- 1Southern Regional Collaborative Innovation Centre for Grain and Oil Crops in China, College of Resources and Environmental Sciences, Hunan Agricultural University, Changsha, China
- 2National Centre of Oilseed Crops Improvement, Hunan Branch, Changsha, China
Abiotic stress induces nitrate (NO3-) allocation to roots, which increases stress tolerance in plants. NRT1.1 is broadly involved in abiotic stress tolerance in plants, but the relationship between NRT1.1 and NO3- allocation under stress conditions is unclear. In this study, we found that Arabidopsis wild-type Col-0 was more cadmium (Cd2+)-tolerant than the nrt1.1 mutant at 20 μM CdCl2. Cd2+ exposure repressed NRT1.5 but upregulated NRT1.8 in roots of Col-0 plants, resulting in increased NO3- allocation to roots and higher [NO3-] root-to-shoot (R:S) ratios. Interestingly, NITRATE REGULATORY GENE2 (NRG2) was upregulated by Cd2+ stress in Col-0 but not in nrt1.1. Under Cd2+ stress, nrg2 and nrg2-3chl1-13 mutants exhibited similar phenotypes and NO3- allocation patterns as observed in the nrt1.1 mutant, but overexpression of NRG2 in Col-0 and nrt1.1 increased the [NO3-] R:S ratio and restored Cd2+ stress tolerance. Our results indicated that NRT1.1 and NRG2 regulated Cd2+ stress-induced NO3- allocation to roots and that NRG2 functioned downstream of NRT1.1. Cd2+ uptake did not differ between Col-0 and nrt1.1, but Cd2+ allocation to roots was higher in Col-0 than in nrt1.1. Stressed Col-0 plants increased Cd2+ and NO3- allocation to root vacuoles, which reduced their cytosolic allocation and transport to the shoots. Our results suggest that NRT1.1 regulates NO3- allocation to roots by coordinating Cd2+ accumulation in root vacuoles, which facilitates Cd2+ detoxification.
Introduction
Heavy metal pollution in soil is an important environmental issue worldwide, which gives rise to agricultural and public health concerns (Bertin and Averbeck, 2006; Mohammed et al., 2011; Åkesson et al., 2014). In China, for example, approximately 7% of the soil is cadmium (Cd) contaminated, 0.5% of which is severely polluted (Zhang et al., 2015). Cd can be released to the soil by excessive use of chemical fertilizers and pesticides, utilization of industrial wastewater and sludge, and atmospheric deposition (Woodis et al., 1977; He and Singh, 1994; Wong et al., 2003; Ottosen et al., 2007; Roberts, 2014). Thus, people are being exposed to Cd-associated toxicity via the consumption of cereals and vegetables grown in Cd-contaminated soils (Chaney, 2015). Susceptibility to Cd2+ stress is species-specific in plants (Chen, 1996), which provides opportunities to select and breed Cd2+-tolerant species/varieties. However, this requires a complete understanding of the underlying mechanisms of Cd2+ tolerance in plants.
Nitrate (NO3-) is one of the two forms of inorganic nitrogen nutrient taken up by terrestrial plants. It also acts as a signal molecule regulating a wide range of genes and biological processes involved in nitrogen utilization, general plant lateral root development, and response to environmental fluctuations (Gowri et al., 1992; Redinbaugh and Campbell, 1993; Gutiérrez et al., 2007; Hirel et al., 2007; Miller et al., 2007; Krouk et al., 2010; Wang et al., 2012; Ruffel et al., 2014; Vidal et al., 2014; Bouguyon et al., 2016). Under normal conditions, most of the absorbed NO3- is transported to the shoots for reduction by NR or as a temporary nitrogen pool stored in vacuoles, which is driven by the H+ transport energized by the tonoplast H+-ATPase and H+-PPase (Martinoia et al., 1981, 2007; Han et al., 2016). NO3- allocation between the roots and the shoots in plants is important for nitrogen utilization and adaptation to abiotic stresses (Fan et al., 2007; Li et al., 2010; Han et al., 2016). The long-distance transport of NO3- is mediated by two nitrate transporters, NRT1.5 and NRT1.8. The former is found in pericycle cells where it is responsible for loading the NO3- to the xylem, whereas the latter is found in xylem parenchyma cells, where it contributes to NO3- unloading from xylem (Lin et al., 2008; Li et al., 2010; Chen et al., 2012). Under adverse environmental conditions, NRT1.5 expression is downregulated and NRT1.8 expression is upregulated in roots. As a result, more NO3- allocates to roots, which subsequently increases plant stress tolerance (Li et al., 2010; Chen et al., 2012). This phenomenon, known as SINAR, has been widely observed under several abiotic stresses, including the Cd2+ stress (Chen et al., 2012; Zhang et al., 2014). Ethylene and jasmonic acid are involved in the regulation of NRT1.5 and NRT1.8 under stress (Zhang et al., 2014).
NRT1.1, which was first cloned in 1993 (Tsay et al., 1993), is essential for NO3- uptake and signaling (Ho et al., 2009). In addition to its function as NO3- transporter, NRT1.1 also plays important roles in vegetative and reproductive growth (Guo et al., 2001), stomatal opening (Guo et al., 2003), root architecture (Remans et al., 2006; Mounier et al., 2014), and transport of chloride and phytohormones (IAA/ABA/jasmonic acid/GAs) (Tsay et al., 1993; Guo et al., 2002; Krouk, 2016; Corratgé-Faillie and Lacombe, 2017). Moreover, it induces tolerance in plants to abiotic stresses, such as proton stress, salt stress, Cd2+ stress, and iron deficiency (Mao et al., 2014; Liu et al., 2015; Abouelsaad et al., 2016; Fang et al., 2016). NRT1.1-mediated plant stress tolerance is closely associated with NO3- uptake, assimilation, and accumulation. It has been reported that NRT1.1 mediates the expression of NO3- regulatory genes such as NRG2 (Ho et al., 2009; Hu et al., 2009; Xu et al., 2016) and NO3- assimilation genes such as NIAs and NiR, as well as some other NRTs (Ho et al., 2009; Undurraga et al., 2017). However, the relationship between NRT1.1 and SINAR, including the control mechanisms, is not well understood.
In this study, we found that NRT1.1 regulated the expression of NRT1.5 and NRT1.8 under Cd2+ stress, which increased NO3- allocation to roots as a mechanism to resist Cd2+ stress. Furthermore, we demonstrated that NRG2 functioned downstream of NRT1.1 in regulating NO3- allocation. NO3- was required to facilitate Cd2+ allocation to the roots, where it was mainly stored in the vacuoles for detoxification. Our results provide insights into the effects of the nitrate regulatory gene network on the regulation of plant stress tolerance.
Materials and Methods
Plant Materials and Growth Conditions
Arabidopsis thaliana wild-type (Col-0), nrt1.1, nrg2 single and double mutants (chl1-1, chl1-5, chl1-13, nrg2-1, nrt2-2, nrg2-3chl1-13), and NRG2 overexpression lines (35S::NRG2/Col-0, 35S::NRG2/chl1-5) were used in this study. Seeds were sown in nutrition soil and placed in a growth chamber (300 μmol photons⋅m-2⋅s-1, 16-h photoperiod, 22°C) to germinate and grow. Ten days after sowing, seedlings with two true leaves were transplanted to 600-ml pots and cultivated hydroponically in nutrient medium. The growth medium contained 1.25 mM KNO3, 0.625 mM KH2PO4, 0.5 mM MgSO4, 0.5 mM Ca(NO3)2, 1.25 μM Fe-EDTA, 17.5 μM H3BO3, 3.5 μM MnCl2, 0.25 μM ZnSO4, 0.05 μM NaMoO4, and 0.125 μM CuSO4. The MES buffer (2.5 mM) was used to maintain the pH of the growth medium at pH 5.8. The growth medium was refreshed every 4 days, and the position of the pots was interchanged when refreshing the solution to eliminate any edge effects. Four weeks after sowing, plants were exposed to Cd stress by adding 20 μM CdCl2 to the growth medium for 3 days. Control plants were grown without CdCl2.
15N Tracer Assay
Plants of Col-0, chl1-1, chl1-5 were grown in the normal nutrient medium for 4 weeks, followed by a 12-h treatment with 200 μM CdCl2. Then, roots were washed with 0.1 mM CaSO4 for 1 min and labeled with 20% atom abundance of Ca(15NO3)2 (pH = 5.8) for 40 min. The roots were washed with 0.1 mM CaSO4 and deionized water. Shoots and roots were sampled separately and oven-dried at 70°C for 48 h. Then the samples were pulverized using a TissuLyser (Tissuelyer-48, Jingxin Co. Ltd., China), and 15N abundance in samples was measured using a continuous-flow isotope ratio mass spectrometer coupled with a carbon-nitrogen elemental analyzer (ANCA-MS; PDZ Europa).
Determination of Photosynthetic Parameters
Photosynthesis of fully expanded rosette leaves was measured between 09:00 and 15:00 with a LI-6400 portable photosynthesis system (Li-Cor Inc., Lincoln, NE, United States). The air temperature in the cuvette was 22°C, the PPED was 200 μmol m-2 s-1, the CO2 concentration (Ca) was 500 μmol mol-1 (controlled with a CO2 mixer), and the VPD was between 1.0 and 1.5 kPa. Before measurement, leaves were placed in the cuvette to adjust for 10 min. Data were recorded after equilibration to a steady state.
Photosynthetic CO2-response curves (A-Ci curves) were measured with a PPFD of 200 μmol m-2 s-1 at nine points of Ca (800, 600, 500, 400, 300, 200, 150, 100, 50 μmol mol-1). Prior to the measurement, leaves were placed in the cuvette to equilibrate for 30 min under a PPFD of 200 μmol m-2 s-1 and Ca of 400 μmol mol-1. Temperature and VPD in the cuvette during measurement were maintained as described above. Data were recorded after equilibration to a steady state. Vc,max, Jmax, and TPU were calculated according to Sharkey et al. (2007). The parameters Kc, Ko, Rd, and Γ∗, which were used to calculate Vc,max, Jmax, and TPU, were estimated as 27.24 Pa, 16.58 Pa, 1 μmol⋅m-2⋅s-1, and 3.74 Pa, respectively.
Determination of Proline, Malondialdehyde (MDA) Concentration, and Superoxide Dismutase (SOD) Activity
Plants were grown in the normal nutrient medium for 4 weeks and then treated with 20 μM CdCl2 for 3 days. Fresh leaves were collected to measure proline and MDA concentrations and SOD activity. Proline concentration was measured using the ninhydrin colorimetry method (Bates et al., 1973; Sharma and Dubey, 2005). Briefly, fresh samples (0.5 g) were homogenized in 5 ml of 3% aqueous sulfosalicylic acid using a mortar and a pestle. Homogenates were centrifuged at 10,000 × g for 10 min at 4°C, and the supernatants were collected and used for proline analysis. In a test tube, 2 ml of supernatant was added to 2 ml of acidic ninhydrin and 2 ml of glacial acetic acid; then, the mixture was placed in a boiling water bath for 15 min. The processed mixture was extracted with 4 ml toluene by thoroughly vigorous stirring. After keeping the tube at room temperature for 30 min, the absorption of the toluene solution was measured spectrophotometrically at 520 nm. A standard curve was created using L-proline.
