- 1College of Agronomy, Sichuan Agricultural University, Chengdu, China
- 2China Key Laboratory of Crop Ecophysiology and Farming System in Southwest, Ministry of Agriculture, Chengdu, China
- 3Maize Research Institute, Sichuan Agricultural University, Chengdu, China
- 4College of Resources, Sichuan Agricultural University, Chengdu, China
In intercropping systems shading conditions significantly impair the seed yield and quality of soybean, and rarely someone investigated the minimum amount of light requirement for soybean growth and development. Therefore, it is an urgent need to determine the threshold light intensity to ensure sustainable soybean production under these systems. An integrated approach combining morphology, physiology, biochemistry and genetic analysis was undertaken to study the light intensity effects on soybean growth and development. A pot experiment was set up in a growth chamber under increasing light intensity treatments of 100 (L100), 200 (L200), 300 (L300), 400 (L400), and 500 (L500) μmol m−2 s−1. Compared with L100, plant height, hypocotyl length, and abaxial leaf petiole angle were decreased, biomass, root:shoot ratio, and stem diameter were increased, extremum was almost observed in L400 and L500. Leaf petiole movement and leaf hyponasty in each treatment has presented a tendency to decrease the leaf angle from L500 to L100. In addition, the cytochrome content (Chl a, Chl b, Car), net photosynthetic rate, chlorophyll fluorescence values of Fv/Fm, /, ETR, ΦPSII, and qP were increased as the light intensity increased, and higher values were noted under L400. Leaf microstructure and chloroplast ultrastructure positively improved with increasing light intensity, and leaf-thickness, palisade, and spongy tissues-thickness were increased by 105, 90, and 370%, under L500 than L100. Moreover, the cross-sectional area of chloroplast (C) outer membrane and starch grains (S), and sectional area ratio (S:C) was highest under L400 and L500, respectively. Compared to L100, the content of starch granules increased by 35.5, 122.0, 157.6, and 145.5%, respectively in L400. The same trends were observed in the enzyme activity of sucrose-synthase, sucrose phosphate synthase, starch synthase, rubisco, phosphoenol pyruvate carboxykinase, and phosphoenol pyruvate phosphatase. Furthermore, sucrose synthesis-related genes were also up-regulated by increasing light intensity, and the highest seed yield and yield related parameters were recorded in the L400. Overall, these results suggested that 400 and 500 μmol m−2 s−1 is the optimum light intensity which positively changed the leaf orientation and adjusts leaf angle to perpendicular to coming light, consequently, soybean plants grow well under prevailing conditions.
Introduction
Light intensity and quality are among the most critical environmental factors for crop physiology and biochemistry (Yang et al., 2018a). For most crop plants, even a slight increase or decrease in light intensity leads to considerable changes in leaf morphology and structure (Wu et al., 2017). According to past comparative studies, the dry matter of roots, stems, leaves, and whole plant as well as the photosynthetic rate, transpiration, and stomatal conductance, and the stem diameter decreased in low light conditions (Yang et al., 2014, 2017). In addition, crop plants produce smaller and thinner leaves under low light conditions than corresponding leaves in full sunlight conditions (Wu et al., 2017). However, shading environments increased the plant height and lodging rate which hinders the transportation of nutrients, water, and photosynthetic products and ultimately causes huge losses to agriculture production. Taken together, light intensity is the main factors which controls the central process of plants such as germination, leaf proliferation and expansion, photosynthesis, buds and flower initiation, and cell division (Kong et al., 2016; Wu et al., 2018; Yang et al., 2018b). Indeed, the numerous plant processes improve with increasing light intensity up to a moderate level which bring dramatic developmental and physiological changes to occur, leading to the rapid increase of these processes (Yang et al., 2015; Wu et al., 2016).
Reductions in light intensity could affect carbon balance of crop plant because the carbohydrate demand increases while its production decreases: rates of physiological processes rise while the photosynthetic yield reduces (Lichtenthaler et al., 1981). Accordingly, tolerance to shade stress increased at high net photosynthetic rate in C3 plants (Su et al., 2014). Moreover, the pattern of carbohydrates into expensive processes, like the biosynthesis of defense proteins (notably light-harvesting chlorophyll protein) raises with increasing shade density (Yang et al., 2018b). In line with this, Rijkers et al. (2010) concluded that plant defense to shade stress is increased at optimum nitrogen supply due to the high light-saturated photosynthetic nitrogen-use. The net photosynthetic rate is the major driver of crop plant carbon balance, optimum and continuous availability of light should also be considered into account to study the plant responses to shade stress.
Leaf positioning directly determines the light interception, it has been considered that phototropism of leaf could be a part of plant responses to shade stress. For example, in different crop species, variations in leaf angle evade the heat stress when maximal light intensity is available (Fu and Ehleringer, 1989; King, 1997; Falster and Westoby, 2003; Vasseur et al., 2011). In crop plants, hyponasty (upward movement of leaf) is one of the first leaf morphological responses to changing light intensity (Pharis and King, 1985). Hyponastic response changes widely among different species and is related to different environmental conditions encountered in collection sites, indicating an adaptive role for this character (van Zanten et al., 2009b). Furthermore, Arabidopsis rosettes showed the hyponastic growth and reduced the leaf angle under high temperature caused by excessive light interception with higher rate of transpiration which could contribute to reducing leaf temperature by enhancing transpiration (Gray et al., 1998; Franklin, 2009). Usually, low light or shading conditions induced the hyponasty response in plants (Hangarter, 1997; Maliakal et al., 1999; Smith, 2000), which is controlled by phytochrome and cryptochrome pathways (Smalle et al., 1997; Vandenbussche et al., 2003; Kozuka et al., 2005; Millenaar et al., 2009). Therefore, hyponasty has been believed to be a typical morphological response of shade avoiding syndrome, which allowed the plants to capture more sun-light and increase carbon gain under the light competition conditions (Pierik et al., 2004; Mullen et al., 2006; van Zanten et al., 2010). Previously, many scientists have studied the effects of light intensity on leaf positioning (van Zanten et al., 2009a) and hypocotyl growth pattern (Wherley et al., 2005). Importantly, decreased light intensity induced the hyponastic response in multiple loss-of-function photoreceptor mutants (Millenaar et al., 2009). Taken together, these findings indicating a tight and close link between leaf angle and light intensity. Therefore, it is important to investigate the response of plant leaf angle under changing light intensity to understand the soybean leaf angle orientation under shading conditions. Crop growth as dry matter production is largely dependent on current photosynthesis and, therefore, one of the main important changes that shade stress affects crop growth is ascribed to its huge reduction of net photosynthesis (Yang et al., 2018b). Reductions in photosynthesis could occur by two main principle mechanisms (Yang et al., 2017): (i) decrease CO2 diffusion into leaves, due to the decrease internal and stomatal conductance (gi and gs, respectively), and (ii) metabolic potential inhibition for photosynthesis by inhibiting the leaf growth and enlargement by controlling the cell proliferation (Wu et al., 2017, 2018). In addition, light is the only source of energy for starch biosynthesis (Stitt and Zeeman, 2012), and the rates of starch biosynthesis and degradation are adjusted to the availability of sunlight, so that when the availability of light increased the starch formation increased and the rate of degradation decreased (Fernandez et al., 2017). Actually, the starch content reduced in sugar beet (Beta vulgaris) and bean leaves as the intensity of light reduced (Fondy et al., 1989; Servaites et al., 1989). However, rarely researchers have investigated the effect of different light intensity on the biosynthesis of starch. Therefore, further investigations are needed due to the relative significance of such mechanisms is debatable.
The amount and activity of important enzymes involved in CO2 fixation and regeneration of rubisco-1, 5-bisphosphate (RuBP) determined the metabolic potential of photosynthesis in plants under different conditions (Seemann and Sharkey, 1986; Delfine et al., 1999; Redondo-Gomez et al., 2007) as well as the activity and content of light capturing components, electron transport fragments, and energy transferring enzymes (Kao et al., 2003; Ranjbarfordoei et al., 2006; Stepien and Kłbus, 2006). In photosynthesis Rubisco (RuBP carboxylase or oxygenase) catalyzes the process of CO2 fixation (Mauser et al., 2001), which is directly involved in first the phase of Calvin Benson cycle and accounting for 12–35% of the leaf protein especially in C3 crop plants (Evans and Seemann, 1989). In past reports, it have revealed that the main biochemical restraint involved in shade-associated down regulation of net photosynthetic rate was reduction in the amount or activity of Rubisco (Seemann and Sharkey, 1986).
In past few years, chlorophyll fluorescence measurements have been known as an informative and useful indicator characterizing different light responses of photosynthesis. Considerable attention was paid to investigate and to determine the important characteristics of this technique (Schreiber et al., 1995). Chlorophyll fluorescence mainly and effectively used to measures the quantum yield of photosystem II and photo-inhibition by determining the potential quantum yield under prevailing light and shade conditions (Rascher et al., 2010). Shade significantly affected the performance and structure of the photosynthetic apparatus (Yao et al., 2017). It blocks the energy transport from PSII to PSI, reduces the leaf thickness, palisade and spongy tissues which results in low chlorophyll fluorescence (Wu et al., 2017; Yao et al., 2017). Thus, in this present study we investigated the effects of different light intensity treatments on leaf positioning and internal structure, the changes in ultra-structure of chloroplast, photosynthetic and chlorophyll fluorescence parameters, leaf carbon status through sucrose and starch contents, enzymatic activity of key enzymes related to photosynthetic process and sugar synthesis, a transcriptional analysis of some targeted genes to investigate the minimum amount of light quantity required by soybean plants for optimum growth and development. Finally, we concluded that changes in yield and yield related components are tightly related to the changes in light quantity.
Materials and Methods
Plant Material and Growth Condition
Glycine max (Linn.) Merr. seeds (Nandou 12, a major soybean cultivar widely planted in Southwest China) were chosen for the phenotypic responses to growth conditions. Before the experiment, soybean seeds were cleaned by 75% ethanol and deionized water for 5 min and germinated on a wet sterile gauze for 48 h in the dark at 25°C. After germination, two seeds were sown in 400 mL pots filled with a mixed matrix of PINDSTRUP organic soil (Pindstrup Mosebrug A/S, Ryomgaard, Denmark) and vermiculite (v:v, 4:1) in a light chamber with 25°C/20°C day/night temperature, 60% relative humidity, 460 μmol mol−1 CO2 and 10/14 h photoperiod. In this experiment, we used growth chamber I-66VL (Percival Scientific Inc., Watson, American), three plates were placed in each chamber and every plate contained 5 plants at a distance of 20.4 cm, and for light intensity treatments we used LED lights. The soybean seedlings have grown up to V2 stage (before the second trifoliate leaf appearance) (Fehr and Caviness, 1977), the pots were transferred to 5 light chambers for light intensity treatment (Supporting Information S1, S2, and S3) by keeping the light quality same in all experimental treatments. When the four trifoliate leaf appearance (about 15 days), the second and third leaves will be harvest for parameters measurement and analyzed. The pots were moved daily to avoid boundary effects and soybean seedlings watered with a one-fifth-strength Hoagland's solution (Hoagland and Arnon, 1950) every 2 days. Light intensity and spectral irradiance (λ = 380–760 nm) measured by HR350 (Hipoint Inc., Gaoxiong, Taiwan). Every treatment was performed with three replicates.
