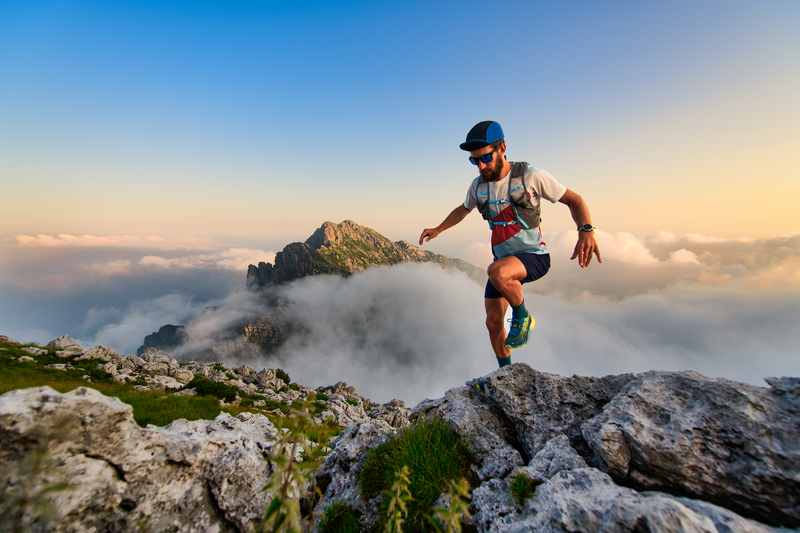
95% of researchers rate our articles as excellent or good
Learn more about the work of our research integrity team to safeguard the quality of each article we publish.
Find out more
PERSPECTIVE article
Front. Plant Sci. , 08 August 2018
Sec. Plant Cell Biology
Volume 9 - 2018 | https://doi.org/10.3389/fpls.2018.01171
Plant growth and development are dependent on chloroplast development and function. Constitutive high level accumulation of a chloroplast stress signal, 3′-phosphoadenosine-5′-phosphate (PAP), confers drought tolerance to plants, but slow downs and alters plant growth and development. PAP, a by-product of sulfur metabolism, is maintained at very low levels by the SAL1 phosphatase during vegetative growth of Arabidopsis and accumulates in rosettes during drought and excess light. Eight independent forward genetic screens in Arabidopsis identified SAL1 as the regulator of multiple phenotypes related to stress responses, hormonal signaling and/or perception. In this perspective article, we collate all the sal1 phenotypes published in the past two decades, and distill the different pathways affected. Our meta-analysis of publicly available sal1 microarray data coupled to preliminary hormonal treatment and profiling results on sal1 indicate that homeostasis and responses to multiple hormones in sal1 are altered during rosette growth, suggesting a potential connection between SAL1-PAP stress retrograde pathway and hormonal signaling. We propose the SAL1-PAP pathway as a case study for integrating chloroplast retrograde signaling, light signaling and hormonal signaling in plant growth and morphogenesis.
Plants are autotrophs that capture light energy from the sun via photosynthesis. The quantity (intensity) and quality (wavelength) of light can affect the assembly and efficiency of photosynthetic machineries in the chloroplasts (Dietz, 2015), which in turn will determine the amount of carbon available for plants to convert into energy for growth and survival. When optimizing and fine-tuning resource allocation for photosynthesis, plants have to account for both the efficiency of light-harvesting through to electron transport chain under limiting conditions such as low light intensities, and the risk of photodamage by reactive oxygen species (ROS) when conditions flip to the other extremes of excessive light exposure and drought. As such, it has been proposed that the chloroplast can act as an environmental sensor that subsequently triggers downstream mechanisms for adjustment of plant function and growth according to the environment. Hence, constant communication in the form of anterograde (nucleus to organelles) and retrograde (organelles to nucleus) signaling takes place between chloroplasts and the nucleus to coordinate and maintain cellular function (Chan et al., 2016b).
Leaf/rosette growth and development appears closely associated with chloroplast development and signaling, as well as with the quantity and quality of light and hormonal levels. Phototropism is a classic example of this, which requires mature chloroplasts (Jin et al., 2001), light signaling and hormonal regulation to take place (Fankhauser and Christie, 2015). There is emerging evidence for developmental abnormalities resulting from changes in chloroplast development (Larkin, 2014; Pogson et al., 2015) and from the presence of cells with non-detectable plastids (Chen et al., 2009). Chloroplasts derived from undeveloped proplastids can propagate via division in a light-responsive manner (Hashimoto and Possingham, 1989; Pogson et al., 2015). Both chloroplast development and plant growth are light-dependent, involving phytochrome-mediated signaling (Okello et al., 2016); and all of these are respectively linked to growth hormonal signaling (Halliday and Fankhauser, 2003; Lau and Deng, 2010; Jiang et al., 2012; Yoshizawa et al., 2014). The key growth-regulating hormones in plants are gibberellic acids (GA), brassinosteroids (BR), and auxin; and some of this hormonal signaling pathway such as GA- and BR-signaling has been demonstrated to crosstalk with light signaling pathway in modulating plant growth (de Lucas et al., 2008; Feng et al., 2008; Bai et al., 2012). However, direct connections that integrate chloroplast retrograde signaling, light signaling and hormonal signaling in regulating plant growth and development are yet to be defined.
Altered levels of chloroplast retrograde signal(s), functioning in either chloroplast biogenesis or operation, ultimately contribute to changes in plant growth and development. For example, Arabidopsis mutants over-accumulating plastid signals such as heme (Chory et al., 1989; Woodson et al., 2011; Espinas et al., 2012), methylerythritol cyclodiphosphate (MEcPP) (Gil et al., 2005; Xiao et al., 2012; Bjornson et al., 2017), dihydroxyacetone phosphate (DHAP) (Chen and Thelen, 2010; Vogel et al., 2014), or 3′-phosphoadenosine 5′-phosphate (PAP) (Rossel et al., 2006; Wilson et al., 2009; Estavillo et al., 2011) are smaller in rosette size with varying extents of altered rosette morphology. As some of these retrograde signals such as MEcPP (Xiao et al., 2012) and PAP (Estavillo et al., 2011) accumulate upon excess light exposure, perhaps these chloroplast retrograde signaling mutants could be used to dissect if and how chloroplast retrograde, light and hormonal signaling crosstalk with one another to impact on plant morphogenesis.