Malondialdehyde concentration was measured using the thiobarbituric acid method (Kramer et al., 1991). Fresh samples (0.5 g) were homogenized in 5 ml 5% (w/v) trichloroacetic acid (TCA) with a mortar and a pestle and centrifuged at 10,000 × g for 10 min at 4°C. Then, 2 ml supernatant were combined with 2.5 ml TBA reagent [0.6% (w/v) TBA in 10% (w/v) TCA], heated at 100°C for 10 min, cooled, and centrifuged at 4000 × g for 10 min. The concentration of MDA was calculated from the absorbance at 600, 532, and 450 nm.
Superoxide dismutase activity was determined according to the method described by Giannopolitis and Ries (1977). The shoot tissues were thoroughly ground with a mortar and a pestle in liquid nitrogen. Then, the samples were homogenized in 0.1 M phosphate buffer containing 0.1 mM EDTA (pH 7.8) and centrifuged at 13,000 × g and 4°C for 10 min. The supernatants were used for determining the SOD activity. The SOD reaction mixture contained 50 mM phosphate buffer (pH 7.8), 13 mM methionine, 75 μM nitro blue tetrazolium (NBT), 10 μM EDTA-Na2, 2.0 μM riboflavin, and modest volume of extract. Ultrapure water was added to a final volume of 3 ml. The mixtures in the glass test tubes were illuminated for 20 min and the absorbance at 560 nm was measured spectrophotometrically. Identical mixtures that were not illuminated served as blank controls (background).
Determination of Chlorophyll Concentration
Chlorophyll concentration in rosette leaves was determined by extraction with 80% acetone for 24 h at room temperature in the dark (Wellburn and Lichtenthaler, 1984). The absorbance of the extract was measured at 663 and 645 nm to calculate chlorophyll a, b, and total chlorophyll concentrations.
Measurements of Biomass, Nitrate, and Cd2+ Concentration
Four-week-old plants treated with CdCl2 for 3 days were sampled and separated into shoots and roots. The samples were oven-dried at 70°C until the weight remained constant (dry weight).
NO3- was extracted from the samples using deionized water in a boiling water bath for 15 min and determined spectrophotometrically at 410 nm by nitration of salicylic acid (Cataldo et al., 1975).
Four-week-old plants exposed to CdCl2 for 3 days were harvested and washed with 0.1 mM CaCl2 for 1 min, followed by rinsing with deionized water for four times. Shoots and roots were separately collected and oven-dried at 70°C until the weight remained constant (dry weight). Samples were digested thoroughly with HNO3 at 180°C and the Cd2+ concentration was determined with an ICP Mass Spectrometer (NexION 350X, PerkinElmer).
Determination of V-ATPase and V-PPase Activities
Root tissues (0.5 g) of four-week-old plants were used for V-ATPase and V-PPase activities determination according to Krebs et al. (2010) with some modifications. V-ATPase and V-PPase activities in 100 μl microsomal membranes were determined calorimetrically by measuring the release of inorganic phosphate (Pi) after an incubation of 30 min at 37°C. The V-ATPase assay medium contained 25 mM Tris-Hepes (pH 7.6), 3 mM MgSO4⋅7 H2O, 50 mM KCl, 0.5 mM NaN3, 0.1 mM NaVO4⋅12 H2O, and 3 mM ATP-Tris. V-PPase activity was assayed in a reaction medium containing 25 mM Tris-Hepes (pH 7.6), 3 mM MgSO4⋅7 H2O, 50 mM KCl, 0.5 mM NaN3, 0.1 mM NaVO4⋅12 H2O, and 3 mM Na4P2O7.
Isolation of Intact Protoplasts and Vacuoles for Determination of Cd2+ and NO3- Levels
Root tissues (0.3 g) of four-week-old plants were used to isolate intact protoplasts and vacuoles according to Robert et al. (2007). The purified protoplasts were divided into two fractions, one of which was used for the releases of vacuoles according to the method described in Dürr et al. (1975). The purified protoplasts and vacuoles were used for the determination of NO3- and Cd2+ concentrations (Vögeli-Lange and Wagner, 1990; Han et al., 2016). NO3- concentrations in protoplasts and vacuoles were measured by a continuous-flow auto-analyzer (Auto Analyzer 3, Bran and Luebbe) as described previously by Han et al. (2016). Cd2+ concentrations in the protoplasts and vacuole were determined with an ICP Mass Spectrometer (NexION 350X, PerkinElmer) as described in Huang et al. (2012).
RNA Extraction and Transcript Analysis
Four-week-old plants were treated with 200 μM CdCl2 for 6 h and the roots were harvested for total RNA analysis. Total RNA was extracted with TRIzol (Invitrogen, United States), precipitated with an equal volume of isopropanol, washed with 75% ethanol, and dissolved with RNase-free water. The cDNA templates were synthesized using the PrimeScriptTM RT Kit with gDNA Eraser (Perfect Real Time) (TAKARA, Japan) following the manufacturer’s protocol. The relative expression of genes in roots was determined by quantitative RT-PCR performed in an Applied Biosystems StepOneTM Real-Time PCR System using SYBR Premix Ex-Taq (TAKARA) according to the manufacturer’s protocol. Primers used in the assays are listed in Supplementary Table S1. The expression data were normalized to Actin2 or sand.
Statistical Analysis
A completely randomized design was applied in the experiments by having four biological replicates in each treatment. The comparisons of the means between Cd2+ stress treatments and controls were performed using the two-tailed Student’s t-test. The effects of Cd2+ stress treatment, along with the genotypes and their interactions, were evaluated using two-way analysis of variance (ANOVA). Multiple comparisons were performed using the least significant difference (LSD) multiple range test. Differences were considered statistically significant at P < 0.05.
Results
Nrt1.1 Improves Cd2+ Stress Tolerance in Plants
Cd2+ stress degraded chlorophyll a, b, and total chlorophyll in nrt1.1 mutants (chl1-1 and chl1-5) by 16.35–24.87, 4.26–12.10, and 11.07–20.75%, respectively, as compared with those in the controls, resulting in more severe chlorosis in nrt1.1 than in Col-0 (Figures 1A,B and Supplementary Figures S1A–C). Cd2+ stress significantly reduced shoot biomass of nrt1.1, but had no effect on Col-0; Cd2+ exposure did not affect the root biomass in any genotype (Figure 1C). Pn, Vc,max, Jmax, and TPU in nrt1.1 were strongly decreased under Cd2+ stress, with significantly lower values than those in Col-0. Photosynthesis in Col-0 was not affected by Cd2+ stress (Supplementary Figures S2A–D). The chl1-9 mutant is defective in nitrate uptake but shows a normal primary nitrate response (Ho et al., 2009). In the presence of Cd2+, chl1-9 had chlorosis similar to chl1-1 and chl1-5 (Supplementary Figure S3), indicating that the nitrate signaling function of NRT1.1 is independent of the underlying mechanism of Cd2+ stress tolerance in Arabidopsis.
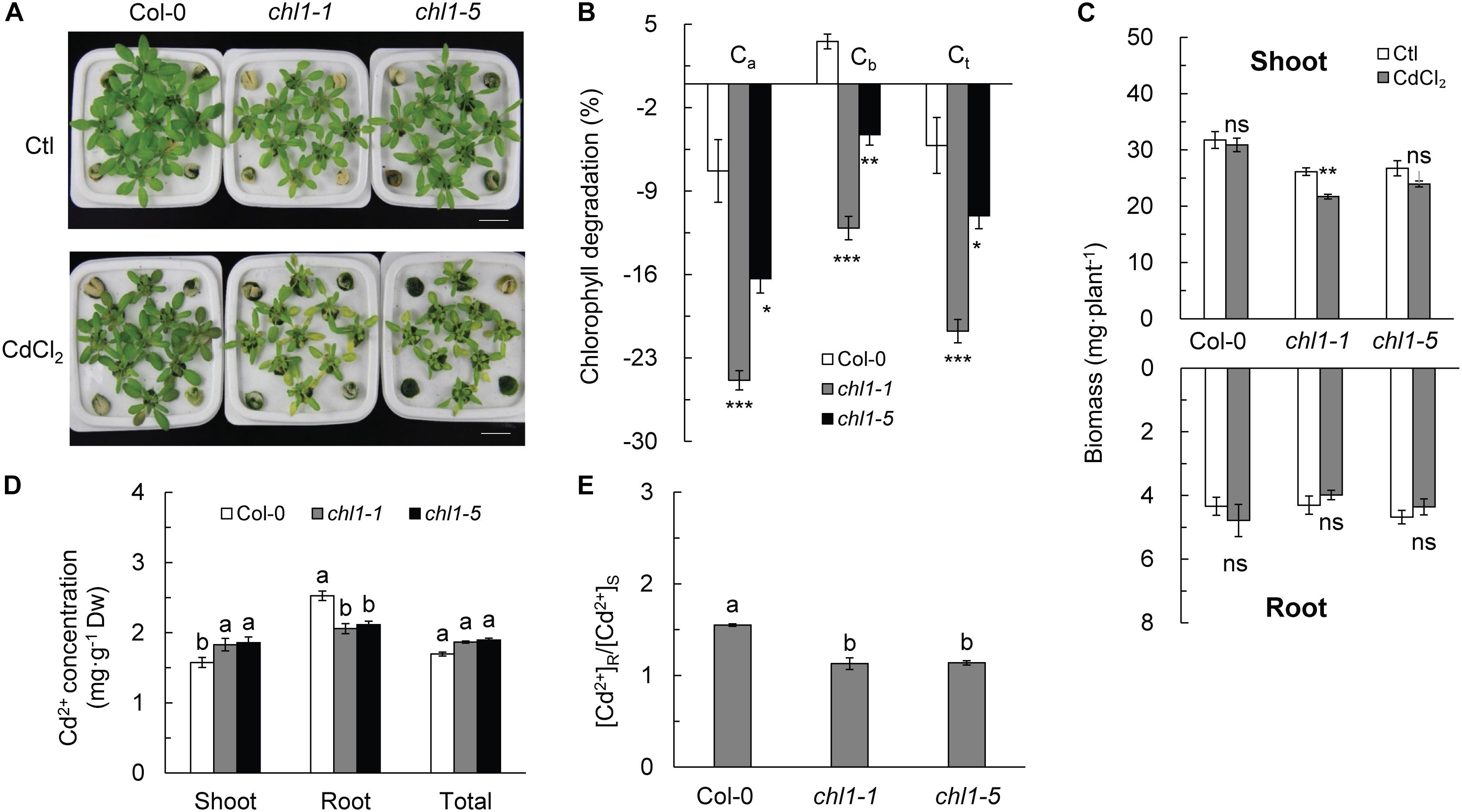
Figure 1. Effects of Cd2+ stress on growth and Cd2+ distribution in Col-0 and nrt1.1 plants. (A) Images of four-week-old Col-0 and the nrt1.1 plants, which were maintained in the absence (Ctl, control) or presence of 20 μM CdCl2 for 3 days. (B) Chlorophyll degradation rate (%) in CdCl2 treated plants relative to untreated control plants. (C) Shoot and root biomasses in Col-0 and nrt1.1. (D) Cd2+ concentration in Col-0 and nrt1.1 plants maintained without or with 20 μM CdCl2 for 3 days. (E) [Cd2+] R:S ratio in Col-0 and nrt1.1 plants. Data represent means ± SE (n = 4). Columns with the same letter indicate no significant difference at P < 0.05 using the LSD method. Scale bars = 1 cm. Bars with one (∗), two (∗∗), and three (∗∗∗) asterisks indicate significant differences from the control at P < 0.05, P < 0.01, and P < 0.001, respectively, using the two-tailed Student’s t-test.
