- State Key Laboratory of Hybrid Rice, College of Life Sciences, Wuhan University, Wuhan, China
In eukaryotes, mitochondrion is an essential organelle which is surrounded by a double membrane system, including the outer membrane, intermembrane space and the inner membrane. The translocase of the outer mitochondrial membrane (TOM) complex has attracted enormous interest for its role in importing the preprotein from the cytoplasm into the mitochondrion. However, little is understood about the potential biological function of the TOM complex in Arabidopsis. The aim of the present study was to investigate how AtTOM40, a gene encoding the core subunit of the TOM complex, works in Arabidopsis. As a result, we found that lack of AtTOM40 disturbed embryo development and its pattern formation after the globular embryo stage, and finally caused albino ovules and seed abortion at the ratio of a quarter in the homozygous tom40 plants. Further investigation demonstrated that AtTOM40 is wildly expressed in different tissues, especially in cotyledons primordium during Arabidopsis embryogenesis. Moreover, we confirmed that the encoded protein AtTOM40 is localized in mitochondrion, and the observation of the ultrastructure revealed that mitochondrion biogenesis was impaired in tom40-1 embryo cells. Quantitative real-time PCR was utilized to determine the expression of genes encoding outer mitochondrial membrane proteins in the homozygous tom40-1 mutant embryos, including the genes known to be involved in import, assembly and transport of mitochondrial proteins, and the results demonstrated that most of the gene expressions were abnormal. Similarly, the expression of genes relevant to embryo development and pattern formation, such as SAM (shoot apical meristem), cotyledon, vascular primordium and hypophysis, was also affected in homozygous tom40-1 mutant embryos. Taken together, we draw the conclusion that the AtTOM40 gene is essential for the normal structure of the mitochondrion, and participates in early embryo development and pattern formation through maintaining the biogenesis of mitochondria. The findings of this study may provide new insight into the biological function of the TOM40 subunit in higher plants.
Introduction
In the past decades, it has been widely studied that the process of embryogenesis in Arabidopsis is highly programmed and controlled by an intricate network of gene expression (ten Hove et al., 2015). The single-celled zygote (fertilized egg cell) initiates cell division and differentiation to produce a mature embryo, while the triploid primary endosperm nucleus (fertilized central polar nucleus) develops into a syncytial endosperm and subsequently undergoes cellularization to form endosperm (Hamamura et al., 2012; Sprunck and Dresselhaus, 2015). The endosperm nourishes the embryo and determines the size of ovule (Lafon-Placette and Köhler, 2014). The onset of early embryogenesis forms the precursors for all stem cells and major tissues (Capron et al., 2009; Lau et al., 2012; Bleckmann et al., 2014). Previous research focused on the molecular mechanisms in the formation of the tissue primordium, and demonstrated that a large number of genes were required for the process of embryo development and morphogenesis in Arabidopsis (Tzafrir et al., 2004; Meinke et al., 2008; Palovaara et al., 2017), which helped to explore the relationship between some critical biological events and embryo development.
The mitochondrion is an essential organelle derived from an endosymbiotic event two billion years ago, and is the main source of ATP to sustain cellular viability and activity (Gray et al., 1999; Cavalier-Smith, 2009; Alcock et al., 2010). In eukaryotes, a small portion of mitochondrial proteins are encoded by the mitochondrial genes, while most proteins are encoded by the nuclear genome. The proteins are synthesized in the cytoplasm and then imported into the mitochondria (Adams and Palmer, 2003; Crimi and Rigolio, 2008; Lithgow and Schneider, 2010). The protein transport process is mediated by the mitochondrial double-membrane system, including the outer membrane (OM), intermembrane space (IMS) and the inner membrane (IM). The translocase of the outer mitochondrial membrane (TOM) complex functions as the entry gate on the OM, it can recognize the preproteins synthesized in the cytosol and then distribute them to the downstream protein sorting machineries (Ahting et al., 1999; Dudek et al., 2013; Bohnert et al., 2015; Fukasawa et al., 2017). It has been reported that the β-barrel protein TOM40 is the core subunit of TOM complex. Previous research about TOM40 focused on the biochemical characterization and its structure (Hill et al., 1998; Ahting et al., 2001; Gessmann et al., 2011; Bausewein et al., 2017). In yeast, TOM40 is a ∼40 kD pore protein that comprises a membrane-inserted, 19-stranded β-barrel domain with three α-helical domains at the N terminus and C terminus (Künkele et al., 1998; Hill et al., 1998; Lackey et al., 2014; Kuszak et al., 2015). These structures form the function area of TOM40 as the major entry gate for the mitochondrial preproteins. To characterize the properties of individual Tom40, single molecule tracking technique was utilized to trace individual Tom40 molecules in yeast, and the results demonstrated that Tom40 movement in the outer mitochondrial membrane is highly dynamic but confined in nature, suggesting anchoring of the TOM complex as a whole (Kuzmenko et al., 2011).
It is known that mitochondria play essential roles in the process of plant growth, development, and morphogenesis (Millar et al., 2008; Martin et al., 2014; Colas des Francs-Small and Small, 2014; Selles et al., 2018). Lately bioinformatic and genetic approaches were utilized in Arabidopsis to detect the genes involved in the process of germination, among the 239 genes queried 29 showed embryo arrested or lethal phenotypes (Law et al., 2012). The mitochondrial protein import component, translocase of the inner membrane17-1, was reported to play a role in defining the timing of germination in Arabidopsis. In mutants lacking AtTIM17-1, it showed a significant increase in the rate of germination, and altered mitochondrial biogenesis fed back to alter the germination rate (Wang et al., 2014). In Arabidopsis, a number of genes were identified to be mitochondrion-targeted and the mutation of these genes led to the aborted seeds at an early stage (Deng et al., 2014; Ju et al., 2016; Fromm et al., 2016). The CA domain is required for the complex I biogenesis in the mitochondrial respiratory chain. In Arabidopsis, mutants are affected in two specific CA subunits, and embryos show impaired mitochondrial membrane potential and mitochondrial reactive oxygen species (ROS) accumulation, and then trigger the lethal phenotype (Córdoba et al., 2016). Similar results were reported in maize (Gutiérrez-Marcos et al., 2007; Sosso et al., 2012; Ren et al., 2017), and rice (Xiao et al., 2018). It was considered that these mutations resulted in defective assembly of respiratory complexes in mitochondria, and then led to disorder in the mitochondria structure such as corruption in mitochondrial membrane, and subsequently caused reduced growth and fertility, or even embryo-lethality.
In plant, the TOM complex is generally composed of six subunits, they are TOM5, TOM6, TOM7, TOM9, TOM22, and TOM40. Previous research work demonstrated that TOM 5, 6, and 7 are small TOMs, they regulate the formation and stability of the TOM complex (Dietmeier et al., 1997; Kato and Mihara, 2008; Thornton et al., 2010; Becker et al., 2011). TOM9 presents as the counterpart of TOM22 in yeast, it is significantly smaller due to a truncation in the cytosolic receptor domain (Jänsch et al., 1998; Macasev et al., 2000; Parvin et al., 2017). TOM20 functions in receptor recognition (Werhahn et al., 2001; Lister et al., 2007), and TOM40 acts as the central porin of the TOM complex (Duncan et al., 2013a). A plant TOM complex was first purified from potato tuber mitochondria, and the sequence similarity between the 36-kDa protein and fungal TOM40 was identified (Jänsch et al., 1998). In Arabidopsis, through using biochemical and cell biology approaches, TOM40 was confirmed to be in the mitochondrial outer membrane and was a transmembrane β-barrel protein (Duncan et al., 2011). However, little was known about how TOM40 works in Arabidopsis. To explore the biological function of AtTOM40 (At3g20000), a gene encoding the core subunit in the TOM complex of Arabidopsis, we obtained two independent T-DNA insertion lines (SALK_128170 and CS873594). With the aid of bioinformatic, genetic, molecular, cellular approaches, we characterize the comprehensive function of AtTOM40. Here we report that AtTOM40 encodes a typical β-barrel protein and is conserved among different species, it is targeted to mitochondria and is widely expressed in different tissues. Knock-out of AtTOM40 caused early embryo-lethal phenotype in Arabidopsis, which may be due to the failure of the mitochondrial biogenesis during embryo development. Further investigation revealed the expression of several important genes encoding outer mitochondrial membrane proteins and embryo pattern formation in Arabidopsis was disordered, suggesting that the AtTOM40 may participate in early embryo development through maintaining the normal mitochondrial biogenesis.
Materials and Methods
Plant Materials and Growth Conditions
Arabidopsis thaliana ecotype Columbia was used as materials. The two T-DNA insertion mutants SALK_128170 (tom40-1/+) and CS873594 (tom40-2/+) were obtained from the Arabidopsis Information Resource1. The Arabidopsis plants were cultivated in a greenhouse at Wuhan University at 22 ± 2°C with a 16-h light and 8-h dark photoperiod.
Bioinformatic Analyses of TOM40 in Different Species
The AtTOM40 protein sequence was used to search for its homologs in other species by using BLAST2. Multiple sequence alignment was performed by ClustalX (version 1.83), and MEGA4 program was used to construct the phylogenetic tree based on the Neighbor-Joining algorithm (Tamura et al., 2007). A three dimensional structure of TOM40 in Arabidopsis and Oryza sativa were constructed according to the crystal structures of their yeast homologs through SWISS-MODEL3. Conserved function domains of TOM40 in different species were predicted on https://www.ebi.ac.uk/interpro/sequencesearch and then were demonstrated on http://ibs.biocuckoo.org/ website.
Mutant Verification and Complementation Analysis
The tom40-1/+ and tom40-2/+ mutant lines were verified by using PCR with specific primers, respectively, and the insertion positions were confirmed by sequencing with the T-DNA primer. To complement the defects of tom40-1/+ and tom40-2/+, the full-length genomic fragment of AtTOM40 was amplified with KOD-Plus DNA polymerase (Toyobo) and cloned into pCambia1300 vector (Cambia), and then transferred into the two mutants by using the floral dip method (Clough and Bent, 1998). Primers used in this work are listed in the Supplementary Table S1.
Clearing Technique of Ovules and Observation of Endosperm Cellularization
Ovules from Arabidopsis were dissected from siliques with self-made needles and were cleared in Hoyer’s solution [chloral hydrate: glycerol: water, 8:1:2 (w/v/v)] for 30 min to 8 h depending on the different embryo developmental stages (Chen et al., 2015). To observe endosperm cellularization, ovules at different developing stages were conducted with the method described before (Li et al., 2017). All the specimens were observed under the Confocal Laser Scanning Microscope (CLSM, Leica SP8).
RNA in situ Hybridization
Wild type ovule collecting, fixating, embedding, and sectioning, along with procedures of RNA in situ hybridization were performed as described previously (Brewer et al., 2006; Deng et al., 2014). The antisense and sense probes used in this study were all generated via PCR with T7 promoter adding primers (listed in Supplementary Table S1), and followed by in vitro transcription with the DIG RNA Labeling Kit (SP6/T7; Roche4). The ovule samples were observed under an Olympus BX60 microscope and photographed with the Olympus DP72 CCD.
GUS Staining Analysis
The promoter fragment of AtTOM40 was amplified by PCR, and its genome-specific primers are listed in Supplementary Table S1. After verification through sequencing, the amplified DNA fragment was cloned into pCAMBIA1381Xb (Cambia, Australia) vector, and then transformed into Arabidopsis wild type plants via the floral dip method described above. The homozygous proAtTOM40::GUS transgenic lines were screened and then used for GUS staining as described before (Chen et al., 2018). The stained samples were observed under an Olympus SZX12 microscope and photographed with a CCD (Cool SNAP, RS Photometric).
Quantitative Real-Time PCR
Total RNAs from various tissues in Arabidopsis were extracted by using RNA isoPlus reagent (TaKaRa, Japan), and transcribed into cDNA with ReverTra Ace qPCR RT Kit (TOYOBO). The cDNAs were amplified as templates with the gene-specific primers, and quantitative Real-Time PCRs were carried out with a Rotor-Gene Q real-time PCR machine (Qiagen). The transcript abundance relative to WT of different tissues was analyzed by the comparative CT method as described previously (Schmittgen and Livak, 2008; Ma and Zhao, 2010), and the GLYCERALDEHYDE-3-PHOSPHATE DEHYDROGENASE (GAPDH) was utilized as an internal gene for normalizing (Zhong and Simons, 1999). Three independent biological replicates and three technical replicates of every sample were conducted for quantitative real-time PCR analysis. Primers used in the experiments are listed in Supplementary Table S1.
