- 1College of Life Sciences, Zhejiang University, Hangzhou, China
- 2Center on Plant Environmental Sensing, College of Life and Environmental Sciences, Hangzhou Normal University, Hangzhou, China
- 3Department of Biology, Duke University, Durham, NC, United States
- 4School of Life Sciences, Zhejiang Sci-Tech University, Hangzhou, China
Salinity is one of the formidable environmental factors that affect plant growth and development and constrain agricultural productivity. Experimentally imposed short-term NaCl treatment triggers a transient increase in cytosolic free Ca2+ concentration ([Ca2+]i) via Ca2+ influx across the plasma membrane. Salinity stress, as well as other stresses, induces the production of reactive oxygen species (ROS), such as H2O2. It is well established that short-term H2O2 treatment also triggers a transient increase in [Ca2+]i. However, whether and how long-term NaCl and H2O2 treatments affect the basal levels of [Ca2+]i as well as plant responses to additional NaCl and H2O2 stresses remain poorly understood. Using an aequorin-based Ca2+ imaging assay, we found that the long-term treatment of Arabidopsis seedlings with both moderate NaCl and H2O2 in the growth media reduced the basal [Ca2+]i levels. Interestingly, we found that the long-term treatment with NaCl, but not H2O2, affected the responses of plants to additional NaCl stress, and remarkably the roots displayed enhanced responses while the leaves showed reduced responses. These findings suggest that plants adapt to the long-term NaCl stress, while H2O2 might be an integrator of many stresses.
Introduction
Soil salinization impacts nearly every aspect of plant growth and development and causes enormous agricultural production losses all over the world (Hasegawa et al., 2000; Zhu, 2002, 2016). High salinity not only leads to continuing loss of arable land but also decreases crop yields. High salinity affects almost a quarter to one-third of global agricultural land, especially in irrigated areas. Previous studies show that high salinity led us to lose about 10 million hectares of agricultural land per year (Zhu, 2001; Munns and Tester, 2008; Deinlein et al., 2014). An additional challenge that compounded these losses is that agriculture needs to provide enough food for a rapidly expanding population in the world and stave off large-scale food shortages (Schroeder et al., 2013). So, it is vital to understand how plants perceive and respond to salt stress.
Researchers have conducted many studies to dissect molecular and genetic mechanisms of plants’ response to salt (NaCl) stress, in which Arabidopsis thaliana was widely used. Excess NaCl in plants causes hyperosmotic stress and cellular ion imbalances (Zhu, 2003; Munns and Tester, 2008). NaCl stress triggers a transient increase in cytosolic Ca2+ concentration ([Ca2+]i) in plants, leading to transcriptional regulation, subsequent growth, as well as developmental responses (Knight et al., 1997; Tracy et al., 2008). Although the molecular nature of initial salt stress perception is unclear, it is highly speculated that this salt-triggered rapid rise in [Ca2+]i, which lasts about 2 min, represents a sensory process in plants (Munns and Tester, 2008; Deinlein et al., 2014). This is in accordance with the notion that cytosolic Ca2+ is a versatile secondary messenger and a vital element in a complex signaling network responding to different abiotic and biotic stimuli, including salt stress (Knight and Knight, 2001; Ru et al., 2008; Dodd et al., 2010; Marti et al., 2013). Given that [Ca2+]i of plants rapidly increased within a few seconds of exposure to NaCl, plant salt sensor is likely to be tightly coupled with Ca2+ channels.
In saline environments, salt stress also triggers other stresses such as osmotic, ionic, and oxidative stresses, and hence the growth of plants also commonly rely on their ability to cope with these stresses (Bose et al., 2015). Environmental stresses including salt stress trigger plants accumulating reactive oxygen species (ROS), and overproduction of ROS such as hydrogen peroxide (H2O2) occurs after salt stress treatment (Vaidyanathan et al., 2003; Borsani et al., 2005; Valderrama et al., 2006; Leshem et al., 2007; Miller et al., 2010; Ben Rejeb et al., 2014). As estimated from previous studies, the time constant for salt-triggered increases in [Ca2+]i is approximately 20 s and for salt-triggered ROS is 400 s (Knight et al., 1997; Leshem et al., 2007; Jiang et al., 2013; Cao et al., 2017). It seems the rapid rise in [Ca2+]i happens earlier than the elevation of ROS imposed by salinity. Considering ROS can also trigger increases in [Ca2+]i (McAinsh et al., 1996; Pei et al., 2000; Leshem et al., 2007; McAinsh and Pittman, 2009; Ma and Berkowitz, 2011), it is possible that, in the signal transduction pathway of salt stress, ROS-triggered [Ca2+]i rise perhaps functions as a feed-forward mechanism. According to recent studies of systemically propagating Ca2+ and ROS waves in plants, a new cell-to-cell communication pathway coupling with electric signals may help us understand how plant cells transmit long-distance signals (Gilroy et al., 2014; Kurusu et al., 2015). It should be noted that we have also found that salt-induced ROS could not trigger a detectable increase in [Ca2+]i under the imposed experimental conditions (Jiang et al., 2013). In plants, every specific [Ca2+]i signature is generated by the accurately manipulated activities of Ca2+ channels and transporters which are located in different tissues, organelles, and membranes. Cytosolic Ca2+ sensors include the calcineurin B-like (CBL) protein families, the Ca2+-dependent protein kinase (CDPK), the calmodulin (CaM), and the calmodulin-like (CML), which could monitor and decode the information loaded in [Ca2+]i signatures, allowing plants to give a quick response and tightly adapt to the ever-changing environment (Harper and Harmon, 2005; Dodd et al., 2010; Zhu et al., 2013). Previously we analyzed that the relationship and interaction of the [Ca2+]i increases are induced by salt stress and ROS in a short-term regime and found that NaCl-gated Ca2+ channels (NaC) and hydrogen peroxide (H2O2)-gated Ca2+ channels (HpC) are different (Jiang et al., 2013). However, whether the long-term treatment of NaCl and H2O2 on the basal [Ca2+]i are the same with plants responses to short-term stresses remains poorly understood.
In this research, we have systematically analyzed the basal [Ca2+]i under various long-term saline and oxidative growth conditions, and the [Ca2+]i signatures in response to short-term stresses under these growth regimes. These findings will not only enable us to better understand how plants perceive high salt and ROS signals but also establish assays for phenotyping potential Arabidopsis mutants with defects in NaCl- or H2O2-induced [Ca2+]i increases aiming to identify these sensitive Ca2+ channels.
Materials and Methods
Plant Materials and Growth Conditions
Arabidopsis thaliana ecotype Columbia-0 (Col-0) constitutively expressing intracellular aequorin (pMAQ2, a kind gift from Dr. M. Knight) under the control of the cauliflower mosaic virus 35S promoter was used (Knight et al., 1997; Tang et al., 2007). Seeds were sterilized with 2.5% PPM (Plant Preservative Mixture; Caisson Labs) at 4°C for 3 days. Arabidopsis plants were grown in 150 mm × 15 mm round Petri dishes in growth room. The culture media contained ½ Murashige and Skoog salts (MS; Sigma), 1.5% (w/v) sucrose (Sigma), and 0.8% (w/v) agar (Becton Dickinson), which was adjusted to pH 6.0 with KOH. We kept the temperature of the environmental rooms at 22 ± 2°C. The photo fluency rate of white light was ∼110 μmol m−2 s−1, and the photoperiods were 16 h light/8 h dark cycles. NaCl and H2O2 were added to the ½ MS media for saline and oxidative environments, respectively.
Aequorin Reconstitution and Measurement of [Ca2+]i
Arabidopsis thaliana plants expressing cytosolic apoaequorin were used for [Ca2+]i measurements (Knight et al., 1991; Tang et al., 2007; Yuan et al., 2014). Seedlings were grown on ½ MS medium for 9 days. Reconstitution of aequorin was conducted in vivo by spraying seedlings with 240 μL of 10 μM coelenterazine per Petri dish followed by incubation at 22°C in the dark for 8 h. Treatments and aequorin luminescence imaging were conducted at room temperature using a ChemiPro HT system including a cryogenically cooled and back-illuminated CCD camera or a newer version Lumazone system (Pylon1300B; Princeton Instruments) equipped with the H-800 light-tight controlled environmental box (Bio-One Scientific Instrument), liquid nitrogen auto filler (Roper Scientific or Bio-One Scientific Instrument), camera controller, and computer-equipped WinView/32 software (Roper Scientific) as described previously (Tang et al., 2007; Yuan et al., 2014). The CCD camera has a 1300 × 1340 pixel resolution and is cooled to −120°C by the cryogenic cooling system before image recording. For the basal [Ca2+]i levels, aequorin luminescence (L) record lasted 30 min in seedlings grown under different concentrations of NaCl or H2O2. For the changes in [Ca2+]i levels in response to NaCl and H2O2 stresses, aequorin luminescence (L) were recorded starting 50 s prior to the treatments, and luminescence images were taken every 10 s as done in our previous research (Jiang et al., 2013). In the dark, the solutions (100 mL) of H2O2 or NaCl solution was added into the Petri dish, and aequorin bioluminescence was recorded continuously (Yuan et al., 2014). Seedlings were treated with a discharging solution containing 0.9 M CaCl2 in 10% (v/v) ethanol and recorded for 5 min to estimate the total remaining aequorin bioluminescence (Lmax) (Knight et al., 1997; Rentel and Knight, 2004; Tang et al., 2007; Yuan et al., 2014). Finally, we used MetaMorph 7.7 to analyze the recorded image data. Considering that the relative [Ca2+]i is relevant to the study, the [Ca2+]i levels were shown as the ratio between aequorin luminescence (L) and total remaining aequorin (Lmax) as mentioned previously (Kiep et al., 2015; Ranf et al., 2015). All the experiments were conducted at room temperature, which was between 22 and 24°C.
