- 1College of Life Science, Sichuan Normal University, Chengdu, China
- 2College of Environment, Chengdu University of Technology, Chengdu, China
Environmentally induced transgenerational plasticity can increase success of progeny and thereby be adaptive if progeny experiences the similarly parental environment. The ecological and evolutionary significance of transgenerational plasticity in plant has been studied mainly in the context of sexual generations. A pot experiment using the stoloniferous herb Centella asiatica was conducted to investigate the effects of high/low light treatment experienced by parental ramets (F0 generation) on morphological and physiological properties of offspring ramets (F2 generation) as well as growth performance. Light environment experienced by parental ramets (F0 generation) significantly influenced petiole length, specific petiole length, internode length of stolon, leaf area, specific leaf area (SLA), leaf nitrogen and chlorophyll contents, potential maximum net photosynthetic rate (Pmax) in offspring ramets subjected to parental or non-parental environments even after they were detached from the parental ramets. Potential maximum net photosynthetic rate (Pmax) of offspring ramets (F2 generation) from parental ramets (F0 generation) subjected to low light treatment was significantly greater than that of offspring ramets (F2 generation) from parental ramets (F0 generation) subjected to high light treatment. Potential maximum net photosynthetic rate (Pmax) of offspring ramets (F2 generation) subjected to parental light environment was greater than that of offspring ramets (F2 generation) subjected to non-parental light environment. The greatest biomass accumulation and total stolon length were observed in offspring ramets (F2 generation) subjected to low light treatment as parental ramets (F0 generation) experienced. When parental ramets (F0 generation) were subjected to low light treatment, biomass accumulation and total stolon length of offspring ramets (F2 generation) experiencing parental light environment were significantly greater than those of offspring ramets (F2 generation) experiencing non-parental light environment. Opposite pattern was observed in offspring ramets (F2 generation) from parental ramets subjected to high light treatment. Our work provides evidence that transgenerational plasticity through both morphological and physiological flexibility was triggered across vegetative generations for stoloniferous herb C. asiatica subjected to high/low light treatment. The transgenerational plasticity can allow offspring ramets to present adaptive phenotype early without lag time in response to the current environment. Thus, it is very important for clonal plants in adapting temporally and spatially heterogeneous habitats.
Introduction
The environmental cues experienced by parents, may influence the phenotype of their progeny. This phenomenon is termed as transgenerational plasticity (Dyer et al., 2010; Fenesi et al., 2014). Transgenerational plasticity elicits phenotypic adjustments to environmental conditions experienced by sexually produced progeny. For example, soil nutrient conditions encountered by parent, affects size and germination of progeny in Senecio sp (Aarssen and Burton, 1990); the competitive ability in Plantago major and P. rugelii is related to the environmental conditions experienced by both parent and progeny (Miao et al., 1991). The parental light environment influences the life history schedule of progeny in Campanulastrum americanum (Galloway and Etterson, 2007). The defensive resistance of progeny is induced by herbivory in the parental generation of Raphanus raphanistrum (Agrawal, 2002). Transgenerational plasticity can be mediated by altered DNA methylation (Rossiter, 1996; Douhovnikoff and Dodd, 2015) or seed quality (Roach and Wulff, 1987). Thereby, transgenerational plasticity may be potentially important for evolutionary dynamics of plant population (Riska, 1989; Räsänen and Kruuk, 2007).
Transgenerational plasticity may be adaptive in progeny grown under the same environmental conditions as experienced by parent (Galloway, 2005; Galloway and Etterson, 2007; Chen et al., 2014; Latzel et al., 2014). For the monocarpic herb C. americanum, fitness of progeny grown under a parental light environment is significantly greater than that of progeny grown under a non-parental light environment (Galloway and Etterson, 2007). Similarly, nutrient conditions experienced by parent, significantly affects biomass and carbon storage of progeny in Plantago lanceolata (Latzel et al., 2014). In addition, transgenerational plasticity may be more important for plant grown under limited resource conditions (such as in soil with low water level and nutrient or in shaded habitat) than one grown under ample resource conditions (Sultan, 1996). However, the ecological and evolutionary significance of transgenerational plasticity mainly focus on studies across sexual generations.
Clonal plant can reproduce a large number of interconnected, potentially independent and genetically identical offspring ramets. Transgenerational plasticity may influence phenotype of offspring ramets (Dong et al., 2017; González et al., 2017). For stoloniferous herb Trifolium repens, greater compensatory growth was observed in offspring ramets propagated from parental ramets subjected to repeated application of jasmonic acid compared to ones from parental ramets subjected to the same volume distilled water without application of jasmonic acid (González et al., 2017); similar pattern was still observed in offspring ramets of Alternanthera philoxeroides propagated from populations suffering from long-time herbivory disturbance (Lu and Ding, 2012). As an alternative to the slower mechanisms of adaptation through natural selection, transgenerational plasticity may confer ecological advantages to clonal plants against the challenges of current and future rapid environmental changes (Verhoeven and Preite, 2014; Douhovnikoff and Dodd, 2015; Dong et al., 2017).
A greenhouse experiment was conducted to explicitly investigate effects of transgenerational plasticity across vegetative generations on morphological and physiological properties of stoloniferous herb Centella asiatica subjected to high/low light treatment. Our first hypothesis is that effects of transgenerational plasticity on morphological and physiological properties persist across vegetative generations. Light may be an important resource for growth, development and reproduction of plants (Madsen and Sand-Jensen, 1994; Wagner et al., 2005; Glover et al., 2015). Morphological plasticity is an adaptive strategy of clonal plants to heterogeneous light conditions. For example, internode extension of stolon and petiole elongation may allow clonal ramets to escape from low light patches and lift leaf blades to higher light zones (Oborny, 1994). The ramets subjected to low light condition can intercept more light by enlarging leaf area (Dong, 1995). So, flexible responses in the internode length of stolon, petiole length and leaf area are crucial for clonal plant in capturing light (Hutchings and Kroon, 1994). As a component of chlorophyll, leaf N content is positively correlated with photosynthetic capacity in plant (Feng et al., 2007; Chen et al., 2015). We predicted that high/low light treatment experienced by parental ramets significantly influenced internode length of stolon, specific internode length of stolon, petiole length, specific petiole length, leaf area, specific leaf area (SLA), leaf nitrogen and chlorophyll contents, potential maximum net photosynthetic rate(Pmax) in offspring ramets subjected to parental or non-parental light environments.
Our second hypothesis is that effects of transgenerational plasticity on growth performance are context-dependent. Then, we predicted that biomass accumulation and total stolon length of offspring ramets experiencing parental light environment significantly increased than those of offspring ramets experiencing non-parental light environment. Our third hypothesis is that offspring ramets reproduced from parental ramets subjected to low resource level environment should be favored in parental or non-parental environments. So, we predicted that whether in parental or non-parental light environment, biomass accumulation and total stolon length of offspring ramets from parental ramets subjected to low light treatment significantly increased than those of offspring ramets from parental ramets subjected to high light treatment.
Materials and Methods
Plant Material
Centella asiatica (Umbelliferae) is a stoloniferous perennial herb, which is generally distributed in ditches, margins of ponds, lawns and roadsides. Each ramet is composed of two zygomorphic leaves with slender petiole. The axillary bud on the vertical stem may grow out and form stolon (Chinese Academy of Sciences, 2004). The stolon usually take roots when in contact with moist substratum, forming a network of stolon above the ground.