Plant and Leaf Traits
Total plant height, stem diameter, biomass, and root: shoot ratio of fully expanded leaf were determined by the light intensity conditions for 15 days treatment and measured at V4 stage. Measurement of abaxial leaf petiole angle (degree) were performed every 2 h in a day at on randomly selected plants.
Photosynthesis and Photosynthetic Pigment Concentration
Li-6400 portable photosynthesis system (LI-COR Inc., Lincoln, NE, USA) was used for photosynthetic parameters measurement of soybean V3 expanding leaves. All parameters including net photosynthetic rate (Pn), transpiration rate (Tr), stomatal conductance (Gs), and intercellular CO2 concentration (Ci) were measured under steady light intensity 600 μmol m−2 s−1, environment temperature 25°C and a CO2 concentration 460 (μmol mol−1) from 9:00 to 11:00.
Soybean V3 trifoliate leaves were collected for photosynthetic pigment concentration measurement and each treatment in three replicates. Chl a, Chl b, Car were extracted from all the leaf samples, and two leaf discs (1.130 cm2) were cut from the middle part of each middle lobules by a puncher (1.2 diameter), and dipped the samples in 10 mL of N,N-dimethyl formamide solution in the dark for 48 h at 4°C (Kutík et al., 2001). The extraction mixture was then measured at wavelengths of 663, 645, and 470 nm by using a spectrophotometer DU-730 (Beck Man Coulter Inc., USA).
Chlorophyll Fluorescence Measurements
The chlorophyll a fluorescence measurement was performed with the miniaturized pulse-amplitude-modulated photosynthesis yield. Before measurement, each plant were transferred to adapt for 30 min in the dark chamber and submitted to chlorophyll fluorescence image capture system (CFImager, Technologica Ltd., Colchester, UK) to estimate maximal PSII quantum yield (Fv/Fm), photochemical efficiency of PSII (/), effective PSII quantum yield (ΦPSII), non-photochemical quenching (NPQ) and coefficient of photochemical quenching (qP). All the parameters were calculated as the methods reported by Maxwell and Johnson (2000). The apparent electron transport rate (ETR) were defined by a uniform absorption of incident light over the whole middle trifoliate leaf at V3 stage.
Leaf Anatomical Features and Chloroplast Ultrastructure
Leaf piece (4 mm2) about 15 replications for each treatment without midribs were fixed in formalin-acetic acid-alcohol solution (FAA; 90% ethanol; 5% formaldehyde; 5% glacial acetic acid, v/v/v) at 4°C for 3 days. The leaf samples were dehydrated in a graded series of ethanol solutions (95, 75, 50, 25, 10% of ethanol for each treatment 30 min and 3 times, respectively), embedded in paraffin and cut the tissue sections to 4 μm thickness with a rotary microtome (RM2235, Leica Microsystems Ltd., Germany). The tissue sections were co-stained by Safranine and Fast Green, observed with a light microscope (ECLIPSE Ts2, Nikon Instruments Inc., Japan) and captured by a digital camera (Digital Sight DS-U3, Nikon Instruments Inc., Japan). The thickness of total leaf, spongy, and palisade mesophyll, and abaxial epidermis were measured by ImageJ.AS described by Yang et al. (2018b), same leaf piece size and replications were cut for chloroplast ultrastructure observation, fixed in glutaric dialdehyde solution (3% glutaraldehyde; 1% osmium tetroxide), and dehydrated in a graded series of acetone solutionsEpon812 were used for tissue embedment, then ultrathin section was cut after uranyl acetate and lead citrate staining. A transmission electron microscope (TEM; HITACHI, H-600IV, Japan) were used for photographs examination. The cross-sectional area of chloroplast, grana, thylakoids, and starch grains were measured by ImageJ.
Real-Time Quantitative PCR Verification
The expanding leaves of Nandou12 at V4 stage under 5 light intensity conditions were harvested on five plants for consideration of RNA abundance and sensitivity of the blade to light intensity. All the leaves were labeled and frozen in liquid nitrogen immediately. RNA was extracted using the TRIzol™ Plus RNA Purification Kit (Invitrogen). Reverse transcription and amplification of cDNA were performed using Super Script III First-Strand Synthesis Super Mix for qRT-PCR (Invitrogen). Real-time quantitative PCR was conducted in Quant Studio 6 Flex Real-Time PCR System (Thermo Fisher Scientific) and 2−ΔΔCT method used for data analysis. Cycle threshold (Ct) values were determined by subtracting the difference of the Ct levels. The reference gene Gmactin11 was selected for the control. All the target genes primers are listed in Supporting Information Table S1.
Enzyme Activity
Before the enzyme activity measurement, the frozen leaves were ground with a pre-cooled mortar and pestle in 1.5 mL ice-cold buffer containing 50 mM Hepes-KOH (pH 7.5), 2 mM EDTA, 10 mM MgCl2, 10 mM dithiothreitol (DTT), 1% (w/v) Triton X-100, 10% (w/v) glycerol, 1% (w/v) bovine serum albumin (BSA), 5% (w/v) polyvinylpolypyrrolidine (PVPP), and 1 mM phenylmethylsulfonyl fluoride (PMSF). The extract was centrifuged at 13,000 g at 4°C for 10 min, and the supernatant was used immediately for activity assay. SS, SPS were determined in 1 mL reaction mixture containing and 500 μL enzyme extract at 34°C for 1 h, added 30% KOH to mixture in a boiling water bath for 10 min to completely inactivate and cooled to room temperature, then applied 3.5 mL 0.15% anthrone-sulfuric acid solution in the last reaction mixture at 40°C for 20 min, the increase in A620 was monitored. Rubisco total activity was measured by injecting 100 μL of the supernatant into 400 μL of an assay mixture consisting of 50 mM Tris-HCl (pH 8.0), 5 mM DTT, 10 mM MgCl2, 0.1 mM EDTA, and 20 mM NaH14CO3 (2.0 GBq mmol−1) at 30°C. After a 5 min activation period, the reaction was initiated by adding RuBP to 0.5 mmol L−1 and terminating after 30 s with 100 μL of 6 mol L−1 HCl.PEPC and PEPP were extracted according to Yang et al. (2012) with some modifications. PEPC was assayed in 1 mL reaction mixture consisting of 50 mM Tris-HCl (pH 8.0), 5 mM MnCl2, 2 mM DTT, 10 mM NaHCO3, 0.2 mM NADH, 5 unit NAD-MDH and 160 μL enzyme extract. The reaction was initiated by adding 2.5 mM phosphoenolpyruvate (PEP). PEPP was determined in 1 mL reaction mixture containing 100 mM imidazole-HCl pH 7.0, 50 mM KCl, 10 mM MgCl2, 0.05% (w/v) BSA, 2 mM DTT, 150 μM NADH, 1 unit LDH, 2 mM ADP and 100 μL enzyme extract. The reaction was initiated with 2 mM PEP, the increase in A412 was monitored. In addition, the activities of adenosine diphosphate glucose pyro-phosphorylase (ADPGPPase), uridine diphosphate glucose pyro-phosphorylase (UDPGPPase), and soluble starch synthase (SSS) were measured by using the previously mentioned method (Doehlert et al., 1988; Liang et al., 1994).
Soluble Sugar, Sucrose, and Starch Content
The samples of soybean leaves at V3 stage were harvest at the end of the day or night and analyzed by enzymatic assay as in Vasseur et al. (2011).
Statistical Analysis of Data
All data analyses were performed by Statistical software (version 7.0; StatSoft). Significance was determined via one-way analysis of variance. Values are presented mean + standard error (SE) from three independent biological replicates, and the least significance difference (LSD) test was employed to compare the means at 5% probability level.
Results
Morphological Characteristics
Figure 1 presents the plant morphological characteristics of the soybean plants under different light intensity treatments. In the present experiment, different light intensity treatments had a significant effect on plant morphological characteristics, the soybean plant height and stem diameter of soybean were considerably increased from L100to L500. Specifically, the maximum soybean plant height 36.5 cm and stem diameter 3.86 mm were measured in treatments L100 and L500, and minimum plant height 7.9 cm and stem diameter 2.76 mm were observed under L500 and L100, respectively. Overall, treatment L100 and L500 significantly increased the plant height and stem diameter increased by 362 and 40%, with respect to L500 and L100, respectively. Table 1 presents the hypocotyl length, plant biomass (PB), and root to shoot ratio of soybean plants in response to different light intensity treatments. The light intensity treatments showed a significant effect on hypocotyl length, PB, and root to shoot ratio of soybean plants, and the PB, and root to shoot ratio of soybean plants in L500 were significantly higher than those under L100, whereas opposite trend was observed for hypocotyl length and it was maximum in L100 and minimum under treatment L500. Mostly, the plant traits showed were non-significant differences in treatments L400 and L500. These results present that increasing light intensity from L100 to L500 not only increased the stem diameter of soybean plants but also improves the other plant traits related to plant growth and development by reducing the plant height because higher plant height increases the rate of plant lodging under shading conditions. In addition, for plant morphological characteristics, vertical elongation of a plant was inhibited with the increase in light intensity, while the transverse extension was improved.
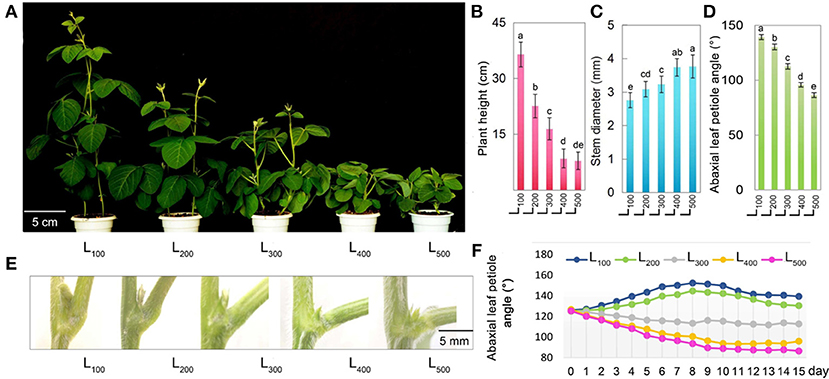
Figure 1. Changes in phenotype and plant traits of soybean as affected by different light intensity treatments. The phenotype (A), Plant height (B), stem diameter (C), and abaxial leaf petiole angle (D), leaf angle picture representation (E), and graphical representation of leaf angle for continuous 15 days (F) of soybean plants under different light intensity treatments. L100, L200, L300, L400, and L500, refer 100, 200, 300, 400, and 500 μmol m−2 s−1, respectively. All the values are representative of three independent experiments. Bars show + standard errors. Within a bar, different lowercase letters show a significant difference at 5% level, between treatments.
