- Molecular Plant Biology and Biotechnology Lab, Research Centre, CSIR-Central Institute of Medicinal and Aromatic Plants, Bengaluru, India
Catharanthus roseus is the sole source of two of the most important anticancer monoterpene indole alkaloids (MIAs), vinblastine and vincristine and their precursors, vindoline and catharanthine. The MIAs are produced from the condensation of precursors derived from indole and terpene secoiridoid pathways. It has been previously reported that the terpene moiety limits MIA biosynthesis in C. roseus. Here, to overcome this limitation and enhance MIAs levels in C. roseus, bifunctional geranyl(geranyl) diphosphate synthase [G(G)PPS] and geraniol synthase (GES) that provide precursors for early steps of terpene moiety (secologanin) formation, were overexpressed transiently by agroinfiltration and stably by Agrobacterium-mediated transformation. Both transient and stable overexpression of G(G)PPS and co-expression of G(G)PPS+GES significantly enhanced the accumulation of secologanin, which in turn elevated the levels of monomeric MIAs. In addition, transgenic C. roseus plants exhibited increased levels of root alkaloid ajmalicine. The dimeric alkaloid vinblastine was enhanced only in G(G)PPS but not in G(G)PPS+GES transgenic lines that correlated with transcript levels of peroxidase-1 (PRX1) involved in coupling of vindoline and catharanthine into 3′,4′-anhydrovinblastine, the immediate precursor of vinblastine. Moreover, first generation (T1) lines exhibited comparable transcript and metabolite levels to that of T0 lines. In addition, transgenic lines displayed normal growth similar to wild-type plants indicating that the bifunctional G(G)PPS enhanced flux toward both primary and secondary metabolism. These results revealed that improved availability of early precursors for terpene moiety biosynthesis enhanced production of MIAs in C. roseus at the whole plant level. This is the first report demonstrating enhanced accumulation of monomeric and dimeric MIAs including root MIA ajmalicine in C. roseus through transgenic approaches.
Introduction
Catharanthus roseus (Madagascar Periwinkle) is the best-characterized monoterpene indole alkaloids (MIAs)-producing plant species. To date, C. roseus remains the only natural source of two medicinally valuable dimeric MIAs vinblastine and vincristine, and their monomeric precursors vindoline and catharanthine (Miettinen et al., 2014; Kellner et al., 2015). While leaf specific dimeric alkaloids vinblastine and vincristine are used either directly or as derivatives in cancer chemotherapy, roots accumulate monomeric alkaloids ajmalicine and serpentine which are used as anti-hypertensive agents (Zhao et al., 2013). Coupling of the monomeric precursors vindoline and catharanthine to α-3′,4′-anhydrovinblastine and its subsequent conversion results in the formation of dimeric vinblastine and vincristine (Costa et al., 2008). Extremely low in planta accumulation of dimeric alkaloids makes them highly expensive. Approximately 500 kg of dry leaves is required to isolate 1 g of vinblastine for pharmaceutical production (Noble, 1990). Moreover, total chemical synthesis of dimeric alkaloids is economically not viable due to their complex structures (Kuboyama et al., 2004; van Der Heijden et al., 2004; Ishikawa et al., 2008). Furthermore, improving the production of monomeric vindoline and dimeric alkaloids in cell suspension and hairy root cultures is challenging as they lack the required level of cellular and tissue differentiation essential for the expression of entire MIAs pathway genes, especially the genes involved in vindoline biosynthesis (Zarate and Verpoorte, 2007; Guirimand et al., 2009). Such a scenario calls for genetic transformation of C. roseus for improvement of MIAs production at the whole plant level.
To date, genetic transformation in C. roseus has been mostly confined to hairy roots and cell cultures (Choi et al., 2004; Peebles et al., 2006; Jaggi et al., 2011; Sun et al., 2017). Moreover, transgenic C. roseus cell suspension cultures do not produce alkaloids in a stable manner and their ability to accumulate MIAs declines by prolonged subculture (Whitmer et al., 2003). Not many efforts have been made with respect to metabolic engineering of C. roseus plants, as they are hard-to-transform owing to their highly recalcitrant nature for genetic transformation. Lately, some reports have demonstrated the generation of transgenic C. roseus through Agrobacterium tumefaciens-mediated transformation (Verma and Mathur, 2011b; Pan et al., 2012; Wang Q. et al., 2012). Direct shoot bud organogenesis and transformation were achieved using pre-plasmolyzed leaf explants and β-glucuronidase (GUS) expression was confirmed in transgenic plants (Verma and Mathur, 2011b). However, there are only few reports pertaining to development of transgenic C. roseus overexpressing MIA pathway genes or regulators (Pan et al., 2012; Wang Q. et al., 2012). It was shown that transgenic overexpression of deacetylvindoline-4-O-acetyltransferase (DAT) in C. roseus plants resulted in improved accumulation of vindoline (Wang Q. et al., 2012). Another study from the same group reported enhanced production of ajmalicine, catharanthine and vindoline in transgenic C. roseus overexpressing geraniol 10-hydroxylase (G10H) and the transcriptional regulator Octadecanoid-derivative Responsive Catharanthus AP2-domain (ORCA3) (Pan et al., 2012; Wang Q. et al., 2012).
Monoterpene indole alkaloids are derived from the central intermediate strictosidine, which is formed by the condensation of indole pathway derived tryptamine and monoterpene iridoid precursor secologanin in a reaction catalyzed by the enzyme strictosidine synthase (STR) (Figure 1). While the indole moiety tryptamine is formed from tryptophan, the iridoid biosynthesis starts from the monoterpene geraniol. Geraniol is formed from geranyl diphosphate (GPP) by the action of a terpene synthase, geraniol synthase (GES). GPP, the universal precursor of monoterpenes, is produced by the condensation of isopentenyl diphosphate (IPP) and dimethylallyl diphosphate (DMAPP) by GPP synthase (GPPS) (Dudareva et al., 2006; Nagegowda, 2010). Geraniol is further oxidized by geraniol-10-hydroxylase/8-oxidase (G8O) to 8-hydroxygeraniol, which is then converted to loganin and finally to secologanin by multiple enzymatic steps (Miettinen et al., 2014). In plants, GPPS functions at the branch point of general and specialized metabolism and regulates flux to monoterpene biosynthesis (Orlova et al., 2009; Nagegowda, 2010). In tobacco, overexpression of snapdragon GPPS small subunit (GPPS.SSU) resulted in an increased monoterpene emission in leaves. However, plants were dwarfed and exhibited strong leaf chlorotic symptoms together with increased light sensitivity due to reduction in the level of primary metabolites (Orlova et al., 2009). In another study, co-expression of peppermint GPS.SSU with four different monoterpene synthases boosted monoterpene production in transgenic tobacco without negatively affecting plant growth (Yin et al., 2017). These studies indicate that, overexpression of either GPPS alone or co-expression of GPPS with a monoterpene synthase could enhance the production of monoterpenes.
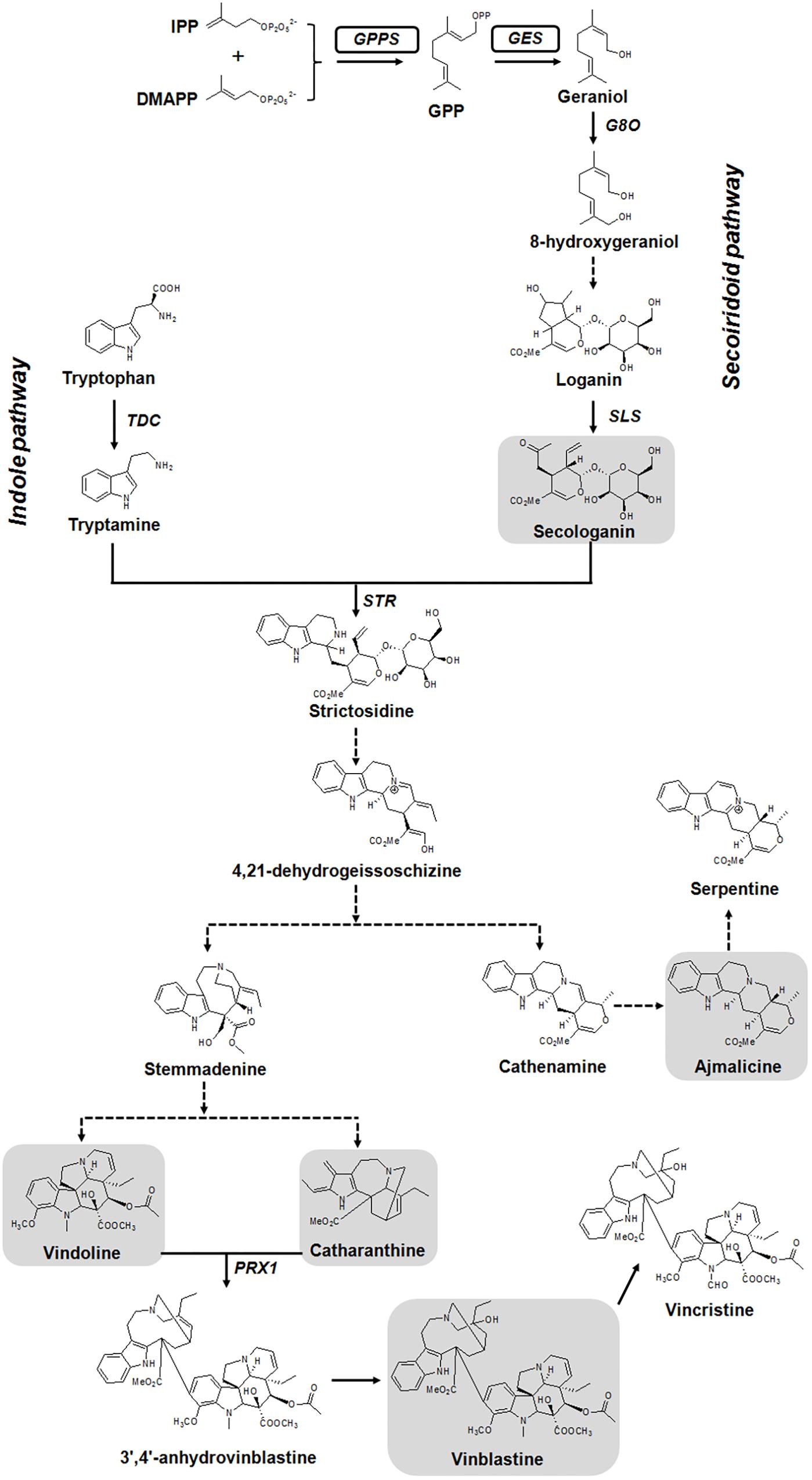
FIGURE 1. Simplified version of monoterpene indole alkaloids (MIAs) biosynthetic pathway in C. roseus. Full and dashed arrows indicate single and multiple enzymatic steps, respectively. The genes used to generate transgenic plants, geranyl(geranyl) diphosphate synthase [G(G)PPS] and geraniol synthase (GES), are boxed. Structures of analyzed metabolites in this study are shown in gray highlighted boxes. DMAPP, dimethylallyl diphosphate; G8O, geraniol-10-hydroxylase/8-oxidase; GPP, geranyl diphosphate; IPP, isopentenyl diphosphate; PRX1, peroxidase 1; SLS, secologanin synthase; STR, strictosidine synthase; TDC, tryptophan decarboxylase.
In C. roseus, the heteromeric GPPS consisting of an enzymatic large subunit (LSU) and an inactive small subunit (SSU) is involved in providing GPP required for MIA biosynthesis. The LSU is a bifunctional G(G)PP synthase [G(G)PPS] that produces both GPP and GGPP in a ∼2:1 ratio (Rai et al., 2013). Further, GPP is utilized by the monoterpene synthase GES, which is transcriptionally regulated and its transient overexpression in leaves enhanced monomeric alkaloids in C. roseus (Kumar et al., 2015). It has been reported in cell cultures that the terpene moiety is limiting for the biosynthesis of MIAs (Peebles et al., 2006). Moreover, we have previously shown by feeding studies that geraniol and not tryptophan is limiting for the biosynthesis of vindoline and catharanthine in C. roseus leaves (Kumar et al., 2015). Thus, enhanced availability of early secoiridoid pathway precursors (GPP and geraniol) could push the flux toward secologanin, which in turn could enhance the accumulation of end products (monomeric/dimeric alkaloids). In this work, we overexpressed a bifunctional G(G)PPS, and co-expressed G(G)PPS and GES in transgenic C. roseus plants that were generated through Agrobacterium-mediated transformation. Transgenic plants accumulated enhanced secologanin, vindoline, catharanthine, ajmalicine and vinblastine without any negative effects on plant growth, thus demonstrating the importance of metabolic engineering at the whole plant level for improvement of monomeric and dimeric MIAs production in C. roseus.
