- 1College of Resources and Environmental Sciences, National Academy of Agriculture Green Development, Key Laboratory of Plant-Soil Interactions, Ministry of Education, China Agricultural University, Beijing, China
- 2College of Agriculture, Ludong University, Yantai, China
Manganese (Mn) and zinc (Zn) play essential roles in plants. Members of the natural resistance-associated macrophage protein (NRAMP) family transport divalent metal ions. In this research, the function of peanut (Arachis hypogaea L.) AhNRAMP1 in transporting Mn and Zn, as well as its potential for iron(Fe) and Zn biofortification was examined. AhNRAMP1 transcription was strongly induced by Mn- or Zn-deficiency in roots and stems of peanut. Yeast complementation assays suggested that AhNRAMP1 encoded a functional Mn and Zn transporter. Exogenous expression of AhNRAMP1 in tobacco and rice enhanced Mn or Zn concentrations, improving tolerance to Mn or Zn deficiency. With higher Mn concentration, transgenic plants exhibited inhibited growth under Mn excess condition; similar results were obtained under excessive Zn treatment. AhNRAMP1 expression increased biomass in transgenic tobacco and rice, as well as yield in transgenic rice grown on calcareous soil. Compared with non-transformed (NT) plants, Fe and Zn concentrations were elevated whereas concentrations of Mn, copper (Cu), and cadmium (Cd) were not enhanced. These results revealed that AhNRAMP1 contributes to Mn and Zn transport in plants and may be a candidate gene for Fe and Zn biofortification.
Introduction
Manganese and Zn are essential micronutrients for plant growth. Mn is not only required for photosynthesis but also acts as a cofactor of numerous enzymes (Ishimaru et al., 2012a). Mn deficiency in plants causes interveinal chlorosis and impairs many physiological processes, leading to reduced biomass (Schmidt et al., 2016). Zn is a structural component of many transcription factors and the only metal ion present in all six enzyme classes (Gupta et al., 2016). Zn deficiency leads to leaf chlorosis, photosynthesis inhibition, biomass reduction, and generation of reactive oxygen species (Sinclair and Krämer, 2012). Nevertheless, excessive Mn or Zn is harmful to plants. Excessive Mn results in oxidative stress and disturbs enzyme activity (Millaleo et al., 2010). Necrosis of old leaves and reduced biomass are also triggered by excessive Zn (Tsonev and Lidon, 2012).
Members of NRAMP family are critical metal transporters in plants. Of particular interest is the functions of NRAMP proteins in transporting divalent trace metals like Fe, Mn, and Zn because of their irreplaceable roles in many metabolic processes (Nevo and Nelson, 2006). Owing to the toxicity of Cd to humans and plants, many researchers explored the function of NRAMP proteins in transporting Cd apart from essential metals (Cailliatte et al., 2009). The five out of six NRAMP genes identified in the model plant Arabidopsis thaliana have been functionally characterized. AtNRAMP1, AtNRAMP3, and AtNRAMP4 participate in the transportation of Fe and Mn (Curie et al., 2000; Lanquar et al., 2005, 2010; Cailliatte et al., 2010). AtNRAMP2 encodes an Mn transporter (Gao et al., 2018). AtNRAMP4 is an identified Zn transporter (Lanquar et al., 2004). AtNRAMP6 functions as an intracellular metal transporter of Cd (Cailliatte et al., 2009). In rice (Oryza sativa), OsNRAMP1 transports Fe and Cd, but not Mn (Takahashi et al., 2011). Whereas both OsNRAMP5 and OsNRAMP6 are Fe and Mn transporter, but OsNRAMP5 can also transport Cd (Ishimaru et al., 2012b; Sasaki et al., 2012; Peris-Peris et al., 2017). OsNRAMP3 participates in remobilization of Mn, but not that of Fe, Cd, and Zn. OsNRAMP4 do not have any role in the transportation of Fe, Mn or Cd. The functional specificity of certain NRAMP proteins is distinct and needs to be elucidated side by side.
In our preliminary research, the peanut AhNRAMP1 was isolated by suppression subtractive hybridization. Evidence showed that AhNRAMP1 functioned as a Fe transporter localized at the plasma membrane (Xiong et al., 2012). Induced AhNRAMP1 expression contributed to improving Fe nutrition in peanut/maize intercropping system, especially in the vegetative growth stage (Xiong et al., 2012; Dai et al., 2018). Zn concentration of peanut shoots was also enhanced by the intercropping system (Inal et al., 2007), but whether AhNRAMP1 was involved in Zn concentration enhancement remained unknown. Besides, AhNRAMP1 also encodes a functional Cd transporter (Chen et al., 2017). These findings propelled us to verify the functions of AhNRAMP1 in transporting Mn and Zn.
Furthermore, Fe or Zn deficiency negatively influences not only yield and quality of crops but also human health (Gupta et al., 2016; Singh et al., 2017). Biofortification is an approach to improve the mineral and vitamin values of crops utilizing plant breeding, transgenic, and agronomic practices (Bouis and Saltzman, 2017). It is essential for human health to elevate Fe and Zn concentrations in the edible tissues of crops. Overexpression of Iron-Regulated Transport 1 (OsIRT1) in rice increased Fe and Zn concentrations in mature seeds and enhanced tolerance to Fe deficiency (Lee and An, 2009). Expression of HvMTP1 (Metal Tolerance Protein 1), a transition metal transporter, improved Zn accumulation in barley endosperm (Menguer et al., 2018). Expression of heterologous transporter genes also improved crop Fe and Zn nutrition. Exogenous overexpression of the MxIRT1 gene enhanced Fe and Zn concentrations in rice seeds (Tan et al., 2015). Briefly, transporter genes are indispensable to transgenic technology for their roles in Fe and Zn biofortification. Given the function of AhNRAMP1 in transporting Fe, it is vital to investigate its Fe or Zn biofortification potential under soil condition.
In the present study, we found the AhNRAMP1 functioning as Mn and Zn transporter. AhNRAMP1 transgenic tobacco and rice were more tolerant to Mn or Zn deficiency as compared to NT plants. Additionally, exogenous expression of AhNRAMP1 increased the yield of rice grown on calcareous soil. Fe and Zn concentrations were higher in transgenic plants while concentrations of Mn, Cu, and Cd were not enhanced by AhNRAMP1 expression. Consequently, we propose AhNRAMP1 as an Mn and Zn transporter and an important candidate transporter for Fe and Zn biofortification.
Materials and Methods
Plant Materials and Growth Conditions
Peanut (A. hypogaea L. cv. Luhua 14) seedlings were grown in continuously aerated nutrient solution. The nutrient solution composition was: 2.00 mM Ca(NO3)2, 0.70 mM K2SO4, 0.10 mM KCl, 0.10 mM KH2PO4, 0.50 mM MgSO4, 10.00 μM H3BO3, 0.50 μM MnSO4, 0.50 μM ZnSO4, 0.20 μM CuSO4, 0.01 μM (NH4)6Mo7O24, and 100.00 μM Fe(III)-EDTA at pH 5.8–6.0. The plants were grown in a greenhouse under a 14 h light [25°C, 300 μmol/(m2⋅s)] and 10 h dark (20°C) cycles. After germination in quartz sand for 14 days followed by growth in complete nutrient solution for 7 days, some peanut plants were transferred to a nutrient solution without additional Mn or Zn for 10 days as Mn or Zn depleted treatments while the others (triplicate replicates) still grown in complete nutrient solution were as a control.
Yeast Functional Complementation
The full-length coding sequence of AhNRAMP1 with restriction sites was amplified with the primers XbaI-AhNRAMP1-F (5′-TCTAGAATGGCAAGCGTTCTTAGACA-3′) and SacI-AhNRAMP1-R (5′-GAGCTCTTATTCCGGTAGTGGGATAT-3′). After digestion, the AhNRAMP1 cDNA was introduced into the yeast expression vector pDR195. The resulting pDR195-AhNRAMP1 constructs were then transformed into the Saccharomyces cerevisiae strains using the LiAc/SS-DNA/PEG method (Xiong et al., 2012): The S. cerevisiae strains used were the Mn uptake-defective mutant Δsmf1 (MATα his3 ade2 leu2 trp1 ura3smf1::URA3ura3::TRP1) (Thomine et al., 2000) and the Zn uptake-defective mutant Δzhy6 (MATα ade6 can1 his3 leu2 trp1 ura3 zap1Δ::TRP1) (Zhao and Eide, 1997). Empty vector pDR195 was transformed into Δsmf1 and Δzhy6 as negative controls and the S. cerevisiae WT strain DEY1457 (MAT ade6 can1 his3 leu2 trp1 ura3; Zhao and Eide, 1997) as a positive control. The transformed cells were selected and cultured on solid synthetic dropout medium without uracil. The transformed cells were diluted to an OD at 600 nm of 0.1 to 0.00001 and spotted onto the plates containing Mn- and Zn-limiting medium. The Mn-limiting medium contained 20 mM ethylene glycol-bis-β-aminoethylether-N, N, N′, N′-tetraacetic acid (EGTA; Sigma-Aldrich, St. Louis, MO, United States). The Zn-limiting medium contained 0.1 mM EDTA (Sigma-Aldrich, St. Louis, MO, United States). The spotted yeast cells were incubated at 30°C for 2∼3 days.
Generation of Transgenic Plants
The coding region of the AhNRAMP1 gene was amplified using the primers 5′-TCTAGAATGGCAAGCGTTCTTAGACAG-3′ and 5′-GAGCTCTTATTCCGGTAGTGGGATATC-3′. XbaI and SacI restriction sites were used to replace AhNRAMP1 cDNA with the β-glucuronidase (GUS) gene of E-90Ω plasmid (Kobayashi et al., 2004). The resultant plasmid has the backbone of the pIG121Hm binary vector (Hiei et al., 1994) and drives AhNRAMP1 cDNA under the control of the –273/–131 region of the barley IDS2 gene containing iron-deficiency-responsive element1 (IDE1) and IDE2, flanked by the -90/+8 region of the cauliflower mosaic virus (CaMV) 35S promoter and the Tobacco mosaic virus 5′ leader (Ω) sequence. The constructed plasmid was introduced into Agrobacterium tumefaciens strain C58 by electroporation and then transformed into tobacco (Nicotiana tabacum L. cv. Petit-Havana SR1) using the standard leaf-disk method. E-90Ω rice lines transformation was conducted via an Agrobacterium-mediated method (Kobayashi et al., 2001) by using the rice cultivar O. sativa L. Tsukinohikari. We also generated AhNRAMP1 transgenic rice using a plasmid driving the GUS gene under control of the 800 bp upstream region of the OsIRT1 gene (Ishimaru et al., 2006). The GUS gene of the OsIRT1 promoter plasmid was replaced by the AhNRAMP1 cDNA using the XbaI and SacI restriction sites. The above construct was introduced into A. tumefaciens strain C58 by electroporation. Rice transformation was conducted according to the above method described for E-90Ω rice line transformation.
