- 1Centre for Cardiovascular Science, The Queen's Medical Research Institute, The University of Edinburgh, Edinburgh, United Kingdom
- 2Department of Molecular Genetics, Faculty of Science and Engineering, Maastricht University, Maastricht, Netherlands
- 3Faculty of Health, Medicine and Life Sciences, Cardiovascular Research Institute Maastricht, Maastricht University, Maastricht, Netherlands
Cardiovascular disease (CVD) is the leading cause of mortality worldwide claiming almost 17. 9 million deaths annually. A primary cause is atherosclerosis within the coronary arteries, which restricts blood flow to the heart muscle resulting in myocardial infarction (MI) and cardiac cell death. Despite substantial progress in the management of coronary heart disease (CHD), there is still a significant number of patients developing chronic heart failure post-MI. Recent research has been focused on promoting neovascularisation post-MI with the ultimate goal being to reduce the extent of injury and improve function in the failing myocardium. Cardiac cell transplantation studies in pre-clinical models have shown improvement in cardiac function; nonetheless, poor retention of the cells has indicated a paracrine mechanism for the observed improvement. Cell communication in a paracrine manner is controlled by various mechanisms, including extracellular vesicles (EVs). EVs have emerged as novel regulators of intercellular communication, by transferring molecules able to influence molecular pathways in the recipient cell. Several studies have demonstrated the ability of EVs to stimulate angiogenesis by transferring microRNA (miRNA, miR) molecules to endothelial cells (ECs). In this review, we describe the process of neovascularisation and current developments in modulating neovascularisation in the heart using miRNAs and EV-bound miRNAs. Furthermore, we critically evaluate methods used in cell culture, EV isolation and administration.
Introduction
CVD is the leading cause of mortality worldwide with the World Health Organization (WHO) reporting that in 2016, 17.9 million people died from CVDs. Of those deaths, an estimated 7.4 million were attributed to CHD alone (WHO Cardiovascular diseases CVDs, 2017). CHD is characterised by narrowing of the coronary arteries due to the gradual formation and the subsequent rupture of plaque within the vessel walls. Blockage of these arteries results in oxygen and nutrient deprivation of the downstream tissue. Consequently, ischaemic damage and cardiomyocyte death occur in the affected region of the heart, a phenomenon known as MI (Thygesen et al., 2019). Various methods, including stem cell (SC) injection, growth factor (GF) delivery, and miRNA treatment, have been proposed to reduce cardiomyocyte death; nonetheless, several limitations have restricted their use. The idea of restoring the lost myocardium was proven to be inefficient since adult mammal cardiomyocytes are terminally differentiated and in contrast to other vertebrate species, such as zebrafish, adult mammal cardiac regenerative capacity is limited (Ye et al., 2018). For this reason, SC injection was proposed as a promising strategy for cardiac repair. The initial hypothesis was that transplanted SCs would differentiate into cardiomyocytes, integrate into the host myocardium, and augment cardiac function through synchronised electromechanical coupling (Garbern and Lee, 2013; Lemcke et al., 2018). Despite some encouraging results, this approach has proven to be largely unsuccessful to date due to several issues, including poor engraftment, immune rejection, genetic instability, and possible teratocarcinoma formation. Importantly, the engrafted cells have also demonstrated relatively poor electrical coupling with the host myocardium, leading to the development of additional safety issues (Liu et al., 2018; Menasché et al., 2018; Romagnuolo et al., 2019). Recently, Vagnozzi et al. (2020), showed that the improvement in cardiac function following SC transplantation may be due to an acute inflammatory wound-healing response, rather than incorporation of delivered cells or production of new cardiomyocytes.
Aiming to improve recovery after MI and, therefore, rescue as many cardiomyocytes as possible, research interest is focused on promoting neovascularisation to create new myocardial blood vessel networks. Paracrine cell communication plays a critical role in the control of this process (Gnecchi et al., 2008; Mirotsou et al., 2011; Hodgkinson et al., 2016). Cell communication in a paracrine manner is regulated by several mechanisms, including EVs. EVs carry and transfer various bioactive molecules, such as small non-coding RNAs, proteins, and lipids, that moderate signalling pathways in the recipient cells. These functional contents depend on several factors, including the cellular origin and the (patho)physiological state of the cells during EV packing and secretion (Raposo and Stoorvogel, 2013). Recently, EV-miRNAs have gained immense interest in the control of cell behaviour in the recipient cells. miRNAs are small non-coding RNAs of approximately 22 nucleotides and have been recognised as critical regulators of gene expression. While most miRNAs regulate gene expression by binding to the 3′ untranslated region of their messenger RNA (mRNA) target, there are also sporadic reports of miRNAs that inhibit protein translation by binding to the 5′ untranslated region of their target. Under certain conditions, miRNAs can also control transcription or activate translation (O'Brien et al., 2018). Nonetheless, there is great controversy regarding the functional relevance of miRNAs in EVs. Several reports suggest that the majority of extracellular miRNAs are protected from plasma ribonucleases by forming complexes with proteins, such as Ago2 and that only a few copies of each miRNA are found in EVs (Arroyo et al., 2011; Chevillet et al., 2014). Moreover, in a non-peer-reviewed study, it was recently argued that miRNAs are not effectively delivered to target cells in a functional manner (Albanese et al., 2020). On the other hand, numerous studies have demonstrated that protected from plasma ribonucleases by their EV carriers, functional miRNAs can be delivered and internalised into recipient cells, acting as novel regulators of gene expression by inhibiting their targets (Johnson et al., 2019; Qiao et al., 2019; Cavallari et al., 2020). Despite this controversy, preclinical studies have demonstrated that EVs hold promise in the regulation of complex processes such as neovascularisation. In this review, we describe the process of neovascularisation and current developments in modulating neovascularisation in the heart using miRNAs and EV-bound miRNAs. Furthermore, we critically evaluate methods used in cell culture, EV isolation and routes of EV administration.
Post-ischaemic Neovascularisation
After the onset of myocardial ischaemia due to coronary artery occlusion, there is an inadequate blood supply to the heart muscle, which results in a pathological change in electrical, contractile or biochemical function of the heart (Grover, 1995). Therefore, post-ischaemic neovascularisation is essential to support the metabolic needs of cardiac cell populations. Angiogenesis and arteriogenesis are regulated by distinct, but partially overlapping, cellular and molecular mechanisms and are responsible for tissue repair and remodelling in acute and chronic ischaemic diseases (Persson and Buschmann, 2011). Angiogenesis refers to the formation of new blood vessels from pre-existing vessels and can be classified as intussusceptive or sprouting angiogenesis (Caduff et al., 1986; Burri and Tarek, 1990; Risau, 1997). Intussusceptive angiogenesis is a dynamic splitting process in which elements of interstitial tissues invade existing blood vessels forming a cylindrical microstructure that spans the lumen of capillaries and small vessels (Short, 1950; Caduff et al., 1986).
As implied by its name, sprouting angiogenesis is characterised by EC sprouts, which usually grow toward an angiogenic stimulus. Hypoxia is one of the key drivers of this process. The primary mechanism of hypoxia-induced neovascularisation involves an increase in hypoxia-inducible factor (HIF-1a) levels (Figure 1). When oxygen-sensing mechanisms detect low oxygen levels, new blood vessels are required to satisfy the metabolic requirements of parenchymal cells. Most of these cells respond to hypoxic stimuli by secreting a critical proangiogenic growth factor, vascular endothelial growth factor (VEGF-A). A particular type of EC, known as tip cells, leads sprouting angiogenesis (Gerhardt et al., 2003). The filopodia of endothelial tip cells are endowed with VEGF-A receptors (VEGFR2) that allow them to sense changes in VEGF-A concentrations and drive them to align with the VEGF gradient. Neighbouring cells, known as stalk cells, divide as they follow behind a tip cell, elongating the new vessel. Maturation and stabilisation of the vessels involve the recruitment of pericytes and ECM deposition along with mechanical stimuli (Stratman and Davis, 2012). This process is highly conserved and regulated by very specific molecular pathways, such as the Notch signalling pathway. In response to VEGF-A, the expression of the ligand Dll4 increases on the surface of tip cells. As a result, these ligands bind to Notch1 receptors of adjacent cells and activate Notch signalling pathway that suppresses tip cell fate and induces the stalk cell phenotype in these cells (Del Toro et al., 2010).
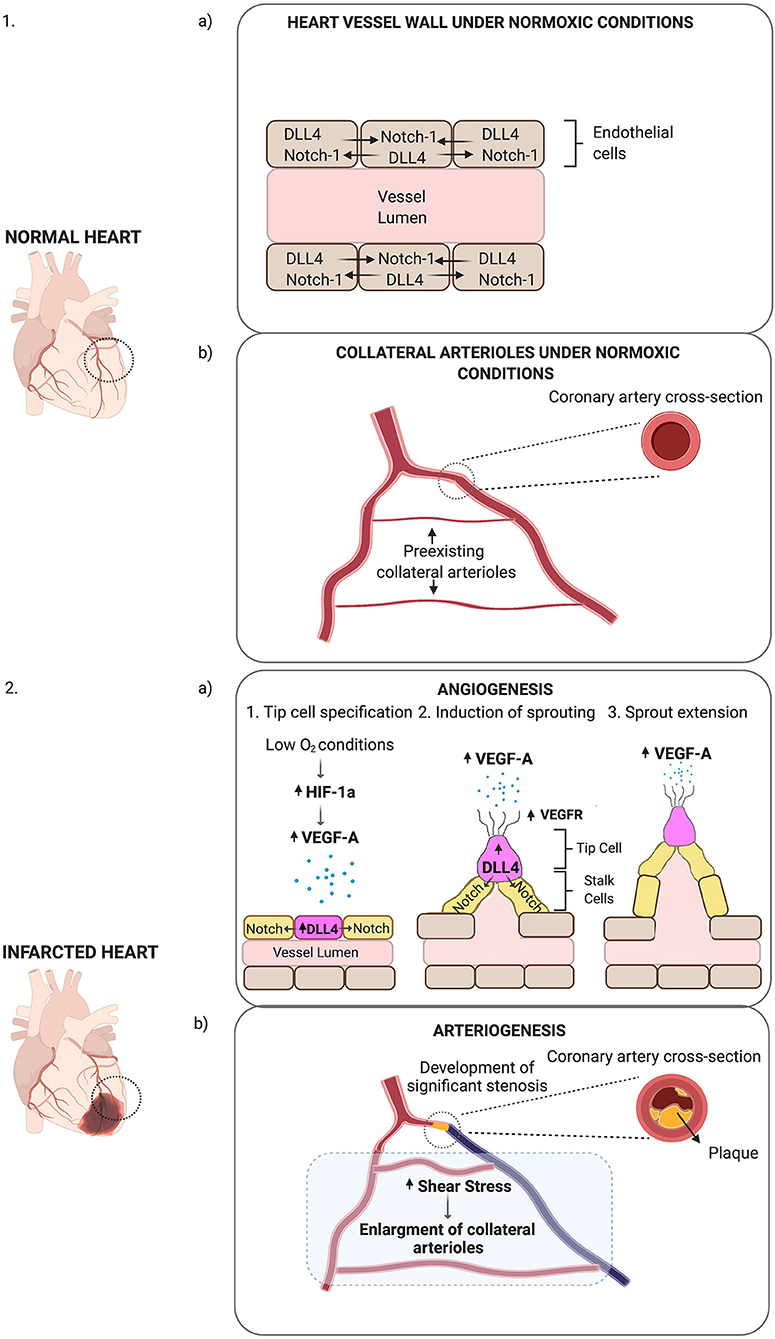
Figure 1. Schematic representation of post-ischaemic neovascularisation. (1a) Under normoxic conditions, DLL4-Notch-1 signalling is balanced in ECs. (1b) Under normoxic conditions, small collateral arterioles may connect main arteries. (2a) Low O2 conditions increase HIF-1a levels, which in turn stimulates the expression of VEGF-A (represented as small blue dots). In response to VEGF-A, Dll4 expression increases on the surface of tip cells (purple cell). Dll4 receptors on tip cells bind to Notch receptors on the surface of stalk cells (yellow cells) activating Dll4/Notch signalling pathway. Dll4—Notch-1 signalling directs ECs into migrating tip or proliferating stalk cells. In response to VEGF-A, VEGFR expression increases on the filopodia of tip cells (represented as protrusions on the tip cell), which then migrate towards VEGF-A with the stalk cells proliferating behind them. This results in the expansion of the vessel lumen and the formation of sprouts on the vessel wall (angiogenesis). (2b) When a coronary artery is occluded due to the formation of plaque within the arterial wall, there is increased shear stress in the collateral arterioles. In response to shear stress, collateral arteriole diameter increases in size (arteriogenesis).
While angiogenesis is stimulated by low-oxygen concentrations and leads to the creation of new capillaries, arteriogenesis is induced by physical forces; mainly fluid shear stress. Arteriogenesis is an adaptive response to transient, repetitive occlusion of a large main artery and is characterised by an enlargement of the collateral arterioles (Figure 1). Once a main artery is occluded the arterial pressure behind the stenosis site decreases, and the blood is redistributed via the collateral arterioles. Thus, collateral arterioles now connect a high-pressure with a low-pressure region (Schaper and Pasyk, 1976). While hypoxia-induced pathways, as well as alterations in haemodynamic forces of the vascular wall, have been proposed as critical regulators of vessel growth after an ischaemic event, emerging evidence supports the idea that tissue-resident EPCs may also be involved in cardiac neovascularisation post-ischaemia (Fujisawa et al., 2019). A better understanding of the cellular frameworks and molecular mechanisms that drive these processes is crucial for the development of new therapeutic neovascularisation strategies.
The use of animal models is essential for a better understanding of the neovascularisation process post-MI at a cellular level. Aiming to identify the mechanism of EC proliferation post-ischaemia, Manavski et al. (2018), utilised Confetti fl/wild type (wt) cadherin 5 (Cdh5)- CreERT2 mice and revealed that ischaemia-induced vascularisation post-acute myocardial infarction (AMI) is mediated by clonally expanded ECs. At day 7 post-AMI 28% of the cardiac sections showed significant clonal expansion of Cdh5-expressing ECs, and this was increased to 33% on day 14. Since Cdh5 is also expressed in bone marrow, the origin of these clonally expanded cells remained inconclusive. Recently, Li et al. (2019) utilised a Pdgfb-iCreER -R26R-Brainbow2.1 mouse. In this system, the expression of Cre is driven by a Pdgfb promoter, which is specific to ECs (Claxton et al., 2008). Using this mouse, they demonstrated that vessel formation and clonal expansion of cardiac ECs was mediated by a subpopulation of resident cardiac ECs with progenitor-like properties.