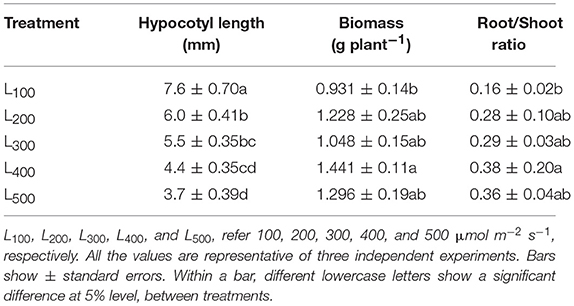
Table 1. Effect of different light intensity treatments on hypocotyl length (mm), biomass (g plant−1), and shoot to root ratio of soybean plants.
Leaf Anatomy
Light quality and quantity, especially light intensity affect positively the leaf anatomy. In this study, the significant differences in leaf thickness, palisade tissues thickness, spongy tissues thickness, and ratio of palisade and spongy thickness were noticed among all the five light intensity treatments (Figures 2A,D–F). Interestingly, soybean leaves showed perfect development of the palisade tissues, and the clearer and compact structure of spongy tissues were observed under treatments L400 and L500. Moreover, the leaf thickness, palisade tissues thickness, and spongy tissues thickness of soybean plants under L500 were significantly higher than those in L100. The maximum and minimum leaf thickness, palisade tissues thickness, and spongy tissues thickness were noted under treatments L100 and L500, respectively. However, the soybean plants grown under L400 and L500 treatments exhibited the decreased ratio of palisade and spongy thickness than treatment L100. On average, leaf thickness, palisade tissues thickness, and spongy tissues thickness increased by 105, 90, and 370%, under L500 in comparison with L100 treatment, the ratio of palisade and spongy thickness was decreased by 147% in L500 than L100 treatment. These findings indicated that shade conditions or low light intensity negatively affected the soybean leaf tissue size, while an optimum light intensity significantly increased the leaf thickness, palisade tissues thickness, and spongy tissues thickness.
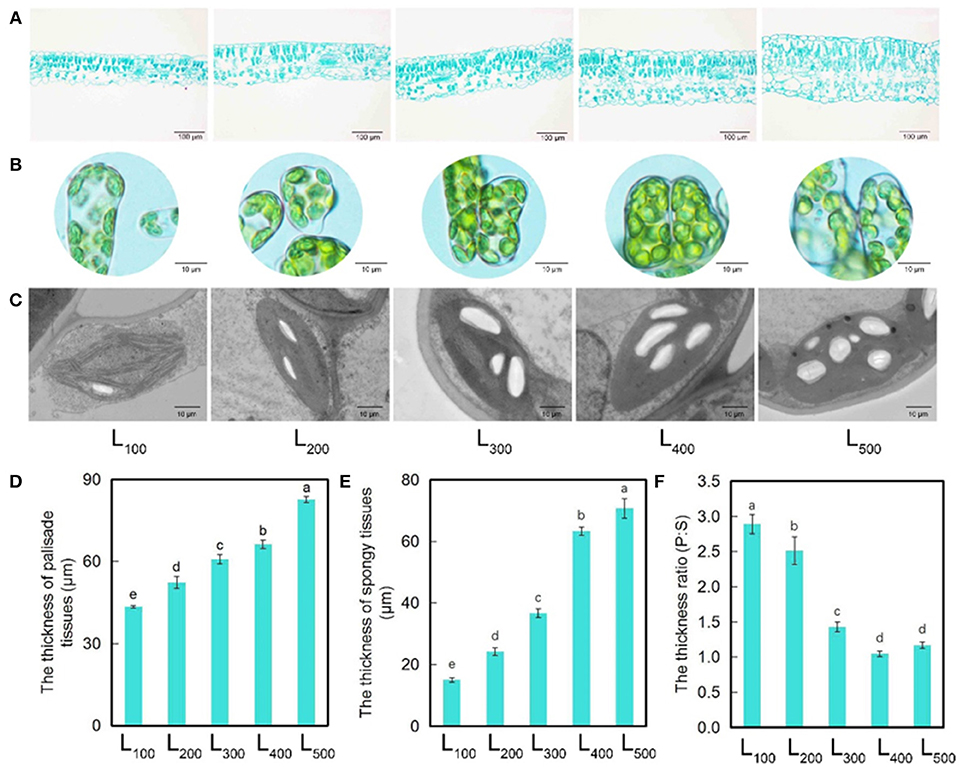
Figure 2. Changes in leaf structure and chloroplast structure of soybean plants as affected by different light intensity treatments. Leaf thickness (A), chloroplast number (B), starch grana (C), thickness of palisade (D), thickness of spongy tissues (E), and ratio of palisade, and spongy thickness (F) of soybean plants under different light intensity treatments. L100, L200, L300, L400, and L500, refer 100, 200, 300, 400, and 500 μmol m−2 s−1, respectively. All the values are representative of three independent experiments. Bars show + standard errors. Within a bar, different lowercase letters show a significant difference at 5% level, between treatments.
Leaf Angle and Carbon Balance
Differences in light intensity induced the epinasty or hyponasty leaf movements in plants. In the present study, different light intensity treatments had a significant impact on the abaxial leaf petiole angle of soybean leaves (Figure 1D). A strong hyponasty (an increase in abaxial leaf petiole angle) was observed under light intensity treatments L100 and L200. The decrease in light intensity from L500 to L100 increase the hyponastic response in soybean plants, and minimum 87.5° abaxial leaf petiole angle was measured under L500 treatments, while maximum 141.6° abaxial leaf petiole angle was noted in L100 treatment. In addition, we measured abaxial leaf angle for continuous 15 days and all the treatments showed consistent effect on leaf angle of soybean plants, maximum under L100, and minimum in treatment L500 (Figures 1E,F). Overall, higher light intensity treatment decreased abaxial leaf petiole angle by 38% compared to the corresponding value under treatment L100. Our results indicated that the shading conditions increased the abaxial leaf petiole angle which negatively affected the light absorption and photosynthetic process in soybean plants.
To further investigate the effect of light intensity solely regulates the hyponastic response in soybean, we determined the sucrose, starch, and total soluble sugar content of soybean shoot and root at the end of day and night. As expected, the sucrose, starch, and total soluble sugar content were significantly increased with increasing light intensity in both shoot and root. The highest sucrose content 0.58 mg g−1, starch content 0.71 mg g−1, and total soluble sugar content 6.74 mg g−1 were measured in treatment L400 as compared with L100 treatment in shoot (Figures 3A–C). The same trend was observed in root. Interestingly, under L400 treatment, this trend of increased sucrose, starch, and total soluble sugar content proved the epinastic movement of soybean leaves because as we mentioned above that increased light intensity (L400 and L500) decreased abaxial leaf petiole angle and increased the light absorption area of soybean leaves which in turn increased the sucrose, starch, and total soluble sugar content of shoot and root due to the higher photosynthetic activity in soybean plants.
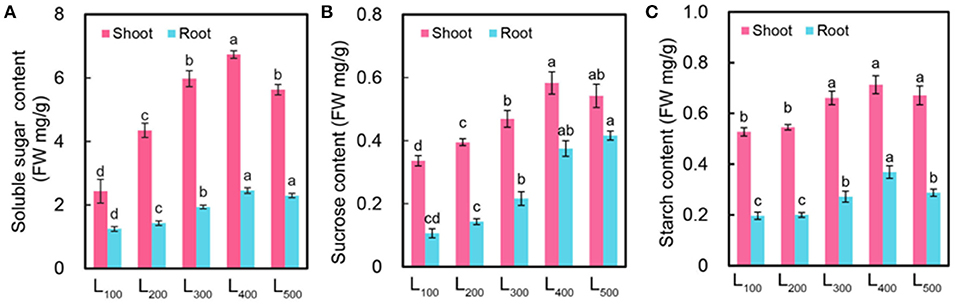
Figure 3. Changes in carbon balance of soybean plants as affected by different light intensity treatments. Soluble sugar content [FW (fresh weight) mg/g] (A), sucrose content [FW (fresh weight) mg/g] (B), and starch content [FW (fresh weight) mg/g] (C) of soybean plants under different light intensity treatments. L100, L200, L300, L400, and L500, refer 100, 200, 300, 400, and 500 μmol m−2 s−1, respectively. All the values are average of three replicates and representative of three independent experiments. Bars show + standard errors. Within a bar, different lowercase letters show a significant difference at 5% level, between treatments.
Chlorophyll Content and Chloroplast Structure
The chlorophyll (Chl) and carotenoids (Car) content of soybean leaves were considerably affected by different light intensity treatments. In this experiment, increasing light intensity from L100 to L500 increased the Chl a, Chl b, Chl a + b, and Car contents while Chl a/b decreased (Table 2). In addition, the Chl a, Chl b, Chl a + b, and Car contents of soybean leaves were found non-significant between light intensity treatments L400 and L500, but Chl a, Chl b, Chl a + b, and Car contents were always higher in L500 than those of under treatment L100. On average, Chl a, Chl b, Chl a + b, and Car contents increased by 43.6, 70, 49, and 20%, under L500 in comparison with L100 treatment, respectively. These improvements suggesting a direct relationship of chlorophyll and carotenoids content with the changes in light intensity.
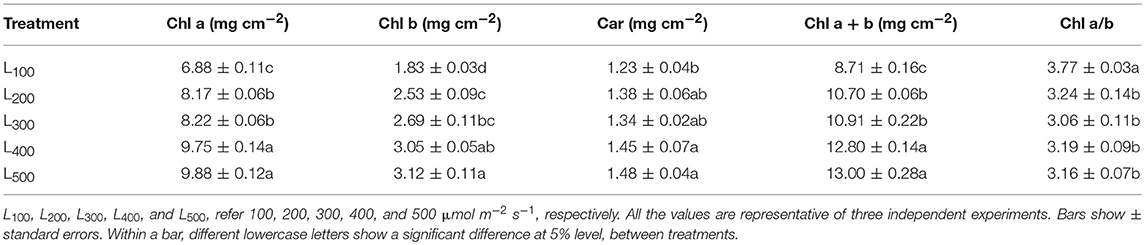
Table 2. Effect of different light intensity treatments on cytochrome content (Chl a, Chl b, Car) and Chl a to b ratio of soybean plants.
In our study, the observations of chloroplast structure shown that chloroplast number and structure were significantly influenced by different light intensity treatments in soybean plants. The highest number of chloroplast was noticed under treatment L400, while the lowest number of chloroplast was observed under L100 (Figure 2B). Moreover, as compared to L100 chloroplasts under L400 were organized centrally in the cell and showed a more compact arrangement, and the grana stacks of chloroplast were clear and large, and every chloroplast contained 4–5 big starch grains (Figure 2C). In addition, the highest cross-sectional area of chloroplast (C) outer membrane, cross-sectional area of starch grains (S), thylakoid to chloroplast ratio (T:C), and sectional area ratio (C:S) were measured under L500 and L400, respectively, while the lowest was determined under treatment L100 Supporting Information Table S2. Taken together, increase light intensity significantly improved the chloroplast structure and arrangement.