Design for Hydroponic and Pot Experiments
T5 transgenic E-90Ω tobacco and rice, as well as transgenic rice induced by the OsIRT1 promoter, were generated and germinated on MS medium containing hygromycin B (50 mg/L). NT seeds were germinated on MS medium without hygromycin B. After growing 2–3 weeks for tobacco or about 4 weeks for rice in MS medium, then plants were transferred to continuously aerated nutrient solution in a greenhouse under 14 h light [25°C, 300 μmol/(m2⋅s)] and 10 h dark (20°C) cycles. The nutrient solution composition for tobacco was the same as described above for peanut. The rice nutrient solution consisted of 1.42 mM NH4NO3, 0.32 mM NaH2PO4⋅2H2O, 0.50 mM K2SO4, 1.00 mM CaCl2, 1.65 mM MgSO4⋅7H2O, 9.00 μM MnCl2⋅4H2O, 0.40 μM ZnSO4⋅7H20, 0.16 μM CuSO4, 20.00 μM Fe(III)-EDTA, 3.00 μM H3BO3, and 0.50 μM (NH4)6Mo7O24⋅2H2O at pH 5.5. After growing 1 week for tobacco or 2 weeks for rice in the normal nutrient solution, the seedlings were transferred to a nutrient solution without additional Mn or Zn as nutrient deficiency treatments, or nutrient solution containing 50-times higher Mn or Zn concentration for the nutrient excess treatments. After growing 10 days for tobacco or 15 days for rice, plants were collected.
After the same progress for generation and culture on MS medium, transgenic, and NT plants were transferred to pots filled with 2 kg calcareous soil for tobacco or 6 kg for rice. The soil was collected from uncultured riverside of the Yongding river (Beijing, China). Soil pH is 8.52 with DTPA-Fe: 2.46 mg/kg, DTPA-Mn: 0.34 mg/kg, DTPA-Zn: 0.53 mg/kg, and DTPA-Cu: 0.49 mg/kg. Before being used, the soil has been amended with basal fertilizers [composition (mg/kg soil): N 100 (Ca(NO3)2⋅4H2O), P 150 (KH2PO4), K 100 (KCl), and Mg 50 (MgSO4⋅7H2O)]. Rice was grown under flooding condition. Tobacco plants were collected after 60 days of growth in a greenhouse with natural lighting, and rice plants were harvested after maturity.
Analyses of Transgenic Tobacco and Rice Plants
The SPAD value of leaves was measured using a SPAD-502 chlorophyll meter (Konica-Minolta, Japan1). Yield composition had been recorded after rice harvested. The harvested plant samples were dried at 65°C for 3 days and weighed. Portions of ∼100 mg were digested with 4 ml of 4.4 M HNO3 and 2 ml of 6.5 M H2O2 for 20 min at 110°C, 20 min at 180°C, and 30 min at 220°C using a MarsXpress oven (CEM, Matthews, NC, United States2). Metal concentrations were measured by inductively coupled plasma atomic emission spectrometry (ICP-AES, Perkin Elmer Optima 3300DV).
Quantitative Real-Time PCR
Total RNA was extracted using TRIzol reagent (TaKaRa, Tokyo, Japan). Genomic DNA elimination and the first cDNA synthesis from RNA was achieved using a PrimeScriptTM RT reagent kit with gDNA Eraser (TaKaRa, Tokyo, Japan). qPCR was performed using a KAPA SYBR fast qPCR kit (Kapa Biosystems, Inc., Woburn, MA, United States) and a qTOWER 2.2 Real-time System (Analytik Jena, Jena, German) with primers listed in Supplementary Table S1. The AhNRAMP1 transcript quality was normalized against peanut AhUbiquitin. The AhNRAMP1 transcript level was normalized against NtActin for transgenic tobacco and OsActin for rice.
Statistical Analysis
Statistical analyses were conducted using R version 3.5.0. The statistical significance of differences between two groups was determined by Student’s t-Test (∗p ≤ 0.05, ∗∗p ≤ 0.01, and ∗∗∗p ≤ 0.001). Differences among multiple groups were assessed by One-way ANOVA followed by post hoc using Duncan’s test (p ≤ 0.05) when data met normal distribution and homogeneity of variance. Otherwise, non-parametric multiple comparisons by Kruskal–Wallis test (p ≤ 0.05) were used.
Results
AhNRAMP1 Was Induced by Mn or Zn Deficiency in Both Roots and Stems of Peanut
AhNRAMP1 expression levels were investigated in different peanut organs under complete culture solution (control), as well as Mn or Zn depleted conditions. With all treatments, AhNRAMP1 expression in stems was lower than that in roots but was higher than that in leaves (Figure 1). AhNRAMP1 transcript level in roots was significantly increased by three-fold after treatment without Mn for 10 days compared with control (Figure 1). Additionally, AhNRAMP1 expression was significantly upregulated (4.8-fold) in Zn-deficient roots (Figure 1). In stems, the mRNA level of AhNRAMP1 was 15.6 times higher under Mn depleted condition and was 8.9 times higher under Zn depleted condition compared with control (Figure 1). By contrast, no changes were induced by Mn or Zn deficiency in new and old leaves (Figure 1).
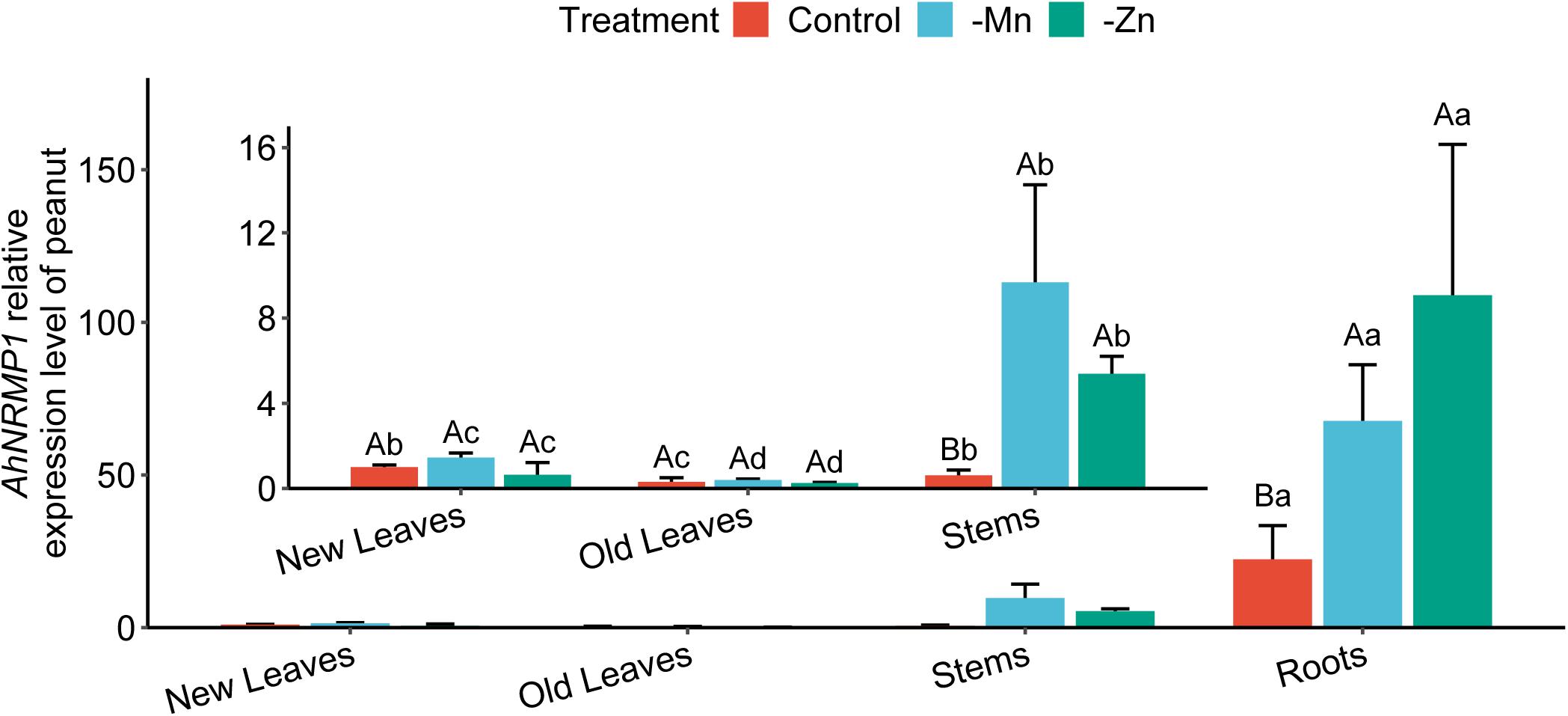
Figure 1. Expression patterns of AhNRAMP1 response to Mn or Zn deficiency in peanut. Peanut plants were grown in the hydroponic system with complete nutrient solution (control) or without Mn, or Zn for 10 days. The results are presented as the mean ±SD of triplicate replicates. Different lowercase letters represent significant differences among the organs of peanut under the same treatment by Kruskal–Wallis test at p ≤ 0.05 while uppercase letters represent significant differences among different treatments of the same organ by Duncan’s test at p ≤ 0.05.
AhNRAMP1 Functionally Complemented the Growth Defect of Mn or Zn Uptake-Defective Yeast Mutants
A yeast functional complementation experiment was conducted using the Δsmf1 (Mn uptake-defective) and Δzhy6 (Zn uptake-defective) yeast mutants to determine the Mn and Zn transport activity of AhNRAMP1. The growth of yeast mutants expressing AhNRAMP1 was significantly improved compared with that of yeast transformed with empty vector when grown on Mn or Zn limiting medium (Figure 2). The results inferred that AhNRAMP1 could complement Δsmf1 and Δzhy6 mutants and transport Mn and Zn.
