Shawky, 2014). Glycinebetaine stabilizes PSII pigment-protein complexes as well as protect the activities of enzymes such as, RuBisCO and rubisco activase that are responsible for fixing CO2 (Talaat and Shawky, 2014). In fact, previous studies have reported that maintenance of PSII activity can help plants to adapt to abiotic stresses (Sheng et al., 2008; Hajiboland et al., 2010; Porcel et al., 2016; Hu et al., 2017); (iv) Mycorrhizal plants maintain higher chlorophyll and carotenoid concentration by improving the uptake of Mg2+ (Evelin et al., 2012; Hashem et al., 2015). Moreover, an increase in NPQ can limit Fv/Fm (Baker, 2008). However, M plants are reported to have lower NPQ (Baker, 2008). Thus, AM symbiosis enhances photosynthetic efficiency by proficient conversion of harvested light into chemical energy, and minimizing NPQ as compared to NM plants (Hu et al., 2017).
Furthermore, salinity also increases the activities of carbon metabolizing enzymes such as, pyruvate orthophosphate dikinase (PPDK), phospho-enol-pyruvate carboxylase (PEPC), NADP-MDH, and NAD-MDH (Hashem et al., 2015). Higher activities of these enzymes are required for better plant growth to overcome stress conditions (Doubnerová and Ryšlavá, 2011). Mycorrhizal plants were found to possess lower activities of these enzymes suggesting lower level of stress. On the other hand, salinity decreased the activity of NADP-ME and AMF colonization induced the activity of this enzyme (Hashem et al., 2015). Higher NADP-ME in M plants can increase carbon metabolism and contribute to stress tolerance (Hashem et al., 2015).
Hormonal Regulation
Phytohormones regulate growth and development of plants under ambient as well as stressed conditions, and hence are also known as growth regulators (Fahad et al., 2015). They are derived from plant biosynthetic pathways and can act at the site of production or away from it. Phytohormones such as, ABA, auxin, BR, CK, GA, JA, SA, SLs, NO, and triazoles have been implicated to play significant roles in imparting salt-stress tolerance in plants (Fahad et al., 2015). In order to initiate suitable plant response to environmental stimulus, these hormones interplay amongst themselves to modulate biochemical and physiological processes that translate into mediation of growth, development, nutrient allocation, and source/sink transitions (Saeed et al., 2017). However, despite the large amount of literature available on phytohormones’ role in salinity tolerance, this aspect has found little interest in studies pertaining to AMF-mediated salinity tolerance in plants. Moreover, during AM symbiosis, ABA, auxin, JA, and SA are known to act as signaling molecules (Gutjahr and Paszkowski, 2009; Miransari, 2012). Therefore, it is postulated that these hormones play significant roles in improving plant tolerance to salinity stress.
Strigolactones consist of a new class of phytohormones that are involved in many aspects of plant development such as coordination of root growth and architecture according to the nutrient availability in soil, suppression of secondary branches in shoot, stimulation of internode length in a cross talk with auxin, regulation of leaf senescence, and induction of AM symbiosis (Agusti et al., 2011; Koltai, 2011; de Saint Germain et al., 2013; Yamada et al., 2014). They also play regulatory roles against abiotic stress. In order to exert its full effect, SLs need to modulate and interact with other phytohormones, especially auxin and ABA. In Sesbania cannabina seedlings, Kong et al. (2017) reported that H2O2 and SLs signaling are involved in AM mediated salt stress alleviation. Ren et al. (2018) observed that that in contrast to NM plants, AM colonization in S. cannabina maintained positive correlation between ABA and SLs. They proposed that AM colonization intensively altered ABA catabolism. This high ABA in M plants, protects it from salt stress by inducing the production of SLs via H2O2 signaling (Ren et al., 2018). On the perception of ABA signal, there is rapid production of H2O2 in the apoplast. The accumulation of H2O2 depends on NADPH oxidase activity, which also plays a significant role in ABA signaling (Kwak et al., 2003). This subsequently leads to increase in accumulation of SLs, and ultimately enhanced salt tolerance (Ren et al., 2018).
Exogenous applications of GA and SA have displayed boost in salinity tolerance in Solanum lycopersicum and Cicer arietinum, respectively (Khalloufi et al., 2017; Garg and Bharti, 2018). Foliar spray of GA3 resulted in better nutrient acquisition and manifold increase in GA concentration in M Solanum lycopersicum indicating that GA can promote salinity tolerance (Khalloufi et al., 2017). AMF colonization has also been shown to impart positive influence on the endogenous concentrations of GA (Shaul-Keinan et al., 2002). Seed priming with SA helped M Cicer arietinum to tolerate salinity stress by improving ionic homeostasis, modulating carbohydrate metabolism, and improving growth and yield. In fact, SA application also promoted AMF colonization (Garg and Bharti, 2018) and vice versa (Hashem et al., 2018). Salicylic acid, in conjunction with AMF have also been shown to improve salinity tolerance by reducing lipid peroxidation while increasing concentration of proline, proteins, reduced sugars, and K+ ion contents in shoots of Ocimum basilicum (Shekoofeh et al., 2012). In a recent study, Hashem et al. (2018) have reported an increase in concentration of JA in Cucumis sativus under salt stress. JA is a phytohormone belonging to octadecanoid family and is involved in plant’s response to biotic as well as abiotic stress. Pedranzani et al. (2016) also reported increased concentrations of JA, 12-OH-JA, and OPDA (precursor of JA) in Digitaria eriantha under salt stress. Their concentrations were further boosted in the presence of Rhizophagus irregularis. Thus, JA has also been implicated to play a key role in imparting salinity tolerance to plants. The underlying mechanisms of GA, SA, and JA in improving salinity tolerance in M plants are yet to be fully understood. Therefore, more studies are required to be directed toward AM-salinity experiments involving phytohormones.
Challenges
This review highlights the mechanisms that AM symbiosis facilitates to impart salt stress tolerance in plants. However, there are several challenges that future research should address for comprehensive understanding of these mechanisms. The challenges are briefly discussed below:
(1) There is no debate on the role of AMF facilitating osmoregulation. However, studies pertaining to osmoregulation are mainly limited to the evaluation of concentrations of osmolytes. There is a need to elucidate these phenomena at the molecular level by investigating the genes that code for these molecules and their biosynthetic enzymes.
(2) AMF have been consistently shown to improve nutrient uptake and maintain ionic homeostasis. However, these reports are focused on determining the concentrations of nutrients in tissues and evaluating ratios, such as K+:Na+, Ca2+:Na+, and Ca2+:Mg2+. Significant progress has been made to understand the mechanisms involved in maintenance of K+:Na+ ratio by studying uptake and translocation of K+ and Na+ ions via transporters. However, there are very few reports that elucidate the uptake of nutrients at the molecular level. CNGCs are non-selective cation channels that take up K+, Na+, and Ca2+ (Kaplan et al., 2007). Till now, there are no investigations on CNGCs in M plants. Therefore, identification of ion transporters and expression studies can provide a better understanding of ion homeostasis in M plants.
(3) Sulfur is a major component of various molecules, such as cysteine, a sulfur donor in ABA synthesis; and glutathione, an antioxidant molecule that helps in detoxification of ROS, thereby helping plants cope with salinity stress. Plants absorb sulfur as sulfate via SULTR. It is also known that AMF can improve sulfur nutrition by enhanced uptake and its translocation to the root in low-sulfate environments (Allen and Shachar-Hill, 2009). However, no studies are available on the influence of AMF on plant sulfate uptake regulation or SULTR genes under saline conditions.
(4) Studies regarding the role of phytohormones are limited and inconclusive. For instance, far and few studies with respect to AMF-salinity have focused on ABA and SLs. Phytohormones, such as BR, JA, NO, and SA have been indicated to improve plant’s tolerance to salinity; however, they are yet to be explored as potential candidates that impart salinity tolerance to M plants. Moreover, SA and JA have been well documented as signals to alert neighboring plants upon biotic stress. On the other hand, M plants are well connected to each other owing to the existence of common mycorrhizal network. Therefore, future research should investigate if SA and JA play any role in eliciting stress signals to its neighbors.
(5) Under salt stress, lipid metabolism undergoes changes that can be associated with profound alterations in cell membrane integrity, composition, and function (Parihar et al., 2015). Though lipid peroxidation has been elucidated in AM-salinity studies, lipid metabolism in salt stressed M plants is yet to be investigated.