Eight original plants of Centella asiatica were collected in Chengdu, Sichuan Province, China (30°05′ 31°26′N; 102°54′ 104°53′E) (Table 1). The original plants were at least 1 km away each other. They may or may not differ in genotype.
In April 2016, they were cultivated in a greenhouse, located in Sichuan Normal University. All pots were filled with substrate (3:1 mixture of humus soil and sand). During the experiment, fertilizer (20% N, 20% P, 20% K; The Scotts Company, United States) was applied to each pot once per week. Tap water was supplied to keep the substrate moist. After 4 months, offspring ramets of each original plant formed a “ramet bank” (EI-Keblawy and Bhatt, 2015).
Experimental Design
F0 generation August 2016, two parental ramets with similar size from each “ramet bank” were grown into plastic pots (42 cm × 34 cm × 11 cm) respectively. We standardized size of the ramets by removing extra leaves and cutting the roots (Wang et al., 2013; Dong et al., 2017). One ramet was subjected to high light treatment (full light) and the other was subjected to low light treatment (50% full light). All reproduced ramets in each pot were named as F0 generation during 10 weeks period.
F1 generation Two ramets with similar size were chosen from each F0 generation and grown in new pots, respectively. One ramet was subjected to high light treatment and the other was subjected to low light treatment. All reproduced ramets in each pot were named as F1 generation during another 10 weeks period.
F2 generation One ramet was chosen from each F1 generation and grown in a new pot. The ramet was subjected to light treatment as its F1 generation experienced. All reproduced ramets in each pot were named as F2 generation during another 10 weeks period. Four treatments were included for F2 generation: F0 generation high light + F1 generation high light + F2 generation high light (HHH); F0 generation high light + F1generation low light + F2 generation low light (HLL); F0 generation low light + F1 generation high light + F2 generation high light (LHH); F0 generation low light + F1 generation low light + F2 generation low light (LLL) (Figure 1). There were eight replicates per treatment. The pots were re-randomized to avoid potential effects of environmental heterogeneity. Offspring ramets from each original plant underwent all treatments.
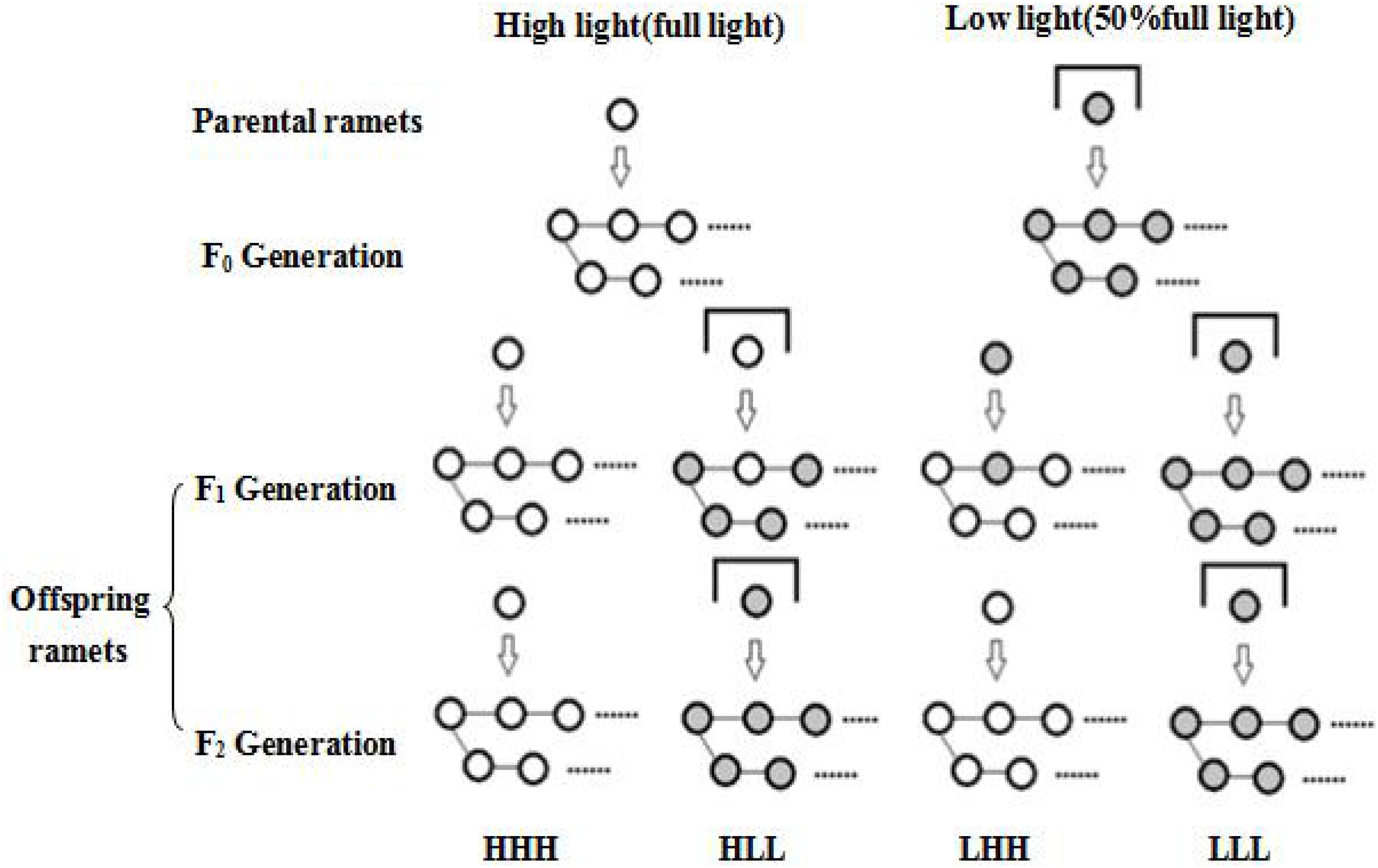
FIGURE 1. Schematic diagram of the experimental design. The experiment is across three vegetative generations. Four treatments were included for F2 generation: F0 generation high light + F1 generation high light + F2 generation high light (HHH); F0 generation high light + F1generation low light + F2 generation low light (HLL); F0 generation low light + F1 generation high light + F2 generation high light (LHH); F0 generation low light + F1 generation low light + F2 generation low light (LLL).
Morphological Properties
After harvesting, offspring ramets (F2 generation) were separated into root, leaf, petiole and stolon. Internode length of stolon and petiole length were measured by ruler. Specific internode length of stolon (internode length of stolon / dry weight) and specific petiole length (petiole length/ dry weight) were counted after drying to constant weight. Leaf area was measured according to the method described by Dong et al. (2015). Specific leaf area (SLA) was counted as follows:
Photosynthetic Properties
A portable photosynthesis system GFS-3000 (Heinz Walz GmbH, Effeltrich, Germany) was used for measurement of photosynthesis during the last week of growth. Eight offspring ramets (F2 generation) with similar size were chosen from each treatment. A fully expanded and mature leaf from each ramet was selected for photosynthetic measurement.
