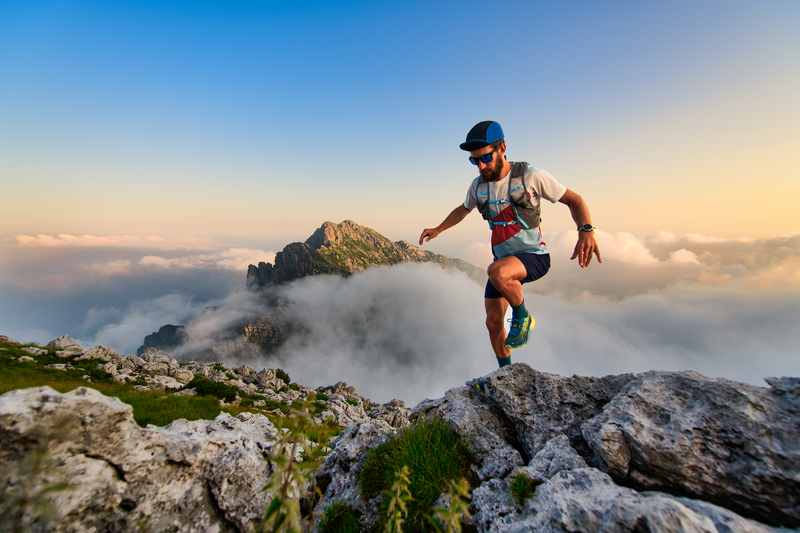
95% of researchers rate our articles as excellent or good
Learn more about the work of our research integrity team to safeguard the quality of each article we publish.
Find out more
ORIGINAL RESEARCH article
Front. Physiol. , 11 September 2020
Sec. Gastrointestinal Sciences
Volume 11 - 2020 | https://doi.org/10.3389/fphys.2020.545184
Hydrogen sulfide (H2S) is a neuromodulator in the central nervous system. However, the physiological role of H2S in the nucleus ambiguus (NA) has rarely been reported. This research aimed to elucidate the role of H2S in the regulation of gastrointestinal motility in rats. Male Wistar rats were randomly assigned to sodium hydrosulfide (NaHS; 4 and 8 nmol) groups, physiological saline (PS) group, capsazepine (10 pmol) + NaHS (4 nmol) group, L703606 (4 nmol) + NaHS (4 nmol) group, and pyrrolidine dithiocarbamate (PDTC, 4 nmol) + NaHS (4 nmol) group. Gastrointestinal motility curves before and after the injection were recorded using a latex balloon attached with a pressure transducer, which was introduced into the pylorus through gastric fundus. The results demonstrated that NaHS (4 and 8 nmol), an exogenous H2S donor, remarkably suppressed gastrointestinal motility in the NA of rats (P < 0.01). The suppressive effect of NaHS on gastrointestinal motility could be prevented by capsazepine, a transient receptor potential vanilloid 1 (TRPV1) antagonist, and PDTC, a NF-κB inhibitor. However, the same amount of PS did not induce significant changes in gastrointestinal motility (P > 0.05). Our findings indicate that NaHS within the NA can remarkably suppress gastrointestinal motility in rats, possibly through TRPV1 channels and NF-κB-dependent mechanism.
Hydrogen sulfide (H2S) is an essential neuromodulator in mammals (Zheng et al., 2018), which regulates numerous pathophysiological functions in the digestive, respiratory, circulatory and nervous systems (Kimura et al., 2012; Drapala et al., 2017; Yang et al., 2017; Yu et al., 2017; Zhou et al., 2018). Endogenous H2S levels have been detected in the brains of rats and humans (Wang, 2012; Yang and He, 2019). The concentrations of endogenous H2S are typically 47–166 μmol L–1 in the brain (Furne et al., 2008). Free H2S is ≤ 9.2 μM in the brain. The release of H2S was maximal at pH 1.5 and gradually decreased with higher pH up to 5.4. H2S released by HCl alone was 161 ± 5 nmol/g protein, whereas that by DTT alone was 1,481 ± 174 nmol/g protein (Warenycia et al., 1989; Ishigami et al., 2009). And such high content suggests that H2S may exert a physiological function (Goodwin et al., 1989; Fiorucci et al., 2006).
H2S is a gasotransmitter produced primarily by cystathionine-β-synthase (CBS) enzyme in the CNS (Swaroop et al., 1992; Calvert et al., 2010; Wang, 2012). Endogenous H2S affects cardiac function in the nucleus tractus solitarii by regulating ATP-sensitive potassium (KATP) channels and/or glutamate receptors (Qiao et al., 2011). Microinjecting L-glutamate into the nucleus ambiguus (NA) can suppress gastrointestinal motility via activating specific N-methyl-D-aspartate receptor (Sun et al., 2010). In our previous works (please see Supplementary Files), we found that CBS is localized in NA. These experimental findings indicate that H2S in the NA can participate in the regulation of gastric functions.