Cd2+ stress strongly increased the MDA concentration in nrt1.1, especially in the roots, where it increased by 310.4% in chl1-1 and 481.4% in chl1-5 as compared to that in the corresponding controls. However, the MDA concentration in Col-0 was not affected in the shoots and only increased by 41.8% in the roots (Supplementary Figure S4A). Cd2+ stress significantly increased the proline concentration in all genotypes, with increases of 84.5 and 59.8%, 54.7 and 55.1%, and 35.4 and 43.3% observed in the shoots and roots of Col-0, chl1-1, and chl1-5, respectively, as compared to the proline concentration in the corresponding controls (Supplementary Figure S4B). Cd2+ stress significantly increased the SOD activity in the shoots of Col-0 but reduced it in the nrt1.1 mutant, which had a significantly lower SOD activity than Col-0 (Supplementary Figure S4C).
We compared the concentration and distribution of Cd2+ in plants exposed to Cd2+ stress and found that the Cd2+ concentration was significantly lower in the shoots of Col-0 plants than in those of the nrt1.1 plants. In contrast, the Cd2+ concentration in the roots of Col-0 plants was markedly higher than that of the nrt1.1 plants (Figure 1D). However, the whole-plant Cd2+ concentration did not vary significantly between Col-0 and nrt1.1 (Figure 1D). Therefore, the [Cd2+] R:S ratio was significantly higher in Col-0 than in nrt1.1 (Figure 1E). These results indicated that Col-0 plants allocated more Cd2+ to the roots during Cd2+ stress, whereas nrt1.1 plants distributed more Cd2+ to shoots, which was consistent with the observed Cd2+ toxicity in the shoots.
Since the iron (Fe) status of plants is critical for Cd2+ uptake and tolerance (He et al., 2017), we measured the Fe concentration but did not detect any genotype-dependent differences, neither under control nor Cd2+ stress conditions (Supplementary Figure S5). This observation suggested that the chlorosis in nrt1.1 leaves under Cd2+ stress did not affect the Fe status.
NRT1.1 Mediates Nitrate Allocation in Roots Under Cd2+ Stress
NRT1.1 is a nitrate transporter. To determine whether the phenotype of Cd2+ stress-induced chlorosis is nitrate-dependent, we used ammonium succinate as the sole nitrogen source during Cd2+ treatment and found that the phenotype of Cd2+ toxicity disappeared (Supplementary Figure S6). The results suggested that NRT1.1-mediated Cd2+ tolerance is nitrate-dependent.
A previous study reported that nitrate allocation in plants is correlated with Cd2+ stress tolerance (Li et al., 2010). Thus, we measured the NO3- concentration and allocation in roots and shoots. The results showed that Cd2+ stress significantly reduced the NO3- concentration in roots and shoots of nrt1.1, whereas the NO3- concentration in roots and shoots of Col-0 was significantly increased by 2.43-fold and 0.13-fold as compared with those in control plants, respectively (Figure 2A). As a result, the [NO3-] R:S ratio in Col-0 was significantly increased by Cd2+ treatment, whereas this ratio remained constant in nrt1.1 at approximately 0.26 (Figure 2B). The short-term 15N trace experiment further confirmed that allocation of absorbed nitrate to the roots was higher in Col-0 (14.00%) than in chl1-1 (9.66%) or chl1-5 (8.25%) under Cd2+ stress (Figures 2C,D).
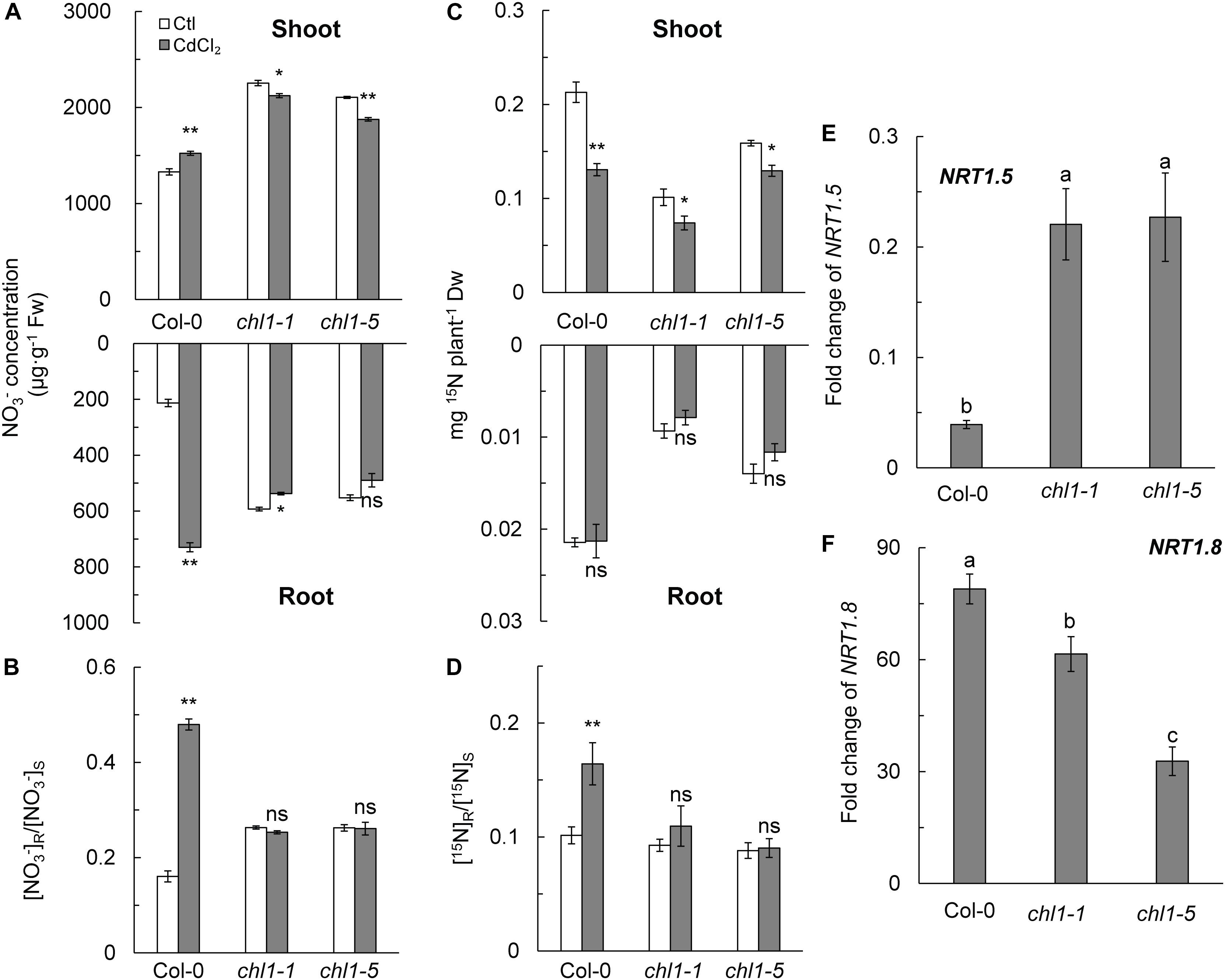
Figure 2. Effects of Cd2+ stress on nitrate allocation and the fold change of NRT1.5 and NRT1.8. (A) NO3- concentration in the shoots and roots of Col-0 and nrt1.1. (B) [NO3-] R:S ratio. (C) 15N levels in the shoots and roots. Four-week-old plants maintained without or with 200 μM CdCl2 for 12 h were labeled with 20% atom abundance of Ca(15NO3)2 for 40 min. (D) [15N] R:S ratio. (E) Fold change of NRT1.5 expression calculated by dividing the expression of NRT1.5 under CdCl2 treatment with the expression of NRT1.5 in the control. (F) Fold change of NRT1.8 expression calculated by dividing the expression of NRT1.8 under CdCl2 treatment with the expression of NRT1.5 in untreated controls. For the gene expression assay, plants were maintained without or with 200 μM CdCl2 for 6 h; roots were harvested for mRNA isolation. Data represent means ± SE (n = 4). Columns with the same letter indicate no significant difference at P < 0.05 using the LSD method. Scale bars = 1 cm. Bars with one (∗) and two (∗∗) asterisks indicate significant differences from the control at P < 0.05, and P < 0.01, respectively, using the two-tailed Student’s t-test.
The expression of nitrate long-distance transport genes NRT1.5 and NRT1.8 were also assayed in this study. As shown in Figure 2E and Supplementary Figure S7A, NRT1.5 expression was strongly inhibited in Col-0 roots during Cd2+ exposure, whereas its expression was only slightly downregulated in nrt1.1 mutants exposed to the same stress. In contrast, Cd2+ stress strongly upregulated the expression of NRT1.8 in roots of all genotypes, with the highest expression levels measured in Col-0 (Figure 2F and Supplementary Figure S7B). The expression of NRT1.5 and NRT1.8 resulted in a higher [NO3-] R:S ratio under Cd2+ stress.
NRG2 Participates in NRT1.1-Mediated Nitrate Allocation
Surprisingly, NRG2 expression in Col-0 roots was also significantly upregulated by Cd2+ stress, whereas the expression of NRG2 in chl1-1 and chl1-5 was not significantly affected (Figure 3A). Therefore, we hypothesized that NRG2 is involved in NRT1.1-mediated Cd2+ tolerance in Arabidopsis. Interestingly, we observed the Cd2+ toxicity phenotype in two NRG2 single mutants, nrg2-1 and nrg2-2, after a 3-day treatment with 20 μM CdCl2 (Figure 3B).