Under a CO2 pressure of 400 μmolmol-1, a light–response curve [net photosynthesis rate (Pn)–photosynthetic photon flux density (PPFD) curve] was generated according to the method described by Chen et al. (2015). The Pmax was calculated according to the Pn–PPFD curves which were fitted with a non-rectangular hyperbola model using the plotting software Origin (Origin Lab, United States) (Gomes et al., 2006; Sorrell et al., 2012):
Where ∅ was the apparent quantum efficiency, 𝜃 was the convexity of the curve and Rd was the dark respiration rate.
Leaf Properties
The leaf for measurement of photosynthetic parameters was then finely ground to determine the nitrogen content with an elemental analyser (vario MACRO CUBE, Elementar Analysensysteme, Hanau, Germany). At the same time, the other zygomorphic leaf originating from the each ramet was selected to measure the absolute chlorophyll content using the dimethylsulphoxide (DMSO) chlorophyll extraction technique (Richardson et al., 2002).
Growth Performance
After harvesting, dry weights of stolon, petiole, root and leaf were recorded after oven drying at 60°C until constant weight was obtained.
Statistical Analysis
Prior to analysis, a square root transformation was used for total stolon length and a logarithmic transformation applied to petiole length. Two-way ANOVA was used to investigate the effects of light treatment experienced by F0 generation (F0), light treatment experienced by F2 generation (F2) and their interaction (F0 × F2) on morphological, leaf and photosynthetic properties of offspring ramets (F2 generation) as well as growth performance. Tukey HSD post hoc test was empolyed to compare difference among different treatments experienced by F2 generation. All analyses were conducted with SPSS 20.0 software (SPSS, Chicago, IL, United States).
Results
Morphological Properties
Specific petiole length and internode length of stolon of offspring ramets (F2 generation) were significantly affected by light treatment experienced by F0 generation (F0), light treatment experienced by F2 generation (F2) and their interaction (F0 × F2) (Table 2). Petiole length and leaf area of offspring ramets (F2 generation) were significantly affected by light treatment experienced by F0 generation (F0) and light treatment experienced by F2 generation (F2) (Table 2). However, specific leaf area (SLA) was significantly affected by light treatment experienced by F0 generation (F0) (Table 2). We did not detect significant effects of the different treatments on specific internode length of stolon of offspring ramets (F2 generation) (Table 2 and Figure 2D).
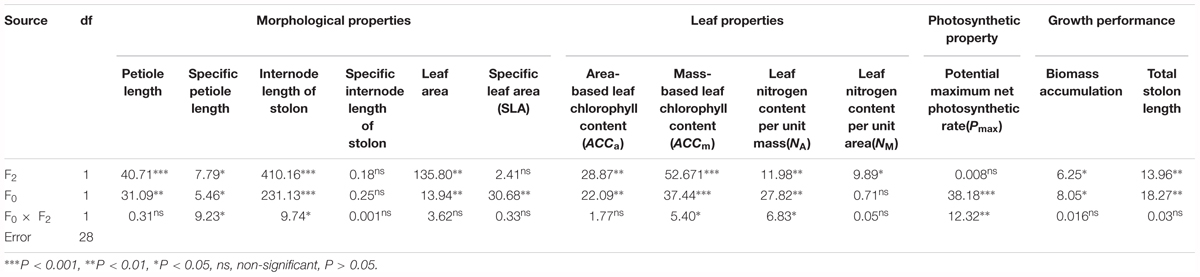
TABLE 2. Two-way ANOVA results for effects of light treatment experienced by F0 generation (F0), light treatment experienced by F2 generation (F2) and their interaction on the morphological, leaf and photosynthetic properties of of offspring ramets (F2 generation) as well as growth performance.
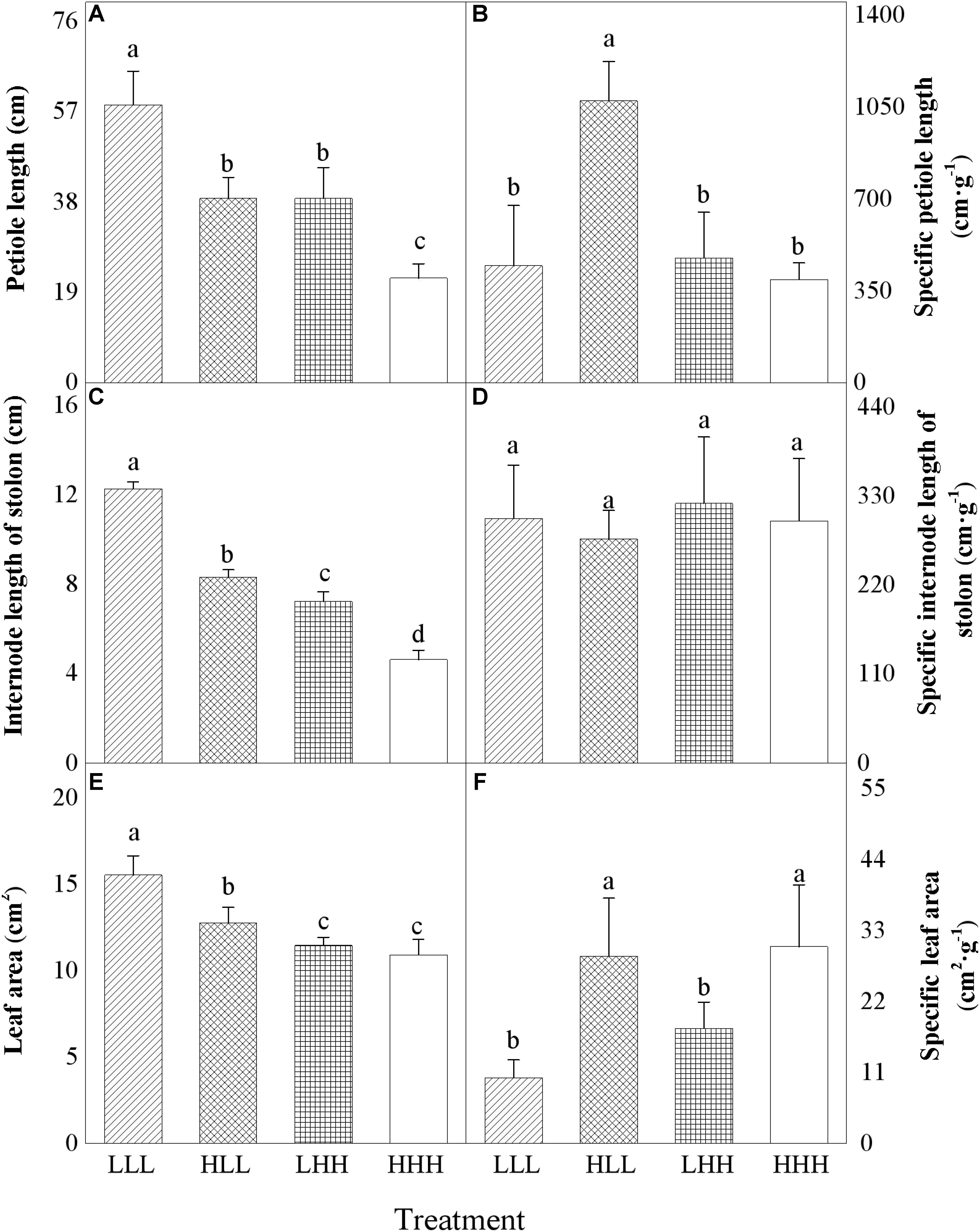
FIGURE 2. Effects of transgenerational plasticity on morphological properties of offspring ranets (F2 generations). The same lower case letters are not significantly different at the P = 0.05 level. Values are means ± s.e. (standard errors), n = 8. HHH: F0 generation high light+ F1 generation high light+ F2 generation high light; HLL: F0 generation high light+ F1 generation low light+ F2 generation low light; LHH: F0 generation low light+ F1 generation high light+ F2 generation high light; LLL: F0 generation low light+ F1 generation low light+ F2 generation low light.