Subcellular Localizations
The CDS (coding sequence) fragment of AtTOM40 was amplified by PCR (the genome-specific primers are listed in Supplementary Table S1). After verification by sequencing, the amplified DNA fragment was cloned into pCAMBIA1300-eGFP vector, and then transformed into Arabidopsis wild type plants via the floral dip method described above. The homozygous proAtTOM40::CDS-GFP transformants were used for the subcellular localizations. Preparation and observation of the mesophyll protoplasts were conducted with the method described before (Deng et al., 2014). Mesophyll protoplasts isolated from the 4-week-old transformants were incubated with 2 mM MitoTracker Red FM (Molecular Probes) for 10 min and examined with a Confocal Laser Scanning Microscope (CLSM, Leica SP8). The images were taken under the eGFP fluorescence (excitation, 488 nm; emission, 505–530 nm) and the MitoTracker channel (excitation, 559 nm; emission, 530–560 nm).
Transmission Electron Microscopy
The ovules at 6DAP (day after pollination) in wild type siliques and the albino ovules 6DAP in tom40-1/+ siliques were collected for fixating, embedding and sectioning as described before (Ke et al., 2017). The ultrathin sections were cut with a Leica UC7 ultramicrotome, and observed under a transmission electron microscope (JEOL, JEM-1400 plus) and photographed with a JOEL digital camera EM (14800RUBY).
Results
AtTOM40 Is a Mitochondrial Translocase of the Outer Membrane and Is Highly Conserved Among Different Species
To determine the identity of TOM40 among different species, we performed a full alignment of the amino acid sequences in AtTOM40 and its homologs from other species. The result showed that all the TOM40 proteins comprise 19 transmembrane β-barrel domains with two α-helical segments (Figure 1A), which are the conserved domains reported before (Kuszak et al., 2015). An extra α-helical segment was found only in Saccharomyces and Candida glabrata. The three-dimensional structure of TOM40 was constructed in Arabidopsis thaliana, Saccharomyces cerevisiae and Oryza sativa, the predicted 3D structure demonstrated the similar 19-stranded β-barrel, which forms the characteristic protein-conducting channel (Figures 1B–E). To further illustrate the evolutionary relationship among monocots, dicots, mammals, yeast and fungi, we analyzed the phylogenetic tree of TOM40s, and found a high degree of homology between AtTOM40 and other TOM40s (Supplementary Figures S1A,B). The conservation of TOM40s in sequence and structure indicates the protein as a common element in the mitochondrial outer membrane.
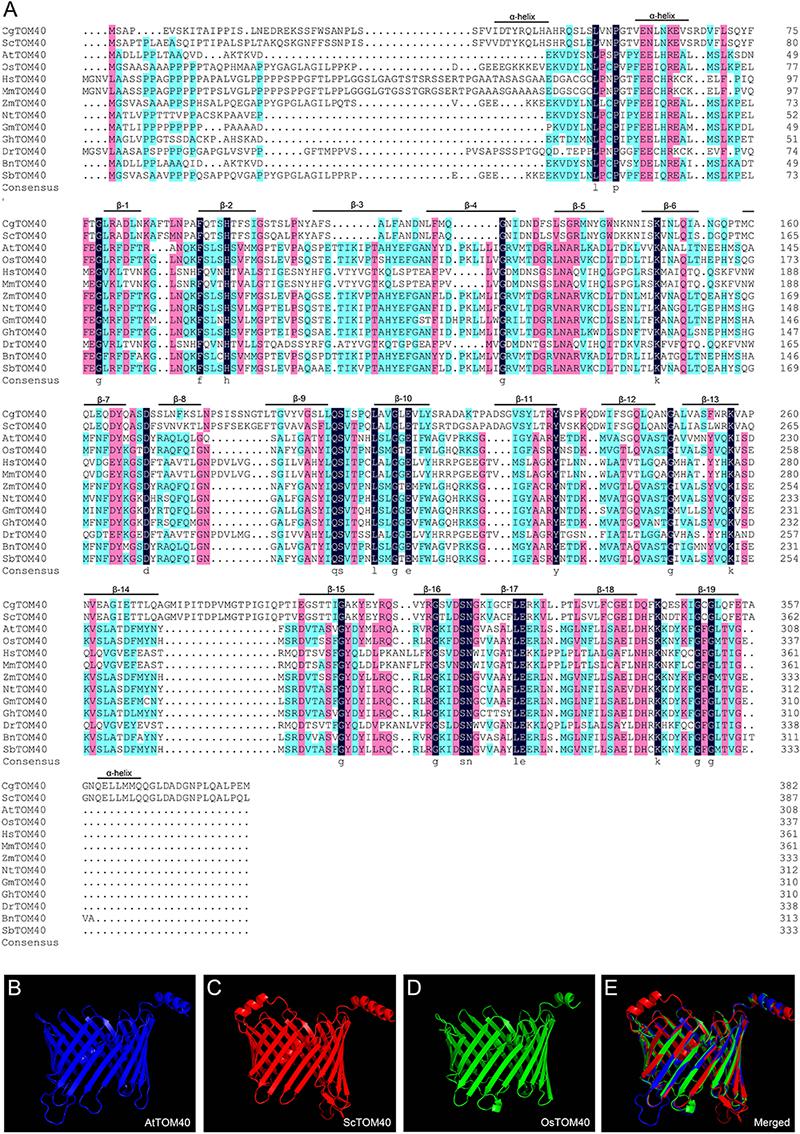
Figure 1. The conservation analysis of TOM40 in different species. (A) Sequence alignment of TOM40 in different species, α-helical segments and β-barrel domain were labeled according to C. glabrata. Cg, Candida glabrata; Sc, Saccharomyces cerevisiae; At, Arabidopsis thaliana; Os, Oryza sativa; Hs, Homo sapiens; Mm, Mus musculus; Zm, Zea mays; Nt, Nicotiana tabacum; Gm, Glycine max; Gh, Gossypium hirsutum; Dr, Danio rerio; Bn, Brassica napus; Sb, Sorghum bicolor. (B–D) Predicted 3-D structures of AtTOM40, ScTOM40 and OsTOM40 according to the crystal structures of their homologs in yeast. (E) The merged view of AtTOM40, ScTOM40 and OsTOM40 predicted 3-D structures.
Knock-Out of AtTOM40 Causes Seed Abortion
To investigate the function of TOM40 in Arabidopsis, we obtained two independent T-DNA insertion mutant lines SALK_128170 (tom40-1) and CS873594 (tom40-2) from the Arabidopsis Information Resource5. The positions of the T-DNA insertions in the tom40-1 and tom40-2 mutants were verified by genomic PCR and sequencing, and were found to be located in the seventh exon and the second exon, respectively (Figure 2A). Due to the loss of kanamycin resistance of the tom40-1 mutant, we utilized PCR to analyze the genetic segregation of its self-fertilized progenies. The results showed that the selective ratio was about 2:1 (resistant: sensitive), instead of the expected 3:1, which suggested that the T-DNA insertion caused embryo lethality in recessive homozygous mutant plants. Moreover, the reciprocal crosses of mutant tom40-1 with wild type plants showed that the segregation ratio was about 1:1 (resistant: sensitive), similar with expected ratio (Table 1), implying the gametophyte fertility of both females and males were normal. Similar results were obtained in the tom40-2 mutant plant, and the segregation analysis of the self-fertilized mutant progenies was conducted with resistance screening (BASTA) instead. Furthermore, we dissected mature seeds from the wild type, tom40-1/+ and tom40-2/+ siliques, and found that the seeds in the wild-type can perform normal development, while both mutant plants possessed a proportion of aborted white seeds (Figure 2B). The seed abortion rates of two mutants were counted to be 24.92 and 25.04% respectively, which are close to the expected 25% (Figure 2C). The results demonstrated that the mutation of AtTOM40 did not affect the gametophyte fertility, but could cause embryo lethality in homozygous progenies.
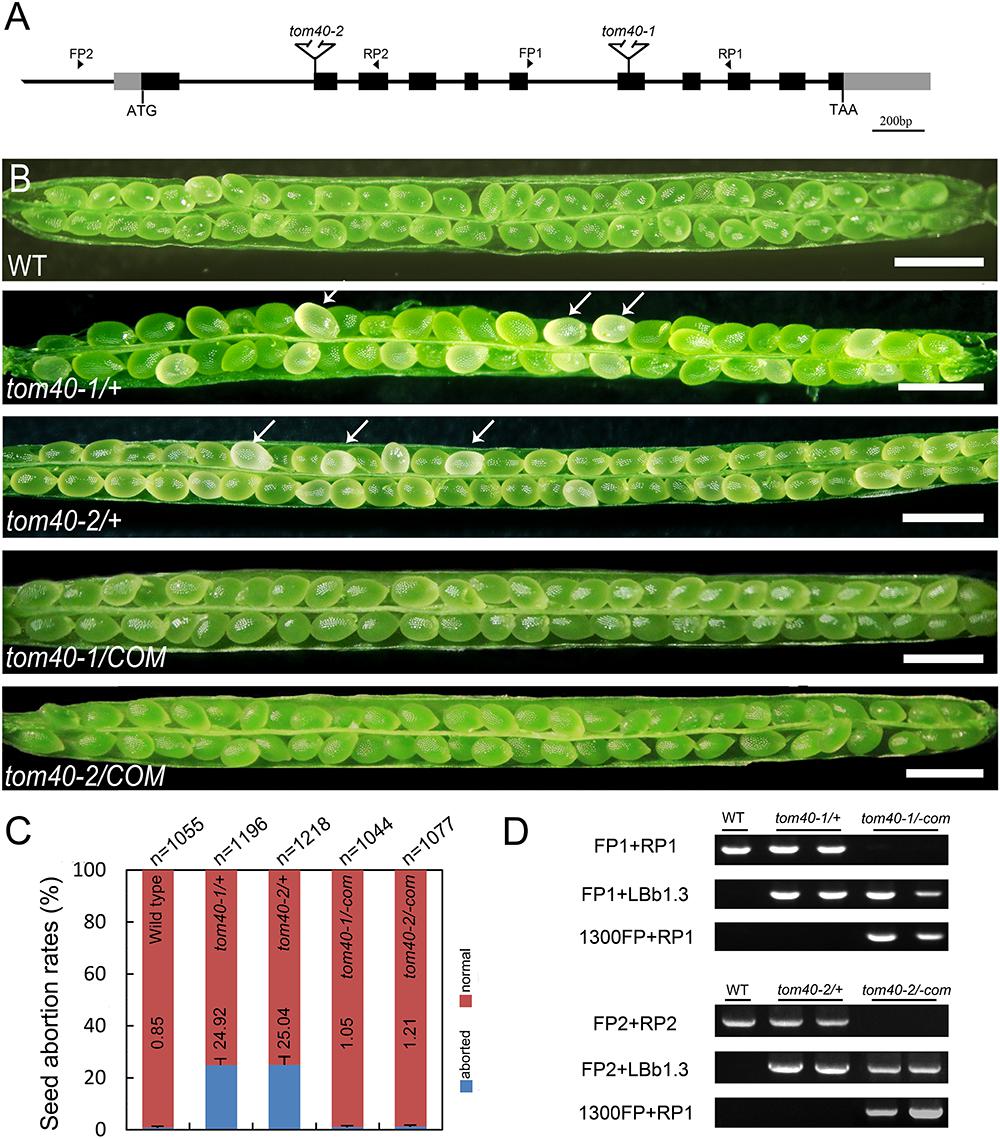
Figure 2. Characterization of AtTOM40 mutation and complementation assays of tom40-1/+ and tom40-2/+ mutants in Arabidopsis. (A) Schematic diagram of the AtTOM40 gene and T-DNA positions of the two mutants. Exons and promoter are shown in black and gray boxes, and introns are shown as lines, respectively. FP and RP indicate forward and reverse primers, Bar = 200 bp. (B) Ovule phenotypes in siliques of the wild type, tom40-1/+, tom40-2/+, functionally complemented tom40-1/+ and tom40-2/+ transgenic plants. The white arrows showed the aborted ovules. Bar = 1 mm. (C) Genotypic analysis of the wild type, tom40-1/+, tom40-2/+ and the complemented mutant plants. (D) The seed abortion rates in the wild type, tom40-1/+, tom40-2/+, and the complemented mutant plants. The total numbers of seeds counted are listed on the top, and the seed abortion rates are shown on the bottom.