H2S regulates many physiological and pathological processes through ion channels. Several recent publications indicated the ability of H2S to activate TRPV1 or TRPA1 receptors in vitro and in vivo experiments (Koroleva et al., 2017). Thus, the TRPV1 antagonist prevented NaHS-evoked luminal chloride secretion (Storti et al., 2015). It initiates the activation of transient receptor potential vanilloid 1 (TRPV1) channels in the urinary bladder of rats and airway of guinea pigs, thus leading to bladder obstruction and airway constriction via neurogenic inflammation (Patacchini et al., 2004; Trevisani et al., 2005). NaHS promotes gastric acid secretion by activating TRPV1 channels in the sensory nerve endings with the subsequent release of substance P (SP) in an NF-κB-dependent manner (Sun et al., 2018). Lu et al. (2014) found that capsazepine, TRPV1 channel antagonists, and L703606, a NK1 receptor antagonist, significantly attenuated the excitatory responses evoked by NaHS, which indicates that NaHS might activate TRPV1 channels in the afferent nerve fibers with the consequent release of SP. However, the mechanism underlies H2S-induced regulation of gastric motility in the NA remains largely unclear.
The transcription factor NF-κB is a pleiotropic mediator of target genes that modulates many physiological functions (Gutierrez et al., 2005; Meffert and Baltimore, 2005; Blank and Prinz, 2014; Haenold et al., 2014). H2S protects gastric mucosal cells from ischemia-reperfusion damage by regulating NF-κB-dependent anti-inflammatory activity. Besides, Keap1 S-sulfhydration anti-apoptosis pathway also protects against water-immersion and restraint stress-induced gastric damage in rats, by opening KATP channel and activating NF-κB-dependent pathway (Guo et al., 2014). Ang et al. (2011) have reported that H2S can regulate TRPV1-induced neurogenic inflammation in sepsis by enhancing SP production and activating ERK-NF-κB pathway. This study aimed to elucidate the role of H2S in regulating gastrointestinal motility in rats, and to investigate whether the effect of H2S is resulted from the activation of TRPV1 channels though a NF-κB-dependent manner.
Wistar rats (male; weighing 270–320 g) were supplied by the Experimental Animal Center of Shandong University. The animals were housed at a constant temperature under a 12:12 h light–dark cycle, and were given ad libitum access to water and food for 7 days. Before starting the experiments, the rats were deprived of food for 24 h. Ethical approval for this study was obtained from the Experimental Animal Ethics Committee of Qilu Normal University, and all experimental protocols were conducted in accordance with the guidelines of the International Association for the Study of Pain (Zimmermann, 1986).
NaHS (4 and 8 nmol) (Sun et al., 2015), capsazepine (10 pmol), L703606 (4 nmol), PDTC (4 nmol), and pontamine sky blue were all supplied by Sigma-Aldrich (St. Louis, MO, United States). NaHS was dissolved in 0.9% saline, while other chemicals were dissolved in DMSO.
Anesthetic procedure was carried out by intraperitoneally injecting chloral hydrate (400 mg/kg body weight) into the rats. Laparotomy was performed, and a warm water-filled balloon (5 mm in diameter) was introduced into the pylorus via a small incision in the forestomach wall. Gastrointestinal motility curves were determined using BL-420F (Biological Function Experimental System; Chengdu Taimeng Company, China) through a pressure transducer.
The anesthetized rats were transferred into a stereotaxic apparatus (Stoelting 68002, Shenzhen Ruiwode Company, China). After performing an occipital craniotomy, a glass micropipette (external tip diameter: 30–50 μm) with pneumatic pump was placed vertically in the right NA. The position was identified based on the Paxinos and Watson rat brain atlas (Paxinos and Watson, 2007).
A series of experiments was perform to assess the effects of H2S on gastrointestinal motility in the NA. (i) Microinjection of NaHS (0.1 μL, 4 nmol) into the NA (n = 6); (ii) microinjection of NaHS (0.1 μL, 8 nmol) into the NA (n = 5); (iii) microinjection of physiological saline (PS; 0.1 μL) the NA (n = 4) as control group; (iv) microinjection of NaHS (0.1 μL, 4 nmol) and capsazepine (0.1 μL, 10 pmol) into the NA (n = 6); (v) microinjection of L703606 (0.1 μL, 4 nmol) and NaHS (0.1 μL, 4 nmol) into the NA (n = 5); and (vi) microinjection of PDTC (0.1 μL, 4 nmol) and NaHS (0.1 μL, 4 nmol) into the NA (n = 5).
After completion of each experiment, 2% pontamine sky blue (0.1 μL) was administered at the same microinjection site. The reaction was terminated though an intravenous bolus injection of 80 mg/kg pentobarbital sodium. Next, the rats were transcardially perfused with PS, followed by fixation in 4% paraformaldehyde. Then, the brains were isolated and placed in 4% paraformaldehyde containing 20% sucrose for approximately 48–72 h. The brainstem samples (40 μm in thickness) were snap-frozen and sectioned, followed by neutral red staining. Statistical analyses were performed for the results in which the tip of the microinjection site was localized within the NA.