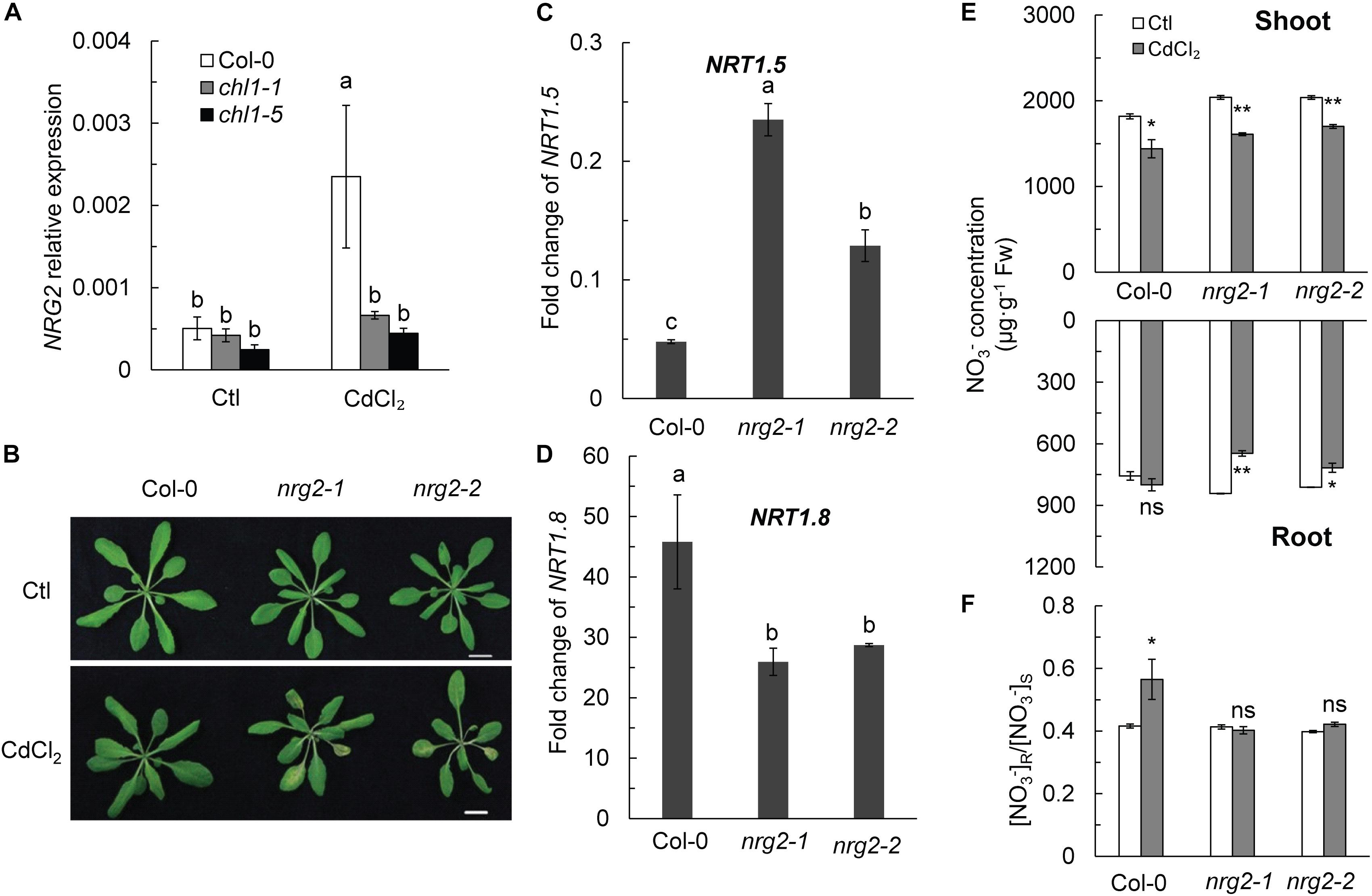
Figure 3. Phenotypes, NO3- concentration, [NO3-] R:S ratio, and gene expression change in Col-0 and nrg2. (A) NRG2 relative expression in Col-0 and nrt1.1 in the absence or presence of Cd2+. (B) Images of four-week-old Col-0 and nrg2 plants grown without or with 20 μM CdCl2 for 3 days. (C) Fold change of NRT1.5 expression calculated as the expression of NRT1.5 under CdCl2 treatment divided by the expression of NRT1.5 in the untreated control. (D) Fold change of NRT1.8 expression calculated as the expression of NRT1.8 under CdCl2 treatment divided by the expression of NRT1.5 in the untreated control. For the gene expression assay, plants were maintained without or with 200 μM CdCl2 for 6 h, roots were harvested for mRNA determination. (E) NO3- concentration in shoot and roots of Col-0 and nrg2 mutants. (F) [NO3-] R:S ratio. Data represent means ± SE (n = 4). Columns with the same letter indicate no significant difference at P < 0.05 using the LSD method. Scale bars = 1 cm. Bars with one (∗) and two (∗∗) asterisks indicate significant differences from the control at P < 0.05, and P < 0.01 respectively, using the two-tailed Student’s t-test.
The expression profile of NRT1.5 and NRT1.8 in nrg2 mutants was similar to that in chl1-1 and chl1-5. NRT1.5 was more significantly downregulated in Col-0 than in nrg2, and NRT1.8 was also more markedly upregulated in Col-0 than in the mutant (Figures 3C,D and Supplementary Figures S7C,D). Accordingly, Col-0 allocated more NO3- to the roots and had an increased [NO3-] R:S ratio under Cd2+ stress (Figures 3E,F). In contrast, the NO3- concentration was lower in ngr2 shoots and roots than in control plant shoots and roots; thus, the ngr2 mutant maintained a constant [NO3-] R:S ratio during the Cd2+ exposure (Figures 3E,F). These results indicated that the Cd2+ stress response in plants with depleted NRG2 gene was similar to that in the nrt1.1 mutant.
An nrg2-3chl1-13 double mutant line was also used in this study. By exposing the double mutant plants to 20 μM Cd2+ for 3 days, we observed a Cd2+ toxicity phenotype that was similar to that of the chl1-13 single mutant (Figure 4A). In the presence of Cd2+, the nrg2-3chl1-13 mutant had an increased NO3- concentration in the shoots and a decreased NO3- concentration in the roots, which lowered the [NO3-] R:S ratio under Cd2+ stress (Figures 4B,C). The results indicated that NRG2 and NRT1.1 are involved in the same pathway regulating the expression of NRT1.5 and NRT1.8, and, consequently, controlling NO3- allocation and Cd2+ stress tolerance.
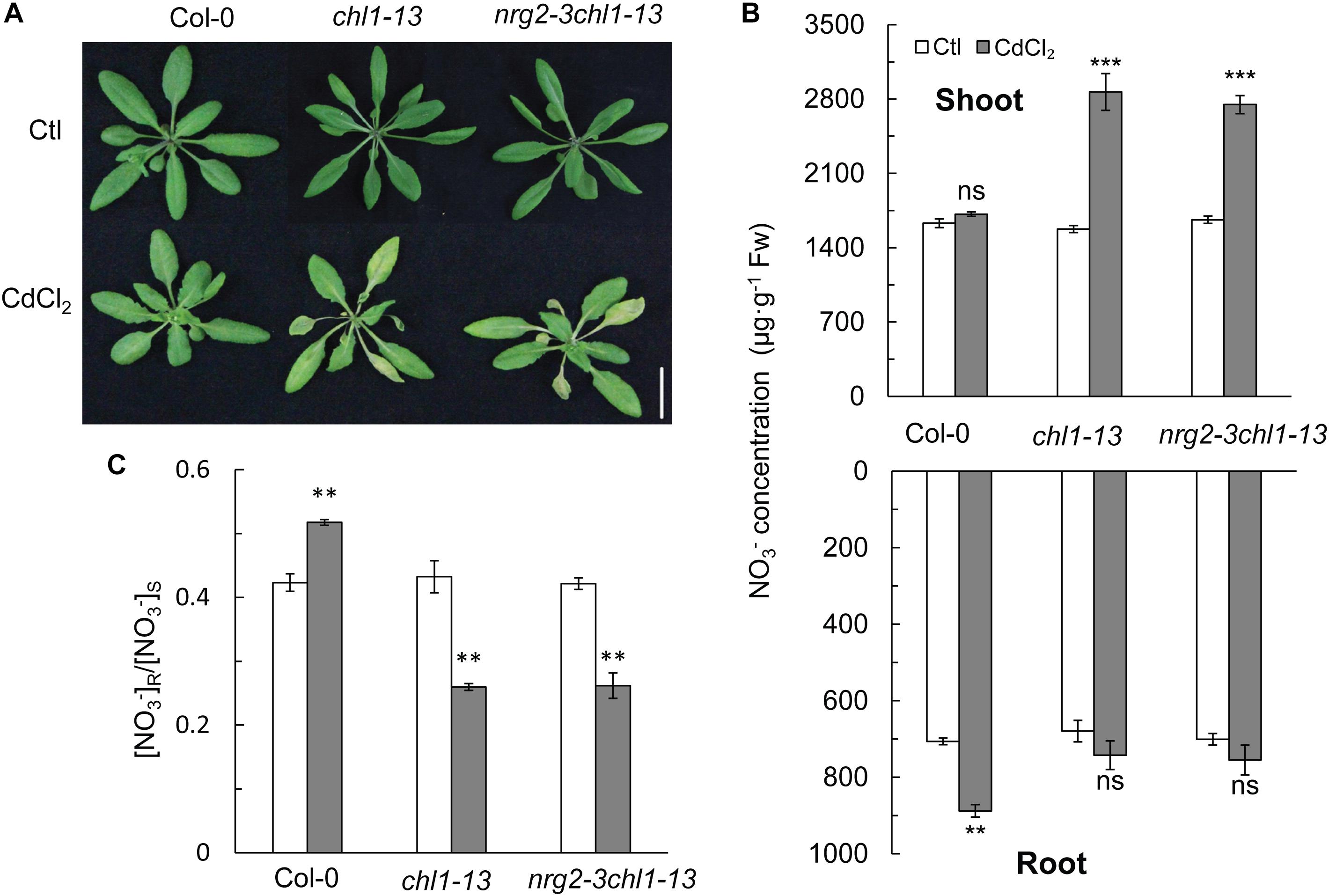
Figure 4. Phenotypes, NO3- concentration, and [NO3-] R:S ratio in Col-0, chl1-13, and nrg2chl1-13 mutants. (A) Images of phenotypes of Col-0, chl1-13, and nrg2-3chl1-13 grown without or with 20 μM CdCl2 for 3 days. (B) NO3-concentration in Col-0, chl1-13, and nrg2chl1-13 in shoots and roots. (C) [NO3-] R:S ratio of Col-0, chl1-13, and nrg2chl1-13. Data represent means ± SE (n = 4). Scale bars = 1 cm. Bars with two (∗∗) and three (∗∗∗) asterisks indicate significant differences from the control at P < 0.01, and P < 0.001, respectively, using the two-tailed Student’s t-test.
To further elucidate the relationship between NRG2 and NRT1.1 in regulating NO3- allocation as a major response to Cd2+ stress, we constitutively overexpressed NRG2 in Col-0 and chl1-5 under the control of the 35S promoter. We found that Cd2+ stress did not induce the Cd2+ toxicity phenotype in 35S::NRG2/Col-0 or 35S::NRG2/chl1-5, both of which with an NRG2 expression that was significantly higher than that in Col-0 (Figures 5A,B). Overexpression of NRG2 in Col-0 and chl1-5 reduced the NO3- concentration in shoots but increased (or maintained) the NO3- concentration in roots under Cd2+ stress (Figure 5C). Hence, the [NO3-] R:S ratio under Cd2+ stress in 35S::NRG2/Col-0 and 35S::NRG2/chl1-5 was significantly increased as compared to that in Col-0 (Figure 5D). These data indicated that NRG2 acted as a downstream element of NRT1.1 to regulate NO3- allocation under Cd2+ stress.
