- 1State Key Laboratory of Crop Stress Biology in Arid Areas, College of Life Sciences, Northwest A&F University, Yangling, China
- 2Department of General Biology, College of Life Science and Agronomy, Zhoukou Normal University, Zhoukou, China
- 3The Key Laboratory of Biotechnology for Medicinal Plant of Jiangsu Province, School of Life Sciences, Jiangsu Normal University, Xuzhou, China
As the key producers of reactive oxygen species (ROS), NADPH oxidases (NOXs), also known as respiratory burst oxidase homologs (RBOHs), play crucial roles in various biological processes in plants with considerable evolutionary selection and functional diversity in the entire terrestrial plant kingdom. However, only limited resources are available on the phylogenesis and functions of this gene family in wheat. Here, a total of 46 NOX family genes were identified in the wheat genome, and these NOXs could be classified into three subgroups: typical TaNOXs, TaNOX-likes, and ferric reduction oxidases (TaFROs). Phylogenetic analysis indicated that the typical TaNOXs might originate from TaFROs during evolution, and the TaFROs located on Chr 2 might be the most ancient forms of TaNOXs. TaNOXs are highly expressed in wheat with distinct tissue or organ-specificity and stress-inducible diversity. A large-scale expression and/or coexpression analysis demonstrated that TaNOXs can be divided into four functional groups with different expression patterns under a broad range of environmental stresses. Different TaNOXs are coexpressed with different sets of other genes, which widely participate in several important intracellular processes such as cell wall biosynthesis, defence response, and signal transduction, suggesting their vital but diversity of roles in plant growth regulation and stress responses of wheat.
Introduction
Reactive oxygen species (ROS) have dual roles in plant growth regulation and environmental stress response. They not only lead to programmed cell death by damaging the cellular components as deleterious factors of oxidative metabolism but are also necessary for plant tolerance to different biotic and abiotic stresses acting as signal molecules (Suzuki et al., 2011; Dietz et al., 2016). Although there are various ROS-generating pathways in plants, the plasma membrane NADPH oxidases (NOXs), also named after respiratory burst oxidase homologs (RBOHs), were considered to be the key enzymes for apoplastic ROS production under both normal and stress conditions and play crucial roles in various biological processes in plants.
NOX homologs are universally present in a broad range of organisms including animals, higher plants, and fungi (Bedard et al., 2007). Until recently, various types of NOXs including the ancestral NOXs, namely ferric reductases (FREs) or ferric reduction oxidases (FROs), were reported in life kingdom with four types. These types were NOXA, NOXB, NOXC, and FRE in fungi (Aguirre et al., 2005). There were also seven types including NOX1–5, DUOX1, and DUOX2 in animals (Bedard et al., 2007) and one type in plants known as NOX5-Like (Sagi and Fluhr, 2006). Although the first NOX gene OsRbohA (OsNOX2) was characterized as a gp91phox homolog of mammalian NOX in rice, multiple NOX members were identified in plants such as Arabidopsis thaliana (Sagi and Fluhr, 2006), rice (Wang et al., 2016), maize (Potocký et al., 2007), tobacco (Yoshioka et al., 2001), potato (Yoshioka et al., 2003), tomato (Amicucci et al., 1999), Medicago truncatula (Marino et al., 2011), and Phaseolus vulgaris (Nestler et al., 2014), and more than 50 FRO and 77 NOX gene homologs were identified (Chang et al., 2016). All the plant NOX members are NOX5-like homologs except the ancestral NOXs (FROs), which were considered as the isoforms of yeast FREs (Sagi and Fluhr, 2006; Bedard et al., 2007). In addition to having their special NADPH_Ox domain and several calcium-binding EF-hand motifs in its N terminus, the NOX5-like homologs in plants also contain membrane-spanning domains, two hemes, NADPH-binding motifs, and FAD-binding motifs just like all the other NOX/DUOX enzymes in animals (Geiszt, 2006). Many plants have multiple NOX members, and each member has its own special function during the growth and development regulation and stress response. In Arabidopsis, there are 10 typical NOX members reported as AtRbohA–J (Sagi and Fluhr, 2006). Among these NOXs, AtRbohD was found to participate in various important processes including abscisic acid-mediated ROS production and stomatal closure (Zhang et al., 2009), lignin assembly (Denness et al., 2011), endosperm development (Penfield et al., 2006) as well as in systemic signal transduction, (Miller et al., 2009), and survival of seedlings under anoxic conditions (Yamauchi et al., 2017). Moreover, it was reported that AtRbohB mainly participates in seed after ripening (Muller et al., 2009), AtRbohC regulates root hair formation and root-hair-cell growth (Takeda et al., 2008), whereas both AtRbohD and AtRbohF not only regulate the immune response (Chaouch et al., 2012) and salt stress tolerance (Xie et al., 2011) but also function in jasmonic acid (JA)-induced gene expression (Maruta et al., 2011). Furthermore, AtRbohE was found to regulate tapetal programmed cell death (PCD) and pollen development (Xie et al., 2011), whereas AtRbohH and AtRbohJ function in pollen tube development (Kaya et al., 2014). In rice, at least nine typical NOX members were identified to be of OsRbohA–I or OsNOX1–9 (Wong et al., 2007; Wang et al., 2013). It was found that OsRbohA (OsNOX2), OsRbohB (OsNOX1), and OsRbohE (OsNOX3) are involved in immune response (Yoshie et al., 2005; Nagano et al., 2016), and OsRbohA (OsNOX2) also plays a crucial role in drought-stress tolerance (Wang et al., 2016). Most recently, OsRbohH (OsNOX9) was found to regulate the aerenchyma formation in roots of rice (Yamauchi et al., 2017). In maize, both ZmRbohH (ZmNOX13) and ROOTHAIRLESS5 (ZmNOX7) were found to function in root development (Rajhi et al., 2011; Nestler et al., 2014).
Moreover, increasing evidence shows that NOXs in plants may serve as molecular “hubs” during ROS-mediated signal transduction pathways (Marino et al., 2012), including receptor-activated C-kinases (RACKs) (Nakashima et al., 2008), Ca2+-dependent protein kinases (CDPKs) (Dubiella et al., 2013), mitogen-activated protein kinases (MAPKs) (Asai et al., 2008), phospholipase Dα1 and phosphatidic acid (Zhang et al., 2009), Ca2+ (Wudick and Feijo, 2014), phosphatidylinositol (Kaye et al., 2011), NO (Delledonne et al., 2002), cGMP (Li et al., 2011), and extracellular ATP-mediated signaling pathways (Song et al., 2006), and hormonal signaling networks (Mittler et al., 2011). Furthermore, AtRbohD was found to participate in the clathrin- and membrane microdomain-dependent endocytic pathways in Arabidopsis (Hao et al., 2014). Although previous studies have highlighted the biochemical properties and physiological functions of NOXs to a certain degree, considering the multiple members in different plant species in which only a few of NOXs were studied in detail, the functions of NOX family proteins are still under investigation.
Wheat (Triticum aestivum) is a worldwide staple crop, necessitating a clear deciphering of its developmental characteristics and stress tolerance mechanisms. However, as of today, only two NOXs in wheat have been reported to participate in brown rust infection response (Dmochowska-Boguta et al., 2013), and the functions of wheat NOX family genes and their regulatory mechanisms in both plant growth regulation and environmental stress response are still largely unknown. In the present study, a comprehensive analysis based on bioinformatics approaches and experimental methods was performed to identify the wheat NOX family genes and their functions during the plant development and stress response. The results obtained here will largely broaden our understanding of the roles of NOXs and their regulation in plants, especially in wheat.
Materials and Methods
Sequence Retrieval and Identification of the NOX Gene Family in Wheat
We retrieved the potential sequences of NOX family members in wheat from IWGSC (http://www.wheatgenome.org/, last accessed May 25, 2017), NCBI (https://www.ncbi.nlm.nih.gov/, last accessed May 25, 2017), and Ensembl Plants (http://plants.ensembl.org/Triticum_aestivum/Info/Index, last accessed May 25, 2017) websites, with Arabidopsis and rice NOX sequences as queries. We identified each NOX member by predicting the conserved domains. For further information, we analyzed some physicochemical parameters, predicted the subcellular localization and the numbers of transmembrane helix, and performed amino acid sequence alignment (see Supplementary Information S1 for detailed procedures).
Chromosomal Location and Exon/Intron Structure Analysis
Using Adobe_Photoshop_CS6 software, a total of 46 candidate genes were mapped to 18 different chromosomes according to the information from scaffolds and Gene ID reported in IWGSC and NCBI websites. The exon/intron logos of individual NOX and FRO genes were obtained from the Gene Structure Display Server (http://gsds.cbi.pku.edu.cn) by aligning the coding or cDNA sequences with their corresponding genomic DNA sequences.
Sequence Alignment and Gene Structure Analysis
The phylogenetic tree of wheat NOX and FRO family members was constructed with MEGA 6.06. The logos of domain organization were obtained from EMBL-EBI (http://pfam.xfam.org/search#tabview=tab1) or SMART (http://smart.embl-heidelberg.de/) websites and were amended with Adobe_Photoshop_CS6. The four conserved domain motifs, namely NADPH_Ox, Ferric_reduct, FAD_binding_8, and NAD_binding_6 in each NOX sequence, were generated by MEME suite (http://meme-suite.org/) (see Supplementary Information S1 for detailed procedures).
Phylogenetic Relationships of NOX and FRO Gene Families in Wheat and Seven Other Plant Species
Multiple sequence alignments and the phylogenetic relationship analysis of NOX and FRO gene families from eight plant species were performed using MEGA 6.06. Meanwhile, the non-synonymous (Ka) and synonymous (Ks) in paralogous and/or orthologous gene pairs from four species were also estimated using the bioinformatics software PAMLX 1.2 (see Supplementary Information S1 for detailed procedures).
Subcellular Localization Analysis
The subcellular location of wheat NOXs was examined in rice protoplasts using transient transformation systems with some modifications (Zhang et al., 2011); at least three monoclones were sent for sequencing upon gene cloning and vector construction for each examined gene (see Supplementary Information S1 for detailed procedures).
Prediction and Functional Analysis of cis-Regulatory Elements
We selected 2,000-bp genomic DNA sequences upstream of the transcriptional start sites of TaNOXs as the promoter sequences (named TaNOX-pros) to analyze the cis-acting elements using the databases: PlantCARE (http://bioinformatics.psb.ugent.be/webtools/plantcare/html/) (Lescot et al., 2002) and PLACE (http://www.dna.affrc.go.jp/PLACE/). Then, the activity of the promoters was analyzed by dual luciferase reporter assay (Zhang et al., 2011; Gu et al., 2013). Three or more monoclones were sent for sequencing to identify each promoter sequence and related vector construction. All values were shown as the average of the data collected from five or more replicates (see Supplementary Information S1 for detailed procedures).
Plant Materials, Treatments, Expression Profiles, and Coexpression Network Analysis
Tissue-specific expression profiles, inducible expression profiles of TaNOX genes, and coexpression analysis between TaNOXs and other genes in wheat (Triticum aestivum cv. Chinese Spring) were performed using the online Genevestigator v3 (https://genevestigator.com/gv/) and/or by qRT-PCR with TaActin (AB181991.1) and TaGAPDH (ABS59297.1) as the internal transcript level controls. The wheat seedlings were harvested from different developmental stages for tissue-specific expression profiles analysis. At the same time, the wheat seedlings treated with abiotic stresses and hormones were also collected for inducible expression profiles and coexpression network analysis. Total RNA was extracted from different samples using RNAiso TM Plus (Takara, Dalian, China) performance, and the subsequent quantitative real-time PCR (qRT-PCR) analysis was referenced to our previous study (Li et al., 2014). The results were presented as heat maps, histogram, and/or also as table lists. All the expression levels represent the mean ± SD of data collected from three independent experiments with each having three or four replicates (see Table S6 and Supplementary Information S1 for detailed procedures).
Results
Identification of NOX Family Genes in Wheat Genome
A hidden markov model (HMM) search was performed to investigate and characterize the NOX gene family in wheat genome, and a total of 46 genes including 36 NOX and 10 FRO candidates were identified (Table S1). These NOX and FRO candidates can be divided into three types: TaNOXs, TaNOX-likes, and TaFROs based on their chromosome localization and domain composition (Table S1, Figures 1, 2). The homologous genes from different subgenomes (A, B, and D) were assigned the same number in gene denomination due to their similarity in gene structure and protein size. The typical NOXs (TaNOXs) have all four conserved domains, namely NADPH_Ox (Pfam accession number PF08414), Ferric_reduct (PF01794), FAD_binding_8 (PF08022), and NAD_binding_6 domain (PF08022). The TaNOX-likes have NADPH_Ox domain but lack one or two other domains, whereas the TaFROs lack NADPH_Ox domain but have the other three domains (Figure 2). Intriguingly, the NOX and FRO family genes in wheat genome are non randomly distributed across the chromosomes. It seems that FRO candidates are mainly distributed on Chr 2 and less on Chr 1; however, all the predicted NOX candidates are mainly distributed on Chr 1, followed by Chr 5, 3, 4, and 6, and none of the NOX members on Chr 2 (Figure S1). Moreover, the characteristics of gene structure and protein size are quite different between the NOX and FRO members (Figure 1, Table S1). TaNOXs are much bigger than TaFROs, whereas the TaNOX-likes are the smallest.