To confirm whether the seed lethality was caused by knock-out of the AtTOM40 gene, we employed complementation tests. The full-length genomic sequence of AtTOM40 was introduced into two mutants, and the self-progenies of T3 generation homozygous transgenic plants were analyzed by genotyping (Figure 2D). The results displayed that no aborted seeds were observed in both complementation lines (Figure 2B), and the ratio of the aborted seeds descended to the similar level with wild type (Figure 2C), indicating that the seed-lethal phenotype could be rescued by the introduction of AtTOM40. All the results support the conclusion that the knock-out of AtTOM40 gene caused seed abortion, suggesting that the gene may play a critical role in embryo development in Arabidopsis.
AtTom40 Is Essential for Embryo Development and Pattern Formation
To clarify how the seed abortion occurred in the mutant plants, we monitored the process of embryo development with a whole mount clearing technique of ovules. The results showed that the difference was not noticeable between wild-type and tom40-1 homozygous embryos before the late globular stage (Figures 3A,E). However, from the time that the wild-type embryos developed into the heart stage (Figure 3B), the embryos in the homozygous tom40-1 mutant began to divide irregularly and could not produce proper organ primordium, including cotyledons and shoot apical meristem (SAM; Figure 3F). As development time goes, wild-type embryos progressed into torpedo and bent cotyledon stages (Figures 3C,D), while the abnormal embryos in the mutant plants still presented irregular globular embryos and could not produce cotyledon primordium (Figures 3G,H). In addition, despite the defect presented in embryo morphological development, the size of embryo propers in homozygous tom40-1 mutant still expanded gradually as developmental time goes, but finally was trapped and arrested (Supplementary Figure S2A). The results indicated that the embryo development and pattern formation were affected in the homozygous mutant plants, and embryo lethality occurred eventually. Next, we calculated ratios of embryos at sequential development stages in wild type and heterozygote plants. The results demonstrated that the tom40-1 mutant delayed growth as developmental progresses, and 24.39% of embryos at 6DAP were arrested at globular stages (Table 2). The frequency was consistent with the seed abortion rate of the mutant (Figure 2C), and similar results were obtained in tom40-2 mutant plants (Figures 3I–L and Table 2), indicating that the proper function of the AtTOM40 gene is required for the programmed embryogenesis in Arabidopsis.
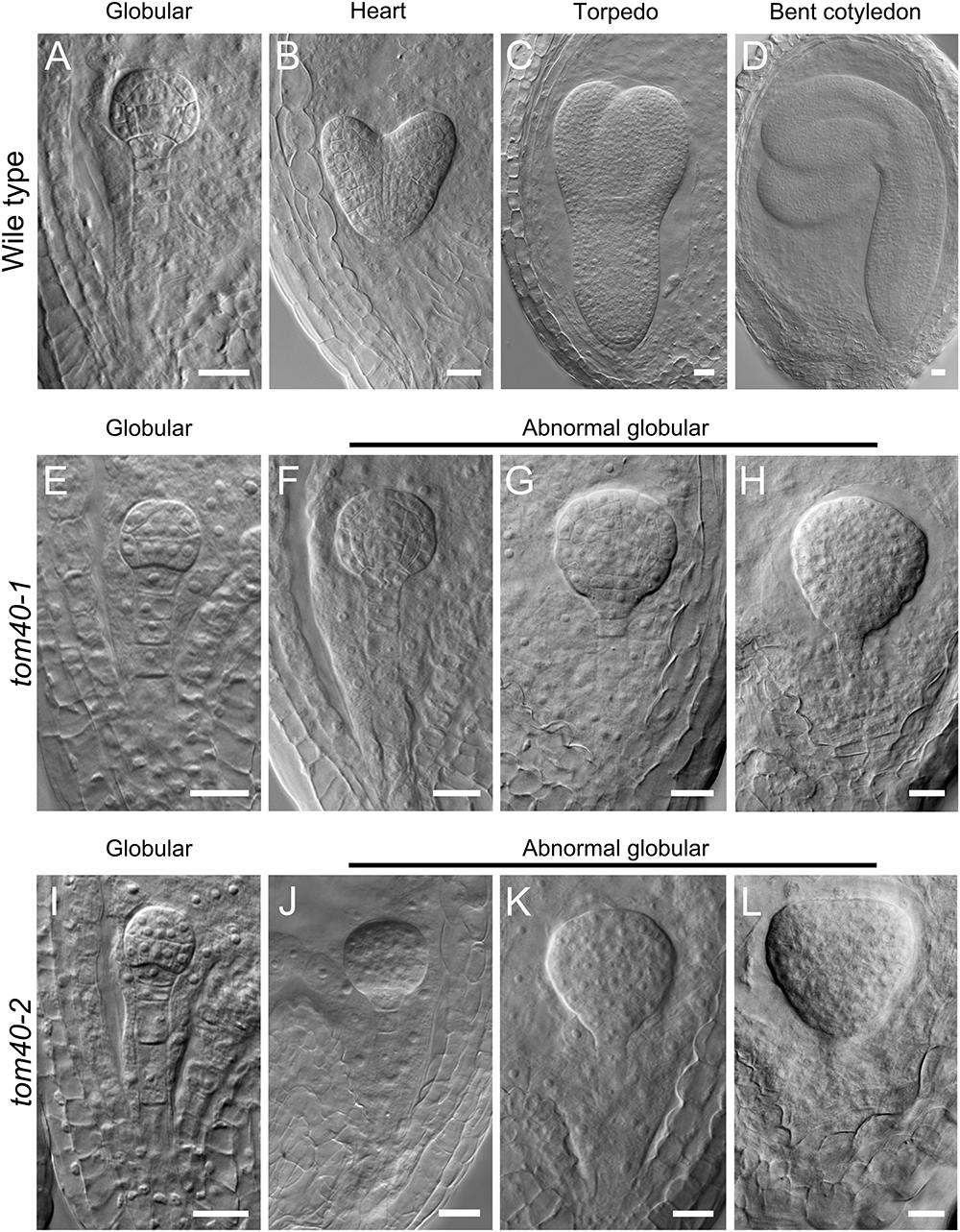
Figure 3. Status of embryo development in plants of the different genotypes. (A–D) Embryos from the globular stage to the bent cotyledon stage in wild-type ovules. (E–H) Embryos in homozygous tom40-1 ovules at parallel developmental stages with A–D. (I–L) Embryos in homozygous tom40-2 ovules at parallel developmental stages with A–D. Bar = 20 μm.
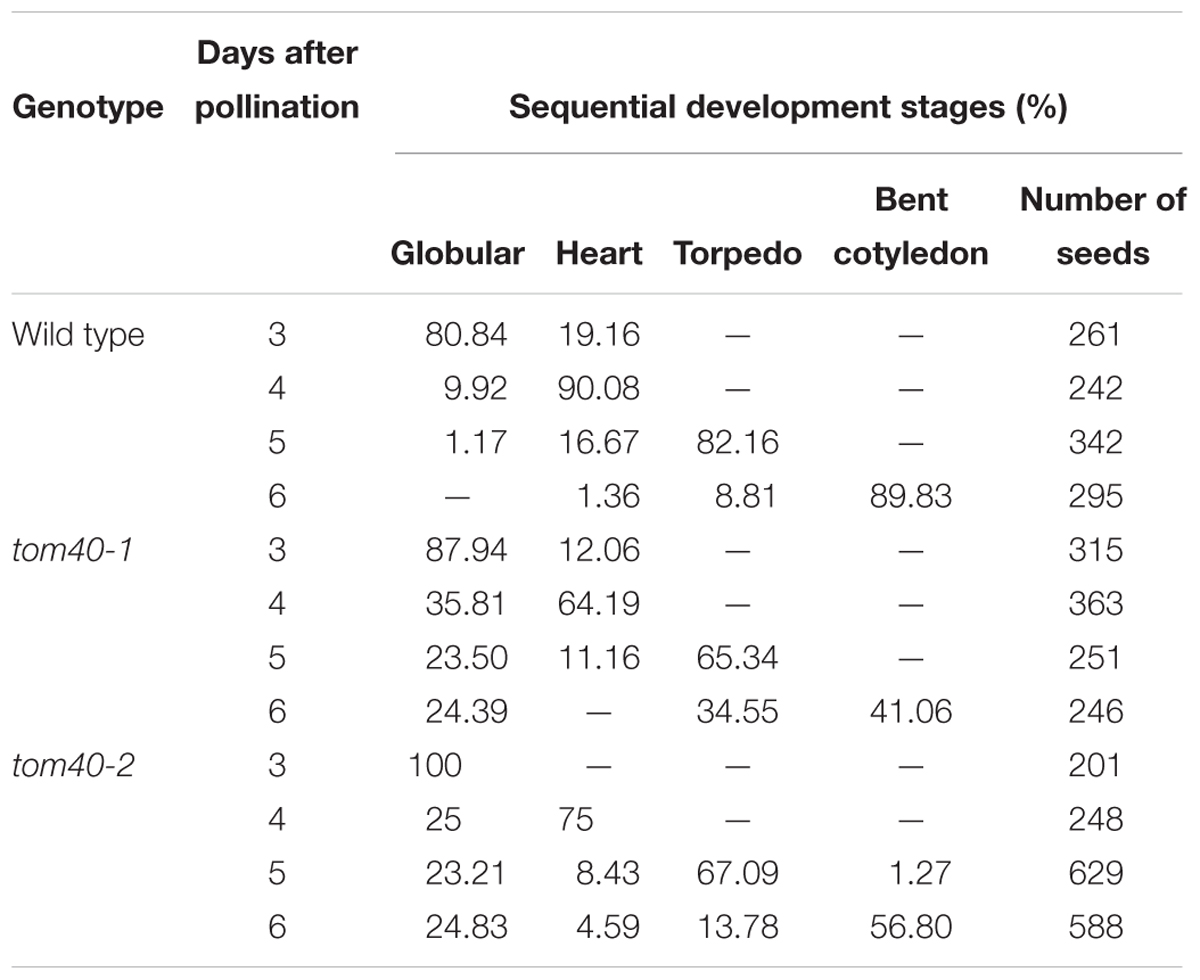
Table 2. Ratios of Arabidopsis embryos in the wild type and the mutants at sequential development stages.
Since the embryo and endosperm are both the major parts of seeds in Arabidopsis, we also examined the endosperm development status in wild type and tom40-1 mutant through its autofluorescence. The results showed that the endosperm free nucleus initiated cellularization at the heart stage in wild type (Supplementary Figure S2B), and the initiation of endosperm cellularization was also observed in both the heterozygous and homozygous tom40-1 mutant ovules at the same developmental stage (Supplementary Figures S2C,D). In brief, we draw the conclusion that AtTOM40 is responsible for seed abortion in mutant plants, and the loss of it can affect embryo differentiation and pattern formation, but not endosperm free nuclear division and cellularization.
AtTOM40 Is Widely Expressed in Different Tissues and Especially in Cotyledons Primordium
To study the expression pattern of the AtTOM40 gene, we obtained transgenic plants with ProAtTOM40::GUS construct. As a result, GUS signals could be detected in the veins of mature leaves (Figure 4A), sepals (Figure 4B), and 7-day-old seedlings (Figure 4C), respectively, especially in shoot meristems, hypocotyls, and vascular bundles of cotyledons (Figure 4C). However, the signal could be barely detected in anthers, stigmas and ovaries (Figure 4D), implying that the absence of AtTOM40 might not affect gametophyte development, which was consistent with the results mentioned above (Table 1). Furthermore, we observed that GUS activity accumulated intensively in the cotyledon of the matured embryos (Figure 4E), hinting the important role of AtTOM40 in cotyledon function.