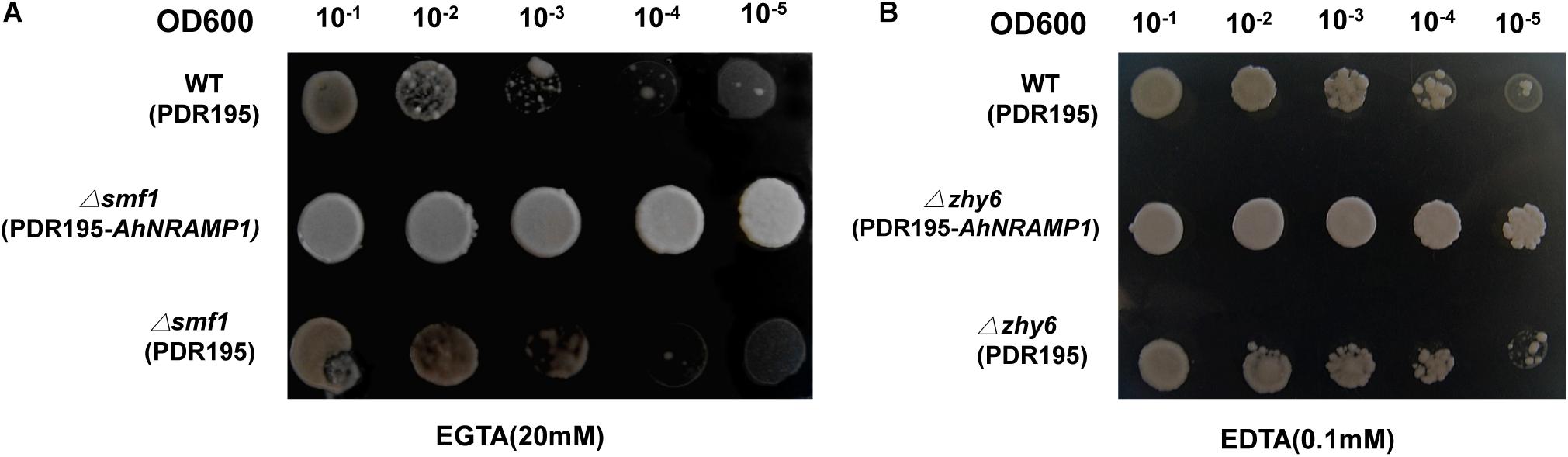
Figure 2. Functional complementation of the Δsmf1 yeast mutant with AhNRAMP1 under Mn-limiting medium (A) and that of the Δzhy6 yeast mutant with AhNRAMP1 under Zn-limiting medium (B). Yeast mutants defective in the uptake of Mn (Δsmf1) and Zn (Δzhy6) transformed with empty pDR195 vector were used as negative controls, and the WT yeast DEY1457 strains transformed with empty pDR195 vector was used as positive controls. Serial dilutions of transformed yeast cells with OD600nm of 0.1–0.00001 were plated on Synthetic Drop-out medium lacking Mn (A) or Zn (B) and grown for 2–3 days at 30°C.
Exogenous Expression of AhNRAMP1 Promoted Mn Uptake in Plants
To investigate the physiological role of AhNRAMP1 in plants, AhNRAMP1 was genetically transformed into tobacco and rice, the model dicot and monocot plant, respectively (Figures 7A,B). Mn concentration was significantly higher in all organs of E-90Ω lines after Mn depleted treatment (Figures 3C,F), as a result of higher expression of AhNRAMP1 in roots (Figures 7A,E). Biomass was also increased in E-90Ω lines (Figures 3A,B,D,E) along with the Mn content as expected (Supplementary Figure S1). Compared with NT rice under Mn depleted condition, more biomass, higher leaf Mn concentration (Figures 3D–F) and higher Mn contents (Supplementary Figure S1B) were also observed in OsIRT1 promoter lines. The primary expression of AhNRAMP1 in roots of OsIRT1 promoter lines may explain the improvement (Figure 7B).
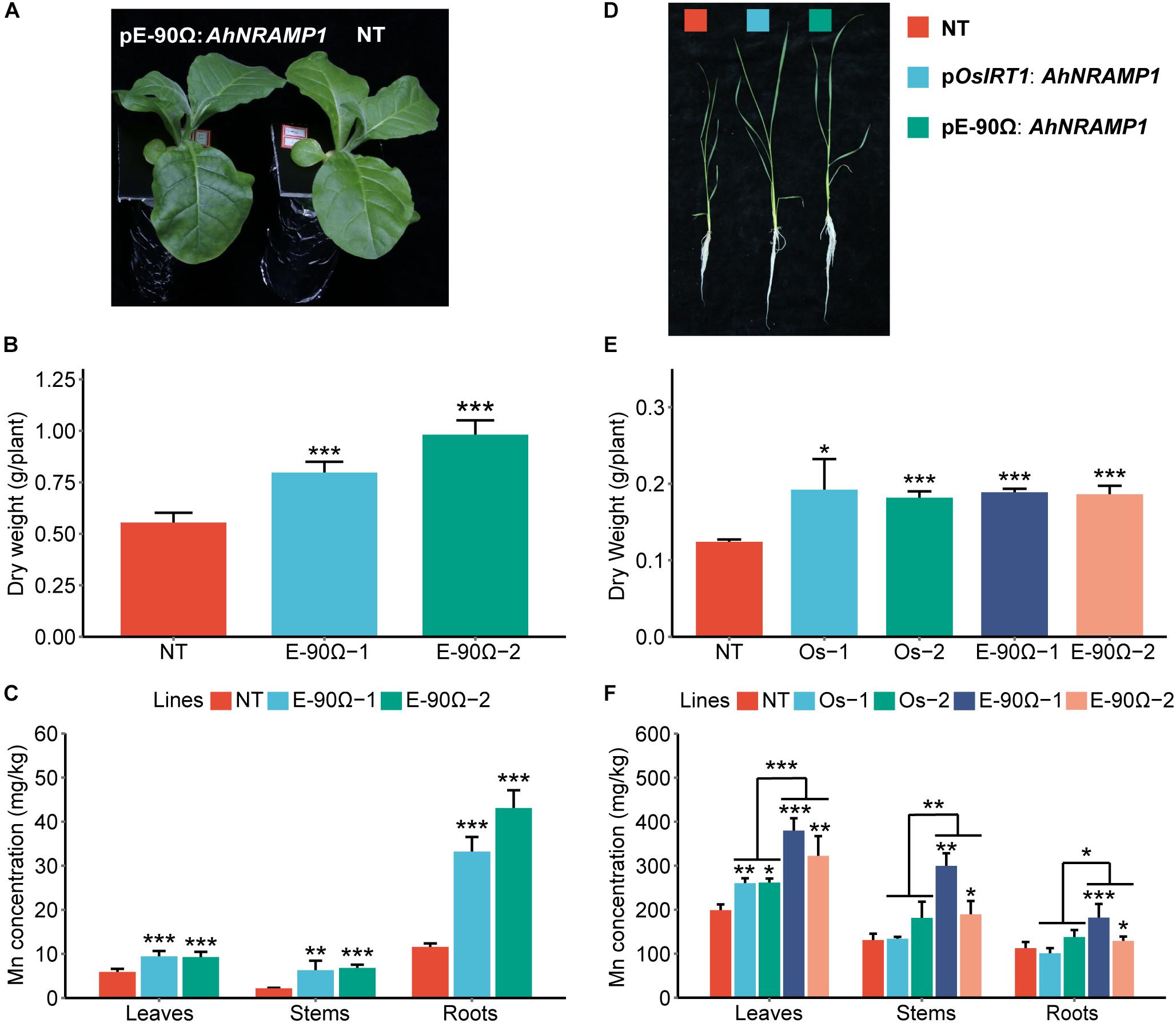
Figure 3. The response to Mn deficiency in AhNRAMP1 transgenic plants. The phenotype (A), biomass (B), and Mn concentration (C) of tobacco (left, A–C) under Mn depleted condition for 10 days, as well as those of rice under Mn depleted condition for 15 days (right, D–F). NT, non-transformed plants; E-90Ω, overexpressing AhNRAMP1 transgenic plants induced by the E-90Ω promoter; Os, AhNRAMP1 transgenic rice induced by the OsIRT1 promoter. The results are presented as the means ± SD of triplicate replicates. Asterisks indicate significant differences of transgenic plants with NT plants or between E-90Ω rice and OsIRT1 promoter rice by Student’s t-Test (∗p ≤ 0.05, ∗∗p ≤ 0.01, and ∗∗∗p ≤ 0.001).
Even under Mn excess condition, the concentration of Mn was still enhanced in all organs of E-90Ω tobacco, as well as in the leaves and roots of E-90Ω rice compared with NT plants (Figures 4C,F). Biomass production was subsequently reduced in E-90Ω lines (Figures 4A,B,D,E). OsIRT1 promoter lines also showed higher leaf Mn concentration and lowered dry weight (Figures 4D–F). Mn content was elevated in only one E-90Ω line compared with NT plants (Supplementary Figure S2). Moreover, AhNRAMP1 expression profiles in E-90Ω lines were similar between Mn depleted and Mn excess conditions (Figures 7A–D). By contrast, AhNRAMP1 expression level in roots of OsIRT1 promoter lines was lower than that in leaves under Mn excess condition (Figure 7D).