The total amplitude and total duration of gastric contraction waves were measured 5 min pre- and post-microinjection. Gastric motility index (GMI) was calculated as a function of the amplitude and duration of each contraction wave. To determine the changes in gastrointestinal motility parameters pre- and post-microinjection, the rates of inhibition were calculated as follows: Inhibition rate (%) = (pre-microinjection value – post-microinjection value) × 100% / pre-microinjection value (Sun et al., 2015).
SPSS v23.0 (IBM SPSS Inc., Chicago, IL, United States) was employed to analyze the results. Statistical differences were evaluated using Student’s t-test followed by post hoc testing with the Student–Newman–Keuls test. All data were presented as mean ± standard error. P values of less than 0.05 was deemed as statistically significant.
As shown in Figures 1A,B, NaHS (4 and 8 nmol), administered at the right NA, was found to exert remarkable inhibitory effect on gastrointestinal motility. In contrast, the same amount of PS exhibited no significant effect on gastrointestinal motility (Figure 1C).
Figure 1. Effects of chemicals microinjected into the nucleus ambiguus (NA) on gastric motility. Effects of NaHS (4 nmol in 0.1 μL) microinjected into the NA on gastric motility (representing curve from a rat) (A). Effects of NaHS (8 nmol in 0.1 μL) microinjected into the NA on gastric motility (representing curve from a rat) (B). Representative curve from a rat showing the Effects of 0.1 μL physiological saline (PS) microinjected into the NA on gastric motility (representing curve from a rat) (C).
Moreover, the data measured 5 min pre- and post-microinjection were analyzed and compared. At a dose of 4 nmol NaHS, the total amplitude of contraction waves (TACW), total duration of contraction waves (TDCW) and GMI were declined from 367.89 ± 30.03 mm 5 min–1 to 239.87 ± 13.51 mm 5 min–1 (P < 0.01), 160.88 ± 13.33 s 5 min–1 to 91.68 ± 4.73 s 5 min–1 (P < 0.01) and 8435.57 ± 251.48 to 4662.21 ± 164.32 (P < 0.01), respectively, before and after microinjection (Figures 2A–C). At a dose of 8 nmol NaHS, the values of TACW, TDCW and GMI reduced from 253.04 ± 11.39 mm 5 min–1 to 138.20 ± 3.85 mm 5 min–1 (P < 0.01), 172.60 ± 7.99 s 5 min–1 to 93.60 ± 3.85 s 5 min–1 (P < 0.01), and 5670.14 ± 159.82 to 2766.05 ± 49.35 (P < 0.01), respectively, before and after microinjection (Figures 2A–C).
Figure 2. Representative data for gastric motility before and after microinjection of NaHS (4 and 8 nmol) or physiological saline (PS) into the nucleus ambiguus. Data of T.A.C.W (A). Data for T.D.C.W (B). Data for gastric motility index (C). Average inhibitory rate of T.A.C.W, T.D.C.W and gastric motility index (D). Micro: microinjection; T.A.C.W: total amplitude of contraction waves; T.D.C.W: total duration of contraction waves; ∗∗P < 0.01, versus before microinjection.
As displayed in Figure 2D, the inhibitory rates of TACW in 4 and 8 nmol NaHS groups were found to be 33.95 and 45.07%, respectively. The inhibitory rates of TDCW in 4 and 8 nmol NaHS groups were determined to be 42.03 and 45.68%, respectively. The inhibitory rates of GMI in 4 and 8 nmol NaHS groups were observed to be 44.54 and 51.14%, respectively. Notably, the inhibitory rates of TACW, TDCW, and GMI in 4 nmol NaHS group were relatively lower than those in 8 nmol NaHS group. These results indicate that NaHS within the NA may have a dose-dependent trend in gastrointestinal motility.
Pretreatment with capsazepine (a TRPV1 antagonist) in the NA could attenuate the suppressive effect of NaHS on gastrointestinal motility (Figure 3A). As shown in Figures 3B–D, the values of TACW changed from 261.71 ± 13.09 mm 5 min–1 (pre-microinjection) to 248.01 ± 15.04 mm 5 min–1 (post-microinjection, P > 0.05); those of TDCW changed from 252.03 ± 14.89 s 5 min–1 (pre-microinjection) to 254.95 ± 18.56 s 5 min–1 (post-microinjection, P > 0.05); and those of GMI changed from 7262.51 ± 415.66 (pre-microinjection) to 7342.42 ± 346.59 (post-microinjection, P > 0.05). These findings reveal that NaHS can regulate gastrointestinal motility through TRPV1 channels.
Figure 3. Representative data for gastric motility in the NaHS (4 nmol in 0.1 μL) group and NaHS (4 nmol in 0.1 μL) + Capsazepine group. Gastric motility curve in NaHS + Capsazepine group (A). Data of T.A.C.W (B). Data for T.D.C.W (C). Data for gastric motility index (D). Micro: microinjection; T.A.C.W: total amplitude of contraction waves; T.D.C.W: total duration of contraction waves; ∗∗P < 0.01, versus before microinjection.
