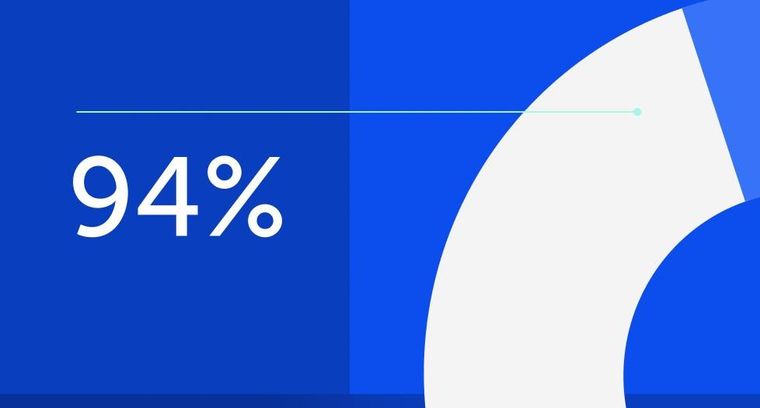
94% of researchers rate our articles as excellent or good
Learn more about the work of our research integrity team to safeguard the quality of each article we publish.
Find out more
REVIEW article
Front. Plant Sci., 02 July 2018
Sec. Plant Nutrition
Volume 9 - 2018 | https://doi.org/10.3389/fpls.2018.00937
This article is part of the Research TopicImproving the Nutritional Content and Quality of Crops: Promises, Achievements, and Future ChallengesView all 29 articles
Micronutrient deficiency, also known as “hidden hunger,” is an increasingly serious global challenge to humankind. Among the mineral elements, Fe (Iron) and Zn (Zinc) have earned recognition as micronutrients of outstanding and diverse biological relevance, as well as of clinical importance to global public health. The inherently low Fe and Zn content and poor bioavailability in cereal grains seems to be at the root of these mineral nutrient deficiencies, especially in the developing world where cereal-based diets are the most important sources of calories. The emerging physiological and molecular understanding of the uptake of Fe and Zn and their translocation in cereal grains regrettably also indicates accumulation of other toxic metals, with chemically similar properties, together with these mineral elements. This review article emphasizes breeding to develop bioavailable Fe- and Zn-efficient cereal cultivars to overcome malnutrition while minimizing the risks of toxic metals. We attempt to critically examine the genetic diversity regarding these nutritionally important traits as well as the progress in terms of quantitative genetics. We sought to integrate findings from the rhizosphere with Fe and Zn accumulation in grain, and to discuss the promoters as well as the anti-nutritional factors affecting Fe and Zn bioavailability in humans while restricting the content of toxic metals.
From the 1950s onwards, the advancement in science and technology together with concerted efforts of international and national agricultural organizations has resulted in significant gains in world food production widely referred to as the “Green Revolution” (Ortiz, 2011). Globally, the availability of sufficient quantities of food is not only a simple achievement of the Green Revolution but has also helped to avert large-scale famines and social and economic upheavals (Khush, 1999). Without the Green Revolution, crop yields in Asia and Latin America would be at least 20% less, food prices would be up 19%, calorie consumption would be down by about 5%, and the number of malnourished children would be up by at least 2% (Evenson and Gollin, 2003). The widespread adoption of these technologies has made it possible to improve the per capita calorie consumption in different continents, especially in the developing world. Overall, the Green Revolution has paid rich dividends in food grain production, particularly cereals, that have led to a significant reduction in the proportion of undernourished people worldwide; however, the problem of malnutrition or lack of quality food still persists, leading to an economic burden for society (Pingali, 2012).
Anemia is the most common human nutritional malaise, resulting from iron (Fe) deficiency and affecting 32.9% people worldwide; meanwhile, zinc (Zn) deficiency affects 17% of the world's population, with the highest risk occurring in sub-Saharan Africa and South Asia (Wessells et al., 2012; Kassebaum et al., 2014). In the twenty-first century, there are strong concerns worldwide regarding the ability to produce nutritionally rich food because cereals are inheritably poor in essential micronutrients. Moreover, owing to a burgeoning human population and industrialization, this situation may be further compounded by the production of cereals in areas with low mineral phytoavailability (White and Broadley, 2009). Thus, there is an urgent global need to cope with the problem of micronutrient deficiencies that contribute to what is referred to as “hidden hunger” and affect at least 2 billion people (or 1 out of 3), mostly in sub-Saharan Africa, South Asia, and Latin America (FAO et al, 2015).
Diversity in diets in order to provide adequate micronutrient consumption is difficult to achieve in the developing world where resource-poor people cannot afford a variety of different foods. For example, many of the relatively cheap and staple crops such as cereals (Table 1), roots [cassava (Manihot esculentaz)], tubers [sweet potato (Ipomoea batatas) and yam (Dioscorea spp.)], and plantain (Musa spp.) that play a very important role in the daily diets of resource-poor people lack high enough amounts of micronutrients (Gibson et al., 2010). Hence, malnutrition and poor health affect these people, who may suffer from blindness or stunting, and sometimes even face death. To overcome this “hidden hunger,” medical supplements and fortification have been pursued (Underwood, 2000). In fact, food fortification has a long history of use in industrialized countries and relies on the addition of micronutrients to processed foods. However, food fortification tends to have a rapid but less sustainable impact, because various safety, technological, and cost considerations may place constraints on such interventions (Allen et al., 2006). Furthermore, such interventions do not always reach the desired target populations (Pfeiffer and McClafferty, 2007). By increasing the micronutrient content of energy-rich crops, micronutrient intakes among the poor can be increased, thereby leading to decreases in the prevalence of micronutrient deficiencies.
Table 1. Information and assumptions used to set target levels for mineral nutrient content in grains of biofortified staple cereals by CGIAR HarvestPlus.
Biofortification is a strategy that involves the use of plant breeding or agronomic practices to increase the density of essential nutrients in the edible part of staple crops that may help to combat deficiencies among poor people who survive on main staples such as cereals (www.harvestplus.org). Agronomic biofortification is a fertilizer-based approach that relies on soil and/or foliar application of micronutrients either alone or in combination with other fertilizers. It is well-established that a Zn fertilizer strategy is an effective way to biofortify cereal crops with Zn, but recurrent cost is involved (Cakmak and Kutman, 2018). By contrast, genetic biofortification is a seed-based approach that complements agronomic biofortification and also current intervention methods such as supplementation and fortification of foods consumed daily. The aim of this strategy is to enhance the content and bioavailability of micronutrients such as minerals and vitamins in crops through plant breeding, thereby impacting favorably the diets of targeted populations, particularly the resource poor worldwide (Bouis and Welch, 2010). Because of its cost effectiveness, the 2008 Copenhagen Consensus ranked biofortification fifth for combating the world's greatest challenges (http://www.copenhagenconsensus.com/publication/second-copenhagen-consensus-biofortification-best-practice-meenakshi). Biofortifying staple crops through plant breeding is therefore a key option to improve micronutrient deficiency in human diets (Bouis and Saltzman, 2017).
Among the mineral nutrients required by humans for their well-being, Fe and Zn play vital roles in numerous metabolic processes and are required in trace amounts by plants as well as animals (Welch and Graham, 2004). For instance, Fe is a well-known essential component of hemoglobin and myoglobin, which are involved in oxygen transport and storage. The greatest effects of Fe deficiency anemia are seen in females during adolescence and pregnancy. Likewise, Fe deficiency affects children's cognitive development until adolescence, and it increases their susceptibility to infectious diseases and mortality (Oliver and Gregory, 2015).
Similarly, Zn is also an essential cofactor for many enzymes and regulatory proteins, and it plays an important role in DNA as well as RNA synthesis and gene expression. Children show stunted growth and neurobehavioral difficulties resulting from Zn deficiency, which may increase the incidence and severity of diarrhea, among other conditions (Nriagu, 2007). Furthermore, Zn deficiency seems to be significantly related to anemia associated with Fe deficiency because Zn controls Fe absorption in the intestines (Chang et al., 2010; Graham et al., 2012). Hence, Fe and Zn are acknowledged as outstanding micronutrients owing to their importance in global public health.
For genetic biofortification, a better understanding of the key steps of mineral nutrient transport from the rhizosphere to grains is needed, which involves coordination of complex physiological steps such as acquisition of Fe and Zn in roots (uptake), subsequent long-distance transport from roots to shoots, and further redistribution toward the developing seeds (Zhao and McGrath, 2009; Carvalho and Vasconcelos, 2013). Although Fe and Zn are known to accumulate in grains, further insights regarding the underpinning physiology and genetics are yet to be revealed. Soil redox potential and pH affect uptake of Fe by roots and Zn accumulation in grains. Fe is mostly available in the rhizosphere as low solubility Fe3+ oxyhydrates, while Fe is oxidized in aerobic soils with high pH, thus occurring as insoluble ferric oxides. Free ferric Fe from the oxides become available at low pH for further uptake by roots (Lindsay and Schwab, 1982).