Genetic lineage tracing has significantly improved our understanding of the neovascularisation process in the post-ischaemic heart. Nonetheless, cardiac neovascularisation potential is limited and does not appear to effectively promote myocardial regeneration. Recently, Kocijan et al. (2020), used an Apln-CreER;R26mT/mG mouse model to compare the angiogenic potential of the heart and skeletal muscle. Apln is highly expressed in ECs during embryonic development and is down-regulated in adulthood. However, in response to hypoxia, under tissue ischaemia or in the context of a tumour, the expression of Apln is reactivated, particularly in tip cells. Using this system, the authors showed that different pro-angiogenic stimuli activated Apln in skeletal muscle, resulting in angiogenic sprouts that could be incorporated into arteries. In the heart, however, Apln+ cells failed to give rise to new vessels. To confirm these data, the authors implanted cancer cells in different organs and showed that the angiogenic response in the heart was reduced. These data confirm that the inherent angiogenic response of the cardiac muscle is limited, emphasising the need for new therapeutic approaches to promote endogenous neovascularisation.
MicroRNAs in Therapeutic Neovascularisation
Over the past few years, miRNAs have gained widespread attention for their role in vascular health and disease, including in neovascularisation. MiRNAs are small (18–22 nucleotide, nt) endogenous non-coding RNA molecules that negatively regulate gene expression by targeting specific mRNAs. Most target sites on mRNAs only share a partial complementarity with their corresponding miRNAs, and thus, a single miRNA can target multiple mRNAs, contributing to biological and pathophysiological processes (Huntzinger and Izaurralde, 2011).
Emerging evidence suggests that miRNAs are critical regulators of both adaptive and maladaptive vascular remodelling and angiogenesis. Table 1 contains a list of all known miRNAs that play a role in cardiovascular neovascularisation as well as their experimentally confirmed targets. Some of these have been extensively studied. MiR-126, for instance, is one of the most abundantly expressed miRNAs in ECs and has a prominent role in controlling angiogenesis by repressing negative regulators of the VEGF pathway, such as the Sprouty-related protein SPRED1 and phosphoinositol-3 kinase regulatory subunit 2 (PIK3R2/p85-beta) (Fish et al., 2008; Wang et al., 2008; Schober et al., 2014). Wang et al. (2008), showed that targeted deletion of miR-126 in mice leads to leaky vessels, haemorrhage and embryonic lethality due to defective vascular integrity. Half of the animals survived 1 week post-MI, while almost all died within 3 weeks post-MI. Another miRNA with angiogenic properties is miR-210. MiR-210 upregulation is a principal element of EC response to hypoxia (Fasanaro et al., 2009). Hu et al. (2010), demonstrated that overexpression of miRNA-210 post-MI in mice increased post-ischaemic neovascularisation by inhibiting ephrin-A3 and improved cardiac function 8 weeks post-MI. miR-23-24-27 cluster has also been reported to play a critical role in the regulation of neovascularisation. Knock-out of miR-27b, a component of this cluster impaired capillary branching in zebrafish embryos by targeting Dll4 and Sprouty (Spry)-2 (Biyashev et al., 2012). Veliceasa et al. (2015), also showed that overexpression of miR-27b in a mouse MI model increased capillary density and reperfusion, and improved cardiac function with an approximately 2-fold increase in ejection fraction over the control 14 days post-MI, and significantly reduced fibrosis at day 28.
On the other hand, several miRNAs have an inhibitory role in post-ischaemic neovascularisation. Overexpression of another component of cluster miR-23-24-27, miR-24, significantly impaired angiogenesis in zebrafish embryos by targeting p21 activated kinase (PAK4) and global transcription factor binding protein 2 (GATA2). Inhibition of this miRNA with chemically engineered cholesterol-conjugated single-strand RNA analogues (antagomirs) in a MI mice model increased capillary and arteriolar density, and improved cardiac function 14 days post-MI (Fiedler et al., 2011). The miRNA 17-92 cluster also has a prominent role in the regulation of post-ischaemic neovascularisation. Overexpression of the miR-92a component of this cluster has been reported to block angiogenesis in-vitro and in-vivo. Among the verified targets of miR-92a, integrin alpha 5 (ITGA5) critically influences EC proliferation and migration (Bonauer et al., 2009; Doebele et al., 2010; Daniel et al., 2014). Interestingly, antimir-92a (MRG-110) was evaluated in clinical trials; the results showed increased angiogenesis and blood perfusion following intradermal injection at the site of a small skin wound in healthy volunteers, as well as reduced alpha-smooth muscle actin (α-SMA) expression, which is correlated with activation of myofibroblasts (Safety Tolerability Pharmacokinetics, 2018).
EVs in Neovascularisation
Over the past few years, EVs have emerged as novel regulators of therapeutic processes, including cardiac neovascularisation due to their ability to transfer molecules, such as miRNAs, in ECs. EVs are defined as heterogeneous plasma membrane vesicles and are released in the extracellular space under normal and pathological conditions (Raposo and Stahl, 2019). According to their size and biogenesis pathway, they can be classified into three main types: exosomes, microvesicles and apoptotic bodies.
Exosomes are a class of cell-derived EVs of endosomal origin that are typically 30–150 nm in diameter and contain various macromolecules derived from the cell of origin. These include miRNAs, proteins, lipids and mRNAs (Isola and Chen, 2016). Exosome biogenesis starts from the intraluminal budding of endosomal compartments. This forms intraluminal vesicles (ILVs) in the endosomal compartments, which are known as multivesicular bodies (MVBs). MVBs can either fuse with lysosomes for subsequent degradation or fuse with the plasma membrane releasing the ILVs, in the extracellular space as exosomes. In contrast to exosomes, microvesicles, are typically 100–1,000 nm in size and are formed by the outward blebbing of the plasma membrane. During the blebbing process, disruption of the actin cytoskeleton and membrane reorganisation occurs (Cocucci and Meldolesi, 2015). The modification of membrane asymmetry results in the redistribution of aminophosholipids to the outer part of the cell membrane. Interestingly, microvesicle formation seems to occur in lipid-rich microdomains of the plasma membrane, such as lipid-rafts or caveolae domains (Del Conde et al., 2005; Morel et al., 2009). On the other hand, apoptotic bodies are exclusively formed during the last steps of apoptosis and range from 800 nm to 5 μm (Caruso and Poon, 2018). Investigating the mechanisms of EV release and uptake by ECs is critical to understand their role in neovascularisation under pathological conditions, such as MI. Recently, a new population of non-membranous nanoparticles ~35 nm in size, termed “exomeres” was identified (Zhang H. et al., 2018; Zhang et al., 2019). Zhang H. et al. (2018), recently showed that exomeres have a distinct protein, lipid, DNA and RNA profile to exosomes, and demonstrate unique organ distribution patterns, suggesting different biological functions. In contrast to EVs, exomere biogenesis remains unclear.
EV Release by ECs
EV release is a complex process that involves cytoskeletal proteins (actin and microtubules), molecular motors (kinesins and myosins), as well as molecular switches (small GTPases) and the fusion machinery (SNAREs and tethering factors) (Raposo and Stoorvogel, 2013). Ras-related proteins in brain (RAB) family, including Rab11, Rab27a/27b, and Rab35 coordinate membrane trafficking events, and have emerged as essential components of exosomal release in several cell types (Savina et al., 2002; Hsu et al., 2010; Ostrowski et al., 2010). Rab7 is another member of RAB family that mediates the maturation of late endosome and mediates their fusion with lysosomes (Vanlandingham and Ceresa, 2009). Jaé et al. (2015), showed that Rab7 and Rab27b regulate the secretion of endothelial miRNA through EVs. Moreover, Ostrowski et al. (2010), demonstrated that knocking down the Rab27a/27b effectors synaptotagmin-like 4 (SYTL4) (also known as Slp4) and exophilin 5 (EXPH5) also inhibits exosome secretion in HEK293 cells. Interestingly, Slp4 was also shown to have a central role in Weibel-Palade body (WPB) exocytosis from ECs (Bierings et al., 2012).
Although our understanding of the exact mechanism of EV-release from EC is lacking, several factors have been shown to trigger the release of EVs from ECs. EC injury is a critical part of the development of CHD and significantly affects the levels of EC-derived EVs (Werner et al., 2006). In general, EC-EVs are present at lower concentrations under physiological conditions and, upon activation, are released from ECs (Koga et al., 2005). Elevated levels of plasma EC microparticles have been reported in several CVD, including CHD (Koga et al., 2005; Werner et al., 2006; Nozaki et al., 2009). Moreover, clinical studies in heart failure patients have revealed that the number of circulating EVs from ECs and EPCs greatly depends on the severity of heart failure. Berezin et al. (2016), for instance, showed that heart failure patients with preserved ejection fraction had an increased number of apoptotic EC-derived EVs and less activated EC-derived EVs than patients with heart failure with reduced injection fraction. Interestingly, EC-derived EVs have been proposed as novel biomarkers of EC dysfunction and may determine the risk of acute heart failure (Horstman, 2004).
EVs of endothelial origin have been found to play a versatile role in neovascularisation since their effect seems to be affected by the dose used. Lacroix et al. (2007), for instance, showed that low amounts of microparticles (2 × 103 particles/well, in a 96-well plate) from TNFa-stimulated HMVECs could increase tube formation of endothelial progenitor derived cells (EPDCs) in-vitro. In contrast, high amounts of microparticles (2 × 105 particles/well, in a 96-well plate) had an inhibitory effect. Liang et al. (2018), showed that hypoxic HUVEC EVs could also inhibit EC migration and angiogenesis probably due to increased levels of miR-19b in these EVs (5 x 104 EVs/well, in a 6-well plate were used). The ability of ECs to stimulate angiogenesis may involve the transfer of miR-214 (Balkom et al., 2013). Interestingly, Chen et al. (2018), showed that EPC-derived EVs could increase angiogenesis but not proliferation in-vitro (7 × 109 EVs, in a 24-well plate). The intramyocardial delivery of EPC-derived EVs incorporated into shear-thinning hydrogels in Wistar rats, could increase angiogenesis, preserve ventricular geometry and improve haemodynamic function post-MI (9.33 × 109 EVs were delivered via 5 × 20 μl injections around the border zone of the infarcted area). Ou et al. (2011), however, demonstrated that endothelial microparticles from HUVECs in increased concentrations (higher than 105 microparticles/ml) could inhibit angiogenesis in mouse heart sections. These data suggest that EC release EVs with diverse roles in neovascularisation.
EV Uptake by ECs
EVs transfer information to the recipient cells by various mechanisms, including clathrin-mediated endocytosis, micropinocytosis, phagocytosis, caveolin-mediated endocytosis, and lipid raft mediated endocytosis (Mulcahy et al., 2014). The glycoproteins [e.g., Heparan sulphate proteoglycans (HSPG) (Christianson et al., 2013)] and proteins [e.g., integrins (Morelli et al., 2004), tetraspanins (Hemler, 2005)] on the surfaces of EVs and their target cells are recognised as critical factors that determine the uptake mechanism. Nazarenko et al. (2010), showed that treating exosomes with antibodies against tetraspanin-8, integrin CD49d, and vascular cell adhesion molecule-1 (VCAM-1, CD106) significantly reduced exosome uptake by RAECs. However, the precise mechanisms of EV uptake by ECs is still unknown. Some EVs can deliver their content to ECs through clathrin-mediated endocytosis. Dynamin is a GTPase essential for membrane fusion during this process. Blocking dynamin activity is a common strategy to study this mechanism (Singh et al., 2017). Chiba et al. (2018), showed that pancreatic cancer cell exosomes may be transferred to ECs through dynamin-dependent clathrin-mediated endocytosis, resulting in increased angiogenesis in-vitro. This was verified by blocking dynamin activity with a small inhibitor, called dynasore. Moreover, Svensson et al. (2013), reported that internalisation of exosomes derived from glioblastoma cells by ECs is significantly decreased post-methyl-β-cyclodextrin (MβCD) treatment. MβCD is a water-soluble oligosaccharide able to remove cholesterol from cell membranes, suggesting that lipid-raft endocytosis is a critical mechanism of EV uptake.
The most commonly used method for detecting EV uptake by ECs in-vitro and in-vivo is by using fluorescent lipid membrane dyes that stain EV membranes, such as PKH67 (Balkom et al., 2013), PKH26 (Lopatina et al., 2014; Lombardo et al., 2016; Zou et al., 2016; Mao et al., 2019) and DiI (Liang et al., 2016). Lopatina et al. (2014), for instance, showed that PKH26-stained MSC-EVs could be internalised into HMVECs and promote angiogenesis in-vitro and in-vivo. Moreover, Liang et al., demonstrated that the uptake of DiI-labelled MSC-exosomes by HUVECs increased tube formation in-vitro. To assess the role of MSC exosomes in EC angiogenesis in-vivo, the authors performed Matrigel plug assays in mice subcutaneously injecting HUVECs mixed with MSC-exosomes and HUVEC alone, and consistently with their in-vitro data, they showed that MSC-exosomes resulted in increased angiogenesis in-vivo. One potential issue with lipid-bound dyes is the leaching of the fluorescent molecules onto the cellular membranes, which can lead to a pattern of internalisation due to membrane recycling rather than EV uptake. To distinguish between surface-bound and internalised fluorescent EVs, the surface of the cell can be treated with trypsin (Franzen et al., 2014). A further limitation of these dyes is that the presence of the fluorescent molecules may affect the physical properties and thus, the normal behaviour of EVs. Membrane permeable dyes, such as carboxyfluorescein succinimidyl ester (CFSE) and 5(6)- carboxyfluorescein diacetate (CFDA), that are confined to the cytosol and fluoresce as a consequence of esterification, as well as Calcein AM are also used to study EV uptake by ECs (Teng et al., 2015; Li et al., 2018; Merckx et al., 2020). Radionuclides and magnetic particles have also been exploited to label EVs. Lee et al. (2020), for instance, recently showed that nanovesicles derived from iron oxide NP-incorporated MSCs could be effectively targeted to the myocardium of rats promoting cardiac function and angiogenesis. Finally, by fusing Gluc to a protein enriched in the membrane of exosomes, such as lactadherin, exosomes can emanate a strong luminescent signal when a Gluc substrate is present (Zhang K. et al., 2018). EVs can also be labelled with GFP by expressing a DNA construct coding for EV markers such as CD63, CD81, and CD9 fused to GFP in parent cells. This way, EVs can be tracked or purified to study their cargo (Garcia et al., 2015; Ribeiro-Rodrigues et al., 2017). Nonetheless, visualising GFP-labelled EV uptake by conventional fluorescent microscopy techniques is challenging due to the small nature of EVs. For this reason, the use of advanced technologies, including electron microscopy and atomic force microscopy are useful in EV characterisation (Mondal et al., 2019).
