- 1Faculty of Electrical Engineering and Information Technology, Institute of Electrodynamics, Microwave and Circuit Engineering, Vienna University of Technology, Vienna, Austria
- 2SzeleSTIM GmbH, Vienna, Austria
- 3General Hospital of the City of Vienna, Vienna, Austria
- 4Division of Vascular Surgery, Department of Surgery, Medical University of Vienna, Vienna, Austria
- 5Faculty of Biology and Faculty of Optics, Complutense University of Madrid, Madrid, Spain
- 6Department of Clinical Epidemiology and Biostatistics, Pontificia Universidad Javeriana, Bogotá, Colombia
- 7The Pediatric Department, Women and Children's Hospital of Hunan, Changsha, China
- 8San Carlos Clinical Hospital, Madrid, Spain
- 9Institute for Health Research, San Carlos Clinical Hospital (IdISSC), Madrid, Spain
- 10Faculty of Medicine, Complutense University of Madrid, Madrid, Spain
- 11Department of Mental and Physical Health and Preventive Medicine, University of Campania “Luigi Vanvitelli”, Naples, Italy
Background: Covid-19 is an infectious disease caused by an invasion of the alveolar epithelial cells by coronavirus 19. The most severe outcome of the disease is the Acute Respiratory Distress Syndrome (ARDS) combined with hypoxemia and cardiovascular damage. ARDS and co-morbidities are associated with inflammatory cytokine storms, sympathetic hyperactivity, and respiratory dysfunction.
Hypothesis: In the present paper, we present and justify a novel potential treatment for Covid19-originated ARDS and associated co-morbidities, based on the non-invasive stimulation of the auricular branch of the vagus nerve.
Methods: Auricular vagus nerve stimulation activates the parasympathetic system including anti-inflammatory pathways (the cholinergic anti-inflammatory pathway and the hypothalamic pituitary adrenal axis) while regulating the abnormal sympatho-vagal balance and improving respiratory control.
Results: Along the paper (1) we expose the role of the parasympathetic system and the vagus nerve in the control of inflammatory processes (2) we formulate our physiological and methodological hypotheses (3) we provide a large body of clinical and preclinical data that support the favorable effects of auricular vagus nerve stimulation in inflammation, sympatho-vagal balance as well as in respiratory and cardiac ailments, and (4) we list the (few) possible collateral effects of the treatment. Finally, we discuss auricular vagus nerve stimulation protective potential, especially in the elderly and co-morbid population with already reduced parasympathetic response.
Conclusions: Auricular vagus nerve stimulation is a safe clinical procedure and it could be either an effective treatment for ARDS originated by Covid-19 and similar viruses or a supplementary treatment to actual ARDS therapeutic approaches.
Introduction
Covid-19 and Acute Respiratory Distress Syndrome (ARDS)
Covid-19 is an infectious disease caused by SARS-CoV-2 which invades alveolar epithelial cells via angiotensin 2-converting enzyme (ACE2) receptors (Hoffmann et al., 2020; Zheng et al., 2020). Infection is triggered by binding of SARS-CoV-1 or SARS-CoV-2 spike (S) protein to ACE2, which is abundantly expressed in alveolar epithelial cells, thus giving rise to respiratory distress (Vellingiri et al., 2020; Zheng et al., 2020).
Covid-19 may lead—especially in elderly people and/or when comorbidities are present- to the Acute Respiratory Distress Syndrome (ARDS) or fulminant pneumonia. Covid-19 may also lead to severe hypoxemia and increased thrombotic and/or thromboembolic events. As a consequence, acute myocardial injury and even chronic damage to the cardiovascular system may occur since ACE2 receptors are also present in the heart making it susceptible to SARS-CoV-2 virus (Guan et al., 2020; Zheng et al., 2020).