When parental ramets were subject to low light treatment, petiole length and internode length of stolon of offspring ramets (F2 generation) experiencing parental light environment significantly increased than those of offspring ramets (F2 generation) experiencing non-parental light environment (Figures 2A,C). However, opposite pattern was observed in petiole length and internode length of stolon of offspring ramets (F2 generation) from parental ramets subjected to high light treatment (Figures 2A,C). When parental ramets were subject to high light treatment, specific petiole length of offspring ramets (F2 generation) experiencing low light environment significantly increased (Figure 2B). Leaf area of offspring ramets (F2 generation) experiencing low light treatment significantly increased than that of offspring ramets (F2 generation) experiencing high light treatment (Figure 2E). When parental ramets were subject to low light treatment, leaf area of offspring ramets (F2 generation) experiencing parental light environment significantly increased than that of offspring ramets (F2 generation) experiencing non-parental light environment (Figure 2E). Compared to offspring ramets from parental ramets subjected to high light treatment, specific leaf area (SLA) of offspring ramets (F2 generation) from parental ramets subjected to low light treatment significantly decreased (Figure 2F).
Leaf Properties
Area-based leaf chlorophyll content (ACCa) of offspring ramets (F2 generation) was significantly affected by light treatment experienced by F0 generation and light treatment experienced by F2 generation (Table 2). Mass-based leaf chlorophyll content (ACCm) of offspring ramets (F2 generation) was significantly affected by light treatment experienced by F0 generation, light treatment experienced by F2 generation and their interaction (F0 × F2) (Table 2). When parental ramets (F0 generation) were subjected to low light treatment, area-based leaf chlorophyll content (ACCa) and mass-based leaf chlorophyll content (ACCm) of offspring ramets (F2 generation) experiencing parental light environment significantly increased than those of offspring ramets (F2 generation) experiencing non-parental light environment (Figures 3A,B). Opposite pattern was observed in area-based leaf chlorophyll content (ACCa) and mass-based leaf chlorophyll content (ACCm) of offspring ramets (F2 generation) from parental ramets subjected to high light treatment (Figures 3A,B).
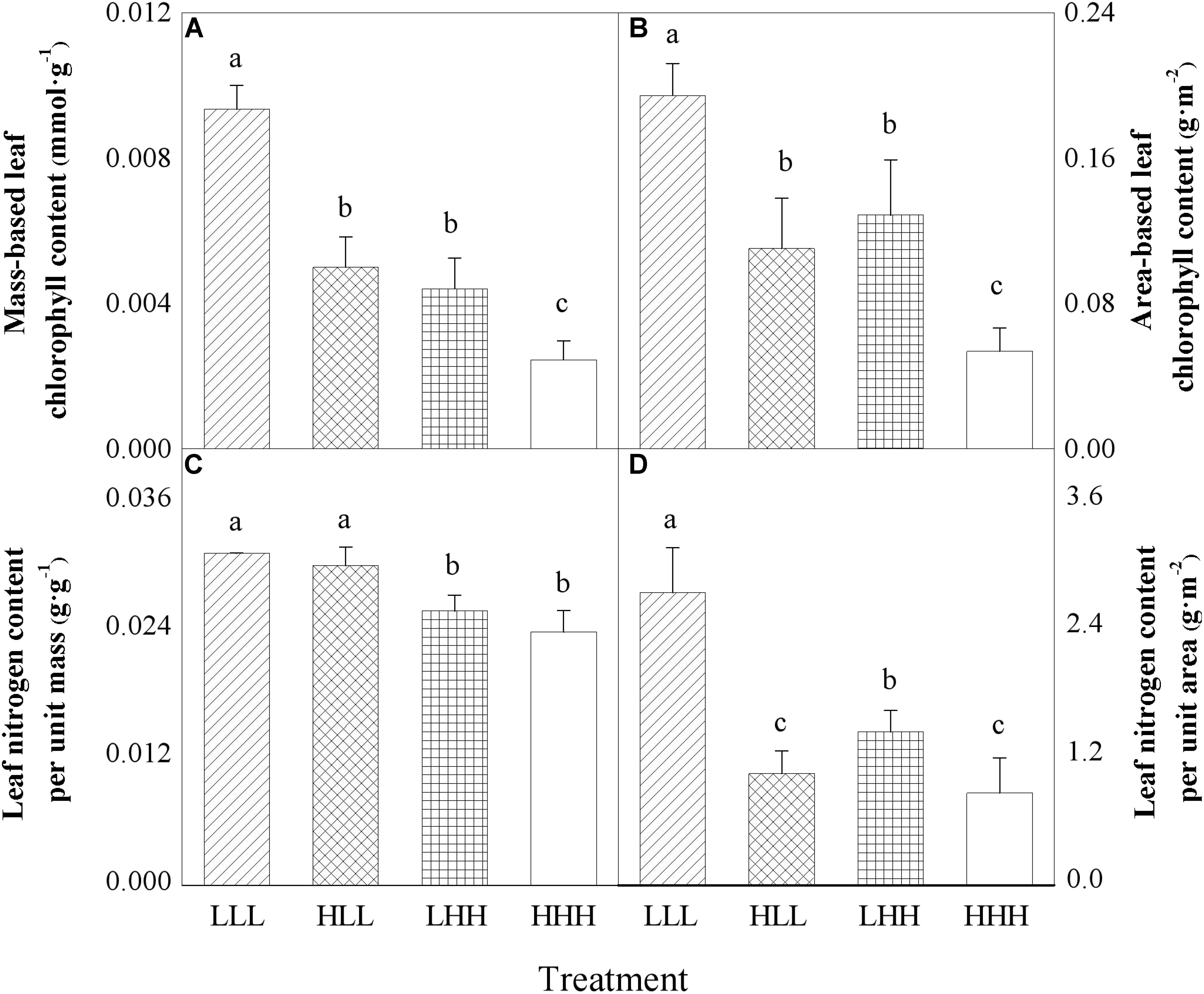
FIGURE 3. Effects of transgenerational plasticity on leaf properties of offspring ramets (F2 generation). The same lower case letters are not significantly different at the P = 0.05 level. Values are means ± s.e. (standard errors), n = 8. HHH: F0 generation high light+ F1 generation high light+ F2 generation high light; HLL: F0 generation high light+ F1 generation low light+ F2 generation low light; LHH: F0 generation low light+ F1 generation high light+ F2 generation high light; LLL: F0 generation low light+ F1 generation low light+ F2 generation low light.
