- 1Centro de Ciencias Genómicas, Universidad Nacional Autónoma de México, Cuernavaca, Mexico
- 2Instituto de Investigación en Ciencias Básicas y Aplicadas, Universidad Autónoma del Estado de Morelos, Cuernavaca, Mexico
- 3Department of Biology, University of Fribourg, Fribourg, Switzerland
Salicylic acid (SA) is a plant hormone that has been described to play an essential role in the activation and regulation of multiple responses to biotic and to abiotic stresses. In particular, during plant-microbe interactions, as part of the defense mechanisms, SA is initially accumulated at the local infected tissue and then spread all over the plant to induce systemic acquired resistance at non-infected distal parts of the plant. SA can be produced by either the phenylalanine or isochorismate biosynthetic pathways. The first, takes place in the cytosol, while the second occurs in the chloroplasts. Once synthesized, free SA levels are regulated by a number of chemical modifications that produce inactive forms, including glycosylation, methylation and hydroxylation to dihydroxybenzoic acids. Glycosylated SA is stored in the vacuole, until required to activate SA-triggered responses. All this information suggests that SA levels are under a strict control, including its intra and extracellular movement that should be coordinated by the action of transporters. However, our knowledge on this matter is still very limited. In this review, we describe the most significant efforts made to date to identify the molecular mechanisms involved in SA transport throughout the plant. Additionally, we propose new alternatives that might help to understand the journey of this important phytohormone in the future.
Discovery of Salicylic Acid
Salicylic acid (SA) belongs to a group of molecules collectively named salicylates, which are phenolic compounds synthesized by plants, that possess an aromatic ring and a hydroxyl group. Even before salicylates were chemically identified, for thousands of years, humans used them as pain killers (Klessig et al., 2018). The first known evidence of this use was discovered on the dental plaque from Neanderthals fossils from El Sidrón cave (Weyrich et al., 2017). These fossils contained remains of poplar bark, suggesting that the individuals chewed the plant to relieve the pain of dental abscesses. Use of plants containing salicylates as analgesics, can be found across many ancient cultures from Europe, Asia and America (Vlot et al., 2009). Nevertheless, it was not until 1828 that salicin was purified from willow bark by Johann A. Buchner. Subsequently around 1838, salicin was separated into a sugar and an aromatic compound that could be converted into an acid, named salicylic acid (2-hydroxybenzoic acid), after the Latin name of the white willow tree Salix alba by Raffaele Piria (Vlot et al., 2009; Kumar, 2014). Free SA is poorly soluble in water and very soluble in polar organic solvents, with a pH value of a saturated aqueous solution of 2.4. SA fluoresces at 412 nm when excited at 301 nm and this property has been used to detect it in several plants (Raskin, 1992).
In 1859, Hermann Kolbe and coworkers synthesized SA, leading to an increase in its consumption due to easy availability and decreased cost. Then, years later, in order to avoid side effects induced by SA consumption (irritation and bleeding in the stomach), Felix Hoffmann reported that acetylsalicylic acid, caused less damage to the digestive system, which finally ended in the product that is nowadays used worldwide – aspirin (Vlot et al., 2009; Klessig et al., 2018). Even though the effects and benefits of aspirin in humans to treat fever, pain or swelling and to reduce the risk of heart attack, stroke and certain cancers, have been well described and studied (Klessig et al., 2018), its role as secondary metabolite in plant biology was only characterized in the late 20th century.
The Plant Hormone SA
Plant hormones have been described to play essential biological roles regulating plant growth, development, reproduction and survival; and many of these mechanisms are regulated by cross-communication and signal-transduction pathways, within which plant hormones fulfill central roles (Verhage et al., 2010; De Vleesschauwer et al., 2013).
For many years, SA was considered just one of the thousands of phenolic compounds produced by the plants, another secondary metabolite with a relatively unimportant biological function (Raskin, 1992; Métraux and Raskin, 1993). However, in 1974, SA was described for the first time as a mobile signaling molecule localized in the phloem, that can induce flowering of Xanthium strumarium and Lemna gibba, suggesting a role as a plant hormone (Cleland, 1974; Cleland and Ajami, 1974). Nevertheless, the evidence that SA was a phytohormone came from the description of its role during the thermogenesis in voodoo lily (Sauromatum guttatum) (Raskin et al., 1989). The authors identified a 100-fold increase of endogenous SA during this event that can be also specifically stimulated by exogenous SA or its derivatives, but not by other structurally related compounds. After this initial characterization, multiple reports studied the role of SA as a phytohormone, including its involvement in the resistance and tolerance to many abiotic stresses, including ozone, UV radiation, paraquat, heat, cold, metal, and salinity/osmotic stresses (Horváth et al., 2007; Yuan and Lin, 2008; Rivas-San Vicente and Plasencia, 2011; Dempsey and Klessig, 2017). In addition, there is evidence that application of SA affects multiple aspects of plant growth and development, including seed germination, vegetative growth, flowering, fruit yield, senescence, stomatal closure, thermogenesis, photosynthesis, respiration, changes in the alternative respiratory pathway, glycolysis and the Krebs cycle (Khan et al., 2015; Dempsey and Klessig, 2017; Klessig et al., 2018). Nevertheless, one of the better- characterized SA-induced responses is that involving plant-microbe interactions, described below.
SA, An Essential Regulator of Plant-Microbe Interactions
Plants are constantly interacting with millions of microorganisms, including bacteria, yeast, fungi, and viruses (Lindow and Brandl, 2003; Baldwin et al., 2017). In response, an intimate dialogue is established between plants and microbes, which modifies the growth and development of the pathogens and the induction of the plant defense responses (Aragón et al., 2017). The first line of defense is grouped into the innate immunity, which can be divided into pathogen-associated molecular pattern-triggered immunity (PTI) and effector triggered immunity (ETI). During PTI, accumulation of reactive oxygen species (ROS), mitogen activated protein kinase (MAPK)-dependent signaling cascades and transcriptional reprograming are induced, while during ETI, induction of the above PTI responses is stronger and/or more rapid, which is usually accompanied by programmed cell death or hypersensitive response at the infection site (Boller and Felix, 2009; Zipfel, 2014; Conrath et al., 2015). The effect of ETI and PTI can block the infection of unadapted pathogens, both at the local infected tissue and systemically in uninfected leaves (Craig et al., 2009). After these early events, secondary or late defense responses are triggered, including the activation of hormone-induced signaling pathways. The main hormones involved during the innate immunity are SA, jasmonic acid, and ethylene (Yang et al., 2013). However, abscisic acid, gibberellins, auxins, cytokinins, and brassinosteroids can also function as modulators of the plant immune signaling networks (Pieterse et al., 2009, 2012).
The role of SA during plant-microbe interactions, was recorded for the first time in tobacco and cucumber plants in 1990. Plants with an increased resistance to tobacco mosaic virus (TMV) showed a strong accumulation of SA, while with TMV-susceptible plants, SA levels were significantly reduced (Malamy et al., 1990). Multiple reports have been published showing the effect of SA regulating the interactions between plants and microbes and recent reviews have made detailed description of this regulation (Dempsey and Klessig, 2017; Klessig et al., 2018). Here, we describe some of the most relevant findings.
After the initial observation with tobacco and cucumber plants, several studies found that tobacco and Arabidopsis thaliana plants transformed with the bacterial gene nahG, encoding a bacterial salicylate hydroxylase that degrades SA to catechol, showed enhanced susceptibility to pathogens (Gaffney et al., 1993; Delaney et al., 1994; Lawton et al., 1995). Through genetic and biochemical analysis, the SA-dependent signaling pathway has been characterized during the past few years (Figure 1). Recently, the protein NPR1 was described as the receptor of SA (Wu et al., 2012). Uninfected plants, with low levels of SA, NPR1 forms an oligomeric complex localized in the cytosol (Figure 1A), modulating the cross talk between SA and jasmonic acid, but not inducing SA-dependent defense genes (Spoel et al., 2003). However, upon pathogen infection or treatment with exogenous SA, a change in the cellular redox system occurs, that has been associated with the SA-triggered suppression of JA responses (Holuigue et al., 2016), which induces NPR1-complex dissociation to monomers, by the reduction of disulfide links (Figure 1B) (Tada et al., 2008). These monomeric forms can then be translocated into the nucleus, where they interact with basic leucine zipper (bZIP) TGA-type transcription factors, inducing the modification of the transcriptome, including the transcriptional activation of the defense-related genes, such as PR-1 (Figure 1B) (Fu and Dong, 2013; Birkenbihl et al., 2017).