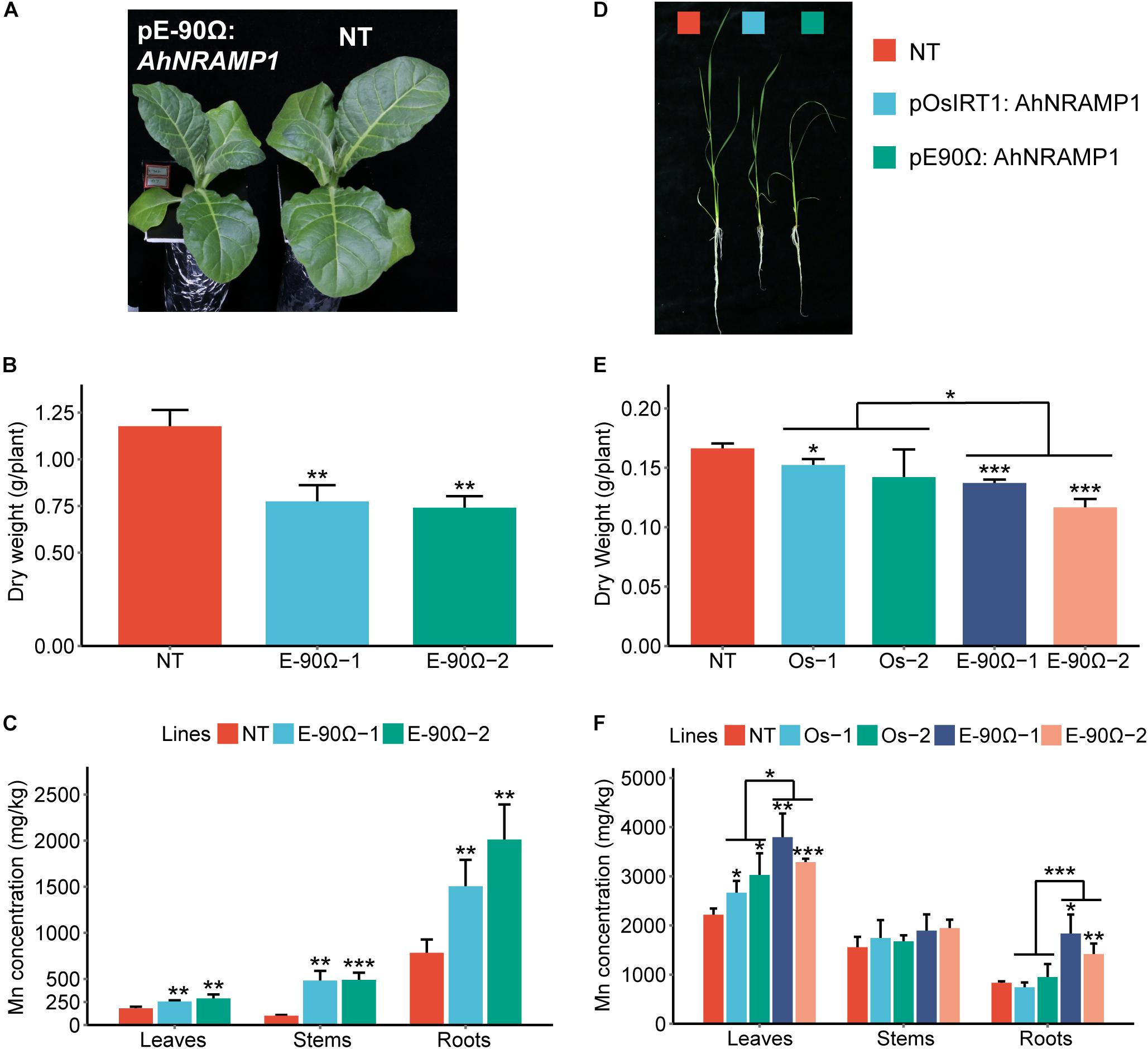
Figure 4. The response to excessive Mn in AhNRAMP1 transgenic plants. The phenotype (A), biomass (B), and Mn concentration (C) of tobacco (left, A–C) under Mn excess condition (25 μm) for 10 days, as well as those of rice (right, D–F) under Mn excess condition (45 μm) for 15 days. NT, non-transformed plants; E-90Ω, overexpressing AhNRAMP1 transgenic plants induced by the E-90Ω promoter; Os, AhNRAMP1 transgenic rice induced by the OsIRT1 promoter. The results are presented as the means ± SD of triplicate replicates. Asterisks indicate significant differences of transgenic plants with NT plants or between E-90Ω rice and OsIRT1 promoter rice by Student’s t-Test (∗p ≤ 0.05, ∗∗p ≤ 0.01, and ∗∗∗p ≤ 0.001).
Besides, AhNRAMP1 expression in all organs of E-90Ω rice was invariably higher than that of OsIRT1 promoter lines under Mn depleted condition (Figure 7B) and excess condition (Figure 7D). Accordingly, with higher Mn concentration, E-90Ω rice was more tolerant to Mn deficiency but more sensitive to excessive Mn compared with OsIRT1 promoter lines (Figures 3D–F, 4D–F). Even the more severely curled leaves were observed in E-90Ω rice under Mn excess condition (Figure 4D).
Exogenous Expression of AhNRAMP1 Enhanced Zn Uptake in Plants
Higher expression of AhNRAMP1 in roots (Figures 7E,F) increased Zn concentration of the leaves and roots in E-90Ω tobacco, as well as enhanced leaf and stem Zn concentration in E-90Ω rice under Zn depleted condition (Figures 5C,F). Leaf Zn concentration was also higher in OsIRT1 promoter lines than NT rice (Figure 5F). As a result, more biomass production (Figures 5A,B,D,E) and higher Zn content (Supplementary Figure S3) were also detected in all transgenic plants.
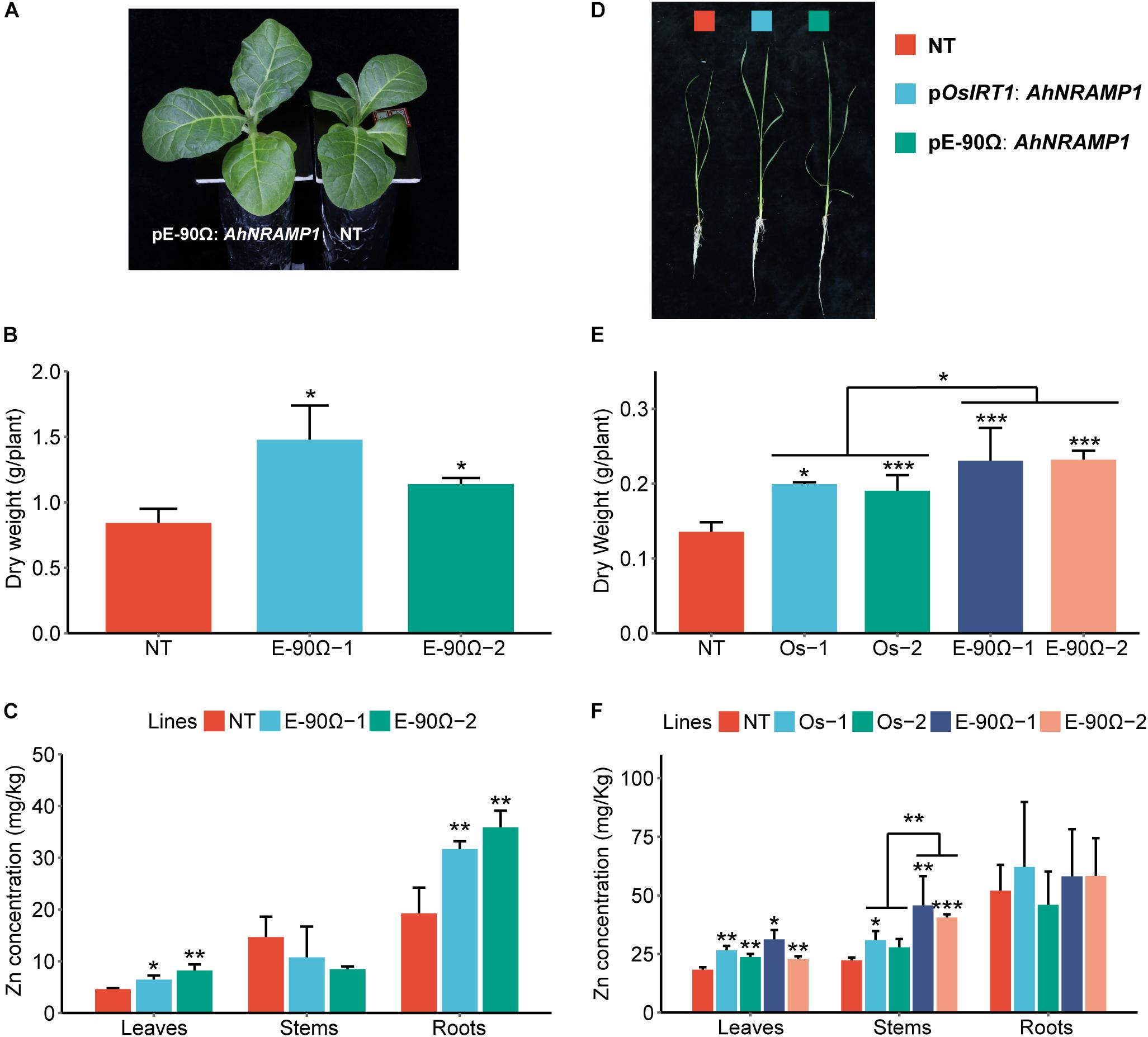
Figure 5. The response to Zn deficiency in AhNRAMP1 transgenic plants. The phenotype (A), biomass (B), and Zn concentration (C) of tobacco (left, A–C) under Zn depleted condition for 10 days, as well as those of rice under Zn depleted condition for 15 days (right, D–F). NT, non-transformed plants; E-90Ω, overexpressing AhNRAMP1 transgenic plants induced by the E-90Ω promoter; Os, AhNRAMP1 transgenic rice induced by the OsIRT1 promoter. The results are presented as the means ±SD of triplicate replicates. Asterisks indicate significant differences of transgenic plants with NT plants or between E-90Ω rice and OsIRT1 promoter rice by Student’s t-Test (∗p ≤ 0.05, ∗∗p ≤ 0.01, and ∗∗∗p ≤ 0.001).
Even after excessive Zn treatment, significantly higher Zn concentration of all organs of transgenic tobacco and increased leaf Zn concentration in transgenic rice was observed (Figures 6C,F). Biomass of all transgenic lines was decreased (Figures 6A,B,D,E). There was no statistical difference in Zn content of the whole plant among different AhNRAMP1 transgenic lines and NT plants (Supplementary Figure S4).