The inhibitory effect of NaHS on gastrointestinal motility could also be reduced by pretreatment with L703606 (a NK1 receptor antagonist; Figure 4A). As shown in Figures 4B–D, The values of TACW changed from 342.51 ± 17.49 mm 5 min–1 (pre-microinjection) to 326.13 ± 15.72 mm 5 min–1 (post-microinjection, P > 0.05); those of TDCW changed from 242.20 ± 5.20 s 5 min–1 (pre-microinjection) to 268.00 ± 13.47 s 5 min–1 (post-microinjection, P > 0.05); and those of GMI changed from 10164.75 ± 310.12 (pre-microinjection) to 10385.65 ± 278.73 (post-microinjection, P > 0.05). These findings demonstrate that NaHS can regulate gastrointestinal motility by enhancing SP production.
Figure 4. Representative data for gastric motility in the NaHS (4 nmol in 0.1 μL) group and NaHS (4 nmol in 0.1 μL) + L703606 group. Gastric motility curve in NaHS + L703606 group (A). Data of T.A.C.W (B). Data for T.D.C.W (C). Data for gastric motility index (D). Micro: microinjection; T.A.C.W: total amplitude of contraction waves; T.D.C.W: total duration of contraction waves; ∗∗P < 0.01, versus before microinjection.
The gastrointestinal motility did not change significantly after administration of PDTC into the NA (Figure 5A). As shown in Figures 5B–D, the values of TACW changed from 368.29 ± 15.44 mm 5 min–1 (pre-microinjection) to 371.68 ± 14.81 mm 5 min–1 (post-microinjection, P > 0.05); those of TDCW changed from 147.20 ± 8.72 s 5 min–1 (pre-microinjection) to 151.40 ± 13.52 s 5 min–1 (post-microinjection, P > 0.05); and those of GMI changed from 10216.24 ± 354.10 (pre-microinjection) to 10107.41 ± 396.45 (post-microinjection, P > 0.05). These findings imply that NaHS may govern gastrointestinal motility by activating NF-κB signaling pathway.
Figure 5. Representative data for gastric motility in the NaHS (4 nmol in 0.1 μL) group and NaHS (4 nmol in 0.1 μL) + PDTC group. Gastric motility curve in NaHS + PDTC group (A). Data of T.A.C.W (B). Data for T.D.C.W (C). Data for gastric motility index (D). Micro: microinjection; T.A.C.W: total amplitude of contraction waves; T.D.C.W: total duration of contraction waves; PDTC: pyrrolidine dithiocarbamate; ∗∗P < 0.01, versus before microinjection.
This study provides strong evidence that exogenous H2S is involved in the regulation of gastrointestinal motility within the NA of rats. The values of TACW, TDCW, and GMI were significantly reduced following microinjection of 4 and 8 nmol NaHS (an exogenous H2S donor) into the NA. However, the frequency of gastrointestinal motility did not change regularly. Two doses (4 and 8 nmol) of NaHS were determined from the pretest values and literature data (Sun et al., 2015). The dose-dependent microinjection of NaHS into the NA remarkably suppressed gastrointestinal motility in rats. Our previous experiments have suggested that CBS, which responsible for H2S production, is highly expressed in the dorsal motor nucleus of the vagus (DMV), and the microinjection of NaHS into the DMV could inhibit gastrointestinal motility and promote gastric acid secretion in rats (Sun et al., 2018). Altogether, these findings indicate that H2S within the NA may act as a neuromodulator or transmitter to inhibit the regulation of gastrointestinal motility.
As the centrum of the parasympathetic preganglionic nerve, the NA and DMV, both act effectively on gastric motility (Zhang et al., 2006, 2009; Wang et al., 2007; Sun et al., 2009), which can represent a source of vagal innervation in the gut. The importance of the activity of this autonomic output in regulating gastrointestinal motility has been well-established (Sun et al., 2010). The vagus nerve is the main autonomic pathway through which these effects are eventually mediated by the NA. Both excitatory and inhibitory fibers are found in the vagus nerves (Cruz et al., 2007). It is speculated that the excitatory fibers may be inhibited, while the inhibitory fibers are excited in the vagus nerve following microinjection of NaHS into the NA.
The present findings revealed that capsazepine, a TRPV1 antagonist, could abolish the suppressive effect of NaHS on gastrointestinal motility, indicating that the suppressive effect of NaHS on gastrointestinal motility is regulated by the initiation of TRPV1 channels. TRPV1 channels are members of the ligand-gated ion channel family, which not only driven by the binding of specific lipophilic molecules but also serve as extracellular protons and physical stimuli, such as heat and osmotic stress (Kauer and Gibson, 2009). Most studies have highlighted on the structure and function of TRPV1, but other thermosensitive TRPV subunits, such as TRPV2, TRPV3 and TRPV4, are also found in the CNS (Caterina, 2007). Besides, activation of TRPV1 receptors can increase glutamate release in the DMV (Derbenev et al., 2006; Derbenev and Smith, 2013). Our previous study has found that microinjection of L-glutamate or NaHS into the DMV can inhibit gastrointestinal motility (Sun et al., 2015). These reports suggest that the microinjection of NaHS into the DMV may increase glutamate release by activating TRPV1 receptors, and the vagal parasympathetic nerve fibers innervating gastric smooth muscles are from both DMV and NA. Thus, it is feasible that the microinjection of NaHS into the NA can inhibit gastrointestinal motility via TRPV1 receptor activation.