Leaf nitrogen content per unit area (NA) of offspring ramets (F2 generation) was significantly affected by light treatment experienced by F0 generation, light treatment experienced by F2 generation and their interaction (F0 × F2) (Table 2). However, leaf nitrogen content per unit mass (NM) of offspring ramets (F2 generation) was significantly affected by light treatment experienced by F2 generation (F2) (Table 2). When parental ramets (F0 generation) were subjected to low light treatment, leaf nitrogen content per unit area (NA) of offspring ramets (F2 generation) experiencing parental light environment was significantly greater than that of offspring ramets (F2 generation) experiencing non-parental light environment (Figure 3D). Leaf nitrogen content per unit mass (NM) of offspring ramets (F2 generation) subjected to low light treatment was significantly greater than that of offspring ramets subjected to high light treatment (Figure 3C).
Photosynthetic Property
Potential maximum net photosynthetic rate (Pmax) of offspring ramets (F2 generation) was significantly affected by light treatment experienced by F0 generation and interaction between light treatment experienced by F0 generation and light treatment experienced by F2 generation (F0 × F2) (Table 2). Potential maximum net photosynthetic rate (Pmax) of offspring ramets (F2 generation) from parental ramets subjected to low light treatment was greater than that of offspring ramets (F2 generation) from parental ramets subjected to high light treatment (Figure 4). Potential maximum net photosynthetic rate (Pmax) of offspring ramets (F2 generation) subjected to parental light environment was greater than that of offspring ramets subjected to non-parental light environment (Figure 4).
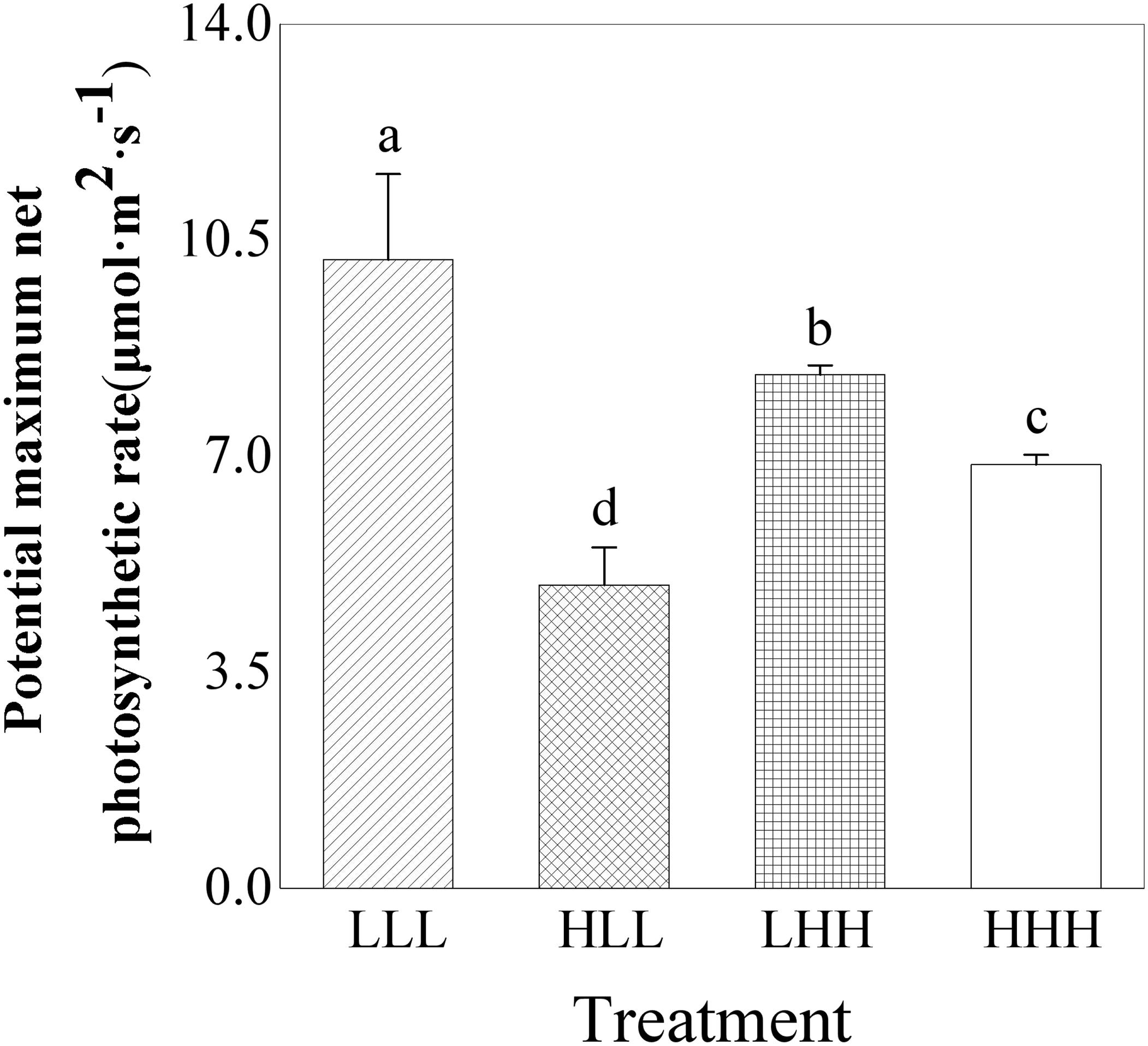
FIGURE 4. Effects of transgenerational plasticity on potential maximum net photosynthetic rate of offspring ramets (F2 generations). The same lower case letters are not significantly different at the P = 0.05 level. Values are means ± s.e. (standard errors), n = 8. HHH: F0 generation high light+ F1 generation high light+ F2 generation high light; HLL: F0 generation high light+ F1 generation low light+ F2 generation low light; LHH: F0 generation low light+ F1 generation high light+ F2 generation high light; LLL: F0 generation low light+ F1 generation low light+ F2 generation low light.
Growth Performance
Biomass accumulation and total stolon length of offspring ramets were significantly affected by light treatment experienced by F0 generation (F0) and light treatment experienced by F2 generation (F2) (Table 2). The greatest biomass accumulation and total stolon length were observed in offspring ramets (F2 generation) subjected to low light treatment as parental ramets (F0 generation) experienced (Figures 5A,B). When parental ramets (F0 generation) were subjected to low light treatment, biomass accumulation and total length of stolon of offspring ramets (F2 generation) experiencing parental light environment were significantly greater than those of offspring ramets (F2 generation) experiencing non-parental light environment (Figures 5A,B). Opposite pattern was observed in offspring ramets (F2 generation) from parental ramets subjected to high light treatment (Figures 5A,B).