Enzymatic Activity and Gene Expression
In this experiment, significant differences in sucrose synthase (SS), sucrose phosphate synthase (SPS), rubisco, phosphoenol pyruvate carboxykinase (PEPC), phosphoenol lpyrute phosphatase (PEPP), adenosine diphosphate glucose pyro-phosphorylase (ADPGPPase), uridine diphosphate glucose pyro-phosphorylase (UDPGPPase), and soluble starch synthase (SSS) were noticed in different light intensity treatments (Figure 4). The SS, PEPC, PEPP, ADPGPPase, UDPGPPase, and SSS activities of soybean plants were gradually increased with increasing light intensity from L100 and L500 and the higher values were measured under L500 than those in other light treatments (Figures 4A–E). On average, the activities of SS, PEPC, PEPP, ADPGPPase, UDPGPPase and SSS were enhanced by 52, 134, 122, 144, 242, and 345% under L500, respectively, with respect to that in L100 treatment. Furthermore, we also measured the activities of SPS and rubisco, there was a significant difference among all light intensity treatments. Acceleration in the activities of SPS and rubisco occurred in all light treatments, the amplitude of acceleration was higher in L400 than L500, L300, L200, and L100 treatments. Moreover, we also measured the activities of starch biosynthesis enzymes (Supporting Information S4) and found maximum values from 400 to 500 μmol m−2 s−1. Overall, the SPS and rubisco activities of soybean plants under L400 was increased by 73 and 89% with respect to those under L100 treatment (Figures 4A,B). These results suggest that the activities of SS, PEPC, PEPP, SPS, and rubisco were directly associated with the changes in light intensity. In our case, using the L400 treatment can be more effective at the enzymatic activities.
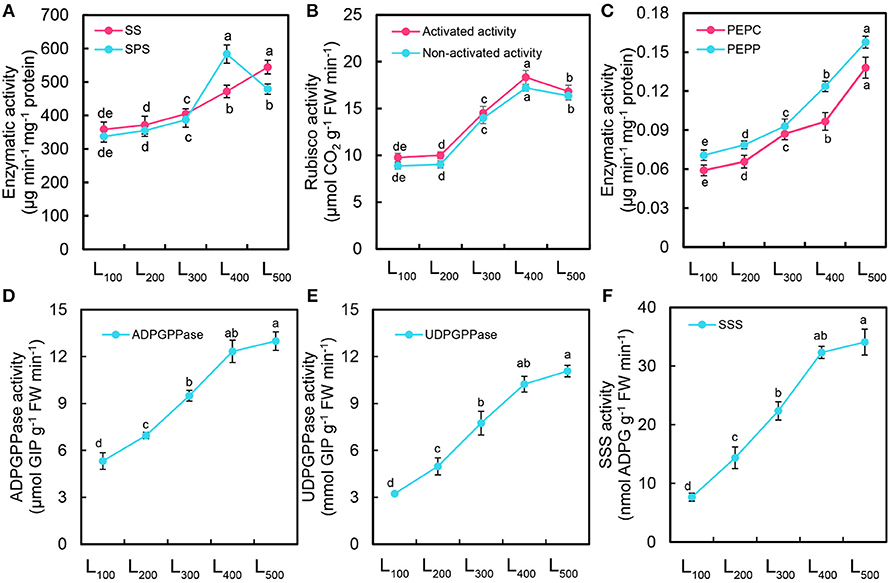
Figure 4. Changes in enzymatic activity of soybean plants as affected by different light intensity treatments. Activity of sucrose synthase (SS) and sucrose phosphate synthase (SPS) (A), activated and non-activated activity of Rubisco (B), activity of phosphoenolpyruvate carboxykinase (PEPC) and phosphoenolpyruvate phosphatase (PEPP) (C), adenosine diphosphate glucose pyro-phosphorylase (ADPGPPase) (D), uridine diphosphate glucose pyro-phosphorylase (UDPGPPase) (E), and soluble starch synthase (SSS) (F) of soybean plants under different light intensity treatments. L100, L200, L300, L400, and L500, refer 100, 200, 300, 400, and 500 μmol m−2 s−1, respectively. All the values are representative of three independent experiments. Bars show ± standard errors. Within a bar, different lowercase letters show a significant difference at 5% level, between treatments.
After blast against Arabidopsis, homologs of soybean were chosen to determine their gene expression levels in our experiment. Two genes expression involves in sucrose phosphate synthase (Gmsps1 and Gmsps2), one gene involves in sucrose synthase (Gmss1), and six genes expression involves in starch synthase (GmAGP1, GmUGP1-1, GmUGP1-2, GmUGP2, GmSSS1-1, and GmSSS1-2) were quantitative analysis. The relative expression levels of all nine genes for sucrose phosphate synthase (2), sucrose synthase (1), and starch synthase (6) were up-regulated with increasing light intensity, and the expression of GmSPS1 and GmSPS2, and GmSS1, and GmAGP1, GmUGP1-1, GmUGP1-2, GmUGP2, GmSSS1-1, and GmSSS1-2, were 1.5 and 1.6, and 5.0 (Figures 5A,B), and 8.3 (Figure 5C), 4.0, 1.8, 3.5, 5.3, and 1.5 (Figures 5D–I), respectively folds in treatment L400 compared with the L100 treatment.
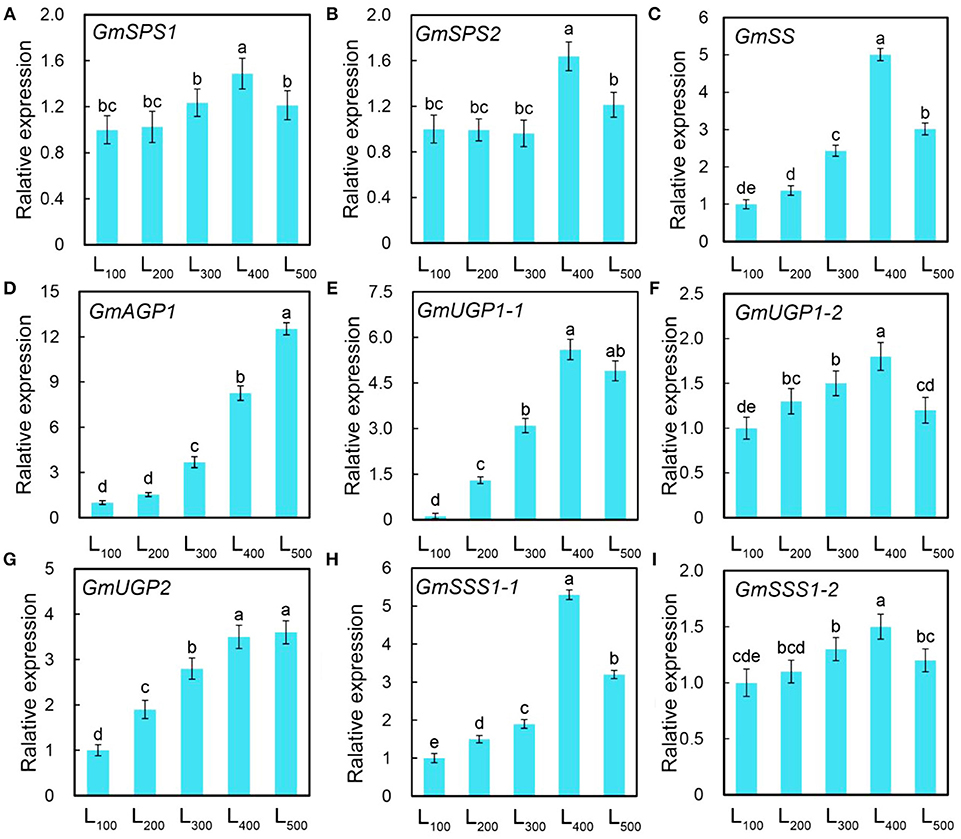
Figure 5. Changes in gene expression level of soybean plants as affected by different light intensity treatments. Gene expression of sucrose phosphate synthase (GmSPSJ) (A), sucrose phosphate synthase (GmSPS2) (B), sucrose synthase (GmSSJ) (C) starch synthase (GmAGPJ; D, GmUGPJ-1; E, GmUGPJ-2, F, GmUGP2,·G, GmSSSJ-1; H, and GmSSSJ-2,·I) of soybean plants under different light intensity treatments. L100, L200, L300, L400, and L500, refer 100, 200, 300, 400, and 500 μmol m−2 s−1, respectively. All the values are representative of three independent experiments. Bars show ± standard errors. Within a bar, different lowercase letters show a significant difference at 5% level, between treatments.
Photosynthetic and Chlorophyll Fluorescence Characteristics
Table 3 shows the photosynthetic (Pn) characteristics of soybean plants in response to different light intensity treatments. The maximum Pn, Gs, and Ci, and Tr, values of soybean plants, appeared in treatments L400 and L500, respectively than those in L100, was 14.43 μ mol CO2 m−2 s−1, 0.59 mol H2O m−2 s−1, and 467.3 mol CO2 mol−1, and 1.93 mmol H2O m−2 s−1. Pn, Gs, and Ci, and Tr, of soybean plants, were increased by 58, 84, and 12%, and 57% under L400 and L500, respectively compared to the corresponding values under L100. This increase in net photosynthetic rate indicating that light intensity is positively related with the decrease abaxial leaf petiole angle and chlorophyll contents, as light intensity (L400 and L500) significantly decreased abaxial leaf petiole angle and chlorophyll contents in soybean plants.
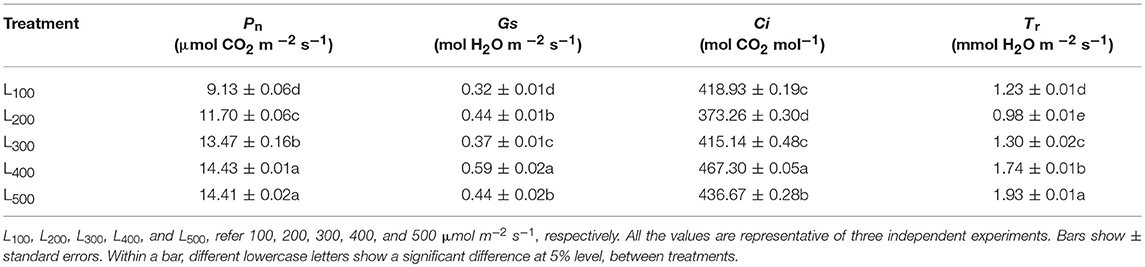
Table 3. Effect of different light intensity treatments on photosynthetic characteristics of soybean plants.
The fate of absorbed radiation energy in soybean leaves was studied in response to different light treatments (Table 4). In this experiment, the chlorophyll fluorescence parameters including Fv/Fm, NPQ, qP, ΦPSII, and ETR were significantly changed in different light intensity treatments. The Fv/Fm, NPQ, qP, ΦPSII, and ETR of soybean leaves under L400 and L500 were significantly higher than those in L100. Furthermore, L400 considerably increased the quantum yields of Fv/Fm, NPQ, qP, ΦPSII, and ETR by 9, 4, 24%, and 24, and 24% respectively, as compared to those under L100, indicating that improve light environment play an important role in improving the chlorophyll fluorescence parameters and photosynthetic capacity of soybean plants.