Our results demonstrated that the suppressive effect of NaHS on gastrointestinal motility could be prevented by PDTC, an NF-κB inhibitor, suggesting that NaHS may act in an NF-κB-dependent manner. H2S, a gaseous transmitter, can diffuse freely across various biological membranes and regulate numerous signaling pathways, including NF-κB signaling pathway (Calvert et al., 2010; Bos et al., 2012). Typically, NF-κB is activated in response to intercellular signals such as cytokines, neurotransmitters, ion channels and neurotrophic factors (Gessi et al., 2016; Numata et al., 2016; Ren et al., 2016; Schlicher et al., 2016; Wang et al., 2016). It has been reported that NaHS can protect against water-immersion and restraint stress-induced gastric damage in rats via NF-κB-dependent pathway (Sun et al., 2017). Several studies have shown that H2S is mediated by the downregulation of NF-κB (Chattopadhyay et al., 2012; Wei et al., 2015; Ma et al., 2017). However, in this study, we found that NaHS inhibited gastric motility in rats, possibly through NF-κB-dependent signaling pathway. The differences between our results and prior findings might be attributed to the different cellular and animal models used.
In conclusion, our findings indicate that exogenous H2S within the NA significantly inhibits gastrointestinal motility in rats. It may occur through the activation of TRPV1 channels and NF-κB-dependent mechanism. This is the first study to elucidate the role of H2S as a promising regulator of gastrointestinal motility in the NA.
All datasets presented in this study are included in the article/supplementary material.
Ethical approval for this study was obtained from the Experimental Animal Ethics Committee of Qilu Normal University.
HoS and HaS conceived and designed the experiments. HD, YS, CL, HJ, XY, ZC, and PT performed the experiments. HoS and HD analyzed the data. HoS and JZ contributed reagents, materials, and analysis tools. HoS wrote the manuscript. HoS and HaS participated in the redaction and correction of the manuscript. All authors contributed to the article and approved the submitted version.
This work was supported by the Natural Science Foundation of Shandong Province (No. ZR2019MC020).
The authors declare that the research was conducted in the absence of any commercial or financial relationships that could be construed as a potential conflict of interest.
We would like to thank Editage (www.editage.cn) and EditSprings (www.editsprings.com) for English language editing.
Ang, S.-F., Moochhala, S. M., MacAry, P. A., and Bhatia, M. (2011). Hydrogen sulfide and neurogenic inflammation in polymicrobial sepsis: involvement of substance P and ERK-NF-kB Signaling. PLoS One 6:e0024535. doi: 10.1371/journal.pone.0024535
Blank, T., and Prinz, M. (2014). NF-kappaB signaling regulates myelination in the CNS. Front. Mol. Neurosci. 7:47. doi: 10.3389/fnmol.2014.00047
Bos, E. M., Snijder, P. M., Jekel, H., Weij, M., Leemans, J. C., van Dijk, M. C., et al. (2012). Beneficial effects of gaseous hydrogen sulfide in hepatic ischemia/reperfusion injury. Transpl. Int. 25, 897–908. doi: 10.1111/j.1432-2277.2012.01514.x
Calvert, J. W., Coetzee, W. A., and Lefer, D. J. (2010). Novel insights into hydrogen sulfide-mediated cytoprotection. Antioxid. Redox. Signal. 12, 1203–1217. doi: 10.1089/ars.2009.2882
Caterina, M. J. (2007). Transient receptor potential ion channels as participants in thermosensation and thermoregulation. Am. J. Physiol. Regul. Integr. Comp. Physiol. 292, 64–76. doi: 10.1152/ajpregu.00446.2006
Chattopadhyay, M., Kodela, R., Nath, N., Barsegian, A., Boring, D., and Kashfi, K. (2012). Hydrogen sulfide-releasing aspirin suppresses NF-κB signaling in estrogen receptor negative breast cancer cells in vitro and in vivo. Biochem. Pharmacol. 83, 723–732. doi: 10.1016/j.bcp.2011.12.019
Cruz, M. T., Murphy, E. C., Sahibzada, N., Verbalis, J. G., and Gillis, R. A. (2007). A reevaluation of the effects of stimulation of the dorsal motor nucleus of the vagus on gastric motility in the rat. Am. J. Physiol. Regul. Integr. Comp. Physiol. 292, 291–307. doi: 10.1152/ajpregu.00863.2005
Derbenev, A. V., Monroe, M. J., Glatzer, N. R., and Smith, B. N. (2006). Vanilloid-mediated heterosynaptic facilitation of inhibitory synaptic input to neurons of the rat dorsal motor nucleus of the vagus. J. Neurosci. 26, 9666–9672. doi: 10.1523/JNEUROSCI.1591-06.2006