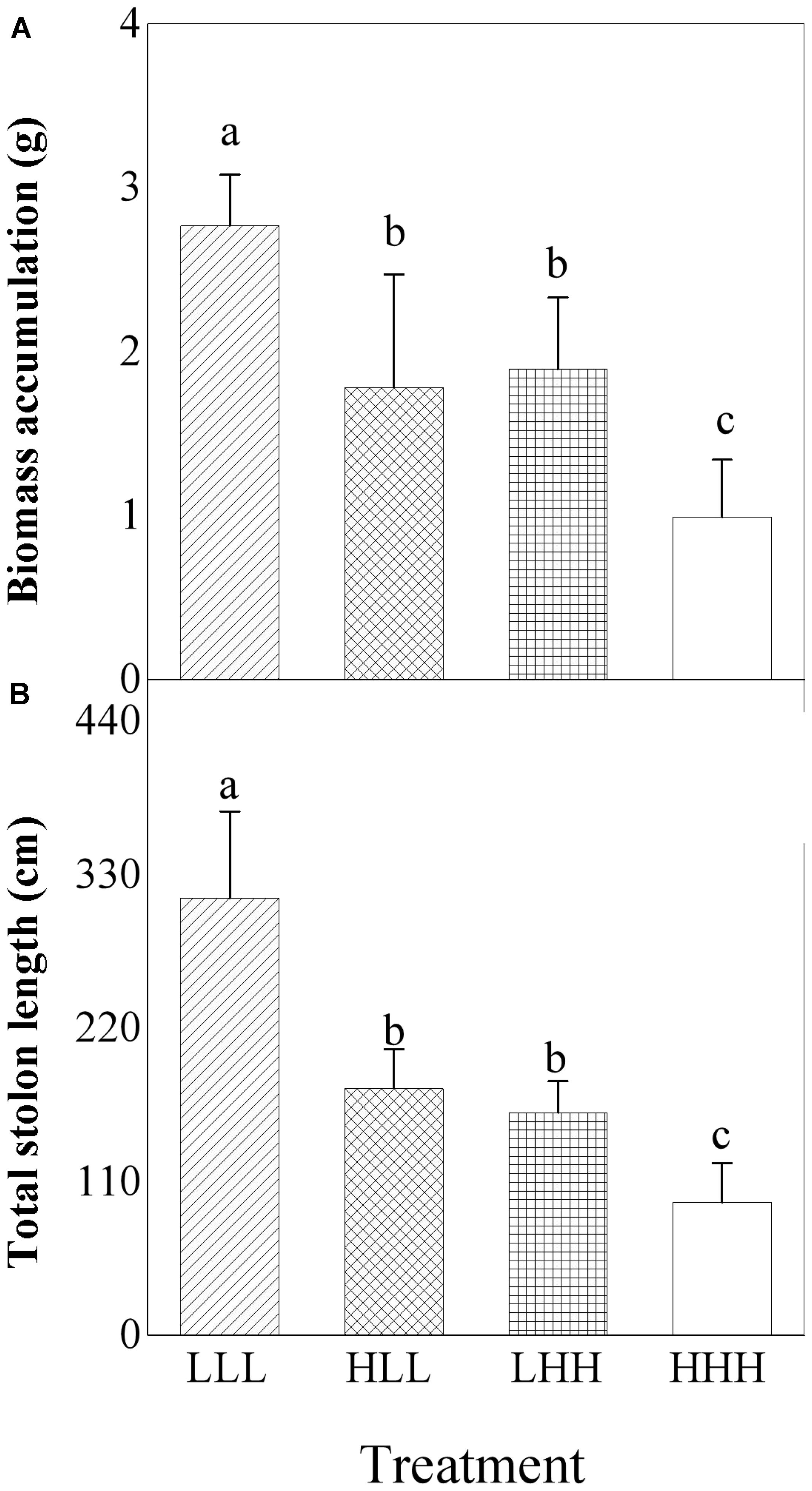
FIGURE 5. Effects of transgenerational plasticity on growth performance of offspring ramets (F2 generation). The same lower case letters are not significantly different at the P = 0.05 level. Values are means ± s.e. (standard errors), n = 8. HHH: F0 generation high light+ F1 generation high light+ F2 generation high light; HLL: F0 generation high light+ F1 generation low light+ F2 generation low light; LHH: F0 generation low light+ F1 generation high light+ F2 generation high light; LLL: F0 generation low light+ F1 generation low light+ F2 generation low light.
Discussion
For stoloniferous herb C.asiatica, the experiment demonstrated transgenerational plasticity triggered by high/low light treatment. Light environment experienced by parental ramets (F0 generation) significantly influenced morphological and physiological properties of offspring ramets (F2 generation) as well as growth performance even after they were detached from the parental ramets. The results supported our first hypothesis that effects of transgenerational plasticity on morphological and physiological properties can transmit across vegetative generations of C. asiatica. Due to limited opportunities to adapt to environmental changes genetically, transgenerational plasticity can impose substantial impact on population dynamics (Benton et al., 2005; Plaistow et al., 2006) and evolution of clonal plants in response to environmental changes (Wade, 1998; Räsänen and Kruuk, 2007). So, transgenerational plasticity would have consequences for population dynamics, and ultimately, evolution, especially given the limited levels of genotypic variation in clonal plants (González et al., 2017).
When parental ramets (F0 generation) were subjected to low light treatment, offspring ramets (F2 generation) experiencing parental light environment presented better growth performance than offspring ramets (F2 generation) experiencing non-parental light environment. This is consistent with previous study that if offspring ramets spread into a new environment as experienced by parental ramets, transgenerational plasticity may facilitate establishment of their populations by enabling adaptation to the new environment more rapidly than natural selection (Latzel and Klimešová, 2010). In addition, opposite pattern was observed in offspring ramets (F2 generation) from parental ramets subjected to high light treatment. The results supported our second hypothesis that effects of transgenerational plasticity on growth performance are context-dependent. Such transgenerational plasticity spanning across vegetative generations is likely adaptive in clonal species (Herman and Sultan, 2011; Holeski et al., 2012).
Compared to offspring ramets (F2 generation) from parental ramets (F0 generation) subjected to high light treatment, growth performance of offspring ramets (F2 generation) from parental ramets (F0 generation) subjected to low light treatment was favored in parental or non-parental light environment. The results supported our third hypothesis. Habitat-specific DNA methylation of clonal genotypes from natural populations may result in locally specialized ecotypes (Verhoeven and Preite, 2014). Further, transgenerational plasticity can affect the evolutionary rate and direction of clonal plants (Latzel et al., 2016; González et al., 2017).
Clonal species often adopted morphological response such as stolon elongation or petiole expansion to escape from environmental stress such as flooding (Luo et al., 2009), low light (González et al., 2017), metal pollutions (Roiloa and Retuerto, 2012) and interspecific competition from neighbor species (Evans and Cain, 1995). Seedlings from parents grown in a CO2-elevated environment reduced photosynthesis compared to seedlings from parents grown in ambient CO2 conditions (Huxman et al., 2001). Parents may enable their offspring to adapt to environmental changes through morphological and photosynthetic adjustment. With plastic changes of morphological properties, photosynthetic capacity and growth performance were significantly improved in offspring ramtes (F2 generation) experiencing parental light environment than in offspring ramtes (F2 generation) experiencing non-parental light environment when parental ramets (F0 generation) were subjected to low light treatment. We tentatively concluded that in response to low light treatment, variation of morphological and physiological properties in parental ramets was transmited to their offspring ramets (Latzel et al., 2009). When parental ramets (F0 generation) were subjected to low light treatment, the greatest leaf nitrogen content per unit area (NA) was observed in offspring ramtes (F2 generation) experiencing parental light environment with a decrease of specific leaf area (SLA). The results implied that effects of transgenerational plasticity on photosynthesis of offspring ramets might be mediated by alternation of resources allocation toward the photosynthetic apparatus (Latzel et al., 2009). It is suggested that environmentally induced epigenetic change and/or inherited resource allocation pattern toward photosynthesis may be responsible for effects of transgenerational plasticity on photosynthesis of offspring ramets.