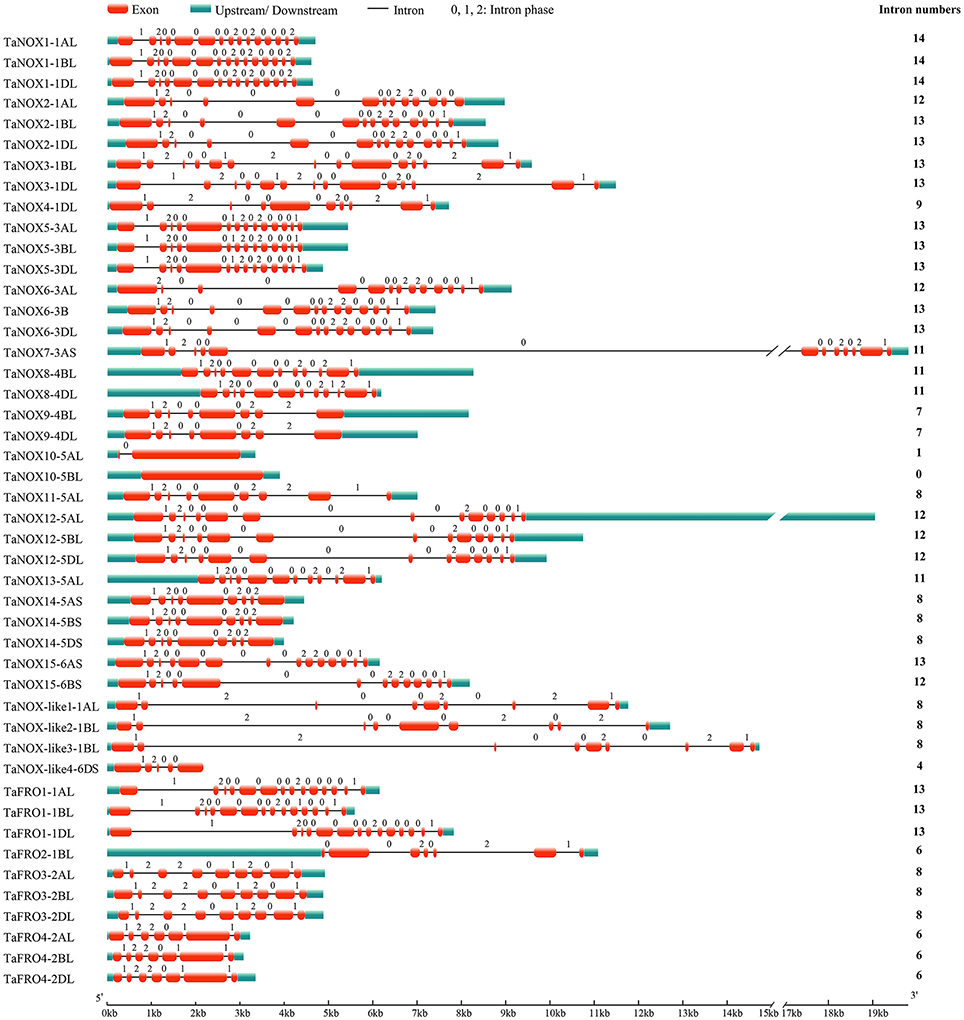
Figure 1. The exon/intron structures of NOX family genes in wheat. The numbers 0, 1, and 2 represent the phase of each intron in the sequence.
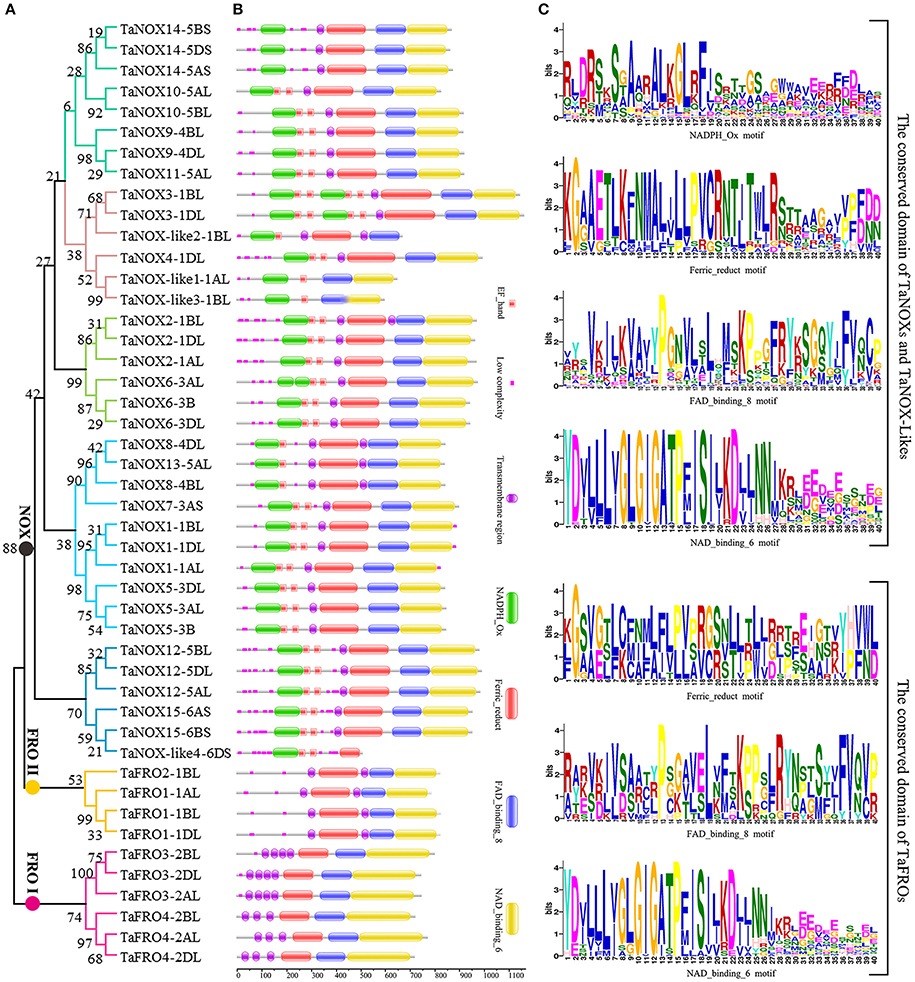
Figure 2. Phylogenetic relationship, domain organization, and conserved motifs analysis of NOX family proteins in wheat. (A) The unrooted maximum-likelihood phylogenetic tree of TaNOXs and TaFROs family members. Numbers above the nodes represent bootstrap values from 1,000 replications. (B) Domain organization of the TaNOXs and TaFROs. The logos of domain organization were obtained from EMBL-EBI and SMART websites and were amended with Adobe_Photoshop_CS6. (C) Four conserved motifs, namely NADPH_Ox, Ferric_reduct, FAD_binding_8, and NAD_binding_6, from all 46 NOX proteins in wheat. The logos of four conserved domain sequences were obtained from MEME Suite website. The bit score means information content of each position in the amino acid sequence.
Gene Structure and Domain Composition of the Wheat NOX Family Proteins
As can be seen in Figure 1, the gene structures are quite diverse between the TaNOXs and TaFROs with different intron numbers for different genes except for the certain homologous genes from different subgenomes (A, B, and D). On the whole, the gene constructions became more and more complicated from TaFROs to TaNOXs for increasing exons and introns. As a special type of NOX, TaNOX-likes are much simple with less exons and introns. According to the phylogenetic tree (Figure 2A), we found that all the homologous genes assigned as the same number in gene denomination were generally clustered into one group earlier than the others, and all the NOX family members could be classified into three big branches: FRO I (including TaFRO3 and 4) on the Chr 2, FRO II (including TaFRO1 and 2) on the Chr 1, and NOX (including TaNOXs and TaNOX-likes) on the Chr 1, 3, 4, 5, and 6, respectively.
As shown in Figure 2B, almost all the typical NOXs contain one or two transmembrane regions, one to four calcium-binding EF-hand motifs, and four conserved constructions, namely one or two typical NADPH_Ox domain, one Ferric_reduction domain, one FAD_binding_8 domain, and one NAD_binding_6 domain. Only TaNOX14 does not have an EF-hand motif (Figure 2B). All TaFROs possess the conserved domains that the typical TaNOXs have but lack the NADPH_Ox domain and the EF-hand motif. In contrast, the TaNOX-likes have only one or two of the three conserved domains similar to TaFROs possession, besides the typical NADPH_Ox and EF-hand motifs. Furthermore, the four functional domains exhibited to be all considerably conserved in construction, and the distribution of amino acid residues in every domain is quite similar but not identical among the NOX members (Figure 2C). Four conservative amino acid residues Y, G, G, and P presented with Y × × G × G × × P motif in the 5′end sequence of NAD_binding_6 domain from TaFROs were the same as those from the TaNOXs and TaNOX-likes. There were also other quite conserved residues, for example, the P in FAD_binding_8 domain and the G in Ferric_reduct domain, as indicated in capital letters in Figure 2C. However, the conservatism of amino acid residues in NADPH _Ox domain was lower than the other three conserved domains. Taken together, all the results mentioned above imply a closer evolutionary relationship between TaFROs and TaNOXs (Figure 2).
Subcellular Localization of the Wheat NOX Family Proteins
As shown in Table S1, all the NOXs including TaNOXs, TaNOX-likes, and TaFROs are located in the plasma membrane of cells as predicted by the Plant-mPLoc. Although no typical transmembrane helixes were identified in TaNOX-likes, these proteins were also predicted to be located at the plasma membrane. To verify the subcellular localization of TaNOXs, the full-length cDNA sequences of four genes, TaNOX7-3AS, TaNOX10-5BL, TaFRO4-2BL, and TaNOX-like4, were cloned and analyzed by a transient transformation system. As shown in Figure 3, the proteins encoded by the four genes are all located on the plasma membrane as expected.
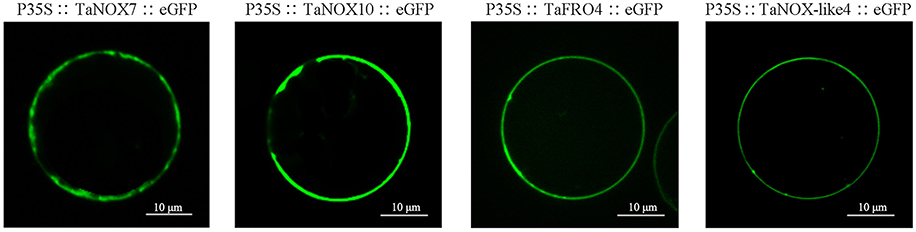
Figure 3. Subcellular localization of TaNOXs. Analysis of the subcellular location was performed using rice protoplast transient transformation systems. The constructs of TaNOX7::eGFP, TaNOX10::eGFP, TaFRO4::eGFP, and TaNOX-like4::eGFP were all under the control of the constitutive 2 × 35S promoters (cauliflower mosaic virus [CaMV]) in pTF486 vectors; at least three monoclones were sent for sequencing upon gene cloning and vector construction for each gene.
Systematic Evolutionary Relationship of NOX Gene Family in Wheat
To comprehensively dissect and characterize the evolutionary relationship of NOX gene family in wheat, a total of 112 NOX and FRO homologs were identified and selected from wheat and other seven plant species for the multiple sequence alignments and phylogenetic relationship analysis (Figure 4; Table S2). The evolutionary relationships between wheat (T. aestivum) and seven other species were presented in Figure 4A, and the numbers of NOX and FRO members were provided in Figure 4B. All the members of NOX and FRO families could be classified into six subgroups I–VI based on the difference of protein topological structure (Figure 4C). The wheat TaNOXs and TaFROs are distributed in every subgroup but dominantly distributed in subgroup I, whereas, the Arabidopsis AtNOXs (AtRbohs) and AtFROs are mainly distributed in subgroup VI (Figure 4D). The members within a certain subgroup exhibited a high identical percentage of amino acid sequences as shown in Table S3.