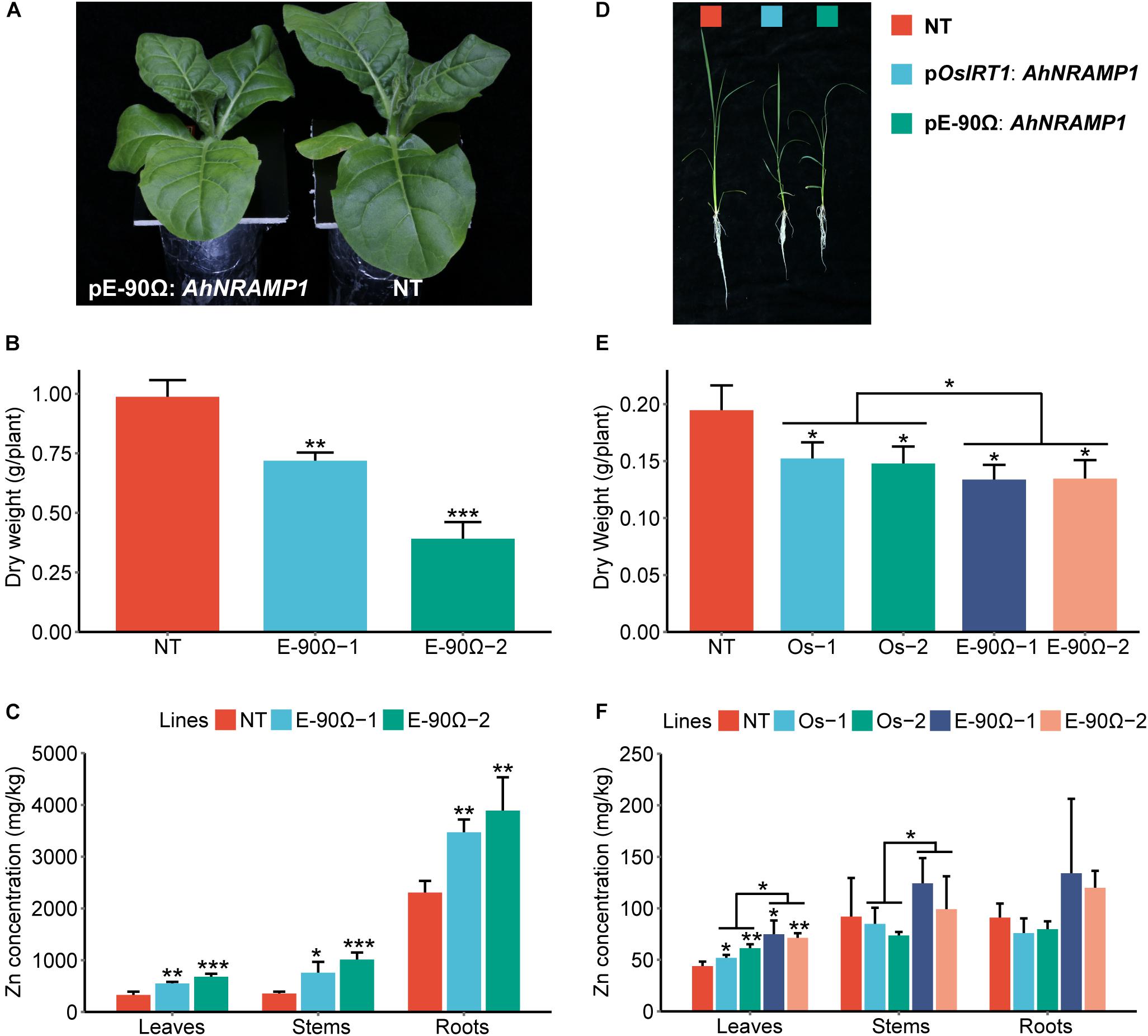
Figure 6. The response to excessive Zn in AhNRAMP1 transgenic plants. The phenotype (A), biomass (B), and Zn concentration (C) of tobacco (left, A–C) under Zn excess condition (25 μm) for 10 days, as well as those of rice (right, D–F) under Zn excess condition (20 μm) for 15 days. NT, non-transformed plants; E-90Ω, overexpressing AhNRAMP1 transgenic plants induced by the E-90Ω promoter; Os, AhNRAMP1 transgenic rice induced by the OsIRT1 promoter. The results are presented as the means ±SD of triplicate replicates. Asterisks indicate significant differences of transgenic plants with NT plants or between E-90Ω rice and OsIRT1 promoter rice by Student’s t-Test (∗p ≤ 0.05, ∗∗p ≤ 0.01, and ∗∗∗p ≤ 0.001).
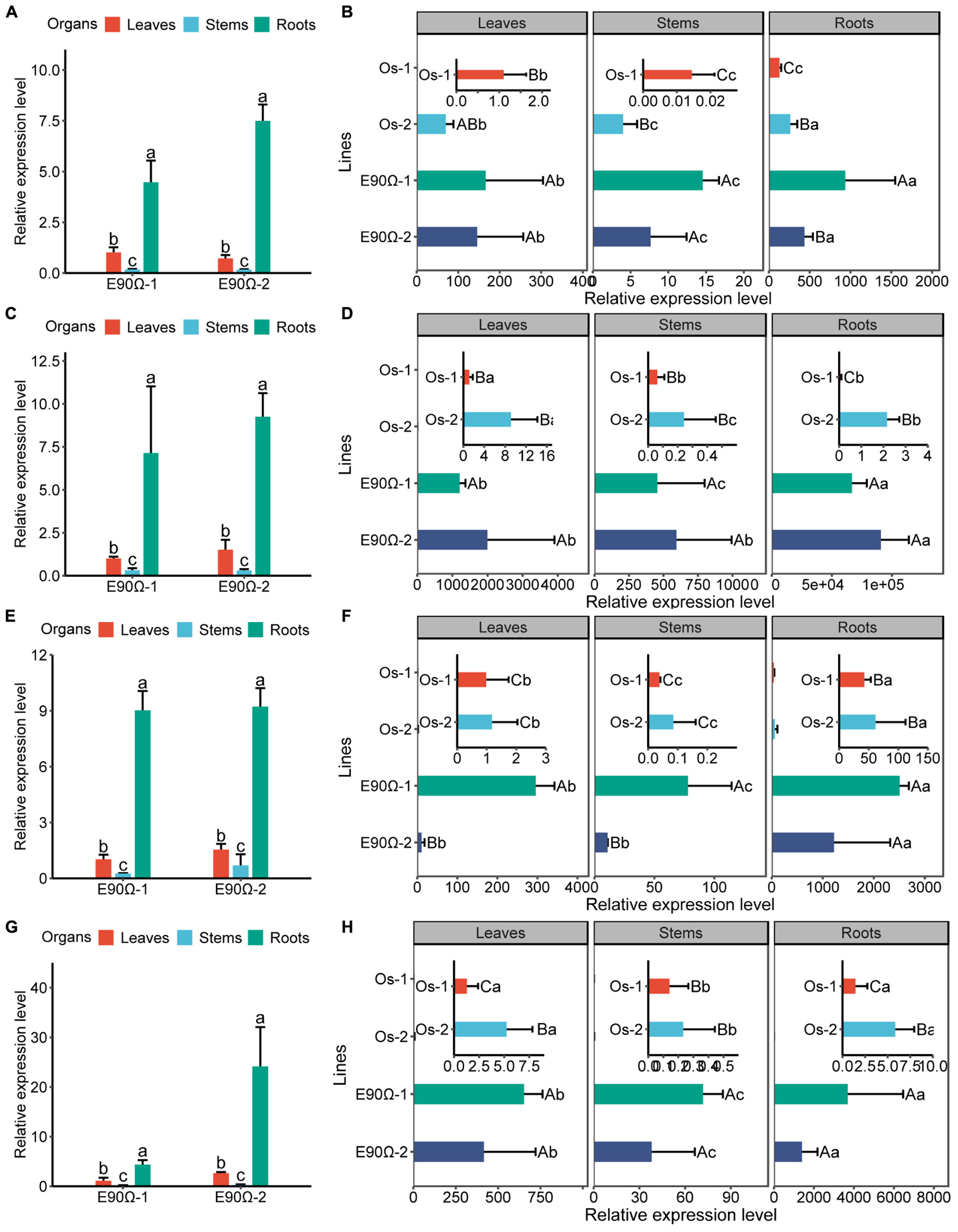
Figure 7. AhNRAMP1 expression profiles of AhNRAMP1 transgenic plants grown in the hydroponic system. AhNRAMP1 expression level in different organs of transgenic tobacco (A) or rice (B) under Mn depleted conditions, as well as those under Mn excess conditions (C,D). AhNRAMP1 expression level in different organs of transgenic tobacco (E) or (F) under Zn depleted conditions, as well as those under Zn excess conditions (G,H). NT, non-transformed plants; E-90Ω, overexpressing AhNRAMP1 transgenic plants induced by the E-90Ω promoter; Os, AhNRAMP1 transgenic rice induced by the OsIRT1 promoter. The results are presented as the means ± SD of triplicate replicates. Different lowercase letters represent significant differences among organs of the same transgenic line while uppercase letters indicate significant differences among different transgenic lines of the same organ at p ≤ 0.05 by Duncan’s test or by Kruskal–Wallis test.
Under Zn depleted condition (Figure 7E) or excess condition (Figure 7H), AhNRAMP1 expression level was also higher in E-90Ω rice lines than OsIRT1 promoter lines (Figures 7F,H). Hence, E-90Ω rice lines exhibited more tolerance to Zn deficiency but more sensitivity to excessive Zn (Figures 5D–F, 6D–F). E-90Ω rice displayed more severely curled leaves under Zn excess condition (Figure 6D).
Exogenous AhNRAMP1 Expression Improved Fe and Zn Nutrition in Plants Grown on Calcareous Soil
For transgenic tobacco grown on calcareous soil for 60 days, biomass production and SPAD value were enhanced by higher Fe concentration of leaves and stems, as well as higher Zn concentration of young leaves (Figures 8A–C,E). The enhancement might be a consequence of the overexpression of AhNRAMP1 in roots (Figure 8D). Stem Mn concentration was higher, but leaf Mn concentration was lower in transgenic tobacco than those in NT plants (Figure 8E). Overexpression AhNRAMP1 did not elevate Cu and Cd concentrations in transgenic tobacco (Figure 8E). The Fe and Zn contents in the aboveground of transgenic tobacco were higher than those of NT plants (Supplementary Figure S5).