Recently, Cre-loxP system was also introduced as a very promising strategy to study EV uptake by cancer cells (Zomer et al., 2016). Adapting this system, de la Cuesta et al. (2019), visualised direct transfer of human pulmonary artery smooth muscle cells (HPASMCs) EVs to human pulmonary arterial endothelial cells (HPAECs). In particular, donor HPASMCs were transduced with a Cre recombinase lentiviral vector and HPAECs with a reporter lentiviral vector, that carried DsRed and eGFP separated by a loxP site. Cells were co-cultured in Boyden chambers, being physically separated by a membrane to prevent direct cell-cell contact. Zomer et al. (2015), previously showed that in this system, only Cre mRNA and not Cre protein is transferred into EVs. By performing qRT-PCR de la Cuesta et al. (2019) confirmed efficient loading of Cre mRNA into HPASMC-EVs. Using this system, the authors showed that HPASMC-EV-mediated Cre recombination resulted in a colour switch from red to green in reporter+ HPAECs, effectively evidencing communication via EVs. Therefore, the advantage of this approach is that EV uptake can be visualised in a more sensitive manner since it results in the fluorescent labelling of whole cells instead of small EVs.
Animal Models to Study EV Trafficking
One of the greatest limitations in using purified labelled EVs from cell culture supernatants into the circulation of animal models is the difficulty to translate the in-vivo implications of EVs at a functional level. The variations observed in studies using EVs from different cell sources, the number of particles injected, the route of administration as well as the fact that most studies are implemented in immunodeficient animals, are all important variables in the design and analysis of experimental data. For this reason, genetically engineered mouse models (GEMM) could provide a promising approach to outreach current limitations allowing tracing of EVs in living organisms. As previously mentioned, a new strategy that allows the study of EV transfer in-vitro and in-vivo using the Cre/loxP system was reported (Zomer et al., 2016). This approach involves the fluorescent labelling of Cre-reporter cells that take up the EVs released from cells which express Cre recombinase. In this method, prior to cell injection into mice ubiquitously expressing the Cre-LoxP reporter tdTomato (tdTomato B6 mice), cells were transfected with a plasmid carrying Cre recombinase and cyan fluorescence protein (CFP). In this way, donor cells expressing Cre recombinase were CFP positive (blue), and EVs derived from these cells carried Cre recombinase mRNA. Upon injection, EVs were taken up by recipient reporter cells that translated Cre mRNA into protein. This resulted in the recombination of the reporter gene and activation of GFP expression leading to a colour switch from red to green fluorescence exclusively in cells that had taken up functional EVs in-vivo Zomer et al. (2015), developed this system to study EV communication between tumour cells. EV-miRNA profiling revealed that malignant tumour cells contain mRNAs involved in tumour migration and metastasis. More importantly, intravital imaging, showed that EVs released by malignant cells can be internalised by less malignant cells within the same or distal tumours and that the less malignant tumour cells display enhanced migratory behaviour and metastatic capacity. The exact mechanism of how the Cre mRNA is transferred into exosomes remains unclear, but it is probably a simple reflection of increased cellular expression and sufficient EV loading. Interestingly, the authors verified that the colour switch was due to Cre mRNA containing EVs, and not by other mechanisms, such as free Cre mRNA or protein.
Other strategies involve the controlled expression of Cre recombinase under specific promotors. For example, Cre mRNA can be expressed selectively in haematopoietic cells under the vav1 promoter and sorted into EVs released into the bloodstream. Upon entering a target cell, this mRNA is translated to a functional protein, leading to excision of the stop-loxP site and induction of marker gene expression in transgenic mice. Based on this, Kur et al. (2020), proved that neuronal activity triggers the uptake of haematopoietic extracellular vesicles in vivo. In particular, they showed that after the induction of peripheral inflammation by intraperitoneal (IP) injection of lipopolysaccharide (LPS), there were frequent recombination events in the hippocampus, substantia nigra, and other regions of the brain as observed by yellow fluorescent protein (EYFP) expression. EYFP expression was mediated by Cre recombinase activity, indicating that haematopoietic cell EVs were transferred and internalised in these regions of the brain. Although most studies utilising GEMM to assess EV uptake are focused on cancer or neuroscience research, the adaptation of these animal models could greatly increase our knowledge in EV communication in cardiovascular disease, including MI. The use of the Cre-loxP system has significantly increased our understanding of EV communication. However, the fact that Cre mRNA can be loaded in and transferred through EVs may doubt the validity of approaches using the Cre-loxP system in a tissue-specific manner, since upon activation of Cre expression in specific cells, Cre mRNA or protein can be transferred to other cell types through EVs, leading to unfavourable recombination events.
Recently, McCann et al. (2020), generated a reporter mouse bearing a CD63-emGFPloxP/stop/loxP knock-in cassette that enables the labelling of cell type-specific EVs in-vivo, without prior in vitro manipulation. Upon crossing with a lineage-specific Cre recombinase driver mice, this system enables the specific labelling of circulating CD63+ vesicles from the cell type of interest. By crossing the mice bearing the CD63-emGFPloxP/stop/loxP knock-in cassette with Cdh5-CreERT2 mice, the authors generated CD63emGFP+ vasculature and showed that following tamoxifen administration to pregnant females the developing vasculature of the embryos was marked with emerald GFP (emGFP). Most importantly, whole plasma-purified EVs contained a subpopulation of emGFP+ vesicles that co-expressed EV markers, including CD9 and CD81, and EC markers, like CD105.
Altogether, the recent development of genetically modified mouse models to study EV trafficking in-vivo holds great promise as valuable tools for unravelling the in-vivo relevance of EVs in physiological and pathophysiological processes such as cardiac neovascularisation after cardiac ischemia.
EVs in Therapeutic Neovascularisation
EVs possess inherent tissue repair properties that make them ideal candidates for regenerative medicine therapeutics. Several studies have demonstrated the ability of EVs to promote neovascularisation post-ischaemia. Gallet et al. (2017), showed that intramyocardial delivery of cardiosphere-derived cell (CDC) exosomes in a pig MI model increased vessel density and cardiomyocyte hypertrophy, while preserving left ventricular (LV) and left ventricular ejection fraction (LVEF) volumes and reducing scar size. Moreover, Potz et al. (2018), showed that intramyocardial injection of human mesenchymal cell-derived EVs in a swine MI model could increase blood flow, capillary and arteriolar density 5 weeks post-left circumflex artery ligation. Chen et al. (2020), aimed to investigate the role of exosomes derived from remote ischemic conditioning (RIC) in cardiac remodelling and function. Exosomes were isolated from the plasma of rats subjected to HLI and injected to the caudal vein of rats once every 3 days post-MI. The authors showed that RIC and RIC exosomes significantly improved cardiac function and blood vessel formation, and decreased collagen deposition 28 days post-MI. Wu et al. (2020), demonstrated that the intramyocardial injection of EVs from ESC-derived cardiovascular progenitor cells (CVPCs) cultured under normoxia or hypoxia could significantly improve cardiac function, vascularisation and cardiomyocyte survival, and reduce fibrosis at 28 days in a mouse MI model. More importantly, EVs secreted from ESC-CVPC cultured under hypoxia had a better benefit in improving cardiac function post-MI.
EVs possess several advantages over cell-based therapeutics and conventional delivery systems. A major advantage is that EVs may be less immunogenic than their parental cells, probably due to the presence of less membrane-bound proteins like MHC complexes on their surface (Ong and Wu, 2015). The number of MHC molecules on EV surface highly depends on the cell of origin and the EV subtype (Wahlund et al., 2017). Recently, Kompa et al. (2020), used a subcutaneously implanted TheraCyte device for sustained delivery of the secretome of human cardiac stem cells (hCSCs) in a rat MI model. Cells can be enclosed in the TheraCyte device, being protected by the host's immune system while allowing the therapeutic secreted products to freely diffuse from within the device. The authors showed that hCSC secretome could preserve LV ejection fraction and cardiac function, reduce fibrotic scar tissue, interstitial fibrosis and cardiomyocyte hypertrophy, while increasing vascular density. To visualise the EV transfer from hCSC to the myocardium, they used CSCs expressing plasma membrane reporters and confirmed that EVs from W8B2+ CSCs could be transferred to the heart and other organs 4 weeks post-implantation. A further advantage of EVs is that they can be easily stored, retaining their function over prolonged periods, overcoming many limitations of the use of viable cells in regenerative medicine. Moreover, EVs are naturally occurring lipid nanoformulations that, compared to other synthetic drug delivery systems, may be promising carriers of therapeutic molecules, exhibiting less toxicity and increased stability under both physiological and pathological conditions. EVs might also be combined with other strategies to optimise therapeutic agent delivery. Liang et al. (2017), showed that exosomes could naturally envelope AAV-vectors (AAVExo) and protected from plasma neutralising antibodies, AAVExo could transduce cardiomyocytes with higher efficiency than free AAVs in a mouse MI model. For these reasons, EVs have emerged as ideal carriers for the delivery of therapeutic molecules, such as miRNAs, to ECs for the promotion of neovascularisation post ischaemia.
EV-bound miRNAs in Neovascularisation
EVs exert their action through the transfer of molecules, such as small RNAs and proteins, able to control molecular pathways in the recipient cells once transferred. For this reason, they have gained immense interest as therapeutic vehicles. Among their multidimensional role, several studies have demonstrated the ability of EVs to stimulate vascular growth and maturation by delivering pro-angiogenic miRNA molecules to ECs. Table 2 contains a list of EV-bound miRNAs as reported in EV-based neovascularisation studies. However, since our intention was to identify EV-bound miRNAs with a role in neovascularisation, this table contains a simple summary of our findings with an emphasis on the miRNAs, and due to space constraints, not all EV-producing cell types are reported.
EV-bound miRNA molecules can be internalised in the cytoplasm of recipient cells and activate molecular pathways controlling cell behaviour (Figure 2). Barile et al. (2014), for instance, showed that EVs from human cardiac progenitor cells (hCPCs) were enriched in the angiogenic and cardioprotective miR-210, miR-132, and miR-146a-3p. As a result, EVs derived from these cells could significantly increase cardiac angiogenesis in a MI rat model. Wang et al. (2017), demonstrated that MSC-derived EVs were also enriched in miR-210. Treatment with MSC-EVs significantly increased HUVEC tube formation, proliferation and migration in-vitro and capillary density of matrigel plugs implanted in a mouse MI model by targeting Efna3. Silencing of miR-210 in MSC-EVs significantly impaired the in-vitro and in-vivo angiogenic effects. Adamiak et al. (2018), showed that treatment with iPSC-derived EVs, promoted murine cardiac EC tube formation, migration and antiapoptotic properties. Injection of iPSCs-EVs in a mouse MI model significantly increased capillary density & reduced LV remodelling and hypertrophy compared to the controls. EV microRNA expression profiling was performed by miRNA array and revealed that several miRNAs were upregulated in these EVs, including miR-16, miR-17-92, miR-19b, miR-20a, miR-34, miR-126-3p, miR-130a-3p, miR-210-3p, miR-294.
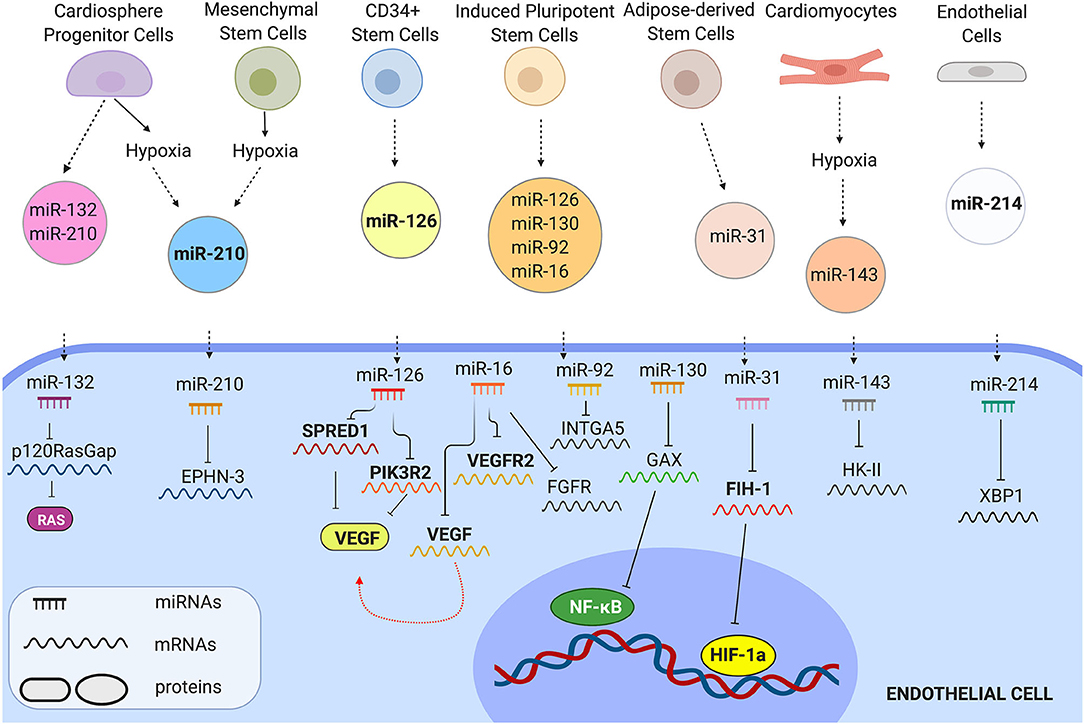
Figure 2. Representative examples of EV miRNAs that control EC behaviour. EVs from different cells carry angiogenic miRNAs. Once transferred in ECs, miRNAs control molecular pathways by inhibiting their mRNA-targets.
Specific conditions, such as hypoxia greatly affect gene expression and favour the production of miRNAs with angiogenic properties. As a result, several studies have confirmed that hypoxia leads to the release of angiogenic EVs. Ribeiro-Rodriquez et al. for instance, demonstrated that exosomes secreted by H9c2 myocardial cells and primary cardiomyocytes cultured under hypoxic conditions significantly increased the formation of new functional vessels post-MI in rats in comparison with exosomes cultured under normoxic conditions. An analysis of the miRNA profile of these exosomes revealed that the pro-angiogenic miR-222 and miR-143 were significantly increased in the hypoxic exosomes providing evidence that these miRNAs may contribute to post-ischemic neovascularisation (Ribeiro-Rodrigues et al., 2017). Similarly, Zhu et al. (2018), showed that exosomes derived from hypoxic MSCs augmented neovascularisation in a MI mouse model. Exosome RNA profiling revealed 145 genes that were upregulated in the hypoxic exosomes compared to the normoxic ones, with miR-210 being one of the most abundant miRNAs. To investigate the role of miR-210 in hypoxic-EV induced angiogenesis, the authors used a miR-210 inhibitor to block the activity of miR-210 in MSCs and revealed that exosomes derived from these cells failed to induce angiogenesis in-vitro. Moreover, overexpression of miR-210 in ECs resulted in improved tube formation similar to that achieved by hypoxic exosomes. Gray et al. (2015), reported that treatment with hypoxic EVs derived from CPCs significantly increased rat cardiac EC tube formation compared to treatment with normoxic EVs. Injection of hypoxic EVs in a mouse MI model significantly improved cardiac function and reduced fibrosis. EV microRNA expression profiling revealed several upregulated miRNAs under hypoxic conditions, including miR-15b, miR-17, miR-20a, miR-103, miR-199a, miR-210, miR-292.