(6) Salt stress causes endocytosis (Hamaji et al., 2009). Earlier, Evelin et al. (2013) reported that NaCl treatment increased the incidence of endocytosis significantly in Trigonella. The vesicular surface increases the surface area for higher exchange capacity (K+ versus Na+), protecting plants from ionic toxicities, imbalances, or interactions between substances in the cytoplasm.
(7) Cell wall of a plant is the interface between plant and environment. Therefore, it is the first organelle that, senses, perceives, and responds to salt stress (Li et al., 2012; Van der Does et al., 2017). Hence, it is crucial that active cell wall integrity is preserved so as to allow plants to sense and respond to stress rapidly. Thus, it necessitates that the composition, biosynthesis of its components and organization into a membrane under stress conditions be well-informed. Therefore, future research should direct studies that evaluate how AMF influence the cell wall under saline conditions.
Directing future AM-salinity research to understand the above-mentioned challenges will immensely improve our understanding of the mechanisms of AMF facilitated salinity tolerance in host plants in order to obtain maximum benefit from AM symbiosis under salinity stress.
Author Contributions
HE, TD, SG, and RK jointly conceptualized and wrote the manuscript, contributing major parts of the literature survey. All the authors have collectively reviewed the manuscript and approved it.
Conflict of Interest Statement
The authors declare that the research was conducted in the absence of any commercial or financial relationships that could be construed as a potential conflict of interest.
Acknowledgments
The authors gratefully acknowledge Research Council of University of Delhi for continued support, New Delhi, India. TD and SG also acknowledge Council of Scientific and Industrial Research for Research Fellowships.
Abbreviations
ABA, abscisic acid; AI, acid invertase; AM, arbuscular mycorrhiza; AMF, arbuscular mycorrhizal fungi; APX, ascorbate peroxidase; AsA, ascorbate; BR, brassinosteroids; CAT, catalase; Ci, intercellular CO2 content; CK, cytokinin; CNGCs, cyclic nucleotide-gated channels; Cu, copper; DHA, dehydroascorbate; F0, minimal fluorescence; FAO, Food and Agriculture Organization; Fm, maximal fluorescence; Fv/Fm, quantum yield of photosystem II; GA, gibberellic acid; GR, glutathione reductase; GSH, reduced glutathione; GSSG, oxidized glutathione; H2O2, hydrogen peroxide; HATs, high affinity transporters; HKT, high affinity potassium transporter; JA, jasmonic acid; K, potassium; M, mycorrhizal; N, nitrogen; NaCl, sodium chloride; NAD-MDH, NAD-dependent malate dehydrogenase; NADP-MDH, NADP-dependent malate dehydrogenase; NADP-ME, NADP-malic enzyme; NHX, sodium/hydrogen exchanger; NM, non-mycorrhizal; NO, nitric oxide; NPQ, non-photochemical quenching; NR, nitrate reductase; .O2-, singlet oxygen; O2-, superoxide anions; OH, hydroxyl ions; P, phosphorus; PC5S, pyrroline-5-carboxylate synthase; PEPC, phospho-enol-pyruvate carboxylase; Pi, intrinsic phosphate concentration; PIP, plasma membrane intrinsic proteins; POX, peroxidase; PPBK, pyruvate orthophosphate dikanase; PSII, photosystem II; Put, putreseine; ROS, reactive oxygen species; SA, salicylic acid; SKOR, outward rectifying K+ channel; SLs, strigolactones; SOD, superoxide dismutase; SOS1, salt overly sensitive 1; Spd, spermidine; Spm, spermine; SPS, sucrose phosphate synthase; SS, sucrose synthase; SULTR, sulfate transporters; TIPs, tonoplast intrinsic proteins; TPP, trehalose-6-phosphate phosphatase; TPS, trehalose-6-phosphate synthase; TRE, trehalase; TSS, total soluble sugar; Zn, zinc; ΦPSII, quantum efficiency of photosystem II.
References
Abd-Allah, E. F., Hashem, A., Alqarawi, A. A., Bahkali, A. H., and Alwhibi, M. S. (2015). Enhancing growth performance and systemic acquired resistance of medicinal plant Sesbania sesban (L.) Merr using arbuscular mycorrhizal fungi under salt stress. Saudi J. Biol. Sci. 22, 274–283. doi: 10.1016/j.sjbs.2015.03.004
Abdel-Fattah, G. M., and Asrar, A. W. A. (2012). Arbuscular mycorrhizal fungal application to improve growth and tolerance of wheat (Triticum aestivum L.) plants grown in saline soil. Acta Physiol. Plant. 34, 267–277. doi: 10.1007/s11738-011-0825-6
Abo-Doma, A., Edrees, S., and Abdel-Aziz, S. H. (2016). The effect of mycorrhiza growth and expression of some genes in barley. Egypt. J. Genet. Cytol. 40, 301–313. doi: 10.21608/ejgc.2011.10794
Agusti, J., Herold, S., Schwarz, M., Sanchez, P., Ljung, K., Dun, E. A., et al. (2011). Strigolactone signaling is required for auxin-dependent stimulation of secondary growth in plants. Proc. Natl. Acad. Sci. U.S.A. 13, 20242–20247. doi: 10.1073/pnas.1111902108
Allen, J. W., and Shachar-Hill, Y. (2009). Sulfur transfer through an arbuscular mycorrhiza. Plant Physiol. 149, 549–560. doi: 10.1104/pp.108.129866
Alqarawi, A. A., Abd-Allah, E. F., and Hashem, A. (2014). Alleviation of salt-induced adverse impact via mycorrhizal fungi in Ephedra aphylla Forssk. J. Plant Interact. 9, 802–810. doi: 10.1080/17429145.2014.949886
Ashraf, M., and Foolad, M. R. (2007). Roles of glycine betaine and proline in improving plant abiotic stress resistance. Environ. Exp. Bot. 59, 206–216. doi: 10.1016/j.envexpbot.2005.12.006
Asins, M. J., Villalta, I., Aly, M. M., Olias, R., Álvarez De Morales, P. A. Z., Huertas, R., et al. (2013). Two closely linked tomato HKT coding genes are positional candidates for the major tomato QTL involved in Na+/K+ homeostasis. Plant Cell Environ. 36, 1171–1191. doi: 10.1111/pce.12051
Augé, R. M., Toler, H. D., and Saxton, A. M. (2014). Arbuscular mycorrhizal symbiosis and osmotic adjustment in response to NaCl stress: a meta-analysis. Front. Plant Sci. 5:562. doi: 10.3389/fpls.2014.00562
Azcon-G de Aguilar, C., Azcón, R., and Barea, J. M. (1979). Endomycorrhizal fungi and Rhizobium as biological fertilisers for Medicago sativa in normal cultivation. Nature 279, 325–327. doi: 10.1038/279325a0
Baker, N. R. (2008). Chlorophyll fluorescence: a probe of photosynthesis in vivo. Annu. Rev. Plant Biol. 59, 89–113. doi: 10.1146/annurev.arplant.59.032607.092759
Ballicora, M. A., Iglesias, A. A., and Preiss, J. (2004). ADP-glucose pyrophosphorylase: a regulatory enzyme for plant starch synthesis. Photosynth. Res. 79, 1–24. doi: 10.1023/B:PRES.0000011916.67519.58
Beltrano, J., Ruscitti, M., Arango, M. C., and Ronco, M. (2013). Effects of arbuscular mycorrhiza inoculation on plant growth, biological and physiological parameters and mineral nutrition in pepper grown under different salinity and P levels. Soil Sci. Plant Nutr. 13, 123–141. doi: 10.4067/S0718-95162013005000012
Benavídes, M. P., Marconi, P. L., Gallego, S. M., Comba, M. E., and Tomaro, M. L. (2000). Relationship between antioxidant defence systems and salt tolerance in Solanum tuberosum. Funct. Plant Biol. 27, 273–278. doi: 10.1071/PP99138
Bolan, N. S. (1991). A critical review on the role of mycorrhizal fungi in the uptake of phosphorus by plants. Plant Soil 134, 189–207. doi: 10.1007/BF00012037
Borde, M., Dudhane, M., and Jite, P. (2011). Growth photosynthetic activity and antioxidant responses of mycorrhizal and non-mycorrhizal bajra (Pennisetum glaucum) crop under salinity stress condition. Crop Prot. 30, 265–271. doi: 10.1016/j.cropro.2010.12.010
Briviba, K., Klotz, L. O., and Sies, H. (1998). Toxic and signaling effects of photochemically or chemically generated singlet oxygen in biological systems. Biol. Chem. 378, 1259–1265.