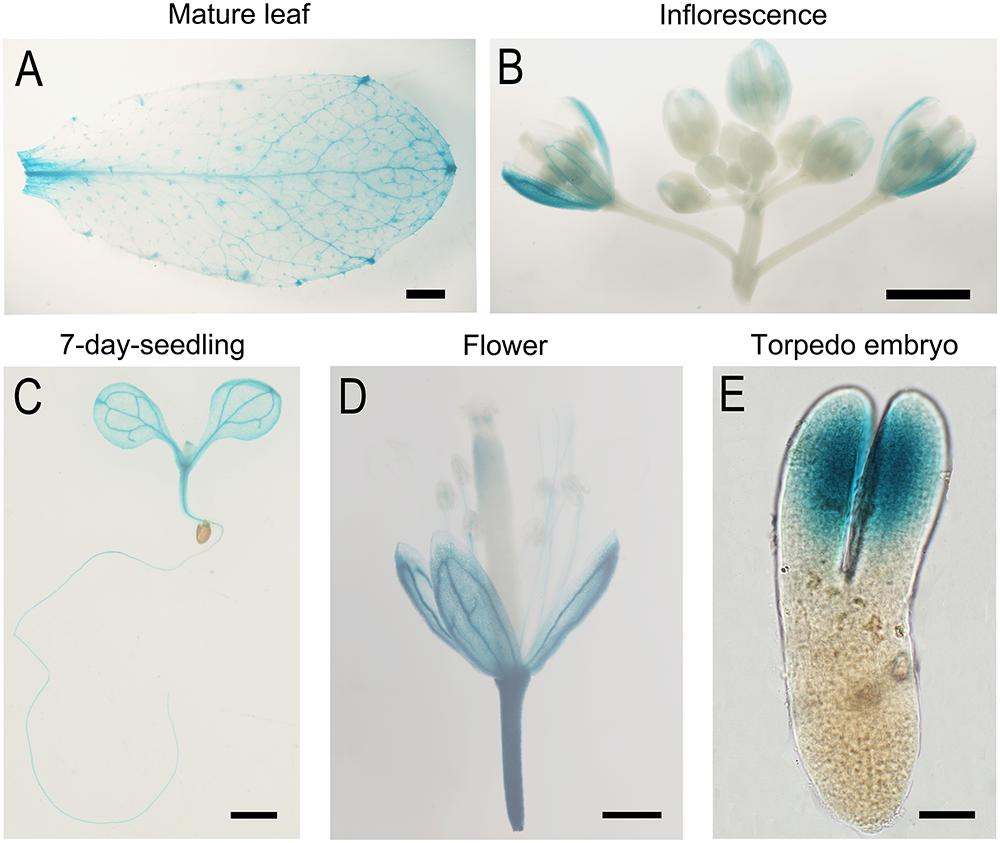
Figure 4. GUS Staining analysis of TOM40 in Arabidopsis. (A–E) GUS staining signals (blue staining) in different tissues of pTOM40::GUS transgenic plants. (A–D) Bar = 1mm, (E) Bar = 20 μm.
Through in situ hybridization technique, we detected the expression pattern of AtTOM40 in wild type ovules. The results showed that the expression signals were present both in embryos and endosperms (Figures 5A–G, arrows), especially in cotyledons of embryos (Figure 4E, arrowhead), demonstrating the role of AtTOM40 in Arabidopsis embryogenesis. To further investigate the expression pattern of the AtTOM40 gene, we conducted quantitative real-time PCR to measure its expression in different tissues and organs. The results showed that AtTOM40 gene is expressed extensively in almost all the organs at different levels, and is especially abundant in flowers (F), inflorescences (INF) and siliques at 1 day after pollination (Figure 5H). Taken together, we can draw the conclusion that AtTOM40 is widely expressed in different tissues, especially in cotyledons primordium.
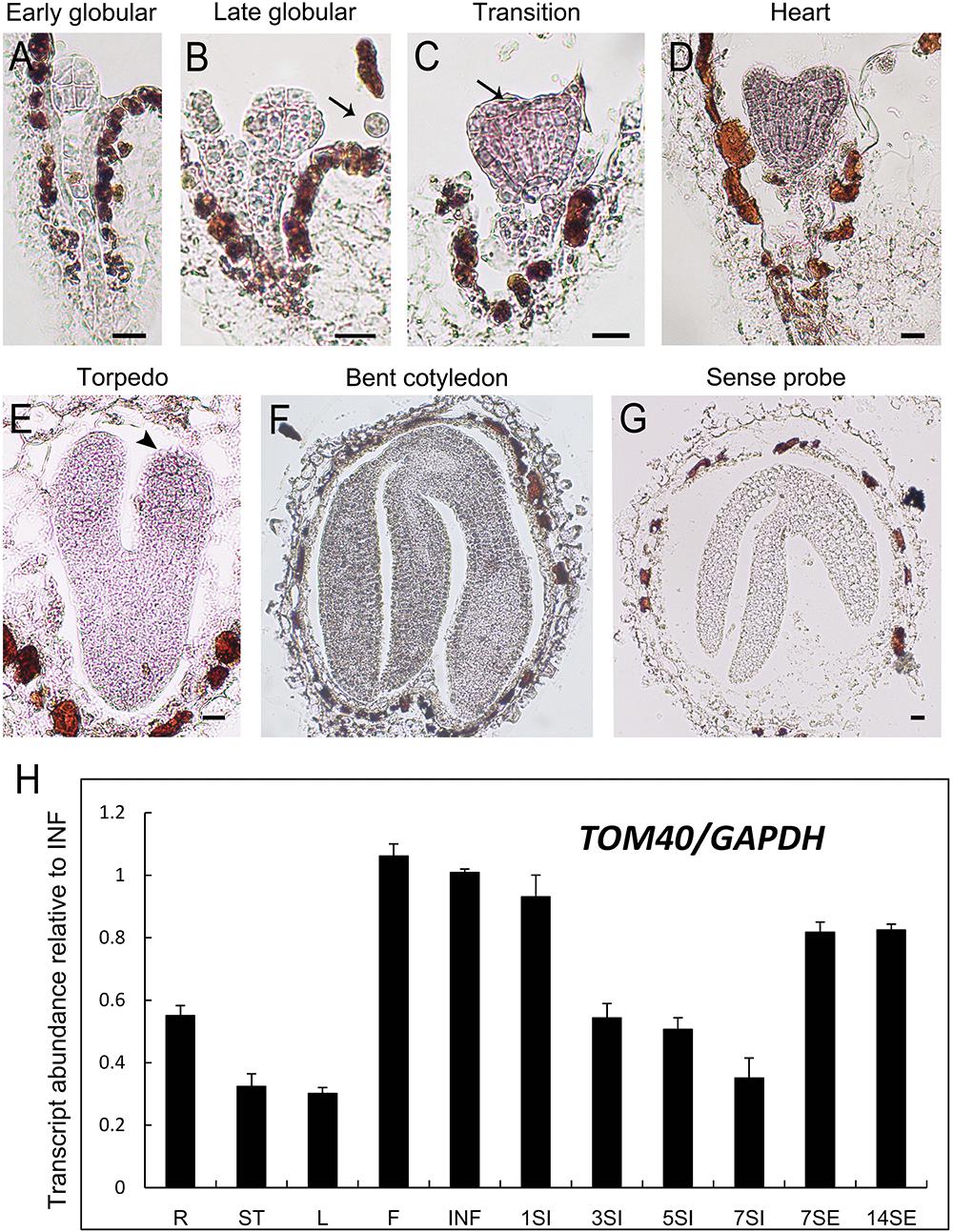
Figure 5. Expression analysis of TOM40 in Arabidopsis. (A–G) In situ hybridization of AtTOM40 transcripts (purple staining) accumulated in wild-type embryos and endosperm. Bar = 20 μm. (H) Quantitative real-time PCR analysis of AtTOM40 in various tissues. R, root; S, stem; L, leaf; F, flower; INF, inflorescence; 1SI–7SI, siliques at 1, 3, 5, 7 days after pollination; 7-14SE, seedlings at 7 and 14 days after germination.
AtTOM40 Is Localized in Mitochondria and Involved in Maintaining Normal Mitochondrial Biogenesis
As predicted above, AtTOM40 is a typical mitochondria translocase of the outer membrane (Figure 1). To confirm this, we fused the AtTOM40 promoter with enhanced GFP (eGFP) at the N-terminus to study the subcellular localization of AtTOM40 protein, in mesophyll protoplasts from the transgenic plants by confocal observation. As a result, the green fluorescence presents the localization of the AtTOM40 protein (Figure 6A), and the red fluorescence presents the localization of mitochondria after incubation with MitoTracker Red FM (Figure 6B). The green fluorescence and the red fluorescence were dotted displayed and could overlapped with each other (Figure 6C), indicating that the AtTOM40 localizes in mitochondria. To further investigate the ultrastructure of mitochondria in embryos, we collected ovules at 6DAP from tom40-1 homozygous mutant and wild type siliques and observed them under TEM (transmission electron microscopy). The mitochondria in wild type embryo proper cells were well developed (Figures 6D,E), but was swollen with less intramitochondrial content and no regular cristae structure in the tom40-1 mutant embryo proper cells (Figures 6F,G). The results demonstrate that AtTOM40 localizes in mitochondria and the lack of it impedes the proper biogenesis of mitochondria, suggesting the critical role of AtTOM40 in maintaining mitochondrial function in Arabidopsis.
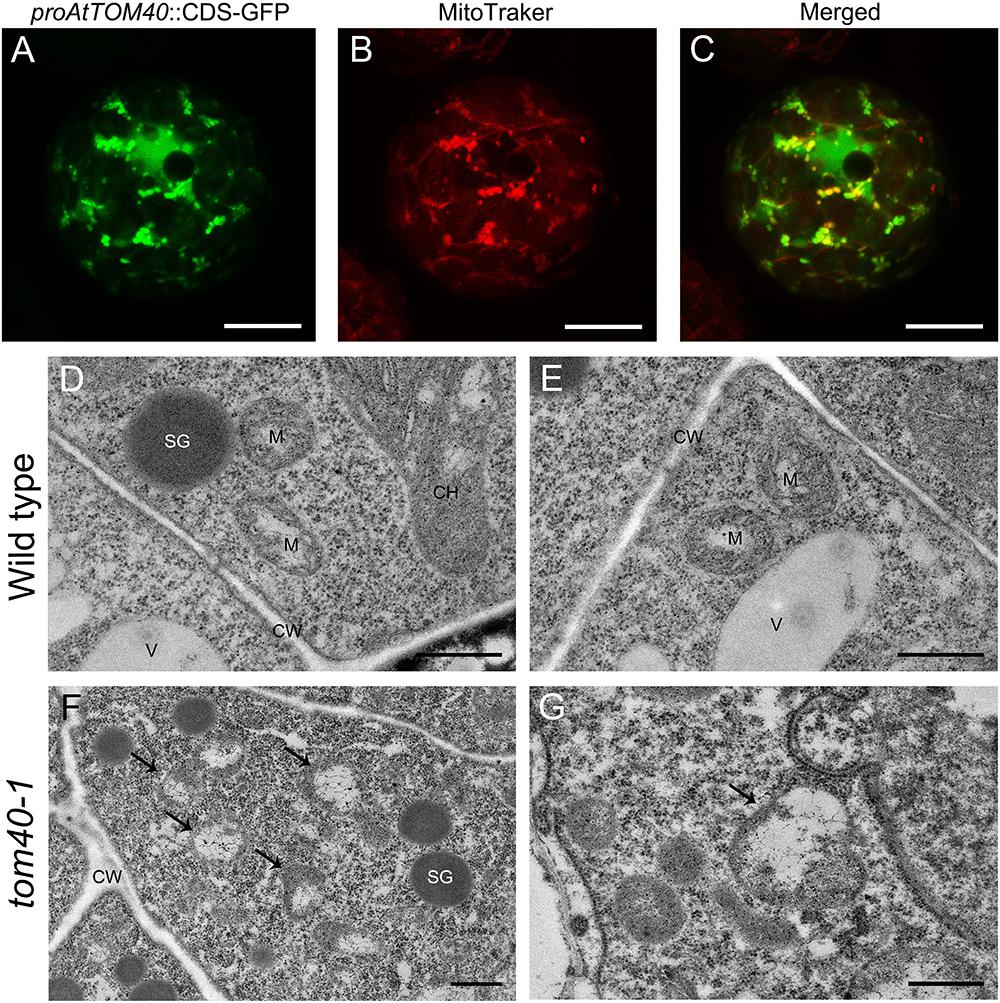
Figure 6. (A–C) Subcellular localization of AtTOM40 protein. Fluorescent signals in mesophyll protoplasts represents AtTOM40 (green), mitochondria (red) and colocalization (merged), respectively. Bar = 10 μm. (D–G) Ultrastructure of mitochondria in wild type (D,E) and tom40-1 (F,G) embryo proper cells at 6DAP. Bar = 500 nm. (F,G) Cells in tom40-1 embryo proper at 6DAP. (D,E,G) Bar = 500 nm, (F) Bar = 1 μm. SG: starch grain; M: mitochondrion; CH: chloroplast; V: vacuole; CW: cell wall.