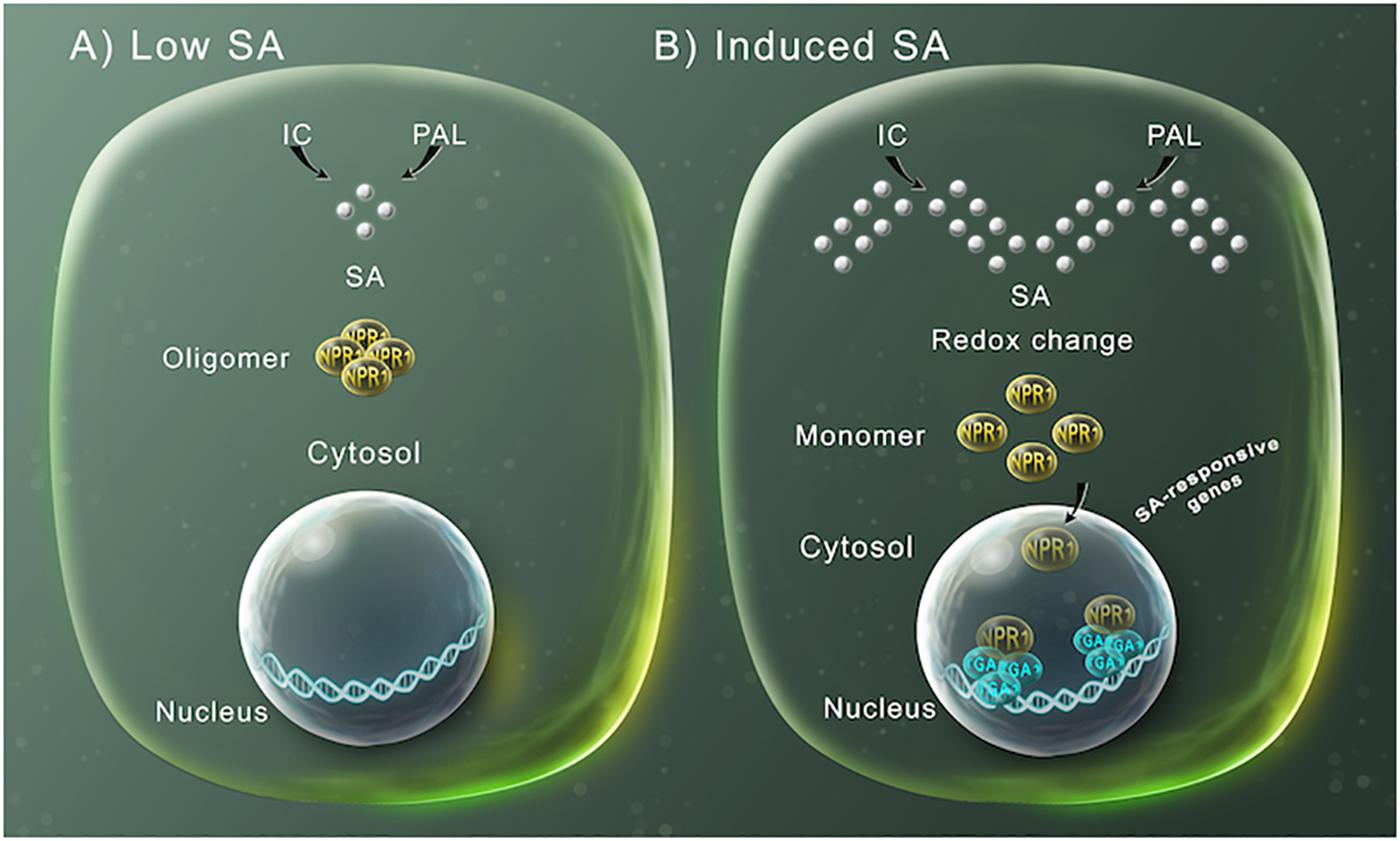
Figure 1. SA-mediated gene expression regulation for plant defense. (A) SA (silver spheres) is synthesized by either the phenylalanine ammonia-lyase (PAL) or the isochorismate (IC) pathways. At low SA levels, NPR1 occurs in the cytosol as an oligomer bound by disulfide bridges. (B) At high SA intracellular concentration, the increase in SA modifies the cellular reduction potential, which leads to NPR1 structure changes to monomers through the reduction of the intermolecular bridges by a change in redox. This allows NPR1 to enter the nucleus, where it binds to specific TGA transcriptions factors inducing the expression of SA-induced defensive response genes.
Additionally, NPR1 activity is regulated by proteasome-mediated degradation. This process is carried out by the NPR1 paralogues, NPR3 and NPR4, which are adaptors for the Cullin 3 ubiquitin E3 ligase and mediates the NPR1 degradation in a SA-dependent manner. In uninfected cells, when SA levels are low, NPR4 is proposed to maintain low NPR1 levels. However, after infection, when SA levels increase, NPR4-NPR1 interaction is disrupted, allowing the accumulation of NPR1. Additionally, when the level of SA is extremely high, NPR3 binds NPR1 leading to NPR1 degradation (Spoel et al., 2009; Fu et al., 2012; Dempsey and Klessig, 2017). NPR1 turnover ensures a correct defense activation and is required for full induction of target genes and the establishment of SA-induced responses (Spoel et al., 2009). However, until now only the ubiquitin ligase adapter function has been attributed to NPR3 and NPR4, but they also might be involved in transcriptional regulation of SA-induced defense genes. Arabidopsis thaliana plants harboring NPR3 and NPR4 mutant versions, unable to bind SA, showed constitutive repression of SA-induced immune responses and enhanced SA-induced defense gene expression, respectively (Ding, 2018).
After these initial SA-triggered responses in the local infected/treated tissue, plants can induce a long-lasting and broad-spectrum defense syndrome called systemic acquired resistance (SAR), which includes the accumulation of PR proteins and the induction of further biosynthesis of SA (Mishina and Zeier, 2007). SAR promotes a priming of defense mechanisms which is a faster and stronger response to a secondary infection inflicted by virus, bacterial and fungal pathogens (Vlot et al., 2009; Conrath et al., 2015). The chemical derivative of SA (MeSA) was initially described as the mobile signal responsible for inducing SAR (Rasmussen et al., 1991; Park et al., 2007; Vlot et al., 2009). However, while several reports suggest that SA is an important molecule to induce SAR, it is not the only one (Smith et al., 1991; Vernooij et al., 1994). Later work has described that together with SA, other compounds participate as inducers of SAR including: dicarboxylic acid azelaic acid (AzA), glycerol-3-phosphate (G3P), dehydroabietinal (DA), and pipecolic acid (Pip) [reviewed by Singh et al. (2017)]. Nevertheless, these results show the important role of SA during the interactions between plants and pathogens.
SA Biosynthesis and Storage Are Compartmentalized
Salicylic acid is probably present in all plants, however, its concentration can fluctuate widely between the species and even between members of the same family (Raskin et al., 1990). For example, tobacco leaves can contain <100 ng/g fresh weight (FW) of SA, while in potato can reach 10 μg/g FW (Malamy et al., 1992; Coquoz et al., 1998). In the model plant Arabidopsis thaliana, the basal level of SA has been quantified between 0.250 and 1 μg/g FW (Nawrath and Métraux, 1999; Wildermuth et al., 2001). Several reviews have recently made a detailed description of SA biosynthesis (Dempsey et al., 2011; Dempsey and Klessig, 2017; Klessig et al., 2018). To date, the biosynthesis of SA has not been fully defined, however, genetic and biochemical evidence pointed out that SA can be produced from two independent and compartmentalized pathways: the isochorismate (IC) pathway localized into the plastids and the phenylalanine ammonia-lyase (PAL) pathway that takes place in the cytosol (Figure 2). Both pathways begin by the accumulation of chorismic acid, produced by shikimic acid biosynthesis. In the IC pathway, chorismic acid is converted into isochorismic acid (IC) by isochorismate synthase and then through the activity of isochorismate pyruvate lyase (IPL) SA is produced in the chloroplast and afterward exported to the cytosol (Fragnière et al., 2011). On the other hand, in the PAL pathway, prephenic acid is produced from the chorismic acid by chorismate mutase (CM), then reduced to L-phenylalanine (Phe) that is converted into trans-cinnamic acid (t-CA) by PAL activity. From t-CA two metabolites can be produced: ortho-courmaric acid (o-CA) and benzaldehyde. SA can be synthesized directly from o-CA, while benzaldehyde is first transformed into benzoic acid (BA) by aldehyde oxidase (AAO) and then to SA by the activity of benzoic acid 2-hydroxylase (BA2H) (Figure 2). Once SA is synthesized, its levels are regulated by a number of chemical modifications, to produce inactive forms, including salicyloyl glucose ester (SGE), SA O-β-glucoside (SAG), methyl salicylate (MeSA), and methyl salicylate O-β-glucoside (MeSAG). These inactive molecules can be stored until required to activate the SA-triggered responses.