Real-Time RT-PCR
Seedlings were grown on normal ½ MS media or ½ MS media added with 40 mM NaCl or 1 mM H2O2, for 10 days. The RNAs of leaves and roots were extracted separately, 50 mg sample of each, using RNA sample Total RNA Kit (TIANGEN). One microgram RNA was used for reverse transcription to generate the first strand of cDNA by FastKing RT Kit (TIANGEN). RT-PCR reaction was carried out using RealUniversal Color PreMix (TIANGEN). Eight-well optical PCR plate was used for each RT-PCR reaction, where each reaction contained 7.5 μL Premix, 1.5 μL forward primer, 1.5 μL reverse primer, 1.5 μL RNase-free H2O, and 3 μL cDNA template. The PCR cycle parameters were set as follows: 1 cycle of 15 min at 95°C, and 40 cycles of 10 s at 95°C, 20 s at 60°C, and 30 s at 72°C. Tublin was used as an internal control to normalize the gene expression level. Primers used in this study are listed in Supplementary Table S1. A melting curve was made after 40 cycles were completed to make sure that only single amplified products were obtained. The standard t-test was used for the statistical analysis.
Results
Long-Term Salt and Oxidative Stresses Lower the Basal [Ca2+]i
Calcium plays fundamentally essential extracellular and intracellular roles and often serves as a life and death signal in animals and plants (Berridge et al., 2003; Hofer and Brown, 2003; Dodd et al., 2010). At the resting level, the basal [Ca2+]i is tightly maintained at a concentration that is ∼20,000-fold below the extracellular Ca2+ concentration (Berridge et al., 2003; Clapham, 2007; Swanson et al., 2011). Since a failure in the regulation of basal [Ca2+]i leads to a vast array of severe diseases in humans, including cancers, neuron degeneration diseases, and cardiovascular diseases, the maintenance of adequate basal [Ca2+]i seems to be essential for all organisms (Roderick and Cook, 2008; Sammels et al., 2010). In plants, the basal [Ca2+]i displays diurnal oscillation patterns (Johnson et al., 1995) and is regulated by soil Ca2+ levels (Tang et al., 2007). In the current study, we observed significant variations of the basal [Ca2+]i levels in these seedlings, when we used NaCl media-grown seedlings of the ecotype Col-0 expressing cytosolic apoaequorin detective in stimulus-induced [Ca2+]i increases, similar to the osmo-sensing mutant osca1, for aequorin-based Ca2+ imaging analyses, we observed remarkably significant variations of the basal [Ca2+]i levels in these seedlings. This observation trigged us to ask a question regarding whether the long-term treatments of NaCl and H2O2 affect the basal [Ca2+]i, which is in line with our previous study on the [Ca2+]i signatures with short-term salt and ROS stresses (Jiang et al., 2013).
We grew Arabidopsis seedlings expressing the Ca2+ indicator aequorin in the MS medium containing 0 to 80 mM NaCl. It should be noted that the concentrations of NaCl were used with the consideration that the seedling growth should not be profoundly inhibited by NaCl, and thus the aequorin measurement would not be significantly affected. In other words, the seedlings looked largely normal without the apparent growth and developmental phenotypes. Aequorin bioluminescence images were taken at 30 min, and the relative value of luminescence (L/Lmax) was calculated and analyzed for the basal [Ca2+]i (Figure 1A). Surprisingly, we observed that the basal [Ca2+]i was decreased in response to the long-term treatment of NaCl (Figure 1A), which was in contrast to the well-known phenomenon of transient increases in [Ca2+]i upon the short-term NaCl treatment. Plants grown on the MS medium without NaCl had a relative basal [Ca2+]i (L/Lmax) of 3.72 ± 0.24 × 10−2 in the roots (Figure 1B). The basal [Ca2+]i levels decreased under NaCl treatment in a concentration-dependent manner (Figure 1B), i.e., the higher the concentration of NaCl in the growth media the lower the basal [Ca2+]i. The basal [Ca2+]i decreased to 1.75 ± 0.33 × 10−2 at 70 mM NaCl and maintained the similar value at 80 mM NaCl in the roots. We also noted that the reduction of basal [Ca2+]i in the roots was more than that in the leaves (Figure 1B). To figure out whether and how the changes in the basal [Ca2+]i affect NaCl- or H2O2-triggered transient increases in [Ca2+]i, we should identify an optimum concentration of NaCl in the growth media, as described previously, for potential up- and down-regulation. The ideal concentration should be used to produce about half of the maximum level of the basal [Ca2+]i (Jiang et al., 2013). Figure 1B shows that the concentration of NaCl required for a half-maximal response was ∼40 mM, and hence we chose 40 mM NaCl for the subsequent experiments.
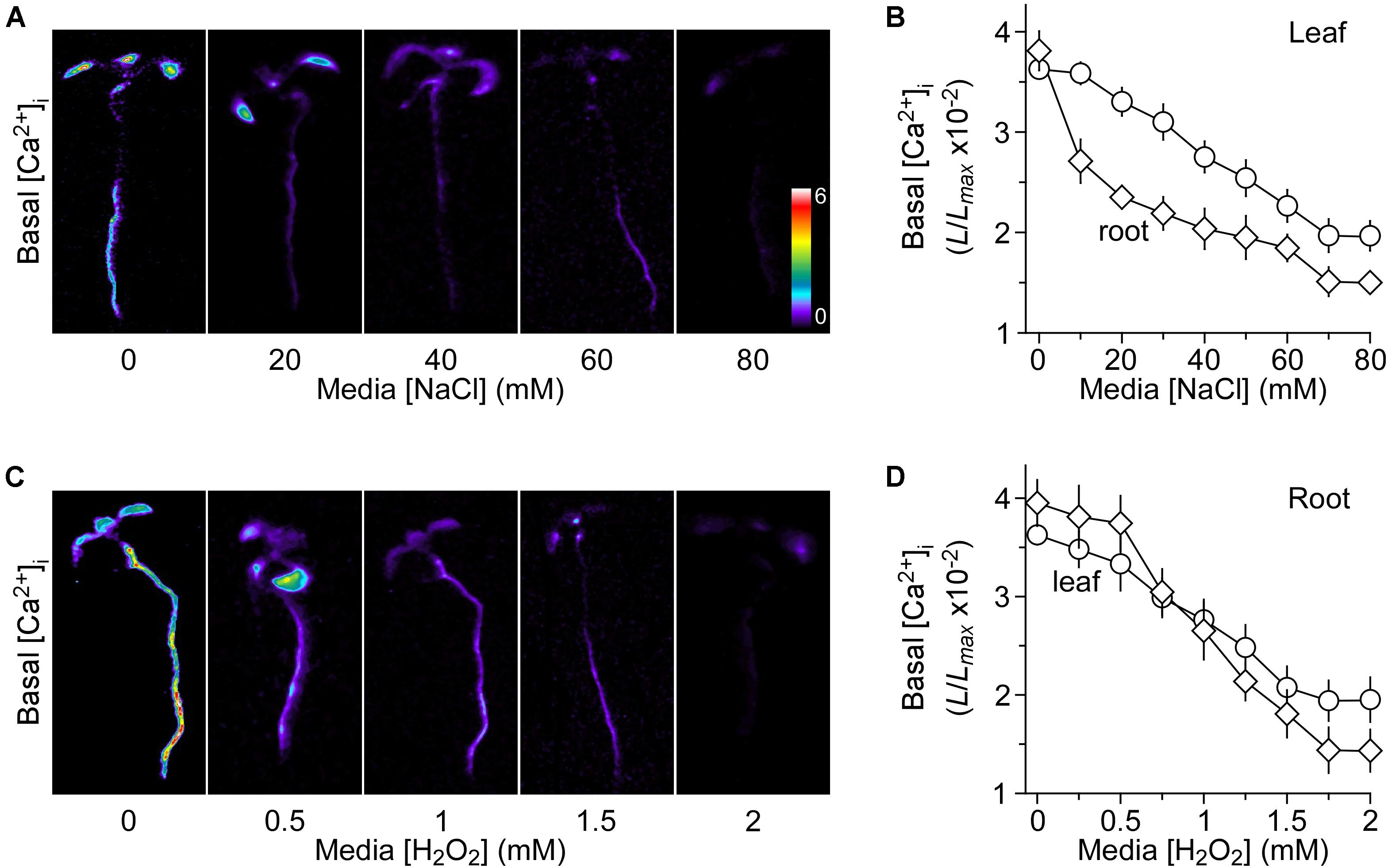
FIGURE 1. Decreases in basal [Ca2+]i in response to long-term salt and oxidative stress. (A,C) Imaging of basal [Ca2+]i in Arabidopsis seedlings grown under salt and oxidative stresses. Seedlings expressing aequorin were grown in the agar media containing several concentrations of NaCl (A) or H2O2 (C) for 9 days. Basal [Ca2+]i in whole seedlings was analyzed by imaging aequorin luminescence (L) for 30 min. Relative [Ca2+]i was shown as L/Lmax (×10−2), and scaled by a pseudo-color bar, where Lmax is the total aequorin luminescence. (B,D) Quantification of basal [Ca2+]i in these seedlings grown under NaCl (B) and H2O2 (D) from experiments as shown in (A,C), respectively. The basal [Ca2+]i levels in leaves and roots were analyzed separately. The data from four independent experiments are shown (mean ± SEM; n = 20).