Expression of Genes Encoding Outer Mitochondrial Membrane Proteins in the Homozygous tom40-1 Mutant Embryos Were Affected
Previous research work has demonstrated that the multi-subunit TOM complex acts as an entry gate on the outer mitochondrial membrane (OMM), and recognizes mitochondrial precursor proteins and passes them through the outer membrane (Ahting et al., 1999; Dudek et al., 2013; Bohnert et al., 2015; Fukasawa et al., 2017). In Arabidopsis, there still exist a series of other proteins on the OMM. They function in import and assembly, transport, morphology and membrane dynamics, energy and metabolism, and synthetase related to the OMM (Duncan et al., 2013b). To further explore how the mutation of AtTOM40 influenced the proteins on OMM, we performed quantitative real-time PCR to determine the changes of expression levels of OMM protein-related genes.
Considering that the albino ovules in tom40-1/+ plants were obvious at 6 days after pollination, we collected 6 DAP ovules in wild type and 6 DAP albino ovules in tom40-1/+ plants respectively for quantitative real-time PCR. At this time, embryos in the wild type had developed to the bent cotyledon stage (Figure 3D), while the abnormal embryos in tom40-1 plants were arrested at the irregular globular stage (Figure 3H). The preliminary experiment showed that the expression level of AtTOM40 in the tom40-1 albino ovules was about one fifth of the expression level in the wild type (Figure 7A), hence the subsequent experiments were reliable and feasible. The expression data showed that among 19 genes tested about three quarters genes were significantly up-regulated or down-regulated (Figures 7B–T). Among the genes involved in import and assembly (Figures 7B–J), transport (Figures 7K–L), morphology and membrane dynamics (Figures 7M–O), most genes were significantly up-regulated, which may due to the absence of AtTOM40 gene. In the meantime, among the genes that function in energy and metabolism (Figures 7P–Q), and synthetase related to the OMM (Figures 7R–T), about a half of the genes were down-regulated, which meant not all the OMM proteins were affected at the transcriptional level. In brief, the mutation of the AtTOM40 gene led to the abnormal expression of genes encoding OMM proteins in Arabidopsis.
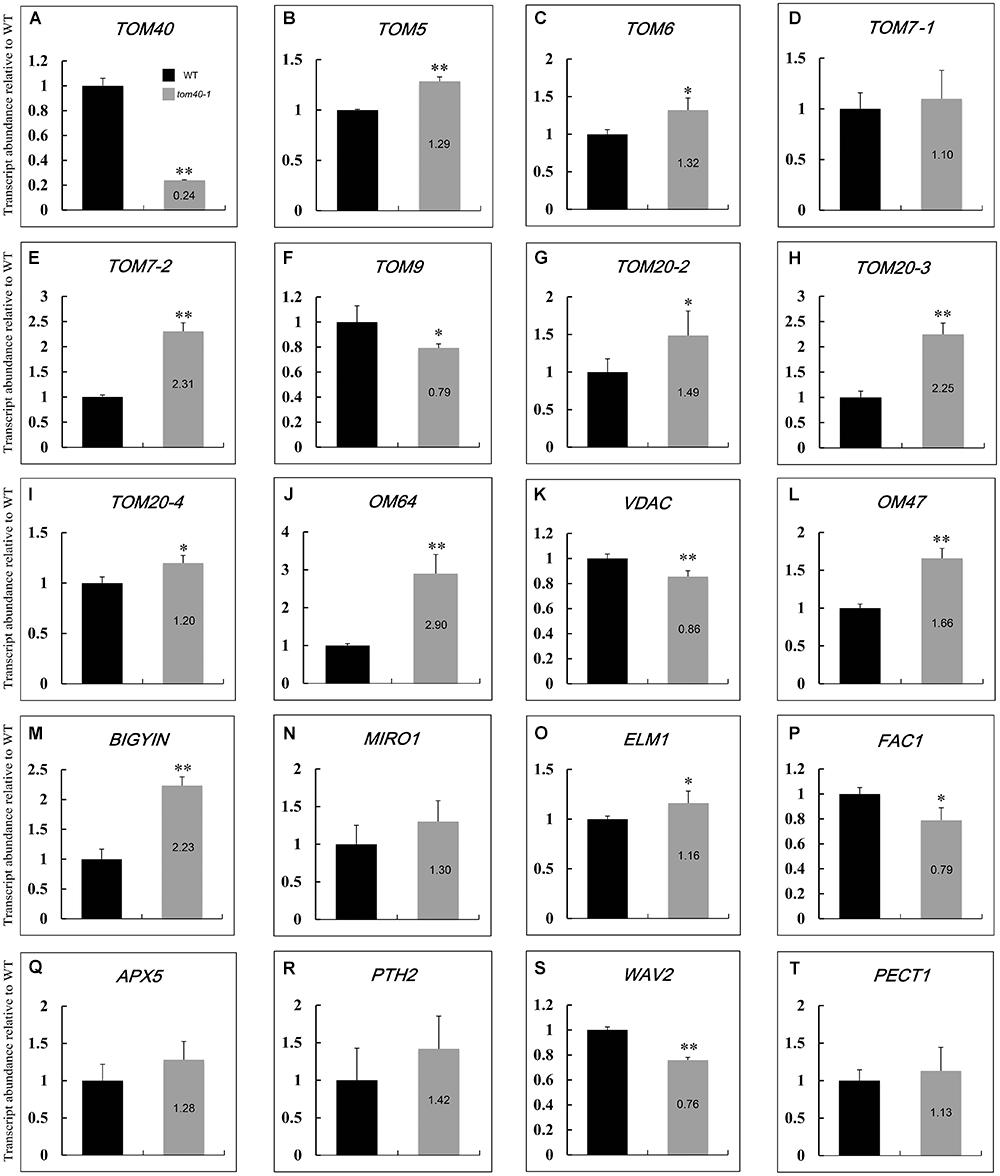
Figure 7. Relative expression levels of genes relevant to outer mitochondrial membrane (OMM) in Arabidopsis between wild type and tom40-1 albino ovules at 6DAP. (A) Expression level of AtTOM40 in the tom40-1 albino ovules was about one fifth of the expression level in the wild type. (B–J) Expression levels of genes involved in import and assembly of mitochondrion proteins. (K–L) Expression levels of genes involved in protein transport in mitochondrion. (M–O) Expression levels of genes involved in morphology and membrane dynamics. (P–Q) Expression levels of genes involved in energy and metabolism. (R–T) Expression levels of genes involved in synthetase related to the OMM. The asterisk indicates a significant difference (Student’s t-test, ∗P < 0.05, ∗∗P < 0.01).
Expression of Genes Relevant to Embryo Development and Pattern Formation in Homozygous tom40-1 Mutant Embryos Were Abnormal
It has been reported that the embryo development and pattern formation in Arabidopsis are precise and predictable. A complicated network control the specification of primordium of various tissue, and a number of genes have been proved to be involved in this process (Tzafrir et al., 2004; ten Hove et al., 2015; Palovaara et al., 2017). In this study, we found that mutation of AtTOM40 led to abnormal embryo development through affecting its pattern formation, and the embryos showed shape alterations and were unable to produce proper tissue primordium, including cotyledon and the shoot apical meristem (SAM; Figure 3). To further evaluate the effects of AtTOM40 mutation on embryo morphological development, we performed quantitative real-time PCR to determine the changes of expression levels of genes known to be essential for embryo pattern formation. The experiment was conducted in the 6 DAP ovules of wild type and 6 DAP albino ovules of tom40-1/+ siliques, respectively.
As a result, of the 19 genes tested, 18 of them were significantly abnormally expressed. The 18 abnormally expressed genes are consistent with the phenotype indicating major defects in patterning of the embryo, demonstrating that the embryo patterning was severely affected at the transcriptional level in the homozygous embryos of tom40-1/+ plants. Except for the WUS (WUSCHEL) gene, the genes expressed in the SAM, including CLV3 (CLAVATA3), LCR (LEAF CURLING RESPONSIVENESS), and STM (SHOOT MERISTEMLESS), were significantly down-regulated, (Figures 8B–E), which was correspondent with the abnormal shoot apical meristem development in the homozygous tom40-1 embryos (Figures 3F–H). Similar results were obtained in genes expressed in cotyledon (Figures 8F–I) and vascular primordium (Figures 8J–M). In addition, two genes expressed in protoderm (ML1, MERISTEM LAYER 1 and PDF2, PROTODERMAL FACTOR 2) were significantly down-regulated (Figures 8N,O), indicating the severe disorder of their expression in the protoderm. Furthermore, among the 5 regulators of hypophysis, 4 of them were significantly abnormally expressed (Figures 8P–S) except that the expression level of WOX5 (WUSCHEL RELATED HOMEOBOX 5) was almost unaffected (Figure 8T). In conclusion, the embryonic pattern formation was abnormal at transcriptional level in the homozygous tom40-1 mutant, indicating that the AtTOM40 gene plays an essential role in the embryo development and pattern formation in Arabidopsis.
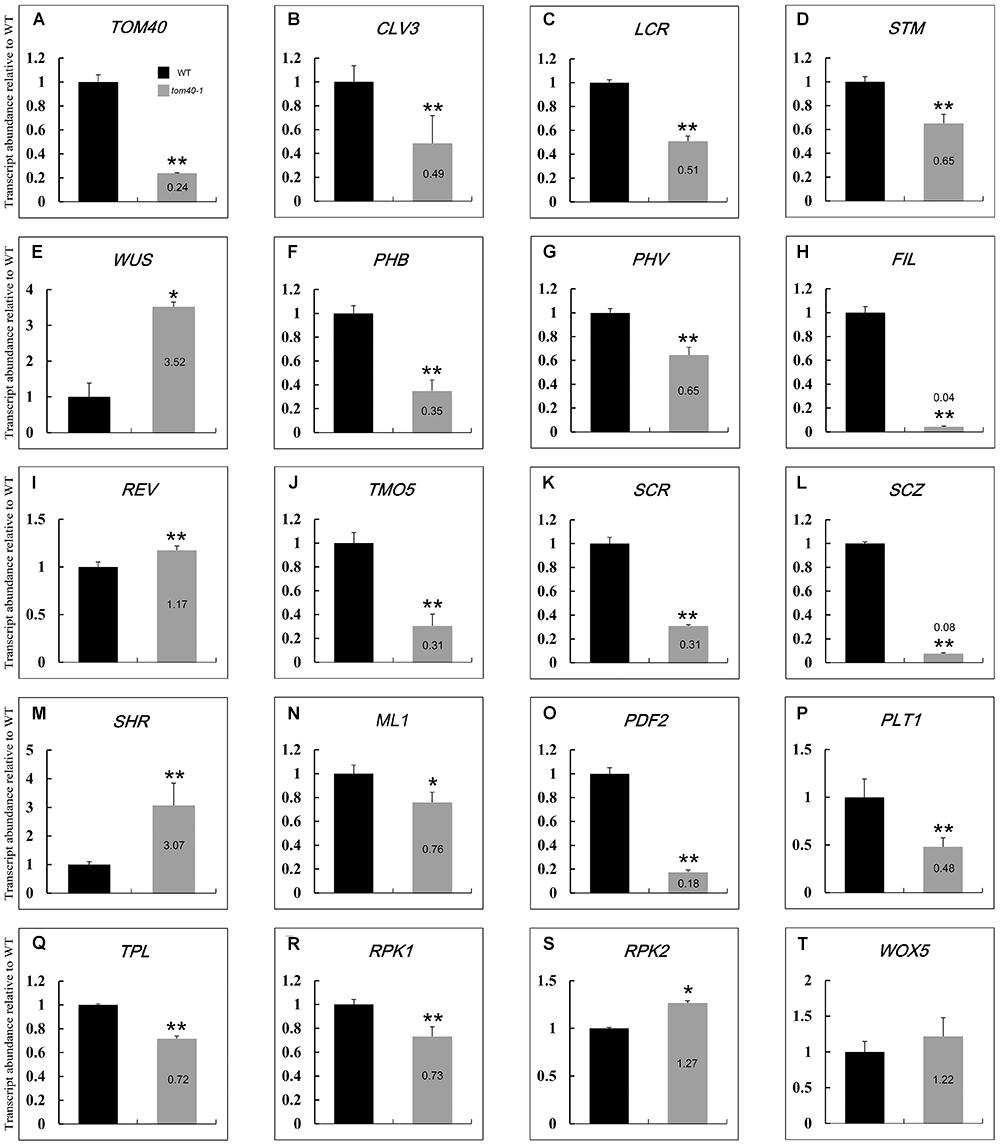
Figure 8. Relative expression levels of genes involved in embryo cell fate decision between wild type and the tom40-1 albino ovules at 6DAP. (A) Expression level of AtTOM40 in the tom40-1 albino ovules was about one fifth of the expression level in the wild type. (B–E) Expression levels of genes involved in SAM. (F–I) Expression levels of genes involved in cotyledon. (J–M) Expression levels of genes involved in vascular primordium. (N,O) Expression levels of genes involved in protoderm. (P–T) Expression levels of genes involved in hypophysis. The asterisk indicates a significant difference (Student’s t-test, ∗P < 0.05, ∗∗P < 0.01).