Burleigh, S. H., Kristensen, B. K., and Bechmann, I. E. (2003). A plasma membrane zinc transporter from Medicago truncatula is up-regulated in roots by Zn fertilization, yet down-regulated by arbuscular mycorrhizal colonization. Plant Mol. Biol. 52, 1077–1088. doi: 10.1023/A:1025479701246
Campanelli, A., Ruta, C., De Mastro, G., and Morone-Fortunato, I. (2013). The role of arbuscular mycorrhizal fungi in alleviating salt stress in Medicago sativa L. var. icon. Symbiosis 59, 65–76. doi: 10.1007/s13199-012-0191-1
Chang, B., Yang, L., Cong, W., Zu, Y., and Tang, Z. (2014). The improved resistance to high salinity induced by trehalose is associated with ionic regulation and osmotic adjustment in Catharanthus roseus. Plant Physiol. Biochem. 77, 140–148. doi: 10.1016/j.plaphy.2014.02.001
Chang, W., Sui, X., Fan, X. X., Jia, T. T., and Song, F. Q. (2018). Arbuscular mycorrhizal symbiosis modulates antioxidant response and ion distribution in salt-stressed Elaeagnus angustifolia seedlings. Front. Microbiol. 9:652. doi: 10.3389/fmicb.2018.00652
Chaves, M. M., Flexas, J., and Pinheiro, C. (2009). Photosynthesis under drought and salt stress: regulation mechanisms from whole plant to cell. Ann. Bot. 103, 551–560. doi: 10.1093/aob/mcn125
Chen, J., Zhang, H., Zhang, X., and Tang, M. (2017). Arbuscular mycorrhizal symbiosis alleviates salt stress in black locust through improved photosynthesis, water status, and K+/Na+ Homeostasis. Front. Plant Sci. 8:1739. doi: 10.3389/fpls.2017.01739
Chen, T. H., and Murata, N. (2011). Glycinebetaine protects plants against abiotic stress: mechanisms and biotechnological applications. Plant Cell Environ. 34, 1–20. doi: 10.1111/j.1365-3040.2010.02232.x
Chinnusamy, V., Jagendorf, A., and Zhu, J. K. (2005). Understanding and improving salt tolerance in plants. Crop Sci. 45, 437–448. doi: 10.2135/cropsci2005.0437
Colmer, T. D., Munns, R., and Flowers, T. J. (2006). Improving salt tolerance of wheat and barley: future prospects. Aust. J. Exp. Agric. 45, 1425–1443. doi: 10.1071/EA04162
Crawford, N. M., and Glass, A. D. (1998). Molecular and physiological aspects of nitrate uptake in plants. Trends Plant Sci. 3, 389–395. doi: 10.1016/S1360-1385(98)01311-9
Cuin, T. A., Bose, J., Stefano, G., Jha, D., Tester, M., Mancuso, S., et al. (2011). Assessing the role of root plasma membrane and tonoplast Na+/H+ exchangers in salinity tolerance in wheat: in planta quantification methods. Plant Cell Environ. 34, 947–961. doi: 10.1111/j.1365-3040.2011.02296.x
da Silva, E. C., Nogueira, R. J. M. C., de Araújo, F. P., de Melo, N. F., and de Azevedo Neto, A. D. (2008). Physiological responses to salt stress in young umbu plants. Environ. Exp. Bot. 63, 147–157. doi: 10.1016/j.envexpbot.2007.11.010
Davenport, R. J., Muñoz-Mayor, A., Jha, D., Essah, P. A., Rus, A., and Tester, M. (2007). The Na+ transporter AtHKT1; 1 controls retrieval of Na+ from the xylem in Arabidopsis. Plant Cell Environ. 30, 497–507. doi: 10.1111/j.1365-3040.2007.01637.x
de Saint Germain, A., Ligerot, Y., Dun, E. A., Pillot, J. P., Ross, J. J., Beveridge, C. A., et al. (2013). Strigolactones stimulate internode elongation independently of gibberellins. Plant Physiol. 163, 1012–1025. doi: 10.1104/pp.113.220541
Doubnerová, V., and Ryšlavá, H. (2011). What can enzymes of C4 photosynthesis do for C3 plants under stress? Plant Sci. 180, 575–583. doi: 10.1016/j.plantsci.2010.12.005
Duan, J. J., Li, J., Guo, S. R., and Kang, Y. Y. (2008). Exogenous spermidine affects polyamine metabolism in salinity stressed Cucumis sativus roots and enhances short term salinity tolerance. J. Plant Physiol. 165, 1620–1635. doi: 10.1016/j.jplph.2007.11.006
El-Tayeb, M. A. (2005). Response of barley grains to the interactive e. ect of salinity and salicylic acid. Plant Growth Regul. 45, 215–224. doi: 10.1007/s10725-005-4928-1
Estrada, B., Aroca, R., Maathuis, F. J., Barea, J. M., and Ruiz-Lozano, J. M. (2013). Arbuscular mycorrhizal fungi native from a Mediterranean saline area enhance maize tolerance to salinity through improved ion homeostasis. Plant Cell Environ. 36, 1771–1782. doi: 10.1111/pce.12082
Evelin, H., Giri, B., and Kapoor, R. (2012). Contribution of Glomus intraradices inoculation to nutrient acquisition and mitigation of ionic imbalance in NaCl-stressed Trigonella foenum-graecum. Mycorrhiza 22, 203–217. doi: 10.1007/s00572-011-0392-0
Evelin, H., Giri, B., and Kapoor, R. (2013). Ultrastructural evidence for AMF mediated salt stress mitigation in Trigonella foenum-graecum. Mycorrhiza 23, 71–86. doi: 10.1007/s00572-012-0449-8
Evelin, H., and Kapoor, R. (2014). Arbuscular mycorrhizal symbiosis modulates antioxidant response in salt-stressed Trigonella foenum-graecum plants. Mycorrhiza 24, 197–208. doi: 10.1007/s00572-013-0529-4
Evelin, H., Kapoor, R., and Giri, B. (2009). Arbuscular mycorrhizal fungi in alleviation of salt stress: a review. Ann. Bot. 104, 1263–1280. doi: 10.1093/aob/mcp251
Fahad, S., Nie, L., Chen, Y., Wu, C., Xiong, D., Saud, S., et al. (2015). “Crop plant hormones and environmental stress,” in Sustainable Agriculture Reviews, ed. E. Lichtfouse (Cham: Springer), 371–400. doi: 10.1007/978-3-319-09132-7_10
Felicia, V., Russia, P., Ingraffia, R., Giambalvo, D., Frenda, A. S., and Martinelli, F. (2017). Arbuscular mycorrhizal symbiosis mitigates the negative effects of salinity on durum wheat. PLoS One 12:e0184158. doi: 10.1371/journal.pone.0184158
Filleur, S., Dorbe, M. F., Cerezo, M., Orsel, M., Granier, F., Gojon, A., et al. (2001). An Arabidopsis T-DNA mutant affected in Nrt2 genes is impaired in nitrate uptake. FEBS Lett. 489, 220224. doi: 10.1016/S0014-5793(01)02096-8
Food and Agriculture Organization [FAO] (2015). Status of the Worlds’s Soil Resources (SWSR) – Main Report, United Nations. Rome: Food and Agriculture Organization
Foyer, C. H., and Halliwell, B. (1976). The presence of glutathione and glutathione reductase in chloroplasts: a proposed role in ascorbic acid metabolism. Planta 133, 21–25. doi: 10.1007/BF00386001
Foyer, C. H., and Noctor, G. (2005). Redox homeostasis and antioxidant signaling: a metabolic interface between stress perception and physiological responses. Plant Cell 17, 1866–1875. doi: 10.1105/tpc.105.0335
Frechilla, S., Lasa, B., Ibarretre, L., Lamfus, C., and Aparico-Tejo, P. (2001). Pea responses to saline stress is affected by the source of nitrogen nutrition (ammonium or nitrate). Plant Growth Regul. 35, 171–179. doi: 10.1023/A:1014487908495
Frosi, G., Barros, V. A., Oliveira, M. T., Santos, M., Ramos, D. G., Maia, L. C., et al. (2016). Symbiosis with AMF and leaf Pi supply increases water deficit tolerance of woody species from seasonal dry tropical forest. J. Plant Physiol. 207, 84–93. doi: 10.1016/j.jplph.2016.11.002
Frosi, G., Barros, V. A., Oliveira, M. T., Santos, M., Ramos, D. G., Maia, L. C., et al. (2017). Arbuscular mycorrhizal fungi and foliar phosphorus inorganic supply alleviate salt stress effects in physiological attributes, but only arbuscular mycorrhizal fungi increase biomass in woody species of a semiarid environment. Tree Physiol. 38, 25–36. doi: 10.1093/treephys/tpx105
Füzy, A., Biró, B., Tóth, T., Hildebrandt, U., and Bothe, H. (2008). Drought, but not salinity, determines the apparent effectiveness of halophytes colonized by arbuscular mycorrhizal fungi. J. Plant Physiol. 165, 1181–1192. doi: 10.1016/j.jplph.2007.08.010