Besides, we researched the basal [Ca2+]i levels in response to added H2O2 in the growth media. Seedlings were grown in MS medium with different concentrations of H2O2, which was from 0 to 2 mM. We also analyzed the basal [Ca2+]i as described above for NaCl. Interestingly, the long-term treatment of H2O2 also lowered the basal [Ca2+]i levels in a dose-dependent manner (Figures 1C,D). Similarly, the basal [Ca2+]i levels were more sensitive to H2O2 in the roots than in the leaves (Figure 1D). Finally, 1 mM H2O2 was chosen as the optimum concentration, in which the magnitude of [Ca2+]i was similar to that in the media with 40 mM NaCl.
Response on [Ca2+]i Induced by Short-Term Stimulus After Long-Term NaCl and H2O2 Pretreatment
We then asked whether the long-term treatment of Arabidopsis with NaCl affects plants’ response to short-term NaCl stress. In other words, we asked whether plants are sensitized or desensitized to NaCl stress when they grow in NaCl media. To determine NaCl-induced [Ca2+]i increases in Arabidopsis seedling grown under salt environments and to investigate these [Ca2+]i increases in roots and leaves separately, we detected the temporal dynamics of NaCl-induced [Ca2+]i increases without NaCl in the grown media as controls for further comparison. After the application of NaCl, the [Ca2+]i elevated immediately, reached a peak at about 20 s, and then declined gradually as described previously (Jiang et al., 2013). Several concentrations of NaCl, ranging from 0 to 600 mM, were applied and the peak values of [Ca2+]i were plotted as a function of the applied NaCl concentrations in the roots (Figure 2A) and leaves (Figure 2B), separately, for celerity. The magnitudes of [Ca2+]i were increased with increases in the concentration of NaCl (Figures 2A,B). The magnitudes of NaCl-induced [Ca2+]i increases were higher in the roots than in the leaves (Figure 2C), similar to those seen in a previous study (Tracy et al., 2008), while similar to another previous finding (Jiang et al., 2013), we found that half of the maximum amplitude [Ca2+]i response was about 200 mM, both in the roots and leaves.
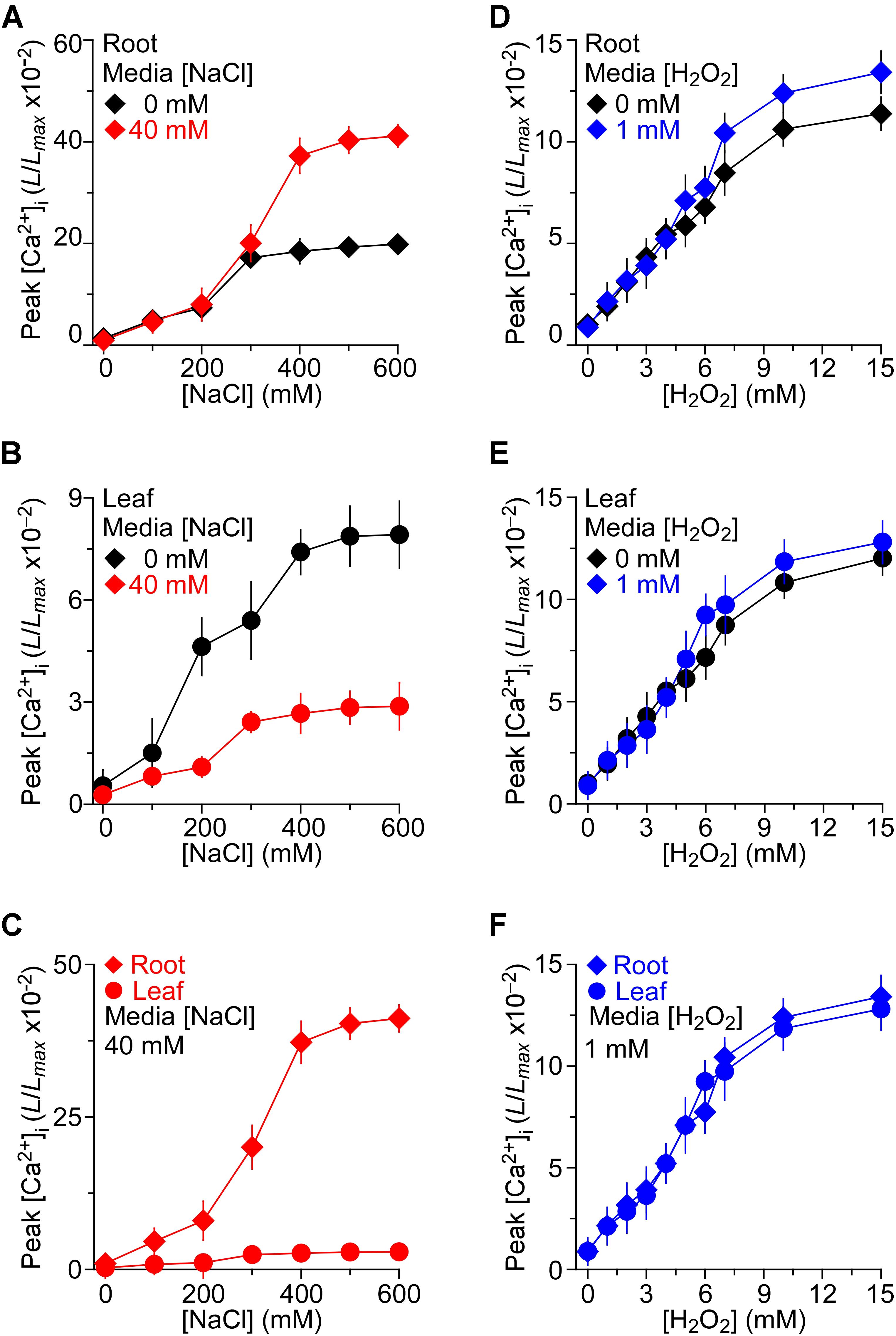
FIGURE 2. The effects of NaCl and H2O2 growth environments on increases in [Ca2+]i induced by NaCl and H2O2, respectively. (A–C) Arabidopsis seedlings expressing aequorin grown under 0 or 40 mM NaCl for 9 days were treated with several concentrations of NaCl, and aequorin luminescence images were taken every 10 s, for 200 s. Data are averaged peak values of increases in [Ca2+]i induced by NaCl in roots (A) and leaves (B), respectively. The sensitivities of [Ca2+]i to NaCl in leaves and roots from seedlings grown under 40 mM NaCl are directly compared in (C). Data from four independent experiments are shown (mean ± SEM; n = 20). (D–F) Seedlings grown under 0 or 1 mM H2O2 for 9 days were treated with several concentrations of H2O2, and aequorin images were taken every 10 s, for 200 s. Data are averaged peak values of [Ca2+]i increases in roots (D) and leaves (E), respectively. The sensitivities of [Ca2+]i to H2O2 in leaves and roots grown under 1 mM H2O2 are directly compared in (F). Data from four independent experiments are shown (mean ± SEM; n = 20).
To access how the consistent presence of NaCl stress in the growth media affects the plants’ response to additional NaCl stress, the seedlings that were grown in the 40 mM NaCl media were treated with gradient concentrations of NaCl from 0 to 600 mM. We found that the [Ca2+]i increases in response to NaCl in MS medium containing 40 mM NaCl were qualitatively consistent with the response to NaCl in general MS, and a higher NaCl induced a greater peak of [Ca2+]i (Figures 2A,B). When changes in [Ca2+]i were detectable in the leaf which was grown in MS containing 40 mM NaCl, the increasing trend was smaller compared with those in MS. On the contrary, the changes in [Ca2+]i that were detectable in the root which grew in salt environment were higher. The root was more sensitive than the leaf under the salt environment compared with the seedling grown in MS (Figure 2C).
Besides, to investigate thoroughly the relationship of H2O2-induced [Ca2+]i increases in the root and leaf, the temporal dynamics of [Ca2+]i increases triggered by H2O2 were further compared. Seedlings were stimulated by concentrations of H2O2 from 0 to 15 mM in MS. After the application of H2O2, the [Ca2+]i increased immediately and reached a peak at about 50 s, and then declined gradually. As expected, H2O2 induced increases in [Ca2+]i in a dose-dependent manner (Figures 2D,E). Current results were similar to those reported previously (Jiang et al., 2013). We found that [Ca2+]i increased in response to H2O2 and the magnitudes of [Ca2+]i depended on the concentration of H2O2, the higher the concentration of H2O2 the higher the magnitude of [Ca2+]i (Figures 2D,E). However, after the application of H2O2, the [Ca2+]i increased immediately, and [Ca2+]i increase in the root was similar to that in the leaf, which was different from the NaCl -induced [Ca2+]i increases (Figures 2D,E). To further study how the changes in H2O2-induced [Ca2+]i increases in the oxidative environment, the seedlings were grown in MS medium containing 1 mM H2O2. We then found that the increases in [Ca2+]i in response to H2O2 from 0 to 15 mM in MS medium containing 1 mM H2O2 were qualitatively the same as those grown in the control MS media. The difference of [Ca2+]i between the root and leaf in the oxidative environment was a little higher than that in the general environment (Figures 2D,E). Additionally, the [Ca2+]i increases induced by H2O2 (0–15 mM) in root were similar to these in leaf of seedlings grown in the oxidative environment (Figure 2F).