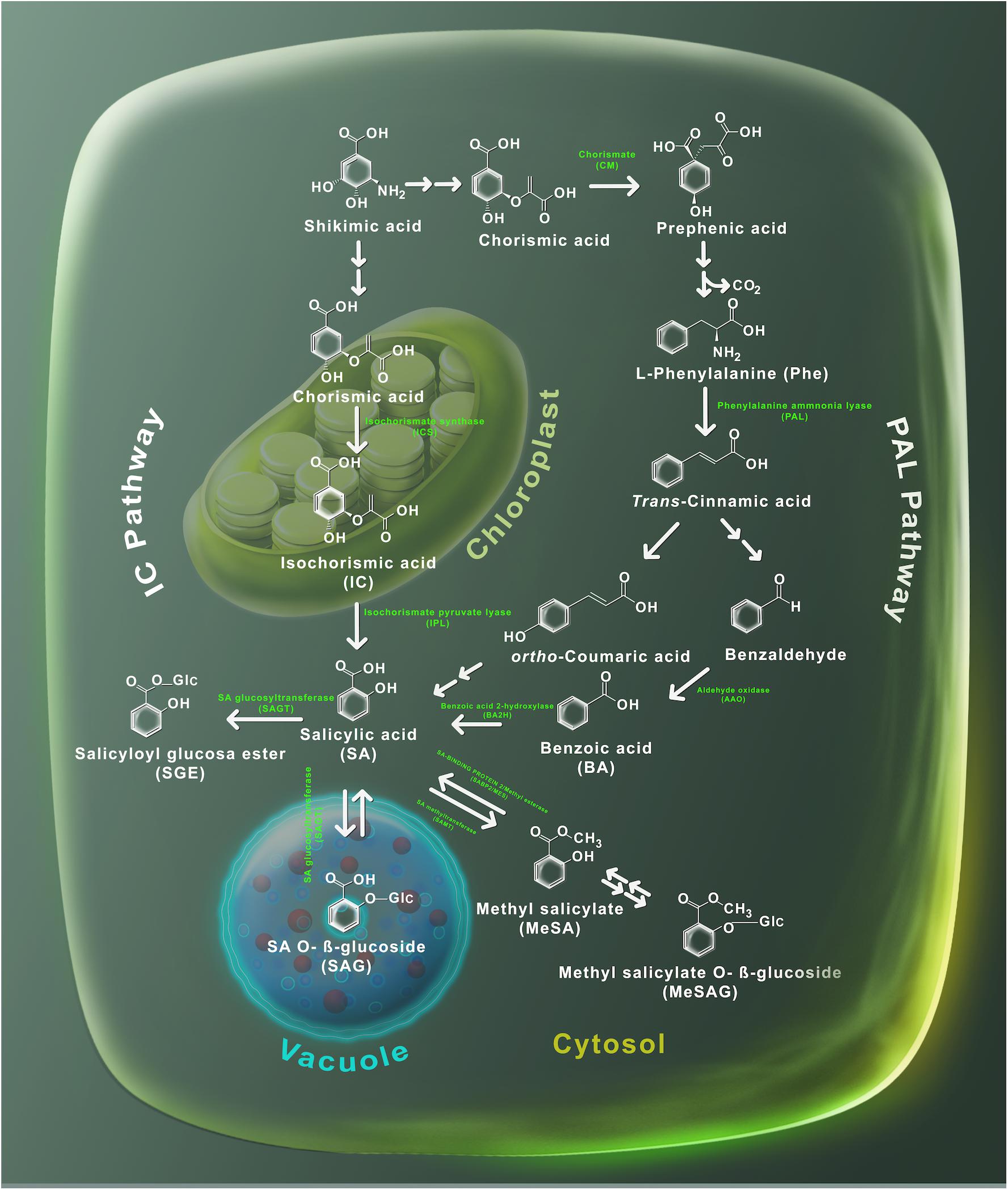
Figure 2. Metabolism of SA. Plants utilize two pathways to produce SA, the phenylalanine ammonia-lyase (PAL) and the isochorismate (IC). Shikimic acid serves as precursor in both routes. The PAL route is carried out in the cytosol, where shikimic acid is transformed into chorismic acid and then into phenylalanine by the action of the chorismic mutase enzyme. Later, the PAL enzyme uses phenylalanine to produce trans-cinnamic acid which in turn is converted into both ortho-coumaric acid and benzaldehyde. Subsequently, benzaldehyde is converted to benzoic (BA) acid by the aldehyde oxidase (AAO) enzyme. BA is transformed to SA through a reaction catalyzed by the benzoic acid hydroxylase (BA2H) enzyme. The IC pathway takes place at the chloroplast – SA is synthetized by the isochorismate synthase to generate isochorismate, which is later transformed into SA through the action of the isochorismate pyruvate lyase enzymes. Salicylic acid glucosides (SAG and SGE) are produced by glucosyltransferases (SAGT); while, methylation of SA is performed by the methyltransferases enzyme. The chemical structures and its subcellular localization of SA substrates and derivatives are shown.
In Arabidopsis thaliana, glucosylation of SA is performed by the action of UDP-glucosyltransferase enzymes UGT74F1 and UGT74F2 (Lim et al., 2002; Dean and Delaney, 2008). Both genes UGT74F1 and UGT74F2 are SA-induced and localized in the cytosol (Dean and Delaney, 2008; Park et al., 2017). The ugt74f1 mutant accumulates less SAG than ugt74f2 mutant and wild-type plants, while SGE was not formed in the ugt74f2 background (Dean and Delaney, 2008). It has been demonstrated that UGT74F1 catalyzes the formation of SAG and UGT74F2 forms primarily SGE, but also synthetizes SAG (Dean and Delaney, 2008; Dempsey et al., 2011). On the other hand, SA produces MeSA by the action of a carboxyl methyltransferase (SAMT) (Ross et al., 1999; Zubieta et al., 2003). In Arabidopsis thaliana, AtBSMT1 encodes for a carboxyl methyltransferase, which may use either BA or SA as substrates to form MeSA (Chen et al., 2003) (Figure 2). It was observed that AtBSMT1 gene expression and hence MeSA production were induced in Arabidopsis thaliana leaves by alamethicin treatment, a pore-forming peptide that emulates pathogen damage (Chen et al., 2003). Therefore, it is suggested that AtBSMT1 performs MeSA production mainly during pathogen infection (Liu et al., 2009). Mutants impaired in the expression of AtBSMT1 did not accumulate MeSA after pathogen attack, while overexpression of AtBSMT1 led to an incremented accumulation of MeSA at the infection zone (Liu et al., 2009). Interestingly, overexpression and mutants of AtBSMT1 were not able to accumulate SA or SAG at the distal leaves and they did not establish SAR (Liu et al., 2009). Likely, the overexpression of AtBSMT1 leads to excessive conversion of free SA to MeSA, which avoids the required accumulation of SA to develop SAR at the systemic leaves. A similar observation was made when Arabidopsis thaliana plants overexpress the OsBSMT1 gene from rice, which accumulated MeSA constitutively and failed to accumulate SA or SAG and were vulnerable to pathogen disease (Koo et al., 2007). Surprisingly, the increase in MeSA levels released by transgenic plants was enough to provoke SAR in nearby wild-type plants in an ICS1-independent process (Koo et al., 2007). Finally, in Nicotiana benthamiana virus-infected plants, SAR was impaired when the SAMT gene was silenced (Zhu et al., 2014).
Taken together, the fact that SA is produced into the chloroplast and in the cytosol, its inactive form (SAG) is stored in different cellular compartments (Figure 2) and afterward spread all over the plant to participate in SAR, suggests an intra and extracellular movement, that should be coordinated by the action of transporters.
The Journey of SA
Similarly, to animal hormones, phytohormones are frequently synthesized in a different place to where they develop their function, thus requiring either local or long-distance communication. Plants employ numerous delivery mechanisms that depend on the distance and direction of the transport (Bonnemain et al., 2013; Park et al., 2017). In the next sections, we examine the most exciting advances about intracellular, cell-to-cell, and long-distance transport of SA (Table 1 and Figure 3).
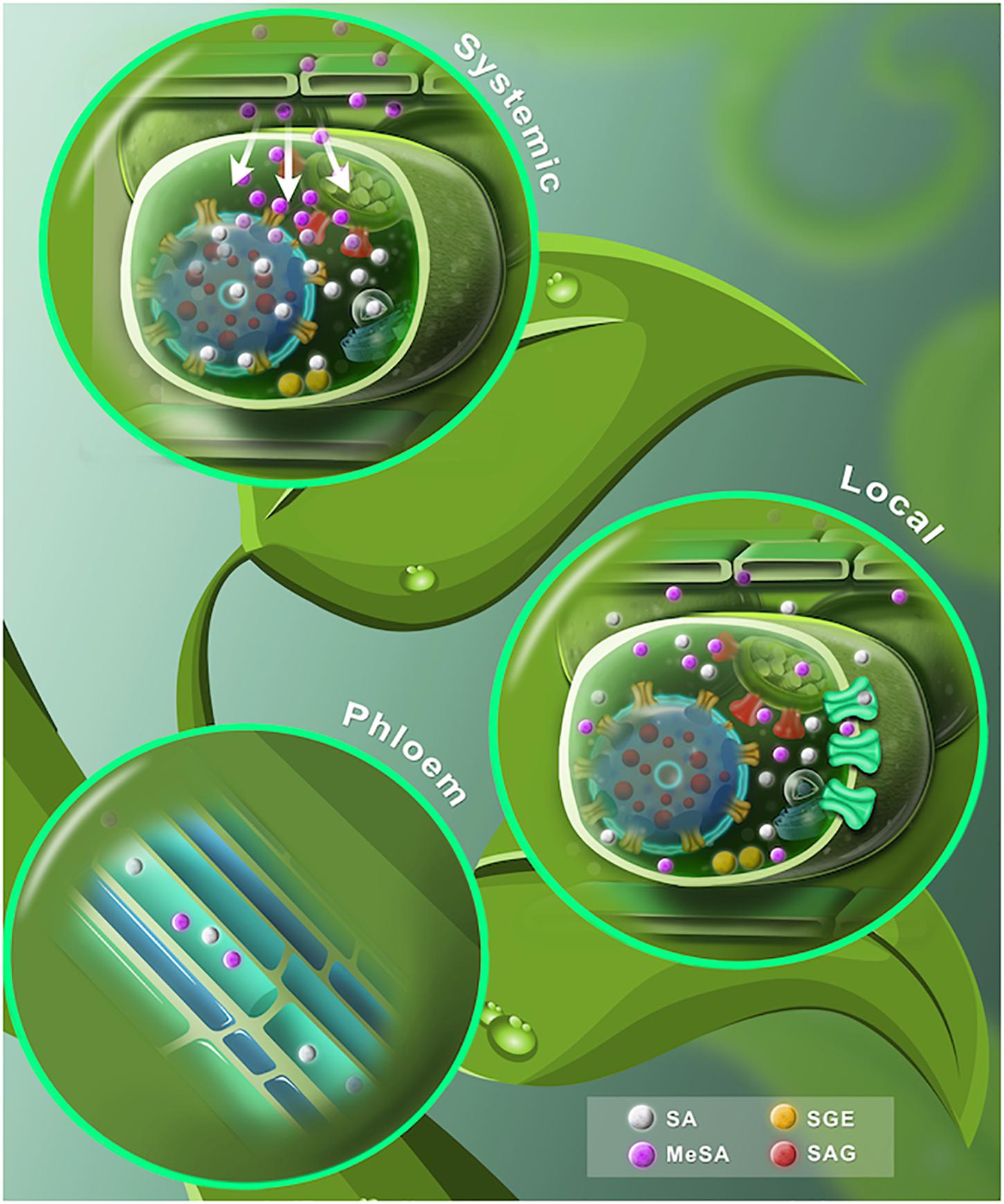
Figure 3. A proposed dynamic model of free and conjugated SA. (Lower leaf) The SA synthesized by ICS and PAL pathways is shown in gray spheres. At the chloroplast, SA is translocated to the cytosol through EDS5 transporters (red carrier). Once extruded, SA is conjugated with glucose to form SAG or SGE (red and yellow spheres, respectively). Later, SAG is transported into the vacuole by ABC transporter/H+ -antiporter systems (yellow carriers). SA may spread out to the apoplast by a carrier-mediated system (green carriers). SA is converted to volatile MeSA (purple spheres) by carboxyl methyltransferase enzyme (SAMT). (Stem) SA phloem transport may be based on a symplastic outer cell transport, phloem apoplast intake through an ion trap mechanism and an apoplast intake mediated by a carrier system. MeSA also can be found in the phloem. (Upper leaf) After a pathogen attack, SA levels rise in the primary infected tissue. SA is converted to MeSA (purple spheres). The accumulating MeSA is translocated to the uninoculated systemic tissue. MeSA is demethylated to form SA and induces de-novo synthesis of SA at the distal tissue.