Discussion
TOM40 Is Essential for the Mitochondria Biogenesis in Different Species
Mitochondria are ubiquitous organelles given rise from endosymbiotic proteobacteria during evolution, and bounded by double-layer membranes (Dolezal et al., 2006; Bohnert et al., 2015). In the course of evolution, copies of genes were transferred from the mitochondrial DNA to the nuclear genome, and vast majority of mitochondrial proteins are synthesized in the cytosol (Perry et al., 2008). Preproteins synthesized in the cytosol are imported and translocated into the mitochondria, and the import process was mediated by membrane protein complexes named translocase. The mitochondrial translocase of the outer mitochondrial membrane (TOM) complex plays important role as an entry gate for nuclear-encoded mitochondrial precursor proteins (Vestweber et al., 1989; Bohnert et al., 2015), and TOM40, a central porin, serves as the core subunit in the complex (Duncan et al., 2013a; Murcha et al., 2014). Previous research demonstrated that TOM40 is a component of the mitochondrial protein import site, and forms the hydrophilic channel of the mitochondrial import pore for preproteins (Perry et al., 2008; Lackey et al., 2014). In yeast, it has been reported that Tom40 functions as the direct binding partner of Vps39 on mitochondria, therefore Vps39 mutant was defective in TOM binding and has lower survival rate during starvation (González Montoro et al., 2018). Furthermore, it was also reported in yeast that the levels of O2- released from the mitochondria coincide with TOM40 expression level. When blocking the TOM complex, it could result in inhibition of O2- release from the mitochondria, indicating the role of TOM40 in respiration of mitochondria (Budzińska et al., 2009). In this study, we found that the TOM40 gene in Arabidopsis was wildly expressed in different tissues and especially in cotyledons primordium (Figures 4, 5), it was also found to be localized in mitochondria and proper mitochondrial biogenesis was disturbed in homozygous tom40-1 mutant embryos (Figure 6), implying that the knock-out of AtTOM40 led to impaired mitochondria. This may be caused by the failure of the preprotein import.
In Arabidopsis, the outer mitochondrial membrane (OMM) proteins function in a series of biological events of mitochondria. TOM9, the plant homolog of yeast TOM22, was reported to be critical for TOM complex assembly, and the Dexamethasone induced RNAi gene silencing of TOM9.2 (the major isoform of TOM9) resulted in a severe reduction in the mature TOM complex (Parvin et al., 2017). Another plant-specific OMM protein AtOM47 was also reported, it plays a role in leaf senescence by transporting metabolic intermediates into and out of mitochondria (Li et al., 2016). In our study, the expression of genes encoding OMM proteins was further detected in the mutant tom40-1 ovules (Figure 7). For the genes encoding the subunits of TOM complex, nearly all the expression level was significantly up-regulated, except that the expression of TOM9 was significantly down- regulated. The results implied that the stability of the TOM complex was disturbed due to the lack of AtTOM40. Moreover, the expression of other OMM proteins, including proteins involved in import and assembly, transport, morphology and membrane dynamics of mitochondria, was also disturbed in the tom40-1 mutant, which strongly implied that AtTOM40 gene may be crucial for normal expression of the OMM genes. Hence, we speculate reasonably that the lack of AtTOM40 gene caused abnormity of the whole TOM complex and then disordered the mitochondrial import apparatus. Thus AtTOM40 is essential for the mitochondria biogenesis.
TOM40 Plays an Important Role in Embryo Development and Pattern Formation Through Maintaining the Function of the Mitochondria Outer Membrane
Previous research has shown that mitochondria played a fundamental role in embryo development in plants (Colas des Francs-Small and Small, 2014), a number of genes located in mitochondria have been found to be essential for embryo development from the early stage in Arabidopsis (Yamaoka et al., 2011; Mansilla et al., 2015), and even caused seed abortion in Arabidopsis (Song and Liu, 2015; Guan et al., 2015; Fromm et al., 2016) and Maize (Wang et al., 2017). In Arabidopsis, the late embryogenesis abundant (LEA) proteins protect the mitochondrial inner membrane in the case of desiccation tolerance, in order to ensure the function of the energy-generating system. And the embryonal mitochondria undergo a process of development before becoming fully functional. Efficient import machinery are needed to allow proper biogenesis of mitochondria (Tolleter et al., 2007). It has also been reported that in rice and Arabidopsis, during the process of embryo development and seed maturation, appropriate preprotein import and mitochondrial biogenesis are necessary for bioenergetic and metabolic functions of mitochondria, including TCA cycle and electron transport chain assembly (Howell et al., 2006; Law et al., 2012). The ETHE1 gene catalyzes the oxidation of persulfides in the mitochondrial matrix and is essential for early embryo development in Arabidopsis (Krüßel et al., 2014). The results in our lab showed that translocase of the inner membrane 9 and 10 (TIM9 and TIM 10) are essential for maintaining mitochondrial function during early embryo cell and endosperm free nucleus divisions in Arabidopsis (Deng et al., 2014). Furthermore, a series of evidence demonstrated that the morphogenesis was crucial for the production of embryo in plant development, and the molecular mechanism of the process was well verified (Tzafrir et al., 2004; Lau et al., 2012; ten Hove et al., 2015). In this study we confirmed that AtTOM40 was a typical translocase of the outer membrane, abnormal embryo development and seed abortion in homozygous tom40 mutant embryos were caused by the absence of AtTOM40 (Figure 2). We also found that embryo development and organ differentiation was suppressed in homozygous mutant embryos (Figure 3), and the failure of morphogenesis was correlated with the misexpression of tissue-specific genes (Figure 8). Taken together, our results revealed that AtTOM40 was essential for the maintenance of normal mitochondrial biogenesis in Arabidopsis, and played an important role in the process of embryo development.
The mechanism of how is TOM40 is involved in embryo development and morphogenesis remains unclear. It is known that from the globular stage to the heart and the later stage, a large number of cellular proliferation and organ primordial differentiation occurred in Arabidopsis embryos (Yoshida et al., 2014). These events laid the foundation for the mature embryo formation and the whole plant development, for which abundant energy productions are needed (Guitton et al., 2004; Xiang et al., 2011). As energy-supply organelles, mitochondria need proper biogenesis and efficient import machinery to provide sufficient ATP for the biological events. Hence, we speculate reasonably that the AtTOM40 gene was involved in the quality control of mitochondria, and the proper function was essential for the biogenesis of mitochondria activities and structure. Therefore, the loss of AtTOM40 led to the functional defect of mitochondria and affected the energy metabolism, and consequently caused the insufficient ATP supply for cell differentiation and embryo organ primordial development, and finally caused embryo lethality. Since mitochondria are maternally inherited in higher plants (Baroux et al., 2008; Nagata, 2010; Xin et al., 2012), it could be derived that in the mutants, the residual maternal energy could enable gametes to complete fertilization and undergo a few rounds of cell division after it. However, the mitochondrial defection may not be able to support the further cell differentiation and embryo organ primordial formation. Our results confirmed that the AtTOM40 localized to the mitochondria and the loss of it would lead to impaired mitochondria structure and embryonic defection. It has been reported that the endosperm undergoes eight rounds of mitosis, and then was followed by cellularization. In the meantime, the embryo development had just reached the dermatogen stage (Boisnard-Lorig et al., 2001; Berger, 2003). Therefore, we can speculate reasonably that in the tom40-1 mutant, the residual maternal energy could support only about eight rounds of cell divisions. Hence, the process of endosperm cellularization could be finished, while the embryo was arrested at the globular stage. Besides, the unparallel development may be derived from a signaling rather than metabolic defect (Sosso et al., 2012). Our work provides a novel insight about the biological function of TOM40 in Arabidopsis, and could help researchers to further understand the relationship between mitochondrial biogenesis and embryo development, but the exact mechanism of AtTOM40 still needs more detailed analyses and further investigation.
Data Availability
All datasets generated for this study are included in the manuscript and/or the Supplementary Files.
Author Contributions
YiH performed most of the experiments, analyzed the research results, and wrote the manuscript. WZ performed the ultrathin section and TEM observation. ZW and YuH performed the Quantitative Real-Time PCR. YZ and JQ participated in the RNA in situ hybridization. XW and YR participated in the mutant verification and complementation analysis. JZ conceived the research plans, guided the whole study, and modified the manuscript.
Funding
This research work was supported by the National Natural Science Foundation of China (Nos. 31870303 and 31670312).
Conflict of Interest Statement
The authors declare that the research was conducted in the absence of any commercial or financial relationships that could be construed as a potential conflict of interest.
Supplementary Material
The Supplementary Material for this article can be found online at: https://www.frontiersin.org/articles/10.3389/fpls.2019.00389/full#supplementary-material
FIGURE S1 | The evolutionary relationship and conservation of TOM40 among different species. (A) Phylogenetic tree of TOM40 among different species. (B) Analyses of conserved domain in TOM40. Gray area represent the whole protein sequence and the blue area represents the conserved porin domain. Cg, Candida glabrata; Sc, Saccharomyces cerevisiae; At, Arabidopsis thaliana; Os, Oryza sativa; Hs, Homo sapiens; Mm, Mus musculus; Zm, Zea mays; Nt, Nicotiana tabacum; Gm, Glycine max; Gh, Gossypium hirsutum; Dr, Danio rerio; Bn, Brassica napus; Sb, Sorghum bicolor.
FIGURE S2 | Developmental status of embryos and endosperm in plants of different genotypes. (A) Size of homozygous embryo propers in tom40-1 and tom40-2 at 3, 5, 7, 9 days after pollination (DAP). (B–D) The process of endosperm cellularization had initiated in wild type (B), heterozygous (C) and homozygous (D) ovules at 4DAP. Bar = 20 μm.
TABLE S1 | Primers (5′ to 3′) used in this study.
Footnotes
- ^https://www.arabidopsis.org
- ^https://blast.ncbi.nlm.nih.gov/Blast.cgi
- ^https://www.swissmodel.expasy.org/
- ^https://www.roche.com
- ^https://www.arabidopsis.org
References
Adams, K. L., and Palmer, J. D. (2003). Evolution of mitochondrial gene content: gene loss and transfer to the nucleus. Mol. Phylogenet. Evol. 29, 380–395. doi: 10.1016/S1055-7903(03)00194-5
Ahting, U., Thieffry, M., Engelhardt, H., Hegerl, R., Neupert, W., and Nussberger, S. (2001). Tom40, the pore-forming component of the protein-conducting TOM channel in the outer membrane of mitochondria. J. Cell Biol. 11, 1151–1160. doi: 10.1083/jcb.153.6.1151
Ahting, U., Thun, C., Hegerl, R., Typke, D., Nargang, F. E., Neupert, W., et al. (1999). The TOM core complex: the general protein import pore of the outer membrane of mitochondria. J. Cell Biol. 29, 959–968. doi: 10.1083/jcb.147.5.959
Alcock, F., Clements, A., Webb, C., Lithgow, T., and Evolution. (2010). Tinkering inside the organelle. Science 327, 649–650. doi: 10.1126/science.1182129
Baroux, C., Autran, D., Gillmor, C. S., Grimanelli, D., and Grossniklaus, U. (2008). The maternal to zygotic transition in animals and plants. Cold Spring Harb. Symp. Quant. Biol. 73, 89–100. doi: 10.1101/sqb.2008.73.053
Bausewein, T., Mills, D. J., Langer, J. D., Nitschke, B., Nussberger, S., and Kühlbrandt, W. (2017). Cryo-EM Structure of the TOM Core Complex from Neurospora crassa. Cell 170, 693–700.e7. doi: 10.1016/j.cell.2017.07.012.