PAP in Arabidopsis is generated from the secondary sulfur metabolism pathway (Kopriva and Gigolashvili, 2016) when sulfotransferases (SOTs) transfer the sulfate group from 3′-phosphoadenosine-5′-phosphosulfate (PAPS) to other acceptor molecules such as desulfoglucosinolates, salicylic acid (SA), and BR (Chan et al., 2013). Under standard growth conditions, PAP is maintained at very low levels by the SAL1 phosphatase, which degrades PAP into adenosine monophosphate (AMP) and inorganic phosphate (Pi). This degradation occurs in chloroplasts and mitochondria, where SAL1 is localized (Chen et al., 2011; Estavillo et al., 2011). Redox-regulation of SAL1 activity allows for its inactivation during oxidative stress in these organelles, causing accumulation of PAP under drought and excess light stresses (Estavillo et al., 2011; Chan et al., 2016a). Genetic studies demonstrate that PAP is able to move intracellularly, targeting nuclear exoribonucleases (XRNs) to up-regulate stress-responsive genes during drought (Estavillo et al., 2011). Multiple independent studies on PAP-accumulating sal1 mutants suggest additional roles for this metabolite and its catabolic enzyme.
Altered phenotypes related to stress (Lee et al., 1999; Xiong et al., 2001, 2004; Wilson et al., 2009), RNA metabolism (Gy et al., 2007), nutrient uptake (Hirsch et al., 2011), leaf morphology (Robles et al., 2010) or plant hormones (Rodríguez et al., 2010; Zhang et al., 2011) have been associated with mutations in SAL1, also known by a diversity of gene names arising from the different alleles characterized (Supplementary Table S1) – hos2 (high expression of osmotically responsive genes), fry1 (fiery 1), alx8 (altered expression of APX2 8), ron1 (rotunda 1), fou8 (fatty acid oxygenation up-regulated), and supo1 (suppressor of PIN1 overexpression phenotype). Despite the different ecotype backgrounds such as Col-0 (fry1-4, -5, -6, alx8, fou8, supo1) (Rossel et al., 2006; Gy et al., 2007; Wilson et al., 2009; Rodríguez et al., 2010; Zhang et al., 2011), Ler (ron1) (Robles et al., 2010), C24 (hos2, fry1-1. -2, -3) (Lee et al., 1999; Xiong et al., 2001, 2004), and Ws (fry1-7) (Hirsch et al., 2011), all sal1 knockout mutants consistently display the following developmental phenotypes: delayed development and flowering, more compact rosette with shorter petiole length and rounder leaf shape. Additionally, sal1 was observed to have thicker leaves (Wilson et al., 2009), open venation patterning on leaves and compromised apical dominance based on its increased number of secondary inflorescences compared to wild type (Robles et al., 2010). Altered root morphology was also observed in sal1 mutants (Robles et al., 2010; Hirsch et al., 2011; Zhang et al., 2011). These growth defects coincide with SAL1 expression being detected in all cell types of wild type Arabidopsis throughout development, with higher expression in vascular or vein tissue of leaves and stamens of flowers (Xiong et al., 2001).
Some sal1 growth phenotypes are related to light responses. Kim and von Arnim (2009) showed that sal1 hypocotyl elongation responded similarly to wild type under white light, but was hypersensitive to low-intensity red light, and to a lesser extent, far red and blue light respectively. Crossing a light perception mutant, phytochrome b (phyb), which has much longer petioles, with the sal1 mutant yielded a partial reversion of the altered sal1 rosette morphology. Additionally, Chen and Xiong (2011) demonstrated that an additional long hypocotyl 5 (hy5) mutation in the sal1 mutant can suppress the enhanced light sensitivity of sal1 hypocotyl elongation, but not its altered rosette phenotype. This suggests that sal1 may indeed exhibit altered light perception, signaling or response. As circadian regulation is closely regulated by light signaling (Millar, 2004; Dalchau et al., 2010; Bordage et al., 2016), the effect of PAP accumulation on slightly delaying circadian period (Litthauer et al., 2018) provides further evidence for potential crosstalk between SAL1/PAP and light signaling, although the mechanism remains unclear. Interestingly, a decrease in transitory starch granules was detected in sal1 chloroplasts (Wilson et al., 2009); whether this could be due to this recently reported circadian phenotype of sal1 deserves further investigation since starch metabolism is also closely associated to light and circadian regulation (Webb and Satake, 2015).
The discovery of PAP as a chloroplast stress retrograde signal was initiated when a sal1 knockout mutant alx8 was identified as having constitutive up-regulated expression of ascorbate peroxidase 2 (APX2) – a chloroplast stress marker gene (Rossel et al., 2006; Wilson et al., 2009; Estavillo et al., 2011). Soil-grown sal1 plants can survive 50% longer than wild type during a terminal drought by retaining water more efficiently and maintaining photosynthetic activity (Rossel et al., 2006; Wilson et al., 2009). Consistent with its improved drought tolerance, sal1 has lower stomatal conductance (Rossel et al., 2006; Pornsiriwong et al., 2017), higher accumulation of various osmoprotectants such as putrescine (Wilson et al., 2009), antioxidants and ROS detoxifying enzymes (Rodríguez et al., 2010), jasmonic acid (JA) (Rodríguez et al., 2010) and an inconsistent increase in abscisic acid (ABA) (Rossel et al., 2006; Pornsiriwong et al., 2017). The stress- and ABA-related phenotypes of sal1 were initially hypothesized to be due to inositol polyphosphates (Xiong et al., 2001), which are candidate secondary substrates of SAL1 in vitro (Quintero et al., 1996). This initial hypothesis has been discounted by multiple independent studies that have shown the primary in vivo substrate of SAL1 is PAP, with any change in inositols likely to be indirect secondary effects of SAL1 inactivation (Kim and von Arnim, 2009; Rodríguez et al., 2010; Chen et al., 2011; Estavillo et al., 2011; Lee et al., 2012; Litthauer et al., 2018).