Derbenev, A. V., and Smith, B. N. (2013). Dexamethasone rapidly increases GABA release in the dorsal motor nucleus of the vagus via retrograde messenger-mediated enhancement of TRPV1 activity. PLoS One 8:70505. doi: 10.1371/journal.pone.0070505
Drapala, A., Koszelewski, D., Tomasova, L., Ostaszewski, R., Grman, M., Ondrias, K., et al. (2017). Parenteral NaHS, a fast-releasing H2S donor, but not GYY4137, a slow-releasing H2S donor, lowers blood pressure in rats. Acta Biochim. Pol. 3, 561–566. doi: 10.18388/abp.2017_1569
Fiorucci, S., Distrutti, E., Cirino, G., and Wallace, J. L. (2006). The emerging roles of hydrogen sulfide in the gastrointestinal tract and liver. Gastroenterology 131, 259–271. doi: 10.4103/2045-9912.260650
Furne, J., Saeed, A., and Levitt, M. D. (2008). Whole tissue hydrogen sulfifide concentrations are orders of magnitude lower than presently accepted values. Am. J. Physiol. Regul. Integr. Comp. Physiol. 295, 1479–1485. doi: 10.1152/ajpregu.90566.2008
Gessi, S., Borea, P. A., Bencivenni, S., Fazzi, D., Varani, K., and Merighi, S. (2016). The activation of mu-opioid receptor potentiates LPS-induced NF-kB promoting an inflammatory phenotype in microglia. FEBS Lett. 590, 2813-2826. doi: 10.1002/1873-3468.12313
Goodwin, L. R., Francom, D., Dieken, F. P., Taylor, J. D., Warenycia, M. W., Reiffenstein, R. J., et al. (1989). Determination of sulfide in brain tissue by gas dialysis/ion chromatography: postmortem studies and two case reports. J. Anal. Toxicol. 13, 105–109. doi: 10.1093/jat/13.2.105
Guo, C., Liang, F., Shah Masood, W., and Yan, X. (2014). Hydrogen sulfide protected gastric epithelial cell from ischemia/reperfusion injury by Keap1 s-sulfhydration, MAPK dependent anti-apoptosis and NF-κB dependent anti-inflammation pathway. Eur. J. Pharmacol. 725, 70–78. doi: 10.1016/j.ejphar.2014.01.009
Gutierrez, H., Hale, V. A., Dolcet, X., and Davies, A. (2005). NFkappaB signalling regulates the growth of neural processes in the developing PNS and CNS. Development 132, 1713–1726. doi: 10.1242/dev.01702
Haenold, R., Weih, F., Herrmann, K. H., Schmidt, K. F., Krempler, K., Engelmann, C., et al. (2014). NF-kappaB controls axonal regeneration and degeneration through cell-specific balance of RelA and p50 in the adult CNS. J. Cell Sci. 127, 3052–3065. doi: 10.1242/jcs.140731
Ishigami, M., Hiraki, K., Umemura, K., Ogasawara, Y., Ishii, K., and Kimura, H. (2009). A source of hydrogen sulfide and a mechanism of its release in the brain. Antioxidants Redox Signal. 11, 205–214. doi: 10.1089/ars.2008.2132
Kauer, J. A., and Gibson, H. E. (2009). Hot flash: TRPV channels in the brain. Trends Neurosci. 4, 215–224. doi: 10.1016/j.tins.2008.12.006
Kimura, H., Shibuya, N., and Kimura, Y. (2012). Hydrogen sulfide is a signaling molecule and a cytoprotectant. Antioxid Redox Signal. 17, 45–57. doi: 10.1089/ars.2011.4345
Koroleva, K., Mustafifina, A., Yakovlev, A., Hermann, A., Giniatullin, R., and Sitdikova, G. (2017). Receptor mechanisms mediating the pro-nociceptive action of hydrogen sulfifide in rat trigeminal neurons and meningeal afferents. Front. Cel. Neurosci. 11:226. doi: 10.3389/fncel.2017.00226
Lu, W., Li, J., Gong, L., Xu, X., Han, T., Ye, Y., et al. (2014). H2S modulates duodenal motility in male rats via activating TRPV1 and KATP channels. Br. J. Pharmacol. 171, 1534–1550. doi: 10.1111/bph.12562
Ma, Y., Shi, Q., Wang, J., Xiao, K., Sun, J., Lv, Y., et al. (2017). Reduction of NF-κB (p65) in scrapie-infected cultured cells and in the brains of scrapie-infected rodents. ACS Chemical Neuroscience 11, 2535–2548. doi: 10.1021/acschemneuro.7b00273
Meffert, M. K., and Baltimore, D. (2005). Physiological functions for brain NF-kappaB. Trends Neurosci. 28, 37–43. doi: 10.1016/j.tins.2004.11.002
Numata, T., Ito, T., Maeda, T., Egusa, C., and Tsuboi, R. (2016). IL-33 promotes ICAM-1 expression via NF-kB in murine mast cells. Allergol. Int. 65, 158–165. doi: 10.1016/j.alit.2015.10.004
Patacchini, R., Santicioli, P., Giuliani, S., and Maggi, C. A. (2004). Hydrogen sulfide (H2S) stimulates capsaicin-sensitive primary afferent neurons in the rat urinary bladder. Br. J. Pharmacol. 142, 31–34. doi: 10.1038/sj.bjp.0705764
Paxinos, G., and Watson, C. (2007). The Rat Brain in Stereotaxic Coordinates, 5th Edn. Elsevier: Academic Press.