Materials And Methods
Generation of Plant Overexpression Vectors
For generation of G(G)PPS plant overexpression construct, the open reading frame corresponding to 1,152 bp was PCR-amplified from leaf cDNA of C. roseus (cv. Dhawal) using full length gene-specific primers (Supplementary Table S1) containing XbaI and SacI sites. The amplified coding regions were cloned into the pJET1.2 vector and nucleotide sequences were confirmed by Sanger sequencing. Resulting pJET constructs were restriction digested and G(G)PPS was sub-cloned into XbaI and SacI sites of pBI121 binary vector under the control of 35S promoter of Cauliflower mosaic virus (CaMV) to form pBI121::G(G)PPS construct (Supplementary Figure S1). Agrobacterium tumefaciens strain GV3101 was transformed with pBI121 empty vector and pBI121::G(G)PPS construct by freeze-thaw method. For GES overexpression, the plant overexpression construct pBI121::GES reported in Kumar et al. (2015) was used.
Transient Overexpression in C. roseus
Transient overexpression was performed following the method described in Kumar et al. (2015). Briefly, overnight grown Agrobacteria cultures were pelleted and resuspended in infiltration buffer (50 mM MES pH 5.6, 2 mM Na3PO4, 0.5% glucose and 100 μM acetosyringone) to a final OD600 of 0.2. The Agrobacteria suspension was incubated at 28°C for 4 h. Infiltration was performed into the first pair of leaves of 3-week-old C. roseus plants using a needle-less syringe. Post-infiltration, plants were covered and maintained in the dark for 48 h. Infiltrated leaves were harvested and used for subsequent gene expression and metabolite analyses.
Generation of Transgenic C. roseus Plants
C. roseus (cv. Dhawal) seeds were surface sterilized using 4% sodium hypochlorite and inoculated in half strength MS (Murashige and Skoog, 1962) medium. In vitro grown hypocotyl and nodal explants were used for generation of G(G)PPS and G(G)PPS+GES transgenic lines, respectively. While G(G)PPS transformants were generated by indirect regeneration using hypocotyl explants according to Wang Q. et al. (2012), plants co-expressing G(G)PPS+GES were generated by direct regeneration using nodal explants following the method reported in Verma and Mathur (2011a).
For generating transgenic plants through indirect regeneration using hypocotyls explants, MS basal medium was supplemented with 250 mg/L proline and 150 mg/L casein hydrolysate (MSCP) with appropriate hormones as follows. Hypocotyl explants were precultured in MSCP1 medium [MSCP with 1.0 mg/L 2,4-dichlorophenoxyacetic acid (2,4-D), 1.0 mg/L α-naphthalene acetic acid (NAA) and 0.1 mg/L zeatin] for 3 days. After co-cultivation with Agrobacteria cultures, explants were transferred to MSCP1 selection medium for a week and subsequently transferred to MSCP2 medium [MSCP with 5.0 mg/L 6-benzyl adenine (BA), 0.5 mg/L NAA] for callus induction. Finally, the initiated shoots were transferred to MSCP3 shoot regeneration medium [MSCP with 1.75 mg/L BA, 0.55 mg/L indole-3-acetic acid (IAA)].
For direct regeneration, nodal explants (1–2 cm) were excised from 1-month-old in vitro grown C. roseus plants. Subsequently, nodal explants were co-cultivated with 1:1 mixture of Agrobacteria harboring pBI121::G(G)PPS and pBI121::GES (OD600 0.4–0.6) for 10 min. The explants were then blot-dried and inoculated into MS-BNT medium containing 1 mg/L BA, 0.1 mg/L NAA and 400 mg/L thiamine HCl. After 3 days of co-cultivation in dark, explants were transferred to fresh MS-BNT medium containing kanamycin and carbenicillin. For shoot proliferation, explants were continued to be cultured in the same medium by subculturing every 10 days. After five rounds of subculturing, well developed shoots were transferred to half strength MS medium for rooting. In both cases, our own modifications were made for rooting as outlined below. After obtaining putative transgenic shoots, they were excised and inoculated initially in rooting media consisting of half strength MS with 2 mg/L indole-3-butyric acid (IBA) and 300 mg/L carbenicillin until root initiation. Later, these plants were transferred to half strength MS with 2.4 μM IBA and 300 mg/L carbenicillin. Plants with well developed roots were transplanted to pots containing 1:1 sterile soilrite:vermicompost mix, and transferred to glass house after acclimation.
PCR Confirmation of Transgenic Lines and Quantitative Reverse Transcriptase-PCR (qRT-PCR) Analysis
Genomic DNA was isolated from wild type (WT) controls and putative transgenic lines following the protocol reported by Doyle and Doyle (1990). Briefly, leaf disks were ground with preheated extraction buffer (1.4 M NaCl, 20 mM EDTA, 100 mM Tris HCl, 0.2% β-Mercaptoethanol, 2% PVP, and 2% CTAB) and incubated at 65°C for 1 h with intermittent shaking. An equal volume of 24:1 chloroform:isoamyl alcohol was added to separate the aqueous phase. The DNA was precipitated by adding 0.6 volume of ice-cold isopropanol to the aqueous phase and incubated overnight at −20°C. Precipitated genomic DNA was then washed with 70% ethanol and finally resuspended in milli Q water. Transgenic lines were confirmed by PCR screening using appropriate primers. In the case of G(G)PPS lines, 35S promoter-specific forward and G(G)PPS-specific reverse primers were used. For screening G(G)PPS+GES lines, 35S/nptII promoter-specific forward and 35S/G(G)PPS/GES/nptII specific reverse primers were used (Supplementary Table S1). RNA isolation, cDNA synthesis and qRT-PCR were performed in transgenic lines following the procedure reported in Rai et al. (2013) and Kumar et al. (2015). Total RNA was extracted from 100 mg leaf tissue using the SpectrumTM Plant Total RNA kit (Sigma-Aldrich, United States) following manufacturer’s instructions. To remove contaminating genomic DNA, on-column DNase digestion was performed with DNase I (Sigma-Aldrich, St. Louis, MO, United States). Total RNA (2 μg) was used for first strand cDNA synthesis with random hexamer primers using High Capacity cDNA Reverse Transcription kit (Applied Biosystems, United States) as per manufacturer’s instructions. qRT-PCR was performed with a linear range of cDNA using StepOne Real-Time PCR System (Applied Biosystems, United States) and expression of transcripts were normalized using N227 that was previously reported as an appropriate endogenous gene in C. roseus (Pollier et al., 2014). qRT-PCR reaction mixture of 5 μl contained 2.5 μl of 2X Maxima SYBR Green/ROX qPCR master mix (Thermo Scientific, United States), 1:3 diluted cDNA and 2 μM gene-specific primers (Supplementary Table S1). The following conditions were used for qRT-PCR: 94°C for 10 min for first cycle, followed by 94°C for 15 s and 60°C for 15 s for 40 cycles. Fold change differences in gene expression were analyzed by the comparative cycle threshold (Ct) method. Relative quantification was carried out by calculating Ct to determine fold difference in gene expression [ΔCt target – ΔCt calibrator]. The relative level was determined as 2−ΔΔCT. All experiments were repeated using three biological replicates with three technical replicates and data were analyzed statistically as mentioned below.
Alkaloid Extraction, Analysis, and Quantification
Secologanin, vindoline, catharanthine, ajmalicine, and vinblastine were quantified using High Performance Liquid Chromatography (HPLC) having Photodiode Array (PDA) detector. HPLC (Model: SCL-10AVP, Shimadzu, Japan) equipped with C18 symmetry reverse phase column (5 μm, 250 mm × 4.6 mm, Waters, Milford, MA, United States) was used for all analyses. Secologanin was extracted and quantified according to Tikhomiroff and Jolicoeur (2002) with minor modifications. Fresh leaves (50 mg) from 2-month-old plants were extracted in 1.5 ml methanol for 60 min in a sonicating bath. The extract was centrifuged and the supernatant was decolorized by adding activated charcoal. Subsequently, the supernatant was transferred to a fresh tube and evaporated to dryness. The dried residue was dissolved in 20 μl methanol and used for HPLC analysis. Mobile phase for HPLC consisted of 15:85 (v/v) mixture of gradient grade acetonitrile:phosphoric acid (0.1 M, pH 2.0) with a flow-rate of 1.5 ml/min. HPLC was performed in isocratic mode for 20 min.
Vindoline and catharanthine were extracted following protocol of Lourdes Miranda-Ham et al. (2007). Briefly, oven dried leaf tissue (10 mg) collected from 2-month-old plants were grounded in 2 ml methanol and incubated at 55°C for 2 h with occasional shaking. The obtained extract was filtered and evaporated to dryness. The residue was dissolved in 700 μl of 2.5% H2SO4 and extracted twice with equal volumes of ethyl acetate. Aqueous phase was retained each time and subsequently, pH of the aqueous phase was adjusted to 9.0 using NH4OH. Finally, alkaloids were extracted with equal volume of ethyl acetate and the resulting organic phase was evaporated to dryness. The residue containing alkaloids was dissolved in 20 μl methanol and used in HPLC analysis. Mobile phase for HPLC consisted of a mixture of A (0.1 M ammonium acetate buffer, pH 7.3) and B (gradient grade acetonitrile) with 1 ml/min flow rate of 70:30 ratio of A:B for first 5 min of run and then was linearly ramped to 36:64 for next 5 min with flow rate of 1.4 ml/min. Subsequently, the ratio was changed to 20:80 with 1.4 ml/min for next 5 min and finally the flow rate was reduced to 1 ml/min with 70:30 ratios in the last 5 min.
Ajmalicine and vinblastine were extracted and quantified following the method described in Pan et al. (2016). Briefly, oven dried young leaves (50 mg) collected from 2-month-old plants for vinblastine and roots (200 mg) from 1-year-old plants maintained in glass house for ajmalicine were extracted with 1 ml methanol by sonication (30 min). Samples were centrifuged at 12,000 rpm for 10 min at room temperature and the supernatant was decolorized (for leaf samples) by adding activated charcoal. The resulting methanolic extract was evaporated to dryness, resuspended in 20 μl methanol and used for HPLC quantification. The mobile phase consisted of a mixture of 5 mM Na2HPO4 (pH 6.0) (solvent A) and gradient grade methanol (solvent B) at a flow rate of 1.5 ml per min. The change in mobile phase was set to a linear gradient from 86:14 to 14:86 at 0–26 min in isocratic mode (14:86 v/v at 26–30 min), a linear gradient from 14:86 to 86:14 at 30–35 min, an isocratic elution with 14:86 (v/v) at 35–40 min. Data was extracted at 238 nm for secologanin, 254 nm for vindoline, catharanthine, ajmalicine, and vinblastine. Peak area obtained from authentic standards (Sigma-Aldrich, St. Louis, MO, United States) (Supplementary Figure S2) and samples were used to quantify alkaloids and expressed as relative content in %.
Chlorophyll and Carotenoids Quantification, and Phenotypic Analyses
The amount of total chlorophyll and carotenoids was quantified according to Lichtenthaler (1987). Briefly, 20 mg of leaf (third developmental stage) was extracted in 98% ethanol for 2 h. The supernatant was used for quantification of chlorophyll and carotenoids. The absorbance was measured at 470, 653, and 666 nm and the amount of chlorophyll a, chlorophyll b, and total carotenoids was calculated. Flowers of T0 transgenic lines were selfed individually and siliques were collected separately from individual transgenic lines. The seeds were germinated and screened by PCR to select the first generation T1 positive plants from each transgenic line. Phenotypic parameters such as number of leaves, branches, flowers and siliques formed in WT and selected T1 transgenic lines were analyzed.