The SAL1-PAP retrograde pathway has been shown to interact with the ABA signaling pathway. Pornsiriwong et al. (2017) demonstrated that PAP-XRN signaling restores ABA-responsiveness of ABA-insensitive mutants [ABA insensitive 1 (abi1), open stomata 1 (ost1)] for stomatal closure; and genetic and exogenous manipulation of PAP increase ABA-responsiveness of seed germination in Arabidopsis. Meanwhile, Chen et al. (2011) observed that the up-regulated ABA signaling in sal1 requires functional ABA Hypersensitive 1 (ABH1), an mRNA cap binding protein that is involved in ABA signaling. These are in line with Wawer et al. (2018)’s observation that both mRNA decapping and 5′–3′ decay contribute to the regulation of ABA signaling. It will be interesting to further dissect the details of how SAL1-PAP intersects with ABA signaling pathway via its impact on RNA metabolism and whether or not other RNA-independent signaling components are involved.
Evidence presented thus far indicate that the SAL1-PAP chloroplast retrograde pathway crosstalks with light signaling for growth regulation and ABA signaling for stress response regulation. There are other biochemical and physiological phenotypes of sal1: earlier onset of increase in JA levels during vegetative growth (Rodríguez et al., 2010), altered auxin perception/response (Robles et al., 2010), reduced sensitivity toward ethylene (Chen and Xiong, 2010), altered sulfur metabolism (Lee et al., 2012) and enhanced susceptibility toward pathogen attacks (Bruggeman et al., 2016; Ishiga et al., 2017). Evidently, multiple hormonal homeostasis and/or signaling are altered in sal1 and it is common for hormones to crosstalk with one another in coordinating plant growth and stress responses (Jaillais and Chory, 2010; Depuydt and Hardtke, 2011). Therefore, we hypothesized that SAL1-PAP retrograde pathway could also interact with growth hormonal signaling pathway for modulating plant growth.
We investigated whether sal1 growth phenotypes could be an output of deficiency or altered perception/signaling in growth-promoting hormones. For example, the sal1 mutant rosette morphology resembles that of BR-insensitive or -deficient mutants (Caño-Delgado et al., 2004; Rodríguez et al., 2010) and sal1 leaf venation patterning and lateral root formation shares similarities to mutants with altered auxin homeostasis (Robles et al., 2010). Indeed, BR-up-regulated genes are down-regulated in sal1 and vice versa (Robles et al., 2010). Therefore, we supplemented soil-grown sal1 mutant (fry1-6) with the three growth promoting hormones – GA3, auxin [indole-3-acetic acid (IAA)] and epi-brassinolide (EBR) – individually and in combination (at least 10 biological replicates per treatment) from 2 weeks old onwards under 16 h photoperiod, as per method described (Ribeiro et al., 2012). We found that sal1 rosette growth improved the most under the triple hormone treatment, resulting in the largest rosette area after 3 weeks of growth relative to the blank treatment control, followed by GA3 and EBR treatments (Figure 1). Importantly, the response to the hormonal treatments was different in sal1; with sal1 growth complemented best by the triple hormone treatment compared to GA3 alone for wild type (seven biological replicates per treatment) (Figure 1 and Supplementary Figure S1). Furthermore, none of the treatments tested here completely rescued sal1 growth phenotypes.
FIGURE 1. Rosette growth of sal1 mutant under different hormonal treatment. Soil grown sal1-6 (fry1-6 allele) mutant were sprayed with different hormones (50 μM of GA3, 50 μM of IAA, or 1 μM of EBR individually, or all of the three hormones together) or blank control [containing 0.5% (v/v) ethanol, which was used to dissolve the commercially available hormones, and 0.1% (v/v) Tween-20] from 2 weeks old onwards (three times per week). The rosette growth since treatment were quantified via LemnaTec Scanalyzer for 3.5 weeks (until bolting stage) based on rosette area (full method see, Phua et al., 2018). Average rosette area ± SD of at least 10 plants per treatment are shown. Significant differences from blank treatment at 38 days old (ANOVA – Dunnett test [GraphPad InStat]) are indicated by ∗∗p < 0.01 and ∗p < 0.05. Example photos of sal1 plants at 31 days old (after 16 days of hormonal treatment).
Partial phenotypic reversion of sal1 was also achieved by crossing the sal1 mutant with the allene oxide synthase (aos) mutant, which has impaired JA biosynthesis and accumulation (Rodríguez et al., 2010). The resulting double mutants have rosette morphology that is partially reverted to wild type-like, specifically the rosette is less compact and has less anthocyanin accumulation but remain smaller than wild type. Hence, part of the altered rosette morphology of sal1 is contributed by the over-accumulation of JA although Ishiga et al. (2017) reported impaired JA signaling in addition to lower levels of SA in sal1 seedlings that correlate with increased its susceptibility to pathogenic attack.
When we analyzed two independent published microarray data of sal1 in Col-0 ecotype background [alx8] (Wilson et al., 2009; Estavillo et al., 2011), we found at least 1500 genes commonly mis-regulated in the same direction (∼960 up-regulated, ∼550 down-regulated) despite some slight differences in plant age (juvenile-adult vs. adult-flowering stages) and photoperiod (12 vs. 16 h light) (Supplementary Table S2). GO enrichment analyses using DAVID Functional Annotation Clustering revealed that genes involved in translation, defense response, ADP binding and tryptophan biosynthetic processes are significantly enriched in alx8 transcriptomes (Supplementary Table S3), which correlates with the pleiotropic sal1 phenotypes discussed earlier. A substantial number of different hormonal biosynthetic and degradation/inactivation genes (as annotated in the AraCyc/Plant Cyc database) are mis-regulated in the alx8 microarray data. These include up-regulation of genes involved in biosynthesis of ABA, auxin, JA, and GA (CYP88A3 – ent-kaurenoic acid hydroxylase 1); while one BR-inactivation gene and a key chloroplast-localized GA biosynthetic gene GA Requiring 2 (GA2; also known as ent-kaurene synthase) are down-regulated (Supplementary Table S4). Since the GA biosynthetic gene CYP88A3 that is up-regulated in alx8 is positioned downstream of GA2 in the GA biosynthetic pathway, the up-regulation of CYP88A3 is likely a feedback response to the reduced substrate availability.