Qiao et al. (2019), compared the effect of exosomes isolated from explant-derived cardiac stromal cells from patients with normal angiography results (NEXO) to exosomes from patients with heart failure (FEXO). They showed that intramyocardial injection of exosomes from the NEXO group in a mouse MI model significantly increased capillary density and decreased the infarct size, while injection of exosomes from the FEXO group exacerbated cardiac function and left ventricular remodelling. Exosomes from the FEXO group exhibited reduced ability to promote HUVEC tube formation in-vitro. EV microRNA expression profiling revealed decreased miR-21-5p expression in the FEXO compared to the NEXO group exosomes. As a result, silencing of miR-21-5p in NEXO group decreased HUVEC tube-formation, while upregulation of miR-21-5p in FEXO group restored HUVEC tube formation. Li et al. (2018) showed that EC treatment with exosomes from coronary blood of patients with myocardial ischaemia significantly increased EC tube formation, proliferation and migration in-vitro. Intramyocardial injection of exosomes in a mouse MI model significantly increased capillary density and reduced scar size. EV microRNA expression revealed decreased miR-939-5p expression in exosomes from coronary blood of patients with myocardial ischaemia compared to exosomes from healthy controls. Transfection of ECs with miR-939-5p mimic decreased EC tube formation and migration in-vitro, whereas transfection with miRNA inhibitor increased tube formation and migration.
Beltrami et al. (2017), showed that human pericardial fluid exosomes increased EC tube formation, proliferation and reduced EC apoptosis. EV microRNA expression profiling revealed that let-7b-5p was increased in these exosomes. Moreover, human pericardial fluid exosomes restored the angiogenic capacity of DICER knock-out-ECs in-vitro, but the reduction of exosomal let-7b-5p in exosomes failed to induce tube formation in the recipient DICER-KD ECs. Exosome injection in a mouse HLI model significantly increased capillary density and blood flow recovery and reduced necrosis. EVs from CD34+ stem cells also appear to be regulators of angiogenesis. In particular, Mathiyalagan et al. (2017), demonstrated that CD34+ cell-derived exosomes are enriched in pro-angiogenic miRNAs, such as miR-126-3p. As a result, injection of these exosomes in an HLI mouse model increased miR-126-3p levels without affecting the endogenous synthesis of this miRNA, implying a direct transfer of exosomal miR-126-3p to the ischaemic limb. Moreover, it was suggested that miR-126-3p enhanced angiogenesis by suppressing its known target, SPRED1. Ranghino et al. (2012), reported that the angiogenic miRNAs miR-126 and miR-296 were upregulated in EPC-derived EVs. Injection of EPC-derived EVs in a mouse HLI model significantly increased capillary density and blood perfusion to the ischaemic muscle. Recently, Johnson et al. (2019), showed that exosomes from induced vascular progenitor cell (iVPC) could significantly increase EC tube formation, proliferation and migration in-vitro and EC sprouting of mouse aortic rings. Exosome injection in a rat HLI model significantly increased capillary density and blood perfusion compared with rat aortic endothelial cell (RAEC) exosomes and controls. EV microRNA expression profiling revealed increased levels of several miRNAs in these exosomes, including miR-20b, miR−27b, miR-29b, miR-42a, miR-100, miR−125b, miR-143, miR-195, miR-291b, miR-497. Taken together, several studies have reported a role of EV-bound miRNAs in post-ischaemic neovascularisation. Nonetheless, a better understanding of their mechanism of action in the recipient cells is essential before clinical application.
Challenges and future Perspectives
The delivery of EVs, carrying therapeutic miRNA molecules, to the heart remains promising for the regulation of therapeutic neovascularisation post-MI, but there are several challenges to the field. Our knowledge of endogenous cell communication via EVs, for instance, both under physiological and pathological conditions is limited. Therefore, in-vivo visualisation of EV spatial and temporal release from the vascular wall, as well as of EV uptake by vascular ECs is of utmost importance for a better understanding of the biological function of EVs. To develop EV-based therapeutics, a more in-depth evaluation of EV pharmacokinetics in-vivo is also crucial. A critical factor that affects EV pharmacokinetics is the route of administration. Gallet et al. (2017), showed that CDC-derived exosomes resulted in improved vessel density and cardiac function post intramyocardial injection, but were ineffective after intracoronary injection. Therefore, preclinical models that allow for the study of endogenous EVs, as well as the biodistribution of exogenously administrated EVs, must be developed for a better understanding of their role in cardiac neovascularisation.
Moreover, clinical trials with EV products will only be possible with the improvement of isolation and purification techniques. The development of highly reproducible methods of isolating GMP-quality EVs, however, remains a great challenge in the field. Due to the lack of standardised cell culture and isolation methods, numerous studies, use relatively impure populations of EVs, which may affect angiogenesis assays, leading to wrong assumptions for their functionality. Lipoproteins are the most common contaminants from serum-containing medium (Yuana et al., 2014; Sódar et al., 2016). In this reason, the choice between culture medium containing EV-depleted serum and culture medium without serum needs to be considered. Serum starvation, however, has been reported to cause significant stress to the cultured cells, which subsequently leads to altered EV secretion (Witwer et al., 2013). Although this issue can be avoided by using EV-depleted serum, there are still several limitations associated with serum EV depletion strategies. Rigorous EV depletion of FBS using long ultracentrifugation protocols, for instance, is time-consuming and cannot remove small serum RNAs which can be mis-annotated as human RNAs (Wei et al., 2016). More recently introduced ultrafiltration-based strategies can overcome these limitations and result in solutions of extremely low EV and small RNA content (Kornilov et al., 2018). Another major dilemma in the EV field is the lack of a consensus methodology for the isolation of pure and intact EVs. Differential centrifugation is the most commonly used method for EV purification. Further purification from co-pelleted protein complexes and lipoproteins can be achieved by a density gradient. Nonetheless, this approach is associated with increased EV aggregation and usually results in fusion or disruption of the isolated EVs. Moreover, the optimal parameters of ultracentrifugation highly dependent on the type of centrifuge rotor used. Thus, it is essential that alternative approaches be used. Interestingly, a combination of ultrafiltration with size-exclusion chromatography has been proposed as a novel EV isolation approach that yields particles of improved purity and quality (Nordin et al., 2015).
Using standardised cell culture and EV isolation methods is extremely important in angiogenesis studies. Depending on the conditions that EVs have been generated and isolated, as well as the EV subtype and the dose used, they may exhibit a powerful proangiogenic or inhibitory effect. Moreover, since cell culture and EV isolation procedures affect the EV cargo, a more in-depth characterisation of the way that therapeutic molecules, such as miRNAs are sorted and released by EVs is essential before clinical application. EV-driven miRNA transfer post-MI is a new promising strategy for the promotion of neovascularisation. Numerous miRNAs including miR-126, miR-92a, miR-210, miR-27b, and miR-24 have been recognised as key regulators of post-ischaemic neovascularisation, but only a limited number have been identified in EVs. KRAS-MEK signalling has been proposed as an important regulator of miRNA sorting in EVs (McKenzie et al., 2016). However, the exact molecular mechanisms that drive miRNA transfer into EVs are not fully understood. Recently, a CRISPR-Cas-9 based system for single-cell detection of EV-mediated functional transfer of RNA was put forward (de Jong et al., 2020). This approach, termed CRISPR Operated Stoplight System for Functional Intercellular RNA Exchange (CROSS-FIRE), is based on activation of a fluorescent protein in recipient reporter cells upon functional delivery of specific sgRNAs, expressed in EV-donor cells. In general, there is a consensus that small RNA composition of cells differs from that in EVs, and emerging evidence supports that the presence of certain motifs on miRNAs may facilitate their transfer into EVs (Villarroya-Beltri et al., 2013; Koppers-Lalic et al., 2014).
Whether miRNA-Ago2 or miRNA-RISC complexes are present in EVs is of great interest to the EV field. Bound to Ago2, EV-miRNAs could efficiently assemble into functional RISCs for downregulation of their mRNA-target in the recipient cells. Interestingly, the entire RISC was reported to be present in EVs secreted from cancer cells, leading to efficient and rapid silencing of mRNA-targets at the recipient cells (Melo et al., 2014). An argument against the presence of Ago2 in EVs is that serum, a typical cell culture additive, contains a significant amount of non-vesicular Ago2 which may contaminate crude EV pellets prepared by standard EV isolation techniques, including ultracentrifugation of the conditioned medium at 100,000 × g (Huang et al., 2013). Size exclusion chromatography or density gradient can be used to separate vesicular from non-vesicular components of cell culture media (Prieto-Fernández et al., 2019). Jeppesen et al. (2019) recently used high-resolution density gradients to separate a crude small EV pellet into non-vesicular and vesicular fractions. Western blot analysis revealed that argonauts, including Ago2, were present in both fractions in media from Gli36 glioblastoma cells, but only in the non-vesicular fraction of media from DKO1 or MDA-MB-231 cells. A possible explanation of these findings could be that both DKO1 and MDA-MB-231 cells carry KRAS mutations. In contrast to this, several pathogens appear to promote Ago2 trafficking in EVs (Bukong et al., 2014; Mantel et al., 2016). Therefore, the ability to detect Ago2 in EVs may be a result of multiple factors, including the cell of origin, experimental conditions and detection methods. Tracing miRNA transfer from the recipient to the donor cells via EVs would significantly increase our knowledge on the way that EV-bound miRNAs affect EC behaviour and therefore, promote neovascularisation post-cardiac ischaemia. Although, several approaches have been proposed (reviewed in Mateescu et al., 2017), the detection and tracing of specific miRNAs in EVs remain challenging due to several issues, including limited probe specificity, limited signal per EV and poor signal-to-background ratios.
Currently, interest has been shifted toward engineering EV surface proteins and cargo for improved targeting (e.g., by the inclusion of peptides) and functionality. In this way, synthetic EVs may be loaded with known angiogenic miRNAs and target ECs to promote neovascularisation. One of the most common strategies used includes the loading of EV-producing cells with exogenous miRNAs and the transfection with plasmids expressing peptides of interest. Thus, EVs derived from these cells may carry therapeutic miRNAs and express targeting peptides on their surface. Alternatively, exogenous cargo can be directly loaded into EVs by several methods, including electroporation, heat-shock or freeze-thaw procedures, detergent treatment or sonication (de Abreu et al., 2020). However, further investigation is needed to define the stoichiometry required for therapeutic effects observed post-EV-miRNA transfer.
Although the focus of this review is on miRNAs, to date, several types of non-coding RNAs have emerged as key regulators of angiogenesis. Long non-coding RNAs (lncRNAs) for instance, may also control EC proliferation (e.g., MALAT1, H19, GAS5) or angiogenesis (MEG3, MANTIS) (Kok and Baker, 2019; Simion et al., 2019). Increasing evidence suggests that the interactions of lncRNAs with miRNAs play a critical role in the regulation of angiogenesis (Zhao et al., 2020). Moreover, lncRNAs may be selectively packaged into EVs and transferred between cells, improving cardiac function and vascularisation and reducing fibrosis post-MI (Wu et al., 2020). Circular RNAs (circRNAs) have also emerged as novel regulators of EC function and angiogenesis (e.g., CANRIL, CZNF292, Circ_0010729,Circ_0003575, Circ_0054633, Circ_0000109) (Zhang and Huang, 2020). CircRNAs can also be transferred to ECs through EVs. In the context of post-ischaemic neovascularisation, Dou et al. (2020), showed that following femoral artery ligation in VSMC specific SIRT1 transgenic (SIRT1-Tg) mice, blood flow and capillary density is significantly decreased due to the delivery of exosomal cZFP609 from VSMCs to ECs. Interestingly, the authors reported that cZFP609 may inhibit angiogenesis via blockade of HIF-1a nuclear translocation and hypoxia-induced VEGF-A expression in ECs. Integrating knowledge of miRNAs with other non-coding RNAs and the way that they are transferred between cells will be critical to our understanding of the biological orchestration that controls neovascularisation in health and disease.
Conclusion
An increasing number of studies have documented the capacity of EV-bound miRNAs to modulate the angiogenic programs of ECs and to control neovascularisation following MI. EV cargo depends on several factors, including the cell source, the conditions in which EVs have been generated, and the isolation and purification techniques employed. These factors, along with the dose used, may determine the beneficial or detrimental effects of EVs on angiogenesis. EV-based therapeutic approaches, however, are still challenging since our knowledge of the mechanisms of miRNA sorting in EVs, EV release, EV-miRNA uptake and the stoichiometry required for the therapeutic effects of EV-miRNA treatment is limited. A broader understanding of these processes, together with the validation of accurate technologies for the clinical-grade quality control of EVs, would significantly delineate the benefit-risk balance, and open up new opportunities for therapeutic strategies.
Author Contributions
DK, AB, MB, and AHB outlined concept and overview of review. DK wrote the manuscript. AB, AHB, MB, PdC, and LdW reviewed and edited the manuscript. DK designed and prepared the figures. All authors listed have made a substantial, direct and intellectual contribution to the work, and approved it for publication.
Funding
DK was funded by a Ph.D. studentship from Medical Research Scotland. AB was supported by European Union Horizon 2020 CardioReGenix Grant no. 825670. AHB was supported by the British Heart Foundation Chair of Translational Cardiovascular Sciences and European Research Council Advanced Grant VASCMIR and Horizon 2020 grant CARDIOREGENIX. MB was supported by a British Heart Foundation Intermediate Basic Science Research Fellowship (FS/16/4/31831) and by the BHF Centre for Vascular Regeneration (RM/17/3/33381). PdC was supported by a Dutch Heart Foundation grant (NHS2015T066).
Conflict of Interest
The authors declare that the research was conducted in the absence of any commercial or financial relationships that could be construed as a potential conflict of interest.