Gadkar, V., and Rillig, M. C. (2006). The arbuscular mycorrhizal fungal protein glomalin is a putative homolog of heat shock protein 60. FEMS Microbiol. Lett. 263, 93–101. doi: 10.1111/j.1574-6968.2006.00412.x
Gamalero, E., Berta, G., Massa, N., Glick, B. R., and Lingua, G. (2010). Interactions between Pseudomonas putida UW4 and Gigaspora rosea BEG9 and their consequences for the growth of cucumber under salt-stress conditions. J. Appl. Microbiol. 108, 236–245. doi: 10.1111/j.1365-2672.2009.04414.x
Garg, A. K., Kim, J. K., Owens, T. G., Ranwala, A. P., Do Choi, Y., Kochian, L. V., et al. (2002). Trehalose accumulation in rice plants confers high tolerance levels to different abiotic stresses. Proc. Natl. Acad. Sci. U.S.A. 99, 15898–15903. doi: 10.1073/pnas.252637799
Garg, N., and Baher, N. (2013). Role of arbuscular mycorrhizal symbiosis in proline biosynthesis and metabolism of Cicer arietinum L. (chickpea) genotypes under salt stress. J. Plant Growth Regul. 32, 767–778. doi: 10.1007/s00344-013-9346-4
Garg, N., and Bhandari, P. (2016a). Interactive effects of silicon and arbuscular mycorrhiza in modulating ascorbate-glutathione cycle and antioxidant scavenging capacity in differentially salt-tolerant Cicer arietinum L. genotypes subjected to long-term salinity. Protoplasma 253, 1325–1345. doi: 10.1007/s00709-015-0892-4
Garg, N., and Bhandari, P. (2016b). Silicon nutrition and mycorrhizal inoculations improve growth, nutrient status, K+/Na+ ratio and yield of Cicer arietinum L. genotypes under salinity stress. Plant Growth Regul. 78, 371–387. doi: 10.1007/s10725-015-0099-x
Garg, N., and Bharti, A. (2018). Salicylic acid improves arbuscular mycorrhizal symbiosis, and chickpea growth and yield by modulating carbohydrate metabolism under salt stress. Mycorrhiza 28, 727–746. doi: 10.1007/s00572-018-0856-6
Garg, N., and Pandey, R. (2016). High effectiveness of exotic arbuscular mycorrhizal fungi is reflected in improved rhizobial symbiosis and trehalose turnover in Cajanus cajan genotypes grown under salinity stress. Fungal Ecol. 21, 57–67. doi: 10.1016/j.funeco.2016.04.001
Gaymard, F., Pilot, G., Lacombe, B., Bouchez, D., Bruneau, D., Boucherez, J., et al. (1998). Identification and disruption of a plant shaker-like outward channel involved in K+ release into the xylem sap. Cell 94, 647–655. doi: 10.1016/S0092-8674(00)81606-2
Geigenberger, P., and Stitt, M. (1993). Sucrose synthase catalyses a readily reversible reaction in vivo in developing potato tubers and other plant tissues. Planta 189, 329–339. doi: 10.1007/BF00194429
Gill, S. S., and Tuteja, N. (2010). Reactive oxygen species and antioxidant machinery in abiotic stress tolerance in crop plants. Plant Physiol. Biochem. 48, 909–930. doi: 10.1016/j.plaphy.2010.08.016
Giri, B., and Mukerji, K. G. (2004). Mycorrhizal inoculant alleviates salt stress in Sesbania aegyptiaca and Sesbania grandiflora under field conditions: evidence for reduced sodium and improved magnesium uptake. Mycorrhiza 14, 307–312. doi: 10.1007/s00572-003-0274-1
Graham, J. H., and Syvertsen, J. P. (1984). Influence of vesicular-arbuscular mycorrhiza on the hydraulic conductivity of roots of two citrus rootstocks. New Phytol. 97, 277–284. doi: 10.1111/j.1469-8137.1984.tb04132.x
Grattan, S. R., and Grieve, C. M. (1992). Mineral element acquisition and growth response of plants grown in saline environments. Agric. Ecosyst. Environ. 38, 275–300. doi: 10.1016/0167-8809(92)90151-Z
Grattan, S. R., and Grieve, C. M. (1999). “Mineral nutrient acquisition and response by plants grown in saline environments,” in Handbook of Plant and Crop Stress, 2, ed. M. Pessarakli (Basel, NY: CRC Press), 203–229.
Guo, L. Q., Shi, D. C., and Wang, D. L. (2010). The key physiological response to alkali stress by the alkali-resistant halophyte Puccinellia tenuiflora is the accumulation of large quantities of organic acids and into the rhyzosphere. J. Agron. Crop. Sci. 196, 123–135. doi: 10.1111/j.1439-037X.2009.00397.x
Gutjahr, C., and Paszkowski, U. (2009). Weights in the balance: jasmonic acid and salicylic acid signaling in root-biotroph interactions. Mol. Plant Microbe Interact. 22, 763–772. doi: 10.1094/MPMI-22-7-0763
Hajiboland, R., Aliasgharzadeh, N., Laiegh, S. F., and Poschenrieder, C. (2010). Colonization with arbuscular mycorrhizal fungi improves salinity tolerance of tomato (Solanum lycopersicum L.) plants. Plant Soil 331, 313–327. doi: 10.1007/s11104-009-0255-z
Hamaji, K., Nagira, M., Yoshida, K., Ohnishi, M., Oda, Y., Uemura, T., et al. (2009). Dynamic aspects of ion accumulation by vesicle traffic under salt stress in Arabidopsis. Plant Cell Physiol. 50, 2023–2033. doi: 10.1093/pcp/pcp143
Hammer, E. C., and Rillig, M. C. (2011). The influence of different stresses on glomalin levels in an arbuscular mycorrhizal fungus-salinity increases glomalin content. PLoS One 6:e28426. doi: 10.1371/journal.pone.0028426
Harris-Valle, C., Esqueda, M., Gutiérrez, A., Castellanos, A. E., Gardea, A. A., and Berbara, R. (2018). Physiological response of Cucurbita pepo var. pepo mycorrhized by Sonoran desert native arbuscular fungi to drought and salinity stresses. Braz. J. Microbiol. 49, 45–53. doi: 10.1016/j.bjm.2017.04.005
Hasegawa, P. M., Bressan, R. A., Zhu, J. K., and Bohnert, H. J. (2000). Plant cellular and molecular responses to high salinity. Annu. Rev. Plant Biol. 51, 463–499. doi: 10.1146/annurev.arplant.51.1.463
Hashem, A., Abd-Allah, E. F., Alqarawi, A. A., Aldubise, A., and Egamberdieva, D. (2015). Arbuscular mycorrhizal fungi enhances salinity tolerance of Panicum turgidum Forssk by altering photosynthetic and antioxidant pathways. J. Plant Interact. 10, 230–242. doi: 10.1080/17429145.2015.1052025
Hashem, A., Alqarawi, A. A., Radhakrishnan, R., Al-Arjani, A. B. F., Aldehaish, H. A., Egamberdieva, D., et al. (2018). Arbuscular mycorrhizal fungi regulate the oxidative system, hormones and ionic equilibrium to trigger salt stress tolerance in Cucumis sativus L. Saudi J. Biol. Sci. 25, 1102–1114. doi: 10.1016/j.sjbs.2018.03.009
Hidri, R., Barea, J. M., Mahmoud, O. M. B., Abdelly, C., and Azcón, R. (2016). Impact of microbial inoculation on biomass accumulation by Sulla carnosa provenances, and in regulating nutrition, physiological and antioxidant activities of this species under non-saline and saline conditions. J. Plant Physiol. 201, 28–41. doi: 10.1016/j.jplph.2016.06.013
Hodge, A., and Fitter, A. H. (2010). Substantial nitrogen acquisition by arbuscular mycorrhizal fungi from organic material has implications for N cycling. Proc. Natl. Acad. Sci. U.S.A. 107, 13754–13759. doi: 10.1073/pnas.1005874107
Hoff, T., Stummann, B. M., and Henningsen, K. W. (1992). Structure, function and regulation of nitrate reductase in higher plants. Physiol. Plant 84, 616–624. doi: 10.1111/j.1399-3054.1992.tb04712.x
Hu, W., Zhang, H., Chen, H., and Tang, M. (2017). Arbuscular mycorrhizas influence Lycium barbarum tolerance of water stress in a hot environment. Mycorrhiza 27, 451–463. doi: 10.1007/s00572-017-0765-0
Ibrahim, A. H., Abdel-Fattah, G. M., Eman, F. M., Abd El-Aziz, M. H., and Shohr, A. E. (2011). Arbuscular mycorrhizal fungi and spermine alleviate the adverse effects of salinity stress on electrolyte leakage and productivity of wheat plants. Phyton 51, 261–268.