Plants have developed different strategies for Fe uptake from the rhizosphere: Strategy I involving ferrous Fe2+ (non-Poaceae) and Strategy II utilizing ferric Fe3+ (Poaceae), referred to as reducing and chelating strategies, respectively, or a combination of strategies I and II (Connorton et al., 2017). Poaceae family members such as rice (Oryza sativa L.), maize (Zea mays L.), and wheat (Triticum aestivum L.) follow Strategy II, and their root epidermis secretes phytosiderophores (PSs) that form stable Fe(III) chelates in the rhizosphere (Roberts et al., 2004). TOM1 (Transporter of Mugineic acid family phytosiderophores)/ZIFL4 belongs to the major facilitator superfamily (MFS) that exports Fe3+-PS chelates in rice and barley (Nozoye et al., 2011). From the rhizosphere, these Fe(III)-PS complexes can be taken up into root cells by Yellow Stripe-Like proteins (YSLs). The maize oligopeptide transporter YS1 is the founding member of the YSL family, which facilitates Fe3+-PS complex uptake from the rhizosphere, and subsequently the role of YSL15 has also been confirmed in rice (Curie et al., 2001; Inoue et al., 2009). Besides Strategy II, both rice and barley have a functional homolog of IRT1 (Iron-Regulated Transporter 1) that allows direct uptake of Fe2+ from the rhizosphere, thus clearly showing the different uptake strategies for Fe2+ and Fe3+ in these cereal crops.
Soil pH significantly influences Zn acquisition and uptake from the rhizosphere by roots because Zn binds tightly to soil elements and plant cell wall parts under high pH. However, under anaerobic conditions in soils, additional factors such as soil redox potential, total sulfur content, and soluble bicarbonate also affect the availability of Zn (Impa and Johnson-Beebout, 2012). As is also the case for Fe(III), which exhibits even lower solubility, Zn solubilization in the rhizosphere is thought to occur via plant-mediated acidification and secretion of low molecular weight organic chelator (Sinclair and Krämer, 2012). Details regarding the role and contribution of Zn acquisition by the plant remain unknown. The uptake of Zn may occur as a divalent cation (Zn2+) or as a Zn-PS complex formed with PSs known as Fe3+-chelators, which are secreted by roots of the plant (von Wiren et al., 1996). ZIP-like transporters may take up Zn as noted in Strategy I plants (Ramesh et al., 2003). Thus, modification of rhizosphere chemistry through root architecture and by secretion of more root exudates that can alter soil pH could be the first promising target for improving Fe and Zn acquisition in cereal roots.
After roots acquire Fe and Zn, their translocation to the shoot and further movement to other vegetative organs depend of several steps, going through symplast, xylem, and phloem. Physiological studies have indicated that chelating molecules such as citrate, nicotianamine, and mugineic acid play a vital role in symplast heavy metal homoeostasis including Fe and Zn. Both minerals move through the xylem into the shoot, where Zn can move as free ions or in a complex with organic acids, while Fe is chelated to organic compounds of low molecular weight that are subsequently translocated by the xylem and phloem to other plant organs (Rellan-Alvarez et al., 2010; Lu et al., 2013). In plants, leaves are the most important sink tissue for these minerals where they are required in the plastids and mitochondria for numerous enzymes essential for photosynthesis and other cellular metabolic processes (Gupta et al., 2015). The FDR3 gene has an important role in transporting Fe (Green and Rogers, 2004); while for Zn transport, heavy metal ATPase (HMA), a member of the P1B ATPase family, is a likely candidate for performing this task (Eren and Arguello, 2004: Hussain et al., 2004). At the molecular level, a plethora of genes associated with influx and efflux transporters has been discovered and extensively characterized in plants, including cereals, and which are involved in translocation of these minerals (Kobayashi and Nishizawa, 2012; Ricachenevsky et al., 2015; Vasconcelos et al., 2017). Moreover, transcription factors have also been identified that regulate the genes involved in the uptake of Fe and Zn and synthesis of PSs in cereals. Despite the tremendous improvement in understanding those components participating in translocation, it remains difficult to define precisely the contribution of each of the components in the metal movement flux for each translocation step.
Plants remobilize and move nutrients from vegetative source organs into seeds during the filling of grains (Waters and Sankaran, 2011). Hence, the amount of both Fe and Zn in the cereal grain is dependent on the former physiological processes: firstly, their acquisition from the soil by roots, and secondly, transportation to the shoots and further remobilization of stored minerals from leaves when they senesce at grain filling. Despite the large amount of these minerals in the vegetative tissues of cereals, their remobilization from leaves is an important process (from senescence to grain filling), contributing to accumulation in the seeds. Fe and Zn accumulate throughout cereal seeds, being primarily concentrated in the aleurone and embryo parts and to lesser extent in the endosperm, except in rice and barley where Zn appears to be less strictly confined to the aleurone than Fe (Persson et al., 2009). From a biofortification perspective, the heterogeneous distribution of these essential mineral elements in cereal grains further complicates the situation for their efficient loading into the core endosperm (Cakmak et al., 2010). Fe, Zn, copper (Cu), and manganese (Mn) are micronutrients that primarily accumulate in the seed aleurone layer, where phytic acid (the main form of Pi storage in seeds) is a strong chelator of metal cations, binding them to form phytate, a salt of inositol phosphate (Raboy, 2009). However, recent studies have shown that Fe is mainly associated with phytic acid, while Zn is bound to proteins, which clearly suggests that Fe and Zn have a different speciation in cereal grain tissues (Persson et al., 2009; Kutman et al., 2010). Future research, hence, is needed to elucidate the molecular aspects of bivalent metal speciation including Fe and Zn in different tissues of seeds for efficient Fe and Zn biofortification strategies in cereals (Persson et al., 2016).
To address the widespread prevalence of micronutrient deficiency, especially pro-vitamin A, Fe, and Zn, the Consultative Group on International Agricultural Research (CGIAR; https://www.cgiar.org) HarvestPlus in collaboration with international and national research institutes emphasized biofortification of staple food crops as a cost-effective, easily applicable, and sustainable approach to benefit low-income households. This may complement other efforts aimed at reaching rural populations in developing countries (www.harvestplus.org). Considering the average content of Fe and Zn in cereal grains and their retention after processing, as well as addressing the issues related to the bioavailability of these mineral nutrients, HarvestPlus established target levels of these nutritionally important traits in cereal grains (Table 1). The initial screening of a large amount of crop germplasm suggested the existence of substantial genetic variation for these traits in cereal crops and their wild relatives. Besides the complex nature of these traits, the assays required to measure micronutrient content in plant samples are tedious and costly.
While plant breeding has been pursued significantly to achieve biofortification in staple crops, the success in breeding for Fe and Zn biofortification in cereal crops lags behind the development of pro-vitamin A enriched cultivars of staple crops (Andersson et al., 2017). The possible reason for this is a better understanding of the carotenoid biosynthesis pathway in plants, especially in maize, that has led to the deployment of functional markers for pro-vitamin A biofortification in maize (Gebremeskel et al., 2018). Although, there have been significant advances in elucidating the mechanisms related to Fe and Zn homeostasis in model plants, detailed understanding is still lacking. It is noteworthy to highlight that several genes controlling Fe and Zn homeostasis in cereal grains—particularly rice—have been characterized, but their role in genotypic variation for the accumulation of these minerals in the grain remains unclear. Hence, a more holistic breeding approach is required for Fe and Zn biofortification of cereal grains that emphasizes the genetic enhancement of the contents of these minerals in cereal grains together with the factors that determine their bioavailability in humans such as inhibitors and/or enhancers (Figure 1). Further, there is the need to be cautious regarding inadvertent enhancement of non-essential/certain toxic elements, such as cadmium (Cd), in cereal grains.
Figure 1. Schematic representation of breeding strategies using different genetic approaches for the development of grain Fe- and Zn-biofortified cereals.
A pre-requisite for breeding for a specific trait is the availability of its genetic variation within the target gene pool. The task is somewhat complex while breeding for Fe and Zn biofortification in cereal grains because their concentration in the grain depends on various physiological processes. Plant breeders rely on additive genetic effects, transgressive segregation, and heterosis for improving desired traits when enough genetic variation exists. Recently, the genetic variability for these minerals in cereals, particularly maize, rice, wheat, barley (Hordeum vulgare L.), sorghum (Sorghum bicolor L.), and pearl millet (Pennisetum glaucum L.), which are the six most important crops and represent 89% of all cereal production worldwide, was reviewed, and the existence of significant genetic differences for these minerals was reported (Teklic et al., 2013; Goudia and Hash, 2015; Gregory et al., 2017). A survey of 1,400 improved maize genotypes and 400 landraces maintained at the genebank of the International Maize and Wheat Improvement Center (CIMMYT, El Batan, México) indicated about four- to six-fold variation for grain Fe and Zn (Bänziger and Long, 2000). Among the tropical-adapted maize inbreds of the International Institute of Tropical Agriculture (IITA, Ibadan, Nigeria), the best inbreds exhibited 32 to 78% more grain Fe and 14 to 180% more grain Zn over their trial mean (Menkir, 2008). Similarly, several-fold variation for grain Fe and Zn in disomic hexaploid bread wheat has also been reported (Velu et al., 2014; Goudia and Hash, 2015). Correspondingly, substantial variation for these minerals in rice grain has also been reported among different cultivars; however, grain polishing removed up to 50% of the Fe from the brown rice grain (Gregorio et al., 2000; Prom-u-thai et al., 2007). About, two-fold higher Zn concentration but slightly lower Fe concentration was reported in indica rice compared with japonica rice (Yang et al., 1998). Significantly lower Fe and Zn contents were found in the seed of modern cultivars of rice than in landraces (Anandan et al., 2011), thus arguing that breeders failed in introducing quality improvement, particularly for micronutrients, because they gave priority to other traits such as size, shape, and appearance of grain, milling quality, and cooking features. However, a notable aspect of the lower content of these nutrients in the seed of modern cultivars compared with landraces/germplasm may be the yield dilution effect; the total grain nutrient content may not differ significantly between landraces and modern cultivars, and part of this effect could be ascribed to higher grain yield in modern cultivars (Pfeiffer and McClafferty, 2007; McDonald et al., 2008). Therefore, grain yield must be kept in mind when discussing breeding solutions in cereals biofortification.