In order to verify if these transcriptomic alterations impacted the sal1 hormonal profile, we performed quantifications of six different hormones (ABA, JA, auxin, GA, cytokinin, and SA) from leaves of two sal1 mutant alleles (alx8 and fry1-6) in Col-0 (n = 3 to 5 biological replicates) as per method described in (Kanno et al., 2010) (Table 1). Our data revealed for the first time that the sal1 mutants have significantly higher auxin (IAA) and lower GA (GA4) levels relative to the Col-0 control, which correlates with the transcriptomic data. Additionally, we observed higher means and substantive increases in the standard deviations relative to mean for the stress hormones (JA-Ile, JA, and ABA), to an extent that renders JA and JA-Ile levels in sal1 to be not statistically different compared to wild type. Variable increases in ABA and JA have already been reported (Rossel et al., 2006; Rodríguez et al., 2010; Ishiga et al., 2017; Pornsiriwong et al., 2017) and this variability would suggest indirect genotype/development/environmental influences on these hormonal content more than direct PAP signaling regulation of biosynthesis. In this context it is relevant that the stomatal regulation by PAP is primarily due to changes in ABA signaling, not ABA levels (Pornsiriwong et al., 2017). In contrast, there was no change in the content for SA and cytokinins (t-zeatin and isopentenyladenine) in sal1 lines compared to that of wild type (Table 1). Collectively, our hormonal profiling data indicate that sal1 has altered hormonal homeostasis not only in an abiotic stress hormone (ABA) but also in growth regulating hormones (auxin and GA) during the juvenile-to-adult stage of development, which are consistent with the sal1 transcriptome (Supplementary Table S4) and growth complementation by GA and triple hormone treatment (Figure 1).
TABLE 1. Hormonal quantification results in fold-change for sal1 mutants in comparison to Col-0 wild type at 4 weeks old.
When considering our hormonal quantification data together with published values it is likely that the effect of PAP accumulation on hormonal homeostasis could be developmental-stage dependent. In contrast to our IAA quantification at the adult stage, Chen and Xiong (2010) did not report differences in auxin levels between the sal1 mutant and wild type at a more juvenile stage. However, sal1 mutant has altered auxin perception (Robles et al., 2010), which could contribute to the increased IAA levels quantified at a later developmental stage, assuming factors like ecotype and growth conditions are negligible. Similarly, no significant changes in SA levels were detected in our experiments in contrast to lower SA levels reported by Ishiga et al. (2017) in sal1 2-week-old seedlings. Since quantification of JA precursor production revealed that the extent of metabolic rate alteration in sal1 differs between developmental stages (Rodríguez et al., 2010) – similar to wild type early on but increase later on – the disparities between the hormonal levels quantified could be due to the different developmental stages of sal1 characterization. Whether or not the increased JA levels at later developmental stages contribute to a readjustment in SA levels in sal1 require further investigation. Therefore, a more systematic and comprehensive profiling of hormones and adenosines throughout sal1 and wild type developmental stages is necessary.
What are the possible intersection points between SAL1-PAP chloroplast signaling, hormonal and light signaling? The signaling network is likely to be complex and comprise several components which have been demonstrated to contribute to sal1 phenotypes; and participate in both hormonal and light signaling. In our view, tempting candidates would include, but not be limited to, the aforementioned RNA metabolism, PHYB, HY5, as well as DELLA and BR signaling proteins.
Our observation of lower GA levels in sal1 could suggest for more abundant DELLA proteins in sal1 compared to wild type since GA promotes DELLA degradation. DELLA proteins can physically interact with key hormone-responsive transcription factors such as JA ZIM-domain family proteins (JAZs) (Hou et al., 2010) and brassinazole resistant 1 (BZR1) (Bai et al., 2012; Gallego-Bartolomé et al., 2012; Li et al., 2012) to promote JA signaling, which can feedback into promoting DELLA expression (Wild et al., 2012) and repress BR signaling (Supplementary Figure S2). Intriguingly, DELLA proteins also mediate light responses via their interactions with phytochrome-interacting factors (PIFs) proteins (de Lucas et al., 2008; Feng et al., 2008). Furthermore, constitutive higher DELLA accumulation as a result of low GA levels can activate ABA biosynthesis (Piskurewicz et al., 2008) and confer drought tolerance to Arabidopsis (Colebrook et al., 2014). These features correlate with multiple reported growth and hormonal-related phenotypes of sal1 discussed above, and it is interesting to note that transcript levels of a few DELLA proteins are up-regulated in alx8 (Estavillo et al., 2011). However, whether this transcriptional up-regulation genuinely reflects increased protein abundance is unclear and detailed work is required to mechanistically dissect how PAP and GA together with other hormonal signaling are interlinked.
It will also be critical to further unravel the interaction between PAP and the secondary messengers ROS and Ca2+. Both of these messengers are known to be key components of hormonal signaling. They are also involved in light signaling and can crosstalk with one another for cellular signaling (Mazars et al., 2010; Gilroy et al., 2014; Görlach et al., 2015). It is intriguing that PAP has contrasting effects on ROS depending on cell and tissue type; inducing a ROS burst in guard cells associated with the restoration of stomatal closure in ABA insensitive mutants (Pornsiriwong et al., 2017) while suppressing ROS levels in vascular tissue (Wilson et al., 2009). Similarly, increased Ca2+ levels in roots was reported in one of the mutant alleles of sal1 (Zhang et al., 2011), but exogenous PAP treatment does not induce any significant changes in cytosolic Ca2+ transients in guard cells (Pornsiriwong et al., 2017). Intracellular ROS and Ca2+ homeostasis are known to regulate cell growth in leaves and germinating seed (Conn et al., 2011; Leymarie et al., 2012); though the exact mechanisms are still unclear. It will be interesting to explore whether and how PAP can contribute to these processes.