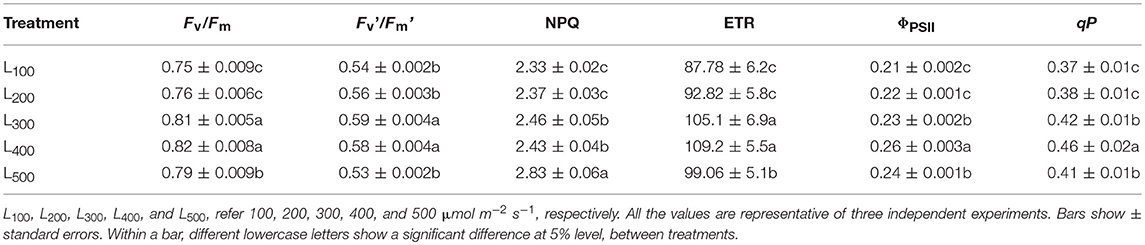
Table 4. Effect of different light intensity treatments on chlorophyll fluorescence characteristics of soybean plants.
Yield and Yield Components
In our study, there was a significant impact of different light intensity treatments on seed yield of soybean plants Table 5. The highest seed yield, 10.1 g p−1, was recorded in the L400 treatment. Relative to L100, soybean plants in L400 obtained a higher seed yield. Yield components also changed among different treatments. The effects of light intensity on pod number per plant (PNP), seed number per plant (SNP) and 100-seed weight (SW) were significant, PNP and SNP under L400 were significantly higher than that in other treatments. Meanwhile, SW was considerably heavier in L100 as compared to L400 treatment. Overall, light treatment L400 increased the PNP and SNP by 70 and 64% as compared to L100, and L100 enhanced the SW by 19% than L400 treatment Table 5.
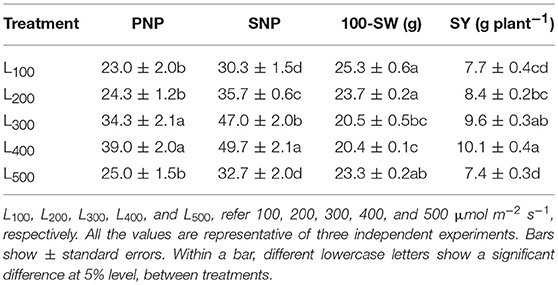
Table 5. Effect of different light intensity treatments on pod number per plant (PNP), seed number per plant (SNP), 100-seed weight (SW), and seed yield per plant (SY) of soybean plants.
Discussion
Variations in Light Intensity: Their Effects on Morphological Characteristics of Soybean
The morphology of crops has certain plasticity, and corresponding adaptation mechanisms exist under different environmental conditions (Gong et al., 2015). Use of higher plant population and intercropping systems are the effective ways for increasing the crop yields especially in developing countries (Xie et al., 2017). However, these methods are typically obstructed by reducing light conditions (Li R. et al., 2014). Numerous reports have confirmed that shade conditions promote the upward growth of stems and petiole while reducing the plant leaf area (Kurepin et al., 2007; Gommers et al., 2013). However, a few experiments pay attention to the impact of changing light intensity on plant morphology. In our experiment, a gradual increase in light intensity significantly improved the stem diameter, PB, and root to shoot ratio, and decreased the plant height and hypocotyl length of soybean plants. These results indicated that any change in light intensity directly affects the morphological parameters of soybean and low light conditions negatively affected the soybean morphology by increasing plant height and reducing stem diameter which in turn caused soybean lodging especially under intercropping conditions (Liu et al., 2016). Similarly, in previous study it has been reported that decrease light intensity significantly changed the soybean morphology by reducing plant dry matter production (Yang et al., 2014). In addition, the plant height, stem diameter, and PB of soybean showed varying responses to different light intensity treatments, and these parameters may be regulated by molecular regulation networks and endogenous plant hormones (Vandenbussche et al., 2005; Sheerin and Hiltbrunner, 2017). Overall, these results showed that L400 greatly improved the soybean morphology, and it is important to increase PB and stem diameter as compared to L100.
Variations in Light Intensity: Their Effects on Leaf Anatomy of Soybean
Leaves are the main part of photosynthesis and any changes in leaf anatomy positively or negatively affected the plants photosynthesis under prevailing conditions. It is apparent that the environmental impacts on plant leaf structure changes for every environmental factor. Results of this study confirm earlier findings (Wu et al., 2017) that improve leaf structure can obtain in crop plants which have been planted under strong light than shading conditions. In addition, higher light intensity mostly increases leaf thickness, palisade tissues thickness, and spongy tissues thickness of leaves as seen in our experiment and previous studies (Fan et al., 2018). This improvement in leaf thickness maybe linked with the increase in mesophyll tissue and lower light intensity produced leaves with large cell gap and loose cell arrangement, therefore palisade tissues and spongy tissues thickness decreases, it might be due to the reduced cell growth and cell layer number in palisade tissues (Kalve et al., 2014). Moreover, the improve light intensity increased the palisade tissue elongation process which enhanced the chloroplast channel area through which carbon dioxide enters, consequently the thickness of leaves and photosynthetic capacity of soybean leaves significantly strengthen (Terashima et al., 2006; Wu et al., 2016) under L400 and L500 treatments. On average, the differences in leaf anatomy under different light intensity treatments suggesting that leaf structural components are the main targets of light and by making adjustments in leaf anatomy plants can perform better under shade stress conditions.
Variations in Light Intensity: Their Effects on Leaf Angle and Carbohydrate Contents
The results of this experiment contribute to better understand soybean leaf angle and leaf movement (epinastic or hyponastic) behavior under changing light intensity treatments. Normally, leaf angle is neglected in studies of photosynthesis while it is the most important factor which influences the process of photosynthesis (Larbi et al., 2015). Obviously, crop leaves with orientations more horizontally to stem or perpendicular to solar radiations will absorb higher amount of solar radiations than those with more perpendicular to stem or parallel orientations to solar radiation (Lovelock et al., 1992); therefore, changes leaf angle can be indicator of plant response to different light intensities available under field conditions. In the present study, the higher light intensity significantly increased leaf angle by decreasing abaxial leaf petiole angle between stem (Figure 6) and leaf in L500 treatment than those of under L100. The increase in light intensity increased the PB as well as leaf biomass and area, which in result increase leaf angle due to the higher gravitational force on those leaves which had more leaf biomass as compared to those which had less leaf biomass. Similarly, Msallem (2002) reported that higher leaf angle under normal light than those under shading conditions. On the contrary, Larbi et al. (2015) found higher leaf angle under low photosynthetically active radiations (PAR) interception as compared with their corresponding values in higher PAR interception condition. However, in accordance to our results van Zanten et al. (2009c) confirmed that increasing light intensity decreased the abaxial leaf petiole angle and also restores many other plant traits associated with leaf structure (Millenaar et al., 2009; van Zanten et al., 2009c). Furthermore, carbohydrate content is the direct expression of the strong photosynthesis. Plants translocate sugar from photosynthesizing leaves to food storing cells that decides the physical fitness of plants (Amiard et al., 2005). Several past experiments concluded the role of light intensity for the synthesis of sucrose, starch, and total soluble sugar content in plants (Preiss, 1998; Michalska et al., 2009). In our results, the sucrose, starch, and total soluble sugar content were significantly increased with increasing light intensity. These results were in agreement with the previous studies (Pilkington et al., 2015). The increase in light intensity played an important role in regulating the enzymes related to sucrose and starch (Eliyahu et al., 2015). Similarly, former studies have reported that cloudy days and low light conditions reduced the soluble sugar content in leaves (Lichtenthaler et al., 1981; Mengin et al., 2017).
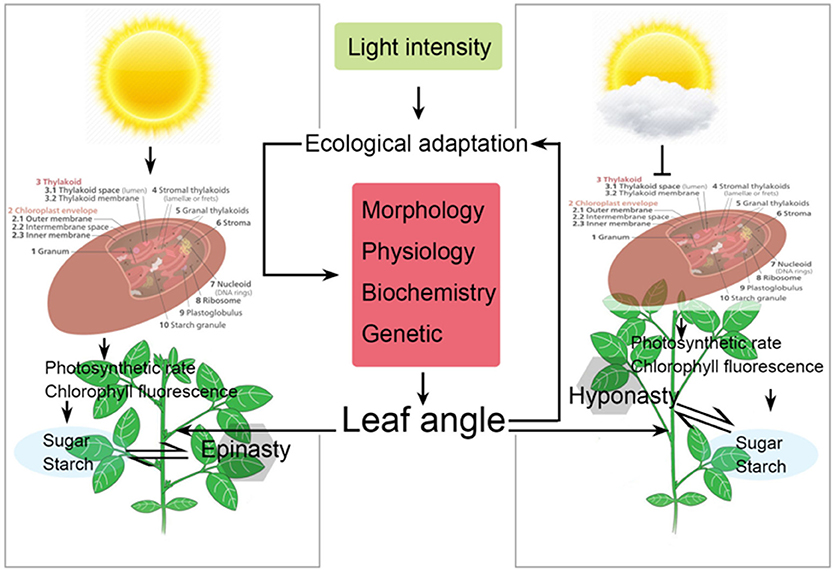
Figure 6. Schematic representation of changes in leaf orientation (leaf angle), physiology, and photosynthetic characteristics of soybean plants as affected by different light intensity treatments. Arrows represent the regulating directions of light intensity on soybean growth and carbon synthesis in this paper. Suppression arrow represent that reduce light intensity which negatively affect the differential growth and carbon synthesis in soybean plants by reducing the chloroplast efficiency.
Variations in Light Intensity: Their Effects on Chlorophyll Content and Chloroplast Structure
One of the plant leaf traits which is most affected by variations in light intensity or shading is Chl a, Chl b, Chl a + b, and Car contents. In our study, significant changes were observed in Chl a, Chl b, Chl a + b, and Car contents, and chlorophyll contents were increased with the increase in light intensity and these results were directly associated with leaf thickness (Figure 6), our results are consistent with previously reported results (Wittmann et al., 2001; Fan et al., 2018). Conversely, several other studies claimed that chlorophyll contents increase with the reduction in light intensity, especially contents of Chl b (Li T. et al., 2014).
Chloroplast ultrastructure controls the photosynthetic performance of crops under changing environmental conditions (Shao et al., 2014). In our study, the number of chloroplast and grana were increased significantly under higher light intensity L400 and L500 treatments as compared to lower light treatment L100, which suggests the beneficial effect of L400 and L500 treatments on photosynthetic apparatus of plant (chloroplast ultrastructure), our findings in line with the results of Yin et al. (2012). Anderson et al. (2008) reported the contradictory results and lower grana number in chloroplast was found under higher light intensity. Furthermore, the improved structure of chloroplast under higher light intensity treatments suggested that it might develop the shade-tolerant mechanism in soybean plants, especially under low light conditions. Therefore, the optimum light intensity (L400) improved the chloroplast ultrastructure and arrangement of soybean leaves.