Simple and reliable phenotyping is always preferred by breeders, but an extensive survey of the literature pertaining to the existence of genetic variability for grain Fe and Zn contents in cereal crops clearly suggests that accurate measurement of these mineral nutrients is a challenging task. For the measurement of Fe and Zn content in plants and related material, a wide range of analytical methods is available ranging from semi-quantitative [Perl's Prussian blue and diphenyl thiocarbazone-based dithizone] to fully quantitative [atomic absorption spectrometry, inductively coupled plasma-optical emission spectrometry (ICP-OES), ICP-mass spectrometry, near-infrared reflectance spectrophotometry, X-ray fluorescence spectrometry, elemental distribution maps secondary ion mass spectrometry, synchrotron X-ray, fluorescence spectroscopy, micro-X-ray fluorescence spectroscopy, and Laser-induced breakdown spectroscopy], which can differ substantially with respect to the many attributes describing method performance (Ihnat, 2003; Pfeiffer and McClafferty, 2007). Therefore, the choice of analytical method would depend on the purpose and precision required in estimation. Alternatively, non-destructive quantitative techniques could be the choice method from a breeding perspective, because initial screening for grain Fe and Zn content together with other mineral elements in an appropriately large number of breeding lines can be obtained with minimal or no sample preparation, thereby enabling the discarding of progenies with the lowest content of these mineral elements. There are numerous possibilities for introducing variation in the results of different studies. Firstly, sensitivity of the method used for the quantification of Fe and Zn contents. Secondly, improper postharvest handling of the samples has also been observed to give erroneous results while estimating grain micronutrient concentrations. Furthermore, it is noteworthy that the variability among microenvironments for Fe and Zn may be significant, and most of the research presenting extremely high or low values of these mineral nutrients is based on single-year data; thus, the results are affected by a significant confounded influence of the sampling and environment. Additionally, extremely high trial mean values of these nutrients reported in some studies appear to be affected by the prior use of manure at some locations, because the level of these nutrients in cereal grains, particularly Zn content, can be even lower when grown in infertile/Zn-deficient soils (Cakmak et al., 2010; Xu et al., 2011; Velu et al., 2014).
Modern cereal cultivars have a lower concentration of Fe and Zn in grains than landraces. This is because breeding has been mainly aimed at increasing grain yield or improving host plant resistance, among other target traits, instead of also improving the micronutrient concentration in grain. Utilization of landraces or crop wild relatives for genetic gain is not a new concept. The genetic variability for content of micronutrients is becoming acknowledged as a desired trait of crop wild relatives, particularly for rice and wheat.
The wild Triticum and Aegilops species have very high grain Fe and Zn contents when compared with both bread and durum wheat (Cakmak et al., 2000; Ortiz-Monasterio and Graham, 2000; Chhuneja et al., 2006; Rawat et al., 2009) as well as synthetic amphiploids (Calderini and Ortiz-Monasterio, 2003). Wild species such as Triticum boeoticum, Triticum monococcum, Triticum dicoccoides (wild emmer), Aegilops tauschii, and Aegilops speltoides were found to have substantially higher levels of these minerals (two- to three-fold) in their grain than modern wheat cultivars (Rawat et al., 2009; Xu et al., 2011; Velu et al., 2014). Compared with the alternative durum allele, recombinant chromosome substitution lines (RSLs) with T. dicoccoides carrying the Gpc-B1 allele had a 12, 18, and 38% higher concentration of Zn, Fe, and protein content, respectively (Cakmak et al., 2004). High concentrations of Fe, Mn, and Zn in grain were stable across sites (Distelfeld et al., 2007). Hence, T. dicoccoides seems to be an interesting source for enhancing both protein and essential mineral content and concentration in wheat cultigens. Similarly, wild accessions of rice such as Oryza rufipogon, Oryza nivara, Oryza latifolia, and Oryza officinalis seem to be assets in rice improvement, showing higher values for Fe and Zn content than cross-bred cultivars (Banerjee et al., 2010; Anuradha et al., 2012).
The finding of quantitative trait loci (QTLs) led to dissection of complex multigenic traits that were difficult to improve through crossbreeding before the progress made in DNA-aided analysis. QTL mapping for mineral nutrients in cereal grains has allowed the identification of many QTLs for both Fe and Zn (Table 2). Most of these QTLs, with a few exceptions, do not seem to be stable across sites. Furthermore, QTL mapping has also clearly indicated the role of epistasis in expression of these traits in cereal grains through interactions with other loci (Table 2).
Table 2. Main effect and epistatic quantitative trait loci (QTLs) associated with Fe and Zn accumulation in different tissues and their co-localization with other traits in cereal crops reported by different groups.
Unfortunately, there is no literature indicating so far a success story for marker-aided selection (MAS) for improving Fe and Zn in cereal grains, but some progress has been made that has laid the foundation stone toward breeding for Fe and Zn biofortification in cereals using MAS. For instance, some of the QTLs identified for Fe and Zn are co-localized, thereby suggesting common mechanisms for their transport. Furthermore, some QTLs for these mineral nutrients are also co-localized with those for other mineral elements such as phosphorus (P) and calcium (Ca), or other agronomically important traits including grain protein content and grain weight (Table 2). Fine mapping of candidate genes related to various QTLs could be a further step for developing biofortified germplasm.
Rice is a model plant for cereal genetics. Chromosome 11 of rice bears a QTL for Zn concentration in the grain, which seems to be associated with OsNAC5—a transcription factor that appears to be related with the remobilization of Zn from green tissues to the seed (Lu et al., 2008; Sperotto et al., 2009, 2010). In unpolished rice grains, 10 candidate genes known for Fe and Zn homeostasis were localized in the QTL regions whereas another six candidate genes were close to QTLs on chromosomes 3, 5, and 7, respectively (Anuradha et al., 2012). Based on these results, Anuradha et al. (2012) emphasized the importance of candidate genes OsYSL1 and OsMTP1 for Fe; OsARD2, OsIRT1, OsNAS1, and OsNAS2 for Zn; and OsNAS3, OsNRAMP1, heavy metal ion transport, and APRT for both Fe and Zn biofortification of grain in rice. Recently, Norton et al. (2014) also found several QTLs for grain Zn and other elements in diverse rice genotypes using genome-wide association mapping, but the known Zn-related genes were not found in these regions, thereby showing the novelty of their results.
The first QTL for grain Fe and Zn in wheat was found by Joppa et al. (1997), who mapped a major QTL (Gpc-B1) for grain protein content to chromosome 6BS in a population of recombinant inbred lines (RILs) that derived after crossing “Langdon” (LDN)—a durum wheat cultivar—and DIC6B—a chromosome substitution LDN line including wild emmer wheat. Subsequently, the Gpc-B1 locus was also found to be related to high concentrations of both Fe and Zn, as well as with fast leaf senescence. The dissection of the Gpc-B1 locus by positional cloning revealed that the gene underlying the Gpc-B1 locus encodes NAM1, which is a NAC transcription factor that belongs to a protein group that includes “No Apical Meristem” (NAM) in Arabidopsis thaliana (Uauy et al., 2006; Distelfeld et al., 2007). The ancestral wild wheat allele NAM-BI leads to fast senescence and enhances the remobilization of nutrients from the leaves to the developing grains. Modern wheat cultivars have instead a non-functional NAM-BI. Both Fe and Zn can be manipulated together because of the co-localization of their QTLs (Shi et al., 2008), whose mapping was facilitated by using RILs or diverse double-haploid (DH) populations (Table 2). The identification and tagging of DNA markers related to both traits provides an aid for crossbreeding, thereby accelerating biofortification for Fe and Zn in grains of cereals.
The ultimate goal of the breeding for Fe and Zn biofortification in cereals is to satisfy the requirement of the human body for these minerals. Thus, the bioavailability of these minerals should be measured according to the cereal-based foods consumed rather than as their quantity in the cereal grains. Considering the low bioavailability of these minerals, it seems to be difficult to meet this demand alone by enhancing the grain Fe and Zn content in cereals. Hence, Fe and Zn should be easily absorbable in the intestines—a difficult task due to inhibitors (e.g., phytic acid) or promoters such as prebiotics enhancing their absorption in the gut—to ensure their effective availability from cereal-based diets (Roberfroid, 2007; White and Broadley, 2009; Dwivedi et al., 2014).
Phytic acid is an effective chelator of positively charged elements such as Ca, Fe, Mn, magnesium (Mg), potassium (K), and Zn, which after human or animal consumption binds to these minerals in the intestines forming mixed salts that are further excreted, thus resulting in mineral deficiency in human populations (Ali et al., 2010). Although phytate is considered an inhibitor of Fe and Zn bioavailability and therefore referred to as an anti-nutritional trait in cereal grains, it may have some health benefits such as being an antioxidant or anticarcinogen (Schlemmer et al., 2009). Furthermore, the important role of phytic acid has also been noted in plant traits such as seedling vigor or protection of seeds against oxidative stress during their lifespan (Doria et al., 2009). Hence, the existence of a minimum concentration of phytic acid in the cereal grains is still under scientific debate from health as well as crop performance perspectives.