Copy Number Determination in Transgenic C. roseus Lines
Copy number of G(G)PPS and GES in transgenic G(G)PPS and G(G)PPS+GES lines were confirmed by using qPCR as described previously (Weng et al., 2004; Yi et al., 2008; Li et al., 2017). For absolute quantification, standard curve was generated using twofold dilutions of genomic DNA extracted from WT controls, selected G(G)PPS and all three lines of G(G)PPS+GES using primers of G(G)PPS, GES, and tryptophan decarboxylase [TDC, the endogenous single copy gene as determined by Goddijn et al. (1994)]. Three separate sets of reactions were performed to amplify G(G)PPS, GES, and TDC. Each reaction (5 μl) contained 2.5 μl of 2X Maxima SYBR Green PCR master mix (Thermo Scientific, United States), 100 ng genomic DNA and 2 μM gene-specific primers. Reaction was performed as follows: 94°C for 10 min for first cycle, followed by 94°C for 15 s and 60°C for 15 s for 40 cycles. The PCR efficiency of target genes and TDC were calculated from slope of standard curve for each gene. The number of copies of G(G)PPS and GES were determined according to Pfaffl (2001).
Statistical Analysis
Average mean, standard error (SE) and number of replicates (n) used for individual experiment were employed for statistical analysis using the GraphPad QUICKCALC online software1. Statistical significance of differences between control and samples were tested according to the unpaired Student’s t-test.
Results
Transient Overexpression of G(G)PPS and G(G)PPS+GES Resulted in Increased Alkaloid Accumulation
Transient overexpression by agroinfiltration either in the host plant or in heterologous systems has been widely used for determining the gene function. Moreover, it is an efficient strategy for functional analyses of genes in those plants where the study of gene function is limited owing to very low transformation efficiency and recalcitrant nature of the plant for genetic transformation. Hence, before proceeding for genetic transformation of C. roseus with G(G)PPS and G(G)PPS+GES, we carried out transient overexpression of these genes to determine their effect on MIA biosynthesis. As G(G)PPS and GES were previously reported to positively influence MIA biosynthesis in C. roseus (Rai et al., 2013; Kumar et al., 2015), we hypothesized that improved availability of precursors (GPP and geraniol) by overexpression of G(G)PPS and co-expression of G(G)PPS+GES could enhance MIA accumulation in C. roseus leaves. As the MIA content varies with leaf developmental stages, both genes were transiently overexpressed in first pair of leaves (that exhibit highest alkaloid accumulation). While, overexpression of G(G)PPS resulted in >30-fold transcript increase, co-expression of G(G)PPS+GES lead to ∼14- and 8-fold increase in transcripts of G(G)PPS and GES, respectively (Figures 2A,B). Next, to determine the effect of G(G)PPS and G(G)PPS+GES overexpression on alkaloid accumulation, first we checked the level of terpenoid intermediate secologanin. HPLC analysis showed ∼2.8- and 2.2-fold increase in secologanin levels in G(G)PPS and G(G)PPS+GES infiltrated leaves, respectively, compared to empty vector pBI121 controls (Figures 2C,D and Supplementary Figures S3A,B). Subsequent analysis of the monomeric alkaloids in G(G)PPS infiltrated leaves revealed ∼3- and ∼6-fold increase in vindoline and catharanthine, respectively (Figure 2C and Supplementary Figure S3A). Similarly, G(G)PPS+GES co-infiltrated leaves exhibited an increase of ∼2.5 and ∼3.2-fold vindoline and catharanthine (Figure 2D and Supplementary Figure S3B). Higher accumulation of secologanin, vindoline and catharanthine could be attributed to dramatic increase in the availability of accumulated transcripts as a result of transient overexpression. Taken together, our results clearly demonstrated that overexpression of G(G)PPS and G(G)PPS+GES can improve the metabolic flux toward MIA biosynthesis in C. roseus.
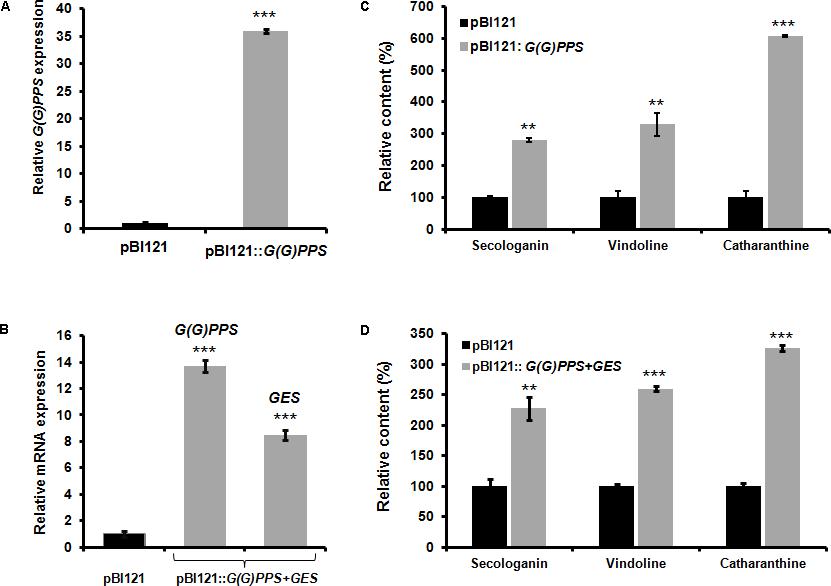
FIGURE 2. Effect of transient overexpression of G(G)PPS and GES in C. roseus leaves. mRNA expression (A,B) and metabolite (C,D) analyses in C. roseus leaves infiltrated with A. tumefaciens carrying pBI121 empty vector (black bar), pBI121::G(G)PPS and co-infiltrated with pBI121::G(G)PPS+pBI121::GES (gray bar) constructs. Transcripts were analyzed by qRT-PCR with CrN227 as an endogenous reference gene. Expression levels were normalized to CrN227 and are represented as expression relative to the pBI121 controls that was set to 1. Relative amounts of secologanin, vindoline, and catharanthine in C. roseus leaves were quantified by HPLC analysis. Secologanin was extracted from 50 mg (fresh weight) of leaves and quantified following Tikhomiroff and Jolicoeur (2002). The monomeric alkaloids vindoline and catharanthine were extracted using 10 mg of oven dried leaves according to Lourdes Miranda-Ham et al. (2007), and quantified following Kumar et al. (2015). In all cases, first pair of infiltrated leaves were used for alkaloid extraction and quantified by HPLC. The levels of quantified metabolites are expressed in % relative to pBI121 vector infiltrated leaves. The bars represent mean ± standard error (SE) of three independent experiments. Significant differences at P < 0.01 and P < 0.001 are represented by “∗∗” and “∗∗∗”, respectively.
Generation of Transgenic C. roseus Plants Overexpressing G(G)PPS and G(G)PPS+GES
In order to substantiate our findings of transient overexpression studies and to demonstrate the in planta role of G(G)PPS and G(G)PPS+GES in improving MIA production, we generated transgenic C. roseus overexpressing G(G)PPS and G(G)PPS+GES. So far, very few efforts have been made with respect to metabolic engineering of C. roseus plant due to its highly recalcitrant nature for genetic transformation together with low transformation efficiency. Lately, some reports have shown the generation of transgenic C. roseus through A. tumefaciens-mediated transformation. For the generation of transgenic C. roseus plants, culture conditions were optimized for callus induction and indirect regeneration following Wang Q. et al. (2012). In vitro grown hypocotyls were used as explants for Agrobacterium-mediated genetic transformation. Hypocotyl explants were precultured in MSCP1 (Figure 3Ai) for 3 days prior to Agrobacterium infection as it has been reported to improve the rate of transformation in C. roseus (Alam et al., 2017). After co-cultivation with Agrobacterium-harboring overexpression constructs, callus initiation was observed in most of the explants in a week in MSCP1 supplemented with 40 mg/L kanamycin and 300 mg/L carbenicillin. The cream colored friable calli developed after about 3 weeks were sub-cultured on to MSCP2 selection medium (Figure 3Aii). However, the calli upon subculturing in MSCP2 medium turned brown in most of the cases and died in about 1–2 weeks. After 2 weeks of sub-culturing in MSCP2 selection medium, only 2–3% of survived calli turned into green friable calli and shoot initiation was observed (Figure 3Aiii). Further, the initiated shoots were transferred to MSCP3 regeneration medium containing appropriate antibiotics. Although the regeneration efficiency was very less, multiple shoots were induced from embryogenic calli in some of the transformation events (Figure 3Aiv). From each calli, 3–4 shoots were induced and after 1 month of shoot maturation, healthy shoots were transferred initially to half strength MS medium supplemented with 2 mg/L IBA. After 2 weeks, though shoots exhibited root initiation, leaves turned yellow and exhibited necrotic symptoms in most of the cases. Hence, a lower concentration of IBA (2.4 μM) was used for root proliferation according to Dhandapani et al. (2008) (Figure 3Av). Plants with well developed roots were transferred to 1:1 sterile soilrite:vermicompost mix and maintained in glass house conditions (Figure 3Avi and Supplementary Figures S4A,B). The complete process of transformation using hypocotyl explants from seed germination to hardening of putative transgenic lines took approximately 4 months. Regeneration efficiency from hypocotyl explants was very low as mentioned above and we were able to generate seven C. roseus lines overexpressing G(G)PPS. For PCR screening of putative transformants, 35S forward and two different G(G)PPS specific reverse primers were used. In both primer combinations, seven plants out of 18 kanamycin-selected plants were positive for G(G)PPS confirming the transgenic nature (Supplementary Figure S5A).
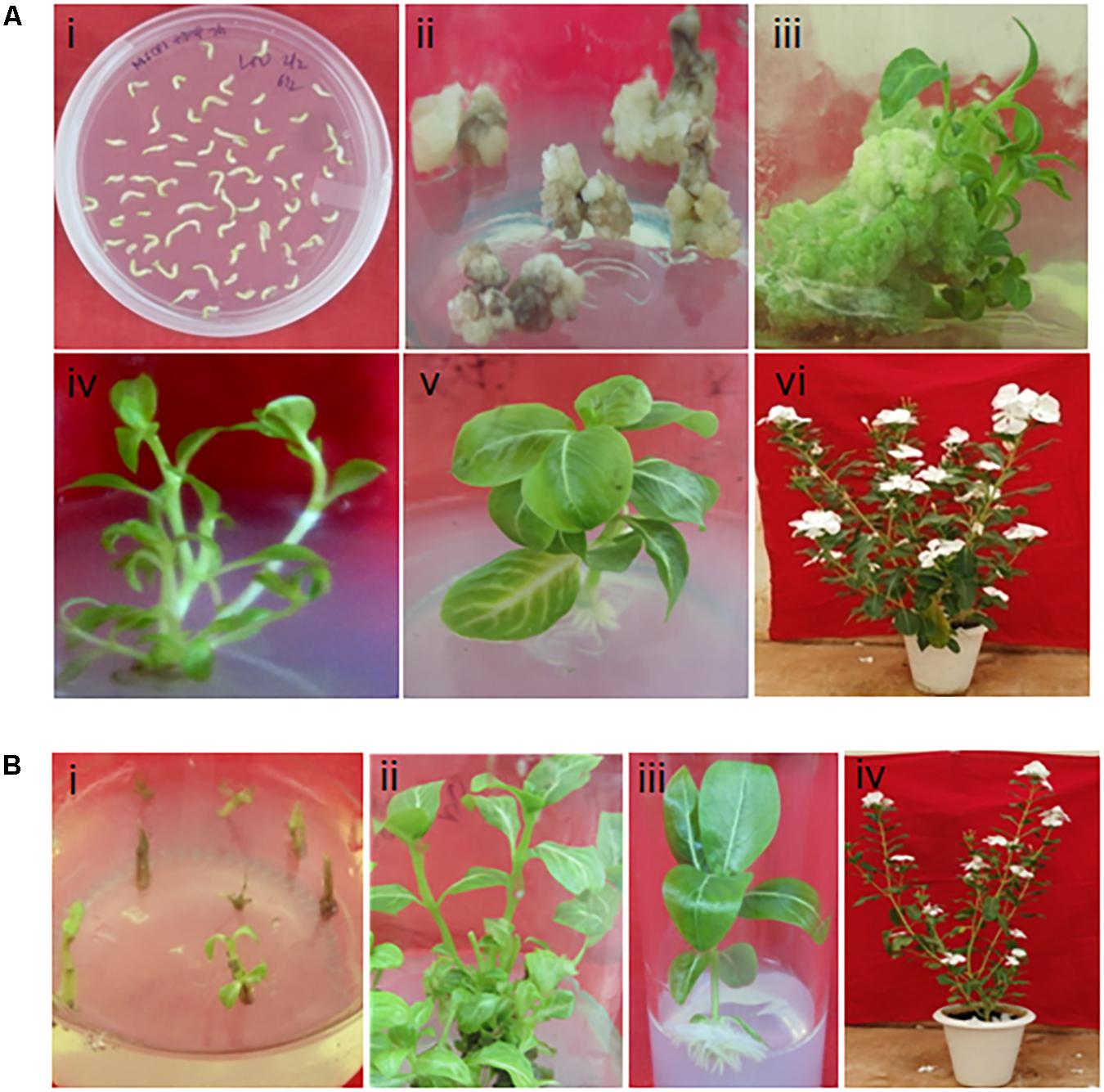
FIGURE 3. Generation of transgenic C. roseus plants overexpressing G(G)PPS and GES. (A) Transformation of C. roseus using hypocotyl explants for G(G)PPS overexpression. (i) Preculture of hypocotyl explants in MS basal media supplemented with 250 mg/L proline and 150 mg/L casein hydrolysate (MSCP) media; (ii) callus induction in MSCP1 media supplemented with 40 mg/L kanamycin and 300 mg/L carbenicillin; (iii) callus proliferation and shoot initiation in MSCP2 with 70 mg/L kanamycin and 300 mg/L carbenicillin; (iv) shoot elongation in MSCP3 with 90 mg/L kanamycin and 300 mg/L carbenicillin; (v) root proliferation in half strength MS media with 2.4 μM IBA; (vi) Established transgenic G(G)PPS plant in the flowering stage. (B) Transformation of C. roseus nodal explants for G(G)PPS+GES overexpression. (i and ii) Selection of nodal explants and multiple shoot induction in MS media supplemented with 1 mg/L BAP + 0.1 mg/L NAA + 400 mg/L thiamine HCl + 70 or 100 mg/L kanamycin + 300 mg/L carbenicillin, respectively; (iii) root proliferation in half strength MS media with 2.4 μM IBA; (iv) Established transgenic G(G)PPS+GES plant in the flowering stage.