Long-Term NaCl Treatment Alters Short-Term Response of [Ca2+]i to NaCl but Not H2O2
Previous studies have reported that the NaCl concentration for a half-maximal response was about 200 mM, and the magnitude of [Ca2+]i was similar to that induced by 4 mM H2O2 (Jiang et al., 2013). The seedlings grown in MS as a control and responses to the stimulus measured using a ChemiPro system were also presented. To further study the changes in [Ca2+]i induced by NaCl and H2O2 in salt environment, we applied 200 mM NaCl or 4 mM H2O2 to stimulate seedlings grown in MS medium and MS medium containing 40 mM NaCl, respectively (Figure 3). The [Ca2+]i increased immediately and reached the peak at approximately 50 s, and then gradually declined. In the roots, the peak [Ca2+]i reached 5.64 ± 0.31 (×10−2) in MS medium, which was similar to the peak [Ca2+]i in seedlings grown in salt medium [5.31 ± 0.20 (×10−2)] (Figure 3A). In the leaves, the peak value reached 5.52 ± 0.22 (×10−2) in MS medium, which was similar to the root (Figure 3B). Nevertheless, the [Ca2+]i increases were markedly declined in the leaf of the seedlings grown in salt medium (Figure 3C).
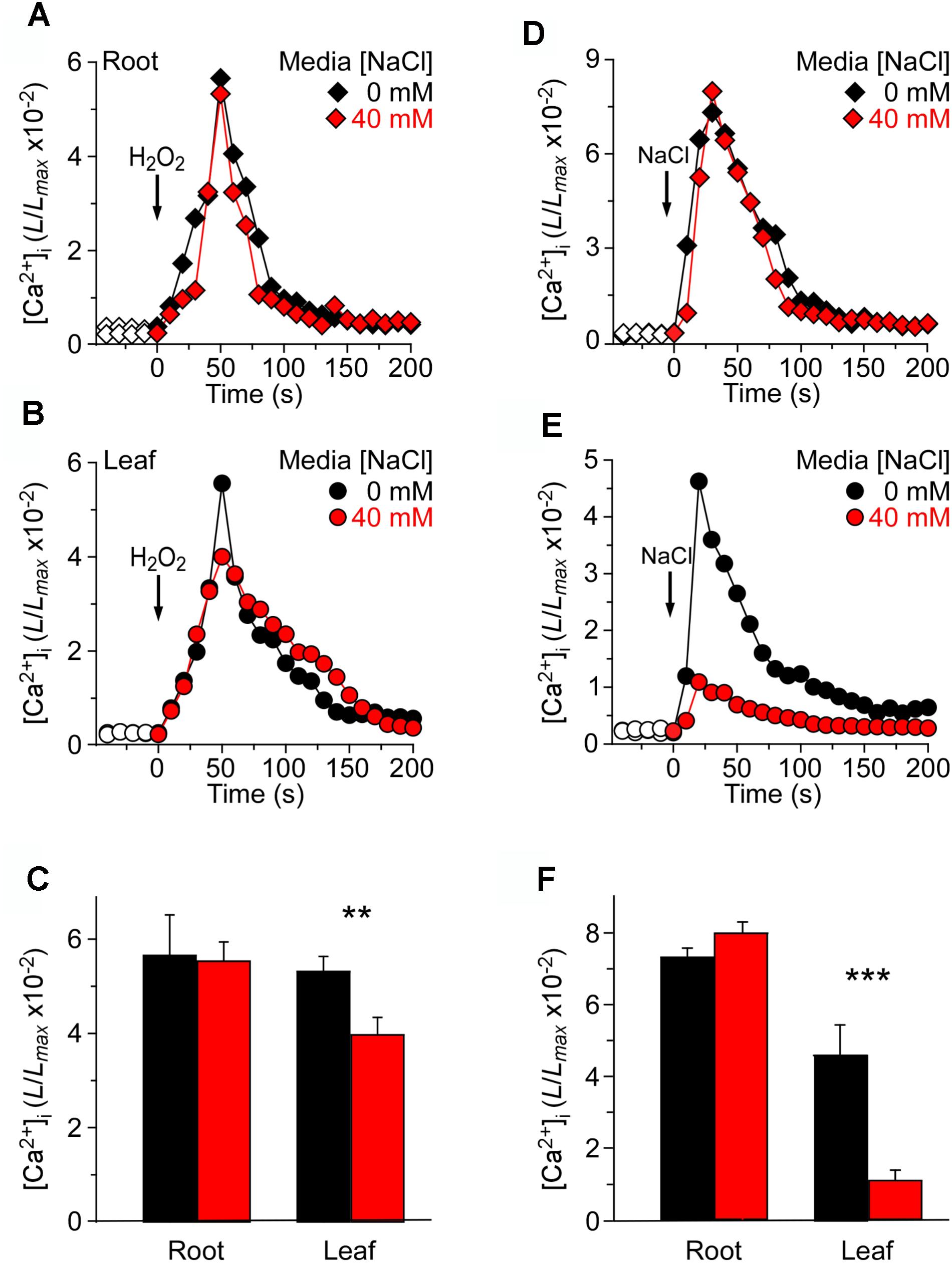
FIGURE 3. Long-term treatment of seedlings by NaCl in the growth media affects [Ca2+]i increases induced by NaCl but not H2O2. (A–C) Arabidopsis seedlings grown in MS media containing 0 or 40 mM NaCl for 9 days were subjected to a 4 mM H2O2 treatment at 0 s, and aequorin luminescence images in roots (A,C) and leaves (B,C) were taken every 10 s throughout the treatment. Representative recordings from individual seedlings were shown. Similar results were seen in four independent experiments using 20 seedlings. (D–F) Similar seedlings as in (A,B) were treated with 200 mM NaCl at time zero, and aequorin images in roots (D,F) and leaves (E,F) were recorded continuously throughout the treatment. Representative recordings from individual seedlings were shown. Similar results were seen in four independent experiments using 20 seedlings (mean ± SD; n = 20; ∗∗∗P < 0.001; ∗∗0.001 < P < 0.01).
At the same time, we analyzed increases in [Ca2+]i in response to 200 mM NaCl in seedlings grown in MS medium and MS medium containing 40 mM NaCl. After the application of 200 mM NaCl, [Ca2+]i was increased immediately, reached a peak at about 20 s, and then declined gradually (Figures 3D,E). Interestingly, although in the root the peak [Ca2+]i reached 7.32 ± 0.35 (×10−2) in the MS medium control, which was similar to the peak [Ca2+]i in the salt medium condition [7.99 ± 0.32 (×10−2)] (Figure 3D), the peak [Ca2+]i was decreased significantly in the leaf under the salt medium condition compared with MS medium (Figures 3E,F). Also, we found that NaCl-induced [Ca2+]i increases in root reached 4.63 ± 0.19 (×10−2), which was higher than that in leaf [1.09 ± 0.11 (×10−2)], although the [Ca2+]i increases of whole seedlings were similar to those under H2O2 treatment.
Long-Term H2O2 Treatment Does Not Alter H2O2- or NaCl-Induced [Ca2+]i Increases
We wished to investigate further how the changes in [Ca2+]i were triggered by NaCl and H2O2 in the oxidative environment. For this, 4 mM H2O2 and 200 mM NaCl were applied to seedlings grown in MS medium and MS medium with 1 mM H2O2, respectively (Figure 4). By analogy, we found that in the root, 4 mM H2O2 induced an immediate and rapid rise in the [Ca2+]i which resulted in similar peaks at about 50 s under MS medium and H2O2 medium conditions, and then declined gradually (Figure 4A). We also found that the temporal dynamics of [Ca2+]i increases was similar in both the root and the leaf (Figures 4A–C). Equally we analyzed the changes in NaCl-induced [Ca2+]i increases in the oxidative environment. The [Ca2+]i in response to 200 mM NaCl under MS medium and MS medium containing 1 mM H2O2 was examined. The [Ca2+]i increased immediately in the root after being treated with 200 mM NaCl, and reached a peak of 7.32 ± 0.33 (×10−2) in normal medium and 8.07 ± 0.26 (×10−2) in H2O2 medium (Figure 4D). However, the [Ca2+]i increases in the leaf were lower than that in the root. Additionally, after 200 mM NaCl treatment, the peak [Ca2+]i that was increased in the H2O2 medium had a remarkable decline compared to that in MS medium (Figure 4F).