Nonetheless, various low-phytic acid (lpa) mutants have been found in barley, maize, rice, and wheat exhibiting 50 to 95% reduced phytic acid P (Rasmussen and Hatzack, 1998; Raboy et al., 2000; Pilu et al., 2003; Shi et al., 2003; Guttieri et al., 2004; Liu et al., 2007). However, the pleiotropic effects of these lpa mutations resulted in significant grain yield loss and also affected other agronomic traits such as poor seed germination along with low grain weight and starch accumulation, and poor plumpness, among other characteristics (Raboy et al., 2000; Pilu et al., 2003; Guttieri et al., 2006; Zhao et al., 2008). Thus, seeking available variability will assist in finding new genetic mechanisms that reduce phytate and avoid any grain yield penalty in cereals. About two-fold variation for seed phytate concentration has been observed in wheat and rice (Liu et al., 2006; Stangoulis et al., 2007). Interestingly, two QTLs for seed phytate concentration have been identified so far in rice; one each on chromosomes 5 and 12 accounting for phenotypic variance of 24 and 15%, respectively (Stangoulis et al., 2007). Genetic markers nearby these QTLs should be used for testing their efficacy as aids for selecting low-phytate lines.
With the growing awareness about diet-related health problems, the presence of health-promoting natural compounds in staple foods, which was earlier considered of minor importance, has attracted greater attention in the food industry. Prebiotics are a group of carbohydrates that are known to confer benefits for human health by selectively promoting the growth or activity of gut microbiota (Dwivedi et al., 2014). Thus, prebiotics in cereal grains should be taken into account for enhancing Fe and Zn bioavailability while undertaking biofortification. To date, scarce research has reported the influence of prebiotics on the absorption of these mineral nutrients in humans and the prevalence of the natural variation and inheritance of these compounds in cereal grains. There is, however, significant genetic variability for inulin concentration in the grains of maize and rice, both of which have lower inulin concentration that those of rye and wheat (Genc et al., 2005; Huynh et al., 2008a). Similarly, substantial genetic variation has also been reported in grain fructan content ranging from 0.7 to 2.9, 3.6 to 6.4, and 0.9 to 4.2% of grain dry weight in the different genotypic lines and cultivars of wheat, rye, and barley, respectively (Boskov-Hansen et al., 2003; Huynh et al., 2008a; Nemeth et al., 2014). There is a relatively high level of low molecular weight soluble dietary fiber in wheat. It includes fructan, which was found in a double mutant sweet wheat (SW) line; however, seeds were severely shrunken and shriveled, and had reduced kernel weight (Shimbata et al., 2011). Nevertheless, the SW mutant can be utilized in breeding programs as a novel source to raise grain fructan levels.
Among cereal crops, genetic mapping studies have been mainly performed in wheat for concentrations of grain prebiotics such as fructan, inulin, and arabinoxylan (Huynh et al., 2008b; Falcon, 2011; Nguyen et al., 2011). A total of five, four, and two QTLs explaining 2–27, 3–19, and 15–20% of phenotypic variation were detected in wheat for grain fructan, inulin, and arabinoxylan concentrations, respectively. Some epistatic QTLs were additionally detected for grain fructan and arabinoxylan concentration, although, their contributions were limited (Huynh et al., 2008b; Nguyen et al., 2011). Despite this, two QTLs each for fructan (6D and 7A), inulin (2BL.2 and 5BS), and arabinoxylan content (2A.1 and 4D.1) were major QTLs (PVE > 10%), suggesting molecular breeding to improve prebiotics significantly in grains of wheat. Recently, Huynh et al. (2012) mapped the fructan biosynthetic pathway gene coding for the enzyme sucrose:sucrose-1-fructosyltransferase (1-SST), which corresponds to the position of a major QTL on wheat chromosome 7A that affects the accumulation of grain fructan (Huynh et al., 2008b). Thus, identification of candidate genes underlying these QTLs would provide a basis for functional analysis and for the development of DNA markers that may assist molecular breeding with the aim of increasing prebiotic concentrations in the grain.
Besides food quality, food safety is also a “hot” topic that encourages scientists to engage in research related to health risks after consuming non-essential metals such as Cd and lead (Pb), and/or metalloids (arsenic, As), which have no beneficial role in plants, animals, or humans (Khan et al., 2015). Among these non-essential heavy metals, Cd particularly is known as highly phytotoxic, having a very low toxicity threshold level, and as a carcinogen, which is a great threat to human health. Nearly 27% of dietary Cd exposure is contributed by grain or grain products (Guttieri et al., 2015). Similarly, arsenic is also carcinogenic and can pose a serious threat to human health even at low concentrations. Moreover, the presence of high concentrations of these non-essential elements in cereal straw is still menacing because cereal straw is mainly used as livestock feed and thus these toxic elements may enter into the human food chain via contaminated meat or milk.
Soil is a natural source of heavy metals, and their elevated concentration in soil can occur either naturally or through anthropogenic activities such as urban and industrial activities as well as from agricultural practices. These toxic metals contamination is a non-reversible accumulation process due to their long estimated half-life in soil. Thus, accumulation of toxic metals in cereal grains impacts significantly on nutritional quality and crop safety. Generally, metals commonly enter plants as divalent cations. It has been reported that increasing accumulation of Fe and Zn in seeds leads to a higher accumulation of Cd, which chemically resembles Fe and Zn. Thus, uptake of Cd in roots and then translocation to seeds appears to occur inside plants along nutrient translocation pathways (Krämer, 2009). The first overlapping QTLs for essential and non-essential metals were identified in the Zn/Cd hyperaccumulator Arabidopsis halleri, and the candidate gene underlying the major QTL was identified as AbHMA4 (Heavy Metal ATPase 4) (Hanikenne et al., 2008). Subsequently, HMA2 was determined to contribute to Cd and Zn translocation in rice (Clemens et al., 2013).
The concentrations of essential mineral nutrients and non-essential metals in grains appear to be independently regulated because some independent grain Cd accumulation loci have been reported in cereals, such as the Cdu1 locus on 5BL in durum wheat (Knox et al., 2009) and one major QTL on 5AL in bread wheat (Guttieri et al., 2015). The identification of causal genes underlying these QTLs will provide more biological insights into Cd accumulation in cereal grains. Similarly, rice genotypes having dysfunctional OsNRAMP5 (Ishikawa et al., 2012) showed a substantial decrease in Cd uptake by roots, as well as Cd content in the straw and grain, but without decreasing the uptake of Fe by the roots, shoots, and straw (Ishimaru et al., 2012; Sasaki et al., 2012). These results suggest that a low grain Cd cereal cultivar can be developed without reducing the concentration of essential mineral nutrients through marker-aided breeding (MAB). Recent research has emphasized the importance of wild relatives for breeding high grain Fe and Zn in cereals crops. Nonetheless, possible pleiotropic effects of the introgression of elevated mineral nutrients need to be investigated by ICP–MS, thereby facilitating joint selection.
Globally, the committed efforts by CGIAR HarvestPlus have led to the integration of essential micronutrients as a core activity in the breeding programs of almost all major cereal crops. Considering the complex genetic mechanism of Fe and Zn accumulation in cereal grains, eradication of these mineral nutrient deficiencies by increasing their levels in cereal grains through conventional breeding is simply too difficult. In the post-genomic and computational systems biology era, the combination of high-throughput genomics and robust statistical analysis, particularly QTL mapping studies, has helped to dissect the molecular basis of natural diversity for complex quantitative traits in a better way. Recent molecular mapping studies clearly indicate the co-localization of QTLs for Fe and Zn with those for other potentially toxic metals such as Cd, Pb, and As. Available knowledge can be used to design targeted crosses for MAB targeting cereal cultivars with high levels of Fe and Zn.
Undoubtedly, QTLs detected only for Fe or Zn have also revealed that plants may be able to differentiate between nutrients and chemically similar toxin ions. Although no information is available so far about the enhancement of toxic metals in cereal grains through cross breeding, there is fear of inadvertent breeding for these non-essential metals that are toxic to both plants and animals even in low concentrations. Moreover, modifications in the accumulation of these toxic elements that are of concern for food safety are rarely determined during research on mineral nutrients. Thus, utilization of natural genetic variation for these mineral nutrients through a molecular breeding approach seems to be more attractive in the future. Furthermore, existence of substantial genetic variability for Fe and Zn bioavailability inhibitors and promoters also offers good opportunities to increase the bioavailable forms of these mineral nutrients in cereal grains. Genes accounting for this variability have rarely, however, been found and, therefore, are not yet being used in breeding; however, this also seems to be a promising approach for the near future. Hence, Fe and Zn bioavailability from cereal grains may be improved through breeding by accumulating either anti-nutrient agents or prebiotics. Furthermore, both functional and genetic evidence along with genome sequencing will provide means for gaining more insights regarding the emerging biofortification genomics.
AG-O conducted the literature survey and together with SC wrote the first draft. RO edited and together with AG-O, SC, MG, and AM improved the manuscript writing. All authors read and approved the final manuscript.
The authors declare that the research was conducted in the absence of any commercial or financial relationships that could be construed as a potential conflict of interest.
Fe, Iron; Zn, Zinc; CGIAR, Consultative Group on International Agricultural Research; QTL, Quantitative Trait Locus; MAS, Marker-aided Selection; MAB, Marker-aided Breeding; ICP-OES, Inductively Coupled Plasma-Optical Emission Spectrometry.
Ali, M., Shuja, M. N., Zahoor, M., and Qadri, I. (2010). Phytic acid: how far have we come?. Afr. J. Biotechnol. 9, 1551–1554 doi: 10.5897/AJB10.045
Allen, L. H., De Benoist, B., Dary, O., and Hurrell, R. (2006). Guidelines on Food Fortification with Micronutrients. Geneva: WHO and FAO, 1–341.