The regeneration efficiency from embryogenic calli was found to be low (3%) and there was no regeneration in the case of explants co-transformed with G(G)PPS+GES. Hence, an alternate approach of genetic transformation using nodal explants was followed for co-transformation. After co-cultivation with Agrobacteria harboring G(G)PPS and GES overexpression constructs, nodal explants were selected in MS-BNT medium supplemented with 70 mg/L kanamycin and 300 mg/L carbenicillin (Figure 3Bi). Most of the co-cultivated explants turned brown and died upon transferring to selection media. Healthy explants were sub-cultured for five more rounds in kanamycin selection media with 70 mg/L. Subsequently, the kanamycin concentration was increased to 100 mg/L for next two rounds. The explants exhibited initiation of leaves and shoot elongation from third round of selection (∼1 month after co-cultivation). In most of the survived explants after five rounds of antibiotic selection, shoot bud induction as well as proliferation occurred from nodes resulting in 3–7 multiple shoots (Figure 3Bii). Individual shoots were then transferred to fresh selection media every 15 days and healthy surviving shoots were inoculated into rooting medium. Although root initiation was observed in rooting media containing 2 mg/L IBA, root proliferation was only obtained in a much lower IBA (2.4 μM) concentration (Figure 3Biii). Well rooted plants were transferred to pots containing 1:1 sterile soilrite:vermicompost mix (Figure 3Biv and Supplementary Figures S4A,B). After obtaining putative transformants of G(G)PPS+GES, PCR analysis using genomic DNA isolated from leaves was carried out to identify transgenic nature of plants. Screening of G(G)PPS+GES lines was carried out by using nptII forward and reverse primers, and 35S forward and gene specific [(G(G)PPS or GES] reverse primers. PCR results indicated three plants positive for G(G)PPS+GES out of 16 kanamycin selected plants (Supplementary Figures S5B,C). The transformation efficiency using nodal explants was also ∼3% similar to transformation using hypocotyls explants. PCR analysis using genomic DNA with primers specific for Agrobacterium chromosomal virulence gene (ChvA) revealed the absence of the corresponding band, thus ruling out the possibility of Agrobacterium contamination in both G(G)PPS and G(G)PPS+GES transformants (Supplementary Figure S6).
Ectopic Expression of G(G)PPS and G(G)PPS+GES Elevates Alkaloid Accumulation in C. roseus Transgenic Plants
Having confirmed the transgenic nature of C. roseus plants, the transcript abundance of G(G)PPS and GES in transgenic lines was analyzed by qRT-PCR. First, the transcript levels in all transgenic lines were determined with respect to WT control plants that were also generated through tissue culture. The various transgenic lines showed increased expression of G(G)PPS with a 2- to 9.5-fold increase compared to WT control plants, with maximum expression in G(G)PPS_5 followed by 8-fold in G(G)PPS_3 and G(G)PPS_7 (Figure 4A). Transgenic plants co-expressing G(G)PPS+GES showed increased expression of both genes (Figure 4B). The expression of G(G)PPS was ∼7-, 3-, and 4-fold in lines 1, 2 and 3, respectively, whereas the expression of GES was ∼5-fold in line 1, and ∼3-fold in lines 2 and 3 (Figure 4B). Next, to determine whether the changes in gene expression were also accompanied by increased MIA accumulation, metabolites were extracted from independent transgenic lines and quantified by HPLC. The content of terpenoid intermediate secologanin exhibited a significant boost of ∼2-fold in lines G(G)PPS_3, G(G)PPS_5 and G(G)PPS_7 that also showed highest transcript abundance (Figure 5A and Supplementary Figure S7A). There was no significant enhancement of secologanin in the remaining four G(G)PPS lines that correlated with the gene expression of G(G)PPS (Figure 5A). Further, to check whether improved availability of secologanin resulted in enhanced accumulation of monomeric alkaloids in leaves, vindoline and catharanthine levels were analyzed in G(G)PPS transgenic lines. HPLC analysis revealed that the trend in accumulation of monomers correlated with the level of secologanin in all seven lines with significant increase of 1.5- to 1.7-fold vindoline and 1.7- to 2.0-fold catharanthine in lines G(G)PPS_3, G(G)PPS_5 and G(G)PPS_7 (Figure 5A and Supplementary Figure S7A). Similarly, G(G)PPS+GES co-expressing lines exhibited a significant increase in secologanin (∼1.8-fold) along with significant increase in vindoline (1.6- to 2.2-fold) and catharanthine (2.5- to 3.0-fold) (Figure 5B and Supplementary Figure S7B). Although all three G(G)PPS+GES lines showed significant accumulation of secologanin and vindoline similar to G(G)PPS lines, the level of catharanthine was much higher (>3.0-fold) in co-expressing lines (Figure 5B and Supplementary Figure S7B).
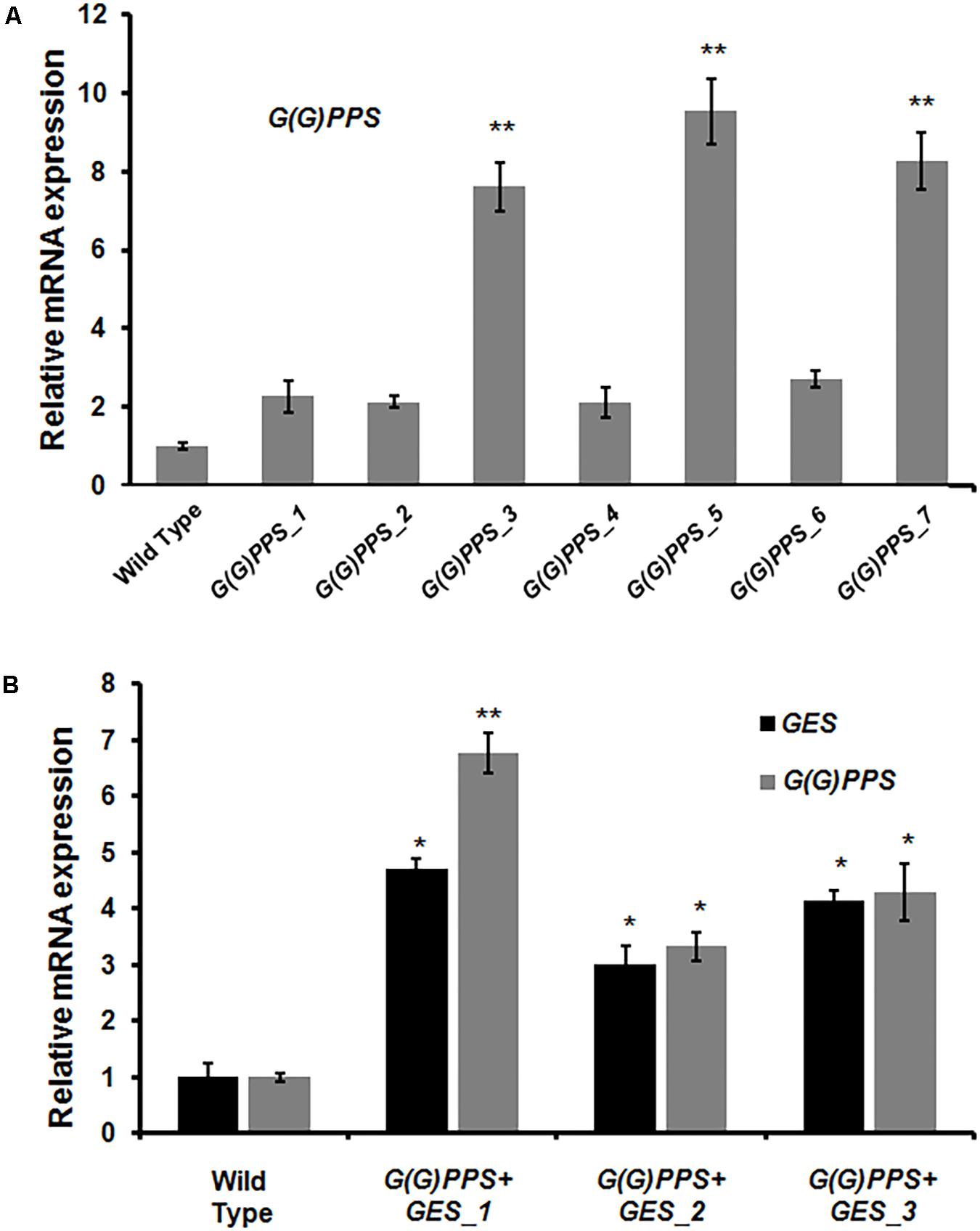
FIGURE 4. Analysis of gene expression in transgenic G(G)PPS and GES C. roseus plants. qRT-PCR analysis of G(G)PPS (gray bar) (A) and G(G)PPS (gray bar)/GES (black bar) (B) in transgenic C. roseus plants. Expression levels of genes were normalized to the endogenous reference gene CrN227 and are represented relative to the wild type (WT) controls, which was set to 1. Error bars represent mean ± standard error (SE) of three independent experiments. Significant differences at P < 0.05 and P < 0.01 are represented by “∗” and “∗∗”, respectively.