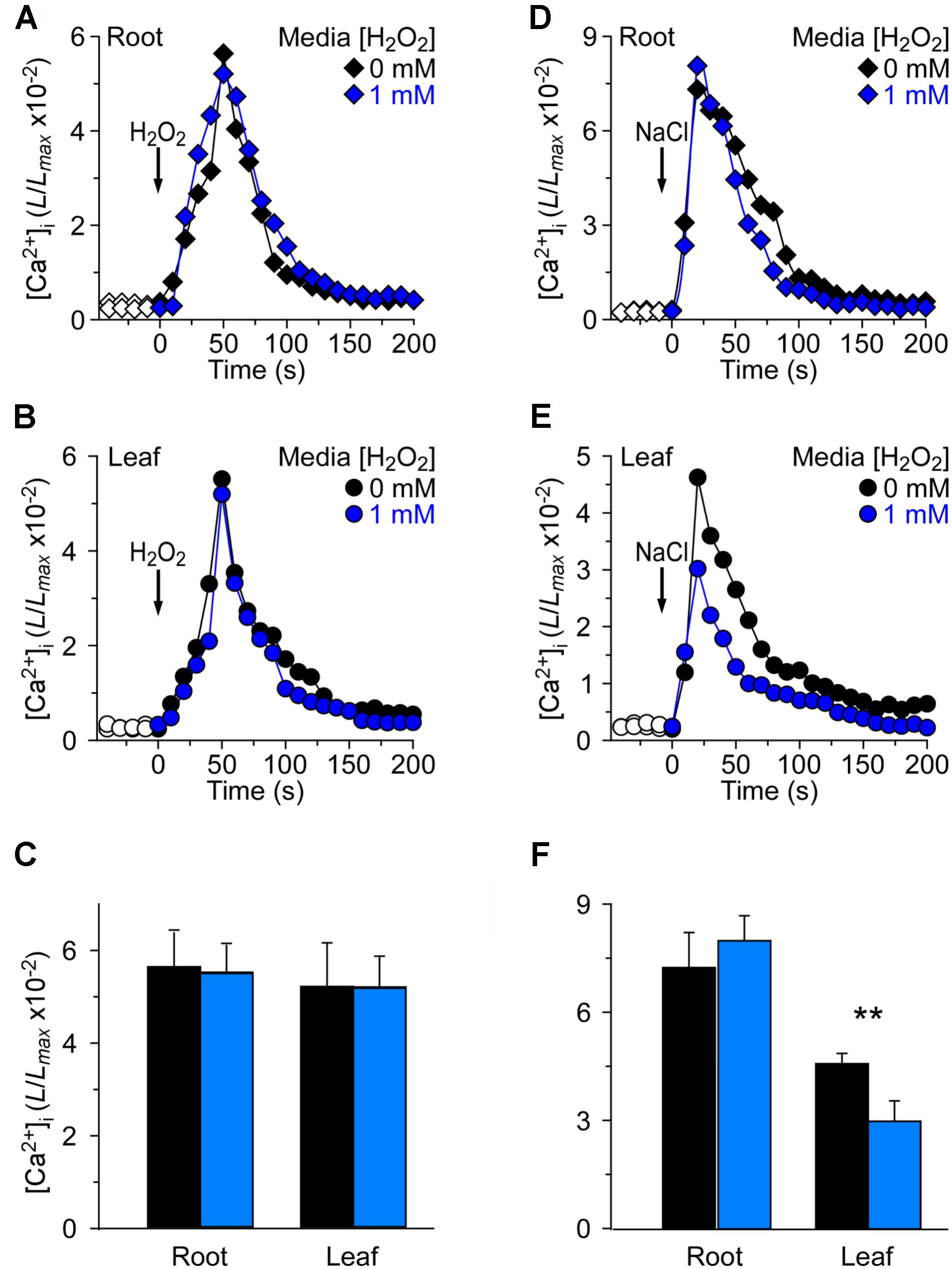
FIGURE 4. Long-term treatment of plants by H2O2 in the growth media has negligible effects on [Ca2+]i increases induced by either H2O2 or NaCl. (A–C) Arabidopsis seedlings grown in MS media containing 0 or 1 mM H2O2 for 9 days were subjected to a 4 mM H2O2 treatment at 0 s, and aequorin luminescence images in roots (A,C) and leaves (B,C) were taken every 10 s throughout the treatment. Representative recordings from individual seedlings were shown. Similar results were seen in four independent experiments using 20 seedlings. (D–F) Similar seedlings as in (A,B) were treated with 200 mM NaCl at time zero, and aequorin images in roots (D,F) and leaves (E,F) were recorded continuously throughout the treatment. Representative recordings from individual seedlings were shown. Similar results were seen in four independent experiments using 20 seedlings (mean ± SD; n = 20; ∗∗0.001 < P < 0.01).
To further assess the reason why the root is more sensitive to NaCl but not H2O2, we measured the expression patterns of Ca2+ signal relative marker genes, such as SOS1, SOS2, SOS3, TPC1, ZAT12, and RBOHD in both the leaf and the root. For ROS stress marker genes, ZAT12, RBOHD, and TPC1, the ZAT12 and RBOHD were all up-regulated both in the leaf and the root, but the TPC1 relative expression was down-regulated in both the leaf and the root (Supplementary Figures S1A,B). It is known that TPC1 expelled Ca2+ from the vacuole to the cytosol in Arabidopsis thaliana, which contributed to long-distance Ca2+ signature (Evans et al., 2016; Guo et al., 2017). The expression of TPC1 was down-regulated, thus Ca2+ released from the vacuole might be also decreased. This might lead to higher cytosolic basal Ca2+ levels, which is clearly not one of the reasons for lower cytosolic Ca2+ seen in Figure 1. Meanwhile, during long-term salt stress, the marker genes, such as SOS1, SOS2, and SOS3, were all up-regulated much more in the root compared to that in the leaf (Supplementary Figures S1C,D). For marker gene TPC1, the expression in the root was reduced, while it was increased in the leaf (Supplementary Figures S1C,D). The TPC1 expression levels may be associated with Ca2+ signaling rather than the basal Ca2+ levels, and more so in the leaf with respect to salt stress (Supplementary Figure S1).
Discussion
Calcium is a critical second messenger in signal transduction in animals and plants (Berridge et al., 2003; Clapham, 2007; McAinsh and Pittman, 2009; Ward et al., 2009). Previous studies have shown that specific stimuli can trigger unique temporal and spatial patterns of [Ca2+]i, known as “[Ca2+]i signatures,” which are thought to function in most aspects of plant growth and development (Tang et al., 2007; Dodd et al., 2010; Jiang et al., 2013). Cytosolic Ca2+ concentrations can be monitored by imaging the Ca2+ indicator aequorin, and the intensity of bioluminescence only depends on the Ca2+ concentration. It is well-known that the basal [Ca2+]i is maintained at 100 nM and about 20,000-fold below the extracellular Ca2+ concentration (Epstein, 1961; Berridge et al., 2003; Hepler, 2005; Clapham, 2007; Swanson et al., 2011). Environmental stimuli activate the Ca2+ channels in general located at the plasma membrane and/or endomembranes, leading to the increases in [Ca2+]i (Hetherington and Brownlee, 2004; Ward et al., 2009). The Ca2+ signature encodes information from the environmental stimuli and is then decoded by intracellular Ca2+ sensors, such as CBL proteins and calmodulins, which initiate the downstream events (Clapham, 2007; Zhu et al., 2013). Over the past decades, a significant progress has been made in understanding the changes in [Ca2+]i that were induced by various abiotic and biotic stresses in plants, including the responses to salt stress, drought, oxidative stress, high and low temperatures, mechanical wounding, and pathogen elicitors (McAinsh and Pittman, 2009; Dodd et al., 2010; Ma and Berkowitz, 2011; Kaya et al., 2014; Yuan et al., 2014). In line with this, we have isolated a mutant with defects in osmotic stress-induced [Ca2+]i increases and identified the osmosensor OSCA1 (Yuan et al., 2014). Interestingly, many abiotic and biotic stresses also induce the accumulation of ROS and generate oxidative stresses, which play an important role in many aspects of plant growth and development (Wise and Naylor, 1987; Mittler, 2002; Vranova et al., 2002; Suzuki et al., 2012; Kaya et al., 2014). For instance, it has been known that salt stress enhances the accumulation of ROS in plants, and hydrogen peroxide has been proposed to function in salt-triggered calcium wave propagations (Vaidyanathan et al., 2003; Borsani et al., 2005; Valderrama et al., 2006; Leshem et al., 2007; Miller et al., 2010; Choi et al., 2016).
It has long been hypothesized that salt stress-triggered increases in [Ca2+]i was involved in the perception of the salt signal, even though much remains to be discovered about the properties of the salt-activated Ca2+ permeable channel and its molecular nature (Zhu, 2001; Munns and Tester, 2008). Additionally, the molecular mechanisms of ROS sensing in plant cells are still unknown, and probably ROS activation of Ca2+ permeable channels may serve as an ROS perception process (Pei et al., 2000; Waring, 2005; Miller et al., 2010). Note that the rapid rise in NaCl-induced [Ca2+]i as well as the NaCl-triggered overproduction of ROS possibly function as critical integrators, mediating stress signal perception, signal transduction, and adaptation (Xie et al., 2011; Ma et al., 2012; Jiang et al., 2013; Ben et al., 2015; Köster et al., 2018). The salt stress-induced [Ca2+]i increases precede the production of H2O2 signaling molecule (Yang and Poovaiah, 2002). NaCl and H2O2 induce [Ca2+]i increases and activate distinct Ca2+ permeable channels (Jiang et al., 2013), suggesting that calcium plays a central role in NaCl and H2O2 signal transduction pathways in plants.