Anandan, A., Rajiv, G., Eswaran, R., and Prakash, M. (2011). Genotypic variation and relationships between quality traits and trace elements in traditional and improved rice (Oryza sativa L.) genotypes. J. Food Sci. 76, 122–130. doi: 10.1111/j.1750-3841.2011.02135.x
Andersson, M. S., Saltzman, A., Virk, P. S., and Pfeiffer, W. H. (2017). Progress update: crop development of biofortified staple food crops under HarvestPlus. Afr. J. Food Agric. Nutr. Dev. 17, 11905–11935. doi: 10.18697/ajfand.78.HarvestPlus05
Anuradha, K., Agarwal, S., Rao, Y. V., Rao, K. V., Viraktamath, B. C., and Sarla, N. (2012). Mapping QTLs and candidate genes for iron and zinc concentrations in unpolished rice of Madhukar × Swarna RILs. Gene 508, 233–240. doi: 10.1016/j.gene.2012.07.054
Bálint, A., Röder, M., Hell, R., Galiba, G., and Börner, A. (2007). Mapping of QTLs affecting copper tolerance and the Cu, Fe, Mn and Zn contents in the shoots of wheat seedlings. Biol. Plant. 51, 129–134 doi: 10.1007/s10535-007-0025-9
Banerjee, S., Sharma, D. J., Verulkar, S. B., and Chandel, G. (2010). Use of in silico and semiquantitative RT-PCR approaches to develop nutrient rich rice (Oryza sativa L.). Indian J. Biotechnol. 9, 203–212. Available online at: http://nopr.niscair.res.in/handle/123456789/7800
Bänziger, M., and Long, J. (2000). The potential for increasing the iron and zinc density of maize through plant-breeding. Food Nutr. Bull. 21, 397–400. doi: 10.1177/156482650002100410
Baxter, I. R., Gustin, J. L., Settles, A. M., and Hoekenga, O. A. (2013). Ionomic characterization of maize kernels in the intermated B73 × Mo17 population. Crop Sci. 53, 208–220. doi: 10.2135/cropsci2012.02.0135
Boskov-Hansen, H., Rasmussen, C. V., Bach-Knudsen, K. E., and Hansen, A. (2003). Effects of genotype and harvest year on content and composition of dietary fibre in rye (Secale cereale L.) grain. J. Sci. Food Agric. 83, 76–85. doi: 10.1002/jsfa.1284
Bouis, H. E., and Saltzman, A. (2017). Improving nutrition through biofortification: a review of evidence from HarvestPlus, 2003 through 2016. Glob. Food Sec. 12, 49–58. doi: 10.1016/j.gfs.2017.01.009
Bouis, H. E., and Welch, R. M. (2010). Biofortification-a sustainable agricultural strategy for reducing micronutrient malnutrition in the global south. Crop Sci. 50, S20–S32. doi: 10.2135/cropsci2009.09.0531
Cakmak, I., Kalayci, M, Kaya, Y., Torun, A. A., Aydin, N., Wang, Y., et al. (2010). Biofortification and localization of zinc in wheat grain. J. Agric. Food Chem. 58, 9092–9102. doi: 10.1021/jf101197h
Cakmak, I., and Kutman, U. B. (2018). Agronomic biofortification of cereals with zinc: a review. Eur. J. Soil Sci. 69, 172–180. doi: 10.1111/ejss.12437
Cakmak, I., Ozkan, H., Braun, H. J., Welch, R. M., and Romheld, V. (2000). Zinc and iron concentrations in seeds of wild, primitive, and modern wheats. Food Nutr. Bull. 21, 401–403. doi: 10.1177/156482650002100411
Cakmak, I., Torun, A., Millet, E., Feldman, M., Fahima, T., Korol, A., et al. (2004). Triticum dicoccoides: an important genetic resource for increasing zinc and iron concentration in modern cultivated wheat. Soil Sci. Plant Nutr. 50, 1047–1054. doi: 10.1080/00380768.2004.10408573
Calderini, D. F., and Ortiz-Monasterio, I. (2003). Are synthetic hexaploides a means of increasing grain element concentrations in wheat? Euphytica 134, 169–178. doi: 10.1023/B:EUPH.0000003849.10595.ac
Carvalho, S. M., and Vasconcelos, M. W. (2013). Producing more with less: strategies and novel technologies for plant-based food biofortification. Food Res. Int. 54, 961–971. doi: 10.1016/j.foodres.2012.12.021
Chang, S., El Arifeen, S., Bari, S., Wahed, M. A., Rahman, K. M., Rahman, M. T., et al. (2010). Supplementing iron and zinc: double blind, randomized evaluation of separate or combined delivery. Eur. J. Clin. Nutr. 64, 153–160. doi: 10.1038/ejcn.2009.127
Chhuneja, P., Dhaliwal, H. S., Bains, N. S., and Singh, K. (2006). Aegilops kotschyi and Ae. tauschii are the sources for high grain iron and zinc. Plant Breed. 125, 1–3. doi: 10.1111/j.1439-0523.2006.01223.x
Clemens, S., Aarts, M. G., Thomine, S., and Verbruggen, N. (2013). Plant science: the key to preventing slow cadmium poisoning. Trends Plant Sci. 18, 92–99. doi: 10.1016/j.tplants.2012.08.003
Connorton, J. M., Balk, J., and Rodríguez-Celma, J. (2017). Iron homeostasis in plants–a brief overview. Metallomics 9, 813–823. doi: 10.1039/c7mt00136c
Crespo-Herrera, L. A., Govindan, V., Stangoulis, J., Hao, Y., and Singh, R. P. (2017). QTL mapping of grain Zn and Fe concentrations in two hexaploid wheat RIL populations with ample transgressive segregation. Front. Plant Sci. 8:1800. doi: 10.3389/fpls.2017.01800
Crespo-Herrera, L. A., Velu, G., and Singh, R. P. (2016). Quantitative trait loci mapping reveals pleiotropic effect for grain iron and zinc concentrations in wheat. Ann. Appl. Biol. 169, 27–35. doi: 10.1111/aab.12276
Curie, C., Panaviene, Z., Loulergue, C., Dellaporta, S. L., Briat, J. F., and Walker, E. L. (2001). Maize yellow stripe1 encodes a membrane protein directly involved in Fe(III) uptake. Nature 409, 346–349. doi: 10.1038/35053080
Distelfeld, A., Cakmak, I., Peleg, Z., Ozturk, L., Yazici, A. M., Budak, H., et al. (2007). Multiple QTL- effects of wheat Gpc-B1 locus on grain protein and micronutrient concentrations. Physiol. Plant. 129, 635–643. doi: 10.1111/j.1399-3054.2006.00841.x
Doria, E., Galleschi, L., Calucci, L., Pinzino, C., Pilu, R., Cassani, E., et al. (2009). Phytic acid prevents oxidative stress in seeds: evidence from a maize (Zea mays L.) low phytic acid mutant. J. Exp. Bot. 60, 967–978. doi: 10.1093/jxb/ern345
Dwivedi, S., Sahrawat, K., Puppala, N., and Ortiz, R. (2014). Plant prebiotics and human health: biotechnology to breed prebiotic-rich nutritious food crops. Electron. J. Biotechnol. 17, 238–245. doi: 10.1016/j.ejbt.2014.07.004
Eren, E., and Arguello, J. M. (2004). Arabidopsis HMA2, a divalent heavy metal-transporting P IB-type ATPase, is involved in cytoplasmic Zn2+ homeostasis. Plant Physiol. 136, 3712–3723. doi: 10.1104/pp.104.046292
Evenson, R. E., and Gollin, D. (2003). Assessing the impact of the Green Revolution, 1960 to 2000. Science 300, 758–762. doi: 10.1126/science.1078710
Falcon, C. M. (2011). Mapping the Genes Controlling Inulin Content in Wheat. Honors Thesis, Cornell University, Ithaca, NY, 1–27.
FAO, IFAD, and W. F. P. (2015). The State of Food Insecurity in the World 2015. Food and Agriculture Organization of the United Nations, Rome.
Garcia-Oliveira, A. L., Tan, L., Fu, Y., and Sun, C. (2009). Genetic identification of quantitative trait loci for contents of mineral nutrients in rice grain. J. Integr. Plant Biol. 51, 84–92. doi: 10.1111/j.1744-7909.2008.00730.x
Gebremeskel, S., Garcia-Oliveira, A. L., Menkir, A., Adetimirin, V., and Gedil, M. (2018). Effectiveness of predictive markers for marker assisted selection of pro-vitamin A carotenoids in medium-late maturing maize (Zea mays L.) inbred lines. J. Cereal Sci. 79, 27–34. doi: 10.1016/j.jcs.2017.09.001
Genc, Y., Humphries, J. M., Lyons, G. H., and Graham, R. D. (2005). Exploiting genotypic variation in plant nutrient accumulation to alleviate micronutrient deficiency in populations. J. Trace Elem. Med. Biol. 18, 319–324. doi: 10.1016/j.jtemb.2005.02.005
Genc, Y., Verbyla, A. P., Torun, A. A., Cakmak, I., Willsmore, K., Wallwork, H., et al. (2009). Quantitative trait loci analysis of zinc efficiency and grain zinc concentration in wheat using whole genome average interval mapping. Plant Soil 314, 49–66. doi: 10.1007/s11104-008-9704-3
Gibson, R. S., Bailey, K. B., Gibbs, M., and Ferguson, E. L. (2010). A review of phytate, iron, zinc, and calcium concentrations in plant-based complementary foods used in low-income countries and implications for bioavailability. Food Nutr. Bull. 31, S134–S146. doi: 10.1177/15648265100312S206
Goudia, B. D., and Hash, C. T. (2015). Breeding for high grain Fe and Zn levels in cereals. Int. J. Innov. Appl. Stud. 12, 342–354.