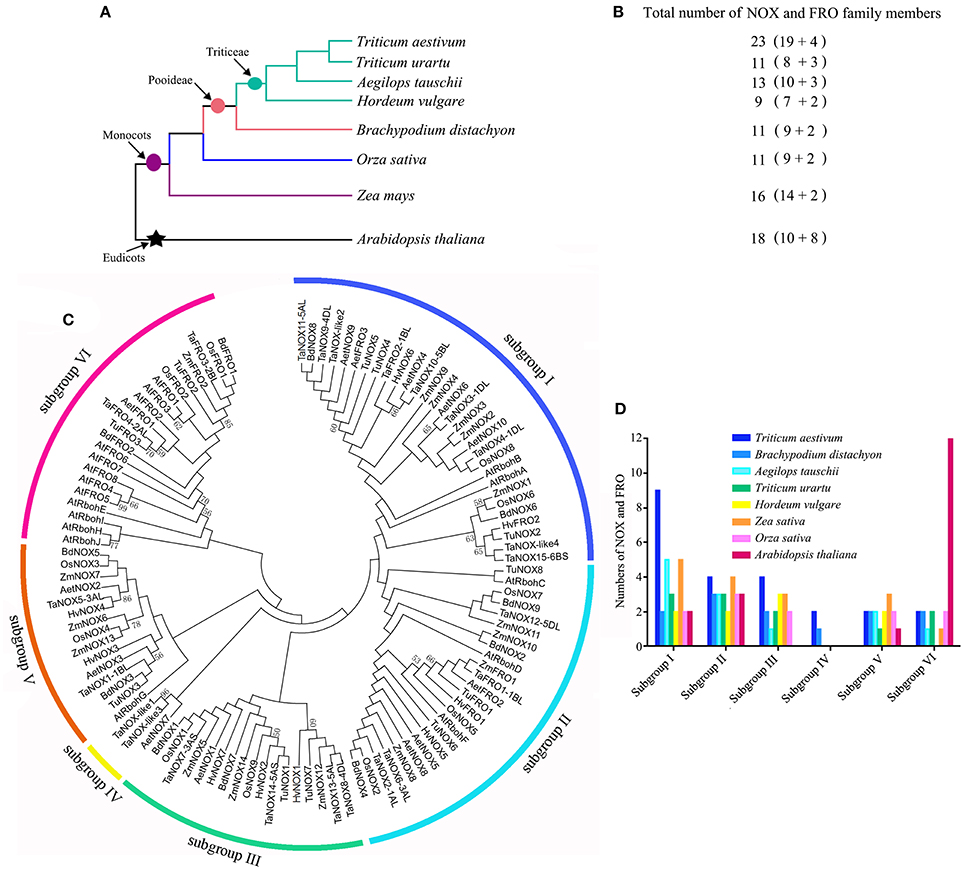
Figure 4. Systematic evolutionary relationships of NOX and FRO gene families in eight plant species. (A) Evolution relationship among the eight grass plant species. (B) The numbers of NOXs and FROs in each species. (C) Phylogenetic tree of NOX and FRO family members in the eight plants. (D) The number of NOXs in the subgroups I–VI.
To estimate evolutionary relatedness and divergence time of NOX family genes between wheat (T. aestivum) and its diploid relatives: Aegilops tauschii, Triticum urartu, and Hordeum vulgare, the rates of non synonymous (Ka) and synonymous (Ks) nucleotide substitutions and their ratios (ω = Ka/Ks) of four putative paralogous and 19 orthologous gene pairs of NOXs were calculated using the bioinformatics software pamlX (Table S4). As can be seen, the majority of Ka/Ks ratios (ω) are more than 1 among the paralogous gene pairs, indicating that the NOX family genes have undergone strong positive selection pressure for functional expansion, and the duplication event was estimated to have occurred 3.16630–6.2533 Mya. On the contrary, the values of ω are almost less than 1 among the orthologous gene pairs, suggesting that the NOX family genes have undergone strong negative selection pressure for functional conservation, and the duplication event was estimated to have occurred 0.0060–0.6466 Mya.
Development and Tissue-Specific Expression of NOX Family Genes in Wheat
To clarify the expression profiles of NOX family genes during the development of wheat, a set of microarray data for the gene expression were obtained from Genevestigator v3. To simplify the phraseology in the following experiments, the homologous genes located on different subchromosomes (Chr A, B, and C) are referred to as TaNOXx; for example, TaNOX1-1AL, -1BL, and/or -1DL were all named as TaNOX1. The expression levels of TaNOXs at 10 developmental stages were presented in Figure 5A. As can be seen, different TaNOXs exhibited different expression patterns with some genes dominantly expressed at certain stages. For example, the genes TaNOX2, 7, and 12 are widely expressed in whole developmental stages with the highest in stem elongation and inflorescence emergence stages, whereas TaNOX15 and TaNOX-like4 are mainly expressed in germinating seeds and at booting stage. The expression of TaFROs has more specificity in wheat. For instance, TaFRO1 and TaFRO2 are only expressed at stem elongation stage, whereas TaFRO3 is mainly expressed at young seedling, inflorescence emergence, and milk stages (Figure 5A).
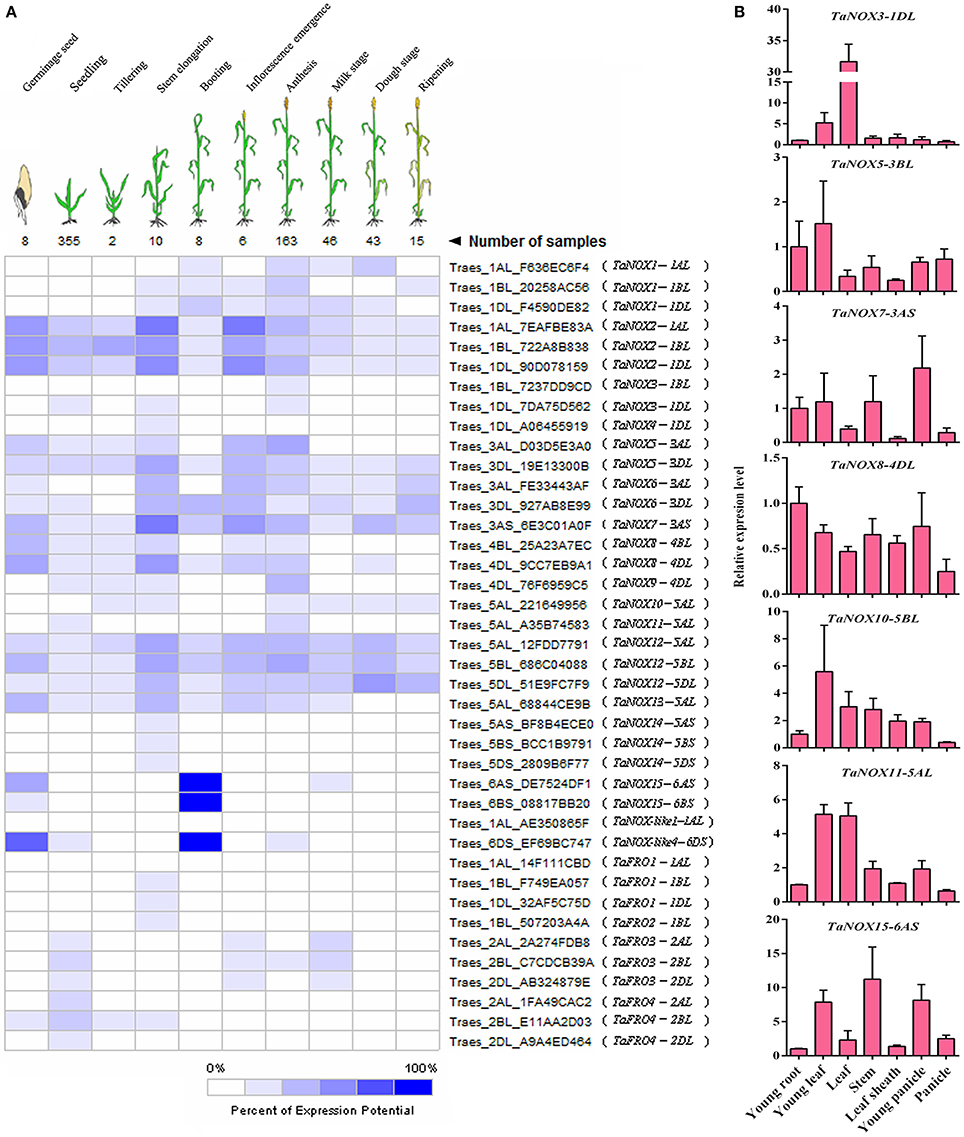
Figure 5. Expression patterns of NOX and FRO family genes in wheat at different developmental stages and in different tissues. (A) The developmental expression pattern analysis of NOX and FRO family genes in 10 developmental stages during wheat growth. All the data were selected from the Ta_mRNASeq_WHEAT_GL-0 database. (B) Expression profiles of seven wheat NOX genes in seven different tissues. The seven tissues are as follows: the young leaf and young root came from 2-week-old seedlings; the leaf, stem, leaf sheath, and young panicle came from the booting stage; the panicle came from the inforescence emergence stage. The developmental expression pattern for each NOX or FRO family gene was performed by the three independent experiments with three replicates for each experiment.
The tissue-specific expression profiles of TaNOXs were examined by qRT-PCR (Figure 5B). Seven wheat tissues from different developmental stages were used for the analysis. According to the tissue-expression profiles obtained from Genevestigator v3, seven TaNOX genes, including TaNOX3, 5, 7, 8, 10, 11, and 15, were selected as the candidates to study the tissue specificities of the genes in wheat. The results showed that every gene had its obvious tissue specificities in expression. The expression of TaNOX3 is the highest in leaves at booting stage, whereas the expression of TaNOX5, 10, and 11 is the highest in young leaves at seedling stage, TaNOX7 in young panicle at booting stage, TaNOX8 in young roots at seedling stage, and TaNOX15 in stems at booting stage. All these results indicated that the expression of each NOX gene is unique and displays a strong spatio-temporal and tissue specificity in wheat.
Analysis of the Promoters of NOX Genes and Their Response to Exogenous Treatments
The promoters of all TaNOX genes were analyzed, and the cis-acting elements existing in the promoters were predicted by the databases of PlantCARE, PLACE, and Neural Network Promoter Prediction. In total, 31 cis-regulatory elements with the prediction score being greater than or equal to 5 remained and were depicted on the corresponding sequences. The elements responsive to environmental stresses and hormones were considered for further analysis (Figure 6, Figure S2). As can be seen, almost all the stress or hormone responsive elements are distributed randomly on the promoter sequences of the TaNOX genes. However, some cis-elements are distributed in clusters in certain promoters, for example, MBS-motif, TGACG-motif, and G-box in the promoters of TaNOX7AS, TaNOX5-3AL and TaNOX5-3BL genes, respectively, implying the essential roles of these elements in the expression of these genes. Interestingly, the numbers and distribution patterns of the cis-elements are also greatly varied among the promoters of homologous genes with the same number in gene denomination although their encoding proteins have an extreme similarity in amino acids sequence and domain construction (Figure 6). This means that the homologous genes might have different regulatory mechanisms in expression, implying their functional divergence during the polyploidization of wheat genome.