Jahromi, F., Aroca, R., Porcel, R., and Ruiz-Lozano, J. M. (2008). Influence of salinity on the in vitro development of Glomus intraradices and on the in vivo physiological and molecular responses of mycorrhizal lettuce plants. Microb. Ecol. 55, 45–53. doi: 10.1007/s00248-007-9249-7
Jansen, M. A., Gaba, V., Greenberg, B. M., Mattoo, A. K., and Edelman, M. (1996). Low threshold levels of ultraviolet-B in a background of photosynthetically active radiation trigger rapid degradation of the D2 protein of photosystem-II. Plant J. 9, 693–699. doi: 10.1046/j.1365-313X.1996.9050693.x
Jung, J., and McCouch, S. (2013). Getting to the roots of it: genetic and hormonal control of root architecture. Front. Plant Sci. 4:186. doi: 10.3389/fpls.2013.00186
Kaldorf, M., Schmelzer, E., and Bothe, H. (1998). Expression of maize and fungal nitrate reductase genes in arbuscular mycorrhiza. Mol. Plant Microbe Interact. 11, 439–448. doi: 10.1094/MPMI.1998.11.6.439
Kaplan, B., Sherman, T., and Fromm, H. (2007). Cyclic nucleotide-gated channels in plants. FEBS Lett. 581, 2237–2246. doi: 10.1016/j.febslet.2007.02.017
Kapoor, R., Sharma, D., and Bhatnagar, A. K. (2008). Arbuscular mycorrhizae in micropropagation systems and their potential applications. Sci. Hortic. 116, 227–239. doi: 10.1016/j.scienta.2008.02.002
Kaur, G., and Asthir, B. (2015). Proline: a key player in plant abiotic stress tolerance. Biol. Plant. 59, 609–619. doi: 10.1007/s10535-015-0549-3
Khalloufi, M., Martínez-Andújar, C., Lachaâl, M., Karray-Bouraoui, N., Pérez-Alfocea, F., and Albacete, A. (2017). The interaction between foliar GA3 application and arbuscular mycorrhizal fungi inoculation improves growth in salinized tomato (Solanum lycopersicum L.) plants by modifying the hormonal balance. J. Plant Physiol. 214, 134–144. doi: 10.1016/j.jplph.2017.04.012
Kishor, P. K., Hong, Z., Miao, G. H., Hu, C. A. A., and Verma, D. P. S. (1995). Overexpression of [delta]-pyrroline-5-carboxylate synthetase increases proline production and confers osmotolerance in transgenic plants. Plant Physiol. 108, 1387–1394. doi: 10.1104/pp.108.4.1387
Köhler, B., and Raschke, K. (2000). The delivery of salts to the xylem. Three types of anion conductance in the plasmalemma of the xylem parenchyma of roots of barley. Plant Physiol. 122, 243–254. doi: 10.1104/pp.122.1.243
Koltai, H. (2011). Strigolactones are regulators of root development. New Phytol. 190, 545–549. doi: 10.1111/j.1469-8137.2011.03678.x
Kong, C. C., Ren, C. G., Li, R. Z., Xie, Z. H., and Wang, J. P. (2017). Hydrogen peroxide and strigolactones signaling are involved in alleviation of salt stress induced by arbuscular mycorrhizal fungus in Sesbania cannabina seedlings. J. Plant Growth Regul. 36, 734–742. doi: 10.1007/s00344-017-9675-9
Kumar, A., Sharma, S., and Mishra, S. (2010). Influence of arbuscular mycorrhizal (AM) fungi and salinity on seedling growth, solute accumulation, and mycorrhizal dependency of Jatropha curcas L. J. Plant Growth Regul. 29, 297–306. doi: 10.1007/s00344-009-9136-1
Kusano, T., Berberich, T., Tateda, C., and Takahashi, Y. (2008). Polyamines: essential factors for growth and survival. Planta 228, 367–381. doi: 10.1007/s00425-008-0772-7
Kuznetsov, V., and Shevyakova, N. I. (2010). “Polyamines and plant adaptation to saline environments,” in Desert Plants: Biology and Biotechnology, ed. K. G. Remawat (Berlin: Springer), 261–298. doi: 10.1007/978-3-642-02550-1_13
Kwak, J. M., Mori, I. C., Pei, Z. M., Leonhardt, N., Torres, M. A., Dangl, J. L., et al. (2003). NADPH oxidase AtrbohD and AtrbohF genes function in ROS-dependent ABA signaling in Arabidopsis. EMBO J. 22, 2623–2633. doi: 10.1093/emboj/cdg277
Latef, A. A. H. A., and Chaoxing, H. (2011). Effect of arbuscular mycorrhizal fungi on growth, mineral nutrition, antioxidant enzymes activity and fruit yield of tomato grown under salinity stress. Sci. Hortic. 127, 228–233. doi: 10.1016/j.scienta.2010.09.020
Läuchli, A., and Lüttge, U. (2002). Salinity: Environment-Plants-Molecules. Dordrecht: Kluwer Academic Publishers.
Levitt, J. (1980). Responses of Plants to Environmental Stress: Chilling, Freezing, and High Temperature Stresses. New York, NY: Academic Press.