Graham, R. D., Knez, M., and Welch, R. M. (2012). How much nutritional iron deficiency in humans globally is due to an underlying zinc deficiency? Adv. Agron. 115, 1–40. doi: 10.1016/B978-0-12-394276-0.00001-9
Green, L., and Rogers, E. E. (2004). FRD3 controls iron localization in Arabidopsis. Plant Physiol. 136, 2523–2531. doi: 10.1104/pp.104.045633
Gregorio, G. B., Senadhira, D., Htut, H., and Graham, R. D. (2000). Breeding for trace mineral density in rice. Food Nutr. Bull. 21, 382–386. doi: 10.1177/156482650002100407
Gregory, P. J., Wahbi, A., Adu-Gyamfi, J., Heiling, M., Gruber, R., Joy, E. J., et al. (2017). Approaches to reduce zinc and iron deficits in food systems. Glob. Food Sec. 15, 1–10. doi: 10.1016/j.gfs.2017.03.003
Gupta, H. S., Hossain, F., Nepolean, T., Vignesh, M., and Mallikarjuna, M. G. (2015). “Understanding genetic and molecular bases of Fe and Zn accumulation towards development of micronutrient-enriched maize,” in Nutrient Use Efficiency: from Basics to Advances, eds A. Rakshit, H. B. Singh, and A. Sen (Springer), 255–282. doi: 10.1007/978-81-322-2169-2-17
Guttieri, M. J., Baenziger, P. S., Frels, K., Carver, B., Arnall, B., Wang, S., et al. (2015). Prospects for selecting wheat with increased zinc and decreased cadmium concentration in grain. Crop Sci. 55, 1712–1728. doi: 10.2135/cropsci2014.08.0559
Guttieri, M. J., Bowen, D., Dorsch, J. A., Raboy, V., and Souza, E. (2004). Identification and characterization of a low phytic acid wheat. Crop Sci. 44, 418–424. doi: 10.2135/cropsci2004.4180
Guttieri, M. J., Peterson, K. M., and Souza, E. J. (2006). Agronomic performance of low phytic acid wheat. Crop Sci. 46, 2623–2629. doi: 10.2135/cropsci2006.01.0008
Hanikenne, M., Talke, I. N., Haydon, M. J., Lanz, C., Nolte, A., Motte, P., et al. (2008). Evolution of metal hyperaccumulation required cis-regulatory changes and triplication of HMA4. Nature 453, 391–395. doi: 10.1038/nature06877
Hao, Y., Velu, G., Peña, R. J., Singh, S., and Singh, R. P. (2014). Genetic loci associated with high grain zinc concentration and pleiotropic effect on kernel weight in wheat (Triticum aestivum L.). Mol. Breed. 34, 1893–1902. doi: 10.1007/s11032-014-0147-7
Hussain, D., Haydon, M. J., Wang, Y., Wong, E., Sherson, S. M., Young, J., et al. (2004). P-type ATPase heavy metal transporters with roles in essential zinc homeostasis in Arabidopsis. Plant Cell 6, 1327–1339. doi: 10.1105/tpc.020487
Huynh, B. L., Mather, D. E., Schreiber, A. W., Toubia, J., Baumann, U., Shoaei, Z., et al. (2012). Clusters of genes encoding fructan biosynthesizing enzymes in wheat and barley. Plant Mol. Biol. 80, 299–314. doi: 10.1007/s11103-012-9949-3
Huynh, B. L., Palmer, L., Mather, D. E., Wallwork, H., Graham, R. D., Welch, R. M., et al. (2008a). Genotypic variation in wheat grain fructan content revealed by a simplified HPLC method. J. Cereal Sci. 48, 369–378. doi: 10.1016/j.jcs.2007.10.004
Huynh, B. L., Wallwork, H., Stangoulis, J. C., Graham, R. D., Willsmore, K. L., Olson, S., et al. (2008b). Quantitative trait loci for grain fructan concentration in wheat (Triticum aestivum L.). Theor. Appl. Genet. 117, 701–709. doi: 10.1007/s00122-008-0811-6
Ihnat, M. (2003). A survey of methods of analysis for minerals in feedstuffs 1, 2. J. Anim. Sci. 81, 3218–3225. doi: 10.2527/2003.81123218x
Impa, S. M., and Johnson-Beebout, S. E. (2012). Mitigating zinc deficiency and achieving high grain Zn in rice through integration of soil chemistry and plant physiology research. Plant Soil 361, 3–41. doi: 10.1007/s11104-012-1315-3
Inoue, H., Kobayashi, T., Nozoye, T., Takahashi, M., Kakei, Y., Suzuki, K., et al. (2009). Rice OsYSL15 is an iron-regulated iron(III)-deoxymugineic acid transporter expressed in the roots and is essential for iron uptake in early growth of the seedlings. J. Biol. Chem. 284, 3470–3479. doi: 10.1074/jbc.M806042200
Ishikawa, S., Ishimaru, Y., Igura, M., Kuramata, M., Abe, T., Senoura, T., et al. (2012). Ion-beam irradiation, gene identification, and marker-assisted breeding in the development of low-cadmium rice. Proc. Natl. Acad. Sci. U.S.A. 109, 19166−19171. doi: 10.1073/pnas.1211132109
Ishimaru, Y., Takahashi, R., Bashir, K., Shimo, H., Senoura, T., Sugimoto, K., et al. (2012). Characterizing the role of rice NRAMP5 in manganese, iron and cadmium transport. Sci. Rep. 2:286. doi: 10.1038/srep00286
Jin, T., Zhou, J., Chen, J., Zhu, L., Zhao, Y., and Huang, Y. (2013). The genetic architecture of zinc and iron content in maize grains as revealed by QTL mapping and meta-analysis. Breed. Sci. 63, 317–324. doi: 10.1270/jsbbs.63.317
Joppa, L. R., Du, C., Hart, G. E., and Hareland, G. A. (1997). Mapping gene (s) for grain protein in tetraploid wheat (Triticum turgidum L.) using a population of recombinant inbred chromosome lines. Crop Sci. 37, 1586–1589.
Kassebaum, N. J., Jasrasaria, R., Naghavi, M., Wulf, S. K., Johns, N., Lozano, R., et al. (2014). A systematic analysis of global anemia burden from 1990 to 2010. Blood 123, 615–624. doi: 10.1182/blood-2013-06-508325
Khan, A., Khan, S., Khan, M. A., Qamar, Z., and Waqas, M. (2015). The uptake and bioaccumulation of heavy metals by food plants, their effects on plants nutrients, and associated health risk: a review. Environ. Sci. Pollut. Res. 22, 13772–13799. doi: 10.1007/s11356-015-4881-0
Knox, R. E., Pozniak, C. J., Clarke, F. R., Clarke, J. M., Houshmand, S., and Singh, A. K. (2009). Chromosomal location of the cadmium uptake gene (Cdu1) in durum wheat. Genome 52, 741–747. doi: 10.1139/G09-042
Kobayashi, T., and Nishizawa, N. K. (2012). Iron uptake, translocation, and regulation in higher plants. Annu. Rev. Plant Biol. 63, 131–152. doi: 10.1146/annurev-arplant-042811-105522
Krämer, U. (2009). The dilemma of controlling heavy metal accumulation in plants. New Phytol. 181, 3–5. doi: 10.1111/j.1469-8137.2008.02699.x
Kumar, J., Jain, S., and Jain, R. K. (2014). Linkage mapping for grain iron and zinc content in F2 population derived from the cross between PAU201 and Palman 579 in rice (Oryza sativa L.). Cereal Res. Commun. 42, 389–400. doi: 10.1556/CRC.42.2014.3.3
Kumar, S., Hash, C, T., Thirunavukkarasu, N., Singh, G., Rajaram, V., Rathore, A., et al. (2016). Mapping quantitative trait loci controlling high iron and zinc content in self and open pollinated grains of pearl millet [Pennisetum glaucum (L.) R. Br.]. Front. Plant Sci. 7:1636. doi: 10.3389/fpls.2016.01636
Kutman, U. B., Yildiz, B., Ozturk, L., and Cakmak, I. (2010). Biofortification of durum wheat with zinc through soil and foliar applications of nitrogen. Cereal Chem. 87, 1–9. doi: 10.1094/CCHEM-87-1-0001
Lindsay, W. L., and Schwab, A. P. (1982). The chemistry of iron in soils and its availability to plants. J. Plant Nutr. 5, 821–840. doi: 10.1080/01904168209363012
Liu, Q. L., Xu, X. H., Ren, X. L., Fu, H. W., Wu, D. X., and Shu, Q. Y. (2007). Generation and characterization of low phytic acid germplasm in rice (Oryza sativa L.) Theor. Appl. Genet. 114, 803–814. doi: 10.1007/s00122-006-0478-9
Liu, Z. H., Wang, H. Y., Wang, X. E., Zhang, G. P., Chen, P. D., and Liu, D. J. (2006). Genotypic and spike positional difference in grain phytase activity, phytate, inorganic phosphorus, iron, and zinc contents in wheat (Triticum aestivum L.). J. Cereal Sci. 44, 212–219. doi: 10.1016/j.jcs.2006.06.001
Lonergan, P. F., Pallotta, M. A., Lorimer, M., Paull, J. G., Barker, S. J., and Graham, R. D. (2009). Multiple genetic loci for zinc uptake and distribution in barley (Hordeum vulgare). New Phytol. 184, 168–179. doi: 10.1111/j.1469-8137.2009.02956.x