Intracellular Transport: From SA Synthesis to Storage
The synthesis of SA, induced by biotic and abiotic stress, is localized in the chloroplast and afterward transported to the cytosol (Dean et al., 2005; Garcion et al., 2008; Fragnière et al., 2011). However, the molecular mechanism involved in this active transport was for a long time unknown. At the beginning of 2000’s, two Arabidopsis thaliana mutants, named SA induction–deficient (sid1 and sid2) were identified (Nawrath and Métraux, 1999). sid mutants were described to be impaired in SA biosynthesis and to show enhanced disease susceptibility to bacterial and fungal pathogens (Nawrath and Métraux, 1999). sid1 was identified to be allelic to ENHANCED DISEASE SUSCEPTIBILITY 5 (EDS5), a member of the multidrug and toxin extrusion (MATE) transporter family (Nawrath and Métraux, 1999; Nawrath et al., 2002). Arabidopsis thaliana plants overexpressing EDS5 show enhanced resistance to viruses, validating the role of EDS5 during the plant defense responses (Ishihara et al., 2008). Additionally, other reports have shown that EDS5 is localized at the chloroplast envelope membrane, suggesting that it might be involved in SA transport (Serrano et al., 2013; Yamasaki et al., 2013). Analyzing the movement of radiolabeled SA in isolated chloroplasts overexpressing EDS5 from Arabidopsis thaliana, and in a yeast heterologous system, it was confirmed that EDS5 is involved in the export of SA from the chloroplast to the cytosol (Serrano et al., 2013).
Interestingly, the EDS5 homolog (EDS5H) also shows a chloroplastic envelope location, but, however, is induced neither by pathogens nor by application of exogenous SA and its mutant eds5h is not impaired in the biosynthesis of SA (Parinthawong et al., 2015). EDS5H most likely transports other phenolic compounds, but not SA, which suggests that the protein-mediated transport system of SA is only performed by specific carriers (Parinthawong et al., 2015). This evidence suggests that EDS5, to our knowledge, is the unique SA plant transporter identified so far involved in its movement from chloroplast to cytosol.
Once synthesized or transported in the cytosol, SA can undergo conjugation with glucose in order to render an inactive form that is water soluble for storage (Figure 3). Depending on where glucose is attached, either the hydroxyl group or the carboxyl group, SA can be transformed into SA-glucoside (SAG) or SA glucose ester (SGE), respectively (Lim et al., 2002; Dean et al., 2003, 2005; Dean and Mills, 2004; Park et al., 2007). It is known that glucosides, such as flavonoid glucosides and SAG, are stable forms for storage of other small phenolic compounds, while glucose esters, such as SGE, may act as intermediary substrates in a biosynthetic pathway (Petrussa et al., 2013; Vaca et al., 2017). Interestingly, reports in tobacco and poplar suggest that the glucose ester of BA and SGE might be precursors for SA formation, indicating that SGE might accumulate in the cytosol to be used as a ready-to-use precursor for SA while SAG is accumulated in the vacuole until the plant needs it (Chong et al., 2001; Ruuhola and Julkunen-Tiitto, 2003).
It has been shown that SAG is transported into the vacuole in various plant species, such as soybean, tobacco, red beet and Arabidopsis thaliana (Table 1) (Dean and Mills, 2004; Dean et al., 2005; Vaca et al., 2017). For soybean, SAG appears to be exported into the vacuole by ATP-binding cassette (ABC) transporters (Dean and Mills, 2004). In tobacco and red beet, transport is carried out by H+-antiporters (Dean et al., 2005). While in Arabidopsis thaliana, SAG is stored in the vacuole and SGE is only found in the cytoplasm (Vaca et al., 2017). An uptake analysis of radiolabeled SAG, in vacuoles of Arabidopsis thaliana, revealed that it is translocated toward the vacuole through a MgATP-dependent process (Vaca et al., 2017). Moreover, vacuolar transport of SAG was blocked by inhibitors of ABC transporters and H+-antiporter systems, indicating that glycosylated SA transport may be performed by these types of pumps (Vaca et al., 2017).
SA-glucoside is reconverted to the active form of SA when hydrolyzed. In tobacco, SAG is reconverted to SA at the apoplast (Hennig et al., 1993), which makes sense with the extracellular localization of β-glucosidases in dicotyledonous plants and also corresponds to the role played by these enzymes during plant pathogen interactions (Morant et al., 2008). However, it is still unknown if vacuolar SAG is transported to the apoplast and/or if it is released after a pathogen-induced response. Additionally, it cannot be discounted that intracellular enzymes may hydrolyze SAG under special conditions (Dempsey et al., 2011). Taking together, these observations indicate that the intracellular level of SA and its inactive forms are under the control of transporters.
Cell to Cell Transport: Influx and Efflux of SA
Once SA is synthesized inside the cells, the next step in the SA journey is its dissemination to neighboring cells (Figure 3) (Kawano et al., 2004). Often SA is spread via the apoplast (Lim et al., 2016; Singh et al., 2017). Because of its chemical features, such as weak acid and poor water solubility, SA crosses through animal and plant cell plasma membranes by pH-dependent diffusion and carrier-mediated mechanisms (Chatton and Roch-Ramel, 1992; Takanaga et al., 1994; Chen et al., 2001; Emoto et al., 2002; Bonnemain et al., 2013). In mammals, a monocarboxylate transporter is localized at the cell plasma membrane (Enerson and Drewes, 2003). In plants, the influx of radiolabeled SA has been reported in the aquatic plant Lemna gibba, where around 90% of 10 μM SA applied to the medium was taken up in a half hour, however, the mechanisms by which the SA was internalized are unknown (Ben-Tal and Cleland, 1982). Nevertheless, after uptake, SA was localized either in the cytosol or vacuole and no further plasmodesmata transport was observed in L. gibba cells (Ben-Tal and Cleland, 1982). It is likely that SA was quickly conjugated with glucose and stored in vacuoles.
Interestingly, SA uptake was faster in a tobacco cell suspension than in L. gibba plants, taking up to 200 μM SA in just 5 min (Chen et al., 2001). Nevertheless, after 5 h, over 90% of the radiolabeled SA absorbed was released to the medium. The SA influx could be inhibited by adding a chelating agent (EGTA), but the efflux was restored by addition of Ca2+ and moreover, a protein synthesis inhibitor blocked SA excretion (Chen et al., 2001; Kawano et al., 2004). The authors suggest the presence of a SA efflux transporter that may be induced at high SA concentrations and which may involve ROS, Ca2+, de-novo protein synthesis and a protein phosphorylation signaling pathway (Chen et al., 2001; Kawano et al., 2004; Bonnemain et al., 2013). However, a constitutive SA efflux carrier involved during low SA concentrations, independent of ROS, Ca2+, and protein kinase cascade signaling has been also proposed (Kawano et al., 2004; Bonnemain et al., 2013).