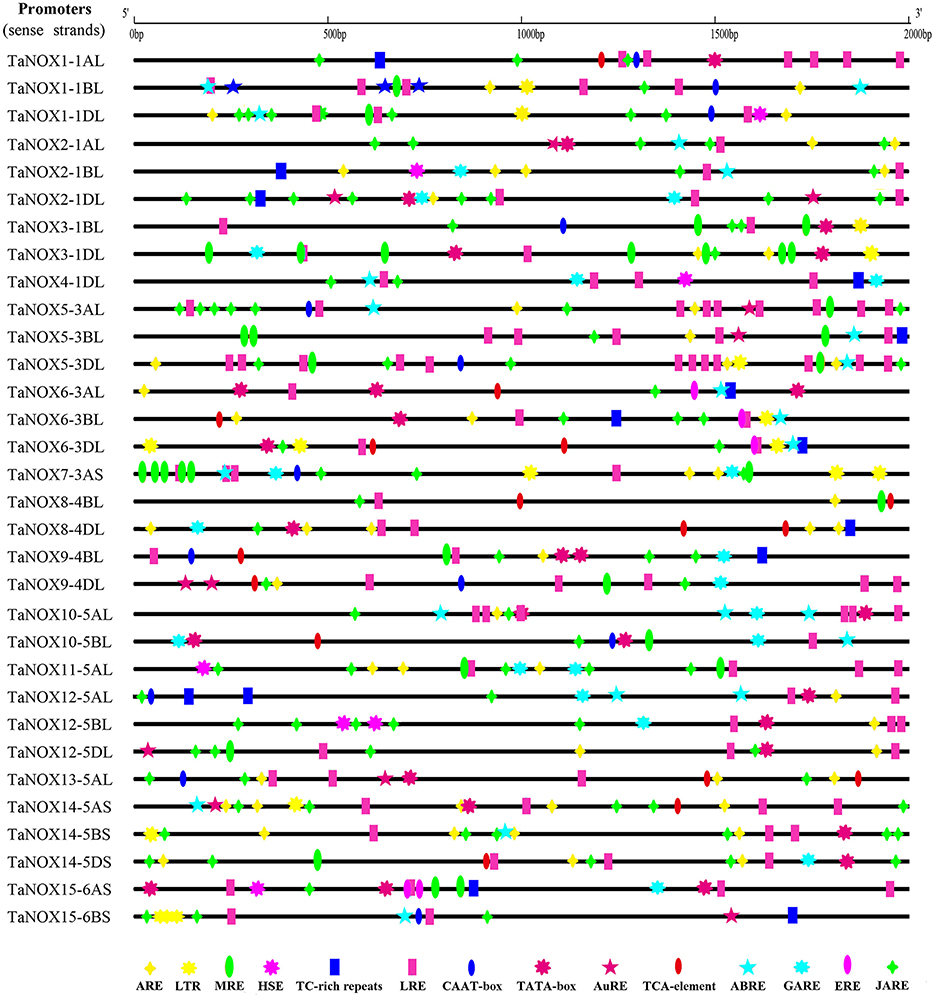
Figure 6. The cis-elements responding to abiotic/biotic stresses and hormone treatments in the sense strands of TaNOX promoters. The cis-elements are as follows. Anaerobic responsive element (ARE): ARE and GC motif; LTR: low-temperature responsiveness; MYB-responsive element (MRE): MBS and MRE; HSE: heat stress responsiveness; TC-rich repeats: defence and stress responsiveness; light-responsive element (LRE): G-box, SPI, MNF1, 4cl-CMA2b, GT1-motif, ACE, and BoxI elements; CAAT-box: common cis-acting element in promoter and enhancer regions; TATA-box: core promoter element around−30 bp of transcription start; auxin-responsive element (AuRE): AuXRR core and TGA element; TCA element: salicylic acid (SA) responsiveness; abscisic acid-responsive element (ABRE): ABRE, motif IIb and CE3 elements; gibberellins (GA)-responsive element (GARE): GARE, TATC-box and p-box; ERE: ethylene-responsive element; methl jasmonic acid (MeJA)-responsive element (JARE): TGACG motif and CGTCA motif.
To get further insight into the regulation of gene expression of TaNOXs, the promoters of seven genes were cloned, and their activities in response to heat, cold, ABA, and MeJA treatments were determined using a dual luciferase reporter system. As shown in Figure 7, each promoter examined exhibited different levels of biological activities in response to different treatments. The activities of almost all the promoters of seven TaNOX genes could be greatly stimulated by heat (Figure 7B), whereas no marked changes in the activities were observed under cold stress compared with the controls. Only the activity of TaNOX7-3AS promoter was obviously decreased by cold (Figure 7C). ABA treatment also significantly stimulated the activities of the promoters of the TaNOX genes except for the promoter of TaNOX3-1DL (Figure 7D). Although the MeJA-responsive cis-elements are widely distributed in the promoters of the seven genes examined here, only the promoters of TaNOX7-3AS, TaNOX12-5BL, and TaNOX13-5AL responded to the exogenous MeJA treatment (Figure 7E). These results indicated that the responses of the TaNOX genes to environmental factors are quite different, and a complicated mechanism might be involved in the control of gene expression in wheat.
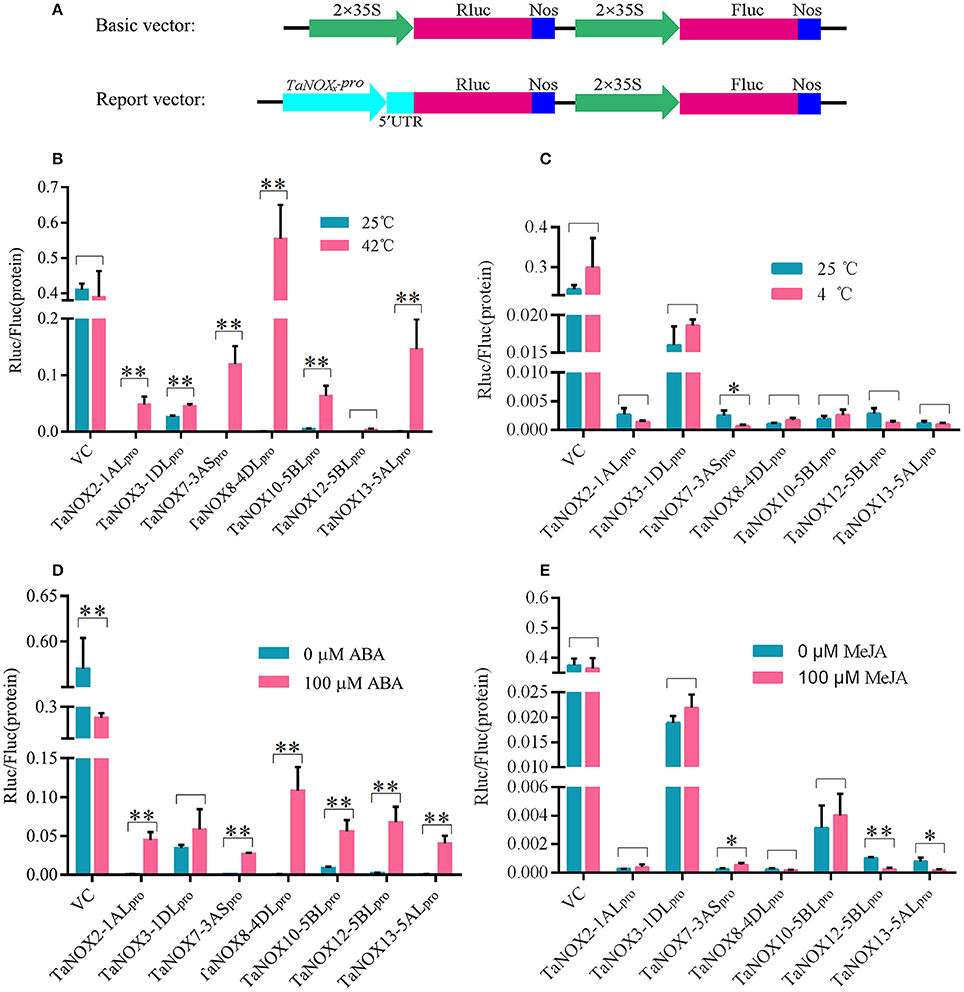
Figure 7. Activity analysis of the promoters of TaNOXs under temperature stress and hormone treatments. (A) Schematic representation of the basic and report vector constructs. (B–E) The relative activity of the promoters of TaNOXs under temperature stress and hormone treatments. The activity analysis of the promoters represents the mean ± SD of data collected at least seven replicates. *P < 0.05; **P < 0.01.
Inducible Expression Profiles Range of TaNOXs Under a Wide Range of Environmental Stresses and Hormone Treatments
To further study the expression characteristics of wheat NOX family genes under suboptimal conditions, we carried out a comprehensive analysis using both the wheat microarray data in Genevestigator v3 and qRT-PCR experiment (Figure 8, Figure S3). As can be seen in Figure 8A, many NOX genes in wheat can respond to a number of environmental stresses. Different inducible expression patterns could be seen in different NOX genes responding to different biotic stresses such as B. graminis, F. graminearum, P. graminis, P. striliformis, and X. translucens and to different abiotic stresses such as cold, heat, drought, and salt. According to the expression patterns, the TaNOX family genes can be simply classified into four groups: Group I including TaNOX1, 2, 6, 7, 8, 12, 13, and 15 and TaNOX-like1 and 4 was upregulated by cold but downregulated by almost all the other environmental treatments; Group II including TaFRO1 and TaNOX4 had no obvious responses to all the environmental treatments; Group III including TaNOX5, TaFRO3, and 4 was upregulated by P. Graminis attack but downregulated by all abiotic treatments and B. graminis and F. graminearum attacks; and Group IV including TaNOX3, 9, 10, 11, 14, and TaFRO2 was upregulated by B. graminis and F. graminearum attacks but downregulated by P. graminis attack (Figure 8A).
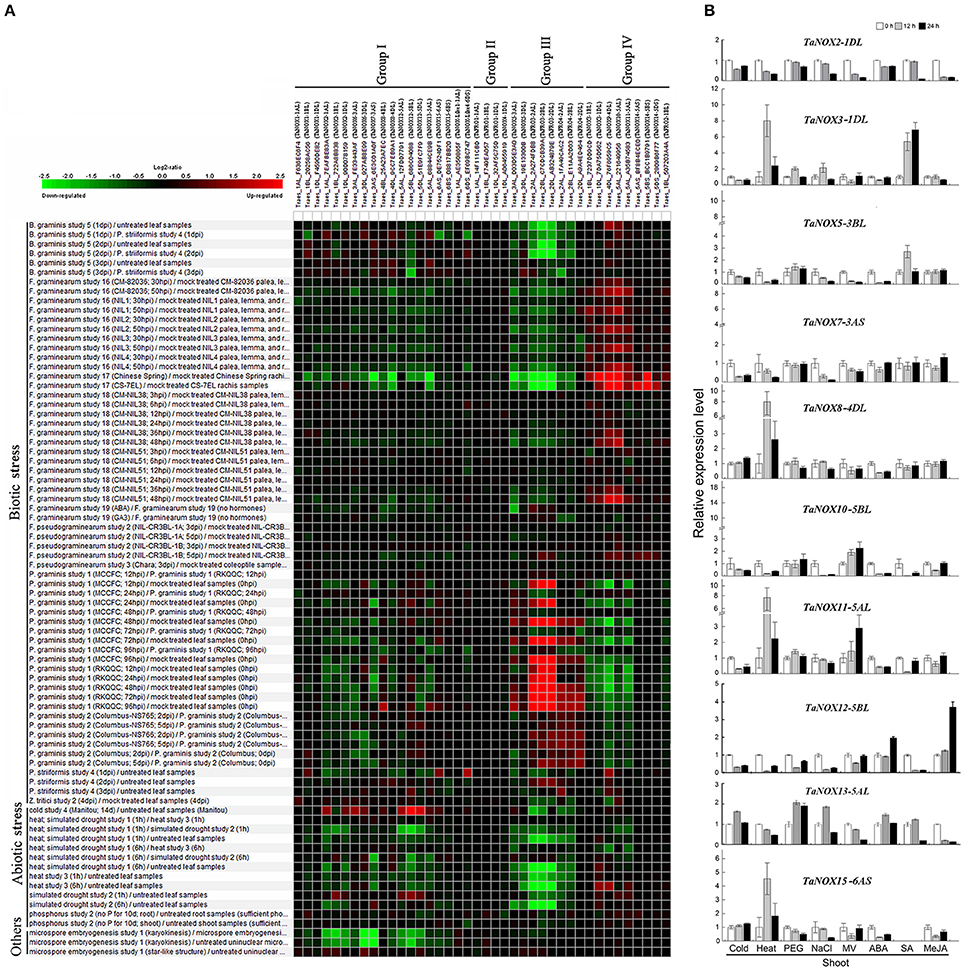
Figure 8. Inducible expression patterns of wheat NOX family genes under different biotic and abiotic stresses. (A) The inducible expression patterns of TaNOXs obtained from the database of Ta_mRNASeq_WHEAT_GL-0 as reported by Genevestigator v3. Results were given as heat maps in green/red coding that reflect relative signal values, where greener represents stronger down regulated expression and redder represents stronger up-regulated expression. (B) The inducible expression patterns performed by qRT-PCR under cold (4°C), heat (40°C), 20% PEG6000, salt (200 mM NaCl), and oxidative (30 μM MV) stresses and by ABA (100 μM), SA (500 μM), MeJA (100 μM) hormone treatments. Two-week-old seedlings were used for the analysis. The expression level of every NOX gene is the mean of results from three independent experiments, each having three or four replicates.