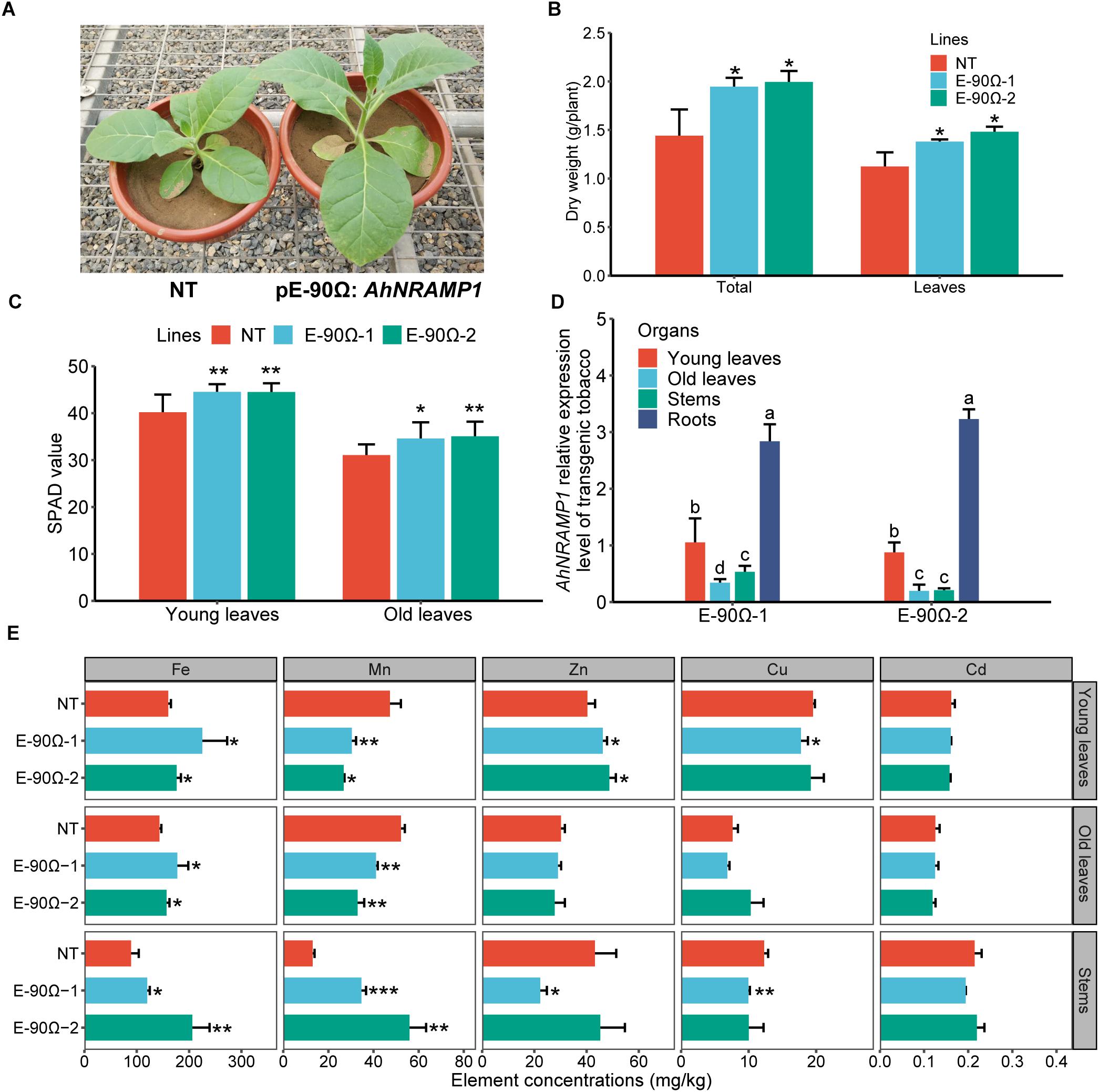
Figure 8. Exogenous AhNRAMP1 improved Fe and Zn deficiencies in transgenic tobacco grown on calcareous soil. The phenotype (A), biomass (B), SPAD value (C), AhNRAMP1 expression profiles (D), and element concentrations (E) of tobacco in the vegetative period. NT, non-transformed plants; E-90Ω, overexpressing AhNRAMP1 transgenic plants induced by the E-90Ω promoter. The results are presented as the means ± SD of twelve replicates for SPAD value, and triplicate replicates for other data. Different lowercase letters represent significant differences of AhNRAMP1 expression level among organs at p ≤ 0.05 by Kruskal–Wallis test. Asterisks indicate significant differences with NT plants by Student’s t-Test (∗p ≤ 0.05, ∗∗p ≤ 0.01, and ∗∗∗p ≤ 0.001).
SPAD value, especially in flag leaves, were higher during the tillering stage and biomass production increased after maturity in AhNRAMP1 transgenic rice compared with NT plants (Figures 9A–C). Exceedingly more productive ears and filled grains, as well as mildly lighter 1000-grain weight, were combined to result in the higher yield of transgenic rice (Figures 9D–H). Fe concentration was higher in the seeds and shoots of all transgenic rice than NT plants (Figure 9I). Zn concentration in the seeds and shoots was higher in overexpressing E-90Ω lines (Figure 9I). Mn, Cu, and Cd concentrations were not enhanced by exogenous expression of AhNRAMP1 (Figure 9I). Moreover, Zn concentration of seeds, Fe, Mn, and Zn concentrations of shoots, SPAD value of flag leaves, dry weight, productive eras, filled grains, and yield were higher in E-90Ω rice compared with OsIRT1 promoter lines (Figures 9A–F,I).
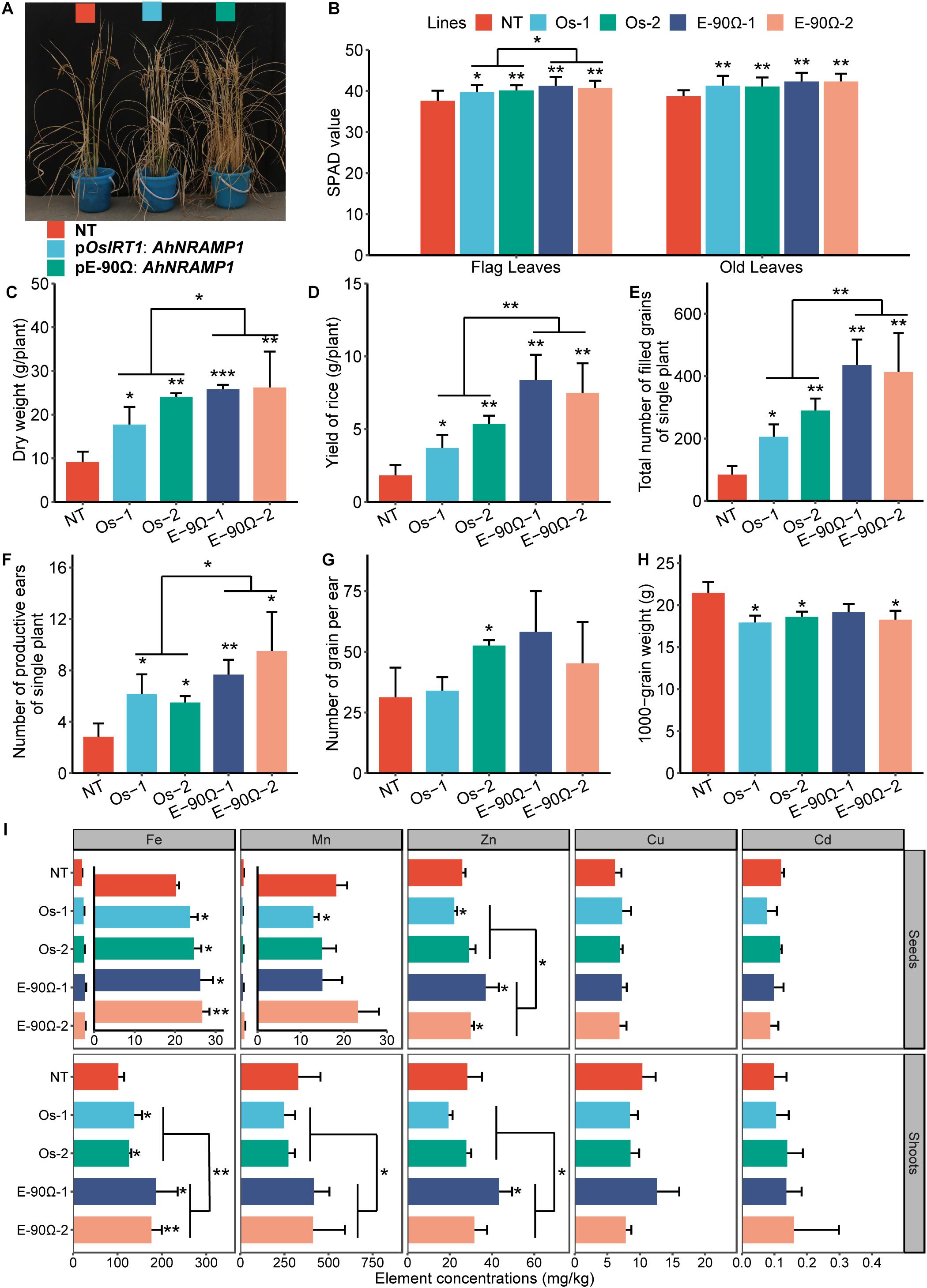
Figure 9. Exogenous AhNRAMP1 improved Fe and Zn deficiencies in transgenic rice grown on calcareous soil. The phenotype (A), biomass (C), yield composition (D–H) and element concentrations (I) of mature rice, as well as the SPAD value of the leaves during the tillering stage (B). NT, non-transformed plants; E-90Ω, overexpressing AhNRAMP1 transgenic plants induced by the E-90Ω promoter; Os, AhNRAMP1 transgenic rice induced by the OsIRT1 promoter. The results are presented as the means ±SD of twelve replicates for the SPAD value, and triplicate replicates for other data. Asterisks indicate significant differences with NT plants or between E-90Ω rice and OsIRT1 promoter rice by Student’s t-Test (∗p ≤ 0.05, ∗∗p ≤ 0.01, and ∗∗∗p ≤ 0.001).