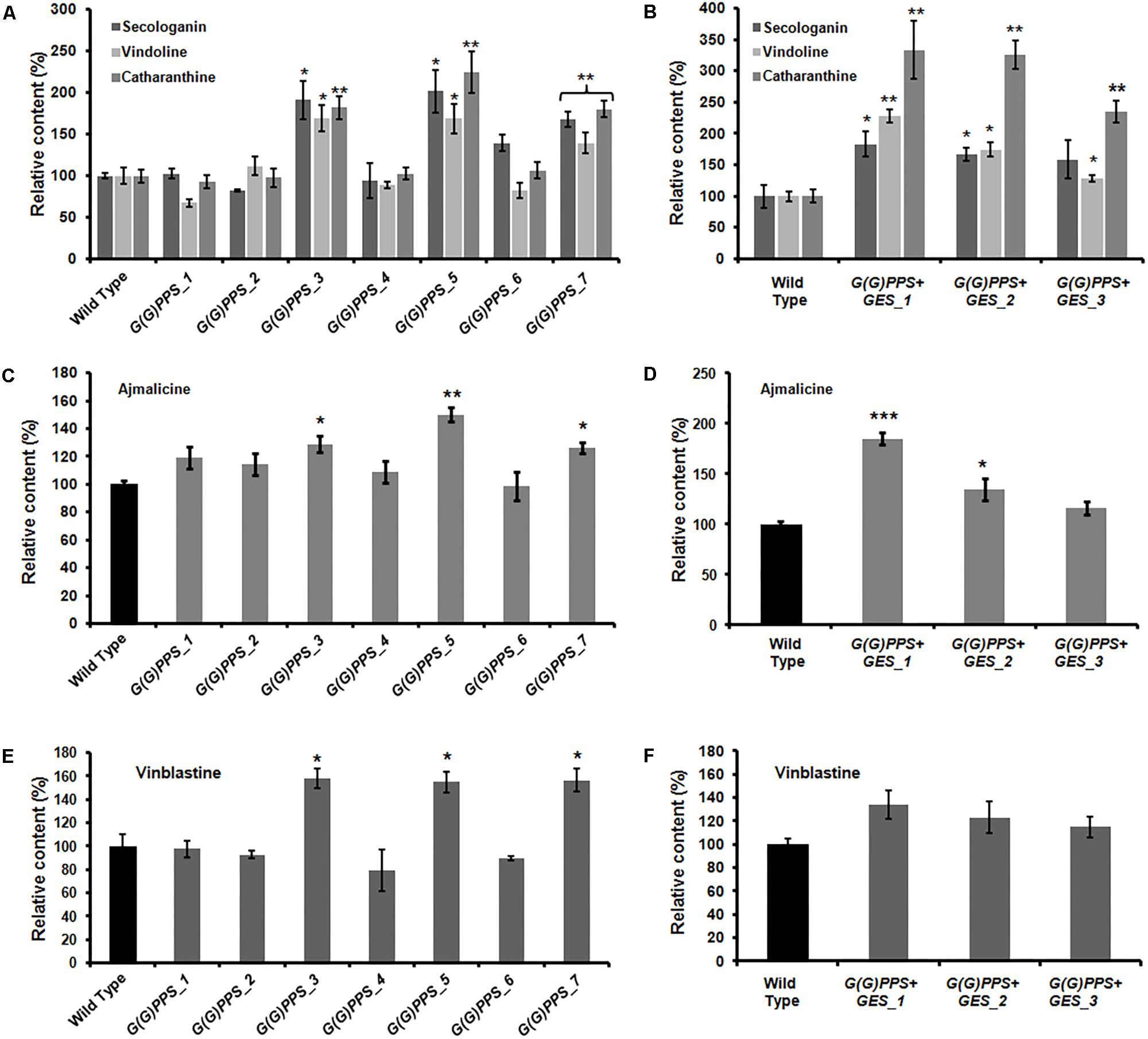
FIGURE 5. Quantification of metabolites in the T0 lines of transgenic G(G)PPS and GES C. roseus plants. Relative amounts of secologanin, vindoline and catharanthine (A,B), ajmalicine (C,D), and vinblastine (E,F) in transgenic G(G)PPS and G(G)PPS+GES C. roseus lines. Secologanin was extracted from 50 mg (fresh weight) of leaves and quantified following Tikhomiroff and Jolicoeur (2002). The monomeric alkaloids vindoline and catharanthine were extracted using 10 mg of oven dried leaves according to Lourdes Miranda-Ham et al. (2007), and quantified following Kumar et al. (2015). Ajmalicine was extracted from roots of wild type (WT) and transgenic plants using 200 mg fresh weight tissue following Pan et al. (2016). The methanolic extract was subjected to HPLC analysis to quantify ajmalicine. The dimeric alkaloid vinblastine was extracted using 50 mg of oven dried leaves and quantified according to Pan et al. (2016). In all cases, young leaves or roots of same developmental stages were used for alkaloid extraction and were quantified by using HPLC. The quantified metabolites are expressed as % levels relative to WT controls. Error bars represent mean ± standard error (SE) of three to four independent experiments. Significant differences at P < 0.05, P < 0.01, and P < 0.001 are represented by “∗”, “∗∗”, and “∗∗∗”, respectively.
In MIA biosynthesis, 4,21-dehydrogeissoschizine acts as a branch point intermediate for the formation of ajmalicine and stemmadenine. While ajmalicine is converted to serpentine, stemmadenine undergoes several transformations to form catharanthine and vindoline (Peebles et al., 2011). In order to assess the impact of G(G)PPS and GES overexpression on accumulation of root alkaloid, ajmalicine was quantified in roots of WT controls and transgenic lines by HPLC analysis. Consistent with the effect on secologanin, catharanthine and vindoline levels, G(G)PPS and GES overexpression enhanced the level of ajmalicine by ∼1.25- to 1.5-fold in three high expressing G(G)PPS transgenic lines (Figure 5C) and 1.3- to 1.8-fold in G(G)PPS+GES co-expressing lines (Figure 5D). This indicated that improved availability of GPP and geraniol due to overexpression of G(G)PPS and GES also influenced the accumulation of alkaloids in roots.
Differential Expression of Peroxidise 1 in Transgenic Lines Correlated With Vinblastine Accumulation
A significant increase of vinblastine (∼1.5-fold) was observed in three G(G)PPS overexpressing lines that exhibited increased secologanin, vindoline and catharanthine (Figures 5A,E). With respect to alkaloid accumulation in G(G)PPS+GES co-expressing plants, although there was elevated accumulation of terpenoid intermediate secologanin, and monomeric alkaloids, there was no significant increase in the level of vinblastine in any of the lines (Figures 5B,F). In a similar manner, when ORCA3 was expressed alone or co-expressed with G10H in transgenic C. roseus plants, monomers were significantly enhanced without any effect on accumulation of α-3′,4′-anhydrovinblastine and vinblastine (Pan et al., 2012). Peroxidise 1 (PRX1) mediates the coupling of monomeric catharanthine and vindoline into dimeric α-3′,4′-anhydrovinblastine, the immediate precursor for vinblastine (Costa et al., 2008). The fact that, the vinblastine level was boosted only in G(G)PPS transgenic plants and not in G(G)PPS+GES lines indicated a possible differential regulation at the terminal step involving PRX1. To verify this, transcript levels of PRX1 were determined in G(G)PPS and G(G)PPS+GES transgenic C. roseus lines. The analyses revealed a significant difference of PRX1 transcripts levels in G(G)PPS and G(G)PPS+GES transgenic lines (Figure 6). All three higher alkaloid accumulating G(G)PPS lines [G(G)PPS_3, G(G)PPS_5, and G(G)PPS_7] showed enhanced PRX1 transcript abundance ranging from fourfold to eightfold (Figure 6A). However, G(G)PPS+GES co-expressing lines exhibited PRX1 expression similar to that of WT controls (Figure 6B). This differential expression of PRX1 could be the possible reason for contrasting levels of vinblastine in G(G)PPS and G(G)PPS+GES transgenic plants.
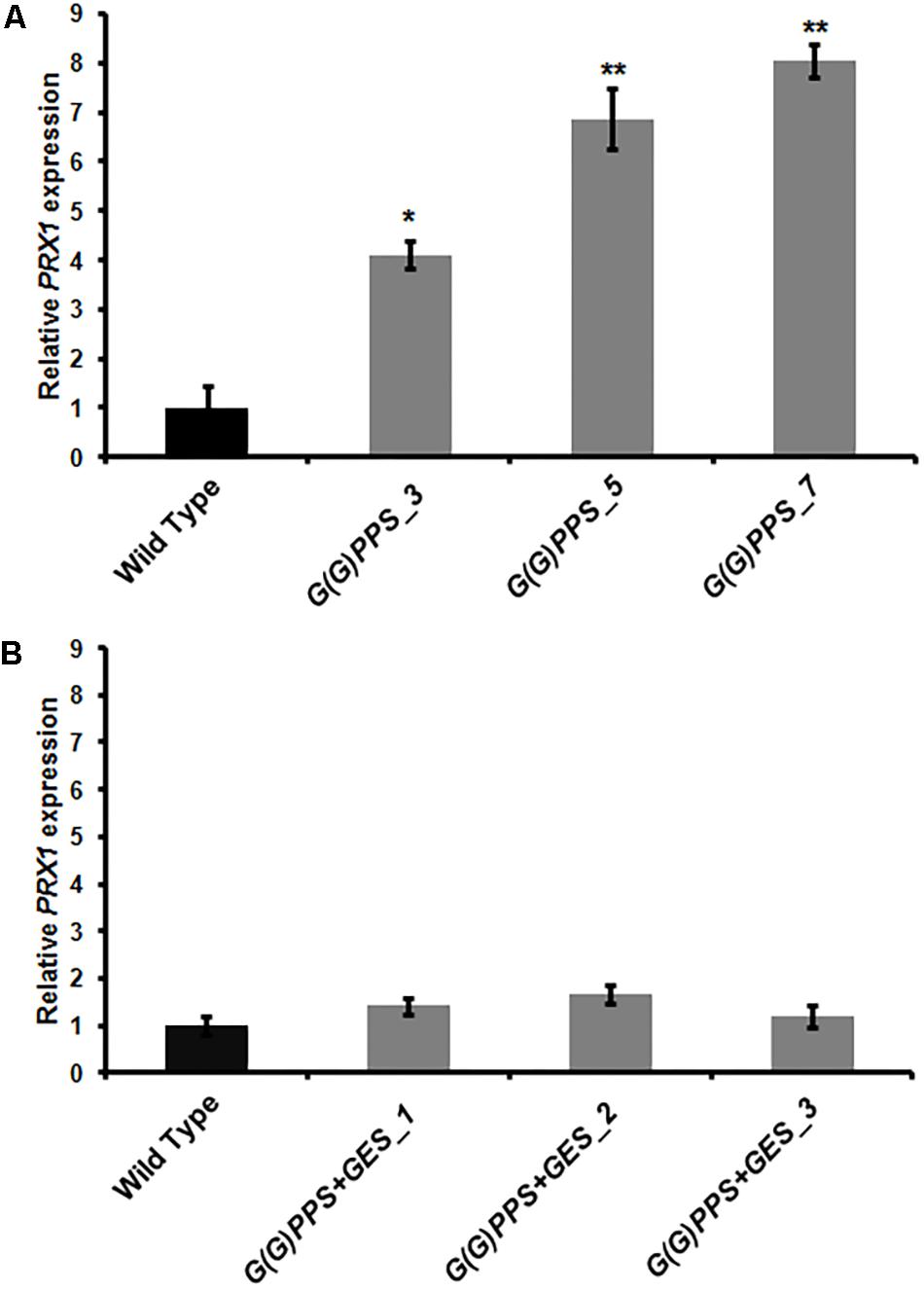
FIGURE 6. PRX1 expression in transgenic C. roseus plants. Analysis of PRX1 expression by qRT-PCR in G(G)PPS (A) and G(G)PPS+ GES (B) overexpressing transgenic C. roseus plants. Expression levels of PRX1 were normalized to the endogenous reference gene CrN227 and are represented relative to the WT controls, which was set to 1. Error bars represent mean ± standard error (SE) of three independent experiments. Significant differences at P < 0.05 and P < 0.01 are represented by “∗” and “∗∗”, respectively.
Transgenic C. roseus Plants Exhibited Stable Levels of Gene Expression and Alkaloids in the T1 Generation
To determine the genetic and chemostability, we checked the transcript and metabolite levels in PCR positive T1 lines of G(G)PPS and G(G)PPS+GES (Figure 7 and Supplementary Figures S8, S9). First, transgene copy numbers in selected G(G)PPS [G(G)PPS_1, G(G)PPS_3, G(G)PPS_5, and G(G)PPS_7] and G(G)PPS+GES transgenic lines were determined by qPCR using genomic DNA extracted from WT controls and transgenic lines. The correlation coefficients of the standard curves of target genes, G(G)PPS, GES, and TDC internal control were 0.996–0.998, and PCR efficiencies were close to 97–100%. This indicated the accuracy and robustness in estimating copy number of genes based on the standard curves. The analysis revealed that G(G)PPS_1, G(G)PPS_3, and G(G)PPS_7 showed the presence of two copies, whereas line G(G)PPS_5 exhibited the presence of three copies of G(G)PPS (Supplementary Table S2). With respect to co-expressing lines, G(G)PPS+GES_1 showed three copies, whereas lines G(G)PPS+GES_2 and G(G)PPS+GES_3 possessed two copies of G(G)PPS. The copy number of GES was found to be three in all the co-expressing transgenic lines (Supplementary Table S2). The WT controls showed one copy each for endogenous G(G)PPS and GES. Hence, the determined copy numbers also include the endogenous copy of G(G)PPS and GES in all transgenic events.