The different responsive profiles of the NOX gene members to different abiotic stresses were also observed by qRT-PCR experiment (Figure 8B, Figure S3B). Here, only a more than two-fold difference in transcript levels was considered to be a true difference for the genes under treatments. Under cold (4°C) stress, most TaNOX genes were downregulated in both shoots and roots at 12 and 24 h time points of the treatment, whereas TaNOX7 was upregulated in roots at 12 h time point. Similarly, most of the genes were downregulated by salt stress (200 mM NaCl) in both shoots and roots at 12 h and/or 24 h time point, whereas TaNOX5 was upregulated in roots at 12 h time point. However, heat stress (40°C) markedly upregulated the transcript levels of TaNOX3, 8, 11, and 15 genes but downregulated TaNOX2, 5, 7, 10, and 12 genes in shoots. In contrast, except for TaNOX12 and 13, almost all the other genes examined were downregulated by heat in roots (Figure S3B). All the examined TaNOXs could be upregulated by the oxidative stress (30 μM MV) in roots. On the contrary, almost all TaNOX genes had no obvious changes by osmotic stress (20% PEG6000) in both roots and shoots except TaNOX13 was upregulated in shoots. Furthermore, except TaNOX7, which showed no changes, and TaNOX12, which was upregulated, all the other genes were downregulated by ABA treatment (100 μM) in shoots, whereas except TaNOX5, which was greatly upregulated, and TaNOX12 and 13, which were downregulated, all the others showed no obvious changes in roots. With the treatment of MeJA (100 μM), the majority of TaNOXs had no significant changes, but TaNOX12 in shoots and TaNOX5, 7, and 10 in roots were exhibited upregulation. For SA treatment (500 μM), the genes of TaNOXs exhibited more complicated expression profiles, and no distinct consistency in expression patterns was observed. Simply, it can be seen that TaNOX3 and 5 genes were upregulated by SA in shoot, whereas TaNOX8 and 10 were upregulated in roots. Although not all the NOX family genes were examined, the results obtained here suggested that each NOX member in wheat has its unique inducible expression profiles and thus has its own functional specificity in the plant stress responses.
Coexpression Relationships Between the Genes of TaNOXs and Others
To further explore the biological functions of TaNOX genes, we generated the coexpression patterns of TaNOXs with others using the wheat microarray data in the database of Ta_mRNASeq_WHEAT_GL-0 from Genevestigator v3 (Figures S4–S6). Eight genes were selected for the analysis according to their inducible expression profiles. Interestingly, several genes showed obvious coexpression relationships with certain NOX genes either at 10 different developmental stages (Figure S4) or in 22 different tissues (Figure S5). These coexpressed genes are mainly involved in the substance transport, cell wall synthesis, redox reaction, phosphorylation, and/or gene expression regulation (Table S5). For instance, TaNOX2 and 3 showed positive correlation with the genes encoding two S-locus lectin protein kinase family proteins; TaNOX3, 7, and TaFRO3 are coexpressed with the genes encoding four leucine-rich repeat protein kinase family proteins. The eight NOX genes also exhibited distinct coexpression with other genes under a lot of biotic and abiotic stress conditions (Figure S6). These coexpression genes are widely involved in stress response, signal transduction, cell wall formation, protein degradation, substance transport, and transcriptional regulation (Table S5). For example, TaNOX2 is highly coexpressed with a gene encoding S-adenosyl-L-methionine-dependent methyltransferase and a gene encoding ubiquitin-specific protease family C19-related protein, whereas TaNOX3 is highly coexpressed with the genes encoding two brassinosteroid-Insensitive 1 precursor kinases and a gene encoding glutathione S-transferase tau 7.
To better understand the coexpression relationships of the TaNOXs with other genes under abiotic stresses, we chose some genes as targets for qRT-PCR. According to the results of tissue and inducible expression profiles, five TaNOX genes and six stress conditions were selected for the experiment. Here, more than two fold difference in transcript levels was considered to be a true difference for the genes as well. As shown in Figure 9, most of the genes selected exhibited significant coexpression patterns with the TaNOXs under a certain stress condition. Only a few genes had an opposite response with the TaNOX gene under some special stress. As can be seen, TaNOX2 exhibited an opposite response with its predicted coexpression genes only under salt stress. For TaNOX3, except under drought and MeJA treatments, it seems that only one predicted gene was not obviously coexpressed with it under four other stress conditions. The distinct coexpression relationships between TaNOX7 and the predicted genes could be observed under heat, natural drying, and ABA treatments. By contrast, TaNOX8 exhibited positive coexpression with the predicted genes under all the six stress treatments. Except drought and salt stresses, TaNOX12 also showed obvious coexpression with its predicted genes examined under other four treatments. These results indicated that the coexpression relationships of TaNOXs with the predicted genes might be stress dependent, implying the specific but complicated functions of NOX genes in wheat development and/or stress responses.
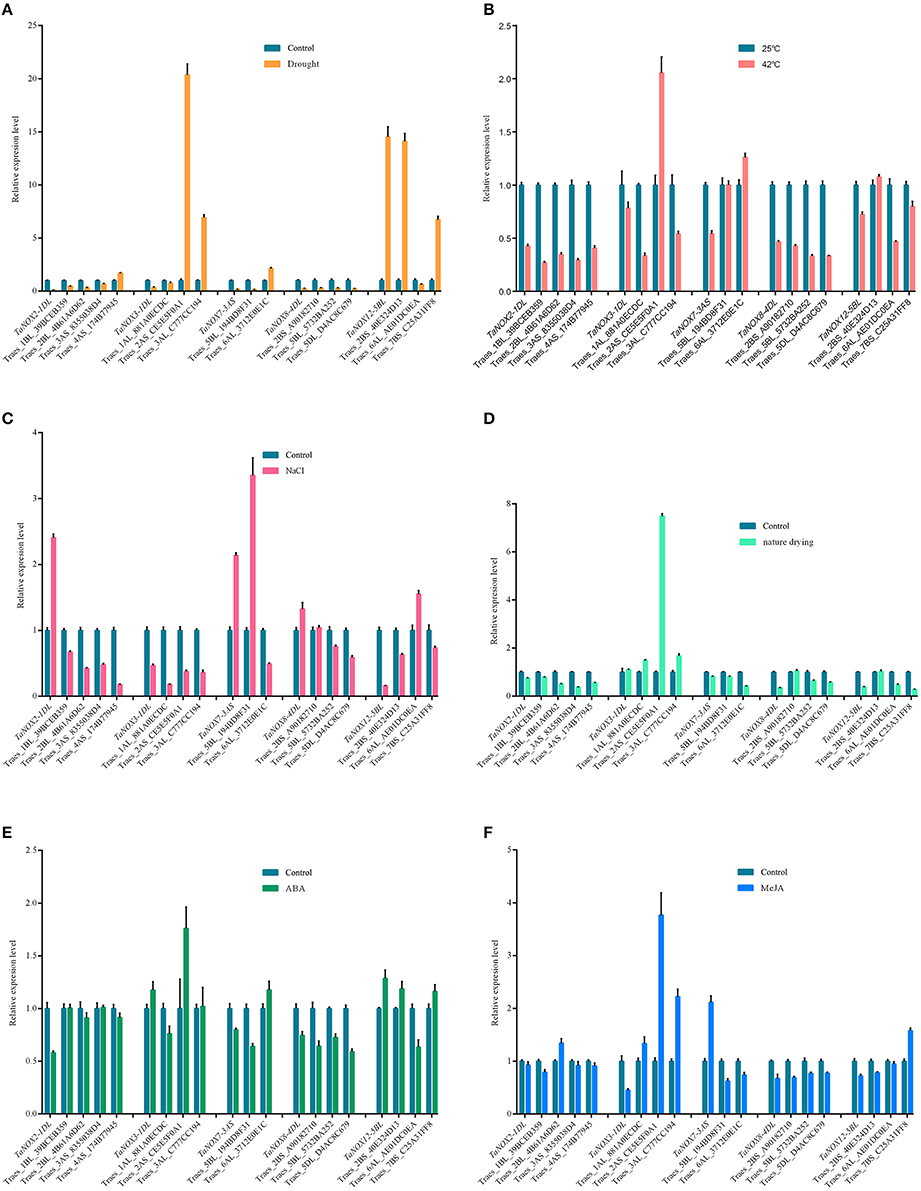
Figure 9. Expression profile analysis of coexpression genes with TaNOXs by qRT-PCR under different abiotic stress treatments on the shoot of wheat seedlings. The 7-day-old wheat seedlings treated with soil drought for 2 days (A), 42°C for 24 h (B), 200 mM NaCl for 6 h (C), nature drying for 30 min (D), 10 μM ABA for 6 h (E), and 10 μM/L MeJA for 6 h (F), respectively, were used as the materials for RNA extraction. Data are mean values from at least five replicates. Abbreviations and locus names of the coexpression genes used here are available in Table S5.
Discussion
Wheat NOXs Are Diverse in Members and Structures With a Complicated Evolution History
As is well-known, common wheat (T. aestivum) is a hexaploid species with three closely related subgenomes (A, B, and D). In this study, a total of 46 NOX family genes, which encode 15 TaNOXs, 4 TaNOX-likes, and 4 TaFROs, were identified according to the sequence analysis and domain composition (Table S1). Phylogenetic analysis showed that the homologous genes from different subgenomes for a certain NOX protein always clustered together first, as expected (Figure 2). Interestingly, not every protein has three homologous genes distributed on the subchromosomes A, B, and D. For example, TaNOX3 includes two homologous genes (TaNOX3-1BL and -1DL) but TaNOX4 has only one (TaNOX4-1DL) (Figure 1). This means that their homologs on a certain subchromosome might be lost during the long-term evolution and natural selection. In fact, many previous studies have shown that in an allopolyploid species, the phenomenon of genovariation including gene rearrangement, structural variation, DNA sequence loss or amplification, and transposon activation occurred frequently during the polyploidization of genome (Jackson and Chen, 2010).
As suggested earlier, gene duplication, fusion, and/or exon shuffling are commonly occurred in eukaryotes for the biological diversity and functional divergence of certain gene family during evolution in plants (Morgante et al., 2005; Van de Peer et al., 2009; Kaessmann, 2010; Magadum et al., 2013). This is also involved in the expansion of NOX gene family in plants (Chang et al., 2016). The typical NOXs of wheat exhibited high similarity in the sequences of the conserved domains but obvious diversity in gene structure and protein size (Table S1, Figures 1, 2). TaFROs also showed very high similarity in sequences of the conserved domains compared with those of TaNOXs although there are still some differences in same conserved amino acids (Figure 2), implying an evolutionary relationship between TaFROs and TaNOXs. In fact, it was suggested that FRO proteins are the ancestral forms of NOXs in both animals and plants (Chang et al., 2016). Interestingly, although the structures of TaNOXs and TaFROs are conserved, they have undergone a diverse structural evolution. It can be seen that the numbers and positions of intron and exon of the genes became more and more complicated from TaFROs to TaNOXs (Figure 1), exhibiting a structural diversity of NOX family genes in wheat, and the evolutionary mechanisms such as gene duplication, gene fusion, and exon shuffling might be also involved in the evolution of wheat NOX gene family. Moreover, although TaFROs are the ancient forms of TaNOXs, the TaFROs genes located on Chr 1 and Chr 2 seem to be somewhat different in evolution and function. The TaFROs located on Chr 1 (TaFRO1 and TaFRO2) and Chr 2 (TaFRO3 and TaFRO4) could not be clustered into the same subgroup within the phylogenetic tree. The TaFROs located on Chr 1 were clustered into the small subgroup and were closer to the TaNOXs and TaNOX-likes than the TaFROs located on Chr 2 (Figure 2A), indicating their differential but complicated evolution of the two types of TaFROs. The TaFROs encoded by the genes located on Chr 2 exhibited different numbers and arrangement of transmembrane regions and different sizes of the three conserved domains compared with those located on Chr 1 (Figure 2), showing their diversity in protein structure and probably also in function. Moreover, it seems that the arrangement and similarity of Ferric_reduct domains and transmembrane regions of the TaFROs encoded by the genes located on Chr 1 are more similar to those of the typical TaNOXs, further suggesting that the TaFROs encoded by the genes located on Chr 1 perhaps are closer to TaNOXs in genetics than those on Chr 2 and the TaFROs on Chr 2 might be the more ancient forms of TaNOXs in wheat.
As reported earlier, typical NOXs only exist in terrestrial plants evolved from the ancient FROs by gaining a NADPH_Ox domain (Chang et al., 2016). The NADPH_Ox domain endowed NOX with a new function of producing ROS as a defence mechanism. Although the FROs in lower organisms are responsible for both ROS production and iron uptake (Aguirre et al., 2005), a number of increasing literatures have shown that in higher plants, NOX-mediated ROS production is mainly responsible for the stress tolerance and development regulation (Kaya et al., 2014; Gupta et al., 2017; Yamauchi et al., 2017), whereas FROs are mainly responsible for iron uptake and iron homeostasis (Sperotto et al., 2010). Furthermore, TaNOXs perhaps will become more complicated in construction; for example, TaNOX3 possessing two NADPH_Ox domains, and this is different from the others that just possesses one NADPH_Ox domain in animo acid sequences (Figure 2B). Further study is needed to check whether it has some biological significance.
The four TaNOX-likes are very special because they have the NADPH_Ox domain but lack one or two other conserved domains that the typical TaNOXs have (Figure 2), and although no typical transmembrane helixes were identified in these proteins, they are also located in the plasma membrane (Figure 3). The analysis for phylogenetic relationships showed that TaNOX-like1 and 3 are much closer to TaNOX4, whereas TaNOX-like2 is much closer to TaNOX3 and TaNOX-like4 is much closer to TaNOX15 (Figures 3, 4), indicating that they might derive from these three typical TaNOXs, respectively, probably by DNA sequence loss during evolution. As no NOX-like proteins were identified in other plant species and no experiments have been done on them, their biological functions are unclear. All this information suggest that wheat NOXs underwent a complicated evolutionary history during the gene expansion and functional divergence.