References
Adamiak, M., Cheng, G., Bobis-Wozowicz, S., Zhao, L., Kedracka-Krok, S., Samanta, A., et al. (2018). Induced pluripotent stem cell (iPSC)-derived extracellular vesicles are safer and more effective for cardiac repair than iPSCs. Circ. Res. 122, 296–309. doi: 10.1161/CIRCRESAHA.117.311769
Albanese, M., Chen, A. Y.-F., Hüls, C., Gärtner, K., Tagawa, T., Keppler, O. T., et al. (2020). Micro RNAs are minor constituents of extracellular vesicles and are hardly delivered to target cells. bioRxiv. doi: 10.1101/2020.05.20.106393
Anand, S., Majeti, B. K., Acevedo, L. M., Murphy, E. A., Mukthavaram, R., Scheppke, L., et al. (2010). MicroRNA-132-mediated loss of p120RasGAP activates the endothelium to facilitate pathological angiogenesis. Nat. Med. 16, 909–914. doi: 10.1038/nm.2186
Arif, M., Pandey, R., Alam, P., Jiang, S., Sadayappan, S., Paul, A., et al. (2017). MicroRNA-210-mediated proliferation, survival, and angiogenesis promote cardiac repair post myocardial infarction in rodents. J. Mol. Med. 95, 1369–1385. doi: 10.1007/s00109-017-1591-8
Arroyo, J. D., Chevillet, J. R., Kroh, E. M., Ruf, I. K., Pritchard, C. C., Gibson, D. F., et al. (2011). Argonaute2 complexes carry a population of circulating microRNAs independent of vesicles in human plasma. Proc. Natl. Acad. Sci. U.S.A. 108, 5003–8. doi: 10.1073/pnas.1019055108
Balkom, B. W. M., de Jong, O. G., Smits, M., Brummelman, J., den Ouden, K., de Bree, P. M., et al. (2013). Endothelial cells require miR-214 to secrete exosomes that suppress senescence and induce angiogenesis in human and mouse endothelial cells. Blood 121, 3997–4006. doi: 10.1182/blood-2013-02-478925
Barile, L., Lionetti, V., Cervio, E., Matteucci, M., Gherghiceanu, M., Popescu, L. M., et al. (2014). Extracellular vesicles from human cardiac progenitor cells inhibit cardiomyocyte apoptosis and improve cardiac function after myocardial infarction. Cardiovasc. Res. 103, 530–541. doi: 10.1093/cvr/cvu167
Bellera, N., Barba, I., Rodriguez-Sinovas, A., Ferret, E., Asín, M. A., Gonzalez-Alujas, M. T., et al. (2014). Single intracoronary injection of encapsulated antagomir-92a promotes angiogenesis and prevents adverse infarct remodeling. J. Am. Heart Assoc. 3:e000946. doi: 10.1161/JAHA.114.000946
Beltrami, C., Besnier, M., Shantikumar, S., Shearn, A. I. U., Rajakaruna, C., Laftah, A., et al. (2017). Human pericardial fluid contains exosomes enriched with cardiovascular-expressed MicroRNAs and promotes therapeutic angiogenesis. Mol. Ther. 25, 679–693. doi: 10.1016/j.ymthe.2016.12.022
Berezin, A. E., Kremzer, A. A., Martovitskaya, Y. V., Berezina, T. A., and Gromenko, E. A. (2016). Pattern of endothelial progenitor cells and apoptotic endothelial cell-derived microparticles in chronic heart failure patients with preserved and reduced left ventricular ejection fraction. EBioMedicine 4, 86–94. doi: 10.1016/j.ebiom.2016.01.018
Bernardo, B. C., Gao, X.-M., Winbanks, C. E., Boey, E. J. H., Tham, Y. K., Kiriazis, H., et al. (2012). Therapeutic inhibition of the miR-34 family attenuates pathological cardiac remodeling and improves heart function, Proc. Natl. Acad. Sci. U.S.A. 109, 17615–17620. doi: 10.1073/pnas.1206432109
Besnier, M., Shantikumar, S., Anwar, M., Dixit, P., Chamorro-Jorganes, A., Sweaad, W., et al. (2019). miR-15a/-16 inhibit angiogenesis by targeting the Tie2 coding sequence: therapeutic potential of a miR-15a/16 decoy system in limb ischemia. Mol. Ther. Nucleic Acids 17, 49–62. doi: 10.1016/j.omtn.2019.05.002
Bierings, R., Hellen, N., Kiskin, N., Knipe, L., Fonseca, A. V., Patel, B., et al. (2012). The interplay between the Rab27A effectors Slp4-a and MyRIP controls hormone-evoked weibel-palade body exocytosis. Blood 120, 2757–2767. doi: 10.1182/blood-2012-05-429936
Biyashev, D., Veliceasa, D., Topczewski, J., Topczewska, J. M., Mizgirev, I., Vinokour, E., et al. (2012). miR-27b controls venous specification and tip cell fate. Blood 119, 2679–2687. doi: 10.1182/blood-2011-07-370635
Bonauer, A., Carmona, G., Iwasaki, M., Mione, M., Koyanagi, M., Fischer, A., et al. (2009). MicroRNA-92a controls angiogenesis and functional recovery of ischemic tissues in mice. Science 324, 1710–1713. doi: 10.1126/science.1174381
Bukong, T. N., Momen-Heravi, F., Kodys, K., Bala, S., and Szabo, G. (2014). Exosomes from hepatitis C infected patients transmit HCV infection and contain replication competent viral RNA in complex with Ago2-miR122-HSP90. PLoS Pathogens 10:e1004424. doi: 10.1371/journal.ppat.1004424
Burri, P. H., and Tarek, M. R. (1990). A novel mechanism of capillary growth in the rat pulmonary microcirculation. Anat. Rec. 228, 35–45. doi: 10.1002/ar.1092280107
Caduff, J. H., Fischer, L. C., and Burri, P. H. (1986). Scanning electron microscope study of the developing microvasculature in the postnatal rat lung. Anat. Rec. 216, 154–164. doi: 10.1002/ar.1092160207
Caporali, A., Meloni, M., Völlenkle, C., Bonci, D., Sala-Newby, G. B., Addis, R., et al. (2011). Deregulation of microRNA-503 contributes to diabetes mellitus–induced impairment of endothelial function and reparative angiogenesis after limb ischemia. Circulation 123, 282–291. doi: 10.1161/CIRCULATIONAHA.110.952325
Caruso, S., and Poon, I. K. H. (2018). Apoptotic cell-derived extracellular vesicles: more than just debris. Front. Immunol. 9:1486. doi: 10.3389/fimmu.2018.01486
Castellan, R. F. P., Vitiello, M., Vidmar, M., Johnstone, S., Iacobazzi, D., Mellis, D., et al. (2020). Mir-96 and miR-183 differentially regulate neonatal and adult post-infarct neovascularisation. JCI Insight. 5:e134888. doi: 10.1172/jci.insight.134888
Cavallari, C., Figliolini, F., Tapparo, M., Cedrino, M., Trevisan, A., Positello, L., et al. (2020). miR-130a and Tgfβ content in extracellular vesicles derived from the serum of subjects at high cardiovascular risk predicts their in-vivo angiogenic potential. Sci. Rep. 10:706. doi: 10.1038/s41598-019-55783-7
Chamorro-Jorganes, A., Araldi, E., Penalva, L. O. F., Sandhu, D., Fernández-Hernando, C., and Suárez, Y. (2011). MicroRNA-16 and MicroRNA-424 regulate cell-autonomous angiogenic functions in endothelial cells via targeting vascular endothelial growth factor receptor-2 and fibroblast growth factor receptor-1. Arterioscler. Thromb. Vascu. Biol. 31, 2595–2606. doi: 10.1161/ATVBAHA.111.236521
Chen, C. W., Wang, L. L., Zaman, S., Gordon, J., Arisi, M. F., Venkataraman, C. M., et al. (2018). Sustained release of endothelial progenitor cell-derived extracellular vesicles from shear-thinning hydrogels improves angiogenesis and promotes function after myocardial infarction. Cardiovasc. Res. 114, 1029–1040. doi: 10.1093/cvr/cvy067
Chen, Q., Huang, M., Wu, J., Jiang, Q., and Zheng, X. (2020). Exosomes isolated from the plasma of remote ischemic conditioning rats improved cardiac function and angiogenesis after myocardial infarction through targeting HSP70. Aging 12, 3682–3693. doi: 10.18632/aging.102837
Chen, Y., and Gorski, D. H. (2008). Regulation of angiogenesis through a microRNA (miR-130a) that down-regulates antiangiogenic homeobox genes GAX and HOXA5. Blood 111, 1217–1226. doi: 10.1182/blood-2007-07-104133
Chevillet, J. R., Kang, Q., Ruf, I. K., Briggs, H. A., Vojtech, L. N., Hughes, S. M., et al. (2014). Quantitative and stoichiometric analysis of the microRNA content of exosomes. Proc. Natl. Acad. Sci. U.S.A. 111, 14888–93. doi: 10.1073/pnas.1408301111
Chiba, M., Kubota, S., Sato, K., and Monzen, S. (2018). Exosomes released from pancreatic cancer cells enhance angiogenic activities via dynamin-dependent endocytosis in endothelial cells in vitro. Sci. Rep. 8:11972. doi: 10.1038/s41598-018-30446-1
Christianson, H. C., Svensson, K. J., Van Kuppevelt, T. H., Li, J. P., and Belting, M. (2013). Cancer cell exosomes depend on cell-surface heparan sulfate proteoglycans for their internalization and functional activity. Proc. Natl. Acad. Sci. U.S.A. 110, 17380–17385. doi: 10.1073/pnas.1304266110
Claxton, S., Kostourou, V., Jadeja, S., Chambon, P., Hodivala-Dilke, K., and Fruttiger, M. (2008). Efficient, inducible Cre-recombinase activation in vascular endothelium. Genesis 46, 74–80. doi: 10.1002/dvg.20367
Climent, M., Quintavalle, M., Miragoli, M., Chen, J., Condorelli, G., and Elia, L. (2015). TGFβ triggers miR-143/145 transfer from smooth muscle cells to endothelial cells, thereby modulating vessel stabilization. Circ. Res. 116, 1753–1764. doi: 10.1161/CIRCRESAHA.116.305178
Cocucci, E., and Meldolesi, J. (2015). Ectosomes and exosomes: shedding the confusion between extracellular vesicles. Trends Cell Biol. 25, 364–372. doi: 10.1016/j.tcb.2015.01.004
Daniel, J.-M., Penzkofer, D., Teske, R., Dutzmann, J., Koch, A., Bielenberg, W., et al. (2014). Inhibition of miR-92a improves re-endothelialization and prevents neointima formation following vascular injury. Cardiovasc. Res. 103, 564–72. doi: 10.1093/cvr/cvu162
de Abreu, R. C., Fernandes, H., da Costa Martins, P. A., Sahoo, S., Emanueli, C., and Ferreira, L. (2020). Native and bioengineered extracellular vesicles for cardiovascular therapeutics. Nat. Rev. Cardiol. 1–13. doi: 10.1038/s41569-020-0389-5
de Jong, O. G., Murphy, D. E., Mäger, I., Willms, E., Garcia-Guerra, A., Gitz-Francois, J. J., et al. (2020). A CRISPR-Cas9-based reporter system for single-cell detection of extracellular vesicle-mediated functional transfer of RNA. Nat. Commun. 11:1113. doi: 10.1038/s41467-020-15347-0
de la Cuesta, F., Passalacqua, I., Rodor, J., Bhushan, R., Denby, L., and Baker, A. H. (2019). Extracellular vesicle cross-talk between pulmonary artery smooth muscle cells and endothelium during excessive TGF-β signalling: implications for PAH vascular remodelling. Cell Commun. Signal. 17:143. doi: 10.1186/s12964-019-0449-9
Del Conde, I., Shrimpton, C. N., Thiagarajan, P., and López, J. A. (2005). Tissue-factor-bearing microvesicles arise from lipid rafts and fuse with activated platelets to initiate coagulation. Blood 106, 1604–1611. doi: 10.1182/blood-2004-03-1095
Del Toro, R., Prahst, C., Mathivet, T., Siegfried, G., Kaminker, J. S., Larrivee, B., et al. (2010). Identification and functional analysis of endothelial tip cell-enriched genes. Blood 116, 4025–4033. doi: 10.1182/blood-2010-02-270819
Dentelli, P., Rosso, A., Orso, F., Olgasi, C., Taverna, D., and Brizzi, M. F. (2010). MicroRNA-222 controls neovascularization by regulating signal transducer and activator of transcription 5A expression. Arterioscler. Thromb. Vasc. Biol. 30, 1562–1568. doi: 10.1161/ATVBAHA.110.206201
Desjarlais, M., Dussault, S., Dhahri, W., Mathieu, R., and Rivard, A. (2017). MicroRNA-150 modulates ischemia-induced neovascularization in atherosclerotic conditions. Arterioscler. Thromb. Vasc. Biol. 37, 900–908. doi: 10.1161/ATVBAHA.117.309189
Doebele, C., Bonauer, A., Fischer, A., Scholz, A., Reiss, Y., Urbich, C., et al. (2010). Members of the microRNA-17-92 cluster exhibit a cell-intrinsic antiangiogenic function in endothelial cells. Blood 115, 4944–4950. doi: 10.1182/blood-2010-01-264812
Dou, Y.-Q., Kong, P., Li, C.-L., Sun, H.-X., Li, W.-W., Yu, Y., et al. (2020). Smooth muscle SIRT1 reprograms endothelial cells to suppress angiogenesis after ischemia. Theranostics 10, 1197–1212. doi: 10.7150/thno.39320
Duan, Q., Yang, L., Gong, W., Chaugai, S., Wang, F., Chen, C., et al. (2015). MicroRNA-214 is upregulated in heart failure patients and suppresses XBP1-mediated endothelial cells angiogenesis. J. Cell. Physiol. 230, 1964–1973. doi: 10.1002/jcp.24942
Fan, J., Li, H., Nie, X., Yin, Z., Zhao, Y., Zhang, X., et al. (2018). MiR-665 aggravates heart failure via suppressing CD34-mediated coronary microvessel angiogenesis Aging 10, 2459–2479. doi: 10.18632/aging.101562
Fasanaro, P., Greco, S., Lorenzi, M., Pescatori, M., Brioschi, M., Kulshreshtha, R., et al. (2009). An integrated approach for experimental target identification of hypoxia-induced miR-210. J. Biol. Chem. 284, 35134–35143. doi: 10.1074/jbc.M109.052779
Fiedler, J., Jazbutyte, V., Kirchmaier, B. C., Gupta, S. K., Lorenzen, J., Hartmann, D., et al. (2011). MicroRNA-24 regulates vascularity after myocardial infarction. Circulation 124, 720–730. doi: 10.1161/CIRCULATIONAHA.111.039008
Fish, J. E., Santoro, M. M., Morton, S. U., Yu, S., Yeh, R. F., Wythe, J. D., et al. (2008). miR-126 regulates angiogenic signaling and vascular integrity. Dev. Cell. 15, 272–284. doi: 10.1016/j.devcel.2008.07.008
Franzen, C. A., Simms, P. E., Huis, A. F., van Foreman, K. E., Kuo, P. C., and Gupta, G. N. (2014). Characterization of uptake and internalization of exosomes by bladder cancer cells. BioMed Res. Int. 2014. doi: 10.1155/2014/619829
Fujisawa, T., Tura-Ceide, O., Hunter, A., Mitchell, A., Vesey, A., Medine, C., et al. (2019). Endothelial progenitor cells do not originate from the bone marrow. Circulation 140, 1524–1526. doi: 10.1161/CIRCULATIONAHA.119.042351
Gallet, R., Dawkins, J., Valle, J., Simsolo, E., de Couto, G., Middleton, R., et al. (2017). Exosomes secreted by cardiosphere-derived cells reduce scarring, attenuate adverse remodelling, and improve function in acute and chronic porcine myocardial infarction. Eur. Heart J. 38, 201–211. doi: 10.1093/eurheartj/ehw240
Garbern, J. C., and Lee, R. T. (2013). Cardiac stem cell therapy and the promise of heart regeneration. Cell Stem Cell 12, 689–698. doi: 10.1016/j.stem.2013.05.008
Garcia, N. A., Ontoria-Oviedo, I., González-King, H., Diez-Juan, A., and Sepúlveda, P. (2015). Glucose starvation in cardiomyocytes enhances exosome secretion and promotes angiogenesis in endothelial cells. PLoS ONE 10:e0138849. doi: 10.1371/journal.pone.0138849
Gerhardt, H., Golding, M., Fruttiger, M., Ruhrberg, C., Lundkvist, A., Abramsson, A., et al. (2003). VEGF guides angiogenic sprouting utilizing endothelial tip cell filopodia. J. Cell Biol. 161, 1163–1177. doi: 10.1083/jcb.200302047
Ghosh, G., Subramanian, I. V., Adhikari, N., Zhang, X., Joshi, H. P., Basi, D., et al. (2010). Hypoxia-induced microRNA-424 expression in human endothelial cells regulates HIF-α isoforms and promotes angiogenesis. J. Clin. Invest. 120, 4141–4154. doi: 10.1172/JCI42980
Gnecchi, M., Zhang, Z., Ni, A., and Dzau, V. J. (2008). Paracrine mechanisms in adult stem cell signaling and therapy. Circ. Res. 103, 1204–19. doi: 10.1161/CIRCRESAHA.108.176826
Gray, W. D., French, K. M., Ghosh-Choudhary, S., Maxwell, J. T., Brown, M. E., Platt, M. O., et al. (2015). Identification of therapeutic covariant microrna clusters in hypoxia treated cardiac progenitor cell exosomes using systems biology. Circ. Res. 116, 255–63. doi: 10.1161/CIRCRESAHA.116.304360
Grover, G. (1995). The definition of myocardial ischaemia. Cardiovasc. Res. 29:141. doi: 10.1016/S0008-6363(95)90114-0
Grundmann, S., Hans, F. P., Kinniry, S., Heinke, J., Helbing, T., Bluhm, F., et al. (2011). MicroRNA-100 regulates neovascularization by suppression of mammalian target of rapamycin in endothelial and vascular smooth muscle cells. Circulation 123, 999–1009. doi: 10.1161/CIRCULATIONAHA.110.000323
Gu, W., Zhan, H., Zhou, X. Y., Yao, L., Yan, M., Chen, A., et al. (2017). MicroRNA-22 regulates inflammation and angiogenesis via targeting VE-cadherin. FEBS Lett. 591, 513–526. doi: 10.1002/1873-3468.12565
Hemler, M. E. (2005). Tetraspanin functions and associated microdomains. Nat. Rev. Mol. Cell Biol. 6, 801–811. doi: 10.1038/nrm1736
Heuslein, J. L., McDonnell, S. P., Song, J., Annex, B. H., and Price, R. J. (2018). MicroRNA-146a regulates perfusion recovery in response to arterial occlusion via arteriogenesis. Front. Bioeng. Biotechnol. 6:1. doi: 10.3389/fbioe.2018.00001
Hinkel, R., Penzkofer, D., Zühlke, S., Fischer, A., Husada, W., Xu, Q. F., et al. (2013). Inhibition of microRNA-92a protects against ischemia/reperfusion injury in a large-animal model. Circulation 128, 1066–1075. doi: 10.1161/CIRCULATIONAHA.113.001904
Hodgkinson, C. P., Gomez, J. A., Bareja, A., and Dzau, V. J. (2016). “Role of paracrine mechanisms,” in Stem Cell and Gene Therapy for Cardiovascular Disease (Amsterdam: Elsevier), 39–48.