Long-Distance Transport
Along with azelaic acid (AzA), glycerol-3-phosphate (G3P), methyl jasmonate (MeJA) and pipecolic acid (Pip), SA participates as a critical long-distance inducer for SAR (Conrath et al., 2015; Klessig et al., 2018). Rapid translocation of radiolabeled SA, injected to the end of tobacco leaf petioles, was observed in systemic neighboring upper and lower leaves (Ohashi et al., 2004). When 14C-labeled BA was applied to cucumber cotyledons infected with tobacco necrosis virus, labeled SA was subsequently found in the phloem and in the upper uninfected leaf (Mölders et al., 1996). During pathogen infection, SA is accumulated in the phloem, via the apoplast in Arabidopsis thaliana and tobacco plants (Yalpani et al., 1991; Zhu et al., 2014; Lim et al., 2016). Additionally, it has been reported that translocation of radiolabeled SA occurs from tobacco virus-inoculated leaves toward uninfected distal zones (Shulaev et al., 1995). SA translocation by the xylem has also been reported in tobacco and Ricinus communis seedlings, although the contribution of xylem in SA-mediated transport to develop SAR is not yet clear (Ohashi et al., 2004; Rocher et al., 2006).
In Ricinus communis seedlings, the SA phloem transport mechanisms comprise a high specificity pH-dependent carrier system, which may be placed in internal tissues, mainly at the cotyledon veins (Table 1) (Rocher et al., 2006, 2009). The authors suggest that SA phloem loading may be based on a symplastic outer cell transport, phloem apoplast intake, through an ion trap mechanism and an apoplast intake mediated by a carrier system (Figure 3) (Rocher et al., 2009).
The major barrier to prevent the free diffusion of SA is the plant cuticle (Niederl et al., 1998; Ohashi et al., 2004). Controversially, it is well known that the exogenous application of SA, either by seed priming (soaked seeds before sowing), the addition of SA to a hydroponic solution or spraying plants with SA solution, is favorable for plant growth and helps to protect them against abiotic stresses (Hayat et al., 2010). For instance, seed priming with SA, increased the activities of antioxidant enzymes in young pea (Pisum sativum L.) and leads to the de-novo synthesis of SA (Szalai et al., 2011). When sweet basil (Ocimum baslicum L.) plants were sprayed with citric and SA, supplemented with a surfactant agent, the root nutrient acquisition pattern changed to assimilating more boron and sulfur, therefore enhancing their uptake to plant shoot, thus increasing fresh biomass and photosynthetic efficiency (Ghazijahani et al., 2014). Moreover, in wheat plants (Triticum aestivum) the abiotic stress caused by the insecticide chlorpyrifos was mitigated by spraying exogenous SA; probably due to an activity improvement of antioxidant enzymes. Also, SA was able to avoid the uptake of chlorpyrifos in wheat plants (Wang and Zhang, 2017). However, exogenous applications of SA are often carried out to run-off, with the possibility that could enter via stomata and that soft mechanical damage can induce the defense mechanisms (Benikhlef et al., 2013). Nevertheless, the mechanisms involved in the assimilation and/or transport of SA under these conditions has not been described.
It has been demonstrated that cuticle is hardly permeable to SA, unless it is converted to a volatile form (Figure 3). MeSA is a volatile SA derivative and it acts as an airborne defense signal (Table 1) (Koo et al., 2007; Park et al., 2007). MeSA is mobilized through the phloem to activate SAR (Yalpani et al., 1991; Kumar and Klessig, 2003). MeSA becomes active when it is reversed to SA by the MeSA esterase activity of SA-binding protein 2 (SABP2) in the systemic tissue (Figure 2) (Seskar et al., 1998; Park et al., 2007, 2009; Vlot et al., 2008; Manosalva et al., 2010). In fact, mutations in either SAMT or SABP2, compromise SAR (Vlot et al., 2008). Altogether, this evidence supports the knowledge that the transport of SA and MeSA serves as a long-distance phloem-mobile signal and that correct homeostasis must be carried out for a successful triggering of SAR. While SA belongs to an orchestrated mechanism that induces SAR, it is not the only one – but it is a fundamental player in the activation of such a significant task of plants when facing the attack of pathogens.
What to Expect About SA Transport in the Future?
Numerous studies confirm that SA plays a crucial role during plant growth and development and in particular during the plant innate immunity (Klessig et al., 2018). In contrast, discovery and characterization of transport systems and signaling pathways remain largely elusive. While genomic approaches, radiolabeled SA tracking analysis and inhibition of channel gating, have provided insights in SA intracellular and long-distance translocation (Dean and Mills, 2004; Park et al., 2007; Serrano et al., 2013; Zhu et al., 2014; Vaca et al., 2017), there are too many open questions to be answered about the molecular and cellular mechanisms that guide the trafficking of intra- and extra-cellular SA.
We now need to move beyond, to approaches that allow identifying new SA carriers, receptors and targets. For example, the mapping of SA-mediated protein-protein interactions can be a suitable tool for this aim. It is likely that more MATE- and ABC-type transporters will be found to be involved in the transport of SA, since these transporter families have been reported to transport such a diversity of chemicals, including other phytohormones (Zhang et al., 2014; Hwang et al., 2016). Membrane protein-protein interaction and transmission surface plasmon resonance (TSPR) approaches among others, might offer tremendous insight in new transport and receptors of SA (Lertvachirapaiboon et al., 2018). For instance, in the future we shall be capable of monitoring the distribution of intracellular SA pools by biosensor imaging development, using these newly identified SA receptors (Jones, 2016).
Additionally, mathematical modeling has been used to predict phytohormone transport and signaling pathways (Voß et al., 2014) e.g., the case of the cellular and long-distance transport of auxin in tobacco and Arabidopsis thaliana, respectively (Hošek et al., 2012; Boot et al., 2016). Also, to predict sequential induction of both auxin efflux and influx carriers in the regulation of lateral root emergence in Arabidopsis thaliana (Péret et al., 2013). Modeling predictions are useful to select appropriate experimental systems and generate hypothetical models of SA transport (Otto et al., 2018), which in parallel with experimental observations will be a powerful tool to develop and establish models of the regulation mechanism for SA transport.
Author Contributions
IM-L, NYA-B, AB, and MS wrote and revised the manuscript. All the authors approved the final version of the manuscript.
Funding
MS laboratory is supported by funds from Dirección General de Asuntos del Personal Académico-UNAM PAPIIT grant IA200218.
Conflict of Interest Statement
The authors declare that the research was conducted in the absence of any commercial or financial relationships that could be construed as a potential conflict of interest.
Acknowledgments
NYA-B acknowledges a fellowship, no. 929445, from Consejo Nacional de Ciencia y Tecnología (CONACyT), México. IM-L acknowledges a post-doctoral fellowship from Dirección General de Asuntos del Personal Académico-UNAM no. CJIC/CTIC/1077/2018. The figures were carried out by Carlos Becerra.
References
Aragón, W., Reina-Pinto, J. J., and Serrano, M. (2017). The intimate talk between plants and microorganisms at the leaf surface. J. Exp. Bot. 68, 5339–5350. doi: 10.1093/jxb/erx327
Baldwin, H. E., Bhatia, N. D., Friedman, A., Eng, R. M., and Seite, S. (2017). The role of cutaneous microbiota harmony in maintaining a functional skin barrier. J. Drugs Dermatol. 16, 12–18. doi: 10.25251/skin.1.supp.138
Benikhlef, L., L’haridon, F., Abou-Mansour, E., Serrano, M., Binda, M., Costa, A., et al. (2013). Perception of soft mechanical stress in Arabidopsis leaves activates disease resistance. BMC Plant Biol. 13:133. doi: 10.1186/1471-2229-13-133
Ben-Tal, Y., and Cleland, C. F. (1982). Uptake and metabolism of (14C) salicylic acid in Lemna gibba G3. Plant Physiol. 70, 291. doi: 10.1104/pp.70.1.291
Birkenbihl, R. P., Liu, S., and Somssich, I. E. (2017). Transcriptional events defining plant immune responses. Curr. Opin. Plant Biol. 38, 1–9. doi: 10.1016/j.pbi.2017.04.004
Boller, T., and Felix, G. (2009). A renaissance of elicitors: perception of microbe-associated molecular patterns and danger signals by pattern-recognition receptors. Ann. Rev. Plant Biol. 60, 379–406. doi: 10.1146/annurev.arplant.57.032905.105346
Bonnemain, J. L., Chollet, J. F., and Rocher, F. (2013). “Transport of salicylic acid and related compounds,” in Efficiency of Salicylic Acid Application on Postharvest Perishable Crops, eds S. Hayat, A. Ahmad, and M.N. Alyemini (Dordrecht: Springer), 43–59.
Boot, K. J. M., Hille, S. C., Libbenga, K. R., Peletier, L. A., Van Spronsen, P. C., Van Duijn, B., et al. (2016). Modelling the dynamics of polar auxin transport in inflorescence stems of Arabidopsis thaliana. J. Exp. Bot. 67, 649–666. doi: 10.1093/jxb/erv471
Chatton, J. Y., and Roch-Ramel, F. (1992). Transport of salicylic acid through monolayers of a kidney epithelial cell line (LLC-PK1). J. Pharmacol. Exp. Therap. 261, 518.