Wheat NOXs Exhibit a Great Specificity in Expression and Play Vital Roles in Both the Plant Growth Regulation and Stress Response
Specific expression is a common characteristic of the genes of a certain protein family in plants, which often reflects the cross-talk and/or difference in functions of the family members. Many studies have shown that different NOXs have different functions both in animals and in plants (Bedard and Krause, 2007; Kaye et al., 2011). In Arabidopsis, 10 NOXs were found, and these NOXs participate in different biological processes and/or stress responses with obvious tissue specificity in the gene expression (Maruta et al., 2011; Chaouch et al., 2012; Kaya et al., 2014; Xie et al., 2014). However, in wheat, although it was reported that NOXs could ameliorate the nickel-induced oxidative stress (Hao et al., 2014), to date, only two genes have been reported functioning in brown rust infection (Dmochowska-Boguta et al., 2013). In the present study, both the results obtained from database research and qRT-PCR analysis indicated that TaNOXs widely function in the plant growth regulation and stress response (Figures 5–8, Table 1). For example, TaNOX2 is expressed in a whole plant with dominantly existing in stem elongation and inflorescence emergence stages, and its transcripts were greatly affected by a set of biotic and abiotic stresses, implying its vital roles in plant growth regulation and also stress responses. TaNOX2 is more similar to Arabidopsis AtRbohD and AtRbohF and rice OsNOX2 (OsRbohA) in phylogenetics (Figure 4). AtRbohD and AtRbohF are all expressed throughout the plant (Sagi and Fluhr, 2006) and are essential not only for the hypersensitive response but also for the ABA-, JA-, and ethylene-induced signaling pathways (Suhita et al., 2004; Desikan et al., 2006; Zhang et al., 2009; Maruta et al., 2011), disease resistance (Chaouch et al., 2012), salt stress tolerance (Xie et al., 2011), and endosperm development (Penfield et al., 2006). OsNOX2 was also found to be expressed in the whole plant and plays crucial roles in both growth regulation and drought stress tolerance (Wang et al., 2016). TaNOX8 and TaNOX12 are also much close to AtRbohD, AtRbohF, and OsNOX2 (Figure 4), and as expected, the two wheat genes exhibit very similar expression profiles with TaNOX2, although they are somewhat different under a certain stress condition, implying their functional identity or coordination. In contrast, TaNOX3 showed marked tissue and/or development specific expression (Figure 5, Table 1) with a great response for many different treatments (Figures 7B, 8, Table 1), suggesting somewhat specificity in functions. TaNOX3 is much closer to Arabidopsis AtRbohB, which was found to be specifically expressed in root and elongation zone and to be involved in seed after ripening (Sagi and Fluhr, 2006; Muller et al., 2009). Similar to TaNOX3, the TaNOXs including TaNOX4, 9, 10, and 11 are also much closer to AtRbohB in phylogenetics, but these TaNOXs exhibit varied tissue and/or development expression profiles as well as the inducible expression (Figures 5, 7, 8, Table 1), implying somewhat divergence in functions among these NOX proteins. TaNOX15 also shows notable tissue- and development-specific expression with more transcripts in stems at booting stage (Figure 5, Table 1), but the transcripts were less induced by different treatments (Figure 8, Table 1). TaNOX15 has high identity in amino acid sequence with Arabdopsis AtRbohC, which was reported participating in root-hair-tip growth and specifically expressed in roots (Sagi and Fluhr, 2006; Takeda et al., 2008), which means that it has different functions in wheat as AtRbohC has in Arabidopsis.
However, TaNOX7, 8, 13, and 14 exhibit much closeness to rice OsNOX1 (OsRbohB) and OsNOX9 (OsRbohH) (Figure 4), and all of them are sensitive to biotic stresses and ABA treatments (Figures 7B, 8, Table 1), implying their possible functions in plant immunity. It was reported that OsNOX1 (OsRbohB) involves in immune response (Nagano et al., 2016), whereas OsNOX9 (OsRbohH) regulates the ethylene-induced aerenchyma formation in roots (Yamauchi et al., 2017). Furthermore, TaNOX1 and TaNOX5 are much closer to OsNOX3, which was also found to play crucial roles in plant immune response (Yoshie et al., 2005; Nagano et al., 2016). TaNOX1 and TaNOX5 having similar functions require further study. Interestingly, due to the inducible expression profiles under a set of biotic and abiotic stresses, all the TaNOXs could be divided into four subgroups (Figure 8), implying their functional divergence between the groups but coordination within the same group. However, as discussed above, different TaNOXs exhibit strong spatio-temporal and tissue-specific expressions, demonstrating an obvious cross-talk and/or divergence in both biological roles and evolutionary relationship of the NOX family genes in wheat.
Coexpression Analysis Reveals the Wide Functions But Complicated Regulatory Mechanisms of the TaNOXs in Wheat
Acting as the key producer of ROS, NOXs play vital roles in plant growth and development including root and root hair elongation (Foreman et al., 2003), endosperm development (Penfield et al., 2006), cellular vesicle cycle (Hao et al., 2014), seed after ripening (Muller et al., 2009), pollen tube growth, lipid microdomain polarization (Potocký et al., 2007; Hao et al., 2014; Nagano et al., 2016), plant-rhizobial symbiosis (Marino et al., 2011, 2012), and also stress responses as reviewed previously (Chang et al., 2016). The functional diversity was also observed in the wheat NOX gene family as discussed above. The results from our thorough coexpression analysis showed that different TaNOXs have different sets of coexpression genes against development stages, tissues, or stress conditions (Figure 9, Figures S4–S6, Table S5), implying the specific but complicated functions of NOX proteins in wheat development regulation and/or stress responses.
Corresponding to the developmental stages and tissue specificity, the coexpression genes with TaNOXs are mainly related to enzymatic glycosyl transfer, energy metabolism, substrate transport, cell wall synthesis, redox reaction, phosphorylation, and/or gene expression regulation (Figures S4, S5, Table S5), suggesting their diverse and vital function in wheat development. For example, TaNOX2 is highly coexpressed with a kinase (casein kinase 1.5) and two cellulose synthases (CESA3 and CESA5) in all the tissues examined, implying that its function may be tightly related to the cell wall synthesis and protein phosphorylation. Casein kinase 1 (CK1) plays a crucial role in regulating growth and development via phosphorylating various substrates throughout the eukaryote kingdom. In Arabidopsis, two CK1s were found to regulate blue light signaling by phosphorylating cryptochrome 2 (Tan et al., 2013). CESA3 and CESA5 are two important proteins functioning in plant cell wall formation (Griffiths et al., 2015) and also in fiber cellulose production (Li et al., 2013). Several RLK family genes and a phytosulfokin receptor 1 gene were found to have significant coexpression with TaNOX3 (Table S5), demonstrating that TaNOX3 might participate in a series of cellular signaling pathways. As reported earlier, RLKs play crucial roles in plant growth, development, and defence response by perceiving and transmitting extracellular or intracellular signals (Wu and Zhou, 2013). As the receptor of phytosulfokine (PSK), the phytosulfokin receptor 1 functions in cell growth, pollen tube guidance, and cell differentiation (Sauter, 2015). Several heavy metal transport-related proteins and DHHC-type zinc finger family proteins are highly coexpressed with TaNOX7 (Table S5), implying its functions in substrate transport and protein anchoring to the membrane. DHHC-type zinc finger family proteins were found to play crucial roles in protein palmitoylation for cell membrane anchoring (Mitchell et al., 2006). Besides being coexpressed with two CESA1-cellulose synthases, TaNOX8 also has high coexpression with a SCAMP family protein and two kinesin motor family proteins, indicating that it might not only participate in cell wall biosynthesis but also function in intracellular substance transport. SCAMP family proteins acting as carriers are responsible for the protein transport functioning to the cell surface in post-Golgi recycling pathways (Fernandez-Chacon et al., 2000), and kinesin family proteins unidirectionally transport various cargos including membranous organelles, protein complexes, and mRNA (Li et al., 2012). TaNOX12, however, is highly coexpressed with several glycosyl transfer-related proteins, demonstrating their roles in enzymatic glycosyl transfer. Moreover, two riboflavin synthase-like superfamily proteins have high coexpression with TaNOX12. Riboflavin synthases are responsible for the biosynthesis of vitamin B2, which is vital for the cellular redox balance (Fischer and Bacher, 2008). TaNOX13 is coexpressed with three CESA proteins and one CK1 protein, suggesting its functions in the cell wall formation and protein phosphorylation. It should be noticed that TaNOX12 has obvious coexpression with TaNOX2 and TaNOX8 at different development stages and in different tissues (Table S4), showing somewhat cross-talk between the function of different TaNOXs. All these results indicate the distinct but crossing functions of the TaNOXs in wheat growth and development.
The coexpression patterns of TaNOXs with other genes are greatly affected by different stresses, and different sets of genes were found to be highly coexpressed with the TaNOXs under stress conditions (Figure 9, Figure S6, Table S5), showing the vital roles of TaNOXs with complicated regulatory mechanisms during their functioning in stress tolerance. The genes that are highly coexpressed with TaNOXs under stress conditions are widely involved in stress signal transduction, cell wall formation, protein degradation, transcriptional regulations, and so on. Among the eight TaNOXs examined here, four genes including TaNOX2, 3, 8, and 13 have distinct coexpression with the genes encoding several protein degradation-related proteins including one ubiquitin-specific protease family C19-related protein, two ARM repeat superfamily proteins, one RING/U-box superfamily protein, and five COP1-interacting protein-related proteins (Figure S6, Table S5). All these coexpressed proteins function as E3 ubiquitin ligase or involve in ubiquitination of proteins (Zeng et al., 2008; Acosta et al., 2012; Xu et al., 2014), implying these TaNOXs might participate in protein degradation in wheat under stress conditions. Furthermore, a set of signal transduction-related genes were found to be highly coexpressed with TaNOXs, especially under stress conditions. At first, it was found that the genes encoding five protein kinase superfamily proteins are highly coexpressed with the TaNOXs, including TaNOX2, 7, and 13 (Table S5). These protein kinases, including an LRR family protein and two CC-NBS-LRR proteins, belong to receptor-like protein kinase (RLK) family, often functioning in signal transduction in plant cells, especially in the pathogen detection and defense response (Wu and Zhou, 2013). Moreover, it was observed that TaNOX3 is highly coexpressed with the genes encoding two BRASSINOSTEROID INSENSITIVE1 (BRI1) precursor kinases, and TaNOX7 is coexpressed with three PLC-like phosphodiesterases superfamily proteins (Figure 9, Figure S6, Table S5). BRI1 is a key component in brassinosteroid (BR) perception and signal transduction and has a broad impact on plant growth and development (Oh et al., 2012). Phospholipase C (PLC) is a class of membrane-associated enzymes playing a crucial role in signal transduction pathways (Singh et al., 2015). Our results obtained here have given much cues for the functions of TaNOXs and the possible working mechanism in stress tolerance but needs further investigation.
The coexpression of TaNOXs with several CESA proteins is another interesting event, especially under stress conditions. A total of nine CESA cellulose synthases were found to be highly coexpressed with the TaNOXs including TaNOX2, 8, and 13 (Table S5), indicating that these TaNOXs have important roles in cell wall formation both under normal and stress conditions. CESA cellulose synthases are the key enzymes for cellulose synthesis during the cell wall formation (Endler and Persson, 2011). It was also proposed that ROS, such as superoxide anion radical and hydrogen peroxide, function in cell wall loosing and stiffing, and in these processes, the NOX-mediated ROS production plays an important role (Karkonen and Kuchitsu, 2015). Furthermore, the ROS production also plays a key role in the process of cell wall remodeling under abiotic stresses (Tenhaken, 2015). All of these results suggest that some complicated regulatory mechanisms might be involved in the developmental regulation and stress response of TaNOXs in wheat.