Furthermore, clonal plants have the potential to selectively place ramets and to avoid unfavorable conditions through morphological plasticity of spacer or branching intensity (Hutchings and Kroon, 1994; Kleunen and Fischer, 2001). For Polygonum persicaria, progeny from parent experiencing drought environment produced longer, more rapidly extending root systems and greater biomass in parental environment than those of progeny in non-parental environment (Galloway and Etterson, 2007). In addition, root-shoot biomass ratio and specific root length of progeny subjected to drought environment experienced by parent significantly increased than those of progeny in ample water environment (Sultan et al., 2009). In our experiment, effects of transgenerational plasticity on morphological and physiological properties of offspring ramets (F2 generation) depended on environmental characteristics experienced by their parent and themselves. Clonal plants thus have the potential to reflect past and current environmental conditions even anticipate future conditions (Latzel et al., 2016). So, the dynamics and genetics of clonal populations may be affected by the interaction of genotypes to phenotypes.
To the best of our knowledge, there has been rare study directly examining the effects of transgenerational plasticity on clonal plants through both morphological and physiological properties. Our work provides evidence that transgenerational plasticity through both morphological and physiological flexibility was triggered across vegetative generations for stoloniferous herb C. asiatica subjected to high/low light treatment. Life-history traits such as clonal integration, intraclonal division of labor and clonal architecture et al may be advantageous to exploitation and colonization of clonal plants in heterogeneous habitats (Latzel and Klimešová, 2010; Martina and Ende, 2013; Chen et al., 2015). The transgenerational plasticity can allow offspring ramets to present adaptive phenotype early without lag time in response to the current environment. Thus, it is very important for clonal plants in adapting temporally and spatially heterogeneous habitats. A wider range of species are needed to understand the generality of this pattern and to assess fully the ecological advantages afforded by these features.
Author Contributions
All authors conceived, designed, and performed the experiments and wrote the paper.
Funding
This project was supported by Education Department of Sichuan Province (14Za0023).
Conflict of Interest Statement
The authors declare that the research was conducted in the absence of any commercial or financial relationships that could be construed as a potential conflict of interest.
References
Aarssen, L. W., and Burton, S. M. (1990). Maternal effects at four levels in Senecio vulgaris (Asteraceae) grown on a soil nutrient gradient. Am. J. Bot. 77, 1231–1240. doi: 10.2307/2444634
Agrawal, A. A. (2002). Herbivory and maternal effects: mechanisms and consequences of transgenerational induced plant resistance. Ecology 83, 3408–3415. doi: 10.2307/3072089
Benton, T. G., Plaistow, S. J., Beckerman, A. P., Lapsley, C. T., and Littlejohns, S. (2005). Changes in maternal investment in eggs can affect population dynamics. Proc. Biol. Sci. 272, 1351–1356. doi: 10.1098/rspb.2005.3081
Chen, B. J., During, H. J., Vermeulen, P. J., and Anten, N. P. (2014). The presence of a below-ground neighbour alters within-plant seed size distribution in Phaseolus vulgaris. Ann. Bot. 114, 937–943. doi: 10.1093/aob/mcu162
Chen, J. S., Li, J., Zhang, Y., and Lei, N. F. (2015). Clonal integration ameliorates the carbon accumulation capacity of a stoloniferous herb, Glechoma longituba, growing in heterogenous light conditions by facilitating nitrogen assimilation in the rhizosphere. Ann. Bot. 115, 127–136. doi: 10.1093/aob/mcu207
Chinese Academy of Sciences (2004). Flora Reipublicae Popularis Sinicae, Vol. 55. Beijing: Science Press, 31–32.
Dong, M. (1995). Morphological responses to local light conditions in clonal herbs from contrasting habitats, and their modification due to physiological integration. Oecologia 101, 282–288. doi: 10.1007/BF00328813
Dong, B. C., Alpert, P., Zhang, Q., and Yu, F. H. (2015). Clonal integration in homogeneous environments increases performance of Alternanthera philoxeroides. Oecologia 179, 393–403. doi: 10.1007/s00442-015-3338-y
Dong, B. C., Fu, T., Luo, F. L., and Yu, F. H. (2017). Herbivory-induced maternal effects on growth and defense traits in the clonal species Alternanthera philoxeroides. Sci. Total Environ. 60, 114–123. doi: 10.1016/j.scitotenv.2017.06.141
Douhovnikoff, V., and Dodd, R. S. (2015). Epigenetics: a potential mechanism for clonal plant success. Plant Ecol. 216, 227–233. doi: 10.1007/s11258-014-0430-z
Dyer, A. R., Brown, C. S., Espeland, E. K., McKayJ, K., Meimberg, H., and Rice, K. J. (2010). The role of adaptive trans-generational plasticity in biological invasions of plants. Evol. Appl. 3, 179–192. doi: 10.1111/j.1752-4571.2010.00118.x
Evans, J. P., and Cain, M. L. (1995). A spatially explicit test of foraging behavior in a clonal plant. Ecology 76, 1147–1155. doi: 10.2307/1940922
Fenesi, A., Dyer, A. R., Geréd, J., Sándor, D., and Ruprecht, E. (2014). Can transgenerational plasticity contribute to the invasion success of annual plant species? Oecologia 176, 95–106. doi: 10.1007/s00442-014-2994-7
Feng, Y. L., Auge, H., and Ebeling, S. K. (2007). Invasive Buddleja davidii allocates more nitrogen to its photosynthetic machinery than five native woody species. Oecologia 153, 501–510. doi: 10.1007/s00442-007-0759-2
Galloway, L. F. (2005). Maternal effects provide phenotypic adaptation to local environmental conditions. New Phytol. 166, 93–100. doi: 10.1111/j.1469-8137.2004.01314.x
Galloway, L. F., and Etterson, J. R. (2007). Transgenerational Plasticity is adaptive in the wild. Science 318, 1134–1136. doi: 10.1126/science.1148766
Glover, R., Drenovsky, R. E., Futrell, C. J., and Grewell, B. J. (2015). Clonal integration in Ludwigia hexapetala, under different light regimes. Aquat. Bot. 122, 40–46. doi: 10.1016/j.aquabot.2015.01.004
Gomes, F. P., Oliva, M. A., Mielke, M. S., de Almeida, A. A. F., and Leite, H. G. (2006). Photosynthetic irradiance-response in leaves of dwarf coconut palm (Cocos nucifera, L. ‘nana’, Arecaceae): comparison of three models. Sci. Hortic. 109, 101–105. doi: 10.1016/j.scienta.2006.02.030
González, A. P. R., Dumalasová, V., Rosenthal, J., Skuhrovec, J., and Latzel, V. (2017). The role of transgenerational effects in adaptation of clonal offspring of white clover (Trifolium repens) to drought and herbivory. Evol. Ecol. 31, 345–361. doi: 10.1007/s10682-016-9844-5