Chen, F., D’auria, J. C., Tholl, D., Ross, J. R., Gershenzon, J., Noel, J. P., et al. (2003). An Arabidopsis thaliana gene for methylsalicylate biosynthesis, identified by a biochemical genomics approach, has a role in defense. Plant J. 36, 577–588. doi: 10.1046/j.1365-313X.2003.01902.x
Chen, H. J., Hou, W. C., Kuc, J., and Lin, Y. H. (2001). Ca2+-dependent and Ca2+-independent excretion modes of salicylic acid in tobacco cell suspension culture. J. Exp. Bot. 52, 1219–1226. doi: 10.1093/jexbot/52.359.1219
Chong, J., Pierrel, M.-A., Atanassova, R., Werck-Reichhart, D., Fritig, B., and Saindrenan, P. (2001). Free and conjugated benzoic acid in tobacco plants and cell cultures. Induced accumulation upon elicitation of defense responses and role as salicylic acid precursors. Plant Physiol. 125, 318. doi: 10.1104/pp.125.1.318
Cleland, C. F. (1974). Isolation of flower-inducing and flower-inhibitory factors from aphid honeydew. Plant Physiol. 54, 899. doi: 10.1104/pp.54.6.899
Cleland, C. F., and Ajami, A. (1974). Identification of the flower-inducing factor isolated from aphid honeydew as being salicylic acid. Plant Physiol. 54, 904. doi: 10.1104/pp.54.6.904
Conrath, U., Beckers, G. J. M., Langenbach, C. J. G., and Jaskiewicz, M. R. (2015). Priming for enhanced defense. Annu. Rev. Phytopathol. 53, 97–119. doi: 10.1146/annurev-phyto-080614-120132
Coquoz, J.-L., Buchala, A., and Métraux, J.-P. (1998). The biosynthesis of salicylic acid in potato plants. Plant Physiol. 117, 1095. doi: 10.1104/pp.117.3.1095
Craig, A., Ewan, R., Mesmar, J., Gudipati, V., and Sadanandom, A. (2009). E3 ubiquitin ligases and plant innate immunity. J. Exp. Bot. 60, 1123–1132. doi: 10.1093/jxb/erp059
De Vleesschauwer, D., Gheysen, G., and Höfte, M. (2013). Hormone defense networking in rice: tales from a different world. Trends Plant Sci. 18, 555–565. doi: 10.1016/j.tplants.2013.07.002
Dean, J. V., and Delaney, S. P. (2008). Metabolism of salicylic acid in wild-type, ugt74f1 and ugt74f2 glucosyltransferase mutants of Arabidopsis thaliana. Physiol. Plant. 132, 417–425. doi: 10.1111/j.1399-3054.2007.01041.x
Dean, J. V., and Mills, J. D. (2004). Uptake of salicylic acid 2-O-β-D-glucose into soybean tonoplast vesicles by an ATP-binding cassette transporter-type mechanism. Physiol. Plant. 120, 603–612. doi: 10.1111/j.0031-9317.2004.0263.x
Dean, J. V., Mohammed, L. A., and Fitzpatrick, T. (2005). The formation, vacuolar localization, and tonoplast transport of salicylic acid glucose conjugates in tobacco cell suspension cultures. Planta 221, 287–296. doi: 10.1007/s00425-004-1430-3
Dean, J. V., Shah, R. P., and Mohammed, L. A. (2003). Formation and vacuolar localization of salicylic acid glucose conjugates in soybean cell suspension cultures. Physiol. Plant. 118, 328–336. doi: 10.1034/j.1399-3054.2003.00117.x
Delaney, T. P., Uknes, S., Vernooij, B., Friedrich, L., Weymann, K., Negrotto, D., et al. (1994). A central role of salicylic acid in plant disease resistance. Science 266:1247. doi: 10.1126/science.266.5188.1247
Dempsey, D. M. A., and Klessig, D. F. (2017). How does the multifaceted plant hormone salicylic acid combat disease in plants and are similar mechanisms utilized in humans? BMC Biol. 15:23. doi: 10.1186/s12915-017-0364-8
Dempsey, D. M. A., Vlot, A. C., Wildermuth, M. C., and Klessig, D. F. (2011). Salicylic Acid Biosynthesis and Metabolism. Bellingham, WA: SPIE.
Ding, Y. (2018). Genetic Analysis of Receptor-Like Protein SNC2-Mediated Plant Resistance in Arabidopsis. PhD Thesis, The University of British Columbia, Vancouver, BC.
Emoto, A., Ushigome, F., Koyabu, N., Kajiya, H., Okabe, K., Satoh, S., et al. (2002). H+-linked transport of salicylic acid, an NSAID, in the human trophoblast cell line BeWo. Am. J. Physiol. Cell Physiol. 282, C1064–C1075. doi: 10.1152/ajpcell.00179.2001
Enerson, B. E., and Drewes, L. R. (2003). Molecular features, regulation, and function of monocarboxylate transporters: implications for drug delivery. J. Pharm. Sci. 92, 1531–1544. doi: 10.1002/jps.10389
Fragnière, C., Serrano, M., Abou-Mansour, E., Métraux, J.-P., and L’ Haridon, F. (2011). Salicylic acid and its location in response to biotic and abiotic stress. FEBS Lett. 585, 1847–1852. doi: 10.1016/j.febslet.2011.04.039
Fu, Z. Q., and Dong, X. (2013). Systemic acquired resistance: turning local infection into global defense. Annu. Rev. Plant Biol. 64, 839–863. doi: 10.1146/annurev-arplant-042811-105606
Fu, Z. Q., Yan, S., Saleh, A., Wang, W., Ruble, J., Oka, N., et al. (2012). NPR3 and NPR4 are receptors for the immune signal salicylic acid in plants. Nature 486:228. doi: 10.1038/nature11162
Gaffney, T., Friedrich, L., Vernooij, B., Negrotto, D., Nye, G., Uknes, S., et al. (1993). Requirement of salicylic acid for the induction of systemic acquired resistance. Science 261:754. doi: 10.1126/science.261.5122.754
Garcion, C., Lohmann, A., Lamodiere, E., Catinot, J., Buchala, A., Doermann, P., et al. (2008). Characterization and biological function of the ISOCHORISMATE SYNTHASE2 Gene of Arabidopsis. Plant Physiol. 147, 1279–1287. doi: 10.1104/pp.108.119420
Ghazijahani, N., Hadavi, E., and Jeong, B. R. (2014). Foliar sprays of citric acid and salicylic acid alter the pattern of root acquisition of some minerals in sweet basil (Ocimum basilicum L.). Front. Plant Sci. 5:573. doi: 10.3389/fpls.2014.00573
Hayat, Q., Hayat, S., Irfan, M., and Ahmad, A. (2010). Effect of exogenous salicylic acid under changing environment: a review. Environ. Exp. Bot. 68, 14–25. doi: 10.1016/j.envexpbot.2009.08.005
Hennig, J., Malamy, J., Grynkiewicz, G., Indulski, J., and Klessig, D. F. (1993). Interconversion of the salicylic acid signal and its glucoside in tobacco. Plant J. 4, 593–600. doi: 10.1046/j.1365-313X.1993.04040593.x
Holuigue, L., Lu, H., and Greenberg, J. T. (2016). Editorial: Salicylic acid signaling networks. Front. Plant Sci. 7:238. doi: 10.3389/fpls.2016.00238
Horváth, E., Szalai, G., and Janda, T. (2007). Induction of abiotic stress tolerance by salicylic acid signaling. J. Plant Growth Regul. 26, 290–300. doi: 10.1007/s00344-007-9017-4
Hošek, P., Kubeš, M., Laòková, M., Dobrev, P. I., Klíma, P., Kohoutová, M., et al. (2012). Auxin transport at cellular level: new insights supported by mathematical modelling. J. Exp. Bot. 63, 3815–3827. doi: 10.1093/jxb/ers074
Hwang, J.-U., Song, W.-Y., Hong, D., Ko, D., Yamaoka, Y., Jang, S., et al. (2016). Plant ABC transporters enable many unique aspects of a terrestrial plant’s lifestyle. Mol. Plant 9, 338–355. doi: 10.1016/j.molp.2016.02.003
Ishihara, T., Sekine, K. T., Hase, S., Kanayama, Y., Seo, S., Ohashi, Y., et al. (2008). Overexpression of the Arabidopsis thaliana EDS5 gene enhances resistance to viruses. Plant Biol. 10, 451–461. doi: 10.1111/j.1438-8677.2008.00050.x
Jones, A. M. (2016). A new look at stress: abscisic acid patterns and dynamics at high-resolution. New Phytol. 210, 38–44. doi: 10.1111/nph.13552
Kawano, T., Furuichi, T., and Muto, S. (2004). Controlled salicylic acid levels and corresponding signaling mechanisms in plants. Plant Biotechnol. 21, 319–335. doi: 10.5511/plantbiotechnology.21.319
Khan, M. I. R., Fatma, M., Per, T. S., Anjum, N. A., and Khan, N. A. (2015). Salicylic acid-induced abiotic stress tolerance and underlying mechanisms in plants. Front. Plant Sci. 6:462. doi: 10.3389/fpls.2015.00462
Klessig, D. F., Choi, H. W., and Dempsey, D. M. A. (2018). Systemic acquired resistance and salicylic acid: past, present, and future. Mol. Plant-Microbe Interact. 31, 871–888. doi: 10.1094/MPMI-03-18-0067-CR
Koo, Y. J., Kim, M. A., Kim, E. H., Song, J. T., Jung, C., Moon, J.-K., et al. (2007). Overexpression of salicylic acid carboxyl methyltransferase reduces salicylic acid-mediated pathogen resistance in Arabidopsis thaliana. Plant Mol. Biol. 64, 1–15. doi: 10.1007/s11103-006-9123-x