The numerous studies on sal1 to date reveal that the SAL1-PAP chloroplast retrograde signaling pathway in Arabidopsis can intersect with light signaling and hormonal signaling pathway in affecting, if not regulating, plant growth and development. While chloroplast retrograde, light and hormonal signaling pathways have been studied independently, and to some extent in a two-way crosstalk manner in the past; the SAL1-PAP pathway may provide the platform to investigate crosstalk between the three pathways altogether in regulating plant growth and development in response to environmental changes (i.e., sun vs. shade) at the molecular level.
SYP performed the transcriptomic mining and hormonal treatment experiment. DY and EN performed the hormonal quantification of sal1. SYP, KXC, GME, and BJP led planning, analysis, and manuscript preparation.
We received financial support from the ARC Centre of Excellence in Plant Energy Biology (CE140100008) and scholarships to SYP (ANU), and KXC (ANU). KXC was also funded by a Postdoctoral Fellowship from Research Foundation-Flanders (FWO).
The authors declare that the research was conducted in the absence of any commercial or financial relationships that could be construed as a potential conflict of interest.
The authors acknowledge Ms. Ayako Nambara (University of Toronto) for her technical assistance to quantify plant hormones.
The Supplementary Material for this article can be found online at: https://www.frontiersin.org/articles/10.3389/fpls.2018.01171/full#supplementary-material
Bai, M.-Y., Shang, J.-X., Oh, E., Fan, M., Bai, Y., Zentella, R., et al. (2012). Brassinosteroid, gibberellin and phytochrome impinge on a common transcription module in Arabidopsis. Nat. Cell Biol. 14, 810–817. doi: 10.1038/ncb2546
Bjornson, M., Balcke, G. U., Xiao, Y., de Souza, A., Wang, J. Z., Zhabinskaya, D., et al. (2017). Integrated omics analyses of retrograde signaling mutant delineate interrelated stress-response strata. Plant J. 91, 70–84. doi: 10.1111/tpj.13547
Bordage, S., Sullivan, S., Laird, J., Millar, A. J., and Nimmo, H. G. (2016). Organ specificity in the plant circadian system is explained by different light inputs to the shoot and root clocks. New Phytol. 212, 136–149. doi: 10.1111/nph.14024
Bruggeman, Q., Mazubert, C., Prunier, F., Lugan, R., Chan, K. X., Phua, S. Y., et al. (2016). Chloroplasts activity and PAP-signaling regulate programmed cell death in Arabidopsis. Plant Physiol. 170, 1745–1756. doi: 10.1104/pp.15.01872
Caño-Delgado, A., Yin, Y., Yu, C., Vafeados, D., Mora-García, S., Cheng, J.-C., et al. (2004). BRL1 and BRL3 are novel brassinosteroid receptors that function in vascular differentiation in Arabidopsis. Development 131, 5341–5351. doi: 10.1242/dev.01403
Chan, K. X., Mabbitt, P. D., Phua, S. Y., Mueller, J. W., Nisar, N., Gigolashvili, T., et al. (2016a). Sensing and signaling of oxidative stress in chloroplasts by inactivation of the SAL1 phosphoadenosine phosphatase. Proc. Natl. Acad. Sci. U.S.A. 113, E4567–E4576. doi: 10.1073/pnas.1604936113
Chan, K. X., Phua, S. Y., Crisp, P., Mcquinn, R., and Pogson, B. J. (2016b). Learning the languages of the chloroplast?: retrograde signaling and beyond. Annu. Rev. Plant Biol. 67, 25–53. doi: 10.1146/annurev-arplant-043015-111854
Chan, K. X., Wirtz, M., Phua, S. Y., Estavillo, G. M., and Pogson, B. J. (2013). Balancing metabolites in drought: the sulfur assimilation conundrum. Trends Plant Sci. 18, 18–29. doi: 10.1016/j.tplants.2012.07.005
Chen, H., and Xiong, L. (2010). The bifunctional abiotic stress signalling regulator and endogenous RNA silencing suppressor FIERY1 is required for lateral root formation. Plant. Cell Environ. 33, 2180–2190. doi: 10.1111/j.1365-3040.2010.02218.x
Chen, H., and Xiong, L. (2011). Genetic interaction of two abscisic acid signaling regulators, HY5 and FIERY1, in mediating lateral root formation. Plant Signal. Behav. 6, 123–125. doi: 10.4161/psb.6.1.14231
Chen, H., Zhang, B., Hicks, L. M., and Xiong, L. (2011). A nucleotide metabolite controls stress-responsive gene expression and plant development. PLoS One 6:e26661. doi: 10.1371/journal.pone.0026661
Chen, M., and Thelen, J. J. (2010). The plastid isoform of triose phosphate isomerase is required for the postgerminative transition from heterotrophic to autotrophic growth in Arabidopsis. Plant Cell 22, 77–90. doi: 10.1105/tpc.109.071837
Chen, Y., Asano, T., Fujiwara, M. T., Yoshida, S., MacHida, Y., and Yoshioka, Y. (2009). Plant cells without detectable plastids are generated in the crumpled leaf mutant of Arabidopsis thaliana. Plant Cell Physiol. 50, 956–969. doi: 10.1093/pcp/pcp047
Chory, J., Peto, C. A., Ashbaugh, M., Saganich, R., Pratt, L., and Ausubel, F. (1989). Different roles for phytochrome in etiolated and green plants deduced from characterization of Arabidopsis thaliana mutants. Plant Cell 1, 867–880. doi: 10.1105/tpc.1.9.867