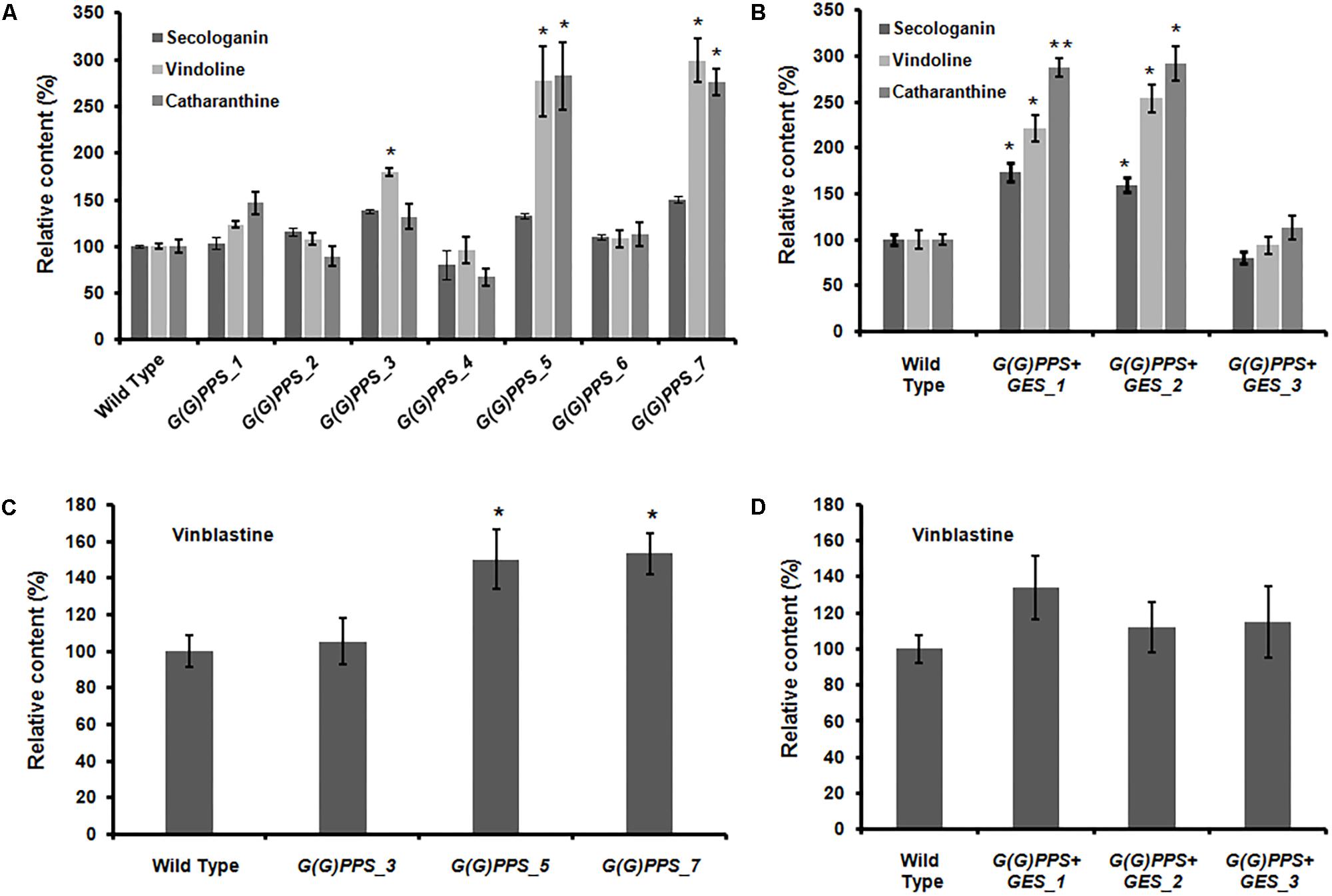
FIGURE 7. Analyses of metabolites in T1 lines of transgenic G(G)PPS and G(G)PPS+ GES in C. roseus plants. Relative levels of secologanin, vindoline and catharanthine (A,B), and vinblastine (C,D) in transgenic G(G)PPS (A,C) and G(G)PPS+GES (B,D) C. roseus lines. In the case of G(G)PPS transgenic plants, only those lines showing significant increase in the monomeric alkaloids catharanthine and vindoline (A) were considered for the dimeric vinblastine analysis (B). Error bars represent mean ± standard error (SE) of three to four independent experiments. Significant differences at P < 0.05 and P < 0.01 are represented by “∗” and “∗∗”, respectively.
Transcript levels in the T1 G(G)PPS transgenic plants exhibited higher G(G)PPS expression (8- to 10-fold) in G(G)PPS_5 and G(G)PPS_7 lines (Supplementary Figure S9A) similar to the levels observed in the T0 lines. However, G(G)PPS_3 line that showed significantly higher transcript accumulation (∼8-fold) in the T0 generation, exhibited reduced expression in the T1 generation was comparable to WT control plants (Figure 4A and Supplementary Figure S9A). In co-transformed C. roseus lines, G(G)PPS transcript abundance was sixfold in line G(G)PPS+GES_1 and ∼3-fold in other two lines (Supplementary Figure S9B), whereas the expression of GES varied between threefold and fourfold in all three lines (Supplementary Figure S9B). To confirm whether increased gene expression was accompanied by elevated MIA accumulation in transgenic lines, alkaloids were quantified in T1 G(G)PPS and G(G)PPS+GES lines. Similar to the accumulation observed in the T0 generation, T1 G(G)PPS lines exhibited improved accumulation of monomeric alkaloids with no significant change in levels of secologanin (Figure 7A and Supplementary Figure S10A). While G(G)PPS_5 and G(G)PPS_7 exhibited ∼2.5-to 2.7-fold accumulation of vindoline and catharanthine, G(G)PPS_3 showed significant increase of only vindoline (∼1.8-fold) (Figure 7A). As for the dimeric alkaloids, only G(G)PPS_5 and G(G)PPS_7 exhibited ∼1.5-fold increase in vinblastine compared to WT controls (Figure 7C and Supplementary Figure S10A). Among the co-expressing G(G)PPS+GES plants, only two T1 plants showed improved accumulation of secologanin, (∼1.5-fold), vindoline (∼2.2-fold), and catharanthine (∼2.8-fold) (Figure 7B and Supplementary Figure S10B). Also, similar to the T0 plants, there was no increase in the level of vinblastine in any of the T1 G(G)PPS+GES co-expressing lines possibly due to regulation of PRX1 (Figure 7D and Supplementary Figure S10B).
Discussion
Vinblastine and vincristine are blockbuster anti-cancer drugs extracted exclusively from C. roseus leaves. As these dimeric MIAs are accumulated at very low levels in leaves, increasing their foliar concentrations would reduce the cost of production. Hence, this study was taken up to enhance the production of monomeric and dimeric MIAs by overexpression of early secoiridoid pathway genes in transgenic C. roseus plants. Previous efforts of overexpression or silencing of transcriptional regulators and pathway genes in C. roseus cell cultures have had limited success in improving MIAs production, indicating a complex regulatory mechanism that balances metabolic flux and thus MIAs accumulation in C. roseus (Murata and De Luca, 2005; Peebles et al., 2009; Jaggi et al., 2011; Van Moerkercke et al., 2015; Sun et al., 2017). In order to allow higher metabolic outputs, improving the availability of upstream precursors is an alternative strategy. Initial elicitation and feeding studies using indole and terpenoid precursors in C. roseus cell cultures indicated inconsistent results on accumulation of different MIAs including serpentine, ajmalicine, tabersonine, and strictosidine (Moreno et al., 1993; Whitmer et al., 1998; Contin et al., 1999). However, later studies in cell suspension and hairy roots of C. roseus indicated that the terpenoid moiety, geraniol (not indole moiety, tryptophan) has a positive effect on accumulation of MIAs like tabersonine and ajmalicine (Morgan and Shanks, 2000; Lee-Parsons and Royce, 2006). In addition, we have previously shown at the whole plant level that GPPS and GES play important roles in providing metabolic flux toward downstream MIA biosynthesis in C. roseus (Rai et al., 2013; Kumar et al., 2015). Since GPPS and GES provide starting precursors (GPP and geraniol) for the terpene moiety formation, we hypothesized that overexpression of G(G)PPS and co-expression of G(G)PPS+GES could push the flux toward secologanin biosynthesis ultimately leading to improved MIA production. To this end, we performed transient overexpression of these genes by agroinfiltration as this strategy has been used to validate gene function in different medicinal plants including C. roseus (Raina et al., 2012; Rai et al., 2013; Kumar et al., 2015). Transient overexpression of G(G)PPS and G(G)PPS+GES lead to enhanced secologanin and the monomeric MIAs (Figure 2 and Supplementary Figure S3), indicating that stable overexpression of these genes in transgenic C. roseus plants could result in improved production of MIAs.
The lack of a reliable genetic transformation method in C. roseus at the whole plant level has been a major impediment for improving MIAs by metabolic engineering approaches (Zarate and Verpoorte, 2007). Owing to the recalcitrant nature and low transformation efficiency, only a few reports have demonstrated genetic transformation in C. roseus using genes related to MIA biosynthesis (Pan et al., 2012; Wang Q. et al., 2012). Here, we generated transgenic C. roseus overexpressing G(G)PPS using transformation protocol of Wang Q. et al. (2012) with minor modifications. Although Wang Q. et al. (2012) reported transformation efficiency of 11% for transgenic C. roseus expressing DAT gene, in our case the efficiency of transformation was ∼3%. As hypocotyl explants co-cultivated with G(G)PPS+GES constructs failed to generate shoots, an alternate regeneration method using nodal explants as reported by Verma and Mathur (2011a) was employed for transformation. This strategy yielded only three transformants with ∼3% transformation efficiency. The lower transformation efficiency in this study could be due to the genotype of C. roseus used as it has been shown in other plant species that transformation efficiency depends on the genotype and cultivar (Wang Y. et al., 2012; Udayakumar et al., 2014). Transgenic G(G)PPS and G(G)PPS+GES lines exhibited varied levels of transcript abundance for corresponding genes (Figure 4). G(G)PPS expression ranged from 2- to 9.5-fold and 3- to 6-fold in G(G)PPS and G(G)PPS+GES transgenic lines, respectively, whereas GES expression was 3- to 4.5-fold in co-expressing lines (Figure 4). Similar to our observation, overexpression of DAT in transgenic C. roseus resulted in twofold to sevenfold expression in different lines (Wang Q. et al., 2012). In another report, overexpression of transcriptional regulator ORCA3 alone in transgenic C. roseus plants resulted only in ∼2-fold expression, whereas transgenic lines co-expressing G10H and ORCA3 resulted in ∼5- and 7-fold increase in transcript levels of G10H and ORCA3, respectively (Pan et al., 2012). Increased transcript accumulation of G(G)PPS and G(G)PPS+GES in transgenic C. roseus plants enhanced the content of secoiridoid secologanin, monomeric vindoline and catharanthine, and root alkaloid ajmalicine (Figures 5 and Supplementary Figure S7). Overall, the level of secologanin and vindoline in three higher expressing G(G)PPS lines and in all G(G)PPS+GES transgenic lines remained similar (Figures 5A,B). However, there was higher accumulation of catharanthine in two G(G)PPS+GES lines compared to G(G)PPS lines (Figures 5A,B), suggesting that improved availability of both GPP and geraniol could have enhanced the accumulation of catharanthine. It was reported that co-expression of 1-deoxy-D-xylulose-5-phosphate synthase (DXS) and G10H in hairy roots resulted in improved accumulation of ajmalicine and tabersonine compared to expression of DXS alone (Peebles et al., 2011). Similarly, C. roseus transgenic plants co-expressing ORCA3 and G10H exhibited higher production of ajmalicine compared to plants expressing ORCA3 alone (Pan et al., 2012). These prior findings along with our present results suggest that overexpression of multiple genes may be necessary to achieve significant gains in product accumulation especially in pathways involving multiple branches, where precursors are channeled into diverse metabolites (Peebles et al., 2011).