Qiao, W., Yang, L., Li, X.-Y., Cao, N., Wang, W.-Z., Chai, C., et al. (2011). The cardiovascular inhibition functions of hydrogen sulfide within the nucleus tractus solitarii are mediated by the activation of KATP channels and glutamate receptors mechanisms. Pharmazie 66, 287–292.
Ren, H. Y., Huang, G. L., Liu, W. M., Zhang, W., Liu, Y., Su, G. Q., et al. (2016). IL-1beta induced RXRalpha overexpression through activation of NF-kappaB signaling in gastric carcinoma. Biomed. Pharmacother. 78, 329–334. doi: 10.1016/j.biopha.2016.01.033
Schlicher, L., Wissler, M., Preiss, F., Brauns-Schubert, P., Jakob, C., Dumit, V., et al. (2016). SPATA2 promotes CYLD activity and regulates TNF-induced NF-kappaB signaling and cell death. EMBO Rep. 17, 1485–1497. doi: 10.15252/embr.201642592
Storti, B., Di Rienzo, C., Cardarelli, F., Bizzarri, R., and Beltram, F. (2015). Unveiling TRPV1 spatio-temporal organization in live cell membranes. PLoS One 10:e0116900. doi: 10.1371/journal.pone.0116900
Sun, H.-Z., Gong, X.-Y., Wu, L., Wang, X.-X., Nie, Y.-N., Shang, R., et al. (2018). Hydrogen sulfide modulates gastric acid secretion in rats Via involvement of substance p and nuclear factor-kb signaling. J. Physiol. Pharmacol. 3, 419–422. doi: 10.26402/jpp.2018.3.08
Sun, H.-Z., Yu, K.-H., and Ai, H.-B. (2015). Role of hydrogen sulfide within the dorsal motor nucleus of the vagus in the control of gastric function in rats. Neurogastroenterol. Motil. 27, 618–626. doi: 10.1111/nmo.12530
Sun, H.-Z., Zhao, S.-Z., Cui, X.-Y., and Ai, H.-B. (2009). Hindbrain effects of L-Glutamate on gastric motility in rats. Gastroenterol. Res. 2, 43–49. doi: 10.4021/gr2009.02.1274
Sun, H.-Z., Zhao, S.-Z., Cui, X.-Y., and Ai, H.-B. (2010). Effects and mechanisms of L-Glutamate microinjected into nucleus ambiguus on gastric motility in rats. Chin. Med. J. 123, 1052–1057. doi: 10.3760/cma.j.issn.0366-6999.2010.08.014
Sun, H.-Z., Zheng, S., Lu, K., Hou, F.-T., Bi, J.-X., Liu, X.-L., et al. (2017). Hydrogen sulfide attenuates gastric mucosal injury induced by restraint water-immersion stress via activation of K(ATP) channel and NF-κB dependent pathway. World J. Gastroenterol. 23, 87–92. doi: 10.3748/wjg.v23.i1.87
Swaroop, M., Bradley, K., Ohura, T., Tahara, T., Roper, M. D., Rosenberg, L. E., et al. (1992). Rat cystathionine beta-synthase Gene organization and alternative splicing. J. Biol. Chem. 267, 11455–11461.