Kumar, D. (2014). Salicylic acid signaling in disease resistance. Plant Sci. 228, 127–134. doi: 10.1016/j.plantsci.2014.04.014
Kumar, D., and Klessig, D. F. (2003). High-affinity salicylic acid-binding protein 2 is required for plant innate immunity and has salicylic acid-stimulated lipase activity. Proc. Natl. Acad. Sci. U.S.A. 100:16101. doi: 10.1073/pnas.0307162100
Lawton, K., Weymann, K., Friedrich, L., Vernooij, B., Uknes, S., and Ryals, J. (1995). Systemic acquired resistance in Arabidopsis requires salicylic acid but not ethylene. Mol. Plant Microbe Interact. 8, 863–870. doi: 10.1094/MPMI-8-0863
Lertvachirapaiboon, C., Baba, A., Ekgasit, S., Shinbo, K., Kato, K., and Kaneko, F. (2018). Transmission surface plasmon resonance techniques and their potential biosensor applications. Biosens. Bioelectron. 99, 399–415. doi: 10.1016/j.bios.2017.07.069
Lim, E.-K., Doucet, C. J., Li, Y., Elias, L., Worrall, D., Spencer, S. P., et al. (2002). The activity of Arabidopsis glycosyltransferases toward salicylic acid, 4-hydroxybenzoic acid, and other benzoates. J. Biol. Chem. 277, 586–592. doi: 10.1074/jbc.M109287200
Lim, G. -H., Shine, M. B., De lorenzo, L., Yu, K., Cui, W., Navarre, D., et al. (2016). Plasmodesmata localizing proteins regulate transport and signaling during systemic acquired immunity in plants. Cell Host Microbe 19, 541–549. doi: 10.1016/j.chom.2016.03.006
Lindow, S. E., and Brandl, M. T. (2003). Microbiology of the phyllosphere. Appl. Environ. Microbiol. 69, 1875–1883. doi: 10.1128/AEM.69.4.1875-1883.2003
Liu, P.-P., Yang, Y., Pichersky, E., and Klessig, D. F. (2009). Altering expression of benzoic acid/salicylic acid carboxyl methyltransferase 1 compromises systemic acquired resistance and PAMP-triggered immunity in Arabidopsis. Mol. Plant-Microbe Interact. 23, 82–90. doi: 10.1094/MPMI-23-1-0082
Liu, P. P., von Dahl, C. C., Park, S. W., and Klessig, D. F. (2011). Interconnection between methyl salicylate and lipid-based long-distance signaling during the development of systemic acquired resistance in Arabidopsis and tobacco. Plant Physiol. 155, 1762–1768. doi: 10.1104/pp.110.171694
Malamy, J., Carr, J. P., Klessig, D. F., and Raskin, I. (1990). Salicylic acid: a likely endogenous signal in the resistance response of tobacco to viral infection. Science 250:1002. doi: 10.1126/science.250.4983.1002
Malamy, J., Hennig, J., and Klessig, D. F. (1992). Temperature-dependent induction of salicylic acid and its conjugates during the resistance response to tobacco mosaic virus infection. Plant Cell 4, 359. doi: 10.1105/tpc.4.3.359
Manosalva, P. M., Park, S.-W., Forouhar, F., Tong, L., Fry, W. E., and Klessig, D. F. (2010). Methyl ESTERASE 1 (StMES1) is required for systemic acquired resistance in potato. Mol. Plant-Microbe Interact. 23, 1151–1163. doi: 10.1094/MPMI-23-9-1151
Métraux, J. P., and Raskin, I. (1993). “Role of phenolics in plant disease resistance,” in Biotechnology in Plant Disease Control, ed. E.J.W.S. Chet. (New York, NY: FBAE), 191–209.
Mishina, T. E., and Zeier, J. (2007). Pathogen-associated molecular pattern recognition rather than development of tissue necrosis contributes to bacterial induction of systemic acquired resistance in Arabidopsis. Plant J. 50, 500–513. doi: 10.1111/j.1365-313X.2007.03067.x
Mölders, W., Buchala, A., and Metraux, J. P. (1996). Transport of salicylic acid in tobacco necrosis virus-infected cucumber plants. Plant Physiol. 112, 787. doi: 10.1104/pp.112.2.787
Morant, A. V., Jørgensen, K., Jørgensen, C., Paquette, S. M., Sánchez-Pérez, R., Møller, B. L., et al. (2008). β-Glucosidases as detonators of plant chemical defense. Phytochemistry 69, 1795–1813. doi: 10.1016/j.phytochem.2008.03.006
Nawrath, C., Heck, S., Parinthawong, N., and Métraux, J. P. (2002). EDS5, an essential component of salicylic acid-dependent signaling for disease resistance in Arabidopsis, is a member of the MATE transporter family. Plant Cell 14, 275–286. doi: 10.1105/tpc.010376
Nawrath, C., and Métraux, J.-P. (1999). Salicylic acid induction–deficient mutants of Arabidopsis express PR-2 and PR-5 and accumulate high levels of camalexin after pathogen inoculation. Plant Cell 11:1393. doi: 10.2307/3870970
Niederl, S., Kirsch, T., Riederer, M., and Schreiber, L. (1998). Co-permeability of (3)H-labeled water and (14)C-labeled organic acids across isolated plant cuticles: investigating cuticular paths of diffusion and predicting cuticular transpiration. Plant Physiol. 116, 117–123. doi: 10.1104/pp.116.1.117
Ohashi, Y., Murakami, T., Mitsuhara, I., and Seo, S. (2004). Rapid down and upward translocation of salicylic acid in tobacco plants. Plant Biotechnol. 21, 95–101. doi: 10.5511/plantbiotechnology.21.95
Otto, P. D., Combrinck, J., Otto, A., Tiedt, R. L., and De Villiers, M. M. (2018). Dissipative particle dynamics investigation of the transport of salicylic acid through a simulated in vitro skin permeation model. Pharmaceuticals 11:134. doi: 10.3390/ph11040134
Parinthawong, N., Cottier, S., Buchala, A., Nawrath, C., and Métraux, J.-P. (2015). Localization and expression of EDS5H a homologue of the SA transporter EDS5. BMC Plant Biol. 15:135. doi: 10.1186/s12870-015-0518-1
Park, J., Lee, Y., Martinoia, E., and Geisler, M. (2017). Plant hormone transporters: what we know and what we would like to know. BMC Biol. 15:93. doi: 10.1186/s12915-017-0443-x
Park, S.-W., Kaimoyo, E., Kumar, D., Mosher, S., and Klessig, D. F. (2007). Methyl salicylate is a critical mobile signal for plant systemic acquired resistance. Science 318, 113–116. doi: 10.1126/science.1147113
Park, S.-W., Liu, P.-P., Forouhar, F., Vlot, A. C., Tong, L., Tietjen, K., et al. (2009). Use of a synthetic salicylic acid analog to investigate the roles of methyl salicylate and its esterases in plant disease resistance. J. Biol. Chem. 284, 7307–7317. doi: 10.1074/jbc.M807968200
Péret, B., Middleton, A. M., French, A. P., Larrieu, A., Bishopp, A., Njo, M., et al. (2013). Sequential induction of auxin efflux and influx carriers regulates lateral root emergence. Mol. Syst. Biol. 9:699. doi: 10.1038/msb.2013.43
Petrussa, E., Braidot, E., Zancani, M., Peresson, C., Bertolini, A., Patui, S., et al. (2013). Plant Flavonoids—Biosynthesis, transport and involvement in stress responses. Int. J. Mol. Sci. 14, 14950–14973. doi: 10.3390/ijms140714950
Pieterse, C. M. J., Leon-Reyes, A., Van Der Ent, S., and Van Wees, S. C. M. (2009). Networking by small-molecule hormones in plant immunity. Nat. Chem. Biol. 5, 308–316. doi: 10.1038/nchembio.164
Pieterse, C. M. J., Van Der Does, D., Zamioudis, C., Leon-Reyes, A., and Van Wees, S. C. M. (2012). Hormonal modulation of plant immunity. Ann. Rev. Cell Dev. Biol. 28, 489–521. doi: 10.1146/annurev-cellbio-092910-154055
Raskin, I. (1992). Role of salicylic acid in plants. Annu. Rev. Plant Physiol. Plant Mol. Biol. 43, 439–463. doi: 10.1146/annurev.pp.43.060192.002255
Raskin, I., Skubatz, H., Tang, W., and Meeuse, B. J. D. (1990). Salicylic acid levels in thermogenic and non-thermogenic plants. Ann. Bot. 66, 369–373. doi: 10.1093/oxfordjournals.aob.a088037
Raskin, I., Turner, I. M., and Melander, W. R. (1989). Regulation of heat production in the inflorescences of an Arum lily by endogenous salicylic acid. Proc. Natl. Acad. Sci. U.S.A. 86, 2214–2218. doi: 10.1073/pnas.86.7.2214
Rasmussen, J. B., Hammerschmidt, R., and Zook, M. N. (1991). Systemic induction of salicylic acid accumulation in cucumber after inoculation with Pseudomonas syringae pv syringae. Plant Physiol. 97, 1342. doi: 10.1104/pp.97.4.1342
Rivas-San Vicente, M., and Plasencia, J. (2011). Salicylic acid beyond defence: its role in plant growth and development. J. Exp. Bot. 62, 3321–3338. doi: 10.1093/jxb/err031
Rocher, F., Chollet, J.-F., Jousse, C., and Bonnemain, J.-L. (2006). Salicylic acid, an ambimobile molecule exhibiting a high ability to accumulate in the phloem. Plant Physiol. 141, 1684–1693. doi: 10.1104/pp.106.082537