Discussion
AhNRAMP1 Functioned as Mn and Zn Transporter in Plants
Mn and Zn are essential micronutrients for plant growth and development. Members of the NRAMP family are functional divalent metal ion transporters (Nevo and Nelson, 2006). Numerous NRAMP proteins have been identified as Mn transporters in plants (Cailliatte et al., 2010; Peris-Peris et al., 2017; Shao et al., 2017; Gao et al., 2018). Zn transport in plants is mainly conducted by members of seven transporter families excluding NRAMP (Gupta et al., 2016). The literature on how NRAMP proteins function to transport Zn in plants is very limited. Only AtNRAMP4 and TcNRAMP4 have been reported to transport Zn (Lanquar et al., 2004; Oomen et al., 2009). The function of AhNRAMP1 in Fe and Cd transport is well established (Xiong et al., 2012; Chen et al., 2017). Perhaps, AhNRAMP1 might be a functional transporter of other divalent metal ions such as Mn and Zn. AhNRAMP1 expression was markedly upregulated in roots and stems under Mn or Zn deficient conditions (Figure 1). AhNRAMP1 expression restored the growth of the yeast mutant Δsmf1 defective in Mn uptake and Δzhy6 detective in Zn uptake (Figure 2), uncovering the Mn and Zn transporting roles of AhNRAMP1.
Constitutive overexpression of NRAMP genes can cause a growth defect (Ishimaru et al., 2012a). Thus, an artificial promoter E-90Ω was used to drive high AhNRAMP1 expression in roots of both tobacco and rice (Xiong et al., 2012). OsIRT1 promoter was also used to generate transgenic rice because OsIRT1 expression was induced in rice roots by Mn- or Zn- deficiency (Lee and An, 2009). In the hydroponic system, Mn concentration of AhNRAMP1 transgenic plants was higher than NT plants under Mn depleted or excess conditions (Figures 3, 4). Exogenous AhNRAMP1 expression also resulted in higher Zn concentration under Zn depleted or excess conditions (Figures 5, 6). As a result, AhNRAMP1 transgenic plants were more resistant to Mn or Zn deficiency, but more sensitive to Mn or Zn excess conditions (Figures 3–6).
Furthermore, AhNRAMP1 expression level in E-90Ω rice was higher than that in OsIRT1 promoter lines under all hydroponic treatments (Figures 7). Thus, the higher expression level of AhNRAMP1 in transgenic plants, the higher Mn or Zn concentrations were detected (Figures 3–6). As a whole, our findings indicated that AhNRAMP1 was a functional transporter of Mn and Zn in plants.
AhNRAMP1 May Be a Candidate Gene for Fe and Zn Biofortification
AhNRAMP1 functioned as Fe (Xiong et al., 2012), Mn, Zn (Figures 2–6), and Cd (Chen et al., 2017) transporters in plants. Accordingly, it is necessary to dissect the role of AhNRAMP1 systematically considering its role in transporting all these metals. Further, Fe and Zn deficiencies frequently disrupted agricultural production (Gupta et al., 2016; Singh et al., 2017). Therefore, we carried out pot experiments using calcareous soil, which frequently causes Fe or Zn deficiency in plants by high pH and high bicarbonate (Marschner and Röhmeld, 1994; Gupta et al., 2016). Expression of AhNRAMP1 improved Fe and Zn nutrition (Figure 8E) in transgenic tobacco, resulting in strikingly enhanced chlorophyll contents, especially in the young leaves (Figure 8C). As poorly movable elements, Fe or Zn deficiency caused chlorosis in young leaves (Sinclair and Krämer, 2012; Xiong et al., 2012). Hence, AhNRAMP1 transgenic tobacco exhibited more tolerance to Fe and Zn deficiency when grown on calcareous soil (Figure 8).
Biomass production was higher in AhNRAMP1 transgenic rice (Figures 9A,C) than NT rice, which was the primary reason of higher yield (Figure 9D; Sakamoto et al., 2006). Besides, another crucial reason behind higher yield in transgenic rice was increased filled grains, which may be due to the enhancement of chlorophyll contents during the tillering stage, especially in flag leaves (Figures 9B–F). After all, flag leaves are the paramount source organs of carbohydrates production (Zhang et al., 2015). Moreover, flag leaves, as youngest leaves, readily suffer from Fe, and Zn deficiency when grown on calcareous soil (Marschner and Röhmeld, 1994; Gupta et al., 2016). From this it follows that the improvement of transgenic rice mentioned above might also be caused by elevated Fe and Zn uptake (Figure 9I and Supplementary Figure S6). Furthermore, breeding crop with an increased ability to accumulate Fe and Zn in their edible portions is beneficial to human health (White and Broadley, 2009). It is exciting that AhNRAMP1 expression enhanced seed Fe concentration in all transgenic rice and seed Zn concentration in E-90Ω rice as compared with NT plants. These results implied that AhNRAMP1 has the potential of Fe and Zn biofortification.
Excessive Mn or Zn is detrimental to plants, which is universal owing to environmental pollution or application of Mn- or Zn- containing fertilizers or pesticides (Millaleo et al., 2010; Tsonev and Lidon, 2012). Nonetheless, Zn is hardly excessive in plants grown on calcareous soil due to its low availability (Sadeghzadeh, 2013). Cd is one of the most toxic heavy metals to both plants and humans (Järup and Åkesson, 2009). AhNRAMP1 functioned as Mn (Figures 2–4) and Cd (Chen et al., 2017) transporters, it is essential to explore how its exogenous expression influence on Mn and Cd uptake in transgenic plants grown on calcareous soil. In our research, concentrations of Mn and Cd were enhanced neither in the leaves of transgenic tobacco nor in the seeds and shoots of transgenic rice by AhNRAMP1 expression when grown on calcareous soil (Figures 8E, 9I). However, even under the excessive Mn or Zn supply in hydroponics, AhNRAMP1 expression still promoted Mn or Zn concentration in transgenic plants (Figures 4, 6). The results indicated the risk of heavy metal accumulation when AhNRAMP1 transgenic plants were grown under the provision of excessive heavy metals. Thus, a series of field experiments should be performed in future to make better use of AhNRAMP1, including drawing on the advantage of alleviating Fe or Zn deficiency and avoiding the disadvantage associated with excessive Mn and Cd.
Conclusion
In conclusion, the present study provides new insights into our understanding of the AhNRAMP1 gene. AhNRAMP1 transported Mn and Zn, and its expression was strongly induced by Mn or Zn deficiency in roots and stems of peanut. Exogenous expression of AhNRAMP1 in tobacco and rice increased Mn or Zn concentrations. Consequently, transgenic plants exhibited improved tolerance to Mn or Zn deficiency but more sensitivity to Mn or Zn excess. Expression of AhNRAMP1 enhanced biomass in transgenic tobacco and rice and yield in transgenic rice grown on calcareous soil. Moreover, Fe and Zn concentrations were higher and Mn, Cu, and Cd concentrations were not enhanced in AhNRAMP1 transgenic plants compared with NT plants. AhNRAMP1 may also be a candidate gene for Fe and Zn biofortification.
Author Contributions
NW and YZ designed the experiments. NW and WQ performed most of the experiments. XG performed the yeast complementation tests. JD, QL, TW, SL, and TL participated in part of the plant analysis. NW wrote the manuscript. WQ, JD, and YZ revised the manuscript. All authors discussed the results and commented on the manuscript. YZ provided funding for this work as the corresponding author.
Funding
This study was supported by a grant from the National Natural Science Foundation of China (NSFC, Grant No. 31872183) and the National Key Research and Development Program of China (2017YFD0202102, 2016YFD0200405, and 2016YFE0101100).
Conflict of Interest Statement
The authors declare that the research was conducted in the absence of any commercial or financial relationships that could be construed as a potential conflict of interest.
Supplementary Material
The Supplementary Material for this article can be found online at: https://www.frontiersin.org/articles/10.3389/fpls.2019.00415/full#supplementary-material
Abbreviations
Cd, Cadmium; cDNA, complementary DNA; Cu, Copper; E-90Ω, AhNRAMP1 transgenic lines induced by the E-90Ω promoter; EDTA, Ethylenediaminetetraacetic acid; EGTA, Ethylene glycol-bis-β-aminoethylether-N, N, N′, N′-tetraacetic acid; Fe, Iron; IRT, iron-regulated transporter; Mn, Manganese; MS, Murashige and Skoog; NRAMP, natural resistance-associated macrophage protein; NT, non-transformed; Os, AhNRAMP1 transgenic lines induced by the OsIRT1 promoter; qPCR, quantitative real-time PCR; SD, standard deviation; WT, wild-type; Zn, Zinc.