Lu, K., Li, L., Zheng, X., Zhang, Z., Mou, T., and Hu, Z. (2008). Quantitative trait loci controlling Cu, Ca, Zn, Mn and Fe content in rice grains. J. Genet. 87, 305–310. doi: 10.1007/s12041-008-0049-8
Lu, L., Tian, S., Zhang, J., Yang, X., Labavitch, J. M., Webb, S. M., et al. (2013). Efficient xylem transport and phloem remobilization of Zn in the hyperaccumulator plant species Sedum alfredii. New Phytol. 198, 721–731. doi: 10.1111/nph.12168
Lung'aho, M. G., Mwaniki, A. M., Szalma, S. J., Hart, J. J., Rutzke, M. A., Kochian, L. V., et al. (2011). Genetic and physiological analysis of iron biofortification in maize kernels. PLoS ONE 6:e20429. doi: 10.1371/journal.pone.0020429
McDonald, G. K., Genc, Y., and Graham, R. D. (2008). A simple method to evaluate genetic variation in grain zinc concentration by correcting for differences in grain yield. Plant Soil 306, 49–55. doi: 10.1007/s11104-008-9555-y
Menkir, A. (2008). Genetic variation for grain mineral content in tropical-adapted maize inbred lines. Food Chem. 110, 454–464. doi: 10.1016/j.foodchem.2008.02.025
Nemeth, C., Andersson, A. A. M., Andersson, R., Mangelsen, E., Sun, C., and Aman, P. (2014). Relationship of grain fructan content to degree of polymerization in different barleys. Food Nutr. Sci. 5, 581–589. doi: 10.4236/fns.2014.56068
Nguyen, V. L., Huynh, B. L., Wallwork, H., and Stangoulis, J. (2011). Identification of quantitative trait loci for grain arabinoxylan concentration in bread wheat. Crop Sci. 51, 1143–1150. doi: 10.2135/cropsci2010.08.0484
Norton, G. J., Deacon, C. M., Xiong, L., Huang, S., Meharg, A. A., and Price, A. H. (2010). Genetic mapping of the rice ionome in leaves and grain: identification of QTLs for 17 elements including arsenic, cadmium, iron and selenium. Plant Soil 329, 139–153. doi: 10.1007/s11104-009-0141-8
Norton, G. J., Douglas, A., Lahner, B., Yakubova, E., Guerinot, M. L., Pinson, S. R., et al. (2014). Genome wide association mapping of grain arsenic, copper, molybdenum and zinc in rice (Oryza sativa L.) grown at four international field sites. PLoS ONE 9:e89685. doi: 10.1371/journal.pone.0089685
Nozoye, T., Nagasaka, S., Kobayashi, T., Takahashi, M., Sato, Y., Sato, Y., et al. (2011). Phytosiderophore efflux transporters are crucial for iron acquisition in graminaceous plants. J. Biol. Chem. 286, 5446–5454. doi: 10.1074/jbc.M110.180026
Nriagu, J. (2007). “Zinc deficiency in human health,” in Encyclopedia of Environmental Health (Amstedam: Elsevier). Available online at: https://pdfs.semanticscholar.org/8d01/a12a1abbffae431db613b7f4e12d575258a8.pdf
Oliver, M. A., and Gregory, P. J. (2015). Soil, food security and human health: a review. Eur. J. Soil Sci. 66, 257–276. doi: 10.1111/ejss.12216
Ortiz, R. (2011). Re-visiting the green revolution. Chron. Horticult. 51, 6–11. doi: 10.1016/j.scienta.2011.09.020
Ortiz-Monasterio, I., and Graham, R. D. (2000). Breeding for trace minerals in wheat. Food Nutr. Bull. 21, 392–396. doi: 10.1177/156482650002100409
Peleg, Z., Cakmak, I., Ozturk, L., Yazici, A., Jun, Y., Budak, H., et al. (2009). Quantitative trait loci conferring grain mineral nutrient concentrations in durum wheat × wild emmer wheat RIL population. Theor. Appl. Genet. 119, 353–369. doi: 10.1007/s00122-009-1044-z
Persson, D. P., de Bang, T. C., Pedas, P. R., Kutman, U. B., Cakmak, I., Andersen,., B., et al. (2016). Molecular speciation and tissue compartmentation of zinc in durum wheat grains with contrasting nutritional status. New Phytol. 211, 1255–1265. doi: 10.1111/nph.13989
Persson, D. P., Hansen, T. H., Laursen, K. H., Schjoerring, J. K., and Husted, S. (2009). Simultaneous iron, zinc, sulfur and phosphorus speciation analysis of barley grain tissues using SEC-ICP-MS and IP-ICP-MS. Metallomics 1, 418–426. doi: 10.1039/b905688b
Pfeiffer, W. H., and McClafferty, B. (2007). HarvestPlus: breeding crops for better nutrition. Crop Sci. 50, S88–S105. doi: 10.2135/cropsci2007.09.0020IPBS
Pilu, R., Panzeri, D., Gavazzi, G., Rasmussen, S. K., Consonni, G., and Nielsen, E. (2003). Phenotypic, genetic and molecular characterization of a maize low phytic-acid mutant (lpa241). Theor. Appl. Genet. 107, 980–987. doi: 10.1007/s00122-003-1316-y
Pingali, P. L. (2012). Green Revolution: Impacts, limits, and the path ahead. Proc. Natl. Acad. Sci. U.S.A. 109, 12302–12308. doi: 10.1073/pnas.0912953109
Prom-u-thai, C., Fukai, S., Godwin, I. D., and Huang, L. (2007). Genotypic variation of iron partitioning in rice grain. J. Sci. Food Agric. 87, 2049–2054. doi: 10.1002/jsfa.2961
Pu, Z. E., Ma, Y., He, Q. Y., Chen, G. Y., Wang, J. R., Liu, Y. X., et al. (2014). Quantitative trait loci associated with micronutrient concentrations in two recombinant inbred wheat lines. J. Integr. Agric. 13, 2322–2329. doi: 10.1016/S2095-3119(13)60640-1
Qin, H., Cai, Y., Liu, Z., Wang, G., Wang, J., Guo, Y., et al. (2012). Identification of QTL for zinc and iron concentration in maize kernel and cob. Euphytica 187, 345–358. doi: 10.1007/s10681-012-0692-2
Raboy, V. (2009). Approaches and challenges to engineering seed phytate and total phosphorus. Plant Sci. 177, 281–296. doi: 10.1016/j.plantsci.2009.06.012
Raboy, V., Gerbasi, P. F., Young, K. A., Stoneberg, S. D., Pickett, S. G., Bauman, A. T., et al. (2000). Origin and seed phenotype of maize low-phytic acid 1-1 and low-phytic acid 2-1. Plant Physiol. 124, 355–368. doi: 10.1104/pp.124.1.355
Ramesh, S. A., Shin, R., Eide, D. J., and Schachtman, D. P. (2003). Differential metal selectivity and gene expression of two zinc transporters from rice. Plant Physiol. 133, 126–134. doi: 10.1104/pp.103.026815
Rasmussen, S. K., and Hatzack, F. (1998). Identification of two low-phytate barley (Hordeum vulgare L.) grain mutants by TLC and genetic analysis. Hereditas 129, 107–112. doi: 10.1111/j.1601-5223.1998.00107.x
Rawat, N., Tiwari, V. K., Singh, N., Randhawa, G. S., Singh, K., Chhuneja, P., et al. (2009). Evaluation and utilization of Aegilops and wild Triticum species for enhancing iron and zinc content in wheat. Genet. Resour. Crop Evol. 56, 53–64. doi: 10.1007/s10722-008-9344-8
Rellan-Alvarez, R., Stastion, A. D. E., Giner-Martinez Sierra, J., Orduna, J., Orera, I., Rodriguez-Castrillon, J. A., et al. (2010). Identification of a tri-iron (III), tri-citrate complex in the xylem sap of iron-deficient tomato [Lycopersicon esculentum] resupplied with iron: new insights into plant iron long-distance transport. Plant Cell Physiol. 51, 91–102. doi: 10.1093/pcp/pcp170
Reuscher, S., Kolter, A., Hoffmann, A., Pillen, K., and Krämer, U. (2016). Quantitative trait loci and inter-organ partitioning for essential metal and toxic analogue accumulation in barley. PLoS ONE 11:e0153392. doi: 10.1371/journal.pone.0153392
Ricachenevsky, F. K., Menguer, P. K., Sperotto, R. A., and Fett, J. P. (2015). Got to hide your Zn away: molecular control of Zn accumulation and biotechnological applications. Plant Sci. 236, 1–17. doi: 10.1016/j.plantsci.2015.03.009
Roberfroid, M. (2007). Prebiotics: the concept revisited. J. Nutr. 137, 830S−837S. doi: 10.1093/jn/137.3.830S
Roberts, L. A., Pierson, A. J., Panaviene, Z., and Walker, E. L. (2004). Yellow stripe1. Expanded roles for the maize iron- phytosiderophore transporter. Plant Physiol. 135, 112–120. doi: 10.1104/pp.103.037572
Roshanzamir, H., Kordenaeej, A., and Bostani, A. (2013). Mapping QTLs related to Zn and Fe concentrations in bread wheat (Triticum aestivum) grain using microsatellite markers. Iran. J. Genet. Plant Breed. 2, 10–17.