Variations in Light Intensity: Their Effects on Photosynthetic and Chlorophyll Fluorescence Characteristics
In addition to the effects of light intensity on morphology, leaf anatomy and chloroplast structure, our findings demonstrate that deleterious impacts of low light abolished by optimum light conditions. There are many reasons why crop plants in shading conditions carbon would be limited. For example, Yang et al. (2017) reported that soybeans assimilate demand increased while photosynthetic capacity decreased under shading conditions (Su et al., 2014). In this study, the increase in light intensity led to enhance the net photosynthetic rate, stomatal conductance, intercellular carbon dioxide levels, and transpiration rate of soybean plants. Thus, this showed that the improved photosynthetic parameters enhanced the carbon gain and promoted the soybean growth (Liao et al., 2005). Moreover, these results suggesting that the increase in net photosynthetic rate under L400 and L500 treatments may be due to the increase in stomatal opening and the changes in net photosynthetic rate were closely associated with the stomatal opening.
Increased photosynthetic capacity is always accompanied with high quantity of electrons passing through PSII (Yao et al., 2017). Chl fluorescence characteristics are one of the main important factors in photosynthetic regulation and plant responses to environmental conditions because of its sensitivity and convenience (Dai et al., 2009). Former studies have reported that low light intensity or shade results in low photosynthesis due to the reduction in qp, PSII and ETR while it increases the NPQ (Huang et al., 2011; Yao et al., 2017). In our present study, similar results were obtained, however, improved Chl fluorescence characteristics were measured under L400 and L500 treatments. These results reveal that higher light intensity enhances the efficiency of PSII and ETR that could enhance the photosynthesis by improving the energy transport from PSII to PSI (Figure 6), our results are consistent with previously reported results of Yang et al. (2018b).
Variations in Light Intensity: Their Effects on Enzymatic Activity and Genes Expression
Results showed from this experiment demonstrating that the enzymatic activities of key enzyme related to sucrose synthesis (SS, SPS, and PEPC), starch synthesis (ADPGPPase, UDPGPPase, and SSS) and photosynthesis (rubisco) process significantly increased with increasing light intensity treatments, higher levels activities of these enzymes were determined under L400 and L500 treatments. These results are in line with the findings of previous reports (Bahaji et al., 2015). In addition, changes in light intensity equally played major roles in accelerating the activities of SS, SPS, and PEPC (Ciereszko et al., 2001). Therefore, plant biomass and net photosynthetic rate, which were largely regulated by improving light intensity might be affected the activities of SS, SPS, and PEPC, and ADPGPPase, UDPGPPase, and SSS, and controlled cell elongation and division in plants by regulating the expression of many genes. These results indicating that the higher sucrose and starch contents were increased by the activities of SS, SPS, and PEPC, and ADPGPPase, UDPGPPase, and SSS with other plant response to higher light intensity treatments, and in our case, using the L400 treatment, can be considered to more effective at the enzymatic activities. Furthermore, the loss of Rubisco activity was recognized to be very early and fast response of crop plants to shade stress (Servaites et al., 1986). Whereas, in this research the activity of Rubisco was increased with increasing light intensity, similar results were reported by Carmo-Silva and Salvucci (2013). This higher rubisco activity under higher light intensity treatments showed that the higher net photosynthetic rate of soybean plants directly correlated with rubisco activity under changing environments (Zhang et al., 2002). The relative expression levels of GmSPS1, GmSPS2, GmSS1, GmAGP1, GmUGP1-1, GmUGP1-2, GmUGP2, GmSSS1-1, and GmSSS1-2 were enhanced and increased the production of sucrose and starch to improve the soybean growth and development. The activities of SPS and SS, and ADPGPPase, UDPGPPase, and SSS enzymes were directly related with the upregulation of these important genes, therefore, in soybeans GmSPS1, GmSPS2, GmSS1, GmAGP1, GmUGP1-1, GmUGP1-2, GmUGP2, GmSSS1-1, and GmSSS1-2 were the important regulators of carbon production and of soybean better growth under low light conditions.
Variations in Light Intensity: Their Effects on Yield and Yield Components
Previously, it has been reported that decrease light intensity or shading conditions significantly decreased the soybean yield and yield related parameters (Wu et al., 2016). However, in our study, increasing light intensity treatments had significant on yield and yield related parameters, and maximum PNP, SNP, and seed yield of soybean plants were recorded under L400 treatment as compared to other treatments. This is might be due to the higher net photosynthetic rate and biomass accumulation, similar to our results Yang et al. (2017) reported that soybean seed yield significantly reduced under shading conditions as compared to normal conditions. Moreover, light enrichment treatments significantly increased the pod number and seed yield of soybean reported by Liu et al. (2008). Hence, PNP and SNP of soybean plants might be improved under high light intensity treatments (L400 and L500), these results implied that higher light intensity at soybean canopy can significantly improve the morphological traits, leaf anatomical characteristics, photosynthetic, and chlorophyll florescence parameters which in turn considerably increased the seed yield by increasing the PNP and SNP.
Conclusion
The significant effects of increasing light intensity on soybean plants have been extensively investigated, but rarely scientist have studied the impacts of different light intensity treatments on soybean to understand the optimum requirement of light for better growth and development. Here, we demonstrated that 400 μmol m−2 s−1 is the threshold level of light intensity which strongly interacts with soybean plant responses to treatment L400 by modifying its carbon balance (Figure 6). Increased light intensity significantly improved the morphological parameters, carbon assimilation rate (production of sucrose and starch), enzymatic activities of key enzymes by up-regulating the important sucrose synthase genes. These energetically expensive pathways positively modify carbon balance which is considerably improved with optimum light intensity. Because the dose response to changed light intensity varies between varieties and crop species, it is likely to play an important role in crop plant strategies and community dynamics. In addition, as compared to low light treatment (L100), higher light improved the leaf structure and anatomy which in turn significantly increased the photosynthetic and chlorophyll florescence especially quantum yield of PSII which in turn substantially increased the yield and yield related parameters. Therefore, we found that 400 and 500 μmol m−2 s−1 is the optimum light intensity which changed the leaf orientation and adjusts leaf angle to perpendicular to coming light, consequently soybean plants grows well under prevailing conditions.
Author Contributions
LF, MR, and ZL performed the experiment. YC and MK performed some analysis. LF, MR, JD, WL, XW, CS, LY, ZZ, and SY conceived the original research plans. FY and WY designed the experiments, analyzed the data, and wrote the article. All authors read and approved the final manuscript.
Funding
This work was funded by the National Key Research and Development Program of China (2016YFD0300602, 2016YFD0300209), the National Nature Science Foundation (31571615), and Program on Industrial Technology System of National Soybean (CARS-04-PS19).
Conflict of Interest Statement
The authors declare that the research was conducted in the absence of any commercial or financial relationships that could be construed as a potential conflict of interest.
Supplementary Material
The Supplementary Material for this article can be found online at: https://www.frontiersin.org/articles/10.3389/fpls.2018.01952/full#supplementary-material
References
Amiard, V., Mueh, K. E., Demmig-Adams, B., Ebbert, V., Turgeon, R., and Adams W. W. III (2005). Anatomical and photosynthetic acclimation to the light environment in species with differing mechanisms of phloem loading. Proc. Natl. Acad. Sci. U.S.A. 102, 12968–12973. doi: 10.1073/pnas.0503784102
Anderson, J. M., Chow, W. S., and De Las Rivas, J. (2008). Dynamic flexibility in the structure and function of photosystem II in higher plant thylakoid membranes: the grana enigma. Photosynth. Res. 98, 575–587. doi: 10.1007/s11120-008-9381-3
Bahaji, A., Sanchez-Lopez, A. M., De Diego, N., Munoz, F. J., Baroja-Fernandez, E., Li, J., et al. (2015). Plastidic phosphoglucose isomerase is an important determinant of starch accumulation in mesophyll cells, growth, photosynthetic capacity, and biosynthesis of plastidic cytokinins in Arabidopsis. PLoS ONE 10:e0119641. doi: 10.1371/journal.pone.0119641
Carmo-Silva, A. E., and Salvucci, M. E. (2013). The regulatory properties of Rubisco activase differ among species and affect photosynthetic induction during light transitions. Plant Physiol. 161, 1645–1655. doi: 10.1104/pp.112.213348
Ciereszko, I., Johansson, H., and Kleczkowski, L. A. (2001). Sucrose and light regulation of a cold-inducible UDP-glucose pyrophosphorylase gene via a hexokinase-independent and abscisic acid-insensitive pathway in Arabidopsis. Biochem. J. 354, 67–72. doi: 10.1042/bj3540067
Dai, Y. J., Shen, Z. G., Ying, L., Wang, L. L., Hannaway, D., and Lu, H. (2009). Effects of shade treatments on the photosynthetic capacity, chlorophyll fluorescence, and chlorophyll content of Tetrastigma hemsleyanum Diels et Gilg. Environ. Exp. Bot. 65, 177–182. doi: 10.1016/j.envexpbot.2008.12.008
Delfine, S., Alvino, A., Villani, M. C., and Loreto, F. (1999). Restrictions to carbon dioxide conductance and photosynthesis in spinach leaves recovering from salt stress. Plant Physiol. 119, 1101–1106. doi: 10.1104/pp.119.3.1101
Doehlert, D. C., Kuo, T. M., and Felker, F. C. (1988). Enzymes of sucrose and hexose metabolism in developing kernels of two inbreds of maize. Plant Physiol. 86, 1013–1019.
Eliyahu, E., Rog, I., Inbal, D., and Danon, A. (2015). ACHT4-driven oxidation of APS1 attenuates starch synthesis under low light intensity in Arabidopsis plants. Proc. Natl. Acad. Sci. U.S.A. 112, 12876–12881. doi: 10.1073/pnas.1515513112
Evans, J. R., and Seemann, J. R. (1989). “The allocation of protein nitrogen in the photosynthetic apparatus: costs, consequences, and control,” in Photosynthesis: Proceedings of the C.S. French Symposium on Photosynthesis, ed W. R. Briggs (Stanford, CA: A.R. Liss), 183–205.
Falster, D. S., and Westoby, M. (2003). Leaf size and angle vary widely across species: what consequences for light interception? New Phytol. 158, 509–525. doi: 10.1046/j.1469-8137.2003.00765.x
Fan, Y., Chen, J., Cheng, Y., Raza, M. A., Wu, X., Wang, Z., et al. (2018). Effect of shading and light recovery on the growth, leaf structure, and photosynthetic performance of soybean in a maize-soybean relay-strip intercropping system. PLoS ONE 13:e0198159. doi: 10.1371/journal.pone.0198159
Fehr, W. R., and Caviness, C. E. (1977). Stages of Soybean Development. Special Report 80, Iowa Agricultural Experiment Station, Iowa Cooperative External Series, Iowa State University, Ames.