Here we found that basal [Ca2+]i decreased under salt and oxidative stress environments (Figure 1). Considering that the basal [Ca2+]i levels are maintained at approximately 100 nM, the low basal [Ca2+]i levels in plants grown in NaCl medium and H2O2 medium, seen in this study (Figure 1), indicates that the low levels may allow plants to adapt well to salt and oxidative environmental stresses. The Ca2+ sensor proteins with diverse Ca2+ affinities, subcellular localizations, and downstream target specificities perceive [Ca2+]i changes and transduce them into downstream signaling responses (Hashimoto and Kudla, 2011). It seems essential for plants to maintain a lower basal [Ca2+]i under stressed environmental conditions. It is possible that the Ca2+ sensors allow the plant to tightly control the appropriate adaptation to changes and provide a robust response to additional stimuli from the environment. The lower basal [Ca2+]i can only activate high affinity Ca2+ sensors, and thus proportionally promote the high-affinity-Ca2+-sensor-mediated signaling pathways. It remains to be addressed why the basal [Ca2+]i of the root is lowered more than that of the leaf in NaCl medium but the [Ca2+]i of the root and leaf is similar in H2O2 medium (Figures 1B,D). Roots grow in the salt stress soil environment, but the leaf is almost not directly contacted with the salt in the soil. Based on the previous research, the salt is absorbed from the site of application of the gel, which triggers the propagation of the Ca2+ wave and induction of systemic molecular responses in the leaf that are unlikely to reflect direct responses to salt stress (Choi et al., 2014). We consider the initial Ca2+ signatures as the main springboard to further research.
Through the application of different concentrations of stimuli to plants, the relationship between the strength of stimulus and magnitude of [Ca2+]i could be found. The magnitudes of [Ca2+]i increases rely on the strength of stimulus, wherein, higher the strength applied the more considerable is the rise in [Ca2+]i, which is observed until the saturation point is reached (Figure 2), and this is consistent with previous reports (Tracy et al., 2008; Jiang et al., 2013). Therefore, the correlation between stimulus strengths and [Ca2+]i magnitudes may be a common dose effect in plants. Analysis of the dose effect of NaCl-induced [Ca2+]i increases demonstrated that the [Ca2+]i in response to NaCl in the root is dramatically stronger than that in the leaf, and the [Ca2+]i magnitudes between root and leaf are further elevated when the seedlings are grown in the NaCl medium (Figures 2A–C), indicating a sensitization mechanism under the imposed experimental conditions. In contrast, in the presence and absence of H2O2 in the medium, no differences of the [Ca2+]i were found between the roots and leaves in the dose effects of H2O2 in both normal environmental and oxidative conditions, which means that the root and the leaf are neither sensitized nor desensitized when the seedlings were grown in oxidative medium (Figures 2D–F).
We have investigated the spatiotemporal patterns of the NaCl- and H2O2-induced [Ca2+]i increases and demonstrated that both NaCl and H2O2 evoke a transient increase in [Ca2+]i which then decays to a new resting level (Figures 3, 4), similar to those seen in previous studies (Knight et al., 1997; Rentel and Knight, 2004; Jiang et al., 2013). The [Ca2+]i elevations in roots from seedlings grown under 40 mM NaCl medium were similar to those from control medium-grown seedlings after application of H2O2 and NaCl stimulus, respectively (Figure 3). However, the [Ca2+]i elevations within the leaf, induced by both H2O2 and NaCl, significantly declined in NaCl medium compared to control medium. Moreover, the [Ca2+]i elevations of leaf stimulated by NaCl reduced more than that stimulated by H2O2 (Figures 3C,F). These results indicate that the sensitivity of leaf is reduced in salt environmental stress. It is most likely that the NaCl-evoked Ca2+ signature within the leaf might be adapted to salt environmental conditions, but the H2O2-evoked Ca2+ signature within the leaf might be only partly adapted to salt environmental conditions. Furthermore, after application of H2O2 and NaCl, respectively, the [Ca2+]i elevations of roots in the H2O2 medium are similar to those in NaCl medium (Figure 4). However, the H2O2-induced [Ca2+]i in leaves was similar to that in roots (Figures 4A,C) and the NaCl-induced [Ca2+]i in leaves has a marked decline in the H2O2 medium (Figure 4F). These results indicate that the NaCl-evoked Ca2+ signatures within the leaf might partly adapt to oxidative environmental stress condition with significant desensitization, while both the root and the leaf that are stimulated by H2O2 under the oxidative environmental conditions can maintain the same sensitivity as in normal environmental conditions.
Our results suggest that the root is the primary sensing tissue to perceive salt stress, and is more sensitive to salt stress when the seedlings are grown in the salt environment. Previous studies have shown that plants have stronger reactions on pre-exposure to drought stress to reduce water loss from leaves (Ding et al., 2012). In addition, plants have long-term somatic memory in epigenome (Sani et al., 2013). With respect to [Ca2+]i signaling, plants exhibit rapid hyperosmotic-induced Ca2+ responses on pre-exposure to osmotic stress, also called “osmo-sensory potentiation” (Stephan et al., 2016). We found similar potentiation phenomenon in salt stress in the root but desensitization in the leaf. However, the oxidative stresses are different from salt and osmotic stresses – no potentiation and desensitization were observed. It is possible that the mechanistic aspects of salt stress (ionic and osmotic stresses) are shared with osmotic stress. When plants suffer from salt stress, they have to maintain enough [Ca2+]i to transduce the Ca2+ signatures to trigger the downstream events in the whole plants, but too much [Ca2+]i can also become toxic. So we speculate that in the process of plants to adapt to salt stress, the basal [Ca2+]i decreases with pre-exposure to salt stress environment, leading to weaker Ca2+ signaling pathways. We have known that salt stress can trigger the SAA (Systemic acquired acclimation) (Gilroy et al., 2014), which enable whole plants to prepare all of their tissues and cells to an upcoming challenge, even though that may initially only be sensed by root tissues. It may indicate why the basal [Ca2+]i in the leaf are decreased for the future upcoming stimulates.
In comparison with plants that cope with salt stress, our data showed that in the process of plant adaption to ROS stress, they have a delicate ROS balance between their production and scavenging, in which ROS does not need to be stored in a particular compartment and could be rapidly generated and/or removed anywhere in the cell or the apoplast (Choi et al., 2014). We have established that the magnitude of salt stress-induced [Ca2+]i increases and the ROS-induced [Ca2+]i increases are affected by both salt and oxidative environments. We also studied the relationship between salt- and ROS-induced [Ca2+]i increases in leaves and roots, respectively. Our data suggest that previous studies on NaCl- and H2O2-induced [Ca2+]i increases are much more complicated in leaf and root under the salt and oxidative environments (Knight et al., 1997; Rentel and Knight, 2004; Jiang et al., 2013). By imaging [Ca2+]i changes, we found the basal [Ca2+]i decrease was dependent on the saline and oxidative environments. Detailed image analyses and comparisons of the [Ca2+]i increases suggest that the roots are more sensitive than the leaves in response to environmental stress conditions. The increases evoked by NaCl are different from those by H2O2 in salt and oxidative environmental stresses, respectively, indicating that there is a different mechanism in the plant evolution process in response to both long-term and transient stress.
Author Contributions
ZJ and Z-MP designed the experiments. LL performed the research. LL, ZJ, SZ, HZ, and WY collected and analyzed the data. LL, ZJ, JS, and Z-MP wrote the manuscript. All authors discussed the results and contributed to the manuscript.
Funding
This work was supported by grants from Chinese NSF (NSFC31301170) to ZJ, Zhejiang NSF (LZ16C020001), NSF (IOS-1457257), and DOE (DE-SC0014077).
Conflict of Interest Statement
The authors declare that the research was conducted in the absence of any commercial or financial relationships that could be construed as a potential conflict of interest.
Acknowledgments
We thank Drs. Marc R. Knight for Arabidopsis seeds expressing aequorin, members in the Pei Lab for discussion and critical reading of the manuscript, and Douglas M. Johnson and Gary B. Swift for the maintenance of the ChemiPro system.
Supplementary Material
The Supplementary Material for this article can be found online at: https://www.frontiersin.org/articles/10.3389/fpls.2018.01390/full#supplementary-material
FIGURE S1 | Long-term H2O2 and NaCl treatment alters relative genes expression. (A,B) Arabidopsis seedlings grown in MS media containing 0 or 1 mM H2O2 and 40 mM NaCl for 10 days. We measured several salt stress relative gene markers such as SOS1, SOS2, SOS3, and TPC1 in leaf (A) and root (B). They are all up-regulated in root except TPC1, and they are all up-regulated in leaf. Similar results were seen in four independent experiments using 40 seedlings. (C,D) Similarly, we measured ROS stress relative gene markers such as ZAT12, RBOHD, and TPC1 in root and leaf. The ZAT12 and RBOHD are all up-regulated both in leaf (C) and root (D), but the TPC1 relative expression is down-regulated both in leaf (C) and root (D). Similar results were seen in four independent experiments using 40 seedlings.
TABLE S1 | Marker Gene Primer.
Abbreviations
AQ, aequorin; [Ca2+]i, cytosolic free Ca2+ concentration; CaM, calmodulin; CBL, calcineurin B-like; CDPK, Ca2+-dependent protein kinase; CML, calmodulin-like; HpC, hydrogen peroxide (H2O2)-gated Ca2+ channels; NaC, NaCl-gated Ca2+ channels; ROS, reactive oxygen species.