Horstman, L. L. (2004). Endothelial microparticles as markers of endothelial dysfunction. Front. Biosci. 9:1118. doi: 10.2741/1270
Hou, J., Liu, L., Zhu, Q., Wu, Y., Tian, B., Cui, L., et al. (2016). MicroRNA-185 inhibits angiogenesis in human microvascular endothelial cells through targeting stromal interaction molecule 1. Cell Biol. Int. 40, 318–328. doi: 10.1002/cbin.10572
Hou, S., Fang, M., Zhu, Q., Liu, Y., Liu, L., and Li, X. (2017). MicroRNA-939 governs vascular integrity and angiogenesis through targeting γ-catenin in endothelial cells. Biochem. Biophys. Res. Commun. 484, 27–33. doi: 10.1016/j.bbrc.2017.01.085
Hsu, C., Morohashi, Y., Yoshimura, S. I., Manrique-Hoyos, N., Jung, S. Y., Lauterbach, M. A., et al. (2010). Regulation of exosome secretion by Rab35 and its GTPase-activating proteins TBC1D10A-C. J. Cell Biol. 189, 223–232. doi: 10.1083/jcb.200911018
Hu, S., Huang, M., Li, Z., Jia, F., Ghosh, Z., Lijkwan, M. A., et al. (2010). MicroRNA-210 as a novel therapy for treatment of ischemic heart disease. Circulation 122(11 Suppl.), S124–S131. doi: 10.1161/CIRCULATIONAHA.109.928424
Huang, X., Yuan, T., Tschannen, M., Sun, Z., Jacob, H., Du, M., et al. (2013). Characterization of human plasma-derived exosomal RNAs by deep sequencing. BMC Genomics 14:319. doi: 10.1186/1471-2164-14-319
Huntzinger, E., and Izaurralde, E. (2011). Gene silencing by microRNAs: contributions of translational repression and mRNA decay. Nat. Rev. Genetics. 12, 99–110. doi: 10.1038/nrg2936
Icli, B., Wara, A. K. M., Moslehi, J., Sun, X., Plovie, E., Cahill, M., et al. (2013). MicroRNA-26a regulates pathological and physiological angiogenesis by targeting BMP/SMAD1 signaling. Circ. Res. 113, 1231–1241. doi: 10.1161/CIRCRESAHA.113.301780
Icli, B., Wu, W., Ozdemir, D., Li, H., Cheng, H. S., Haemmig, S., et al. (2019a). MicroRNA-615-5p regulates angiogenesis and tissue repair by targeting AKT/eNOS (protein kinase B/endothelial nitric oxide synthase) signaling in endothelial cells. Arterioscler. Thromb. Vasc. Biol. 39, 1458–1474. doi: 10.1161/ATVBAHA.119.312726
Icli, B., Wu, W., Ozdemir, D., Li, H., Haemmig, S., Liu, X., et al. (2019b). MicroRNA-135a-3p regulates angiogenesis and tissue repair by targeting p38 signaling in endothelial cells. FASEB J. 33, 5599–5614. doi: 10.1096/fj.201802063RR
Isola, A., and Chen, S. (2016). Exosomes: the messengers of health and disease. Curr. Neuropharmacol. 15, 157–165. doi: 10.2174/1570159X14666160825160421
Jaé, N., McEwan, D. G., Manavski, Y., Boon, R. A., and Dimmeler, S. (2015). Rab7a and Rab27b control secretion of endothelial microRNA through extracellular vesicles. FEBS Lett. 589, 3182–3188. doi: 10.1016/j.febslet.2015.08.040
Jakob, P., Doerries, C., Briand, S., Mocharla, P., Kränkel, N., Besler, C., et al. (2012). Loss of angiomir-126 and 130a in angiogenic early outgrowth cells from patients with chronic heart failure: role for impaired in vivo neovascularization and cardiac repair capacity. Circulation 126, 2962–2975. doi: 10.1161/CIRCULATIONAHA.112.093906
Jeppesen, D. K., Fenix, A. M., Franklin, J. L., Higginbotham, J. N., Zhang, Q., Zimmerman, L. J., et al. (2019). Reassessment of exosome composition. Cell 177, 428–445.e18. doi: 10.1016/j.cell.2019.02.029
Jiang, Q., Lagos-Quintana, M., Liu, D., Shi, Y., Helker, C., Herzog, W., et al. (2013). MiR-30a regulates endothelial tip cell formation and arteriolar branching. Hypertension 62, 592–598. doi: 10.1161/HYPERTENSIONAHA.113.01767
Jin, Y., Yang, C. J., Xu, X., Cao, J. N., Feng, Q. T., and Yang, J. (2015). MiR-214 regulates the pathogenesis of patients with coronary artery disease by targeting VEGF. Mol. Cell. Biochem. 402, 111–122. doi: 10.1007/s11010-014-2319-5
Johnson, T. K., Zhao, L., Zhu, D., Wang, Y., Xiao, Y., Oguljahan, B., et al. (2019). Exosomes derived from induced vascular progenitor cells promote angiogenesis in vitro and in an in vivo rat hindlimb ischemia model. Am. J. Physiol. Heart Circ. Physiol. 317, H765–H776. doi: 10.1152/ajpheart.00247.2019
Joris, V., Gomez, E. L., Menchi, L., Lobysheva, I., Di Mauro, V., Esfahani, H., et al. (2018). MicroRNA-199a-3p and MicroRNA-199a-5p take part to a redundant network of regulation of the NOS (NO Synthase)/NO pathway in the endothelium. Arterioscler. Thromb. Vasc. Biol. 38, 2345–2357. doi: 10.1161/ATVBAHA.118.311145
Kang, T., Jones, T. M., Naddell, C., Bacanamwo, M., Calvert, J. W., Thompson, W. E., et al. (2016). Adipose-derived stem cells induce angiogenesis via microvesicle transport of miRNA-31. Stem Cells Transl. Med. 5, 440–450. doi: 10.5966/sctm.2015-0177
Kim, J.-H., Lee, K.-S., Lee, D.-K., Kim, J., Kwak, S.-N., Ha, K.-S., et al. (2014). Hypoxia-responsive MicroRNA-101 promotes angiogenesis via heme oxygenase-1/vascular endothelial growth factor axis by targeting cullin 3. Antioxid. Redox Signal. 21, 2469–2482. doi: 10.1089/ars.2014.5856
Kocijan, T., Rehman, M., Colliva, A., Groppa, E., Leban, M., Vodret, S., et al. (2020). Genetic lineage tracing reveals poor angiogenic potential of cardiac endothelial cells. Cardiovasc. Res. doi: 10.1093/cvr/cvaa012. [Epub ahead of print].
Koga, H., Sugiyama, S., Kugiyama, K., Watanabe, K., Fukushima, H., Tanaka, T., et al. (2005). Elevated levels of VE-cadherin-positive endothelial microparticles in patients with type 2 diabetes mellitus and coronary artery disease. J. Am. Coll. Cardiol. 45, 1622–1630. doi: 10.1016/j.jacc.2005.02.047
Kok, F. O., and Baker, A. H. (2019). The function of long non-coding RNAs in vascular biology and disease. Vascular Pharmacol. 114, 23–30. doi: 10.1016/j.vph.2018.06.004
Kompa, A. R., Greening, D. W., Kong, A. M., McMillan, P. J., Fang, H., Saxena, R., et al. (2020). Sustained subcutaneous delivery of secretome of human cardiac stem cells promotes cardiac repair following myocardial infarction. Cardiovasc. Res. Cvaa088. doi: 10.1093/cvr/cvaa088
Koppers-Lalic, D., Hackenberg, M., Bijnsdorp, I. V., van Eijndhoven, M. A. J., Sadek, P., Sie, D., et al. (2014). Nontemplated nucleotide additions distinguish the small RNA composition in cells from exosomes. Cell Rep. 8, 1649–1658. doi: 10.1016/j.celrep.2014.08.027
Kornilov, R., Puhka, M., Mannerström, B., Hiidenmaa, H., Peltoniemi, H., Siljander, P., et al. (2018). Efficient ultrafiltration-based protocol to deplete extracellular vesicles from fetal bovine serum. J. Extracell. Vesicles 7:1422674. doi: 10.1080/20013078.2017.1422674
Kuehbacher, A., Urbich, C., Zeiher, A. M., and Dimmeler, S. (2007). Role of dicer and drosha for endothelial microRNA expression and angiogenesis. Circ. Res. 101, 59–68. doi: 10.1161/CIRCRESAHA.107.153916
Kur, I.-M., Prouvot, P.-H., Fu, T., Fan, W., Müller-Braun, F., Das, A., et al. (2020). Neuronal activity triggers uptake of hematopoietic extracellular vesicles in vivo. PLoS Biol. 18:e3000643. doi: 10.1371/journal.pbio.3000643
Lacroix, R., Sabatier, F., Mialhe, A., Basire, A., Pannell, R., Borghi, H., et al. (2007). Activation of plasminogen into plasmin at the surface of endothelial microparticles: a mechanism that modulates angiogenic properties of endothelial progenitor cells in vitro. Blood 110, 2432–2439. doi: 10.1182/blood-2007-02-069997
Lalwani, M. K., Sharma, M., Singh, A. R., Chauhan, R. K., Patowary, A., Singh, N., et al. (2012). Reverse genetics screen in zebrafish identifies a role of miR-142a-3p in vascular development and integrity. PLoS ONE 7:e52588. doi: 10.1371/journal.pone.0052588
Lee, J.-R., Park, B.-W., Kim, J., Choo, Y. W., Kim, H. Y., Yoon, J.-K., et al. (2020). Nanovesicles derived from iron oxide nanoparticles–incorporated mesenchymal stem cells for cardiac repair. Sci. Adv. 6:eaaz0952. doi: 10.1126/sciadv.aaz0952
Lei, Z., van Mil, A., Brandt, M. M., Grundmann, S., Hoefer, I., Smits, M., et al. (2015). MicroRNA-132/212 family enhances arteriogenesis after hindlimb ischaemia through modulation of the Ras-MAPK pathway. J. Cell. Mol. Med. 19, 1994–2005. doi: 10.1111/jcmm.12586
Lemcke, H., Voronina, N., Steinhoff, G., and David, R. (2018). Recent progress in stem cell modification for cardiac regeneration. Stem Cells Int. 2018, 1–22. doi: 10.1155/2018/1909346
Li, H., Liao, Y., Gao, L., Zhuang, T., Huang, Z., Zhu, H., et al. (2018). Coronary serum exosomes derived from patients with myocardial ischemia regulate angiogenesis through the miR-939-mediated nitric oxide signaling pathway. Theranostics 8, 2079–2093. doi: 10.7150/thno.21895
Li, Z., Solomonidis, E. G., Meloni, M., Taylor, R. S., Duffin, R., Dobie, R., et al. (2019). Single-cell transcriptome analyses reveal novel targets modulating cardiac neovascularization by resident endothelial cells following myocardial infarction. Eur. Heart J. 40, 2507–2520. doi: 10.1093/eurheartj/ehz305
Liang, H. Z., Li, S. F., Zhang, F., Wu, M. Y., Li, C. L., Song, J. X., et al. (2018). Effect of endothelial microparticles induced by hypoxia on migration and angiogenesis of human umbilical vein endothelial cells by delivering MicroRNA-19b. Chin. Med. J. 131, 2726–2733. doi: 10.4103/0366-6999.245271
Liang, X., Zhang, L., Wang, S., Han, Q., and Zhao, R. C. (2016). Exosomes secreted by mesenchymal stem cells promote endothelial cell angiogenesis by transferring miR-125a. J. Cell Sci. 129, 2182–2189. doi: 10.1242/jcs.170373
Liang, Y., Prabhu, M., Erik, K., Elena, C., Dongtak, J., Delaine, C., et al. (2017). Abstract 15439: AAV-containing exosomes as a novel vector to improve AAV-mediated myocardial gene delivery in resistance to neutralizing antibody | circulation. Circulation 136.