Li, T., Run-Jin, L., Xin-Hua, H., and Bao-Shan, W. (2012). Enhancement of superoxide dismutase and catalase activities and salt tolerance of euhalophyte Suaeda salsa L. by mycorrhizal fungus Glomus mosseae. Pedosphere 22, 217–224. doi: 10.1016/S1002-0160(12)60008-3
Li, X., and Christie, P. (2001). Changes in soil solution Zn and pH and uptake of Zn by arbuscular mycorrhizal red clover in Zn-contaminated soil. Chemosphere 42, 201–207. doi: 10.1016/S0045-6535(00)00126-0
Lima Neto, M. C., Martins, M. D. O., Ferreira-Silva, S. L., and Silveira, J. A. G. (2015). Jatropha curcas and Ricinus communis display contrasting photosynthetic mechanisms in response to environmental conditions. Sci. Agric. 72, 260–269. doi: 10.1590/0103-9016-2014-0325
Lin, J., Wang, Y., Sun, S., Mu, C., and Yan, X. (2017). Effects of arbuscular mycorrhizal fungi on the growth, photosynthesis and photosynthetic pigments of Leymus chinensis seedlings under salt-alkali stress and nitrogen deposition. Sci. Total Environ. 576, 234–241. doi: 10.1016/j.scitotenv.2016.10.091
Liu, A., Hamel, C., Hamilton, R. I., Ma, B. L., and Smith, D. L. (2000). Acquisition of Cu, Zn, Mn and Fe by mycorrhizal maize (Zea mays L.) grown in soil at different P and micronutrient levels. Mycorrhiza 9, 331–336. doi: 10.1007/s005720050277
Liu, K. H., Huang, C. Y., and Tsay, Y. F. (1999). CHL1 is a dual-affinity nitrate transporter of Arabidopsis involved in multiple phases of nitrate uptake. Plant Cell 11, 865–874. doi: 10.1105/tpc.11.5.865
Liu, S., Guo, X., Feng, G., Maimaitiaili, B., Fan, J., and He, X. (2016). Indigenous arbuscular mycorrhizal fungi can alleviate salt stress and promote growth of cotton and maize in saline fields. Plant Soil 398, 195–206. doi: 10.1007/s11104-015-2656-5
Lunn, J. E., Delorge, I., Figueroa, C. M., Van Dijck, P., and Stitt, M. (2014). Trehalose metabolism in plants. Plant J. 79, 544–567. doi: 10.1111/tpj.12509
Maathuis, F. J. (2013). Sodium in plants: perception, signalling, and regulation of sodium fluxes. J. Exp. Bot. 65, 849–858. doi: 10.1093/jxb/ert326
Maathuis, F. J., and Amtmann, A. (1999). K+ nutrition and Na+ toxicity: the basis of cellular K+/Na+ ratios. Ann. Bot. 84, 123–133. doi: 10.1006/anbo.1999.0912
Marschner, H., and Dell, B. (1994). Nutrient uptake in mycorrhizal symbiosis. Plant Soil 159, 89–102. doi: 10.1007/BF00000098
Minocha, R., Majumdar, R., and Minocha, S. C. (2014). Polyamines and biotic stress in plants: a complex relationship. Front. Plant Sci. 5:175. doi: 10.3389/fpls.2014.00175
Miransari, M. (2010). Arbuscular mycorrhizal fungi and nitrogen uptake. Arch. Microbiol. 193, 77–81. doi: 10.1007/s00203-010-0657-6
Miransari, M. (2012). “Role of phytohormone signaling during stress,” in Environmental Adaptations and Stress Tolerance of Plants in the Era of Climate Change, eds P. Ahmad and M. Prasad (New York, NY: Springer), 381–393. doi: 10.1007/978-1-4614-0815-4_17
Mittler, R. (2002). Oxidative stress, antioxidants and stress tolerance. Trends Plant Sci. 7, 405–410. doi: 10.1016/S1360-1385(02)02312-9
Munns, R. (1993). Physiological processes limiting plant growth in saline soils: some dogmas and hypotheses. Plant Cell Environ. 16, 15–24. doi: 10.1111/j.1365-3040.1993.tb00840.x
Munns, R. (2005). Genes and salt tolerance: bringing them together. New Phytol. 167, 645–663. doi: 10.1111/j.1469-8137.2005.01487.x
Munns, R., James, R. A., and Läuchli, A. (2006). Approaches to increasing the salt tolerance of wheat and other cereals. J. Exp. Bot. 57, 1025–1043. doi: 10.1093/jxb/erj100
Munns, R., and Tester, M. (2008). Mechanisms of salinity tolerance. Annu. Rev. Plant Biol. 59, 651–681. doi: 10.1146/annurev.arplant.59.032607.092911
Murkute, A. A., Sharma, S., and Singh, S. K. (2006). Studies on salt stress tolerance of citrus rootstock genotypes with arbuscular mycorrhizal fungi. Hortic Sci. 33, 70–76. doi: 10.17221/3742-HORTSCI
Navarro, J. M., Pérez-Tornero, O., and Morte, A. (2014). Alleviation of salt stress in citrus seedlings inoculated with arbuscular mycorrhizal fungi depends on the rootstock salt tolerance. J. Plant Physiol. 171, 76–85. doi: 10.1016/j.jplph.2013.06.006
Noctor, G., and Foyer, C. H. (1998). Ascorbate and glutathione: keeping active oxygen under control. Annu. Rev. Plant Biol. 49, 249–279. doi: 10.1146/annurev.arplant.49.1.249
Olías, R., Eljakaoui, Z., Li, J. U. N., De Morales, P. A., Marín-Manzano, M. C., Pardo, J. M., et al. (2009). The plasma membrane Na+/H+ antiporter SOS1 is essential for salt tolerance in tomato and affects the partitioning of Na+ between plant organs. Plant Cell Environ. 32, 904–916. doi: 10.1111/j.1365-3040.2009.01971.x
Ouziad, F., Wilde, P., Schmelzer, E., Hildebrandt, U., and Bothe, H. (2006). Analysis of expression of aquaporins and Na+/H+ transporters in tomato colonized by arbuscular mycorrhizal fungi and affected by salt stress. Environ. Exp. Bot. 57, 177–186. doi: 10.1016/j.envexpbot.2005.05.011
Pandey, R., and Garg, N. (2017). High effectiveness of Rhizophagus irregularis is linked to superior modulation of antioxidant defence mechanisms in Cajanus cajan (L.) Millsp. genotypes grown under salinity stress. Mycorrhiza 27, 669–682. doi: 10.1007/s00572-017-0778-8
Pang, X. M., Zhang, Z. Y., Wen, X. P., Ban, Y., and Moriguchi, T. (2007). Polyamines, all-purpose players in response to environment stresses in plants. Plant Stress 1, 173–188.
Pardo, J. M., Cubero, B., Leidi, E. O., and Quintero, F. J. (2006). Alkali cation exchangers: roles in cellular homeostasis and stress tolerance. J. Exp. Bot. 57, 1181–1199. doi: 10.1093/jxb/erj114
Parihar, P., Singh, S., Singh, R., Singh, V. P., and Prasad, S. M. (2015). Effect of salinity stress on plants and its tolerance strategies: a review. Environ. Sci. Pollut. R. 22, 4056–4075. doi: 10.1007/s11356-014-3739-1
Parvaiz, A., and Satyawati, S. (2008). Salt stress and phyto-biochemical responses of plants-a review. Plant Soil Environ. 54, 89–99. doi: 10.17221/2774-PSE
Pedranzani, H., Rodríguez-Rivera, M., Gutiérrez, M., Porcel, R., Hause, B., and Ruiz-Lozano, J. M. (2016). Arbuscular mycorrhizal symbiosis regulates physiology and performance of Digitaria eriantha plants subjected to abiotic stresses by modulating antioxidant and jasmonate levels. Mycorrhiza 26, 141–152. doi: 10.1007/s00572-015-0653-4
Peng, J., Liu, J., Zhang, L., Luo, J., Dong, H., Ma, Y., et al. (2016). Effects of soil salinity on sucrose metabolism in cotton leaves. PLoS One 11:e0156241. doi: 10.1371/journal.pone.0156241
Pitman, M. G., and Läuchli, A. (2002). “Global impact of salinity and agricultural ecosystems,” in Salinity: Environment- Plants- Molecules, eds A. Läuchli and U. Lüttge (Dordrecht: Springer), 3–20. doi: 10.1007/0-306-48155-3_1
Porcel, R., Aroca, R., Azcon, R., and Ruiz-Lozano, J. M. (2016). Regulation of cation transporter genes by the arbuscular mycorrhizal symbiosis in rice plants subjected to salinity suggests improved salt tolerance due to reduced Na+ root-to-shoot distribution. Mycorrhiza 26, 673–684. doi: 10.1007/s00572-016-0704-5
Porcel, R., Aroca, R., and Ruiz-Lozano, J. M. (2012). Salinity stress alleviation using arbuscular mycorrhizal fungi. A review. Agron. Sustain. Dev. 32, 181–200. doi: 10.1007/s13593-011-0029-x
Porcel, R., Redondo-Gómez, S., Mateos-Naranjo, E., Aroca, R., Garcia, R., and Ruiz-Lozano, J. M. (2015). Arbuscular mycorrhizal symbiosis ameliorates the optimum quantum yield of photosystem II and reduces non-photochemical quenching in rice plants subjected to salt stress. J. Plant Physiol. 185, 75–83. doi: 10.1016/j.jplph.2015.07.006
Rabie, G. H. (2005). Influence of arbuscular mycorrhizal fungi and kinetin on the response of mungbean plants to irrigation with seawater. Mycorrhiza 15, 225–230. doi: 10.1007/s00572-004-0345-y
Rabie, G. H., and Almadini, A. M. (2005). Role of bioinoculants in development of salt-tolerance of Vicia faba plants under salinity stress. Afr. J. Biotechnol. 4, 210–222. doi: 10.5897/AJB2005.000-3041
Rahnama, A., Munns, R., Poustini, K., and Watt, M. (2011). A screening method to identify genetic variation in root growth response to a salinity gradient. J. Exp. Bot. 62, 69–77. doi: 10.1093/jxb/erq359
Ramel, F., Birtic, S., Ginies, C., Soubigou-Taconnat, L., Triantaphylidès, C., and Havaux, M. (2012). Carotenoid oxidation products are stress signals that mediate gene responses to singlet oxygen in plants. Proc. Natl. Acad. Sci. U.S.A. 109, 5535–5540. doi: 10.1073/pnas.1115982109
Redillas, M. C., Park, S. H., Lee, J. W., Kim, Y. S., Jeong, J. S., Jung, H., et al. (2012). Accumulation of trehalose increases soluble sugar contents in rice plants conferring tolerance to drought and salt stress. Plant Biotechnol. Rep. 6, 89–96. doi: 10.1007/s11816-011-0210-3
Redondo-Gómez, S., Mateos-Naranjo, E., Figueroa, M. E., and Davy, A. J. (2010). Salt stimulation of growth and photosynthesis in an extreme halophyte, Arthrocnemum macrostachyum. Plant Biol. 12, 79–87. doi: 10.1111/j.1438-8677.2009.00207.x
Ren, C. G., Kong, C. C., and Xie, Z. H. (2018). Role of abscisic acid in strigolactone-induced salt stress tolerance in arbuscular mycorrhizal Sesbania cannabina seedlings. BMC Plant Biol. 18:74. doi: 10.1186/s12870-018-1292-7
Rinaldelli, E., and Mancuso, S. (1996). Response of young mycorrhizal and non-mycorrhizal plants of olive tree (Olea europaea L.) to saline conditions.I. short-term electrophysiological and long-term vegetative salt effects. Adv. Hortic. Sci. 10, 126–134.