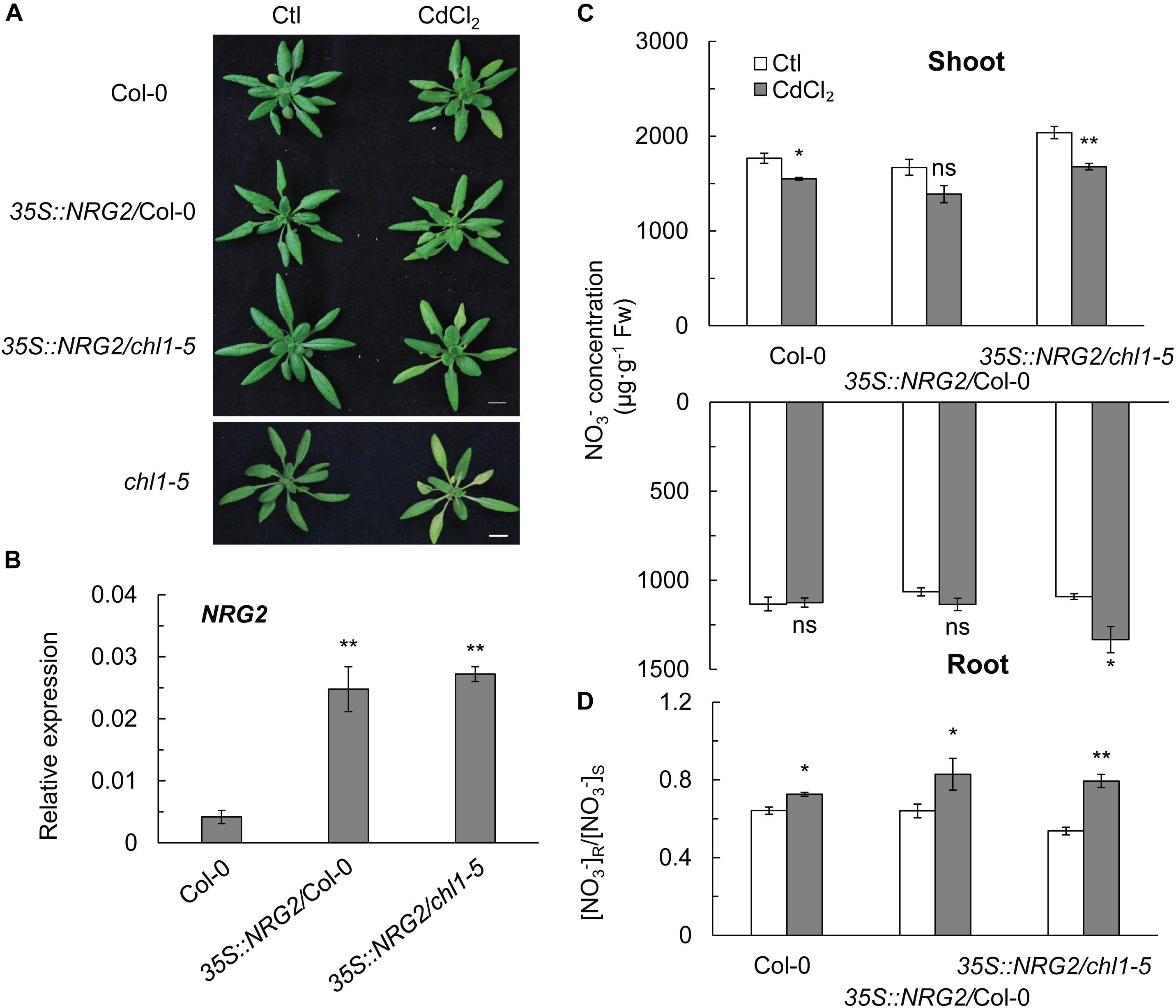
Figure 5. Phenotypes, NO3- concentration, and [NO3-] R:S ratio in Col-0, chl1-5, and NRG2 overexpression lines. Four-week-old plants were grown without or with 20 μM CdCl2 for 3 days, and shoots and roots were harvested for nitrate determination. (A) Phenotypes of Col-0, 35S::NRG2/Col-0, 35S::NRG2/chl1-5, and chl1-5 in maintained in the absence or presence of 20 μM CdCl2. (B) NRG2 relative expression in Col-0 and NRG2 overexpression plants. (C) NO3- concentration of Col-0, 35S::NRG2/Col-0, and 35S::NRG2/chl1-5 in shoots and roots. (D) [NO3-] R:S ratio in Col-0 and NRG2 overexpression plants. Scale bars = 1 cm. Data represent means ± SE (n = 4). Bars with one (∗) and two (∗∗) asterisks indicate significant differences from the control at P < 0.05, and P < 0.01, respectively, using the two-tailed Student’s t-test.
Coordination of Cd2+ and NO3- Allocation in Root Tissues
Cd2+ stress triggered both Cd2+ and NO3- allocation in roots of Col-0 plants (Figures 1D,E, 2A,B, 3E,F). Previous studies showed that the cellular vacuoles function in nitrogen storage and heavy metal sequestration (Andreev, 2001; Sharma et al., 2016). In our study, we measured the distribution of Cd2+ and NO3- in the vacuole and protoplast, along with the proton pump activity of the tonoplast, which promotes ion accumulation in the vacuole.
As shown in Figure 6, the activities of the V-ATPase and V-PPase in roots tissues of Col-0 were increased under Cd2+ stress, whereas the activities of those two enzymes were significantly decreased in chl1-1 and chl1-5 roots (Figures 6A,B). Cd2+ stress reduced the NO3- content in both protoplasts and vacuoles, with a greater reduction in the vacuole NO3- content in chl1-1 and chl1-5 mutant plants, resulting in a lower proportion of vacuole NO3- as compared to the total NO3- in nrt1.1 protoplasts (Figure 6C). NO3- accumulation in the cytosol (P-V) was significantly increased in nrt1.1 mutant plants and was markedly higher than that in Col-0 plants (Figure 6D).
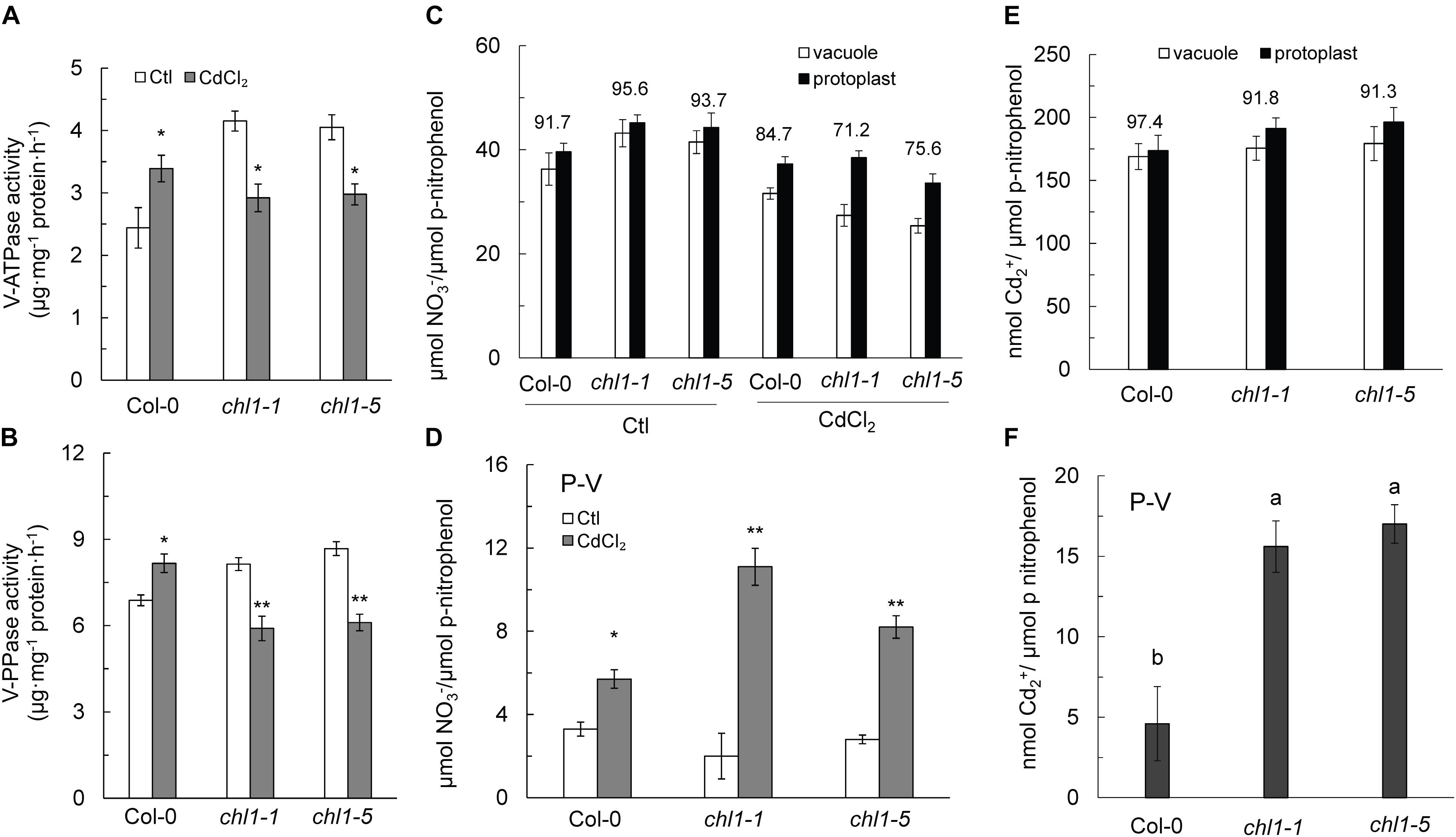
Figure 6. Reduced VSC of NO3- and Cd2+ in the roots of nrt1.1 mutant plants maintained without or with 20 μM CdCl2. (A) V-ATPase and (B) V-PPase (tonoplast proton-pump) activities in root tissue of Col-0 and nrt1.1 mutant. (C) NO3- distribution between the vacuole and protoplast in Col-0 and nrt1.1 root tissue maintained without or with 20 μM CdCl2. (D) Total NO3- in the cytosol under 20 μM CdCl2 treatment, calculated by total NO3- in the protoplast minus total NO3- in vacuoles. (E) Cd2+ distribution between the vacuole and protoplast in Col-0 and nrt1.1 root tissue maintained without or with 20 μM CdCl2 treatment. (F) Total Cd2+ in the cytosol maintained without or with 20 μM CdCl2 treatment, calculated as the difference between total Cd2+ in the protoplast and total Cd2+ in vacuoles. Data represent means ± SE (n = 4). Values above the bars represent the percentage of total vacuolar NO3- or Cd2+ in root tissue divided by total NO3- or Cd2+ in total protoplasts in root tissue. Bars with one (∗) and two (∗∗) asterisks indicate significant differences from the control at P < 0.01, and P < 0.001, respectively, using the two-tailed Student’s t-test. Bars with the same letter indicate no significant difference at P < 0.05 using the LSD method.
Under Cd2+ stress, the proportion of vacuole Cd2+ relative to the total Cd2+ in root protoplasts was lower in nrt1.1 than in Col-0 (Figure 6E), indicating that substantial amounts of Cd2+ accumulated in the cytosol of those nrt1.1 root protoplasts (Figure 6F). Thus, we concluded that the increased allocation of NO3- and Cd2+ to the root vacuoles is an effective strategy for Col-0 to increase the tolerance to Cd2+ stress.
Discussion
NRT1.1 Improves Cd2+ Stress Tolerance by Nitrate Allocation in Roots
We found that Col-0 plants were more tolerant to Cd2+ stress than nrt1-1 and nrt1-5 plants. Interestingly, Col-0 also allocated more nitrate to roots, which was not observed in the nrt1.1 mutant. It is well known that nitrate is reallocated to the roots under abiotic stress, including heavy metal stress (Hernandez et al., 1997). Nitrate reallocation to plant roots has been characterized as a typical mechanism that increases the stress tolerance in plants (Li et al., 2010; Chen et al., 2012). Our results suggested that the difference in nitrate allocation between Col-0 and nrt1.1 is linked to their difference in Cd2+ tolerance. In this study, the data indicated that the enhanced nitrate allocation to the roots is correlated with transcript level changes of NRT1.5 and NRT1.8, which corroborated earlier reports by Li et al. (2010) and Chen et al. (2012) using nrt1.5 and nrt1.8 mutants. Our results, along with the earlier reports, indicated that nitrate plays an essential role in the tolerance to Cd2+ stress via the regulatory function of NRT1.1.