Liu, L.-Z., Li, C., Chen, Q., Jing, Y., Carpenter, R., Jiang, Y., et al. (2011). MiR-21 induced angiogenesis through AKT and ERK activation and HIF-1α expression. PLoS ONE 6:e19139. doi: 10.1371/journal.pone.0019139
Liu, Y., Li, Q., Hosen, M. R., Zietzer, A., Flender, A., Levermann, P., et al. (2019). Atherosclerotic conditions promote the packaging of functional MicroRNA-92a-3p into endothelial microvesicles. Circ. Res. 124, 575–587. doi: 10.1161/CIRCRESAHA.118.314010
Liu, Y.-W., Chen, B., Yang, X., Fugate, J. A., Kalucki, F. A., Futakuchi-Tsuchida, A., et al. (2018). Human embryonic stem cell-derived cardiomyocytes restore function in infarcted hearts of non-human primates. Nat. Biotechnol. 36, 597–605. doi: 10.1038/nbt.4162
Lombardo, G., Dentelli, P., Togliatto, G., Rosso, A., Gili, M., Gallo, S., et al. (2016). Activated stat5 trafficking via endothelial cell-derived extracellular vesicles controls IL-3 pro-angiogenic paracrine action. Sci. Rep. 6:25689. doi: 10.1038/srep25689
Lopatina, T., Bruno, S., Tetta, C., Kalinina, N., Porta, M., and Camussi, G. (2014). Platelet-derived growth factor regulates the secretion of extracellular vesicles by adipose mesenchymal stem cells and enhances their angiogenic potential. Cell Commun. Signal. 12:26. doi: 10.1186/1478-811X-12-26
Manavski, Y., Lucas, T., Glaser, S. F., Dorsheimer, L., Günther, S., Braun, T., et al. (2018). Clonal expansion of endothelial cells contributes to ischemia-induced neovascularization. Circ. Res. 122, 670–677. doi: 10.1161/CIRCRESAHA.117.312310
Mantel, P.-Y., Hjelmqvist, D., Walch, M., Kharoubi-Hess, S., Nilsson, S., Ravel, D., et al. (2016). Infected erythrocyte-derived extracellular vesicles alter vascular function via regulatory Ago2-miRNA complexes in malaria. Nat. Commun. 7:2727. doi: 10.1038/ncomms12727
Mao, Y., Wang, Y., Dong, L., Zhang, Y., Zhang, Y., Wang, C., et al. (2019). Hypoxic exosomes facilitate angiogenesis and metastasis in esophageal squamous cell carcinoma through altering the phenotype and transcriptome of endothelial cells. J. Exp. Clin. Cancer Res. 38:389. doi: 10.1186/s13046-019-1384-8
Marchetti, M., Meloni, M., Anwar, M., Zen, A. A. H., Sala-Newby, G., Slater, S., et al. (2020). MicroRNA-24-3p targets notch and other vascular morphogens to regulate post-ischemic microvascular responses in limb muscles. Int. J. Mol. Sci. 21:1733. doi: 10.3390/ijms21051733
Martello, A., Mellis, D., Meloni, M., Howarth, A., Ebner, D., Caporali, A., et al. (2018). Phenotypic miRNA screen identifies miR-26b to promote the growth and survival of endothelial cells. Mol. Ther. Nucleic Acids 13, 29–43. doi: 10.1016/j.omtn.2018.08.006
Mateescu, B., Kowal, E. J. K., van Balkom, B. W. M., Bartel, S., Bhattacharyya, S. N., Buzás, E. I., et al. (2017). Obstacles and opportunities in the functional analysis of extracellular vesicle RNA - an ISEV position paper. J. Extracell. Vesicles 6:1286095. doi: 10.1080/20013078.2017.1286095
Mathiyalagan, P., Liang, Y., Kim, D., Misener, S., Thorne, T., Kamide, C. E., et al. (2017). Angiogenic mechanisms of human CD34+ stem cell exosomes in the repair of ischemic hindlimb. Circ. Res. 120, 1466–1476. doi: 10.1161/CIRCRESAHA.116.310557
McCann, J. V., Bischoff, S. R., Zhang, Y., Cowley, D. O., Sanchez-Gonzalez, V., Daaboul, G. D., et al. (2020). Reporter mice for isolating and auditing cell type-specific extracellular vesicles in vivo. Genesis 58:e23369. doi: 10.1002/dvg.23369
McKenzie, A. J., Hoshino, D., Hong, N. H., Cha, D. J., Franklin, J. L., Coffey, R. J., et al. (2016). KRAS-MEK signaling controls Ago2 sorting into exosomes. Cell Rep. 15, 978–987. doi: 10.1016/j.celrep.2016.03.085
Melo, S. A., Sugimoto, H., OConnell, J. T., Kato, N., Villanueva, A., Vidal, A., et al. (2014). Cancer exosomes perform cell-independent microRNA biogenesis and promote tumorigenesis. Cancer Cell 26, 707–21. doi: 10.1016/j.ccell.2014.09.005
Menasché, P., Vanneaux, V., Hagège, A., Bel, A., Cholley, B., Parouchev, A., et al. (2018). Transplantation of human embryonic stem cell–derived cardiovascular progenitors for severe ischemic left ventricular dysfunction. J. Am. Coll. Cardiol. 71, 429–438. doi: 10.1016/j.jacc.2017.11.047
Menghini, R., Casagrande, V., Cardellini, M., Martelli, E., Terrinoni, A., Amati, F., et al. (2009). MicroRNA 217 modulates endothelial cell senescence via silent information regulator 1. Circulation 120, 1524–1532. doi: 10.1161/CIRCULATIONAHA.109.864629
Merckx, G., Hosseinkhani, B., Kuypers, S., Deville, S., Irobi, J., Nelissen, I., et al. (2020). Angiogenic effects of human dental pulp and bone marrow-derived mesenchymal stromal cells and their extracellular vesicles. Cells 9:312. doi: 10.3390/cells9020312
Mirotsou, M., Jayawardena, T. M., Schmeckpeper, J., Gnecchi, M., and Dzau, V. J. (2011). Paracrine mechanisms of stem cell reparative and regenerative actions in the heart. J. Mol. Cell. Cardiol. 50, 280–9. doi: 10.1016/j.yjmcc.2010.08.005
Mondal, A., Ashiq, K. A., Phulpagar, P., Singh, D. K., and Shiras, A. (2019). Effective visualization and easy tracking of extracellular vesicles in glioma cells. Biol. Proced. Online 21:4. doi: 10.1186/s12575-019-0092-2
Morel, O., Toti, F., Morel, N., and Freyssinet, J.-M. (2009). Microparticles in endothelial cell and vascular homeostasis: are they really noxious? Haematologica 94, 313–317. doi: 10.3324/haematol.2008.003657
Morelli, A. E., Larregina, A. T., Shufesky, W. J., Sullivan, M. L. G., Stolz, D. B., Papworth, G. D., et al. (2004). Endocytosis, intracellular sorting, and processing of exosomes by dendritic cells. Blood 104, 3257–3266. doi: 10.1182/blood-2004-03-0824
Mulcahy, L. A., Pink, R. C., and Carter, D. R. F. (2014). Routes and mechanisms of extracellular vesicle uptake. J. Extracell. Vesicles 3. doi: 10.3402/jev.v3.24641
Nazarenko, I., Rana, S., Baumann, A., McAlear, J., Hellwig, A., Trendelenburg, M., et al. (2010). Cell surface tetraspanin Tspan8 contributes to molecular pathways of exosome-induced endothelial cell activation. Cancer Res. 70, 1668–1678. doi: 10.1158/0008-5472.CAN-09-2470
Nordin, J. Z., Lee, Y., Vader, P., Mäger, I., Johansson, H. J., Heusermann, W., et al. (2015). Ultrafiltration with size-exclusion liquid chromatography for high yield isolation of extracellular vesicles preserving intact biophysical and functional properties. Nanomed. Nanotechnol. Biol. Med. 11, 879–883. doi: 10.1016/j.nano.2015.01.003
Nozaki, T., Sugiyama, S., Koga, H., Sugamura, K., Ohba, K., Matsuzawa, Y., et al. (2009). Significance of a multiple biomarkers strategy including endothelial dysfunction to improve risk stratification for cardiovascular events in patients at high risk for coronary heart disease. J. Am. Coll. Cardiol. 54, 601–608. doi: 10.1016/j.jacc.2009.05.022
O'Brien, J., Hayder, H., Zayed, Y., and Peng, C. (2018). Overview of MicroRNA biogenesis, mechanisms of actions, and circulation. Front. Endocrinol. 9:402. doi: 10.3389/fendo.2018.00402
Ong, S.-G., and Wu, J. C. (2015). Exosomes as potential alternatives to stem cell therapy in mediating cardiac regeneration. Circul. Res. 117, 7–9. doi: 10.1161/CIRCRESAHA.115.306593
Ostrowski, M., Carmo, N. B., Krumeich, S., Fanget, I., Raposo, G., Savina, A., et al. (2010). Rab27a and Rab27b control different steps of the exosome secretion pathway. Nat. Cell Biol. 12, 19–30. doi: 10.1038/ncb2000
Ou, Z.-J., Chang, F.-J., Luo, D., Liao, X.-L., Wang, Z.-P., Zhang, X., et al. (2011). Endothelium-derived microparticles inhibit angiogenesis in the heart and enhance the inhibitory effects of hypercholesterolemia on angiogenesis. Am. J. Physiol. Endocrinol. Metab. 300, E661–E668. doi: 10.1152/ajpendo.00611.2010
Pankratz, F., Bemtgen, X., Zeiser, R., Leonhardt, F., Kreuzaler, S., Hilgendorf, I., et al. (2015). MicroRNA-155 exerts cell-specific antiangiogenic but proarteriogenic effects during adaptive neovascularization. Circulation 131, 1575–1589. doi: 10.1161/CIRCULATIONAHA.114.014579
Persson, A. B., and Buschmann, I. R. (2011). Vascular growth in health and disease. Front. Mol. Neurosci. 4:14. doi: 10.3389/fnmol.2011.00014
Poliseno, L., Tuccoli, A., Mariani, L., Evangelista, M., Citti, L., Woods, K., et al. (2006). MicroRNAs modulate the angiogenic properties of HUVECs. Blood 108, 3068–3071. doi: 10.1182/blood-2006-01-012369
Potz, B. A., Scrimgeour, L. A., Pavlov, V. I., Sodha, N. R., Abid, M. R., and Sellke, F. W. (2018). Extracellular vesicle injection improves myocardial function and increases angiogenesis in a swine model of chronic ischemia. J. Am. Heart Assoc. 7:e008344. doi: 10.1161/JAHA.117.008344
Prieto-Fernández, E., Aransay, A. M., Royo, F., González, E., Lozano, J. J., Santos-Zorrozua, B., et al. (2019). A comprehensive study of vesicular and non-vesicular mirnas from a volume of cerebrospinal fluid compatible with clinical practice. Theranostics 9, 4567–4579. doi: 10.7150/thno.31502
Qiao, L., Hu, S., Liu, S., Zhang, H., Ma, H., Huang, K., et al. (2019). microRNA-21-5p dysregulation in exosomes derived from heart failure patients impairs regenerative potential. The J. Clin. Invest. 129, 2237–2250. doi: 10.1172/JCI123135
Qiao, Y., Ma, N., Wang, X., Hui, Y., Li, F., Xiang, Y., et al. (2011). MiR-483-5p controls angiogenesis in vitro and targets serum response factor. FEBS Lett. 585, 3095–3100. doi: 10.1016/j.febslet.2011.08.039
Ranghino, A., Cantaluppi, V., Grange, C., Vitillo, L., Fop, F., Biancone, L., et al. (2012). Endothelial progenitor cell-derived microvesicles improve neovascularization in a murine model of hindlimb ischemia. Int. J. Immunopathol. Pharmacol. 25, 75–85. doi: 10.1177/039463201202500110
Rao, X., Wan, L., Jie, Z., Zhu, X., Yin, J., and Cao, H. (2019). Upregulated miR-27a-3p indicates a poor prognosis in pancreatic carcinoma patients and promotes the angiogenesis and migration by epigenetic silencing of GATA6 and activating VEGFA/VEGFR2 signaling pathway. OncoTargets Ther. 12, 11241–11254. doi: 10.2147/OTT.S220621
Raposo, G., and Stahl, P. D. (2019). Extracellular vesicles: a new communication paradigm? Nat. Rev. Mol. Cell Biol. 20, 509–510. doi: 10.1038/s41580-019-0158-7
Raposo, G., and Stoorvogel, W. (2013). Extracellular vesicles: exosomes, microvesicles, and friends. J. Cell Biol. 200, 373–383. doi: 10.1083/jcb.201211138
Ribeiro-Rodrigues, T. M., Laundos, T. L., Pereira-Carvalho, R., Batista-Almeida, D., Pereira, R., Coelho-Santos, V., et al. (2017). Exosomes secreted by cardiomyocytes subjected to ischaemia promote cardiac angiogenesis. Cardiovasc. Res. 113, 1338–1350. doi: 10.1093/cvr/cvx118
Risau, W. (1997). Mechanisms of Angiogenesis. Available online at: https://www.nature.com/articles/386671a0.pdf (accessed April 20, 2020).
Romagnuolo, R., Masoudpour, H., Porta-Sánchez, A., Qiang, B., Barry, J., Laskary, A., et al. (2019). Human embryonic stem cell-derived cardiomyocytes regenerate the infarcted pig heart but induce ventricular tachyarrhythmias. Stem Cell Rep. 12, 967–981. doi: 10.1016/j.stemcr.2019.04.005
Safety Tolerability Pharmacokinetics Pharmacodynamics of MRG. 110 Following Intradermal Injection in Healthy Volunteers - Full Text View - ClinicalTrials.gov. (2018). Available online at: https://clinicaltrials.gov/ct2/show/NCT03603431 (accessed April 28, 2020).
Savina, A., Vidal, M., and Colombo, M. I. (2002). The exosome pathway in K562 cells is regulated by Rab11. J. Cell Sci. 115, 2505–15.
Schaper, W., and Pasyk, S. (1976). Influence of collateral flow on the ischemic tolerance of the heart following acute and subacute coronary occlusion. Circulation 53 (3 Suppl.), I57–62.
Schober, A., Nazari-Jahantigh, M., Wei, Y., Bidzhekov, K., Gremse, F., Grommes, J., et al. (2014). MicroRNA-126-5p promotes endothelial proliferation and limits atherosclerosis by suppressing Dlk1. Nat. Med. 20, 368–376. doi: 10.1038/nm.3487
Semo, J. (2013) The 106b~25 microRNA cluster is essential for neovascularization after hindlimb ischaemia in mice. Eur. Heart J. 35, 3212–3223. doi: 10.1093/eurheartj/eht041.