Several other stress response-related genes were also found to be highly coexpressed with certain TaNOXs, further demonstrating the wide functions but complicated regulatory mechanisms of the TaNOXs during the plant development and stress responses. For instance, TaNOX3 is coexpressed with glutathione S-transferase tau 7, and TaNOX13 has coexpression with NAD kinase 1 (NADK1). Glutathione S-transferase (GST) is a multifunctional ROS scavenging enzyme that is involved in a variety of cellular processes. In Arabidopsis, a homolog of glutathione S-transferase tau 7 was found to be related to detoxification, secondary metabolism, and stress responses to Botrytis cinerea or Pseudomonas syringae (Fode et al., 2008). NADKs are the enzymes found in both prokaryotes and eukaryotes, generating pyridine nucleotide NADP(H) from substrates ATP and NAD(H). NADP(H) can be used as the electron donor to NOXs for generating ROS in plants (Turner et al., 2004). In Arabidopsis, NADK1 was found to be induced by environmental stresses and participated in plant pathogen interaction (Berrin et al., 2005). Moreover, the TaNOXs including TaNOX7, 12, and 13 also exhibit high coexpression with the genes encoding two O-fucosyltransferase family proteins, one starch synthase 2, and two melibiase family proteins, respectively, which all play important roles in glycometabolism. All these results suggest that the TaNOXs themselves or with their coexpression genes, synergistically or antagonistically, display pleiotropic functions in a wide range of processes of substance and energy metabolism, detoxification, substance transport, development regulation, and defence response, again implying the specific but complicated functions of the NOX genes in the wheat development and/or stress responses.
Taken together, wheat has multiple members of NOXs with diverse but vital functions in plant growth and development regulation and stress responses. Every member of TaNOXs has its specific expression pattern and function. Meanwhile, the functions of each wheat NOX and its regulatory mechanism in development regulation and defence response are still unclear. The interactions among TaNOXs and other genes are still barely reported in detail. The results obtained have provided a valuable foundation for further exploring the functions of NOX genes in wheat and in other plants as well.
Author Contributions
K-MC proposed concept. C-HH and P-QW collected data. C-HH, X-YW, and BY made the abiotic stress treatments. C-HH, X-YW, L-BY, T-TM, P-PZ, XW, W-TL, W-QL, and L-SM contributed for coexpression data. K-MC and C-HH wrote the manuscript.
Funding
This work was supported by the National Natural Science Foundation of China (grant nos. 31770204 and 31270299) and the Program for New Century Excellent Talents in University of China (NCET-11-0440).
Conflict of Interest Statement
The authors declare that the research was conducted in the absence of any commercial or financial relationships that could be construed as a potential conflict of interest.
Supplementary Material
The Supplementary Material for this article can be found online at: https://www.frontiersin.org/articles/10.3389/fpls.2018.00906/full#supplementary-material
References
Acosta, M. G., Ahumada, M. Á., Lassaga, S. L., and Casco, V. H. (2012). PUB16 gene expression under abiotic stress and their putative role as an ARM repeat protein in Arabidopsis thaliana self-pollination pathway. Adv. Biosci. Biotechnol. 3, 609–619. doi: 10.4236/abb.2012.35079
Aguirre, J., Ríos-Momberg, M., Hewitt, D., and Hansberg, W. (2005). Reactive oxygen species and development in microbial eukaryotes. Trends Microbiol. 13, 111–118. doi: 10.1016/j.tim.2005.01.007
Amicucci, E., Gaschler, K., and Ward, J. M. (1999). NADPH oxidase genes from tomato (Lycopersicon esculentum) and curly-leaf pondweed (Potamogeton crispus). Plant Biol. 1, 524–528. doi: 10.1055/s-2007-978547
Asai, S., Ohta, K., and Yoshioka, H. (2008). MAPK signaling regulates nitric oxide and NADPH oxidase-dependent oxidative bursts in Nicotiana benthamiana. Plant Cell 20, 1390–1406. doi: 10.1105/tpc.107.055855
Bedard, K., and Krause, K. H. (2007). The NOX family of ROS-generating NADPH oxidases: physiology and pathophysiology. Physiol. Rev. 87, 245–313. doi: 10.1152/physrev.00044.2005
Bedard, K., Lardy, B., and Krause, K. H. (2007). NOX family NADPH oxidases: not just in mammals. Biochimie 89, 1107–1112. doi: 10.1016/j.biochi.2007.01.012
Berrin, J. G., Pierrugues, O., Brutesco, C., Alonso, B., Montillet, J. L., Roby, D., et al. (2005). Stress induces the expression of AtNADK-1, a gene encoding a NAD(H) kinase in Arabidopsis thaliana. Mol. Genet. Genomics 273, 10–19. doi: 10.1007/s00438-005-1113-1
Chang, Y. L., Li, W. Y., Miao, H., Yang, S. Q., Li, R., Wang, X., et al. (2016). Comprehensive genomic analysis and expression profiling of the NOX gene families under abiotic stresses and hormones in plants. Genome Biol. Evol. 8, 791–810. doi: 10.1093/gbe/evw035
Chaouch, S., Queval, G., and Noctor, G. (2012). AtRbohF is a crucial modulator of defence-associated metabolism and a key actor in the interplay between intracellular oxidative stress and pathogenesis responses in Arabidopsis. Plant J. 69, 613–627. doi: 10.1111/j.1365-313X.2011.04816.x
Delledonne, M., Murgia, I., Ederle, D., Sbicego, P. F., Biondani, A., Polverari, A., et al. (2002). Reactive oxygen intermediates modulate nitric oxide signaling in the plant hypersensitive disease-resistance response. Plant Physiol. Biochem. 40, 605–610. doi: 10.1016/S0981-9428(02)01397-9
Denness, L., McKenna, J. F., Segonzac, C., Wormit, A., Madhou, P., Bennett, M., et al. (2011). Cell wall damage-induced lignin biosynthesis is regulated by a reactive oxygen species- and jasmonic acid-dependent process in Arabidopsis. Plant Physiol. 156, 1364–1374. doi: 10.1104/pp.111.175737
Desikan, R., Last, K., Harrett-Williams, R., Tagliavia, C., Harter, K., Hooley, R., et al. (2006). Ethylene-induced stomatal closure in Arabidopsis occurs via AtrbohF-mediated hydrogen peroxide synthesis. Plant J. 47, 907–916. doi: 10.1111/j.1365-313X.2006.02842.x
Dietz, K. J., Mittler, R., and Noctor, G. (2016). Recent progress in understanding the role of reactive oxygen species in plant cell signaling. Plant Physiol. 171, 1535–1539. doi: 10.1104/pp.16.00938
Dmochowska-Boguta, M., Nadolska-Orczyk, A., and Orczyk, W. (2013). Roles of peroxidases and NADPH oxidases in the oxidative response of wheat (Triticum aestivum) to brown rust (Puccinia triticina) infection. Plant Pathol. 62, 993–1002. doi: 10.1111/ppa.12009
Dubiella, U., Seybold, H., Durian, G., Komander, E., Lassig, R., Witte, C. P., et al. (2013). Calcium-dependent protein kinase/NADPH oxidase activation circuit is required for rapid defense signal propagation. Proc. Natl. Acad. Sci. U.S.A. 110, 8744–8749. doi: 10.1073/pnas.1221294110
Endler, A., and Persson, S. (2011). Cellulose synthases and synthesis in Arabidopsis. Mol. Plant 4, 199–211. doi: 10.1093/mp/ssq079
Fernández-Chacón, R., Achiriloaie, M., Janz, R., Albanesi, J. P., and Südhof, T. C. (2000). SCAMP1 function in endocytosis. J. Biol. Chem. 275, 12752–12756. doi: 10.1074/jbc.275.17.12752
Fischer, M., and Bacher, A. (2008). Biosynthesis of vitamin B2: structure and mechanism of riboflavin synthase. Arch. Biochem. Biophys. 474, 252–265. doi: 10.1016/j.abb.2008.02.008
Fode, B., Siemsen, T., Thurow, C., Weigel, R., and Gatz, C. (2008). The Arabidopsis GRAS protein SCL14 interacts with class II TGA transcription factors and is essential for the activation of stress-inducible promoters. Plant Cell 20, 3122–3135. doi: 10.1105/tpc.108.058974
Foreman, J., Demidchik, V., Bothwell, J. H., Mylona, P., Miedema, H., Torres, M. A., et al. (2003). Reactive oxygen species produced by NADPH oxidase regulate plant cell growth. Nature 422, 442–446. doi: 10.1038/nature01485
Geiszt, M. (2006). NADPH oxidases: new kids on the block. Cardiovasc. Res. 71, 289–299. doi: 10.1016/j.cardiores.2006.05.004
Griffiths, J. S., Šola, K., Kushwaha, R., Lam, P., Tateno, M., Young, R., et al. (2015). Unidirectional movement of cellulose synthase complexes in Arabidopsis seed coat epidermal cells deposit cellulose involved in mucilage extrusion, adherence, and ray formation. Plant Physiol. 168, 502–520. doi: 10.1104/pp.15.00478
Gu, L., Han, Z., Zhang, L., Downie, B., and Zhao, T. (2013). Functional analysis of the 5' regulatory region of the maize GALACTINOL SYNTHASE2 gene. Plant Sci. 213, 38–45. doi: 10.1016/j.plantsci.2013.09.002
Gupta, D. K., Pena, L. B., Romero-Puertas, M. C., Hernández, A., Inouhe, M., and Sandalio, L. M. (2017). NADPH oxidases differentially regulate ROS metabolism and nutrient uptake under cadmium toxicity. Plant Cell Environ. 40, 509–526. doi: 10.1111/pce.12711
Hao, H., Fan, L., Chen, T., Li, R. L., Li, X. J., He, Q., et al. (2014). Clathrin and membrane microdomains cooperatively regulate RbohD dynamics and activity in Arabidopsis. Plant Cell 26, 1729–1745. doi: 10.1105/tpc.113.122358
Jackson, S., and Chen, Z. J. (2010). Genomic and expression plasticity of polyploidy. Curr. Opin. Plant Biol. 13, 153–159. doi: 10.1016/j.pbi.2009.11.004
Kaessmann, H. (2010). Origins, evolution, and phenotypic impact of new genes. Genome Res. 20, 1313–1326. doi: 10.1101/gr.101386.109
Kärkönen, A., and Kuchitsu, K. (2015). Reactive oxygen species in cell wall metabolism and development in plants. Phytochemistry 112, 22–32. doi: 10.1016/j.phytochem.2014.09.016
Kaya, H., Nakajima, R., Iwano, M., Kanaoka, M. M., Kimura, S., Takeda, S., et al. (2014). Ca2+-activated reactive oxygen species production by Arabidopsis RbohH and RbohJ is essential for proper pollen tube tip growth. Plant Cell 26, 1069–1080. doi: 10.1105/tpc.113.120642
Kaye, Y., Golani, Y., Singer, Y., Leshem, Y., Cohen, G., Ercetin, M., et al. (2011). Inositol polyphosphate 5-phosphatase7 regulates the production of reactive oxygen species and salt tolerance in Arabidopsis. Plant Physiol. 157, 229–241. doi: 10.1104/pp.111.176883
Lescot, M., Déhais, P., Thijs, G., Marchal, K., Moreau, Y., Van de Peer, Y., et al. (2002). PlantCARE, a database of plant cis-acting regulatory elements and a portal to tools for in silico analysis of promoter sequences. Nucleic Acids Res. 30, 325–327. doi: 10.1093/nar/30.1.325
Li, A., Xia, T., Xu, W., Chen, T., Li, X., Fan, J., et al. (2013). An integrative analysis of four CESA isoforms specific for fiber cellulose production between Gossypium hirsutum and Gossypium barbadense. Planta 237, 1585–1597. doi: 10.1007/s00425-013-1868-2
Li, J., Wang, X., Zhang, Y., Jia, H., and Bi, Y. (2011). cGMP regulates hydrogen peroxide accumulation in calcium-dependent salt resistance pathway in Arabidopsis thaliana roots. Planta 234, 709–722. doi: 10.1007/s00425-011-1439-3
Li, J., Xu, Y., and Chong, K. (2012). The novel functions of kinesin motor proteins in plants. Protoplasma 249(Suppl. 2), S95–S100. doi: 10.1007/s00709-011-0357-3
Li, W. Y., Wang, X., Li, R., Li, W. Q., and Chen, K. M. (2014). Genome-wide analysis of the NADK gene family in plants. PLoS ONE 9:e101051. doi: 10.1371/journal.pone.0101051