Although nitrate allocation to roots improves stress tolerance in plants, the relevant physiological and molecular mechanisms are not well characterized. Cd2+ induces oxidative stress in plants (Mendoza-Cózatl et al., 2005; Hayat et al., 2007; Cuypers et al., 2010). Our results revealed that nitrate accumulation in roots is correlated with increases in antioxidant capacity, as indicated by less membrane lipid peroxidation, higher proline level, and stronger SOD activity (Supplementary Figures S2A–C), suggesting that the anti-oxidative system protected the plants from Cd2+ damage. As predicted, Col-0 maintained a higher photosynthetic rate (Supplementary Figure S1A). Nitrate reduction is an energy-intensive process (Sunil et al., 2013). Assimilation of nitrate in the leaves directly involves reducing compounds such as NADH, NADPH, reduced ferredoxin, and ATP derived from photosynthesis (Gonzalez-Dugo et al., 2010). Storage of nitrate in the roots can reduce the energy competition between nitrate reduction and photosynthesis, thus, reducing the adverse effect of Cd2+ on photosynthesis.
NRG2 Works Together With NRT1.1 in the Regulation of Nitrate Allocation
NRG2 is an important nitrate regulatory gene that has been reported to regulate nitrate signaling in Arabidopsis in part by modulating NRT1.1 expression (Xu et al., 2016). The nrg2 mutant showed lower nitrate accumulation in the roots than the wild-type due to reduced expression of NRT1.1 and upregulation of NRT1.8 (Xu et al., 2016). However, the relationship between these genes in regulating nitrate allocation remained unknown. Our results showed distinct expression patterns of NRT1.5 and NRT1.8 in Col-0 and the nrt1.1 mutant, indicating that NRT1.1 regulates the expression of NRT1.5 and NRT1.8. Interestingly, we observed a significant upregulation of NRG2 in Col-0 plants when grown under Cd2+ stress (Figure 3A), indicating that NRG2 is involved in the response of nitrate-dependent Cd2+ stress.
By testing the nrg2 single mutant, we observed a Cd2+ stress phenotype, and the expression patterns of NRT1.5 and NRT1.8 were similar to that in the nrt1.1 mutant (Figures 3B–D). The nrg2-3chl1-13 double mutant also had chlorosis similar to the nrt1.1 plants and a reduced [NO3-] R:S ratio under Cd2+ stress (Figures 4A–C). The results indicated that NRG2 and NRT1.1 are involved in the same regulatory pathway for nitrate allocation under Cd2+ stress. Xu et al. (2016) reported that NRG2 functions upstream of NRT1.1 in nitrate signaling. By overexpressing the NRG2 gene in chl1-5 plants, we found that both Cd2+ stress induced chlorosis and nitrate allocation to the roots were restored in 35S::NRG2/chl1-5 plants (Figures 5A–D). The results demonstrated that NRG2 functions downstream of NRT1.1 to regulate Cd2+ stress-induced nitrate allocation. Our results also suggested that NRG2 can cooperate with NRT1.1 in nitrate signaling, depending on the growth conditions.
Cd2+ Coordinates NO3- Allocation to Root Vacuoles to Improve Cd2+ Stress Tolerance in Plant
Previous studies have demonstrated that stress-induced alterations of the expression of NRT1.5 and NRT1.8 depend on the presence of nitrate in the growth medium (Chen et al., 2012). Interestingly, the phenomenon of Cd2+ toxicity disappeared, as was also observed in previous studies, when the plants were supplied with ammonium as the sole nitrogen nutrient (Supplementary Figure S5). It indicated that Cd2+ toxicity is closely correlated with NO3- allocation in plants. This observation was corroborated by studies performed in Arabidopsis, which showed that NO3- and Cd2+ uptake increase simultaneously (Mao et al., 2014). Studies in rice showed that an excess of NO3- increases the uptake and accumulation of Cd2+ (Yang et al., 2016). In this study, however, the uptake of Cd2+ in Col-0 was not different from that in nrt1.1 mutants, but Col-0 plants allocated more Cd2+ to the roots (Figures 1D,E), which was consistent with the NO3- allocation pattern (Figures 2A,B). These results suggest that the presence of NO3- facilitates the allocation of Cd2+ to the roots, which increases the tolerance to Cd2+ stress.
The cellular vacuole plays important roles in maintaining the ion homeostasis in the plant cell and in regulating the responses to several abiotic and biotic stresses (Andreev, 2001). It acts as a storage pool of NO3-, in which the NO3- concentration is an order of magnitude higher than that in the cytoplasm (Martinoia et al., 1981, 2000). Sequestration into the vacuole is one of the key mechanisms of heavy metal detoxification in plants (Sharma et al., 2016). The transfer of NO3- and Cd2+ into the vacuole depends on a set of different transporters (De Angeli et al., 2006; Korenkov et al., 2006, 2007, 2009; Morel et al., 2009; Ueno et al., 2011). Nevertheless, both processes are mediated by V-ATPase and V-PPase energy pumps located on the membrane of tonoplast (Krebs et al., 2010; Sharma et al., 2016). We found that the activities of V-ATPase and V-PPase in Col-0 plants exposed to Cd2+ stress were significantly higher than those in the nrt1.1 mutants (Figures 6A,B), leading to higher Cd2+ and NO3- levels in root vacuoles (Figures 6C,E) and a stronger reduction of cytosolic Cd2+ and NO3- levels in Col-0 plants as compared with those in nrt1.1 plants (Figures 6D,F). Thus, there was less Cd2+ transport from the roots to the shoots, diminishing Cd2+ stress-induced injuries in the leaves. However, further studies are required to elucidate how the distribution of Cd2+ and NO3- between root vacuoles and cytosol is regulated.
Conclusion
Our results indicate that Cd2+ stress-initiated nitrate allocation to roots (SINAR) is associated with the antioxidant system to diminish stress-induced chlorosis. Furthermore, we found that root vacuoles are involved in the coordinated accumulation of Cd2+ and NO3- under Cd2+ stress.
In Col-0 plants, NRG2 acted as a downstream element of NRT1.1 in the regulation of NO3- allocation to the roots by downregulating NRT1.5 and upregulating NRT1.8 under Cd2+ stress. Moreover, a larger proportion of absorbed Cd2+ remained in the roots, where Cd2+ and NO3- were allocated from the cytosol to the vacuole by increasing the tonoplast proton-pump activities (V-ATPase and V-PPase). Thus, intracellular sequestration reduced the transport of Cd2+ from roots to shoot, which is beneficial for Cd2+ detoxification and growth (Figure 7A).
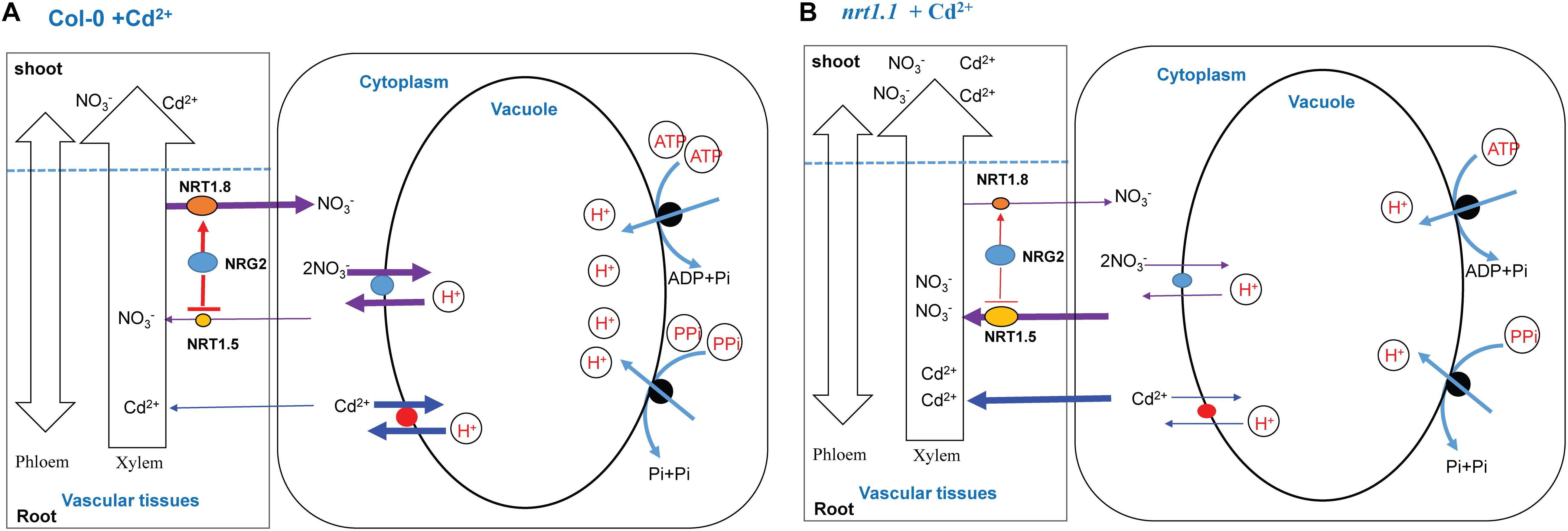
Figure 7. A schematic model of the coordinated regulation by NRT1.1 and NRG2 in plants exposed to Cd2+ stress. The red lines indicate the route of the regulation pathway. The size of arrows represents the flow rate. (A) When Col-0 plants are exposed to Cd2+, NRT1.1 induces NRG2 expression in roots and further downregulates NRT1.5 expression and upregulates NRT1.8 expression. As a result, more nitrate allocates to the roots. In addition, Cd2+ absorbed by the root system remains in the roots, and Cd2+ and NO3- are coordinatively allocated between vacuole and cytosol by enhancing tonoplast proton-pump activities (V-ATPase and V-PPase). Thus, the Cd2+ and NO3- contents are higher in the vacuole than in the cytoplasm, which facilitates Cd2+ detoxification and promotes growth. (B) In the nrt1.1 mutant, NRG2 expression is not changed by Cd2+ stress. The expression profiles of NRT1.5 and NRT1.8 are not affected as significantly as in Col-0 under Cd2+ stress. Hence, a greater proportion of nitrate absorbed by the root system is transported to the aboveground plant parts. Moreover, as compared with the control, the tonoplast proton-pump activities (V-ATPase and V-PPase) in nrt1.1 mutants are lower under Cd2+ stress, resulting in enhanced Cd2+ and NO3- allocation to the cytosol and transport to aboveground plant parts. Ultimately, nrt1.1 plants are damaged under Cd2+ stress.
In the nrt1.1 mutant, NRG2 expression was not changed by Cd2+ stress. Furthermore, the expression patterns of NRT1.5 and NRT1.8 in nrt1.1 were not affected as significantly as those in Col-0 under Cd2+ stress. Hence, a larger proportion of NO3- absorbed by the root system was transported to the aboveground parts. Moreover, as compared to those in the controls, the tonoplast proton-pump activities (V-ATPase and V-PPase) in the nrt1.1 mutant were lower under Cd2+ stress, resulting in enhanced Cd2+ and NO3- allocation to the cytosol and transport to aboveground parts. Ultimately, the nrt1.1 mutant was more seriously injured under Cd2+ stress (Figure 7B). Our findings provide an insight into the underlying mechanism of the network of NRT1.1 and NRG2 in regulating Cd2+ stress tolerance in plants.
Author Contributions
SJ and ZZ conceived the original screening and research plans and supervised the experiments. SJ performed most of the experiments and agreed to serve as the author responsible for contact and ensured communication. JL and QLiao provided technical assistance to SJ. SJ and ZZ designed the experiments and analyzed the data. QLiu and CG interpreted the result. ZZ conceived the project and wrote the article with contributions of all the authors and supervised and completed the writing.