Fernandez, O., Ishihara, H., George, G. M., Mengin, V., Flis, A., Sumner, D., et al. (2017). Foliar starch turnover occurs in long days and in falling light at the end of the day. Plant Physiol. 2017:00601. doi: 10.1104/pp.17.00601
Fondy, B. R., Geiger, D. R., and Servaites, J. C. (1989). Photosynthesis, carbohydrate metabolism, and export in Beta vulgaris L. and Phaseolus vulgaris L. during square and sinusoidal light regimes. Plant Physiol. 89, 396–402. doi: 10.1104/pp.89.2.396
Franklin, K. A. (2009). Light and temperature signal crosstalk in plant development. Curr. Opin. Plant Biol. 12, 63–68. doi: 10.1016/j.pbi.2008.09.007
Fu, Q. A., and Ehleringer, J. R. (1989). Heliotropic leaf movements in common beans controlled by air temperature. Plant Physiol. 91, 1162–1167. doi: 10.1104/pp.91.3.1162
Gommers, C. M., Visser, E. J., St Onge, K. R., Voesenek, L. A., and Pierik, R. (2013). Shade tolerance: when growing tall is not an option. Trends Plant Sci. 18, 65–71. doi: 10.1016/j.tplants.2012.09.008
Gong, W. Z., Jiang, C. D., Wu, Y. S., Chen, H. H., Liu, W. Y., Yang, W. Y., et al. (2015). Tolerance vs . avoidance: two strategies of soybean (Glycine max) seedlings in response to shade in intercropping. Photosynthetica. 53, 259–268. doi: 10.1007/s11099-015-0103-8
Gray, W. M., Ostin, A., Sandberg, G., Romano, C. P., and Estelle, M. (1998). High temperature promotes auxin-mediated hypocotyl elongation in Arabidopsis. Proc. Natl. Acad. Sci. U.S.A. 95, 7197–7202. doi: 10.1073/pnas.95.12.7197
Hangarter, R. P. (1997). Gravity, light and plant form. Plant Cell Environ. 20, 796–800. doi: 10.1046/j.1365-3040.1997.d01-124.x
Hoagland, D. R., and Arnon, D. I. (1950). Growing plants without soil by the water-culture method. Circ. Calif. Agric. Exp. Stn. 367, 1–24.
Huang, D., Wu, L., Chen, J. R., and Dong, L. (2011). Morphological plasticity, photosynthesis and chlorophyll fluorescence of Athyrium pachyphlebium at different shade levels. Photosynthetica 49, 611–618. doi: 10.1007/s11099-011-0076-1
Kalve, S., Fotschki, J., Beeckman, T., Vissenberg, K., and Beemster, G. T. (2014). Three-dimensional patterns of cell division and expansion throughout the development of Arabidopsis thaliana leaves. J. Exp. Bot. 65, 6385–6397. doi: 10.1093/jxb/eru358
Kao, W. Y., Tsai, T. T., and Shih, C. N. (2003). Photosynthetic gas exchange and chlorophyll a fluorescence of three wild soybean species in response to NaCl treatments. Photosynthetica 41, 415–419. doi: 10.1023/B:PHOT.0000015466.22288.23
King, D. A. (1997). The functional significance of leaf angle in Eucalyptus. Aust. J. Bot. 45, 619–639. doi: 10.1071/BT96063
Kong, D. X., Li, Y. Q., Wang, M. L., Bai, M., Zou, R., Tang, H., et al. (2016). Effects of light intensity on leaf photosynthetic characteristics, chloroplast structure, and alkaloid content of Mahonia bodinieri (Gagnep.) Laferr. Acta Physiol. Plant. 38:120. doi: 10.1007/s11738-016-2147-1
Kozuka, T., Horiguchi, G., Kim, G. T., Ohgishi, M., Sakai, T., and Tsukaya, H. (2005). The different growth responses of the Arabidopsis thaliana leaf blade and the petiole during shade avoidance are regulated by photoreceptors and sugar. Plant Cell Physiol. 46, 213–223. doi: 10.1093/pcp/pci016
Kurepin, L. V., Emery, R. J., Pharis, R. P., and Reid, D. M. (2007). The interaction of light quality and irradiance with gibberellins, cytokinins and auxin in regulating growth of Helianthus annuus hypocotyls. Plant Cell Environ. 30, 147–155. doi: 10.1111/j.1365-3040.2006.01612.x
Kutík, J., Holá, D., Vičánková, A., Šmídová, M., Kočová, M., Körnerová, M., et al. (2001). The heterogeneity of structural and functional photosynthetic characteristics of mesophyll chloroplasts in various parts of mature or senescing leaf blade of two maize (Zea Mays L.) genotypes. Photosynthetica 39, 497–506. doi: 10.1023/A:1015639609268
Larbi, A., Vazquez, S., El-Jendoubi, H., Msallem, M., Abadia, J., Abadía, A., et al. (2015). Canopy light heterogeneity drives leaf anatomical, eco-physiological, and photosynthetic changes in olive trees grown in a high-density plantation. Photosynth. Res. 123, 141–155. doi: 10.1007/s11120-014-0052-2
Li, R., Wen, T., Tang, Y. P., Sun, X., and Xia, C. (2014). Effect of shading on photosynthetic and chlorophyll fluorescence characteristics of soybean. Acta Pratac. Sin. 23, 198–206.
Li, T., Liu, L. N., Jiang, C. D., Liu, Y. J., and Shi, L. (2014). Effects of mutual shading on the regulation of photosynthesis in field-grown sorghum. J. Photochem. Photobiol. B 137, 31–38. doi: 10.1016/j.jphotobiol.2014.04.022
Liang, J. S., Cao, X. Z., Xu, S., Zhu, Q. S., and Song, P. (1994). Studies on the relations between grain sink intensity and starch accumulation of rice. Acta Agron. Sin. 20, 685–691.
Liao, J. X., Ge, Y., Huang, C. C., Zhang, J., Liu, Q. X., Chang, J., et al. (2005). Effects of irradiance on photosynthetic characteristics and growth of Mosla chinensis and M. scabra. Photosynthetica 43, 111–115. doi: 10.1007/s11099-005-1115-6
Lichtenthaler, H. K., Buschmann, C., Doll, M., Fietz, H. J., Bach, T., Kozel, U., et al. (1981). Photosynthetic activity, chloroplast ultrastructure, and leaf characteristics of high-light and low-light plants and of sun and shade leaves. Photosynth. Res. 2, 115–141. doi: 10.1007/BF00028752
Liu, B., Wang, C., Jin, J., Liu, J. D., Zhang, Q. Y., Liu, X. B., et al. (2008). Effects of light enrichment and shade during reproductive stage on yield and yield components in soybean. Soy. Sci. 27, 764–772.
Liu, W., Deng, Y., Hussain, S., Zou, J., Yuan, J., Luo, L., et al. (2016). Relationship between cellulose accumulation and lodging resistance in the stem of relay intercropped soybean [Glycine max (L.) Merr.]. Field Crop Res. 196, 261–267. doi: 10.1016/j.fcr.2016.07.008
Lovelock, C. E., Clough, B. F., and Woodrow, I. E. (1992). Distribution and accumulation of ultraviolet-radiation-absorbing compounds in leaves of tropical mangroves. Planta 188, 143–154. doi: 10.1007/BF00216808
Maliakal, S. K., Mcdonnell, K., Dudley, S. A., and Schmitt, J. (1999). Effects of red to far-red ratio and plant density on biomass allocation and gas exchange in impatiens capensis. Int. J. Plant Sci.160, 723–733. doi: 10.1086/314157
Mauser, H., King, W. A., Gready, J. E., and Andrews, T. J. (2001). CO(2) fixation by Rubisco: computational dissection of the key steps of carboxylation, hydration, and C-C bond cleavage. J. Am. Chem. Soc. 123, 10821–10829. doi: 10.1021/ja011362p
Maxwell, K., and Johnson, G. N. (2000). Chlorophyll fluorescence–a practical guide. J. Exp. Bot. 51, 659–668. doi: 10.1093/jexbot/51.345.659
Mengin, V., Pyl, E. T., Alexandre Moraes, T., Sulpice, R., Krohn, N., Encke, B., et al. (2017). Photosynthate partitioning to starch in Arabidopsis thaliana is insensitive to light intensity but sensitive to photoperiod due to a restriction on growth in the light in short photoperiods. Plant Cell Environ. 40, 2608–2627. doi: 10.1111/pce.13000
Michalska, J., Zauber, H., Buchanan, B. B., Cejudo, F. J., and Geigenberger, P. (2009). NTRC links built-in thioredoxin to light and sucrose in regulating starch synthesis in chloroplasts and amyloplasts. Proc. Natl. Acad. Sci. U.S.A. 106, 9908–9913. doi: 10.1073/pnas.0903559106
Millenaar, F. F., van Zanten, M., Cox, M. C., Pierik, R., Voesenek, L. A., and Peeters, A. J. (2009). Differential petiole growth in Arabidopsis thaliana: photocontrol and hormonal regulation. New Phytol. 184, 141–152. doi: 10.1111/j.1469-8137.2009.02921.x
Msallem, M. (2002). Etude de la Juvénilité Chez L'olivier (Olea europaea L.). Aspects Morphologiques, Anatomiques et Biochimiques. Thèse Doctorat d'Etat, Institut National Agronomique de Tunisie.
Mullen, J. L., Weinig, C., and Hangarter, R. P. (2006). Shade avoidance and the regulation of leaf inclination in Arabidopsis. Plant Cell Environ. 29, 1099–1106. doi: 10.1111/j.1365-3040.2005.01484.x
Pharis, R. P., and King, R. W. (1985). Gibberellins and reproductive development in seed plants. Annu. Rev. Plant Physiol. 36, 517–568. doi: 10.1146/annurev.pp.36.060185.002505
Pierik, R., Cuppens, M. L., Voesenek, L. A., and Visser, E. J. (2004). Interactions between ethylene and gibberellins in phytochrome-mediated shade avoidance responses in tobacco. Plant Physiol. 136, 2928–2936. doi: 10.1104/pp.104.045120
Pilkington, P. D., Milne, L. C., Cairns, K. E., Lewis, J., and Whelan, T. A. (2015). Modifiable partner factors associated with perinatal depression and anxiety: a systematic review and meta-analysis. J. Affect. Disord. 178, 165–180. doi: 10.1016/j.jad.2015.02.023
Preiss, J. (1998). “Biosynthesis of starch and its regulation,” in Plant Carbohydrates I. Encyclopedia of Plant Physiology (New Series), Vol. 13/A, eds F. A. Loewus and W. Tanner (Berlin; Heidelberg: Springer), 397–417.
Ranjbarfordoei, A., Samson, R., and Damme, P. V. (2006). Chlorophyll fluorescence performance of sweet almond [Prunus dulcis (Miller) D. Webb] in response to salinity stress induced by NaCl. Photosynthetica 44, 513–522. doi: 10.1007/s11099-006-0064-z
Rascher, U., Liebig, M., and Lüttge, U. (2010). Evaluation of instant light-response curves of chlorophyll fluorescence parameters obtained with a portable chlorophyll fluorometer on site in the field. Plant Cell Environ. 23, 1397–1405. doi: 10.1046/j.1365-3040.2000.00650.x
Redondo-Gomez, S., Mateos-Naranjo, E., Davy, A. J., Fernandez-Munoz, F., Castellanos, E. M., Luque, T., et al. (2007). Growth and photosynthetic responses to salinity of the salt-marsh shrub Atriplex portulacoides. Ann. Bot. 100, 555–563. doi: 10.1093/aob/mcm119
Rijkers, T., Pons, T. L., and Bongers, F. (2010). The effect of tree height and light availability on photosynthetic leaf traits of four neotropical species differing in shade tolerance. Funct. Ecol. 14, 77–86. doi: 10.1046/j.1365-2435.2000.00395.x
Schreiber, U., Bilger, W., and Neubauer, C. (1995). “Chlorophyll Fluorescence as a nonintrusive indicator for rapid assessment of in vivo photosynthesis,” in Ecophysiology of Photosynthesis. Springer Study Edition, Vol. 100, eds E. D. Schulze and M. M. Caldwell (Berlin; Heidelberg: Springer), 49–70.