References
Ben, R. K., Lefebvre-De, V. D., Le, D. I., Leprince, A. S., Bordenave, M., Maldiney, R., et al. (2015). Hydrogen peroxide produced by NADPH oxidases increases proline accumulation during salt or mannitol stress in Arabidopsis thaliana. New Phytol. 208, 1138–1148. doi: 10.1111/nph.13550
Ben Rejeb, K., Abdelly, C., and Savoure, A. (2014). How reactive oxygen species and proline face stress together. Plant Physiol. Biochem. 80, 278–284. doi: 10.1016/j.plaphy.2014.04.007
Berridge, M. J., Bootman, M. D., and Roderick, H. L. (2003). Calcium signalling: dynamics, homeostasis and remodelling. Nat. Rev. Mol. Cell Biol. 4, 517–529. doi: 10.1038/nrm1155
Borsani, O., Zhu, J. H., Verslues, P. E., Sunkar, R., and Zhu, J. K. (2005). Endogenous siRNAs derived from a pair of natural cis-antisense transcripts regulate salt tolerance in Arabidopsis. Cell 123, 1279–1291. doi: 10.1016/j.cell.2005.11.035
Bose, J., Rodrigomoreno, A., Lai, D., Xie, Y., Shen, W., and Shabala, S. (2015). Rapid regulation of the plasma membrane H+-ATPase activity is essential to salinity tolerance in two halophyte species, Atriplex lentiformis and Chenopodium quinoa. Ann. Bot. 115, 481–494. doi: 10.1093/aob/mcu219
Cao, X. Q., Jiang, Z. H., Yi, Y. Y., Yang, Y., Ke, L. P., Pei, Z. M., et al. (2017). Biotic and abiotic stresses activate different Ca2+ permeable channels in Arabidopsis. Front. Plant Sci. 8:83. doi: 10.3389/Fpls.2017.00083
Choi, W. G., Hilleary, R., Swanson, S. J., Kim, S. H., and Gilroy, S. (2016). Rapid, long-distance electrical and calcium signaling in plants. Annu. Rev. Plant Biol. 67, 287–307. doi: 10.1146/annurev-arplant-043015-112130
Choi, W. G., Toyota, M., Kim, S. H., Hilleary, R., and Gilroy, S. (2014). Salt stress-induced Ca2+ waves are associated with rapid, long-distance root-to-shoot signaling in plants. Proc. Natl. Acad. Sci. U.S.A. 111, 6497–6502. doi: 10.1073/pnas.1319955111
Deinlein, U., Stephan, A. B., Horie, T., Luo, W., Xu, G., and Schroeder, J. I. (2014). Plant salt-tolerance mechanisms. Trends Plant Sci. 19, 371–379. doi: 10.1016/j.tplants.2014.02.001
Ding, Y., Fromm, M., and Avramova, Z. (2012). Multiple exposures to drought ’train’ transcriptional responses in Arabidopsis. Nat. Commun. 3:740. doi: 10.1038/ncomms1732
Dodd, A. N., Kudla, J., and Sanders, D. (2010). The language of calcium signaling. Annu. Rev. Plant Biol. 61, 593–620. doi: 10.1146/annurev-arplant-070109-104628
Epstein, E. (1961). The essential role of calcium in selective cation transport by plant cells. Plant Physiol. 36, 437–444. doi: 10.1104/pp.36.4.437
Evans, M. J., Choi, W. G., Gilroy, S., and Morris, R. J. (2016). A ROS-assisted calcium wave dependent on the AtRBOHD NADPH oxidase and TPC1 cation channel propagates the systemic response to salt stress. Plant Physiol. 171, 1771. doi: 10.1104/pp.16.00215
Gilroy, S., Suzuki, N., Miller, G., Choi, W. G., Toyota, M., Devireddy, A. R., et al. (2014). A tidal wave of signals: calcium and ROS at the forefront of rapid systemic signaling. Trends Plant Sci. 19, 623–630. doi: 10.1016/j.tplants.2014.06.013
Guo, J., Zeng, W., and Jiang, Y. (2017). Tuning the ion selectivity of two-pore channels. Proc. Natl. Acad. Sci. U.S.A. 114, 1009–1014. doi: 10.1073/pnas.1616191114
Harper, J. F., and Harmon, A. (2005). Plants, symbiosis and parasites: a calcium signalling connection. Nat. Rev. Mol. Cell Biol. 6, 555–566. doi: 10.1038/nrm1679
Hasegawa, P. M., Bressan, R. A., Zhu, J. K., and Bohnert, H. J. (2000). Plant cellular and molecular responses to high salinity. Ann. Rev. Plant Physiol. Plant Mol. Biol. 51, 463–499. doi: 10.1146/annurev.arplant.51.1.463
Hashimoto, K., and Kudla, J. (2011). Calcium decoding mechanisms in plants. Biochimie 93, 2054–2059. doi: 10.1016/j.biochi.2011.05.019
Hepler, P. K. (2005). Calcium: a central regulator of plant growth and development. Plant Cell 17, 2142–2155. doi: 10.1105/tpc.105.032508
Hetherington, A. M., and Brownlee, C. (2004). The generation of Ca2+ signals in plants. Annu. Rev. Plant Biol. 55, 401–427. doi: 10.1146/annurev.arplant.55.031903.141624
Hofer, A. M., and Brown, E. M. (2003). Extracellular calcium sensing and signalling. Nat. Rev. Mol. Cell Biol. 4, 530–538. doi: 10.1038/nrm1154
Jiang, Z. H., Zhu, S., Ye, R., Xue, Y., Chen, A., An, L. Z., et al. (2013). Relationship between NaCl- and H2O2-induced cytosolic Ca2+ increases in response to stress in Arabidopsis. PLoS One 8:e76130. doi: 10.1371/journal.pone.0076130
Johnson, C. H., Knight, M. R., Kondo, T., Masson, P., Sedbrook, J., Haley, A., et al. (1995). Circadian oscillations of cytosolic and chloroplastic free calcium in plants. Science 269, 1863–1865. doi: 10.1126/science.7569925
Kaya, H., Nakajima, R., Iwano, M., Kanaoka, M. M., Kimura, S., Takeda, S., et al. (2014). Ca2+-activated reactive oxygen species production by Arabidopsis RbohH and RbohJ is essential for proper pollen tube tip growth. Plant Cell 26, 1069–1080. doi: 10.1105/tpc.113.120642
Kiep, V., Vadassery, J., Lattke, J., Maass, J. P., Boland, W., Peiter, E., et al. (2015). Systemic cytosolic Ca2+ elevation is activated upon wounding and herbivory in Arabidopsis. New Phytol. 207, 996–1004. doi: 10.1111/nph.13493
Knight, H., and Knight, M. R. (2001). Abiotic stress signalling pathways: specificity and cross-talk. Trends Plant Sci. 6, 262–267. doi: 10.1016/s1360-1385(01)01946-x
Knight, H., Trewavas, A. J., and Knight, M. R. (1997). Calcium signalling in Arabidopsis thaliana responding to drought and salinity. Plant J. 12, 1067–1078. doi: 10.1046/j.1365-313X.1997.12051067.x
Knight, M. R., Campbell, A. K., Smith, S. M., and Trewavas, A. J. (1991). Transgenic plant aequorin reports the effects of touch and cold-shock and elicitors on cytoplasmic calcium. Nature 352, 524–526. doi: 10.1038/352524a0
Köster, P., Wallrad, L., Edel, K. H., Faisal, M., Alatar, A. A., and Kudla, J. (2018). The battle of two ions: Ca2+ signalling against Na+ stress. Plant Biol. doi: 10.1111/plb.12704 [Epub ahead of print].