In addition to enhancing secologanin and monomeric alkaloids, G(G)PPS overexpressing lines also accumulated higher level of vinblastine (Figure 5E and Supplementary Figure S7A). In contrast, G(G)PPS+GES lines did not show any increase in the levels of vinblastine despite of significant increase in secologanin, vindoline, and catharanthine (Figure 5F). This result suggested that improved metabolic flux (in the form of monomeric alkaloids) is somehow not being utilized for dimeric alkaloid formation. These findings prompted us to check whether gene expression of PRX1 has any influence on the accumulation of vinblastine. Transcript analysis revealed that G(G)PPS and G(G)PPS+GES overexpressors had a differential expression of PRX1 that correlated with the accumulation of vinblastine (Figures 5E,F and Supplementary Figure S7). It is not clear at this point, how PRX1 is differentially expressed in G(G)PPS and G(G)PPS+GES overexpressors. However, PRX1 may be transcriptionally regulated by some unknown mechanism in G(G)PPS overexpressors leading to enhanced vinblastine. It has been previously reported that the expression of PRX1 and accumulation of 3′,4′-anhydrovinblastine is under complex regulation and also depends on the availability of hydrogen peroxide (Costa et al., 2008). In addition, PRX1 has been suggested to act as positive regulator and interacts with ethylene responsive factor (ERF) regulating accumulation of 3′,4′-anhydrovinblastine in C. roseus (Wang et al., 2016).
The success of genetic engineering depends on genetic and chemical stability of transgenic plants in subsequent generations (Verma et al., 2015). The copy number of integrated gene affects the expression level and genetic stability in transgeniclines. One or two copies of target gene integration in transgenic plants have been reported ideal for genetic stability (Tang et al., 2007). The integration of multiple copies of transgene into one or more chromosomes might result in low genetic stability, transcriptional or post transcriptional gene silencing. Lately, qPCR has been successfully utilized for determining transgene copy number in transgenic plants of different species (Weng et al., 2004; Yi et al., 2008; Li et al., 2017). In the present study, qPCR determination of copy number revealed that one to two copies of G(G)PPS in different G(G)PPS and G(G)PPS+GES overexpressing lines, whereas it was found to be two for GES in all three G(G)PPS+GES transgenic lines (Supplementary Table S2). Previous transgenic studies in C. roseus plants have analyzed the metabolites in only T0 plants (Pan et al., 2012; Wang Q. et al., 2012). Hence to check the chemo-stability in subsequent generations, the level of secologanin and downstream alkaloids were measured in T1 lines of G(G)PPS and G(G)PPS+GES transgenic lines. While catharanthine and vindoline displayed better accumulation in two T1 G(G)PPS lines [G(G)PPS_5 and G(G)PPS_7] compared to that of T0 plants (Figures 5A, 7A), the vinblastine levels were comparable in both T0 and T1 lines (Figures 5B, 7B). Among the three T1 G(G)PPS+GES lines, G(G)PPS+GES_1 and G(G)PPS+GES_2 lines exhibited a similar trend to that of T0 plants for all analyzed metabolites including vinblastine (Figures 5B,F, 7B,D). As observed in T0 lines, PRX1 exhibited differential expression that correlated with vinblastine levels in T1 transgenic lines with higher levels of vindoline and catharanthine (Figure 6 and Supplementary Figure S9C).
Overexpression of GPPS and some of the terpene synthases redirects metabolic flux toward secondary metabolite biosynthesis, thus lowering the flux for the synthesis of other primary metabolites thereby affecting plant growth. For instance, transgenic tobacco plants expressing snapdragon GPS.SSU were dwarf and displayed strong chlorosis with reduced chlorophyll content (Orlova et al., 2009). Also, overexpression of strawberry linalool/nerolidol synthase and taxadiene synthase in Arabidopsis resulted in a dwarf phenotype due to reduction in gibberellic acid levels (Aharoni et al., 2003; Besumbes et al., 2004). In contrast, co-expression of peppermint GPPS.SSU with different monoterpene synthases enhanced the monoterpene production without affecting plant growth in tobacco (Yin et al., 2017). Here, overexpression of G(G)PPS alone or together with GES did not alter the C. roseus plant phenotype (Supplementary Table S3 and Supplementary Figure S4C). Selected transgenic lines were tested for growth parameters and no differences were found (Supplementary Table S3). As we have overexpressed a bifunctional G(G)PPS, it could have provided higher flux of GGPP and GPP, respectively, required for generalized and specialized metabolism in transgenic lines, thereby not affecting the plant growth. Indeed, two best expressing G(G)PPS transgenic lines exhibited a significant increase in the level of total chlorophyll indicating that enhanced GGPP production by the bifunctional enzyme could have resulted in improved chlorophyll content (Supplementary Figure S11). Although overexpression of Picea abies bifunctional G(G)PPS resulted in increased accumulation of geranylgeranyl fatty acid esters, chlorophylls and carotenoids remained unaffected (Nagel et al., 2014).
In summary, we demonstrate that the level of monomeric MIAs and pharmaceutically important vinblastine can be enhanced in plants by transgenic overexpression of genes involved in early steps of secoiridoid pathway. While overexpression of bifunctional G(G)PPS enhanced both monomeric and dimeric MIAs, co-expression of G(G)PPS and GES led to increase of monomeric MIAs higher than G(G)PPS overexpressors. Improved accumulation of MIAs in transgenic periwinkle without compromising the plant growth indicated the positive role of bifunctional G(G)PPS in both generalized and specialized metabolism. Commercial exploitation of G(G)PPS transgenic lines could reduce the cost of dimeric alkaloids production and co-expressing lines could be exploited for vindoline and catharanthine production or may be used in molecular breeding approaches to further improve the dimeric alkaloids content. Moreover, future research focusing on stacking of a combination of MIA pathway genes including PRX1 and transcriptional regulators could enhance the flux resulting in further improvement of alkaloids in C. roseus.
Author Contributions
SK and HS performed the experiments. SK, HS, and DN analyzed the data. DN conceived and coordinated the research. SK and DN wrote the manuscript.
Funding
This work was supported by Department of Biotechnology, Government of India funded project (BT/PR6109/AGII/106/857/2012; GAP-272) to DN. SK was initially supported by Research Associateship by GAP-272 and later by CSIR Senior Research Associateship. HS acknowledges ICMR for the Research Fellowship.
Conflict of Interest Statement
The authors declare that the research was conducted in the absence of any commercial or financial relationships that could be construed as a potential conflict of interest.
Acknowledgments
The authors express their sincere gratitude to the Director, CSIR-CIMAP for his support throughout the study. Authors are also thankful to Dr. Micheal Long for his help with English corrections. Institutional communication number for this article is CIMAP/PUB/2018/01.
Supplementary Material
The Supplementary Material for this article can be found online at: https://www.frontiersin.org/articles/10.3389/fpls.2018.00942/full#supplementary-material
Footnotes
References
Aharoni, A., Giri, A. P., Deuerlein, S., Griepink, F., de Kogel, W. J., Verstappen, F. W., et al. (2003). Terpenoid metabolism in wild-type and transgenic Arabidopsis plants. Plant Cell 15, 2866–2884. doi: 10.1105/tpc.016253
Alam, P., Khan, Z. A., Abdin, M. Z., Khan, J. A., Ahmad, P., Elkholy, S. F., et al. (2017). Efficient regeneration and improved sonication-assisted Agrobacterium transformation (SAAT) method for Catharanthus roseus. 3 Biotech. 7:26.
Besumbes,Ó., Sauret-Gueto, S., Phillips, M. A., Imperial, S., Rodriguez-Concepcion, M., and Boronat, A. (2004). Metabolic engineering of isoprenoid biosynthesis in Arabidopsis for the production of taxadiene, the first committed precursor of taxol. Biotechnol. Bioeng. 88, 168–175. doi: 10.1002/bit.20237
Choi, P. S., Kim, Y. D., Choi, K. M., Chung, H. J., Choi, D. W., and Liu, J. R. (2004). Plant regeneration from hairy-root cultures transformed by infection with Agrobacterium rhizogenes in Catharanthus roseus. Plant Cell Rep. 22, 828–831. doi: 10.1007/s00299-004-0765-3
Contin, A., van der Heijden, R., and Verpoorte, R. (1999). Effects of alkaloid precursor feeding and elicitation on the accumulation of secologanin in a Catharanthus roseus cell suspension culture. Plant Cell Tissue Organ Cult. 56, 111–119. doi: 10.1023/A:1006257125191
Costa, M. M., Hilliou, F., Duarte, P., Pereira, L. G., Almeida, I., Leech, M., et al. (2008). Molecular cloning and characterization of a vacuolar class III peroxidase involved in the metabolism of anticancer alkaloids in Catharanthus roseus. Plant Physiol. 146, 403–417. doi: 10.1104/pp.107.107060
Dhandapani, M., Kim, D. H., and Hong, S. (2008). Efficient plant regeneration via somatic embryogenesis and organogenesis from explants of Catharanthus roseus. In Vitro Cell Dev. Biol. Plant. 44, 18–25. doi: 10.1007/s11627-007-9094-x
Dudareva, N., Negre, F., Nagegowda, D. A., and Orlova, I. (2006). Plant volatiles: recent advances and future perspectives. Crit. Rev. Plant Sci. 25, 417–440. doi: 10.1080/07352680600899973
Goddijn, O. J., Lohman, F. P., de Kam, R. J., Schilperoort, R. A., and Hoge, J. H. (1994). Nucleotide sequence of the tryptophan decarboxylase gene of Catharanthus roseus and expression of tdc-gusA gene fusions in Nicotiana tabacum. Mol. Gen. Genet. 242, 217–225. doi: 10.1007/BF00391016
Guirimand, G., Burlat, V., Oudin, A., Lanoue, A., St-Pierre, B., and Courdevault, V. (2009). Optimization of the transient transformation of Catharanthus roseus cells by particle bombardment and its application to the sub-cellular localization of hydroxymethylbutenyl 4-diphosphate synthase and geraniol 10-hydroxylase. Plant Cell Rep. 28, 1215–1234. doi: 10.1007/s00299-009-0722-2
Ishikawa, H., Colby, D. A., and Boger, D. L. (2008). Direct coupling of catharanthine and vindoline to provide vinblastine: total synthesis of (+)- and ent-(-)-vinblastine. J. Am. Chem. Soc. 130, 420–421. doi: 10.1021/ja078192m
Jaggi, M., Kumar, S., and Sinha, A. K. (2011). Overexpression of an apoplastic peroxidase gene CrPrx in transgenic hairy root lines of Catharanthus roseus. Appl. Microbiol. Biot. 90, 1005–1016. doi: 10.1007/s00253-011-3131-8
Kellner, F., Kim, J., Clavijo, B. J., Hamilton, J. P., Childs, K. L., Vaillancourt, B., et al. (2015). Genome-guided investigation of plant natural product biosynthesis. Plant J. 82, 680–692. doi: 10.1111/tpj.12827
Kuboyama, T., Yokoshima, S., Tokuyama, H., and Fukuyama, T. (2004). Stereocontrolled total synthesis of (+)-vincristine. Proc. Natl. Acad. Sci. U.S.A. 101, 11966–11970. doi: 10.1073/pnas.0401323101
Kumar, K., Kumar, S. R., Dwivedi, V., Rai, A., Shukla, A. K., Shanker, K., et al. (2015). Precursor feeding studies and molecular characterization of geraniol synthase establish the limiting role of geraniol in monoterpene indole alkaloid biosynthesis in Catharanthus roseus leaves. Plant Sci. 239, 56–66. doi: 10.1016/j.plantsci.2015.07.007