Trevisani, M., Patacchini, R., Nicoletti, P., Gatti, R., Gazzieri, D., Lissi, N., et al. (2005). Hydrogen sulfide causes vanilloid receptor 1-mediated neurogenic inflammation in the airways. Br. J. Pharmacol. 145, 1123–1131. doi: 10.1038/sj.bjp.0706277
Wang, N., Zhou, Z., Wu, T., Liu, W., Yin, P., Pan, C., et al. (2016). TNF-alpha-induced NF-kappaB activation upregulates microRNA-150-3p and inhibits osteogenesis of mesenchymal stem cells by targeting beta-catenin. Open Biol. 6:150258. doi: 10.1098/rsob.150258
Wang, R. (2012). Physiological implications of hydrogen sulfide: a whiff exploration that blossomed. Physiol. Rev. 92, 791–896. doi: 10.1152/physrev.00017.2011
Wang, Y. H., Ai, H. B., Zhang, Y. Y., and Cui, X. Y. (2007). Effects and mediated pathway of electrical stimulation of nucleus ambiguus on gastric motility and gastric barrier mucus secretion in rats. Scand. J. Clin. Lab. Invest. 67, 489–497. doi: 10.1080/00365510601161505
Warenycia, M. W., Goodwin, L. R., Benishin, C. G., Reiffenstein, R. J., Francom, D. M., Taylor, J. D., et al. (1989). Acute hydrogen sulfide poisoning. Demonstration of selective uptake of sulfide by the brainstem by measurement of brain sulfide levels. Biochem. Pharmacol. 38, 973–981. doi: 10.1016/0006-2952(89)90288-8
Wei, X., Zhang, B., Zhang, Y., Li, H., Cheng, L., Zhao, X., et al. (2015). Hydrogen sulfide inhalation improves neurological outcome via NF-κB-mediated inflammatory pathway in a rat model of cardiac arrest and resuscitation. Cell Physiol. Biochem. 36, 1527–1538. doi: 10.1159/000430316
Yang, C. T., Lai, Z. Z., Zheng, Z. H., Kang, J. M., Xian, M., Wang, R. Y., et al. (2017). A novel pH-controlled hydrogen sulfide donor protects gastric mucosa from aspirin-inducedinjury. J. Cell Mol. Med. 10, 2441–2451. doi: 10.1111/jcmm.13166
Yang, Q., and He, G. (2019). Imbalance of homocysteine and H2S: significance, mechanisms, and therapeutic promise in vascular injury. Oxid. Med. Cell. Longev. 2019, 1–11. doi: 10.1155/2019/7629673.7629673
Yu, Q., Wang, B., Zhao, T., Zhang, X., Tao, L., Shi, J., et al. (2017). NaHS protects against the impairments induced by oxygen-glucose deprivation in different ages of primary hippocampal neurons. Front. Cell. Neurosci. 11:67. doi: 10.3389/fncel.2017.00067
Zhang, X. Y., Ai, H. B., and Cui, X. Y. (2006). Effects of nucleus ambiguus and dorsal motor nuclei of vagus on gastric H(+) and HCO(3)(-) secretion in rats. World J. Gastroenterol. 12, 3271–3274. doi: 10.3748/wjg.v12.i20.3271
Zhang, Y. Y., Cao, G. H., Zhu, W. X., Cui, X. Y., and Ai, H. B. (2009). Comparative study of c-Fos expression in rat dorsal vagal complex and nucleus ambiguus induced by different durations of restraint water-immersion stress. Chin. J. Physiol. 52, 143–150. doi: 10.4077/CJP.2009
Zheng, F., Han, J., Lu, H., Cui, C., Yang, J., Cui, Q., et al. (2018). Cystathionine beta synthase-hydrogen sulfide system in paraventricular nucleus reduced high fatty diet induced obesity and insulin resistance by brain-adipose axis. Biochim. Biophys. Acta Mol. Basis Dis. 10, 3281–3291. doi: 10.1016/j.bbadis.2018.07.014
Zhou, J., Lv, X. H., Fan, J. J., Dang, L. Y., Dong, K., Gao, B., et al. (2018). GYY4137 promotes mice feeding behavior via arcuate nucleus sulfur-sulfhydrylation and AMPK activation. Front. Pharmacol. 9:966. doi: 10.3389/fphar.2018.00966
Keywords: nucleus ambiguus, hydrogen sulfide, gastric motility, TRPV1, NF-κB
Citation: Sun H, Ding H, Shi Y, Li C, Jin H, Yang X, Chen Z, Tian P, Zhu J and Sun H (2020) Exogenous Hydrogen Sulfide Within the Nucleus Ambiguus Inhibits Gastrointestinal Motility in Rats. Front. Physiol. 11:545184. doi: 10.3389/fphys.2020.545184
Received: 24 March 2020; Accepted: 21 August 2020;
Published: 11 September 2020.
Edited by:
Stephen J. Pandol, Cedars-Sinai Medical Center, United StatesReviewed by:
Hongmei Xue, Hebei Medical University, ChinaCopyright © 2020 Sun, Ding, Shi, Li, Jin, Yang, Chen, Tian, Zhu and Sun. This is an open-access article distributed under the terms of the Creative Commons Attribution License (CC BY). The use, distribution or reproduction in other forums is permitted, provided the original author(s) and the copyright owner(s) are credited and that the original publication in this journal is cited, in accordance with accepted academic practice. No use, distribution or reproduction is permitted which does not comply with these terms.
*Correspondence: Hongzhao Sun, c3VuaG9uZ3poYW8xOEAxMjYuY29t; Haiji Sun, c3VuaGo1MDE4QDEyNi5jb20=
†These authors have contributed equally to this work
Disclaimer: All claims expressed in this article are solely those of the authors and do not necessarily represent those of their affiliated organizations, or those of the publisher, the editors and the reviewers. Any product that may be evaluated in this article or claim that may be made by its manufacturer is not guaranteed or endorsed by the publisher.
Research integrity at Frontiers
Learn more about the work of our research integrity team to safeguard the quality of each article we publish.