Kurusu, T., Kuchitsu, K., and Tada, Y. (2015). Plant signaling networks involving Ca2+ and Rboh/Nox-mediated ROS production under salinity stress. Front. Plant Sci. 6:427. doi: 10.3389/fpls.2015.00427
Leshem, Y., Seri, L., and Levine, A. (2007). Induction of phosphatidylinositol 3-kinase-mediated endocytosis by salt stress leads to intracellular production of reactive oxygen species and salt tolerance. Plant J. 51, 185–197. doi: 10.1111/j.1365-313X.2007.03134.x
Ma, L., Zhang, H., Sun, L., Jiao, Y., Zhang, G., Miao, C., et al. (2012). NADPH oxidase AtrbohD and AtrbohF function in ROS-dependent regulation of Na+/K+homeostasis in Arabidopsis under salt stress. J. Exp. Bot. 63, 305–317. doi: 10.1093/jxb/err280
Ma, W., and Berkowitz, G. A. (2011). Ca2( conduction by plant cyclic nucleotide gated channels and associated signaling components in pathogen defense signal transduction cascades. New Phytol. 190, 566–572. doi: 10.1111/j.1469-8137.2010.03577.x
Marti, M. C., Stancombe, M. A., and Webb, A. A. (2013). Cell- and stimulus type-specific intracellular free Ca2+ signals in Arabidopsis. Plant Physiol. 163, 625–634. doi: 10.1104/pp.113.222901
McAinsh, M. R., Clayton, H., Mansfield, T. A., and Hetherington, A. M. (1996). Changes in stomatal behavior and guard cell cytosolic free calcium in response to oxidative stress. Plant Physiol. 111, 1031–1042. doi: 10.1104/pp.111.4.1031
McAinsh, M. R., and Pittman, J. K. (2009). Shaping the calcium signature. New Phytol. 181, 275–294. doi: 10.1111/j.1469-8137.2008.02682.x
Miller, G., Suzuki, N., Ciftci-Yilmaz, S., and Mittler, R. (2010). Reactive oxygen species homeostasis and signalling during drought and salinity stresses. Plant Cell Environ. 33, 453–467. doi: 10.1111/j.1365-3040.2009.02041.x
Mittler, R. (2002). Oxidative stress, antioxidants and stress tolerance. Trends Plant Sci. 7, 405–410. doi: 10.1016/S1360-1385(02)02312-9
Munns, R., and Tester, M. (2008). Mechanisms of salinity tolerance. Annu. Rev. Plant Biol. 59, 651–681. doi: 10.1146/annurev.arplant.59.032607.092911
Pei, Z.-M., Murata, Y., Benning, G., Thomine, S., Klusener, B., Allen, G. J., et al. (2000). Calcium channels activated by hydrogen peroxide mediate abscisic acid signalling in guard cells. Nature 406, 731–734. doi: 10.1038/35021067
Ranf, S., Gisch, N., Schaffer, M., Illig, T., Westphal, L., Knirel, Y. A., et al. (2015). A lectin S-domain receptor kinase mediates lipopolysaccharide sensing in Arabidopsis thaliana. Nat. Immunol. 16, 426–433. doi: 10.1038/ni.3124
Rentel, M. C., and Knight, M. R. (2004). Oxidative stress-induced calcium signaling in Arabidopsis. Plant Physiol. 135, 1471–1479. doi: 10.1104/pp.104.042663
Roderick, H. L., and Cook, S. J. (2008). Ca2+ signalling checkpoints in cancer: remodelling Ca2+ for cancer cell proliferation and survival. Nat. Rev. Cancer 8, 361–375. doi: 10.1038/nrc2374
Ru, Q. M., Pei, Z. M., and Zheng, H. L. (2008). In vitro studies on antioxidant and antimicrobial activities of polysaccharide from Lycoris aurea. Zhong Yao Cai 31, 1536–1540.
Sammels, E., Parys, J. B., Missiaen, L., De Smedt, H., and Bultynck, G. (2010). Intracellular Ca2+ storage in health and disease: a dynamic equilibrium. Cell Calcium 47, 297–314. doi: 10.1016/j.ceca.2010.02.001
Sani, E., Herzyk, P., Perrella, G., Colot, V., and Amtmann, A. (2013). Hyperosmotic priming of Arabidopsis seedlings establishes a long-term somatic memory accompanied by specific changes of the epigenome. Genome Biol. 14:R59. doi: 10.1186/gb-2013-14-6-r59
Schroeder, J. I., Delhaize, E., Frommer, W. B., Guerinot, M. L., Harrison, M. J., Herrera-Estrella, L., et al. (2013). Using membrane transporters to improve crops for sustainable food production. Nature 497, 60–66. doi: 10.1038/nature11909
Stephan, A. B., Kunz, H. H., Yang, E., and Schroeder, J. I. (2016). Rapid hyperosmotic-induced Ca2+ responses in Arabidopsis thaliana exhibit sensory potentiation and involvement of plastidial KEA transporters. Proc. Natl. Acad. Sci. U.S.A. 113, E5242. doi: 10.1073/pnas.1519555113
Suzuki, N., Koussevitzky, S., Mittler, R., and Miller, G. (2012). ROS and redox signalling in the response of plants to abiotic stress. Plant Cell Environ. 35, 259–270. doi: 10.1111/j.1365-3040.2011.02336.x
Swanson, S. J., Choi, W. G., Chanoca, A., and Gilroy, S. (2011). In vivo imaging of Ca2+, pH, and reactive oxygen species using fluorescent probes in plants. Annu. Rev. Plant Biol. 62, 273–297. doi: 10.1146/annurev-arplant-042110-103832
Tang, R. H., Han, S., Zheng, H., Cook, C. W., Choi, C. S., Woerner, T. E., et al. (2007). Coupling diurnal cytosolic Ca2+ oscillations to the CAS-IP3 pathway in Arabidopsis. Science 315, 1423–1426. doi: 10.1126/science.1134457
Tracy, F. E., Gilliham, M., Dodd, A. N., Webb, A. A., and Tester, M. (2008). NaCl-induced changes in cytosolic free Ca2+ in Arabidopsis thaliana are heterogeneous and modified by external ionic composition. Plant Cell Environ. 31, 1063–1073. doi: 10.1111/j.1365-3040.2008.01817.x
Vaidyanathan, H., Sivakumar, P., Chakrabarty, R., and Thomas, G. (2003). Scavenging of reactive oxygen species in NaCl-stressed rice (Oryza sativa L.) - differential response in salt-tolerant and sensitive varieties. Plant Sci. 165, 1411–1418. doi: 10.1016/j.plantsci.2003.08.005
Valderrama, R., Corpas, F. J., Carreras, A., Gomez-Rodriguez, M. V., Chaki, M., Pedrajas, J. R., et al. (2006). The dehydrogenase-mediated recycling of NADPH is a key antioxidant system against salt-induced oxidative stress in olive plants. Plant Cell Environ. 29, 1449–1459. doi: 10.1111/j.1365-3040.2006.01530.x
Vranova, E., Inze, D., and Van Breusegem, F. (2002). Signal transduction during oxidative stress. J. Exp. Bot. 53, 1227–1236. doi: 10.1093/jexbot/53.372.1227
Ward, J. M., Maser, P., and Schroeder, J. I. (2009). Plant ion channels: gene families, physiology, and functional genomics analyses. Annu. Rev. Physiol. 71, 59–82. doi: 10.1146/annurev.physiol.010908.163204
Waring, P. (2005). Redox active calcium ion channels and cell death. Arch. Biochem. Biophys. 434, 33–42. doi: 10.1016/j.abb.2004.08.001
Wise, R. R., and Naylor, A. W. (1987). Chilling-enhanced photooxidation: the peroxidative destruction of lipids during chilling injury to photosynthesis and ultrastructure. Plant Physiol. 83, 272–277. doi: 10.1104/pp.83.2.272
Xie, Y. J., Xu, S., Han, B., Wu, M. Z., Yuan, X. X., Han, Y., et al. (2011). Evidence of Arabidopsis salt acclimation induced by up-regulation of HY1 and the regulatory role of RbohD-derived reactive oxygen species synthesis. Plant J. Cell Mol. Biol. 66, 280–292. doi: 10.1111/j.1365-313X.2011.04488.x
Yang, T., and Poovaiah, B. W. (2002). Hydrogen peroxide homeostasis: activation of plant catalase by calcium/calmodulin. Proc. Natl. Acad. Sci. U.S.A. 99, 4097–4102. doi: 10.1073/pnas.052564899
Yuan, F., Yang, H., Xue, Y., Kong, D., Ye, R., Li, C., et al. (2014). OSCA1 mediates osmotic-stress-evoked Ca2+ increases vital for osmosensing in Arabidopsis. Nature 514, 367–371. doi: 10.1038/nature13593
Zhu, J. K. (2001). Plant salt tolerance. Trends Plant Sci. 6, 66–71. doi: 10.1016/S1360-1385(00)01838-0
Zhu, J. K. (2002). Salt and drought stress signal transduction in plants. Annu. Rev. Plant Biol. 53, 247–273. doi: 10.1146/annurev.arplant.53.091401.143329
Zhu, J. K. (2003). Regulation of ion homeostasis under salt stress. Curr. Opin. Plant Biol. 6, 441–445. doi: 10.1016/S1369-5266(03)00085-2
Zhu, J. K. (2016). Abiotic stress signaling and responses in plants. Cell 167, 313–324. doi: 10.1016/j.cell.2016.08.029
Keywords: Arabidopsis thaliana, calcium imaging, aequorin, calcium signaling, basal cytosolic Ca2+ level, salt stress, oxidative stress
Citation: Liu L, Jiang Z, Zhang S, Zhao H, Yang W, Siedow JN and Pei Z-M (2018) Both NaCl and H2O2 Long-Term Stresses Affect Basal Cytosolic Ca2+ Levels but Only NaCl Alters Cytosolic Ca2+ Signatures in Arabidopsis. Front. Plant Sci. 9:1390. doi: 10.3389/fpls.2018.01390
Received: 08 March 2018; Accepted: 31 August 2018;
Published: 23 October 2018.
Edited by:
Paul E. Verslues, Academia Sinica, TaiwanReviewed by:
Won-Gyu Choi, University of Nevada, Reno, United StatesMelanie Krebs, Centre for Organismal Studies Heidelberg, Germany
Copyright © 2018 Liu, Jiang, Zhang, Zhao, Yang, Siedow and Pei. This is an open-access article distributed under the terms of the Creative Commons Attribution License (CC BY). The use, distribution or reproduction in other forums is permitted, provided the original author(s) and the copyright owner(s) are credited and that the original publication in this journal is cited, in accordance with accepted academic practice. No use, distribution or reproduction is permitted which does not comply with these terms.
*Correspondence: Zhen-Ming Pei, enBlaUBkdWtlLmVkdQ==
†These authors have contributed equally to this work