Rocher, F., Chollet, J.-F., Legros, S., Jousse, C., Lemoine, R., Faucher, M., et al. (2009). Salicylic acid transport in Ricinus communis Involves a pH-dependent carrier system in addition to diffusion. Plant Physiol. 150:2081. doi: 10.1104/pp.109.140095
Ross, J. R., Nam, K. H., D’auria, J. C., and Pichersky, E. (1999). S-adenosyl-l-methionine:salicylic acid carboxyl methyltransferase, an enzyme involved in floral scent production and plant defense, represents a new class of plant methyltransferases. Arch. Biochem. Biophys. 367, 9–16. doi: 10.1006/abbi.1999.1255
Ruuhola, T., and Julkunen-Tiitto, R. (2003). Trade-off between synthesis of salicylates and growth of micropropagated salix pentandra. J. Chem. Ecol. 29, 1565–1588. doi: 10.1023/A:1024266612585
Serrano, M., Wang, B., Aryal, B., Garcion, C., Abou-Mansour, E., Heck, S., et al. (2013). Export of salicylic acid from the chloroplast requires the multidrug and toxin extrusion-like transporter EDS5. Plant Physiol. 162, 1815–1821. doi: 10.1104/pp.113.218156
Seskar, M., Shulaev, V., and Raskin, I. (1998). Endogenous methyl salicylate in pathogen-inoculated tobacco plants. Plant Physiol. 116, 387. doi: 10.1104/pp.116.1.387
Shulaev, V., Leon, J., and Raskin, I. (1995). Is salicylic acid a translocated signal of systemic acquired resistance in tobacco? Plant Cell 7:1691. doi: 10.1105/tpc.7.10.1691
Singh, A., Lim, G.-H., and Kachroo, P. (2017). Transport of chemical signals in systemic acquired resistance. J. Integr. Plant Biol. 59, 336–344. doi: 10.1111/jipb.12537
Smith, J. A., Hammerschmidt, R., and Fulbright, D. W. (1991). Rapid induction of systemic resistance in cucumber by Pseudomonas syringae pv. syringae. Physiol. Mol. Plant Pathol. 38, 223–235. doi: 10.1016/S0885-5765(05)80126-2
Spoel, S. H., Koornneef, A., Claessens, S. M. C., Korzelius, J. P., Van Pelt, J. A., Mueller, M. J., et al. (2003). NPR1 modulates cross-talk between salicylate- and jasmonate-dependent defense pathways through a novel function in the cytosol. Plant Cell 15, 760–770. doi: 10.1105/tpc.009159
Spoel, S. H., Mou, Z., Tada, Y., Spivey, N. W., Genschik, P., and Dong, X. (2009). Proteasome-mediated turnover of the transcription coactivator NPR1 plays dual roles in regulating plant immunity. Cell 137, 860–872. doi: 10.1016/j.cell.2009.03.038
Szalai, G., Horgosi, S., Soós, V., Majláth, I., Balázs, E., and Janda, T. (2011). Salicylic acid treatment of pea seeds induces its de novo synthesis. J. Plant Physiol. 168, 213–219. doi: 10.1016/j.jplph.2010.07.029
Tada, Y., Spoel, S. H., Pajerowska-Mukhtar, K., Mou, Z., Song, J., Wang, C., et al. (2008). Plant immunity requires conformational charges of NPR1 via S-nitrosylation and thioredoxins. Science 321:952. doi: 10.1126/science.1156970
Takanaga, H., Tamai, I., and Tsuji, A. (1994). pH-dependent and carrier-mediated transport of salicylic acid across caco-2 cells. J. Pharm. Pharmacol. 46, 567–570. doi: 10.1111/j.2042-7158.1994.tb03858.x
Vaca, E., Behrens, C., Theccanat, T., Choe, J.-Y., and Dean, J. V. (2017). Mechanistic differences in the uptake of salicylic acid glucose conjugates by vacuolar membrane-enriched vesicles isolated from Arabidopsis thaliana. Physiol. Plant. 161, 322–338. doi: 10.1111/ppl.12602
Verhage, A., Van Wees, S. C. M., and Pieterse, C. M. J. (2010). Plant immunity: it’s the hormones talking, but what do they say? Plant Physiol. 154, 536. doi: 10.1104/pp.110.161570
Vernooij, B., Friedrich, L., Morse, A., Reist, R., Kolditz-Jawhar, R., Ward, E., et al. (1994). Salicylic acid is not the translocated signal responsible for inducing systemic acquired resistance but is required in signal transduction. Plant Cell 6:959. doi: 10.1105/tpc.6.7.959
Vlot, A. C., Dempsey, D. M. A., and Klessig, D. F. (2009). Salicylic acid, a multifaceted hormone to combat disease. Annu. Rev. Phytopathol. 47, 177–206. doi: 10.1146/annurev.phyto.050908.135202
Vlot, A. C., Klessig, D. F., and Park, S.-W. (2008). Systemic acquired resistance: the elusive signal(s). Curr. Opin. Plant Biol. 11, 436–442. doi: 10.1016/j.pbi.2008.05.003
Voß, U., Bishopp, A., Farcot, E., and Bennett, M. J. (2014). Modelling hormonal response and development. Trends Plant Sci. 19, 311–319. doi: 10.1016/j.tplants.2014.02.004
Wang, C., and Zhang, Q. (2017). Exogenous salicylic acid alleviates the toxicity of chlorpyrifos in wheat plants (Triticum aestivum). Ecotoxicol. Environ. Saf. 137, 218–224. doi: 10.1016/j.ecoenv.2016.12.011
Weyrich, L. S., Duchene, S., Soubrier, J., Arriola, L., Llamas, B., Breen, J., et al. (2017). Neanderthal behaviour, diet, and disease inferred from ancient DNA in dental calculus. Nature 544:357. doi: 10.1038/nature21674
Wildermuth, M. C., Dewdney, J., Wu, G., and Ausubel, F. M. (2001). Isochorismate synthase is required to synthesize salicylic acid for plant defence. Nature 417, 562–565. doi: 10.1038/35107108
Wu, Y., Zhang, D., Chu, J. Y., Boyle, P., Wang, Y., Brindle, I. D., et al. (2012). The Arabidopsis NPR1 protein is a receptor for the plant defense hormone salicylic acid. Cell Rep. 1, 639–647. doi: 10.1016/j.celrep.2012.05.008
Yalpani, N., Silverman, P., Wilson, T. M., Kleier, D. A., and Raskin, I. (1991). Salicylic acid is a systemic signal and an inducer of pathogenesis-related proteins in virus-infected tobacco. Plant Cell 3, 809–818. doi: 10.1105/tpc.3.8.809
Yamasaki, K., Motomura, Y., Yagi, Y., Nomura, H., Kikuchi, S., Nakai, M., et al. (2013). Chloroplast envelope localization of EDS5, an essential factor for salicylic acid biosynthesis in Arabidopsis thaliana. Plant Signal. Behav. 8:e23603. doi: 10.4161/psb.23603
Yang, D.-L., Yang, Y., and He, Z. (2013). Roles of plant hormones and their interplay in rice immunity. Mol. Plant 6, 675–685. doi: 10.1093/mp/sst056
Yuan, S., and Lin, H. H. (2008). Role of salicylic acid in plant abiotic stress. Z. Naturforsch C 63, 313–320. doi: 10.1515/znc-2008-5-601
Zhang, H., Zhu, H., Pan, Y., Yu, Y., Luan, S., and Li, L. (2014). A DTX/MATE-type transporter facilitates abscisic acid efflux and modulates ABA sensitivity and drought tolerance in Arabidopsis. Mol. Plant 7, 1522–1532. doi: 10.1093/mp/ssu063
Zhu, F., Xi, D.-H., Yuan, S., Xu, F., Zhang, D.-W., and Lin, H.-H. (2014). Salicylic acid and jasmonic acid are essential for systemic resistance against Tobacco mosaic virus in Nicotiana benthamiana. Mol. Plant-Microbe Interact. 27, 567–577. doi: 10.1094/MPMI-11-13-0349-R
Zipfel, C. (2014). Plant pattern-recognition receptors. Trends Immunol. 35, 345–351. doi: 10.1016/j.it.2014.05.004
Keywords: salicylic acid, transport, phytohormone, defense response, systemic acquired resistance, plant-microbe interactions
Citation: Maruri-López I, Aviles-Baltazar NY, Buchala A and Serrano M (2019) Intra and Extracellular Journey of the Phytohormone Salicylic Acid. Front. Plant Sci. 10:423. doi: 10.3389/fpls.2019.00423
Received: 18 February 2019; Accepted: 20 March 2019;
Published: 16 April 2019.
Edited by:
Viktor Zarsky, Charles University in Prague, CzechiaReviewed by:
Tibor Janda, Centre for Agricultural Research (MTA), HungaryDaniel F. Klessig, Boyce Thompson Institute, United States
Copyright © 2019 Maruri-López, Aviles-Baltazar, Buchala and Serrano. This is an open-access article distributed under the terms of the Creative Commons Attribution License (CC BY). The use, distribution or reproduction in other forums is permitted, provided the original author(s) and the copyright owner(s) are credited and that the original publication in this journal is cited, in accordance with accepted academic practice. No use, distribution or reproduction is permitted which does not comply with these terms.
*Correspondence: Israel Maruri-López, aXNtYXJ1cmlAY2NnLnVuYW0ubXg= Mario Serrano, c2VycmFub0BjY2cudW5hbS5teA==