Becker, T., Wenz, L. S., Thornton, N., Stroud, D., Meisinger, C., Wiedemann, N., et al. (2011). Biogenesis of mitochondria: dual role of Tom7 in modulating assembly of the preprotein translocase of the outer membrane. J. Mol. Biol. 405, 113–124. doi: 10.1016/j.jmb.2010.11.002
Berger, F. (2003). Endosperm: the crossroad of seed development. Curr. Opin. Plant Biol. 6, 42–50. doi: 10.1016/S1369526602000043
Bleckmann, A., Alter, S., and Dresselhaus, T. (2014). The beginning of a seed: regulatory mechanisms of double fertilization. Front. Plant Sci. 11:452. doi: 10.3389/fpls.2014.00452
Bohnert, M., Pfanner, N., and van der Laan, M. (2015). Mitochondrial machineries for insertion of membrane proteins. Curr. Opin. Struct. Biol. 33, 92–102. doi: 10.1016/j.sbi.2015.07.013
Boisnard-Lorig, C., Colon-Carmona, A., Bauch, M., Hodge, S., Doerner, P., Bancharel, E., et al. (2001). Dynamic analyses of the expression of the HISTONE::YFP fusion protein in Arabidopsis show that syncytial endosperm is divided in mitotic domains. Plant Cell 13, 495–509. doi: 10.1105/tpc.13.3.495
Brewer, P. B., Heisler, M. G., Hejátko, J., Friml, J., and Benková, E. (2006). In situ hybridization for mRNA detection in Arabidopsis tissue sections. Nat. Protoc. 1, 1462–1467. doi: 10.1038/nprot.2006.226
Budzińska, M., Gałgańska, H., Karachitos, A., Wojtkowska, M., and Kmita, H. (2009). The TOM complex is involved in the release of superoxide anion from mitochondria. J. Bioenerg. Biomembr. 41, 361–367. doi: 10.1007/s10863-009-9231-9
Capron, A., Chatfield, S., Provart, N., and Berleth, T. (2009). Embryogenesis: pattern formation from a single cell. Arabidopsis Book 7, e0126. doi: 10.1199/tab.0126
Cavalier-Smith, T. (2009). Predation and eukaryote cell origins: a coevolutionary perspective. Int. J. Biochem. Cell Biol. 41, 307–322. doi: 10.1016/j.biocel.2008.10.002
Chen, H., Li, S., Li, L., Hu, H., and Zhao, J. (2018). Arabidopsis EMB1990 Encoding a Plastid-Targeted YlmG protein is required for chloroplast biogenesis and embryo development. Front. Plant Sci. 16:181. doi: 10.3389/fpls.2018.00181
Chen, H., Zou, W., and Zhao, J. (2015). Ribonuclease J is required for chloroplast and embryo development in Arabidopsis. J. Exp. Bot. 66, 2079–2091. doi: 10.1093/jxb/erv010
Clough, S. J., and Bent, A. F. (1998). Floral dip: a simplified method for Agrobacterium-mediated transformation of Arabidopsis thaliana. Plant J. 16, 735–743. doi: 10.1046/j.1365-313x.1998.00343.x
Colas, des Francs-Small, C., and Small, I. (2014). Surrogate mutants for studying mitochondrially encoded functions. Biochimie 100, 234–242. doi: 10.1016/j.biochi.2013.08.019
Córdoba, J. P., Marchetti, F., Soto, D., Martin, M. V., Pagnussat, G. C., and Zabaleta, E. (2016). The CA domain of the respiratory complex I is required for normal embryogenesis in Arabidopsis thaliana. J. Exp. Bot. Mar. 67, 1589–1603. doi: 10.1093/jxb/erv556
Crimi, M., and Rigolio, R. (2008). The mitochondrial genome, a growing interest inside an organelle. Int. J. Nanomedicine 3, 51–57.
Deng, Y., Zou, W., Li, G., and Zhao, J. (2014). TRANSLOCASE OF THE INNER MEMBRANE9 and 10 are essential for maintaining mitochondrial function during early embryo cell and endosperm free nucleus divisions in Arabidopsis. Plant Physiol. 166, 853–868. doi: 10.1104/pp.114.242560
Dietmeier, K., Hönlinger, A., Bömer, U., Dekker, P. J., Eckerskorn, C., Lottspeich, F., et al. (1997). Tom5 functionally links mitochondrial preprotein receptors to the general import pore. Nature 388, 195–200. doi: 10.1038/40663
Dolezal, P., Likic, V., Tachezy, J., and Lithgow, T. (2006). Evolution of the molecular machines for protein import into mitochondria. Science 313, 314–318. doi: 10.1126/science.1127895
Dudek, J., Rehling, P., and van der Laan, M. (2013). Mitochondrial protein import: common principles and physiological networks. Biochim. Biophys. Acta 1833, 274–285. doi: 10.1016/j.bbamcr.2012.05.028
Duncan, O., Murcha, M. W., and Whelan, J. (2013a). Unique components of the plant mitochondrial protein import apparatus. Biochim Biophys Acta 1833, 304–313. doi: 10.1016/j.bbamcr.2012.02.015
Duncan, O., Taylor, N. L., Carrie, C., Eubel, H., Kubiszewski-Jakubiak, S., Zhang, B., et al. (2011). Multiple lines of evidence localize signaling, morphology, and lipid biosynthesis machinery to the mitochondrial outer membrane of Arabidopsis. Plant Physiol. 157, 1093–1113. doi: 10.1104/pp.111.183160
Duncan, O., van der Merwe, M. J., Daley, D. O., and Whelan, J. (2013b). The outer mitochondrial membrane in higher plants. Trends Plant Sci. 18, 207–217. doi: 10.1016/j.tplants.2012.12.004
Fromm, S., Braun, H. P., and Peterhansel, C. (2016). Mitochondrial gamma carbonic anhydrases are required for complex I assembly and plant reproductive development. New Phytol. 211, 194–207. doi: 10.1111/nph.13886
Fukasawa, Y., Oda, T., Tomii, K., and Imai, K. (2017). Origin and evolutionary alteration of the mitochondrial import system in eukaryotic lineages. Mol. Biol. Evol. 34, 1574–1586. doi: 10.1093/molbev/msx096
Gessmann, D., Flinner, N., Pfannstiel, J., Schlösinger, A., Schleiff, E., Nussberger, S., et al. (2011). Structural elements of the mitochondrial preprotein-conducting channel Tom40 dissolved by bioinformatics and mass spectrometry. Biochim. Biophys. Acta 1807, 1647–1657. doi: 10.1016/j.bbabio.2011.08.006
González Montoro, A., Auffarth, K., Hönscher, C., Bohnert, M., Becker, T., Warscheid, B., et al. (2018). Vps39 Interacts with Tom40 to establish one of two functionally distinct vacuole-mitochondria contact sites. Dev. Cell 45, 621-636.e7. doi: 10.1016/j.devcel.2018.05.011
Gray, M. W., Burger, G., and Lang, B. F. (1999). Mitochondrial evolution. Science 283, 1476–1481. doi: 10.1126/science.283.5407.1476
Guan, X., Chen, H., Abramson, A., Man, H., Wu, J., Yu, O., et al. (2015). A phosphopantetheinyl transferase that is essential for mitochondrial fatty acid biosynthesis. Plant J. 84, 718–732. doi: 10.1111/tpj.13034
Guitton, A. E., Page, D. R., Chambrier, P., Lionnet, C., Faure, J. E., Grossniklaus, U., et al. (2004). Identification of new members of Fertilisation Independent Seed Polycomb Group pathway involved in the control of seed development in Arabidopsis thaliana. Development 131, 2971–2981. doi: 10.1242/dev.01168
Gutiérrez-Marcos, J. F., Dal Prà, M., Giulini, A., Costa, L. M., Gavazzi, G., Cordelier, S., et al. (2007). empty pericarp4 encodes a mitochondrion-targeted pentatricopeptide repeat protein necessary for seed development and plant growth in maize. Plant Cell 19, 196–210. doi: 10.1105/tpc.105.039594
Hamamura, Y., Nagahara, S., and Higashiyama, T. (2012). Double fertilization on the move. Curr. Opin. Plant Biol. 15, 70–77. doi: 10.1016/j.pbi.2011.11.001
Hill, K., Model, K., Ryan, M. T., Dietmeier, K., Martin, F., Wagner, R., et al. (1998). Tom40 forms the hydrophilic channel of the mitochondrial import pore for preproteins. Nature 395, 516–521. doi: 10.1038/26780
Howell, K. A., Millar, A. H., and Whelan, J. (2006). Ordered assembly of mitochondria during rice germination begins with pro-mitochondrial structures rich in components of the protein import apparatus. Plant Mol. Biol. 60, 201–223. doi: 10.1007/s11103-005-3688-7
Jänsch, L., Kruft, V., Schmitz, U. K., and Braun, H. P. (1998). Unique composition of the preprotein translocase of the outer mitochondrial membrane from plants. J. Biol. Chem. 273, 17251–17257. doi: 10.1074/jbc.273.27.17251
Ju, Y., Liu, C., Lu, W., Zhang, Q., and Sodmergen. (2016). Arabidopsis mitochondrial protein slow embryo development1 is essential for embryo development. Biochem. Biophys. Res. Commun. 474, 371–376. doi: 10.1016/j.bbrc.2016.04.114
Kato, H., and Mihara, K. (2008). Identification of Tom5 and Tom6 in the preprotein translocase complex of human mitochondrial outer membrane. Biochem. Biophys. Res. Commun. 369, 958–963. doi: 10.1016/j.bbrc.2008.02.150
Ke, X., Zou, W., Ren, Y., Wang, Z., Li, J., Wu, X., et al. (2017). Functional divergence of chloroplast Cpn60α subunits during Arabidopsis embryo development. PLoS Genet. 13:e1007036. doi: 10.1371/journal.pgen.1007036
Krüßel, L., Junemann, J., Wirtz, M., Birke, H., Thornton, J. D., Browning, L. W., et al. (2014). The mitochondrial sulfur dioxygenase ETHYLMALONIC ENCEPHALOPATHY PROTEIN1 is required for amino acid catabolism during carbohydrate starvation and embryo development in Arabidopsis. Plant Physiol. 165, 92–104. doi: 10.1104/pp.114.239764
Künkele, K. P., Heins, S., Dembowski, M., Nargang, F. E., Benz, R., Thieffry, M., et al. (1998). The preprotein translocation channel of the outer membrane of mitochondria. Cell 93, 1009–1019. doi: 10.1016/S0092-8674(00)81206-4
Kuszak, A. J., Jacobs, D., Gurnev, P. A., Shiota, T., Louis, J. M., Lithgow, T., et al. (2015). Evidence of distinct channel conformations and substrate binding affinities for the mitochondrial outer membrane protein translocase pore Tom40. J. Biol. Chem. 290, 26204–26217. doi: 10.1074/jbc.M115.642173
Kuzmenko, A., Tankov, S., English, B. P., Tarassov, I., Tenson, T., Kamenski, P., et al. (2011). Single molecule tracking fluorescence microscopy in mitochondria reveals highly dynamic but confined movement of Tom40. Sci. Rep. 1:195. doi: 10.1038/srep00195
Lackey, S. W., Taylor, R. D., Go, N. E., Wong, A., Sherman, E. L., and Nargang, F. E. (2014). Evidence supporting the 19 β-strand model for Tom40 from cysteine scanning and protease site accessibility studies. J. Biol. Chem. 289, 21640–21650. doi: 10.1074/jbc.M114.578765
Lafon-Placette, C., and Köhler, C. (2014). Embryo and endosperm, partners in seed development. Curr. Opin. Plant. Biol. 17, 64–69. doi: 10.1016/j.pbi.2013.11.008
Lau, S., Slane, D., Herud, O., Kong, J., and Jürgens, G. (2012). Early embryogenesis in flowering plants: setting up the basic body pattern. Annu. Rev. Plant Biol. 63, 483–506. doi: 10.1146/annurev-arplant-042811-105507