Seemann, J. R., and Sharkey, T. D. (1986). Salinity and nitrogen effects on photosynthesis, ribulose-1,5-bisphosphate carboxylase and metabolite pool sizes in Phaseolus vulgaris L. Plant Physiol. 82, 555–560. doi: 10.1104/pp.82.2.555
Servaites, J. C., Geiger, D. R., Tucci, M. A., and Fondy, B. R. (1989). Leaf carbon metabolism and metabolite levels during a period of sinusoidal light. Plant Physiol. 89, 403–408. doi: 10.1104/pp.89.2.403
Servaites, J. C., Parry, M. A., Gutteridge, S., and Keys, A. J. (1986). Species variation in the predawn inhibition of ribulose-1,5-bisphosphate carboxylase/oxygenase. Plant Physiol. 82, 1161–1163. doi: 10.1104/pp.82.4.1161
Shao, Q., Wang, H., Guo, H., Zhou, A., Huang, Y., Sun, Y., et al. (2014). Effects of shade treatments on photosynthetic characteristics, chloroplast ultrastructure, and physiology of Anoectochilus roxburghii. PLoS ONE 9:e85996. doi: 10.1371/journal.pone.0085996
Sheerin, D. J., and Hiltbrunner, A. (2017). Molecular mechanisms and ecological function of far-red light signalling. Plant Cell Environ. 40, 2509–2529. doi: 10.1111/pce.12915
Smalle, J., Haegman, M., Kurepa, J., Van Montagu, M., and Straeten, D. V. (1997). Ethylene can stimulate Arabidopsis hypocotyl elongation in the light. Proc. Natl. Acad. Sci. U.S.A. 94, 2756–2761. doi: 10.1073/pnas.94.6.2756
Smith, H. (2000). Phytochromes and light signal perception by plants–an emerging synthesis. Nature 407, 585–591. doi: 10.1038/35036500
Stepien, P., and Kłbus, G. (2006). Water relations and photosynthesis in Cucumis sativus L. leaves under salt stress. Biol. Plant. 50, 610–616. doi: 10.1007/s10535-006-0096-z
Stitt, M., and Zeeman, S. C. (2012). Starch turnover: pathways, regulation and role in growth. Curr. Opin. Plant Biol. 15, 282–292. doi: 10.1016/j.pbi.2012.03.016
Su, B. Y., Song, Y. X., Song, C., Cui, L., Yong, T. W., and Yang, W. Y. (2014). Growth and photosynthetic responses of soybean seedlings to maize shading in relay intercropping system in Southwest China. Photosynthetica 52, 332–340. doi: 10.1007/s11099-014-0036-7
Terashima, I., Hanba, Y. T., Tazoe, Y., Vyas, P., and Yano, S. (2006). Irradiance and phenotype: comparative eco-development of sun and shade leaves in relation to photosynthetic CO2 diffusion. J. Exp. Bot. 57, 343–354. doi: 10.1093/jxb/erj014
van Zanten, M., Millenaar, F. F., Cox, M. C., Pierik, R., Voesenek, L. A., and Peeters, A. J. M. (2009a). Auxin perception and polar auxin transport are not always a prerequisite for differential growth. Plant Signal. Behav. 4, 899–901. doi: 10.4161/psb.4.9.9528
van Zanten, M., Pons, T. L., Janssen, J. M., Voesenek, L. C. J., and Peeters, A. J. M. (2010). On the relevance and control of leaf angle. Crit. Rev. Plant. Sci. 29, 300–316. doi: 10.1080/07352689.2010.502086
van Zanten, M., Voesenek, L. A., Peeters, A. J., and Millenaar, F. F. (2009b). Hormone- and light-mediated regulation of heat-induced differential petiole growth in Arabidopsis. Plant Physiol. 151, 1446–1458. doi: 10.1104/pp.109.144386
van Zanten, T. S., Cambi, A., Koopman, M., Joosten, B., Figdor, C. G., et al. (2009c). Hotspots of GPI-anchored proteins and integrin nanoclusters function as nucleation sites for cell adhesion. Proc. Natl. Acad. Sci. U.S.A. 106, 18557–18562. doi: 10.1073/pnas.0905217106
Vandenbussche, F., Pierik, R., Millenaar, F. F., Voesenek, L. A., and Van Der Straeten, D. (2005). Reaching out of the shade. Curr. Opin. Plant Biol. 8, 462–468. doi: 10.1016/j.pbi.2005.07.007
Vandenbussche, F., Vriezen, W. H., Smalle, J., Laarhoven, L. J., Harren, F. J., Van Der Straeten, D. et al. (2003). Ethylene and auxin control the Arabidopsis response to decreased light intensity. Plant Physiol. 133, 517–527. doi: 10.1104/pp.103.022665
Vasseur, F., Pantin, F., and Vile, D. (2011). Changes in light intensity reveal a major role for carbon balance in Arabidopsis responses to high temperature. Plant Cell Environ. 34, 1563–1576. doi: 10.1111/j.1365-3040.2011.02353.x
Wherley, B. G., Gardner, D. S., and Metzger, J. D. (2005). Tall fescue photomorphogenesis as influenced by changes in the spectral composition and light intensity. Crop. Sci. 45, 562–568. doi: 10.2135/cropsci2005.0562
Wittmann, C., Aschan, G., and Pfanz, H. (2001). Leaf and twig photosynthesis of young beech (Fagus sylvatica) and aspen (Populus tremula) trees grown under different light regime. Basic Appl. Ecol. 2, 145–154. doi: 10.1078/1439-1791-00047
Wu, Y., Gong, W., Wang, Y., Yong, T., Yang, F., Liu, W., et al. (2018). Leaf area and photosynthesis of newly emerged trifoliolate leaves are regulated by mature leaves in soybean. J. Plant. Res. 131, 671–680. doi: 10.1007/s10265-018-1027-8
Wu, Y., Gong, W., Yang, F., Wang, X., Yong, T., and Yang, W. (2016). Responses to shade and subsequent recovery of soya bean in maize-soya bean relay strip intercropping. Plant Prod. Sci. 15, 1–9. doi: 10.1080/1343943X.2015.1128095
Wu, Y., Gong, W., and Yang, W. (2017). Shade inhibits leaf size by controlling cell proliferation and enlargement in soybean. Sci. Rep. 7:9259. doi: 10.1038/s41598-017-10026-5
Xie, Y., Liu, Y., Wang, H., Ma, X., Wang, B., Wu, G., et al. (2017). Phytochrome-interacting factors directly suppress MIR156 expression to enhance shade-avoidance syndrome in Arabidopsis. Nat. Commun. 8:348. doi: 10.1038/s41467-017-00404-y
Yang, F., Fan, Y., Wu, X., Cheng, Y., Liu, Q., Feng, L., et al. (2018a). Auxin-to-gibberellin ratio as a signal for light intensity and quality in regulating soybean growth and matter partitioning. Front. Plant. Sci. 9:56. doi: 10.3389/fpls.2018.00056
Yang, F., Feng, L., Liu, Q., Wu, X., Fan, Y., Ali Raza, M., et al. (2018b). Effect of interactions between light intensity and red-to- far-red ratio on the photosynthesis of soybean leaves under shade condition. Environ. Exp. Bot. 150, 79–87. doi: 10.1016/j.envexpbot.2018.03.008
Yang, F., Huang, S., Gao, R., Liu, W., Yong, T., Wang, X., et al. (2014). Growth of soybean seedlings in relay strip intercropping systems in relation to light quantity and red:far-red ratio. Field Crop Res. 155, 245–253. doi: 10.1016/j.fcr.2013.08.011
Yang, F., Liao, D., Wu, X., Gao, R., Fan, Y., Ali Raza, M., et al. (2017). Effect of aboveground and belowground interactions on the intercrop yields in maize-soybean relay intercropping systems. Field Crop Res. 203, 16–23. doi: 10.1016/j.fcr.2016.12.007
Yang, F., Wang, X., Liao, D., Lu, F., Gao, R., Liu, W., et al. (2015). Yield response to different planting geometries in maize–soybean relay strip intercropping systems. Agron. J. 107:296. doi: 10.2134/agronj14.0263
Yang, L., Chen, L., Peng, H., Guo, P., Wang, P., Ma, C.-L., et al. (2012). Organic acid metabolism in Citrus grandis, leaves and roots is differently affected by nitric oxide and aluminum interactions. Sci. Hortic. 133, 40–46. doi: 10.1016/j.scienta.2011.10.011
Yao, X., Li, C., Li, S., Zhu, Q., Zhang, H., Wang, H., et al. (2017). Effect of shade on leaf photosynthetic capacity, light-intercepting, electron transfer and energy distribution of soybeans. Plant Growth Regul. 83, 1–8. doi: 10.1007/s10725-017-0307-y
Yin, L., Fristedt, R., Herdean, A., Solymosi, K., Bertrand, M., Andersson, M. X., et al. (2012). Photosystem II function and dynamics in three widely used Arabidopsis thaliana accessions. PLoS ONE 7:e46206. doi: 10.1371/journal.pone.0046206
Keywords: light intensity, sucrose synthase, soybean, starch synthase, photosynthesis
Citation: Feng L, Raza MA, Li Z, Chen Y, Khalid MHB, Du J, Liu W, Wu X, Song C, Yu L, Zhang Z, Yuan S, Yang W and Yang F (2019) The Influence of Light Intensity and Leaf Movement on Photosynthesis Characteristics and Carbon Balance of Soybean. Front. Plant Sci. 9:1952. doi: 10.3389/fpls.2018.01952
Received: 18 June 2018; Accepted: 14 December 2018;
Published: 08 January 2019.
Edited by:
Angeles Calatayud, Instituto Valenciano de Investigaciones Agrarias, SpainReviewed by:
Dawei Zhang, Sichuan University, ChinaSuriyan Cha-um, National Science and Technology Development Agency (NSTDA), Thailand
Copyright © 2019 Feng, Raza, Li, Chen, Khalid, Du, Liu, Wu, Song, Yu, Zhang, Yuan, Yang and Yang. This is an open-access article distributed under the terms of the Creative Commons Attribution License (CC BY). The use, distribution or reproduction in other forums is permitted, provided the original author(s) and the copyright owner(s) are credited and that the original publication in this journal is cited, in accordance with accepted academic practice. No use, distribution or reproduction is permitted which does not comply with these terms.
*Correspondence: Wenyu Yang, mssiyangwy@sicau.edu.cn
Feng Yang, f.yang@sicau.edu.cn
† These authors have contributed equally to this work