Ruiz-Lozano, J. M., and Azcón, R. (1996). Mycorrhizal colonization and drought stress as factors affecting nitrate reductase activity in lettuce plants. Agric. Ecosyst. Environ. 60, 175–181. doi: 10.1016/S0167-8809(96)01074-2
Ruiz-Lozano, J. M., Porcel, R., Azcón, C., and Aroca, R. (2012). Regulation by arbuscular mycorrhizae of the integrated physiological response to salinity in plants: new challenges in physiological and molecular studies. J. Exp. Bot. 63, 695–709. doi: 10.1093/jxb/ers126
Saeed, W., Naseem, S., and Ali, Z. (2017). Strigolactones biosynthesis and their role in abiotic stress resilience in plants: a critical review. Front. Plant Sci. 8:1487. doi: 10.3389/fpls.2017.01487
Saia, S., Rappa, V., Ruisi, P., Abenavoli, M. R., Sunseri, F., Giambalvo, D., et al. (2015). Soil inoculation with symbiotic microorganisms promotes plant growth and nutrient transporter genes expression in durum wheat. Front. Plant Sci. 6:815. doi: 10.3389/fpls.2015.00815
Sannazzaro, A. I., Echeverría, M., Albertó, E. O., Ruiz, O. A., and Menéndez, A. B. (2007). Modulation of polyamine balance in Lotus glaber by salinity and arbuscular mycorrhiza. Plant Physiol. Biochem. 45, 39–46. doi: 10.1016/j.plaphy.2006.12.008
Schnepf, A., Leitner, D., Klepsch, S., Pellerin, S., and Mollier, A. (2011). “Modelling phosphorus dynamics in the soil-plant system,” in Phosphorus in Action, eds E. Bünemann, A. Oberson, and E. Frossard (Berlin: Springer), 113–133. doi: 10.1007/978-3-642-15271-9_5
Schrader, S., and Sauter, J. J. (2002). Seasonal changes of sucrose-phosphate synthase and sucrose synthase activities in poplar wood (Populus x canadensis Moench < robusta > ) and their possible role in carbohydrate metabolism. J. Plant Physiol. 159, 833–843. doi: 10.1078/0176-1617-00730
Seemann, J. R., and Critchley, C. (1985). Effects of salt stress on the growth, ion content, stomatal behaviour and photosynthetic capacity of a salt-sensitive species. Phaseolus vulgaris L. Planta 164, 151–162. doi: 10.1007/BF00396077
Selvaraj, T., and Chellappan, P. (2006). Arbuscular mycorrhizae: a diverse personality. J. Cent. Eur. Agric. 7, 349–358.
Serbinova, E. A., and Packer, L. (1994). Antioxidant properties of α-tocopherol and α-tocotrienol. Methods Enzymol. 234, 354–366. doi: 10.1016/0076-6879(94)34105-2
Shaul, O. (2002). Magnesium transport and function in plants: the tip of the iceberg. Biometals 15, 307–321. doi: 10.1023/A:1016091118585
Shaul-Keinan, O., Gadkar, V., Ginzberg, I., Grünzweig, J. M., Chet, I., Elad, Y., et al. (2002). Hormone concentrations in tobacco roots change during arbuscular mycorrhizal colonization with Glomus intraradices. New Phytol. 154, 501–507. doi: 10.1046/j.1469-8137.2002.00388.x
Shekoofeh, E., Sepideh, H., and Roya, R. (2012). Role of mycorrhizal fungi and salicylic acid in salinity tolerance of Ocimum basilicum resistance to salinity. Afr. J. Biotechnol. 11, 2223–2235. doi: 10.5897/AJB11.1672
Sheng, M., Tang, M., Chen, H., Yang, B., Zhang, F., and Huang, Y. (2008). Influence of arbuscular mycorrhizae on photosynthesis and water status of maize plants under salt stress. Mycorrhiza 18, 287–296. doi: 10.1007/s00572-008-0180-7
Sheng, M., Tang, M., Zhang, F., and Huang, Y. (2011). Influence of arbuscular mycorrhiza on organic solutes in maize leaves under salt stress. Mycorrhiza 21, 423–430. doi: 10.1007/s00572-010-0353-z
Singh, M., Kumar, J., Singh, S., Singh, V. P., and Prasad, S. M. (2015). Roles of osmoprotectants in improving salinity and drought tolerance in plants: a review. Rev. Environ. Sci. Biotechnol. 14, 407–426. doi: 10.1007/s11157-015-9372-8
Smirnoff, N. (1993). The role of active oxygen in the response of plants to water deficit and desiccation. New Phytol. 125, 27–58. doi: 10.1111/j.1469-8137.1993.tb03863.x
Smith, S. E., Facelli, E., Pope, S., and Smith, F. A. (2010). Plant performance in stressful environments: interpreting new and established knowledge of the roles of arbuscular mycorrhizas. Plant Soil 326, 3–20. doi: 10.1007/s11104-009-9981-5
Subramanian, K. S., Tenshia, V., Jayalakshmi, K., and Ramach, V. (2009). Role of arbuscular mycorrhizal fungus (Glomus intraradices) (fungus aided) in zinc nutrition of maize. J. Agric. Biotech. Sustain. Dev. 1, 29–38. doi: 10.5897/JABSD/5DE37E539765
Sultana, N., Ikeda, T., and Itoh, R. (1999). Effect of NaCl salinity on photosynthesis and dry matter accumulation in developing rice grains. Environ. Exp. Bot. 42, 211–220. doi: 10.1016/S0098-8472(99)00035-0
Talaat, N. B., and Shawky, B. T. (2011). Influence of arbuscular mycorrhizae on yield, nutrients, organic solutes, and antioxidant enzymes of two wheat cultivars under salt stress. J. Plant Nutr. Soil Sci. 174, 283–291. doi: 10.1371/journal.pone.0196408
Talaat, N. B., and Shawky, B. T. (2013). Modulation of nutrient acquisition and polyamine pool in salt-stressed wheat (Triticum aestivum L.) plants inoculated with arbuscular mycorrhizal fungi. Acta Physiol. Plant. 35, 2601–2610. doi: 10.1007/s11738-013-1295-9
Talaat, N. B., and Shawky, B. T. (2014). Protective effects of arbuscular mycorrhizal fungi on wheat (Triticum aestivum L.) plants exposed to salinity. Environ. Exp. Bot. 98, 20–31. doi: 10.1016/j.envexpbot.2013.10.005
Thomas, C. E., McLean, L. R., Parker, R. A., and Ohlweiler, D. F. (1992). Ascorbate and phenolic antioxidant interactions in prevention of liposomal oxidation. Lipids 27, 543–550. doi: 10.1007/BF02536138
Van den Ende, W., Michiels, A., Le Roy, K., and Van Laere, A. (2002). Cloning of a vacuolar invertase from Belgian endive leaves (Cichorium intybus). Physiol. Plant. 115, 504–512. doi: 10.1034/j.1399-3054.2002.1150404.x
Van der Does, D., Boutrot, F., Engelsdorf, T., Rhodes, J., McKenna, J. F., Vernhettes, S., et al. (2017). The Arabidopsis leucine-rich repeat receptor kinase MIK2/LRR-KISS connects cell wall integrity sensing, root growth and response to abiotic and biotic stresses. PLoS Genet. 13:e1006832. doi: 10.1371/journal.pgen.1006832