Lee-Parsons, C. W. T., and Royce, A. J. (2006). Precursor limitations in methyljasmonate-induced Catharanthus roseus cell cultures. Plant Cell Rep. 25, 607–612. doi: 10.1007/s00299-005-0109-y
Li, S., Cong, Y., Liu, Y., Wang, T., Shuai, Q., and Chen, N., et al. (2017). Optimization of Agrobacterium-mediated transformation in soybean. Front. Plant Sci. 8:246. doi: 10.3389/fpls.2017.00246
Lichtenthaler, H. K. (1987). Chlorophylls and carotenoids: pigments of photosynthetic biomembranes. Method Enzymol. 148, 350–382. doi: 10.1016/0076-6879(87)48036-1
Lourdes Miranda-Ham, M. D., Islas-Flores, I., and Vázquez-Flota, A. F. (2007). Accumulation of monoterpenoid indole alkaloids in periwinkle seedlings (Catharanthus roseus) as a model for the study of plant-environment interactions. Biochem. Mol. Biol. Educ. 35, 206–210. doi: 10.1002/bmb.60
Miettinen, K., Dong, L., Navrot, N., Schneider, T., Burlat, V., Pollier, J., et al. (2014). The seco-iridoid pathway from Catharanthus roseus. Nat. Commun. 5:3606. doi: 10.1038/ncomms4606
Moreno, P. R., van der Heijden, R., and Verpoorte, R. (1993). Effect of terpenoid precursor feeding and elicitation on formation of indole alkaloids in cell suspension cultures of Catharanthus roseus. Plant Cell Rep. 12, 702–705. doi: 10.1007/BF00233423
Morgan, J. A., and Shanks, J. V. (2000). Determination of metabolic rate-limitations by precursor feeding in Catharanthus roseus hairy root cultures. J. Biotechnol. 79, 137–145. doi: 10.1016/S0168-1656(00)00221-2
Murashige, T., and Skoog, F. (1962). A revised medium for rapid growth and bioassays with tobacco tissue cultures. Physiol. Plant 15, 473–497. doi: 10.1111/j.1399-3054.1962.tb08052.x
Murata, J., and De Luca, V. (2005). Localization of tabersonine 16-hydroxylase and 16-OH tabersonine-16-O-methyltransferase to leaf epidermal cells defines them as a major site of precursor biosynthesis in the vindoline pathway in Catharanthus roseus. Plant J. 44, 581–594. doi: 10.1111/j.1365-313X.2005.02557.x
Nagegowda, D. A. (2010). Plant volatile terpenoid metabolism: biosynthetic genes, transcriptional regulation and subcellular compartmentation. FEBS Lett. 584, 2965–2973. doi: 10.1016/j.febslet.2010.05.045
Nagel, R., Berasategui, A., Paetz, C., Gershenzon, J., and Schmidt, A. (2014). Overexpression of an isoprenyl diphosphate synthase in spruce leads to unexpected terpene diversion products that function in plant defense. Plant Physiol. 164, 555–569. doi: 10.1104/pp.113.228940
Noble, R. L. (1990). The discovery of the vinca alkaloids- chemotherapeutic agents against cancer. Biochem. Cell. Biol. 68, 1344–1351. doi: 10.1139/o90-197
Orlova, I., Nagegowda, D. A., Kish, C. M., Gutensohn, M., Maeda, H., Varbanova, M., et al. (2009). The small subunit of snapdragon geranyl diphosphate synthase modifies the chain length specificity of tobacco geranylgeranyl diphosphate synthase in planta. Plant Cell 21, 4002–4017. doi: 10.1105/tpc.109.071282
Pan, Q., Saiman, M. Z., Mustafa, N. R., Verpoorte, R., and Tang, K. (2016). A simple and rapid HPLC-DAD method for simultaneously monitoring the accumulation of alkaloids and precursors in different parts and different developmental stages of Catharanthus roseus plants. J. Chromatogr. B Analyt. Technol. Biomed. Life Sci. 1, 10–16. doi: 10.1016/j.jchromb.2016.01.034
Pan, Q., Wang, Q., Yuan, F., Xing, S., Zhao, J., Choi, Y. H., et al. (2012). Overexpression of ORCA3 and G10H in Catharanthus roseus plants regulated alkaloid biosynthesis and metabolism revealed by NMR-metabolomics. PLoS ONE 7:e43038. doi: 10.1371/journal.pone.0043038
Peebles, C. A., Hughes, E. H., Shanks, J. V., and San, K. Y. (2009). Transcriptional response of the terpenoid indole alkaloid pathway to the overexpression of ORCA3 along with jasmonic acid elicitation of Catharanthus roseus hairy roots over time. Metab. Eng. 11, 76–86. doi: 10.1016/j.ymben.2008.09.002
Peebles, C. A., Sander, G. W., Hughes, E. H., Peacock, R., Shanks, J. V., and San, K. Y. (2011). The expression of 1-deoxy-D-xylulose synthase and geraniol-10-hydroxylase or anthranilate synthase increases terpenoid indole alkaloid accumulation in Catharanthus roseus hairy roots. Metab. Eng. 13, 234–240. doi: 10.1016/j.ymben.2010.11.005
Peebles, C. A. M., Hong, S. B., Gibson, S. I., Shanks, J. V., and San, K. Y. (2006). Effects of terpenoid precursor feeding on Catharanthus roseus hairy roots overexpressing the alpha or alpha and beta subunits of anthranilate synthase. Biotechnol. Bioeng. 93, 534–540. doi: 10.1002/bit.20739
Pfaffl, M. W. (2001). A new mathematical model for relative quantification in real-time RT-PCR. Nucleic Acids Res. 29:e45. doi: 10.1093/nar/29.9.e45
Pollier, J., Vanden Bossche, R., Rischer, H., and Goossens, A. (2014). Selection and validation of reference genes for transcript normalization in gene expression studies in Catharanthus roseus. Plant Physiol. Biochem. 83, 20–25. doi: 10.1016/j.plaphy.2014.07.004
Rai, A., Smita, S. S., Singh, A. K., Shanker, K., and Nagegowda, D. A. (2013). Heteromeric and homomeric geranyl diphosphate synthases from Catharanthus roseus and their role in monoterpene indole alkaloid biosynthesis. Mol. Plant. 6, 1531–1549. doi: 10.1093/mp/sst058
Raina, S. K., Wankhede, D. P., Jaggi, M., Singh, P., Jalmi, S. K., Raghuram, B., et al. (2012). CrMPK3, a mitogen activated protein kinase from Catharanthus roseus and its possible role in stress induced biosynthesis of monoterpenoid indole alkaloids. BMC Plant Biol. 12:134. doi: 10.1186/1471-2229-12-134
Sun, J., Zhao, L., Shao, Z., Shanks, J., and Peebles, C. A. M. (2017). Expression of tabersonine 16-hydroxylase and 16-hydroxytabersonine-O-methyltransferase in Catharanthus roseus hairy roots. Biotechnol. Bioeng. 115, 673–683. doi: 10.1002/bit.26487
Tang, W., Newton, R. J., and Weidner, D. A. (2007). Genetic transformation and gene silencing mediated by multiple copies of a transgene in eastern white pine. J. Exp. Bot. 58, 545–554. doi: 10.1093/jxb/erl228
Tikhomiroff, C., and Jolicoeur, M. (2002). Screening of Catharanthus roseus secondary metabolites by high-performance liquid chromatography. J. Chromatogr. A 955, 87–93. doi: 10.1016/S0021-9673(02)00204-2
Udayakumar, R., Kasthurirengan, S., Mariashibu, T. S., Rayan, J. J. S., Ganapathi, A., Kim, S. C., et al. (2014). Agrobacterium-mediated genetic transformation of Withania somnifera using nodal explants. Acta Physiol. Plant. 36, 1969–1980. doi: 10.1007/s11738-014-1572-2
van Der Heijden, R., Jacobs, D. I., Snoeijer, W., Hallard, D., and Verpoorte, R. (2004). The Catharanthus alkaloids: pharmacognosy and biotechnology. Curr. Med. Chem. 11, 607–628. doi: 10.2174/0929867043455846
Van Moerkercke, A., Steensma, P., Schweizer, F., Pollier, J., Gariboldi, I., Payne, R., et al. (2015). The bHLH transcription factor BIS1 controls the iridoid branch of the monoterpenoid indole alkaloid pathway in Catharanthus roseus. Proc. Natl. Acad. Sci. U.S.A.112, 8130–8135. doi: 10.1073/pnas.1504951112
Verma, P., and Mathur, A. K. (2011a). Direct shoot bud organogenesis and plant regeneration from pre-plasmolysed leaf explants in Catharanthus roseus. Plant Cell Tissue Organ Cult. 106, 401–408. doi: 10.1007/s11240-011-9936-4
Verma, P., and Mathur, A. K. (2011b). Agrobacterium tumefaciens-mediated transgenic plant production via direct shoot bud organogenesis from pre-plasmolyzed leaf explants of Catharanthus roseus. Biotechnol. Lett. 33, 1053–1060. doi: 10.1007/s10529-010-0515-2
Verma, P., Sharma, A., Khan, S. A., Mathur, A. K., and Shanker, K. (2015). Morphogenetic and chemical stability of long-term maintained Agrobacterium-mediated transgenic Catharanthus oseus plants. Nat. Prod. Res. 29, 315–320. doi: 10.1080/14786419.2014.940348
Wang, Q., Xing, S., Pan, Q., Yuan, F., Zhao, J., Tian, Y., et al. (2012). Development of efficient Catharanthus roseus regeneration and transformation system using Agrobacterium tumefaciens and hypocotyls as explants. BMC Biotechnol. 12:34. doi: 10.1186/1472-6750-12-34
Wang, X., Pan, Y. J., Chang, B. W., Hu, Y. B., Guo, X. R., and Tang, Z. H. (2016). Ethylene-induced vinblastine accumulation is related to activated expression of downstream TIA pathway genes in Catharanthus roseus. Biomed. Res. Int. 2016:3708187. doi: 10.1155/2016/3708187
Wang, Y., van Kronenburg, B., Menzel, T., Maliepaard, C., Shen, X., and Krens, F. (2012). Regeneration and Agrobacterium-mediated transformation of multiple lily cultivars. Plant Cell Tissue Org. 111, 113–122. doi: 10.1007/s11240-012-0172-3
Weng, H., Pan, A., Yang, L., Zhang, C., Liu, Z., and Zhang, D. (2004). Estimating number of transgene copies in transgenic rapeseed by real-time PCR assay with HMG I/Y as an endogenous reference gene. Plant Mol. Biol. Rep. 22, 289–300. doi: 10.1007/BF02773139
Whitmer, S., Canel, C., Hallard, D., Gonçalves, C., and Verpoorte, R. (1998). Influence of precursor availability on alkaloid accumulation by transgenic cell line of Catharanthus roseus. Plant Physiol. 116, 853–857. doi: 10.1104/pp.116.2.853
Whitmer, S., Canel, C., van der Heijden, R., and Verpoorte, R. (2003). Long-term instability of alkaloid production by stably transformed cell lines of Catharanthus roseus. Plant Cell Tissue Org. 74, 73–80. doi: 10.1023/A:1023368309831
Yi, C. X., Zhang, J., Chan, K. M., Liu, X. K., and Hong, Y. (2008). Quantitative real-time PCR assay to detect transgene copy number in cotton (Gossypium hirsutum). Anal. Biochem. 375, 150–152. doi: 10.1016/j.ab.2007.11.022
Yin, J. L., Wong, W. S., Jang, I. C., and Chua, N. H. (2017). Co-expression of peppermint geranyl diphosphate synthase small subunit enhances monoterpene production in transgenic tobacco plants. New Phytol. 213, 1133–1144. doi: 10.1111/nph.14280
Zarate, R., and Verpoorte, R. (2007). Strategies for the genetic modification of the medicinal plant Catharanthus roseus (L.) G. Don. Phytochem. Rev. 6, 475–491. doi: 10.1007/s11101-006-9020-6
Keywords: Catharanthus roseus, geraniol synthase, geranyl(geranyl) diphosphate synthase, overexpression, monoterpene indole alkaloids, transgenic plant
Citation: Kumar SR, Shilpashree HB and Nagegowda DA (2018) Terpene Moiety Enhancement by Overexpression of Geranyl(geranyl) Diphosphate Synthase and Geraniol Synthase Elevates Monomeric and Dimeric Monoterpene Indole Alkaloids in Transgenic Catharanthus roseus. Front. Plant Sci. 9:942. doi: 10.3389/fpls.2018.00942
Received: 13 April 2018; Accepted: 12 June 2018;
Published: 06 July 2018.
Edited by:
Philipp Zerbe, University of California, Davis, United StatesReviewed by:
Carsten Kulheim, Australian National University, AustraliaHenrik Toft Simonsen, Technical University of Denmark, Denmark
Sibongile Mafu, University of Massachusetts Amherst, United States
Copyright © 2018 Kumar, Shilpashree and Nagegowda. This is an open-access article distributed under the terms of the Creative Commons Attribution License (CC BY). The use, distribution or reproduction in other forums is permitted, provided the original author(s) and the copyright owner(s) are credited and that the original publication in this journal is cited, in accordance with accepted academic practice. No use, distribution or reproduction is permitted which does not comply with these terms.
*Correspondence: Dinesh A. Nagegowda, ZGEubmFnZWdvd2RhQGNpbWFwLnJlcy5pbg==