Herman, J. J., and Sultan, S. E. (2011). Adaptive transgenerational plasticity in plants: case studies, mechanisms, and implications for natural populations. Front. Plant Sci. 2:102. doi: 10.3389/fpls.2011.00102
Holeski, L. M., Jander, G., and Agrawal, A. A. (2012). Transgenerational defense induction and epigenetic inheritance in plants. Trends Ecol. Evol. 27, 618–626. doi: 10.1016/j.tree.2012.07.011
Hutchings, M. J., and Kroon, H. D. (1994). Foraging in plants: the role of morphological plasticity in resource acquisition. Adv. Ecol. Res. 25, 159–238. doi: 10.1016/S0065-2504(08)60215-9
Huxman, T. E., Charlet, T. N., Grant, C., and Smith, S. D. (2001). The effects of parental CO2 and offspring nutrient environment on initial growth and photosynthesis in an annual grass. Int. J. Plant Sci. 162, 617–623. doi: 10.1086/320132
EI-Keblawy, A. A., and Bhatt, A. (2015). Aerial seed bank affects germination in two small-seeded halophytes in Arab Gulf desert. J. Arid Environ. 117, 10–17. doi: 10.1016/j.jaridenv.2015.02.001
Kleunen, M. V., and Fischer, M. (2001). Adaptive evolution of plastic foraging responses in a clonal plant. Ecology 82, 3309–3319. doi: 10.1890/0012-9658(2001)082[3309:AEOPFR]2.0.CO;2
Latzel, V., Hájek, T., Klimešová, J., and Gómez, S. (2009). Nutrients and disturbance history in two Plantago species: maternal effects as a clue for observed dichotomy between resprouting and seeding strategies. Oikos 118, 1669–1678. doi: 10.1111/j.1600-0706.2009.17767.x
Latzel, V., Janecek, S., Dolezal, J., Klimesova, J., and Bossdorf, O. (2014). Adaptive transgenerational plasticity in the perennial Plantago lanceolata. Oikos 123, 41–46. doi: 10.1111/j.1600-0706.2013.00537.x
Latzel, V., and Klimešová, J. (2010). Transgenerational plasticity in clonal plants. Evol. Ecol. 24, 1537–1543. doi: 10.1007/s10682-010-9385-2
Latzel, V., Rendina, G. A. P., and Jonathan, R. (2016). Epigenetic memory as a basis for intelligent behavior in clonal plants. Front. Plant Sci. 7:1354. doi: 10.3389/fpls.2016.01354
Lu, X., and Ding, J. (2012). History of exposure to herbivores increases the compensatory ability of an invasive plant. Biol. Invasions 14, 649–658. doi: 10.1007/s10530-011-0106-8
Luo, F. L., Nagel, K. A., Zeng, B., Schurr, U., and Matsubara, S. (2009). Photosynthetic acclimation is important for post-submergence recovery of photosynthesis and growth in two riparian species. Ann. Bot. 104, 1435–1444. doi: 10.1093/aob/mcp257
Madsen, T. V., and Sand-Jensen, K. (1994). The interactive effects of light and inorganic carbon on aquatic plant growth. Plant Cell Environ. 17, 955–962. doi: 10.1111/j.1365-3040.1994.tb00324.x
Martina, J. P., and Ende, C. N. V. (2013). Increased spatial dominance in high nitrogen, saturated soil due to clonal architecture plasticity of the invasive wetland plant, Phalaris arundinacea. Plant Ecol. 214, 1443–1453. doi: 10.1007/s11258-013-0265-z
Miao, S. L., Bazzaz, F. A., and Primack, R. B. (1991). Persistence of maternal nutrient effects in Plantago major: the third generation. Ecology 72, 1634–1642. doi: 10.2307/1940963
Oborny, B. (1994). Growth rules in clonal plants and environmental predictability–a simulation study. J. Ecol. 82, 341–351. doi: 10.2307/2261302
Plaistow, S. J., Lapsley, C. T., and Benton, T. G. (2006). Context-dependent intergenerational effects: the interaction between past and present environments and its effect on population dynamics. Am. Nat. 167, 206–215. doi: 10.1086/499380
Räsänen, K., and Kruuk, L. (2007). Maternal effects and evolution at ecological time-scales. Funct. Ecol. 21, 408–421. doi: 10.1111/j.1365-2435.2007.01246.x
Richardson, A. D., Duigan, S. P., and Berlyn, G. P. (2002). An evaluation of noninvasive methods to estimate foliar chlorophyll content. New Phytol. 153, 185–194. doi: 10.1046/j.0028-646X.2001.00289.x
Riska, B. (1989). Composite traits, selection response, and evolution. Evolution 43, 1172–1191. doi: 10.2307/2409355
Roach, D. A., and Wulff, R. D. (1987). Maternal effects in plants. Annu. Rev. Ecol. Syst. 18, 209–235. doi: 10.1146/annurev.es.18.110187.001233
Roiloa, S. R., and Retuerto, R. (2012). Clonal integration in Fragaria vesca growing in metal-polluted soils: parents face penalties for establishing their offspring in unsuitable environments. Ecol. Res. 27, 95–106. doi: 10.1007/s11284-011-0876-6
Rossiter, M. C. (1996). Incidence and consequences of inherited environmental effects. Annu. Rev. Ecol. Syst. 27, 451–476. doi: 10.1146/annurev.ecolsys.27.1.451
Sorrell, B. K., Brix, H., Fitridge, I., Konnerup, D., and Lambertini, C. (2012). Gas exchange and growth responses to nutrient enrichment in invasive Glyceria maxima and native New Zealand Carex species. Aquat. Bot. 103, 37–47. doi: 10.1016/j.aquabot.2012.05.008
Sultan, S. E. (1996). Phenotypic plasticity for offspring traits in Polygonum persicaria. Ecology 77, 1791–1807. doi: 10.2307/2265784
Sultan, S. E., Barton, K., and Wilczek, A. M. (2009). Contrasting patterns of transgenerational plasticity in ecologically distinct congeners. Ecology 90, 1831–1839. doi: 10.1890/08-1064.1
Verhoeven, K. J., and Preite, V. (2014). Epigenetic variation in asexually reproducing organisms. Evolution 68, 644–655. doi: 10.1111/evo.12320
Wade, M. J. (1998). The Evolutionary Genetics of Maternal Effects. New York, NY: Oxford University Press.
Wagner, H., Jakob, T., and Wilhelm, C. (2005). Balancing the energy flow from captured light to biomass under fluctuating light conditions. New Phytol. 169, 95–108. doi: 10.1111/j.1469-8137.2005.01550.x
Keywords: maternal effects, potential maximum net photosynthetic rate (Pmax), leaf nitrogen content, internode length of stolon, leaf area
Citation: Li K, Chen J, Wei Q, Li Q and Lei N (2018) Effects of Transgenerational Plasticity on Morphological and Physiological Properties of Stoloniferous Herb Centella asiatica Subjected to High/Low Light. Front. Plant Sci. 9:1640. doi: 10.3389/fpls.2018.01640
Received: 13 June 2018; Accepted: 22 October 2018;
Published: 14 November 2018.
Edited by:
Bi-Cheng Dong, Beijing Forestry University, ChinaReviewed by:
Yao-Bin Song, Hangzhou Normal University, ChinaFang-Li Luo, Beijing Forestry University, China
Copyright © 2018 Li, Chen, Wei, Li and Lei. This is an open-access article distributed under the terms of the Creative Commons Attribution License (CC BY). The use, distribution or reproduction in other forums is permitted, provided the original author(s) and the copyright owner(s) are credited and that the original publication in this journal is cited, in accordance with accepted academic practice. No use, distribution or reproduction is permitted which does not comply with these terms.
*Correspondence: Jinsong Chen, cjs74@163.com Ningfei Lei, 470226504@qq.com