Colebrook, E. H., Thomas, S. G., Phillips, A. L., and Hedden, P. (2014). The role of gibberellin signalling in plant responses to abiotic stress. J. Exp. Biol. 217, 67–75. doi: 10.1242/jeb.089938
Conn, S. J., Gilliham, M., Athman, A., Schreiber, A. W., Baumann, U., Moller, I., et al. (2011). Cell-specific vacuolar calcium storage mediated by CAX1 regulates apoplastic calcium concentration, gas exchange, and plant productivity in Arabidopsis. Plant Cell 23, 240–257. doi: 10.1105/tpc.109.072769
Dalchau, N., Hubbard, K. E., Robertson, F. C., Hotta, C. T., Briggs, H. M., Stan, G.-B., et al. (2010). Correct biological timing in Arabidopsis requires multiple light-signaling pathways. Proc. Natl. Acad. Sci. U.S.A. 107, 13171–13176. doi: 10.1073/pnas.1001429107
de Lucas, M., Davière, J.-M., Rodríguez-Falcón, M., Pontin, M., Iglesias-Pedraz, J. M., Lorrain, S., et al. (2008). A molecular framework for light and gibberellin control of cell elongation. Nature 451, 480–484. doi: 10.1038/nature06520
Depuydt, S., and Hardtke, C. S. (2011). Hormone signalling crosstalk in plant growth regulation. Curr. Biol. 21, R365–R373. doi: 10.1016/j.cub.2011.03.013
Dietz, K. J. (2015). Efficient high light acclimation involves rapid processes at multiple mechanistic levels. J. Exp. Bot. 66, 2401–2414. doi: 10.1093/jxb/eru505
Espinas, N. A., Kobayashi, K., Takahashi, S., Mochizuki, N., and Masuda, T. (2012). Evaluation of unbound free heme in plant cells by differential acetone extraction. Plant Cell Physiol. 53, 1344–1354. doi: 10.1093/pcp/pcs067
Estavillo, G. M., Crisp, P. A., Pornsiriwong, W., Wirtz, M., Collinge, D., Carrie, C., et al. (2011). Evidence for a SAL1-PAP chloroplast retrograde pathway that functions in drought and high light signaling in Arabidopsis. Plant Cell 23, 3992–4012. doi: 10.1105/tpc.111.091033
Fankhauser, C., and Christie, J. M. (2015). Plant phototropic growth. Curr. Biol. 25, R384–R389. doi: 10.1016/j.cub.2015.03.020
Feng, S., Martinez, C., Gusmaroli, G., Wang, Y., Zhou, J., Wang, F., et al. (2008). Coordinated regulation of Arabidopsis thaliana development by light and gibberellins. Nature 451, 475–479. doi: 10.1038/nature06448.
Gallego-Bartolomé, J., Minguet, E. G., Grau-Enguix, F., Abbas, M., Locascio, A., Thomas, S. G., et al. (2012). Molecular mechanism for the interaction between gibberellin and brassinosteroid signaling pathways in Arabidopsis. Proc. Natl. Acad. Sci. U. S. A. 109, 13446–13451. doi: 10.1073/pnas.1119992109
Gil, M. J., Coego, A., Mauch-Mani, B., Jordá, L., and Vera, P. (2005). The Arabidopsis csb3 mutant reveals a regulatory link between salicylic acid-mediated disease resistance and the methyl-erythritol 4-phosphate pathway. Plant J. 44, 155–166. doi: 10.1111/j.1365-313X.2005.02517.x
Gilroy, S., Suzuki, N., Miller, G., Choi, W. G., Toyota, M., Devireddy, A. R., et al. (2014). A tidal wave of signals: calcium and ROS at the forefront of rapid systemic signaling. Trends Plant Sci. 19, 623–630. doi: 10.1016/j.tplants.2014.06.013
Görlach, A., Bertram, K., Hudecova, S., and Krizanova, O. (2015). Calcium and ROS: a mutual interplay. Redox Biol. 6, 260–271. doi: 10.1016/j.redox.2015.08.010
Gy, I., Gasciolli, V., Lauressergues, D., Morel, J.-B., Gombert, J., Proux, F., et al. (2007). Arabidopsis FIERY1, XRN2, and XRN3 are endogenous RNA silencing suppressors. Plant Cell 19, 3451–3461. doi: 10.1105/tpc.107.055319
Halliday, K. J., and Fankhauser, C. (2003). Phytochrome-hormonal signalling networks. New Phytol. 157, 449–463. doi: 10.1046/j.1469-8137.2003.00689.x
Hashimoto, H., and Possingham, J. V. (1989). Effect of light on the chloroplast division cycle and DNA synthesis in cultured leaf discs of spinach. Plant Physiol. 89, 1178–1183. doi: 10.1104/pp.89.4.1178
Hirsch, J., Misson, J., Crisp, P. A., David, P., Bayle, V., Estavillo, G. M., et al. (2011). A novel fry1 allele reveals the existence of a mutant phenotype unrelated to 5′ to 3′ exoribonuclease (XRN) activities in Arabidopsis thaliana roots. PLoS One 6:e16724. doi: 10.1371/journal.pone.0016724
Hou, X., Lee, L. Y. C., Xia, K., Yan, Y., and Yu, H. (2010). DELLAs modulate jasmonate signaling via competitive binding to JAZs. Dev. Cell 19, 884–894. doi: 10.1016/j.devcel.2010.10.024
Ishiga, Y., Watanabe, M., Ishiga, T., Tohge, T., Matsuura, T., Ikeda, Y., et al. (2017). The SAL-PAP chloroplast retrograde pathway contributes to plant immunity by regulating glucosinolate pathway and phytohormone signaling. Mol. Plant-Microbe Interact. 30, 829–841. doi: 10.1094/MPMI-03-17-0055-R
Jaillais, Y., and Chory, J. (2010). Unraveling the paradoxes of plant hormone signaling integration. Nat. Struct. Mol. Biol. 17, 642–645. doi: 10.1038/nsmb0610-642
Jiang, X., Li, H., Wang, T., Peng, C., Wang, H., Wu, H., et al. (2012). Gibberellin indirectly promotes chloroplast biogenesis as a means to maintain the chloroplast population of expanded cells. Plant J. 72, 768–780. doi: 10.1111/j.1365-313X.2012.05118.x