Law, S. R., Narsai, R., Taylor, N. L., Delannoy, E., Carrie, C., Giraud, E., et al. (2012). Nucleotide and RNA metabolism prime translational initiation in the earliest events of mitochondrial biogenesis during Arabidopsis germination. Plant Physiol. 158, 1610–1627. doi: 10.1104/pp.111.192351
Li, G., Zou, W., Jian, L., Qian, J., Deng, Y., and Zhao, J. (2017). Non-SMC elements 1 and 3 are required for early embryo and seedling development in Arabidopsis. J. Exp. Bot. 68, 1039–1054. doi: 10.1093/jxb/erx016
Li, L., Kubiszewski-Jakubiak, S., Radomiljac, J., Wang, Y., Law, S. R., Keech, O., et al. (2016). Characterization of a novel β-barrel protein (AtOM47) from the mitochondrial outer membrane of Arabidopsis thaliana. J. Exp. Bot. 67, 6061–6075. doi: 10.1093/jxb/erw366
Lister, R., Carrie, C., Duncan, O., Ho, L. H., Howell, K. A., Murcha, M. W., et al. (2007). Functional definition of outer membrane proteins involved in preprotein import into mitochondria. Plant Cell 19, 3739–3759. doi: 10.1105/tpc.107.050534
Lithgow, T., and Schneider, A. (2010). Evolution of macromolecular import pathways in mitochondria, hydrogenosomes and mitosomes. Philos. Trans. R. Soc. Lond. B Biol. Sci. 365, 799–817. doi: 10.1098/rstb.2009.0167
Ma, H., and Zhao, J. (2010). Genome-wide identification, classification, and expression analysis of the arabinogalactan protein gene family in rice (Oryza sativa L.). J Exp Bot. 61, 2647–2668. doi: 10.1093/jxb/erq104
Macasev, D., Newbigin, E., Whelan, J., and Lithgow, T. (2000). How do plant mitochondria avoid importing chloroplast proteins? Components of the import apparatus Tom20 and Tom22 from Arabidopsis differ from their fungal counterparts. Plant Physiol. 123, 811–816. doi: 10.1104/pp.123.3.811
Mansilla, N., Garcia, L., Gonzalez, D. H., and Welchen, E. (2015). AtCOX10, a protein involved in haem o synthesis during cytochrome c oxidase biogenesis, is essential for plant embryogenesis and modulates the progression of senescence. J. Exp. Bot. 66, 6761–6775. doi: 10.1093/jxb/erv381
Martin, M. V., Distéfano, A. M., Bellido, A., Córdoba, J. P., Soto, D., Pagnussat, G. C., et al. (2014). Role of mitochondria during female gametophyte development and fertilization in A. thaliana. Mitochondrion 19(Pt B), 350–356. doi: 10.1016/j.mito.2014.01.005
Meinke, D., Muralla, R., Sweeney, C., and Dickerman, A. (2008). Identifying essential genes in Arabidopsis thaliana. Trends Plant Sci. 13, 483–491. doi: 10.1016/j.tplants.2008.06.003
Millar, A. H., Small, I. D., Day, D. A., and Whelan, J. (2008). Mitochondrial biogenesis and function in Arabidopsis. Arabidopsis Book 6:e0111. doi: 10.1199/tab.0111
Murcha, M. W., Wang, Y., Narsai, R., and Whelan, J. (2014). The plant mitochondrial protein import apparatus - the differences make it interesting. Biochim. Biophys. Acta 1840, 1233–1245. doi: 10.1016/j.bbagen.2013.09.026
Nagata, N. (2010). Mechanisms for independent cytoplasmic inheritance of mitochondria and plastids in angiosperms. J. Plant Res. 123, 193–199. doi: 10.1007/s10265-009-0293-x
Palovaara, J., Saiga, S., Wendrich, J. R., van ’t Wout Hofland, N., van Schayck, J. P., Hater, F., et al. (2017). Transcriptome dynamics revealed by a gene expression atlas of the early Arabidopsis embryo. Nat. Plants 3, 894–904. doi: 10.1038/s41477-017-0035-3
Parvin, N., Carrie, C., Pabst, I., Läßer, A., Laha, D., Paul, M. V., et al. (2017). TOM9.2 Is a Calmodulin-Binding Protein Critical for TOM complex assembly but not for mitochondrial protein import in Arabidopsis thaliana. Mol. Plant 10, 575–589. doi: 10.1016/j.molp.2016.12.012
Perry, A. J., Rimmer, K. A., Mertens, H. D., Waller, R. F., Mulhern, T. D., Lithgow, T., et al. (2008). Structure, topology and function of the translocase of the outer membrane of mitochondria. Plant Physiol. Biochem. 46, 265–274. doi: 10.1016/j.plaphy.2007.12.012
Ren, X., Pan, Z., Zhao, H., Zhao, J., Cai, M., Li, J., et al. (2017). EMPTY PERICARP11 serves as a factor for splicing of mitochondrial nad1 intron and is required to ensure proper seed development in maize. J. Exp. Bot. 68, 4571–4581. doi: 10.1093/jxb/erx212
Schmittgen, T. D., and Livak, K. J. (2008). Analyzing real-time PCR data by the comparative C(T) method. Nat. Protoc. 3, 1101–1108. doi: 10.1038/nprot.2008.73
Selles, B., Michaud, C., Xiong, T. C., Leblanc, O., and Ingouff, M. (2018). Arabidopsis pollen tube germination and growth depend on the mitochondrial calcium uniporter complex. New Phytol. 19, 58–65. doi: 10.1111/nph.15189
Song, L., and Liu, D. (2015). Mutations in the three Arabidopsis genes that encode the E2 subunit of the mitochondrial pyruvate dehydrogenase complex differentially affect enzymatic activity and plant growth. Plant Cell Rep. 34, 1919–1926. doi: 10.1007/s00299-015-1838-1
Sosso, D., Mbelo, S., Vernoud, V., Gendrot, G., Dedieu, A., Chambrier, P., et al. (2012). PPR2263, a DYW-Subgroup Pentatricopeptide repeat protein, is required for mitochondrial nad5 and cob transcript editing, mitochondrion biogenesis, and maize growth. Plant Cell 24, 676–691. doi: 10.1105/tpc.111.091074
Sprunck, S., and Dresselhaus, T. (2015). Three cell fusions during double fertilization. Cell 161, 708–709. doi: 10.1016/j.cell.2015.04.032
Tamura, K., Dudley, J., Nei, M., and Kumar, S. (2007). MEGA4: molecular evolutionary genetics analysis (MEGA) software version 4.0. Mol. Biol. Evol. 24, 1596–1599. doi: 10.1093/molbev/msm092
ten Hove, C. A., Lu, K. J., and Weijers, D. (2015). Building a plant: cell fate specification in the early Arabidopsis embryo. Development 142, 420–430. doi: 10.1242/dev.111500
Thornton, N., Stroud, D. A., Milenkovic, D., Guiard, B., Pfanner, N., and Becker, T. (2010). Two modular forms of the mitochondrial sorting and assembly machinery are involved in biogenesis of alpha-helical outer membrane proteins. J. Mol. Biol. 396, 540–549. doi: 10.1016/j.jmb.2009.12.026
Tolleter, D., Jaquinod, M., Mangavel, C., Passirani, C., Saulnier, P., Manon, S., et al. (2007). Structure and function of a mitochondrial late embryogenesis abundant protein are revealed by desiccation. Plant Cell 19, 1580–1589. doi: 10.1105/tpc.107.050104
Tzafrir, I., Pena-Muralla, R., Dickerman, A., Berg, M., Rogers, R., Hutchens, S., et al. (2004). Identification of genes required for embryo development in Arabidopsis. Plant Physiol. 135, 1206–1220. doi: 10.1104/pp.104.045179
Vestweber, D., Brunner, J., Baker, A., and Schatz, G. (1989). A 42K outer-membrane protein is a component of the yeast mitochondrial protein import site. Nature 21 341, 205–209. doi: 10.1038/341205a0
Wang, G., Zhong, M., Shuai, B., Song, J., Zhang, J., Han, L., et al. (2017). E+ subgroup PPR protein defective kernel 36 is required for multiple mitochondrial transcripts editing and seed development in maize and Arabidopsis. New Phytol. 214, 1563–1578. doi: 10.1111/nph.14507
Wang, Y., Law, S. R., Ivanova, A., van Aken, O., Kubiszewski-Jakubiak, S., and Uggalla, V. (2014). The mitochondrial protein import component, TRANSLOCASE OF THE INNER MEMBRANE17-1, plays a role in defining the timing of germination in Arabidopsis. Plant Physiol. 166, 1420–1435. doi: 10.1104/pp.114.245928
Werhahn, W., Niemeyer, A., Jänsch, L., Kruft, V., Schmitz, U. K., and Braun, H. (2001). Purification and characterization of the preprotein translocase of the outer mitochondrial membrane from Arabidopsis. Identification of multiple forms of TOM20. Plant Physiol. 125, 943–954. doi: 10.1104/pp.125.2.943
Xiang, D., Venglat, P., Tibiche, C., Yang, H., Risseeuw, E., Cao, Y., et al. (2011). Genome-wide analysis reveals gene expression and metabolic network dynamics during embryo development in Arabidopsis. Plant Physiol. 156, 346–356. doi: 10.1104/pp.110.171702
Xiao, H., Zhang, Q., Qin, X., Xu, Y., Ni, C., Huang, J., et al. (2018). Rice PPS1 encodes a DYW motif-containing pentatricopeptide repeat protein required for five consecutive RNA-editing sites of nad3 in mitochondria. New Phytol. 220, 878–892. doi: 10.1111/nph.15347
Xin, H. P., Zhao, J., and Sun, M. X. (2012). The maternal-to-zygotic transition in higher plants. J. Integr. Plant Biol. 54, 610–615. doi: 10.1111/j.1744-7909.2012.01138.x
Yamaoka, S., Nakajima, M., Fujimoto, M., and Tsutsumi, N. (2011). MIRO1 influences the morphology and intracellular distribution of mitochondria during embryonic cell division in Arabidopsis. Plant Cell Rep. 30, 239–244. doi: 10.1007/s00299-010-0926-5
Yoshida, S., Barbier, de Reuille, P., Lane, B., Bassel, G. W., Prusinkiewicz, P., et al. (2014). Genetic control of plant development by overriding a geometric division rule. Dev. Cell 29, 75–87. doi: 10.1016/j.devcel.2014.02.002
Keywords: Arabidopsis, mitochondria biogenesis, translocase of the outer mitochondrial membrane 40 (TOM40), embryo development, pattern formation
Citation: Hu Y, Zou W, Wang Z, Zhang Y, Hu Y, Qian J, Wu X, Ren Y and Zhao J (2019) Translocase of the Outer Mitochondrial Membrane 40 Is Required for Mitochondrial Biogenesis and Embryo Development in Arabidopsis. Front. Plant Sci. 10:389. doi: 10.3389/fpls.2019.00389
Received: 25 January 2019; Accepted: 13 March 2019;
Published: 02 April 2019.
Edited by:
Mary Byrne, The University of Sydney, AustraliaReviewed by:
Eduardo Zabaleta, CONICET, ArgentinaMalgorzata Heidorn-Czarna, University of Wrocław, Poland
Copyright © 2019 Hu, Zou, Wang, Zhang, Hu, Qian, Wu, Ren and Zhao. This is an open-access article distributed under the terms of the Creative Commons Attribution License (CC BY). The use, distribution or reproduction in other forums is permitted, provided the original author(s) and the copyright owner(s) are credited and that the original publication in this journal is cited, in accordance with accepted academic practice. No use, distribution or reproduction is permitted which does not comply with these terms.
*Correspondence: Jie Zhao, anpoYW9Ad2h1LmVkdS5jbg==