Funding
This study was financially supported in part by the National Key R&D Program of China (2017YFD0200100), National Natural Science Foundation of China (Grant No. 31101596, 31372130), Hunan Provincial Recruitment Program of Foreign Experts, the National Oilseed Rape Production Technology System of China; “2011 Plan” supported by The Chinese Ministry of Education, and Research and Innovation Project of Postgraduates in Hunan Province (CX2015B242).
Conflict of Interest Statement
The authors declare that the research was conducted in the absence of any commercial or financial relationships that could be construed as a potential conflict of interest.
Acknowledgments
We thank Dr. Ji-Ming Gong, Shanghai Institute of Plant Physiology and Ecology, Shanghai Institutes for Biological Sciences, for providing A. thaliana mutant materials (chl1-1, chl1-5), Dr. Yi-Fang Tsay, Institute of Molecular Biology, Academia Sinica, Tai Wan District, for providing the A. thaliana mutant lines chl1-9, and Dr. Yong Wang, Shan Dong Agricultural University, for providing NRG2 mutant lines.
Supplementary Material
The Supplementary Material for this article can be found online at: https://www.frontiersin.org/articles/10.3389/fpls.2019.00384/full#supplementary-material
Abbreviations
Jmax, maximum electron transport rate; MDA, malondialdehyde; NR, nitrate reductase; NRG2, NITRATE REGULATORY GENE2; PPED, photosynthetic photon flux intensity; R:S, root-to-shoot; SINAR, stress-initiated nitrate allocation in roots; SOD, superoxide dismutase; TPU, triose phosphate utilization; Vc,max, maximum carboxylation rate of Rubisco.
References
Abouelsaad, I., Weihrauch, D., and Renault, S. (2016). Effects of salt stress on the expression of key genes related to nitrogen assimilation and transport in the roots of the cultivated tomato and its wild salt-tolerant relative. Sci. Hortic. 211, 70–78. doi: 10.1016/j.scienta.2016.08.005
Åkesson, A., Barregard, L., Bergdahl, I. A., Nordberg, G. F., Nordberg, M., and Skerfving, S. (2014). Non-renal effects and the risk assessment of environmental cadmium exposure. Environ. Health Persp. 122, 431–438. doi: 10.1289/ehp.1307110
Andreev, M. (2001). Functions of the vacuole in higher plant cells. Russ. J. Plant Physiol. 48, 777–787. doi: 10.1023/a:1016776523371
Bates, L. S., Waldren, R. P., and Teare, I. D. (1973). Rapid determination of free proline for water-stress studies. Plant Soil 39, 205–207. doi: 10.1007/BF00018060
Bertin, G., and Averbeck, D. (2006). Cadmium: cellular effects, modifications of biomolecules, modulation of DNA repair and genotoxic consequences. Biochimie 88, 1549–1559. doi: 10.1016/j.biochi.2006.10.001
Bouguyon, E., Perrine-Walker, F., Pervent, M., Rochette, J., Cuesta, C., Benkova, E., et al. (2016). Nitrate controls root development through post-transcriptional regulation of the NRT1.1/NPF6.3 transporter/sensor. Plant Physiol. 172, 1237–1248. doi: 10.1104/pp.16.01047
Cataldo, D. A., Maroon, M., Schrader, L. E., and Youngs, V. L. (1975). Rapid colorimetric determination of nitrate in plant tissue by nitration of salicylic acid. Commun. Soil Sci. Plant Anal. 6, 71–80. doi: 10.1080/00103627509366547
Chaney, R. L. (2015). How does contamination of rice soils with Cd and Zn cause high incidence of human Cd disease in subsistence rice farmers. Curr. Pollut. Rep. 1, 13–22. doi: 10.1007/s40726-015-0002-4
Chen, C. Z., Lv, X. F., Li, J. Y., Yi, H. Y., and Gong, J. M. (2012). Arabidopsis NRT1.5 is another essential component in the regulation of nitrate reallocation and stress tolerance. Plant Physiol. 159, 1582–1590. doi: 10.1104/pp.112.199257
Corratgé-Faillie, C., and Lacombe, B. (2017). Substrate (un)specificity of Arabidopsis NRT1/PTR FAMILY (NPF) proteins. J. Exp. Bot. 68, 3107–3113. doi: 10.1093/jxb/erw499
Cuypers, A., Plusquin, M., Remans, T., Jozefczak, M., Keunen, E., Gielen, H., et al. (2010). Cadmium stress: an oxidative challenge. Biometals 23, 927–940. doi: 10.1007/s10534-010-9329-x
De Angeli, A., Monachello, D., Ephritikhine, G., Frachisse, J. M., Thomine, S., Gambale, F., et al. (2006). The nitrate/proton antiporter AtCLCa mediates nitrate accumulation in plant vacuoles. Nature 442, 939–942. doi: 10.1038/nature05013
Dürr, M., Boller, T., and Wiemken, A. (1975). Polybase induced lysis of yeast spheroplasts. A new gentle method for preparation of vacuoles. Arch. Microbiol. 105, 319–327. doi: 10.1007/BF00447152
Fan, X., Jia, L., Li, Y., Smith, S. J., Miller, A. J., and Shen, Q. (2007). Comparing nitrate storage and remobilization in two rice cultivars that differ in their nitrogen use efficiency. J. Exp. Bot. 58, 1729–1740. doi: 10.1093/jxb/erm033
Fang, X. Z., Tian, W. H., Liu, X. X., Lin, X. Y., Jin, C. W., and Zheng, S. J. (2016). Alleviation of proton toxicity by nitrate uptake specifically depends on nitrate transporter 1.1 in Arabidopsis. New Phytol. 211, 149–158. doi: 10.1111/nph.13892
Giannopolitis, C. N., and Ries, S. K. (1977). Superoxide dismutases I. occurrence in higher plants. Plant Physiol. 59, 309–314. doi: 10.2307/4264724
Gonzalez-Dugo, V., Durand, J. L., and Gastal, F. (2010). Water deficit and nitrogen nutrition of crops. A review. Agron. Sustain. Dev. 30, 529–544. doi: 10.1051/agro/2009059
Gowri, G., Kenis, J. D., Ingemarsson, B., Redinbaugh, M. G., and Campbell, W. H. (1992). Nitrate reductase transcript is expressed in the primary response of maize to environmental nitrate. Plant Mol. Biol. 18, 55–64. doi: 10.1007/bf00018456
Guo, F. Q., Wang, R., Chen, M., and Crawford, N. M. (2001). The Arabidopsis dual-affinity nitrate transporter gene AtNRT1.1 (CHL1) is activated and functions in nascent organ development during vegetative and reproductive growth. Plant Cell 13, 1761–1777. doi: 10.2307/3871317
Guo, F. Q., Wang, R., and Crawford, N. M. (2002). The Arabidopsis dual-affinity nitrate transporter gene AtNRT1.1 (CHL1) is regulated by auxin in both shoots and roots. J. Exp. Bot. 53, 835–844. doi: 10.1093/jexbot/53.370.835
Guo, F. Q., Young, J., and Crawford, N. M. (2003). The nitrate transporter AtNRT1.1 (CHL1) functions in stomatal opening and contributes to drought susceptibility in Arabidopsis. Plant Cell 15, 1–11. doi: 10.1105/tpc.006312
Gutiérrez, R. A., Gifford, M. L., Poultney, C., Wang, R., Shasha, D. E., Coruzzi, G. M., et al. (2007). Insights into the genomic nitrate response using genetics and the sungear software system. J. Exp. Bot. 58, 2359–2367. doi: 10.1093/jxb/erm079
Han, Y. L., Song, H. X., Liao, Q., Yu, Y., Jian, S. F., Lepo, J. E., et al. (2016). Nitrogen use efficiency is mediated by vacuolar nitrate sequestration capacity in roots of Brassica napus. Plant Physiol. 170, 1684–1698. doi: 10.1104/pp.15.01377
Hayat, S., Ali, B., Hasan, S. A., and Ahmad, A. (2007). Brassinosteroid enhanced the level of antioxidants under cadmium stress in Brassica juncea. Environ. Exp. Bot. 60, 33–41. doi: 10.1016/j.envexpbot.2006.06.002
He, Q. B., and Singh, B. R. (1994). Crop uptake of cadmium from phosphorus fertilizers: I. Yield and cadmium content. Water Air Soil Pollut. 74, 251–265. doi: 10.1007/BF00479793
He, X. L., Fan, S. K., Zhu, J., Guan, M. Y., Liu, X. X., Zhang, Y. S., et al. (2017). Iron supply prevents Cd uptake in Arabidopsis by inhibiting IRT1 expression and favoring competition between Fe and Cd uptake. Plant Soil 416, 453–462. doi: 10.1007/s11104-017-3232-y
Hernandez, L. E., Gárate, A., and Carpena-Ruiz, R. (1997). Effects of cadmium on the uptake, distribution and assimilation of nitrate in Pisum sativum. Plant Soil 189, 97–106. doi: 10.1023/a:1004252816355
Hirel, B., Le Gouis, J., Ney, B., and Gallais, A. (2007). The challenge of improving nitrogen use efficiency in crop plants: towards a more central role for genetic variability and quantitative genetics within integrated approaches. J. Exp. Bot. 58, 2369–2387. doi: 10.1093/jxb/erm097
Ho, C. H., Lin, S. H., Hu, H. C., and Tsay, Y. F. (2009). CHL1 functions as a nitrate sensor in plants. Cell 138, 1184–1194. doi: 10.1016/j.cell.2009.07.004
Hu, H. C., Wang, Y. Y., and Tsay, Y. F. (2009). AtCIPK8, a CBL-interacting protein kinase, regulates the low-affinity phase of the primary nitrate response. Plant J. 57, 264–278. doi: 10.1111/j.1365-313X.2008.03685.x
Huang, J., Zhang, Y., Peng, J. S., Zhong, C., Yi, H. Y., Ow, D. W., et al. (2012). Fission yeast HMT1 lowers seed cadmium through phytochelatin-dependent vacuolar sequestration in Arabidopsis. Plant Physiol. 158, 1779–1788. doi: 10.1104/pp.111.192872
Korenkov, V., Hirschi, K., Crutchfield, J. D., and Wagner, G. J. (2007). Enhancing tonoplast Cd/H antiport activity increases Cd, Zn, and Mn tolerance, and impacts root/shoot Cd partitioning in Nicotiana tabacum L. Planta 226, 1379–1387. doi: 10.2307/23389824
Korenkov, V., King, B., Hirschi, K., and Wagner, G. J. (2009). Root-selective expression of AtCAX4 and AtCAX2 results in reduced lamina cadmium in field-grown Nicotiana tabacum L. Plant Biotechnol. J. 7, 219–226. doi: 10.1111/j.1467-7652.2008.00390.x
Korenkov, V., Park, S., Cheng, N. H., Sreevidya, C., Lachmansingh, J., Morris, J., et al. (2006). Enhanced Cd2+-selective root-tonoplast-transport in tobaccos expressing Arabidopsis cation exchangers. Planta 225, 403–411. doi: 10.2307/23389558
Kramer, G. F., Norman, H. A., Krizek, D. T., and Mirecki, R. M. (1991). Influence of UV-B radiation on polyamines, lipid peroxidation and membrane lipids in cucumber. Phytochemistry 30, 2101–2108. doi: 10.1016/0031-9422(91)83595-C