Short, R. H. D. (1950). Alveolar epithelium in relation to growth of the lung. Philos. Trans. R Soc. Lond. B Biol. Sci. 235, 35–86. doi: 10.1098/rstb.1950.0014
Simion, V., Haemmig, S., and Feinberg, M. W. (2019). LncRNAs in vascular biology and disease. Vasc. Pharmacol. 114, 145–156. doi: 10.1016/j.vph.2018.01.003
Singh, M., Jadhav, H. R., and Bhatt, T. (2017). Dynamin functions and ligands: classical mechanisms behind. Mol. Pharmacol. 91, 123–134. doi: 10.1124/mol.116.105064
Sódar, B. W., Kittel, Á., Pálóczi, K., Vukman, K. V., Osteikoetxea, X., Szabó-Taylor, K., et al. (2016). Low-density lipoprotein mimics blood plasma-derived exosomes and microvesicles during isolation and detection. Sci. Rep. 6:24316. doi: 10.1038/srep24316
Stratman, A. N., and Davis, G. E. (2012). Endothelial cell-pericyte interactions stimulate basement membrane matrix assembly: influence on vascular tube remodeling, maturation, and stabilization. Microsc. Microana.l 18, 68–80. doi: 10.1017/S1431927611012402
Svensson, K. J., Christianson, H. C., Wittrup, A., Bourseau-Guilmain, E., Lindqvist, E., Svensson, L. M., et al. (2013). Exosome uptake depends on ERK1/2-heat shock protein 27 signaling and lipid raft-mediated endocytosis negatively regulated by caveolin-1. J. Biol. Chem. 288, 17713–17724. doi: 10.1074/jbc.M112.445403
Teng, X., Chen, L., Chen, W., Yang, J., Yang, Z., and Shen, Z. (2015). Mesenchymal stem cell-derived exosomes improve the microenvironment of infarcted myocardium contributing to angiogenesis and anti-inflammation. Cell. Physiol. Biochem. 37, 2415–2424. doi: 10.1159/000438594
Thygesen, K., Alpert, J. S., Jaffe, A. S., Chaitman, B. R., Bax, J. J., Morrow, D. A., et al. (2019). Fourth universal definition of myocardial infarction 2018. Eur. Heart J. 40, 237–269. doi: 10.1093/eurheartj/ehy462
Vagnozzi, R. J., Maillet, M., Sargent, M. A., Khalil, H., Johansen, A. K. Z., Schwanekamp, J. A., et al. (2020). An acute immune response underlies the benefit of cardiac stem cell therapy. Nature 577, 405–409. doi: 10.1038/s41586-019-1802-2
Vanlandingham, P. A., and Ceresa, B. P. (2009). Rab7 regulates late endocytic trafficking downstream of multivesicular body biogenesis and cargo sequestration. J. Biol. Chem. 284, 12110–12124. doi: 10.1074/jbc.M809277200
Veliceasa, D., Biyashev, D., Qin, G., Misener, S., Mackie, A. R., Kishore, R., et al. (2015). Therapeutic manipulation of angiogenesis with miR-27b. Vasc. Cell 7:6. doi: 10.1186/s13221-015-0031-1
Villarroya-Beltri, C., Gutiérrez-Vázquez, C., Sánchez-Cabo, F., Pérez-Hernández, D., Vázquez, J., Martin-Cofreces, N., et al. (2013). Sumoylated hnRNPA2B1 controls the sorting of miRNAs into exosomes through binding to specific motifs. Nat. Commun. 4:3980. doi: 10.1038/ncomms3980
Wahlund, C. J. E., Güclüler, G., Hiltbrunner, S., Veerman, R. E., Näslund, T. I., and Gabrielsson, S. (2017). Exosomes from antigen-pulsed dendritic cells induce stronger antigen-specific immune responses than microvesicles in vivo. Sci. Rep. 7:17095. doi: 10.1038/s41598-017-16609-6
Wang, H.-W., Huang, T.-S., Lo, H.-H., Huang, P.-H., Lin, C.-C., Chang, S.-J., et al. (2014). Deficiency of the MicroRNA-31–MicroRNA-720 pathway in the plasma and endothelial progenitor cells from patients with coronary artery disease. Arterioscler. Thromb. Vasc. Biol. 34, 857–869. doi: 10.1161/ATVBAHA.113.303001
Wang, N., Chen, C., Yang, D., Liao, Q., Luo, H., Wang, X., et al. (2017). Mesenchymal stem cells-derived extracellular vesicles, via miR-210, improve infarcted cardiac function by promotion of angiogenesis. Biochim. Biophys. Acta. 1863, 2085–2092. doi: 10.1016/j.bbadis.2017.02.023
Wang, S., Aurora, A. B., Johnson, B. A., Qi, X., McAnally, J., Hill, J. A., et al. (2008). The endothelial-specific MicroRNA miR-126 governs vascular integrity and angiogenesis. Dev. Cell 15, 261–271. doi: 10.1016/j.devcel.2008.07.002
Wang, X., Ling, C. C., Li, L., Qin, Y., Qi, J., Liu, X., et al. (2016). MicroRNA-10a/10b represses a novel target gene mib1 to regulate angiogenesis. Cardiovasc. Res. 110, 140–150. doi: 10.1093/cvr/cvw023
Wei, Z., Batagov, A. O., Carter, D. R. F., and Krichevsky, A. M. (2016). Fetal bovine serum RNA interferes with the cell culture derived extracellular RNA. Sci. Rep. 6:31175. doi: 10.1038/srep31175
Welten, S. M. J., Bastiaansen, A. J. N. M., de Jong, R. C. M., de Vries, M. R., Peters, E. A. B., Boonstra, M. C., et al. (2014). Inhibition of 14q32 MicroRNAs miR-329, miR-487b, miR-494, and miR-495 increases neovascularization and blood flow recovery after ischemia. Circ. Res. 115, 696–708. doi: 10.1161/CIRCRESAHA.114.304747
Werner, N., Wassmann, S., Ahlers, P., Kosiol, S., and Nickenig, G. (2006). Circulating CD31 + /annexin V + apoptotic microparticles correlate with coronary endothelial function in patients with coronary artery disease. Arterioscler. Thromb. Vasc. Biol. 26, 112–116. doi: 10.1161/01.A.T.V.0000191634.13057.15
WHO Cardiovascular diseases CVDs (2017). Available online at: http://www.who.int/en/news-room/fact-sheets/detail/cardiovascular-diseases-(cvds) (accessed May 19, 2018).
Witwer, K. W., Buzás, E. I., Bemis, L. T., Bora, A., Lässer, C., Lötvall, J., et al. (2013). Standardization of sample collection, isolation and analysis methods in extracellular vesicle research. J. Extracell. Vesicles 2:20360. doi: 10.3402/jev.v2i0.20360
Wu, Q., Wang, J., Tan, W. L. W., Jiang, Y., Wang, S., Li, Q., et al. (2020). Extracellular vesicles from human embryonic stem cell-derived cardiovascular progenitor cells promote cardiac infarct healing through reducing cardiomyocyte death and promoting angiogenesis. Cell Death Dis. 11:354. doi: 10.1038/s41419-020-2508-y
Xu, X., Huang, J., Yu, L., Xiang, Q., Chen, Q., Long, X., et al. (2019). Exosomal miR-423-5p mediates the proangiogenic activity of human adipose-derived stem cells by targeting sufu. Stem Cell Res. Ther. 10:106. doi: 10.1186/s13287-019-1196-y
Yamada, N., Tsujimura, N., Kumazaki, M., Shinohara, H., Taniguchi, K., Nakagawa, Y., et al. (2014). Colorectal cancer cell-derived microvesicles containing microRNA-1246 promote angiogenesis by activating Smad 1/5/8 signaling elicited by PML down-regulation in endothelial cells. Biochim. Biophys. Acta. 1839, 1256–1272. doi: 10.1016/j.bbagrm.2014.09.002
Yan, X. C., Cao, J., Liang, L., Wang, L., Gao, F., Yang, Z. Y., et al. (2016). miR-342-5p is a notch downstream molecule and regulates multiple angiogenic pathways including notch, vascular endothelial growth factor and transforming growth factor β signaling. J. Am. Heart Assoc. 5:e003042. doi: 10.1161/JAHA.115.003042
Yang, F., Liu, W., Yan, X., Zhou, H., Zhang, H., Liu, J., et al. (2016). Effects of mir-21 on cardiac microvascular endothelial cells after acute myocardial infarction in rats: role of phosphatase and tensin homolog (PTEN)/vascular endothelial growth factor (VEGF) signal pathway. Med. Sci. Monitor 22, 3562–3575. doi: 10.12659/MSM.897773
Yang, Y., Luo, H., Zhou, C., Zhang, R., Liu, S., Zhu, X., et al. (2019). Regulation of capillary tubules and lipid formation in vascular endothelial cells and macrophages via extracellular vesicle-mediated microRNA-4306 transfer. J. Int. Med. Res. 47, 453–469. doi: 10.1177/0300060518809255
Yang, Z., Wu, L., Zhu, X., Xu, J., Jin, R., Li, G., et al. (2013). MiR-29a modulates the angiogenic properties of human endothelial cells. Biochem. Biophys. Res. Commun. 434, 143–149. doi: 10.1016/j.bbrc.2013.03.054
Ye, L., DAgostino, G., Loo, S. J., Wang, C. X., Su, L. P., Tan, S. H., et al. (2018). Early regenerative capacity in the porcine heart. Circulation 138, 2798–2808. doi: 10.1161/CIRCULATIONAHA.117.031542
Yin, K. J., Olsen, K., Hamblin, M., Zhang, J., Schwendeman, S. P., and Chen, Y. E. (2012). Vascular endothelial cell-specific MicroRNA-15a inhibits angiogenesis in hindlimb ischemia. J. Biol. Chem. 287, 27055–27064. doi: 10.1074/jbc.M112.364414
Yuana, Y., Levels, J., Grootemaat, A., Sturk, A., and Nieuwland, R. (2014). Co-isolation of extracellular vesicles and high-density lipoproteins using density gradient ultracentrifugation. J. Extracell. Vesicles 3:10.3402/jev.v3.23262. doi: 10.3402/jev.v3.23262
Zhang, H., Freitas, D., Kim, H. S., Fabijanic, K., Li, Z., Chen, H., et al. (2018). Identification of distinct nanoparticles and subsets of extracellular vesicles by asymmetric flow field-flow fractionation. Nat. Cell Biol. 20, 332–343. doi: 10.1038/s41556-018-0040-4
Zhang, K., Zhao, X., Chen, X., Wei, Y., Du, W., Wang, Y., et al. (2018). Enhanced therapeutic effects of mesenchymal stem cell-derived exosomes with an injectable hydrogel for hindlimb ischemia treatment. ACS Appl. Mater. Interfaces. 10:30081–30091. doi: 10.1021/acsami.8b08449
Zhang, Q., Higginbotham, J. N., Jeppesen, D. K., Yang, Y.-P., Li, W., McKinley, E. T., et al. (2019). Transfer of functional cargo in exomeres. Cell Rep. 27, 940–954.e6. doi: 10.1016/j.celrep.2019.01.009
Zhang, T.-R., and Huang, W.-Q. (2020). Angiogenic circular RNAs: a new landscape in cardiovascular diseases. Microvasc. Res. 129:103983. doi: 10.1016/j.mvr.2020.103983
Zhao, Y., Yan, M., Chen, C., Gong, W., Yin, Z., Li, H., et al. (2018). MiR-124 aggravates failing hearts by suppressing CD151-facilitated angiogenesis in heart. Oncotarget 9, 14382–14396. doi: 10.18632/oncotarget.24205
Zhao, Z., Sun, W., Guo, Z., Zhang, J., Yu, H., and Liu, B. (2020). Mechanisms of lncRNA/microRNA interactions in angiogenesis. Life Sci. 254:116900. doi: 10.1016/j.lfs.2019.116900
Zhou, Q., Gallagher, R., Ufret-Vincenty, R., Li, X., Olson, E. N., and Wang, S. (2011). Regulation of angiogenesis and choroidal neovascularization by members of microRNA-23~27~24 clusters. Proc. Natl. Acad. Sci. U. S. A. 108, 8287–8292. doi: 10.1073/pnas.1105254108
Zhu, J., Lu, K., Zhang, N., Zhao, Y., Ma, Q., Shen, J., et al. (2018). Myocardial reparative functions of exosomes from mesenchymal stem cells are enhanced by hypoxia treatment of the cells via transferring microRNA-210 in an nSMase2-dependent way. Artif. Cells Nanomed. Biotechnol. 46, 1659–1670. doi: 10.1080/21691401.2017.1388249
Zhu, L. P., Zhou, J. P., Zhang, J. X., Wang, J. Y., Wang, Z. Y., Pan, M., et al. (2017). MiR-15b-5p regulates collateral artery formation by targeting AKT3 (Protein Kinase B-3). Arterioscler. Thromb. Vasc. Biol. 37, 957–968. doi: 10.1161/ATVBAHA.116.308905
Zomer, A., Maynard, C., Verweij, F. J., Kamermans, A., Schäfer, R., Beerling, E., et al. (2015). In vivo imaging reveals extracellular vesicle-mediated phenocopying of metastatic behavior. Cell 161, 1046–1057. doi: 10.1016/j.cell.2015.04.042
Zomer, A., Steenbeek, S. C., Maynard, C., and Van Rheenen, J. (2016). Studying extracellular vesicle transfer by a Cre-loxP method. Nat. Protoc. 11, 87–101. doi: 10.1038/nprot.2015.138
Keywords: extracellular vesicles (EV), microRNA (miR), neovascularisation, angiogenesis, cardiac, myocardial infarct, exosome (EXO), regeneration
Citation: Kesidou D, da Costa Martins PA, de Windt LJ, Brittan M, Beqqali A and Baker AH (2020) Extracellular Vesicle miRNAs in the Promotion of Cardiac Neovascularisation. Front. Physiol. 11:579892. doi: 10.3389/fphys.2020.579892
Received: 03 July 2020; Accepted: 25 August 2020;
Published: 25 September 2020.
Edited by:
Reinier Boon, Goethe University Frankfurt, GermanyReviewed by:
Jason E. Fish, University Health Network (UHN), CanadaKasey C. Vickers, Vanderbilt University Medical Center, United States
Peifeng Li, Qingdao University, China
Copyright © 2020 Kesidou, da Costa Martins, de Windt, Brittan, Beqqali and Baker. This is an open-access article distributed under the terms of the Creative Commons Attribution License (CC BY). The use, distribution or reproduction in other forums is permitted, provided the original author(s) and the copyright owner(s) are credited and that the original publication in this journal is cited, in accordance with accepted academic practice. No use, distribution or reproduction is permitted which does not comply with these terms.
*Correspondence: Andrew Howard Baker, YW5keS5iYWtlciYjeDAwMDQwO2VkLmFjLnVr; Abdelaziz Beqqali, YS5iZXFxYWxpJiN4MDAwNDA7ZWQuYWMudWs=