Magadum, S., Banerjee, U., Murugan, P., Gangapur, D., and Ravikesavan, R. (2013). Gene duplication as a major force in evolution. J. Genet. 92, 155–161. doi: 10.1007/s12041-013-0212-8
Marino, D., Andrio, E., Danchin, E. G., Oger, E., Gucciardo, S., Lambert, A., et al. (2011). A Medicago truncatula NADPH oxidase is involved in symbiotic nodule functioning. New Phytol. 189, 580–592. doi: 10.1111/j.1469-8137.2010.03509.x
Marino, D., Dunand, C., Puppo, A., and Pauly, N. (2012). A burst of plant NADPH oxidases. Trends Plant Sci. 17, 9–15. doi: 10.1016/j.tplants.2011.10.001
Maruta, T., Inoue, T., Tamoi, M., Yabuta, Y., Yoshimura, K., Ishikawa, T., et al. (2011). Arabidopsis NADPH oxidases, AtrbohD and AtrbohF, are essential for jasmonic acid-induced expression of genes regulated by MYC2 transcription factor. Plant Sci. 180, 655–660. doi: 10.1016/j.plantsci.2011.01.014
Miller, G., Schlauch, K., Tam, R., Cortes, D., Torres, M. A., Shulaev, V., et al. (2009). The plant NADPH oxidase RBOHD mediates rapid systemic signaling in response to diverse stimuli. Sci. Signal. 2, ra45. doi: 10.1126/scisignal.2000448
Mitchell, D. A., Vasudevan, A., Linder, M. E., and Deschenes, R. J. (2006). Protein palmitoylation by a family of DHHC protein S-acyltransferases. J. Lipid Res. 47, 1118–1127. doi: 10.1194/jlr.R600007-JLR200
Mittler, R., Vanderauwera, S., Suzuki, N., Miller, G., Tognetti, V. B., Vandepoele, K., et al. (2011). ROS signaling: the new wave? Trends Plant Sci. 16, 300–309. doi: 10.1016/j.tplants.2011.03.007
Morgante, M., Brunner, S., Pea, G., Fengler, K., Zuccolo, A., and Rafalski, A. (2005). Gene duplication and exon shuffling by helitron-like transposons generate intraspecies diversity in maize. Nat. Genet. 37, 997–1002. doi: 10.1038/ng1615
Müller, K., Carstens, A. C., Linkies, A., Torres, M. A., and Leubner-Metzger, G. (2009). The NADPH-oxidase AtrbohB plays a role in Arabidopsis seed after-ripening. New Phytol. 184, 885–897. doi: 10.1111/j.1469-8137.2009.03005.x
Nagano, M., Ishikawa, T., Fujiwara, M., Fukao, Y., Kawano, Y., Kawai-Yamada, M., et al. (2016). Plasma membrane microdomains are essential for Rac1-RbohB/H-mediated immunity in rice. Plant Cell 28, 1966–1983. doi: 10.1105/tpc.16.00201
Nakashima, A., Chen, L., Thao, N. P., Fujiwara, M., Wong, H. L., Kuwano, M., et al. (2008). RACK1 functions in rice innate immunity by interacting with the Rac1 immune complex. Plant Cell 20, 2265–2279. doi: 10.1105/tpc.107.054395
Nestler, J., Liu, S., Wen, T. J., Paschold, A., Marcon, C., Tang, H. M., et al. (2014). Roothairless5, which functions in maize (Zea mays L.) root hair initiation and elongation encodes a monocot-specific NADPH oxidase. Plant J. 79, 729–740. doi: 10.1111/tpj.12578
Oh, M. H., Kim, H. S., Wu, X., Clouse, S. D., Zielinski, R. E., and Huber, S. C. (2012). Calcium/calmodulin inhibition of the Arabidopsis BRASSINOSTEROID-INSENSITIVE 1 receptor kinase provides a possible link between calcium and brassinosteroid signalling. Biochem. J. 443, 515–523. doi: 10.1042/BJ20111871
Penfield, S., Li, Y., Gilday, A. D., Graham, S., and Graham, I. A. (2006). Arabidopsis ABA INSENSITIVE4 regulates lipid mobilization in the embryo and reveals repression of seed germination by the endosperm. Plant Cell 18, 1887–1899. doi: 10.1105/tpc.106.041277
Potocký, M., Jones, M. A., Bezvoda, R., Smirnoff, N., and Zárský, V. (2007). Reactive oxygen species produced by NADPH oxidase are involved in pollen tube growth. New Phytol. 174, 742–751. doi: 10.1111/j.1469-8137.2007.02042.x
Rajhi, I., Yamauchi, T., Takahashi, H., Nishiuchi, S., Shiono, K., Watanabe, R., et al. (2011). Identification of genes expressed in maize root cortical cells during lysigenous aerenchyma formation using laser microdissection and microarray analyses. New Phytol. 190, 351–368. doi: 10.1111/j.1469-8137.2010.03535.x
Sagi, M., and Fluhr, R. (2006). Production of reactive oxygen species by plant NADPH oxidases. Plant Physiol. 141, 336–340. doi: 10.1104/pp.106.078089
Sauter, M. (2015). Phytosulfokine peptide signalling. J. Exp. Bot. 66, 5160–5168. doi: 10.1093/jxb/erv071
Singh, A., Bhatnagar, N., Pandey, A., and Pandey, G. K. (2015). Plant phospholipase C family: regulation and functional role in lipid signaling. Cell Calcium 58, 139–146. doi: 10.1016/j.ceca.2015.04.003
Song, C. J., Steinebrunner, I., Wang, X., Stout, S. C., and Roux, S. J. (2006). Extracellular ATP induces the accumulation of superoxide via NADPH oxidases in Arabidopsis. Plant Physiol. 140, 1222–1232. doi: 10.1104/pp.105.073072
Sperotto, R. A., Boff, T., Duarte, G. L., Santos, L. S., Grusak, M. A., and Fett, J. P. (2010). Identification of putative target genes to manipulate Fe and Zn concentrations in rice grains. J. Plant Physiol. 167, 1500–1506. doi: 10.1016/j.jplph.2010.05.003
Suhita, D., Raghavendra, A. S., Kwak, J. M., and Vavasseur, A. (2004). Cytoplasmic alkalization precedes reactive oxygen species production during methyl jasmonate- and abscisic acid-induced stomatal closure. Plant Physiol. 134, 1536–1545. doi: 10.1104/pp.103.032250
Suzuki, N., Miller, G., Morales, J., Shulaev, V., Torres, M. A., and Mittler, R. (2011). Respiratory burst oxidases: the engines of ROS signaling. Curr. Opin. Plant Biol. 14, 691–699. doi: 10.1016/j.pbi.2011.07.014
Takeda, S., Gapper, C., Kaya, H., Bell, E., Kuchitsu, K., and Dolan, L. (2008). Local positive feedback regulation determines cell shape in root hair cells. Science 319, 1241–1244. doi: 10.1126/science.1152505
Tan, S. T., Dai, C., Liu, H. T., and Xue, H. W. (2013). Arabidopsis casein kinase1 proteins CK1.3 and CK1.4 phosphorylate cryptochrome2 to regulate blue light signaling. Plant Cell 25, 2618–2632. doi: 10.1105/tpc.113.114322
Tenhaken, R. (2015). Cell wall remodeling under abiotic stress. Front. Plant Sci. 5:771. doi: 10.3389/fpls.2014.00771
Turner, W. L., Waller, J. C., Vanderbeld, B., and Snedden, W. A. (2004). Cloning and characterization of two NAD kinases from Arabidopsis. Identification of a calmodulin binding isoform. Plant Physiol. 135, 1243–1255. doi: 10.1104/pp.104.040428
Van de Peer, Y., Fawcett, J. A., Proost, S., Sterck, L., and Vandepoele, K. (2009). The flowering world: a tale of duplications. Trends Plant Sci. 14, 680–688. doi: 10.1016/j.tplants.2009.09.001
Wang, G. F., Li, W. Q., Li, W. Y., Wu, G. L., Zhou, C. Y., and Chen, K. M. (2013). Characterization of rice NADPH oxidase genes and their expression under various environmental conditions. Int. J. Mol. Sci. 14, 9440–9458. doi: 10.3390/ijms14059440
Wang, X., Zhang, M. M., Wang, Y. J., Gao, Y. T., Li, R., Wang, G. F., et al. (2016). The plasma membrane NADPH oxidase OsRbohA plays a crucial role in developmental regulation and drought-stress response in rice. Physiol. Plant. 156, 421–443. doi: 10.1111/ppl.12389
Wong, H. L., Pinontoan, R., Hayashi, K., Tabata, R., Yaeno, T., Hasegawa, K., et al. (2007). Regulation of rice NADPH oxidase by binding of Rac GTPase to its N-terminal extension. Plant Cell 19, 4022–4034. doi: 10.1105/tpc.107.055624
Wu, Y., and Zhou, J. M. (2013). Receptor-like kinases in plant innate immunity. J. Integr. Plant Biol. 55, 1271–1286. doi: 10.1111/jipb.12123
Wudick, M. M., and Feijo, J. A. (2014). At the intersection: merging Ca2+ and ROS signaling pathways in pollen. Mol. Plant 7, 1595–1597. doi: 10.1093/mp/ssu096
Xie, H. T., Wan, Z. Y., Li, S., and Zhang, Y. (2014). Spatiotemporal production of reactive oxygen species by NADPH oxidase is critical for tapetal programmed cell death and pollen development in Arabidopsis. Plant Cell 26, 2007–2023. doi: 10.1105/tpc.114.125427
Xie, Y. J., Xu, S., Han, B., Wu, M. Z., Yuan, X. X., Han, Y., et al. (2011). Evidence of Arabidopsis salt acclimation induced by up-regulation of HY1 and the regulatory role of RbohD-derived reactive oxygen species synthesis. Plant J. 66, 280–292. doi: 10.1111/j.1365-313X.2011.04488.x
Xu, D., Lin, F., Jiang, Y., Huang, X., Li, J., Ling, J., et al. (2014). The RING-finger E3 ubiquitin ligase COP1 SUPPRESSOR1 negatively regulates COP1 abundance in maintaining COP1 homeostasis in dark-grown Arabidopsis seedlings. Plant Cell 26, 1981–1991. doi: 10.1105/tpc.114.124024
Yamauchi, T., Yoshioka, M., Fukazawa, A., Mori, H., Nishizawa, N. K., Tsutsumi, N., et al. (2017). An NADPH oxidase RBOH functions in rice roots during lysigenous aerenchyma formation under oxygen-deficient conditions. Plant Cell 29, 775–790. doi: 10.1105/tpc.16.00976
Yoshie, Y., Goto, K., Takai, R., Iwano, M., Takayama, S., Isogai, A., et al. (2005). Function of the rice gp91phox homologs OsrbohA and OsrbohE genes in ROS-dependent plant immune responses. Plant Biotechnol. 22, 127–135. doi: 10.5511/plantbiotechnology.22.127
Yoshioka, H., Numata, N., Nakajima, K., Katou, S., Kawakita, K., Rowland, O., et al. (2003). Nicotiana benthamiana gp91phox homologs NbrbohA and NbrbohB participate in H2O2 accumulation and resistance to Phytophthora infestans. Plant Cell 15, 706–718. doi: 10.1105/tpc.008680
Yoshioka, H., Sugie, K., Park, H. J., Maeda, H., Tsuda, N., Kawakita, K., et al. (2001). Induction of plant gp91phox homolog by fungal cell wall, arachidonic acid, and salicylic acid in potato. Mol. Plant Microbe Interact. 14, 725–736. doi: 10.1094/MPMI.2001.14.6.725
Zeng, L. R., Park, C. H., Venu, R. C., Gough, J., and Wang, G. L. (2008). Classification, expression pattern, and E3 ligase activity assay of rice U-box-containing proteins. Mol. Plant 1, 800–815. doi: 10.1093/mp/ssn044
Zhang, Y., Zhu, H., Zhang, Q., Li, M., Yan, M., Wang, R., et al. (2009). Phospholipase dalpha1 and phosphatidic acid regulate NADPH oxidase activity and production of reactive oxygen species in ABA-mediated stomatal closure in Arabidopsis. Plant Cell 21, 2357–2377. doi: 10.1105/tpc.108.062992
Keywords: wheat (Triticum aestivum), NADPH oxidases, phylogenetic analysis, abiotic/biotic stress response, coexpression analysis
Citation: Hu C-H, Wei X-Y, Yuan B, Yao L-B, Ma T-T, Zhang P-P, Wang X, Wang P-Q, Liu W-T, Li W-Q, Meng L-S and Chen K-M (2018) Genome-Wide Identification and Functional Analysis of NADPH Oxidase Family Genes in Wheat During Development and Environmental Stress Responses. Front. Plant Sci. 9:906. doi: 10.3389/fpls.2018.00906
Received: 26 February 2018; Accepted: 08 June 2018;
Published: 23 July 2018.
Edited by:
Viswanathan Chinnusamy, Indian Agricultural Research Institute (ICAR), IndiaReviewed by:
Zhaoqing Chu, Shanghai Chenshan Plant Science Research Center (CAS), ChinaBaris Uzilday, Ege University, Turkey
Copyright © 2018 Hu, Wei, Yuan, Yao, Ma, Zhang, Wang, Wang, Liu, Li, Meng and Chen. This is an open-access article distributed under the terms of the Creative Commons Attribution License (CC BY). The use, distribution or reproduction in other forums is permitted, provided the original author(s) and the copyright owner(s) are credited and that the original publication in this journal is cited, in accordance with accepted academic practice. No use, distribution or reproduction is permitted which does not comply with these terms.
*Correspondence: Kun-Ming Chen, a3VubWluZ2NoZW5AbndzdWFmLmVkdS5jbg==; a3VubWluZ2NoZW5AMTYzLmNvbQ==
†These authors have contributed equally to this work.