Sasaki, A., Yamaji, N., Yokosho, K., and Ma, J. F. (2012). Nramp5 is a major transporter responsible for manganese and cadmium uptake in rice. Plant Cell 24, 2155–2167. doi: 10.1105/tpc.112.096925
Schlemmer, U., Frølich, W., Prieto, R. M., and Grases, F. (2009). Phytate in foods and significance for humans: food sources, intake, processing, bioavailability, protective role and analysis. Mol. Nutr. Food Res. 53, S330–S375. doi: 10.1002/mnfr.200900099
Shi, J., Wang, H., Wu, Y., Hazebroek, J., Meeley, R. B., and Ertl, D. S. (2003). The maize low-phytic acid mutant lpa2 is caused by mutation in an inositol phosphate kinase gene. Plant Physiol. 131, 507–515. doi: 10.1104/pp.014258
Shi, R., Li, H., Tong, Y., Jing, R., Zhang, F., and Zou, C. (2008). Identification of quantitative trait locus of zinc and phosphorus density in wheat (Triticum aestivum L.) grain. Plant Soil 306, 95–104. doi: 10.1007/s11104-007-9483-2
Shimbata, T., Inokuma, T., Sunohara, A., Vrinten, P., Saito, M., Takiya, T., et al. (2011). High levels of sugars and fructan in mature seed of sweet wheat lacking GBSSI and SSIIa enzymes. J. Agric. Food Chem. 59, 4794–4800. doi: 10.1021/jf200468c
Šimić, D., Mladenović Drinić, S., Zdunić, Z., Jambrović, A., Ledenčan, T., Brkić, J., et al. (2011). Quantitative trait loci for biofortification traits in maize grain. J. Hered. 103, 47–54. doi: 10.1093/jhered/esr122
Sinclair, S. A., and Krämer, U. (2012). The zinc homeostasis network of land plants. Biochim. Biophys. Acta 1823, 1553–1567. doi: 10.1016/j.bbamcr.2012.05.016
Sorić, R., Ledenčan, T., Zdunić, Z., Jambrović, A., Brkić, I., Lončarić, Z., et al. (2012). Quantitative trait loci for metal accumulation in maize leaf. Maydica 56, 323–329.
Sperotto, R. A., Boff, T., Duarte, G. L., Santos, L. S., Grusak, M. A., and Fett, J. P. (2010). Identification of putative target genes to manipulate Fe and Zn concentrations in rice grains. J. Plant Physiol. 167, 1500–1506. doi: 10.1016/j.jplph.2010.05.003
Sperotto, R. A., Ricachenevsky, F. K., Duarte, G. L., Boff, T., Lopes, K. L., Sperb, E. R., et al. (2009). Identification of up-regulated genes in flag leaves during rice grain filling and characterization of OsNAC5, a new ABA-dependent transcription factor. Planta 230, 985–1002. doi: 10.1007/s00425-009-1000-9
Srinivasa, J., Arun, B., Mishra, V. K., Singh, G. P., Velu, G., Babu, R., et al. (2014). Zinc and iron concentration QTL mapped in a Triticum spelta × T. aestivum cross. Theor. Appl. Genet. 127, 1643–1651. doi: 10.1007/s00122-014-2327-6
Stangoulis, J. C., Huynh, B. L., Welch, R. M., Choi, E. Y., and Graham, R. D. (2007). Quantitative trait loci for phytate in rice grain and their relationship with grain micronutrient content. Euphytica 154, 289–294. doi: 10.1007/s10681-006-9211-7
Teklic, T., Lončarić, Z., Kovačević, V., and Singh, B. R. (2013). Metallic trace elements in cereal grain–a review: how much metal do we eat? Food Energy Sec. 2, 81–95. doi: 10.1002/fes3.24
Tiwari, V. K., Rawat, N., Chhuneja, P., Neelam, K., Aggarwal, R., Randhawa, G. S., et al. (2009). Mapping of quantitative trait loci for grain iron and zinc concentration in diploid A genome wheat. J. Hered. 100, 771–776. doi: 10.1093/jhered/esp030
Uauy, C., Distelfeld, A., Fahima, T., Blechl, A., and Dubcovsky, J. (2006). A NAC gene regulating senescence improves grain protein, zinc, and iron content in wheat. Science 314, 1298–1301. doi: 10.1126/science.1133649
Underwood, B. A. (2000). Overcoming micronutrient deficiency in developing countries: is there a role for agriculture? Food Nutr. Bull. 21, 356–360. doi: 10.1177/156482650002100403
Vasconcelos, M. W., Gruissem, W., and Bhullar, N. K. (2017). Iron biofortification in the 21st century: setting realistic targets, overcoming obstacles, and new strategies for healthy nutrition. Curr. Opin. Biotechnol. 44, 8–15. doi: 10.1016/j.copbio.2016.10.001
Velu, G., Ortiz-Monasterio, I., Cakmak, I., Hao, Y., and Singh, R. P. (2014). Biofortification strategies to increase grain zinc and iron concentrations in wheat. J. Cereal Sci. 59, 365–372. doi: 10.1016/j.jcs.2013.09.001
Velu, G., Tutus, Y., Gomez-Becerra, H. F., Hao, Y., Demir, L., Kara, R., et al. (2017). QTL mapping for grain zinc and iron concentrations and zinc efficiency in a tetraploid and hexaploid wheat mapping populations. Plant Soil 411, 81–99. doi: 10.1007/s11104-016-3025-8
von Wiren, N., Marschner, H., and Romheld, V. (1996). Roots of iron-efficient maize also absorb phytosiderophore-chelated zinc. Plant Physiol. 111, 1119–1125. doi: 10.1104/pp.111.4.1119
Waters, B. M., and Sankaran, R. P. (2011). Moving micronutrients from the soil to the seeds: genes and physiological processes from a biofortification perspective. Plant Sci. 180, 562–574. doi: 10.1016/j.plantsci.2010.12.003
Welch, R. M., and Graham, R. D. (2004). Breeding for micronutrients in staple food crops from a human nutrition perspective. J. Exp. Bot. 55, 353–364. doi: 10.1093/jxb/erh064
Wessells, K. R., Singh, G. M., and Brown, K. H. (2012). Estimating the global prevalence of inadequate zinc intake from national food balance sheets: effects of methodological assumptions. PLoS ONE 7:e50565. doi: 10.1371/journal.pone.0050565
White, P. J., and Broadley, M. R. (2009). Biofortification of crops with seven mineral elements often lacking in human diets – iron, zinc, copper, calcium, magnesium, selenium and iodine. New Phytol. 182, 49–84. doi: 10.1111/j.1469-8137.2008.02738.x
Xu, Y., An, D., Li, H., and Xu, H. (2011). Review: breeding wheat for enhanced micronutrients. Can. J. Plant Sci. 91, 231–237. doi: 10.4141/CJPS10117
Xu, Y., An, D., Liu, D., Zhang, A., Xu, H., and Li, B. (2012). Molecular mapping of QTLs for grain zinc, iron and protein concentration of wheat across two environments. Field Crops Res. 138, 57–62. doi: 10.1016/j.fcr.2012.09.017
Yang, X., Ye, Z. Q., Shi, C. H., Zhu, M. L., and Graham, R. D. (1998). Genotypic differences in concentrations of iron, manganese, copper, and zinc in polished rice grains. J. Plant Nutr. 21, 1453–1462.
Zdunić, Z., Grljušić, S., Ledenčan, T., Duvnjak, T., and Šimić, D. (2014). Quantitative trait loci mapping of metal concentrations in leaves of the maize IBM population. Hereditas 151, 55–60. doi: 10.1111/hrd2.00048
Zhang, X., Zhang, G., Guo, L., Wang, H., Zeng, D., Dong, G., et al. (2011). Identification of quantitative trait loci for Cd and Zn concentrations of brown rice grown in Cd-polluted soils. Euphytica 180, 173–179. doi: 10.1007/s10681-011-0346-9
Zhao, F. J., and McGrath, S. P. (2009). Biofortification and phytoremediation. Curr. Opin. Plant Biol. 12, 373–380. doi: 10.1016/j.pbi.2009.04.005
Keywords: biofortification, cereals, iron, zinc, micronutrient deficiency, toxic risks
Citation: Garcia-Oliveira AL, Chander S, Ortiz R, Menkir A and Gedil M (2018) Genetic Basis and Breeding Perspectives of Grain Iron and Zinc Enrichment in Cereals. Front. Plant Sci. 9:937. doi: 10.3389/fpls.2018.00937
Received: 16 January 2018; Accepted: 11 June 2018;
Published: 02 July 2018.
Edited by:
Felipe Klein Ricachenevsky, Universidade Federal de Santa Maria, BrazilReviewed by:
Ümit Bariş Kutman, Gebze Technical University, TurkeyCopyright © 2018 Garcia-Oliveira, Chander, Ortiz, Menkir and Gedil. This is an open-access article distributed under the terms of the Creative Commons Attribution License (CC BY). The use, distribution or reproduction in other forums is permitted, provided the original author(s) and the copyright owner(s) are credited and that the original publication in this journal is cited, in accordance with accepted academic practice. No use, distribution or reproduction is permitted which does not comply with these terms.
*Correspondence: Ana Luisa Garcia-Oliveira, YS5vbGl2ZWlyYUBjZ2lhci5vcmc=
Rodomiro Ortiz, cm9kb21pcm8ub3J0aXpAc2x1LnNl
Disclaimer: All claims expressed in this article are solely those of the authors and do not necessarily represent those of their affiliated organizations, or those of the publisher, the editors and the reviewers. Any product that may be evaluated in this article or claim that may be made by its manufacturer is not guaranteed or endorsed by the publisher.
Research integrity at Frontiers
Learn more about the work of our research integrity team to safeguard the quality of each article we publish.