Jin, X., Zhu, J., and Zeiger, E. (2001). The hypocotyl chloroplast plays a role in phototropic bending of Arabidopsis seedlings: developmental and genetic evidence. J. Exp. Bot. 52, 91–97. doi: 10.1093/jxb/52.354.91
Kanno, Y., Jikumaru, Y., Hanada, A., Nambara, E., Abrams, S. R., Kamiya, Y., et al. (2010). Comprehensive hormone profiling in developing Arabidopsis seeds: examination of the site of ABA biosynthesis, ABA transport and hormone interactions. Plant Cell Physiol. 51, 1988–2001. doi: 10.1093/pcp/pcq158
Kim, B.-H., and von Arnim, A. G. (2009). FIERY1 regulates light-mediated repression of cell elongation and flowering time via its 3′(2′),5′-bisphosphate nucleotidase activity. Plant J. 58, 208–219. doi: 10.1111/j.1365-313X.2008.03770.x
Kopriva, S., and Gigolashvili, T. (2016). Glucosinolate synthesis in the context of plant metabolism. Adv. Botan. Res. 80, 99–124. doi: 10.1016/bs.abr.2016.07.002
Larkin, R. M. (2014). Influence of plastids on light signalling and development. Philos. Trans. R. Soc. London. Ser. B 369:20130232. doi: 10.1098/rstb.2013.0232
Lau, O. S., and Deng, X. W. (2010). Plant hormone signaling lightens up: integrators of light and hormones. Curr. Opin. Plant Biol. 13, 571–577. doi: 10.1016/j.pbi.2010.07.001
Lee, B.-R., Huseby, S., Koprivova, A., Chételat, A., Wirtz, M., Mugford, S. T., et al. (2012). Effects of fou8/fry1 mutation on sulfur metabolism: is decreased internal sulfate the trigger of sulfate starvation response? PLoS One 7:e39425. doi: 10.1371/journal.pone.0039425
Lee, H., Xiong, L., Ishitani, M., Stevenson, B., and Zhu, J. K. (1999). Cold-regulated gene expression and freezing tolerance in an Arabidopsis thaliana mutant. Plant J. 17, 301–308. doi: 10.1046/j.1365-313X.1999.00375.x
Leymarie, J., Vitkauskaité, G., Hoang, H. H., Gendreau, E., Chazoule, V., Meimoun, P., et al. (2012). Role of reactive oxygen species in the regulation of Arabidopsis seed dormancy. Plant Cell Physiol. 53, 96–106. doi: 10.1093/pcp/pcr129
Li, Q.-F., Wang, C., Jiang, L., Li, S., Sun, S. S. M., and He, J.-X. (2012). An interaction between BZR1 and DELLAs mediates direct signaling crosstalk between brassinosteroids and gibberellins in Arabidopsis. Sci. Signal. 5:ra72. doi: 10.1126/scisignal.2002908
Litthauer, S., Chan, K. X., and Jones, M. A. (2018). 3′-phosphoadenosine 5′-phosphate accumulation delays the circadian system. Plant Physiol. 176, 3120–3135. doi: 10.1104/pp.17.01611
Mazars, C., Thuleau, P., Lamotte, O., and Bourque, S. (2010). Cross-talk between ROS and calcium in regulation of nuclear activities. Mol. Plant 3, 706–718. doi: 10.1093/mp/ssq024
Millar, A. J. (2004). Input signals to the plant circadian clock. J. Exp. Bot. 55, 277–283. doi: 10.1093/jxb/erh034
Okello, R. C. O., de Visser, P. H. B., Heuvelink, E., Marcelis, L. F. M., and Struik, P. C. (2016). Light mediated regulation of cell division, endoreduplication and cell expansion. Environ. Exp. Bot. 121, 39–47. doi: 10.1016/j.envexpbot.2015.04.003
Phua, S. Y., Pornsiriwong, W., Chan, K. X., Estavillo, G. M., and Pogson, B. J. (2018). Development of strategies for genetic manipulation and fine-tuning of a chloroplast retrograde signal 3′-phosphoadenosine 5′-phosphate. Plant Direct 2, 1–15. doi: 10.1002/pld3.31
Piskurewicz, U., Jikumaru, Y., Kinoshita, N., Nambara, E., Kamiya, Y., and Lopez-Molina, L. (2008). The gibberellic acid signaling repressor RGL2 inhibits Arabidopsis seed germination by stimulating abscisic acid synthesis and ABI5 activity. Plant Cell 20, 2729–2745. doi: 10.1105/tpc.108.061515
Pogson, B. J., Ganguly, D., and Albrecht-Borth, V. (2015). Insights into chloroplast biogenesis and development. Biochim. Biophys. Acta – Bioenerg. 1847, 1017–1024. doi: 10.1016/j.bbabio.2015.02.003
Pornsiriwong, W., Estavillo, G. M., Chan, K. X., Tee, E. E., Ganguly, D., Crisp, P. A., et al. (2017). A chloroplast retrograde signal, 3′-phosphoadenosine 5′-phosphate, acts as a secondary messenger in abscisic acid signaling in stomatal closure and germination. Elife 6, 1–34. doi: 10.7554/eLife.23361
Quintero, F., Garciadeblás, B., and Rodríguez-Navarro, A. (1996). The SAL1 gene of Arabidopsis, encoding an enzyme with 3′ (2′), 5′-bisphosphate nucleotidase and inositol polyphosphate 1-phosphatase activities, increases salt tolerance in yeast. Plant Cell 8, 529–537. doi: 10.2307/3870330
Ribeiro, D. M., Araújo, W. L., Fernie, A. R., Schippers, J. H. M., and Mueller-Roeber, B. (2012). Translatome and metabolome effects triggered by gibberellins during rosette growth in Arabidopsis. J. Exp. Bot. 63, 2769–2786. doi: 10.1093/jxb/err463
Robles, P., Fleury, D., Candela, H., Cnops, G., Alonso-Peral, M. M., Anami, S., et al. (2010). The RON1/FRY1/SAL1 gene is required for leaf morphogenesis and venation patterning in Arabidopsis. Plant Physiol. 152, 1357–72. doi: 10.1104/pp.109.149369