Krebs, M., Beyhl, D., Görlich, E., Al-Rasheid, K. A., Marten, I., Stierhof, Y. D., et al. (2010). Arabidopsis V-ATPase activity at the tonoplast is required for efficient nutrient storage but not for sodium accumulation. PNAS 107, 3251–3256. doi: 10.1073/pnas.0913035107
Krouk, G. (2016). Hormones and nitrate: a two-way connection. Plant Mol. Biol. 91, 599–606. doi: 10.1007/s11103-016-0463-x
Krouk, G., Crawford, N. M., Coruzzi, G. M., and Tsay, Y. F. (2010). Nitrate signaling: adaptation to fluctuating environments. Curr. Opin. Plant Biol. 13, 265–272. doi: 10.1016/j.pbi.2009.12.003
Li, J. Y., Fu, Y. L., Pike, S. M., Bao, J., Tian, W., Zhang, Y., et al. (2010). The Arabidopsis nitrate transporter NRT1.8 functions in nitrate removal from the xylem sap and mediates cadmium tolerance. Plant Cell 22, 1633–1646. doi: 10.1105/tpc.110.075242
Lin, S. H., Kuo, H. F., Canivenc, G., Lin, C. S., Lepetit, M., and Hsu, P. K. (2008). Mutation of the Arabidopsis NRT1.5 nitrate transporter causes defective root-to-shoot nitrate transport. Plant Cell 20, 2514–2528. doi: 10.1105/tpc.108.060244
Liu, X., Cui, H., Li, A., Zhang, M., and Teng, Y. (2015). The nitrate transporter NRT1.1 is involved in iron deficiency responses in Arabidopsis. J. Plant Nutr. Soil Sci. 178, 601–608. doi: 10.1002/jpln.201400480
Mao, Q. Q., Guan, M. Y., Lu, K. X., Du, S. T., Fan, S. K., Ye, Y. Q., et al. (2014). Inhibition of nitrate transporter 1.1-controlled nitrate uptake reduces cadmium uptake in Arabidopsis. Plant Physiol. 166, 934–944. doi: 10.1104/pp.114.243766
Martinoia, E., Heck, U., and Wiemken, A. (1981). Vacuoles as storage compartments of nitrate in barley leaves. Nature 289, 292–294. doi: 10.1038/289292a0
Martinoia, E., Maeshima, M., and Neuhaus, H. E. (2007). Vacuolar transporters and their essential role in plant metabolism. J. Exp. Bot. 58, 83–102. doi: 10.1093/jxb/erl183
Martinoia, E., Massonneau, A., and Frangne, N. (2000). Transport processes of solutes across the vacuolar membrane of higher plants. Plant Cell Physiol. 41, 1175–1186. doi: 10.1093/pcp/pcd059
Mendoza-Cózatl, D., Loza-Tavera, H., Hernández-Navarro, A., and Moreno-Sánchez, R. (2005). Sulfur assimilation and glutathione metabolism under cadmium stress in yeast, protists and plants. FEMS Microbiol. Rev. 29, 653–671. doi: 10.1016/j.femsre.2004.09.004
Miller, A. J., Fan, X., Orsel, M., Smith, S. J., and Wells, D. M. (2007). Nitrate transport and signalling. J. Exp. Bot. 58, 2297–2306. doi: 10.1093/jxb/erm066
Mohammed, A. S., Kapri, A., and Goel, R. (2011). Heavy metal pollution: source, impact, and remedies. Environ. Pollut. 20, 1–28. doi: 10.1007/978-94-007-1914-9_1
Morel, M., Crouzet, J., Gravot, A., Auroy, P., Leonhardt, N., Vavasseur, A., et al. (2009). AtHMA3, a P1B-ATPase allowing Cd/Zn/Co/Pb vacuolar storage in Arabidopsis. Plant Physiol. 149, 894–904. doi: 10.1104/pp.108.130294
Mounier, E., Pervent, M., Ljung, K., Gojon, A., and Nacry, P. (2014). Auxin-mediated nitrate signalling by NRT1.1 participates in the adaptive response of Arabidopsis root architecture to the spatial heterogeneity of nitrate availability. Plant Cell Environ. 37, 162–174. doi: 10.1111/pce.12143
Ottosen, L. M., Pedersen, A. J., Hansen, H. K., and Ribeiro, A. B. (2007). Screening the possibility for removing cadmium and other heavy metals from wastewater sludge and bio-ashes by an electrodialytic method. Electrochim. Acta 52, 3420–3426. doi: 10.1016/j.electacta.2006.06.048
Redinbaugh, M. G., and Campbell, W. H. (1993). Glutamine synthetase and ferredoxin-dependent glutamate synthase expression in the maize (Zea mays) root primary response to nitrate (evidence for an organ-specifc response). Plant Physiol. 101, 1249–1255. doi: 10.1104/pp.101.4.1249
Remans, T., Nacry, P., Pervent, M., Filleur, S., Diatloff, E., Mounier, E., et al. (2006). The Arabidopsis nrt1.1 transporter participates in the signaling pathway triggering root colonization of nitrate-rich patches. PNAS 103, 19206–19211. doi: 10.1073/pnas.0605275103
Robert, S., Zouhar, J., Carter, C., and Raikhel, N. (2007). Isolation of intact vacuoles from Arabidopsis rosette leaf-derived protoplasts. Nat. Protoc. 2, 259–262. doi: 10.1038/nprot.2007.26
Roberts, T. L. (2014). Cadmium and phosphorous fertilizers: the issues and the science. Procedia Eng. 83, 52–59. doi: 10.1016/j.proeng.2014.09.012
Ruffel, S., Gojon, A., and Lejay, L. (2014). Signal interactions in the regulation of root nitrate uptake. J. Exp. Bot. 65, 5509–5517. doi: 10.1093/jxb/eru321
Sharkey, T. D., Bernacchi, C. J., Farquhar, G. D., and Singsaas, E. L. (2007). Fitting photosynthetic carbon dioxide response curves for C3 leaves. Plant Cell Environ. 30, 1035–1040. doi: 10.1111/j.1365-3040.2007.01710.x
Sharma, P., and Dubey, R. S. (2005). Modulation of nitrate reductase activity in rice seedlings under aluminium toxicity and water stress: role of osmolytes as enzyme protectant. J. Plant Physiol. 162, 854–864. doi: 10.1016/j.jplph.2004.09.011
Sharma, S. S., Dietz, K. J., and Mimura, T. (2016). Vacuolar compartmentalization as indispensable component of heavy metal detoxification in plants. Plant Cell Environ. 39, 1112–1126. doi: 10.1111/pce.12706
Sunil, B., Talla, S. K., Aswani, V., and Raghavendra, A. S. (2013). Optimization of photosynthesis by multiple metabolic pathways involving interorganelle interactions: resource sharing and ROS maintenance as the bases. Photosynth. Res. 117, 61–71. doi: 10.1007/s11120-013-9889-z
Tsay, Y. F., Schroeder, J. I., Feldmann, K. A., and Crawford, N. M. (1993). The herbicide sensitivity gene CHL1 of Arabidopsis encodes a nitrate-inducible nitrate transporter. Cell 72, 705–713. doi: 10.1016/0092-8674(93)90399-B
Ueno, D., Milner, M. J., Yamaji, N., Yokosho, K., Koyama, E., Clemencia, Z. M., et al. (2011). Elevated expression of TcHMA3 plays a key role in the extreme Cd tolerance in a Cd hyperaccumulating ecotype of Thlaspi caerulescens. Plant J. 66, 852–862. doi: 10.1111/j.1365-313X.2011.04548.x
Undurraga, S. F., Ibarrahenríquez, C., Fredes, I., Álvarez, J. M., and Gutiérrez, R. A. (2017). Nitrate signaling and early responses in Arabidopsis roots. J. Exp. Bot. 68, 2541–2551. doi: 10.1093/jxb/erx041
Vidal, E. A., Moyano, T. C., Canales, J., and Gutierrez, R. A. (2014). Nitrogen control of developmental phase transitions in Arabidopsis thaliana. J. Exp. Bot. 65, 5611–5618. doi: 10.1093/jxb/eru326
Vögeli-Lange, R., and Wagner, G. J. (1990). Subcellular localization of cadmium and cadmium-binding peptides in tobacco leaves: implication of a transport function for cadmium-binding peptides. Plant Physiol. 92, 1086–1093. doi: 10.2307/4272746
Wang, Y. Y., Hsu, P. K., and Tsay, Y. F. (2012). Uptake, allocation and signaling of nitrate. Trends Plant Sci. 17, 458–467. doi: 10.1016/j.tplants.2012.04.006
Wellburn, A. R., and Lichtenthaler, H. K. (1984). Formulae and program to determine total carotenoids and chlorophylls a and b of leaf extracts in different solvents. Adv. Photosynth. Res. 2, 9–12. doi: 10.1007/978-94-017-6368-4_3
Wong, C. S. C., Li, X. D., Zhang, G., Qi, S. H., and Peng, X. Z. (2003). Atmospheric deposition of heavy metals in the pearl river delta. China. Atmos. Environ. 37, 767–776. doi: 10.1016/s1352-2310(02)00929-9
Woodis, T. C., Hunter, G. B., and Johnson, F. J. (1977). Statistical studies of matrix effects on the determination of cadmium and lead in fertilizer materials and plant tissue by flameless atomic absorption spectrometry. Anal. Chim. Acta 90, 127–136. doi: 10.1016/S0003-2670(01)82301-1
Xu, N., Wang, R., Zhao, L., Zhang, C., Li, Z., Lei, Z., et al. (2016). The Arabidopsis NRG2 protein mediates nitrate signaling and interacts with and regulates key nitrate regulators. Plant Cell 28, 485–504. doi: 10.1105/tpc.15.00567
Yang, Y., Xiong, J., Chen, R., Fu, G., Chen, T., and Tao, L. (2016). Excessive nitrate enhances cadmium (Cd) uptake by up-regulating the expression of OSIRT1, in rice (Oryza sativa). Environ. Expl. Bot. 122, 141–149. doi: 10.1016/j.envexpbot.2015.10.001
Zhang, G. B., Yi, H. Y., and Gong, J. M. (2014). The Arabidopsis ethylene/jasmonic acid-NRT signaling module coordinates nitrate reallocation and the trade-off between growth and environmental adaptation. Plant Cell 26, 3984–3998. doi: 10.1105/tpc.114.129296
Keywords: CHL1/NRT1.1/NPF6.3, NITRATE REGULATORY GENE 2, nitrate allocation, cadmium stress, vacuole, Arabidopsis
Citation: Jian S, Luo J, Liao Q, Liu Q, Guan C and Zhang Z (2019) NRT1.1 Regulates Nitrate Allocation and Cadmium Tolerance in Arabidopsis. Front. Plant Sci. 10:384. doi: 10.3389/fpls.2019.00384
Received: 22 December 2018; Accepted: 13 March 2019;
Published: 27 March 2019.
Edited by:
Eric Ruelland, Centre National de la Recherche Scientifique (CNRS), FranceReviewed by:
Abdelilah Chaoui, University of Carthage, TunisiaKo Noguchi, Tokyo University of Pharmacy and Life Sciences, Japan
Copyright © 2019 Jian, Luo, Liao, Liu, Guan and Zhang. This is an open-access article distributed under the terms of the Creative Commons Attribution License (CC BY). The use, distribution or reproduction in other forums is permitted, provided the original author(s) and the copyright owner(s) are credited and that the original publication in this journal is cited, in accordance with accepted academic practice. No use, distribution or reproduction is permitted which does not comply with these terms.
*Correspondence: Zhenhua Zhang, emh6aDE0NjhAMTYzLmNvbQ==