Footnotes
References
Bouis, H. E., and Saltzman, A. (2017). Improving nutrition through biofortification: a review of evidence from HarvestPlus, 2003 through 2016. Glob. Food Sec. 12, 49–58. doi: 10.1016/j.gfs.2017.01.009
Cailliatte, R., Lapeyre, B., Briat, J. F., Mari, S., and Curie, C. (2009). The NRAMP6 metal transporter contributes to cadmium toxicity. Biochem. J. 422, 217–228. doi: 10.1042/BJ20090655
Cailliatte, R., Schikora, A., Briat, J. F., Mari, S., and Curie, C. (2010). High-affinity manganese uptake by the metal transporter NRAMP1 is essential for Arabidopsis growth in low manganese conditions. Plant Cell 22, 904–917. doi: 10.1105/tpc.109.073023
Chen, C., Xia, S., Deng, R., Liu, C., and Shi, G. (2017). AhIRT1 and AhNRAMP1 metal transporter expression correlates with Cd uptake in peanuts under iron deficiency. PLoS One 12:e0185144. doi: 10.1371/journal.pone.0185144
Curie, C., Alonso, J. M., Le Jean, M., Ecker, J. R., and Briat, J. F. (2000). Involvement of NRAMP1 from Arabidopsis thaliana in iron transport. Biochem. J. 347(Pt 3), 749–755. doi: 10.1042/bj3470749
Dai, J., Qiu, W., Wang, N., Nakanishi, H., and Zuo, Y. (2018). Comparative transcriptomic analysis of the roots of intercropped peanut and maize reveals novel insights into peanut iron nutrition. Plant Physiol. Biochem. 127, 516–524. doi: 10.1016/j.plaphy.2018.04.024
Gao, H., Xie, W., Yang, C., Xu, J., Li, J., Wang, H., et al. (2018). NRAMP2, a trans-Golgi network-localized manganese transporter, is required for Arabidopsis root growth under manganese deficiency. New Phytol. 217, 179–193. doi: 10.1111/nph.14783
Gupta, N., Ram, H., and Kumar, B. (2016). Mechanism of Zinc absorption in plants: uptake, transport, translocation and accumulation. Rev. Environ. Sci. Biotechnol. 15, 89–109. doi: 10.1007/s11157-016-9390-1
Hiei, Y., Ohta, S., Komari, T., and Kumashiro, T. (1994). Efficient transformation of rice (Oryza sativa L.) mediated by Agrobacterium and sequence analysis of the boundaries of the T-DNA. Plant J. 6, 271–282. doi: 10.1046/j.1365-313X.1994.6020271.x
Inal, A., Gunes, A., Zhang, F., and Cakmak, I. (2007). Peanut/maize intercropping induced changes in rhizosphere and nutrient concentrations in shoots. Plant Physiol. Biochem. 45, 350–356. doi: 10.1016/j.plaphy.2007.03.016
Ishimaru, Y., Bashir, K., Nakanishi, H., and Nishizawa, N. K. (2012a). OsNRAMP5, a major player for constitutive iron and manganese uptake in rice. Plant Signal. Behav. 7, 763–766. doi: 10.4161/psb.20510
Ishimaru, Y., Takahashi, R., Bashir, K., Shimo, H., Senoura, T., Sugimoto, K., et al. (2012b). Characterizing the role of rice NRAMP5 in manganese, iron and cadmium transport. Sci. Rep. 2:286. doi: 10.1038/srep00286
Ishimaru, Y., Suzuki, M., Tsukamoto, T., Suzuki, K., Nakazono, M., Kobayashi, T., et al. (2006). Rice plants take up iron as an Fe3+-phytosiderophore and as Fe2+. Plant J. 45, 335–346. doi: 10.1111/j.1365-313X.2005.02624.x
Järup, L., and Åkesson, A. (2009). Current status of cadmium as an environmental health problem. Toxicol. Appl. Pharmacol. 238, 201–208. doi: 10.1016/j.taap.2009.04.020
Kobayashi, T., Nakanishi, H., Takahashi, M., Kawasaki, S., Nishizawa, N. K., and Mori, S. (2001). In vivo evidence that Ids3 from Hordeum vulgare encodes a dioxygenase that converts 2’-deoxymugineic acid to mugineic acid in transgenic rice. Planta 212, 864–871. doi: 10.1007/s004250000453
Kobayashi, T., Nakayama, Y., Takahashi, M., Inoue, H., Nakanishi, H., Yoshihara, T., et al. (2004). Construction of artificial promoters highly responsive to iron deficiency. Soil Sci. Plant Nutr. 50, 1167–1175. doi: 10.1080/00380768.2004.10408590
Lanquar, V., Lelièvre, F., Barbier-Brygoo, H., and Thomine, S. (2004). Regulation and function of AtNRAMP4 metal transporter protein. Soil Sci. Plant Nutr. 50, 1141–1150. doi: 10.1080/00380768.2004.10408587
Lanquar, V., Lelièvre, F., Bolte, S., Hamès, C., Alcon, C., Neumann, D., et al. (2005). Mobilization of vacuolar iron by AtNRAMP3 and AtNRAMP4 is essential for seed germination on low iron. EMBO J. 24, 4041–4051. doi: 10.1038/sj.emboj.7600864
Lanquar, V., Ramos, M. S., Lelièvre, F., Barbier-Brygoo, H., Krieger-Liszkay, A., Krämer, U., et al. (2010). Export of vacuolar manganese by AtNRAMP3 and AtNRAMP4 is required for optimal photosynthesis and growth under manganese deficiency. Plant Physiol. 152, 1986–1999. doi: 10.1104/pp.109.150946
Lee, S., and An, G. (2009). Over-expression of OsIRT1 leads to increased iron and zinc accumulations in rice. Plant Cell Environ. 32, 408–416. doi: 10.1111/j.1365-3040.2009.01935.x
Marschner, H., and Röhmeld, V. (1994). Strategies of plants for acquisition of iron. Plant Soil 165, 261–274. doi: 10.1007/BF00008069
Menguer, P. K., Vincent, T., Miller, A. J., Brown, J. K. M., Vincze, E., Borg, S., et al. (2018). Improving zinc accumulation in cereal endosperm using HvMTP1, a transition metal transporter. Plant Biotechnol. J. 16, 63–71. doi: 10.1111/pbi.12749
Millaleo, R., Reyes-Díaz, M., Ivanov, A. G., Mora, M. L., and Alberdi, M. (2010). Manganese as essential and toxic element for plants: transport, accumulation and resistance mechanisms. J. Soil Sci. Plant Nutr. 10, 470–481. doi: 10.4067/S0718-95162010000200008
Nevo, Y., and Nelson, N. (2006). The NRAMP family of metal-ion transporters. Biochim. Biophys. Acta 1763, 609–620. doi: 10.1016/j.bbamcr.2006.05.007
Oomen, R. J., Wu, J., Lelièvre, F., Blanchet, S., Richaud, P., Barbier-Brygoo, H., et al. (2009). Functional characterization of NRAMP3 and NRAMP4 from the metal hyperaccumulator Thlaspi caerulescens. New Phytol. 181, 637–650. doi: 10.1111/j.1469-8137.2008.02694.x
Peris-Peris, C., Serra-Cardona, A., Sanchez-Sanuy, F., Campo, S., Ariño, J., and San Segundo, B. (2017). Two NRAMP6 isoforms function as iron and manganese transporters and contribute to disease resistance in rice. Mol. Plant Microbe Interact. 30, 385–398. doi: 10.1094/MPMI-01-17-0005-R
Sadeghzadeh, B. (2013). A review of zinc nutrition and plant breeding. J. Soil Sci. Plant Nutr. 13, 905–927. doi: 10.4067/S0718-95162013005000072
Sakamoto, T., Morinaka, Y., Ohnishi, T., Sunohara, H., Fujioka, S., Ueguchi-Tanaka, M., et al. (2006). Erect leaves caused by brassinosteroid deficiency increase biomass production and grain yield in rice. Nat. Biotechnol. 24, 105–109. doi: 10.1038/nbt1173
Sasaki, A., Yamaji, N., Yokosho, K., and Ma, J. F. (2012). Nramp5 is a major transporter responsible for manganese and cadmium uptake in rice. Plant Cell 24, 2155–2167. doi: 10.1105/tpc.112.096925
Schmidt, S. B., Jensen, P. E., and Husted, S. (2016). Manganese deficiency in plants: the impact on photosystem II. Trends Plant Sci. 21, 622–632. doi: 10.1016/j.tplants.2016.03.001
Shao, J. F., Yamaji, N., Shen, R. F., and Ma, J. F. (2017). The key to Mn homeostasis in plants: regulation of Mn transporters. Trends Plant Sci. 22, 215–224. doi: 10.1016/j.tplants.2016.12.005
Sinclair, S. A., and Krämer, U. (2012). The zinc homeostasis network of land plants. Biochim. Biophys. Acta 1823, 1553–1567. doi: 10.1016/j.bbamcr.2012.05.016
Singh, S. P., Keller, B., Gruissem, W., and Bhullar, N. K. (2017). Rice nicotianamine synthase 2 expression improves dietary iron and zinc levels in wheat. Theor. Appl. Genet. 130, 283–292. doi: 10.1007/s00122-016-2808-x
Takahashi, R., Ishimaru, Y., Senoura, T., Shimo, H., Ishikawa, S., Arao, T., et al. (2011). The OsNRAMP1 iron transporter is involved in Cd accumulation in rice. J. Exp. Bot. 62, 4843–4850. doi: 10.1093/jxb/err136
Tan, S., Han, R., Li, P., Yang, G., Li, S., Zhang, P., et al. (2015). Over-expression of the MxIRT1 gene increases iron and zinc content in rice seeds. Transgen. Res. 24, 109–122. doi: 10.1007/s11248-014-9822-z
Thomine, S., Wang, R., Ward, J. M., Crawford, N. M., and Schroeder, J. I. (2000). Cadmium and iron transport by members of a plant metal transporter family in Arabidopsis with homology to Nramp genes. Proc. Natl. Acad. Sci. U.S.A. 97, 4991–4996. doi: 10.1073/pnas.97.9.4991
Tsonev, T., and Lidon, F. J. C. (2012). Zinc in plants-an overview. Emir. J. Food Agric. 24, 322–333.
White, P. J., and Broadley, M. R. (2009). Biofortification of crops with seven mineral elements often lacking in human diets-iron, zinc, copper, calcium, magnesium, selenium and iodine. New Phytol. 182, 49–84. doi: 10.1111/j.1469-8137.2008.02738.x
Xiong, H., Kobayashi, T., Kakei, Y., Senoura, T., Nakazono, M., Takahashi, H., et al. (2012). AhNRAMP1 iron transporter is involved in iron acquisition in peanut. J. Exp. Bot. 63, 4437–4446. doi: 10.1093/jxb/ers117
Zhang, B., Ye, W., Ren, D., Tian, P., Peng, Y., Gao, Y., et al. (2015). Genetic analysis of flag leaf size and candidate genes determination of a major QTL for flag leaf width in rice. Rice 8:39. doi: 10.1186/s12284-014-0039-9
Keywords: AhNRAMP1, manganese, zinc, iron, cadmium, transporter, peanut, biofortification
Citation: Wang N, Qiu W, Dai J, Guo X, Lu Q, Wang T, Li S, Liu T and Zuo Y (2019) AhNRAMP1 Enhances Manganese and Zinc Uptake in Plants. Front. Plant Sci. 10:415. doi: 10.3389/fpls.2019.00415
Received: 27 November 2018; Accepted: 19 March 2019;
Published: 07 May 2019.
Edited by:
Hatem Rouached, Institut National de la Recherche Agronomique (INRA), FranceReviewed by:
Levent Ozturk, Sabancı University, TurkeySantosh B. Satbhai, Indian Institute of Science Education and Research Mohali, India
Copyright © 2019 Wang, Qiu, Dai, Guo, Lu, Wang, Li, Liu and Zuo. This is an open-access article distributed under the terms of the Creative Commons Attribution License (CC BY). The use, distribution or reproduction in other forums is permitted, provided the original author(s) and the copyright owner(s) are credited and that the original publication in this journal is cited, in accordance with accepted academic practice. No use, distribution or reproduction is permitted which does not comply with these terms.
*Correspondence: Yuanmei Zuo, enVveW1AY2F1LmVkdS5jbg==
†These authors have contributed equally to this work