Rodríguez, V. M., Chételat, A., Majcherczyk, P., and Farmer, E. E. (2010). Chloroplastic phosphoadenosine phosphosulfate metabolism regulates basal levels of the prohormone jasmonic acid in Arabidopsis leaves. Plant Physiol. 152, 1335–45. doi: 10.1104/pp.109.150474
Rossel, J. B., Walter, P. B., Hendrickson, L., Chow, W. S., Poole, A., Mullineaux, P. M., et al. (2006). A mutation affecting ASCORBATE PEROXIDASE 2 gene expression reveals a link between responses to high light and drought tolerance. Plant Cell Environ. 29, 269–281. doi: 10.1111/j.1365-3040.2005.01419.x
Vogel, M. O., Moore, M., König, K., Pecher, P., Alsharafa, K., Lee, J., et al. (2014). Fast retrograde signaling in response to high light involves metabolite export, MITOGEN-ACTIVATED PROTEIN KINASE6, and AP2/ERF transcription factors in Arabidopsis. Plant Cell 26, 1151–1165. doi: 10.1105/tpc.113.121061
Wawer, I., Golisz, A., Sulkowska, A., Kawa, D., Kulik, A., and Kufel, J. (2018). mRNA decapping and 5′-3′ decay contribute to the regulation of ABA signaling in Arabidopsis thaliana. Front. Plant Sci. 9:312. doi: 10.3389/fpls.2018.00312
Webb, A. A. R., and Satake, A. (2015). Understanding circadian regulation of carbohydrate metabolism in arabidopsis using mathematical models. Plant Cell Physiol. 56, 586–593. doi: 10.1093/pcp/pcv033
Wild, M., Davière, J.-M., Cheminant, S., Regnault, T., Baumberger, N., Heintz, D., et al. (2012). The Arabidopsis DELLA RGA-LIKE3 is a direct target of MYC2 and modulates jasmonate signaling responses. Plant Cell 24, 3307–3319. doi: 10.1105/tpc.112.101428
Wilson, P. B., Estavillo, G. M., Field, K. J., Pornsiriwong, W., Carroll, A. J., Howell, K. A., et al. (2009). The nucleotidase/phosphatase SAL1 is a negative regulator of drought tolerance in Arabidopsis. Plant J. 58, 299–317. doi: 10.1111/j.1365-313X.2008.03780.x
Woodson, J. D., Perez-Ruiz, J. M., and Chory, J. (2011). Heme synthesis by plastid ferrochelatase I regulates nuclear gene expression in plants. Curr. Biol. 21, 897–903. doi: 10.1016/j.cub.2011.04.004
Xiao, Y., Savchenko, T., Baidoo, E. E. K., Chehab, W. E., Hayden, D. M., Tolstikov, V., et al. (2012). Retrograde signaling by the plastidial metabolite MEcPP regulates expression of nuclear stress-response genes. Cell 149, 1525–1535. doi: 10.1016/j.cell.2012.04.038
Xiong, L., Lee BH., Ishitani, M., Lee, H., Zhang, C., and Zhu, J. K. (2001). FIERY1 encoding an inositol polyphosphate 1-phosphatase is a negative regulator of abscisic acid and stress signaling in Arabidopsis. Genes Dev. 15, 1971–1984. doi: 10.1101/gad.891901
Xiong, L., Lee, H., Huang, R., and Zhu, J.-K. (2004). A single amino acid substitution in the Arabidopsis FIERY1/HOS2 protein confers cold signaling specificity and lithium tolerance. Plant J. 40, 536–545. doi: 10.1111/j.1365-313X.2004.02225.x
Yoshizawa, E., Kaizuka, M., Yamagami, A., Higuchi-Takeuchi, M., Matsui, M., Kakei, Y., et al. (2014). BPG3 is a novel chloroplast protein that involves the greening of leaves and related to brassinosteroid signaling. Biosci. Biotechnol. Biochem. 78, 420–429. doi: 10.1080/09168451.2014.885831
Keywords: phosphoadenosines, chloroplast retrograde, light, hormones, signaling, plant growth
Citation: Phua SY, Yan D, Chan KX, Estavillo GM, Nambara E and Pogson BJ (2018) The Arabidopsis SAL1-PAP Pathway: A Case Study for Integrating Chloroplast Retrograde, Light and Hormonal Signaling in Modulating Plant Growth and Development? Front. Plant Sci. 9:1171. doi: 10.3389/fpls.2018.01171
Received: 15 May 2018; Accepted: 23 July 2018;
Published: 08 August 2018.
Edited by:
Julian Eaton-Rye, University of Otago, New ZealandReviewed by:
Saijaliisa Kangasjärvi, University of Turku, FinlandCopyright © 2018 Phua, Yan, Chan, Estavillo, Nambara and Pogson. This is an open-access article distributed under the terms of the Creative Commons Attribution License (CC BY). The use, distribution or reproduction in other forums is permitted, provided the original author(s) and the copyright owner(s) are credited and that the original publication in this journal is cited, in accordance with accepted academic practice. No use, distribution or reproduction is permitted which does not comply with these terms.
*Correspondence: Barry J. Pogson, QmFycnkuUG9nc29uQGFudS5lZHUuYXU=
Disclaimer: All claims expressed in this article are solely those of the authors and do not necessarily represent those of their affiliated organizations, or those of the publisher, the editors and the reviewers. Any product that may be evaluated in this article or claim that may be made by its manufacturer is not guaranteed or endorsed by the publisher.
Research integrity at Frontiers
Learn more about the work of our research integrity team to safeguard the quality of each article we publish.