Viljanen, K., Sundberg, S., Ohshima, T., and Heinonen, M. (2002). Carotenoids as antioxidants to prevent photooxidation.Eur. J. Lipid Sci. Technol. 104, 353–359. doi: 10.1002/1438-9312(200206)104:6<353::AID-EJLT353>3.0.CO;2-5
Wang, R., Liu, D., and Crawford, N. M. (1998). The Arabidopsis CHL1 protein plays a major role in high-affinity nitrate uptake. Proc. Natl. Acad. Sci. U.S.A. 95, 15134–15139. doi: 10.1073/pnas.95.25.15134
Wang, Y., Wang, M., Li, Y., Wu, A., and Huang, J. (2018). Effects of arbuscular mycorrhizal fungi on growth and nitrogen uptake of Chrysanthemum morifolium under salt stress. PLoS One 13:e0196408. doi: 10.1371/journal.pone.0196408
Wu, D., Cai, S., Chen, M., Ye, L., Chen, Z., Zhang, H., et al. (2013). Tissue metabolic responses to salt stress in wild and cultivated barley. PLoS One 8:e55431. doi: 10.1371/journal.pone.0055431
Wu, N., Li, Z., Liu, H., and Tang, M. (2015). Influence of arbuscular mycorrhiza on photosynthesis and water status of Populus cathayana Rehder males and females under salt stress. Acta Physiol. Plant. 37, 183–197. doi: 10.1007/s11738-015-1932-6
Wu, Q. S., Zou, Y. N., Liu, W., Ye, X. F., Zai, H. F., and Zhao, L. J. (2010). Alleviation of salt stress in citrus seedlings inoculated with mycorrhiza: changes in leaf antioxidant defense systems. Plant Soil Environ. 56, 470–475. doi: 10.17221/54/2010-PSE
Xu, H., Lu, Y., and Tong, S. (2018). Effects of arbuscular mycorrhizal fungi on photosynthesis and chlorophyll fluorescence of maize seedlings under salt stress. Emir. J. Food Agric. 30, 199–204. doi: 10.9755/ejfa.2018.v30.i3.1642
Yamada, Y., Furusawa, S., Nagasaka, S., Shimomura, K., Yamaguchi, S., and Umehara, M. (2014). Strigolactone signaling regulates rice leaf senescence in response to a phosphate deficiency. Planta 240, 399–408. doi: 10.1007/s00425-014-2096-0
Yang, S., and Cui, L. (2009). The action of aquaporins in cell elongation, salt stress and photosynthesis. Chin. J. Biotechnol. 25, 321–327.
Yang, X., Liang, Z., Wen, X., and Lu, C. (2008). Genetic engineering of the biosynthesis of glycinebetaine leads to increased tolerance of photosynthesis to salt stress in transgenic tobacco plants. Plant Mol. Biol. 66, 73–86. doi: 10.1007/s11103-007-9253-9
Yang, Y., and Guo, Y. (2017). Elucidating the molecular mechanisms mediating plant salt-stress responses. New Phytol. 217, 523–539. doi: 10.1111/nph.14920
Yang, Y., Tang, M., Sulpice, R., Chen, H., Tian, S., and Ban, Y. (2014). Arbuscular mycorrhizal fungi alter fractal dimension characteristics of Robinia pseudoacacia L. seedlings through regulating plant growth, leaf water status, photosynthesis, and nutrient concentration under drought stress. J. Plant Growth Regul. 33, 612–625. doi: 10.1007/s00344-013-9410-0
Yu, J., Sun, L., Fan, N., Yang, Z., and Huang, B. (2015). Physiological factors involved in positive effects of elevated carbon dioxide concentration on Bermudagrass tolerance to salinity stress. Environ. Exp. Bot. 115, 20–27. doi: 10.1016/j.envexpbot.2015.02.003
Zapata, P. J., Serrano, M., Garcíalegaz, M. F., Pretel, M. T., and Botella, M. A. (2017). Short term effect of salt shock on ethylene and polyamines depends on their plant sensitivity. Front. Plant Sci. 8:855. doi: 10.3389/fpls.2017.00855
Zhang, H. S., Qin, F. F., Qin, P., and Pan, S. M. (2014). Evidence that arbuscular mycorrhizal and phosphate-solubilizing fungi alleviate NaCl stress in the halophyte Kosteletzkya virginica: nutrient uptake and ion distribution within root tissues. Mycorrhiza 24, 383–395. doi: 10.1007/s00572-013-0546-3
Zhang, W., Wang, C., Lu, T., and Zheng, Y. (2018). Cooperation between arbuscular mycorrhizal fungi and earthworms promotes the physiological adaptation of maize under a high salt stress. Plant Soil 423, 125–140. doi: 10.1007/s11104-017-3481-9
Zhu, X., Song, F., Liu, S., and Liu, F. (2016). Role of arbuscular mycorrhiza in alleviating salinity stress in wheat (Triticum aestivum L.) grown under ambient and elevated CO2. J. Agron. Crop Sci. 202, 486–496. doi: 10.1111/jac.12175
Zhu, X., Song, F., Liu, S., Liu, F., and Li, X. (2018). Arbuscular mycorrhiza enhances nutrient accumulation in wheat exposed to elevated CO2 and soil salinity. J. Plant Nutr. Soil Sci. 81, 836–846. doi: 10.1002/jpln.201700575
Zou, Y. N., Wu, Q. S., Huang, Y. M., Ni, Q. D., and He, X. H. (2013). Mycorrhizal-mediated lower proline accumulation in Poncirus trifoliata under water deficit derives from the integration of inhibition of proline synthesis with increase of proline degradation. PLoS One 8:e80568. doi: 10.1371/journal.pone.0080568
Keywords: antioxidants, arbuscular mycorrhizal fungi, aquaporins, ionic homeostasis, photosynthetic efficiency, osmotic balance, salinity
Citation: Evelin H, Devi TS, Gupta S and Kapoor R (2019) Mitigation of Salinity Stress in Plants by Arbuscular Mycorrhizal Symbiosis: Current Understanding and New Challenges. Front. Plant Sci. 10:470. doi: 10.3389/fpls.2019.00470
Received: 29 January 2019; Accepted: 28 March 2019;
Published: 12 April 2019.
Edited by:
Ricardo Aroca, Estación Experimental del Zaidín (EEZ), SpainReviewed by:
Juan Manuel Ruiz-Lozano, Spanish National Research Council (CSIC), SpainYoshihiro Kobae, Rakuno Gakuen University, Japan
Rosa Porcel, Universitat Politècnica de València, Spain
Copyright © 2019 Evelin, Devi, Gupta and Kapoor. This is an open-access article distributed under the terms of the Creative Commons Attribution License (CC BY). The use, distribution or reproduction in other forums is permitted, provided the original author(s) and the copyright owner(s) are credited and that the original publication in this journal is cited, in accordance with accepted academic practice. No use, distribution or reproduction is permitted which does not comply with these terms.
*Correspondence: Rupam Kapoor, kapoor_rupam@yahoo.com