“ARDS is a clinically and biologically heterogeneous disorder associated with many disease processes that injure the lung, culminating in increased non-hydrostatic extravascular lung water, reduced compliance, and severe hypoxemia” (Nanchal and Truwit, 2018). In ARDS, tiny lung blood vessels become leaky, which cause fluid to fill up alveoli and thus to prevent the lungs from effectively providing O2 to the rest of the body and clearing CO2 (Stevens et al., 2018). ARDS is characterized by widespread inflammation in the lungs, inflammatory cytokine storms, and an imbalance of the sympathetic-parasympathetic activity of the autonomic nervous system (Guo et al., 2020; Zhou et al., 2020).
The Vagus Nerve and the Immune System
The vagus nerve, the major nerve of the parasympathetic nervous system, mediates and modulates the immune response to inflammatory processes in the body (Pavlov and Tracey, 2012). It is composed by both, sensory (≈80%) and motor fibers (Berthoud and Neuhuber, 2000).
Inflammatory mediators (e.g., pro-inflammatory cytokines and/or endotoxins) activate the vagal afferent fibers; the associated afferent fibers convey inflammatory information to the nucleus of the solitary tract (NST) and generate postsynaptic excitatory potentials in NST neurons (Figure 1) (Bonaz et al., 2017). NST somatotopic organization allows the detection and precise localization of any inflammatory process (Pavlov and Tracey, 2012). Once an inflammatory process has been detected, NST neurons activate the dorsal motor nucleus of the vagus nerve (DMNV) whose efferent fibers trigger two different mechanisms of immune reply: the cholinergic anti-inflammatory pathway (ChAP) and the hypothalamic pituitary adrenal axis (HPAA) (Bonaz et al., 2017).
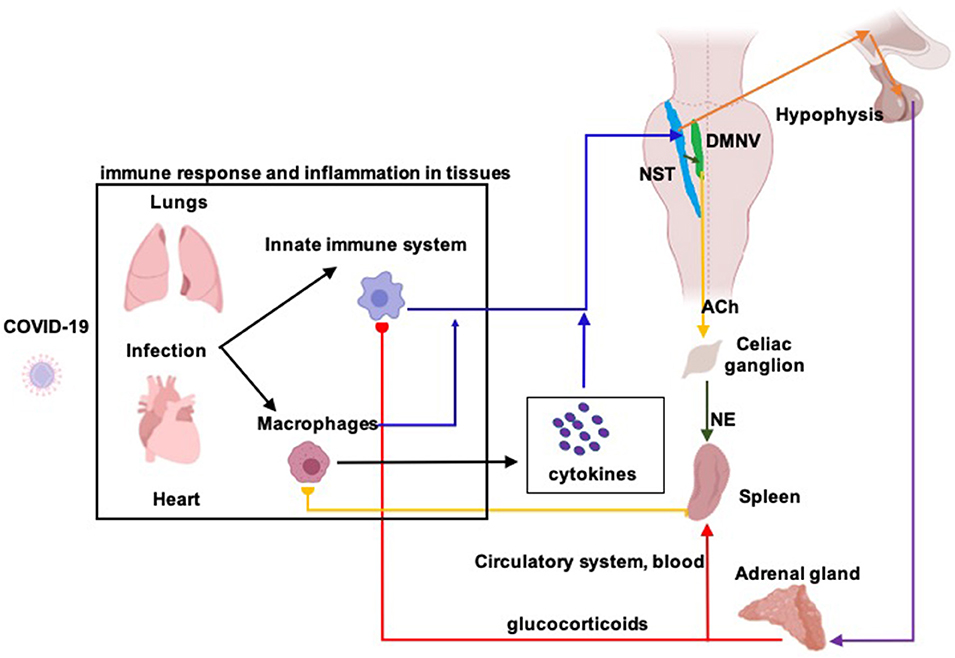
Figure 1. Diagram of the vagus nerve mediated anti-inflammatory responses. The vagus nerve plays a key role in the neuro-endocrine-immune axis, having a dual anti-inflammatory role through its afferent and efferent fibers. In an infection, such as that caused by Covid-19, a primary immune response leads to a release of pro-inflammatory cytokines, generating an inflammatory process at the site of infection, in this case, in the lungs and heart. Released cytokines are recognized by afferent fibers of the vagus nerve (blue arrows; information about inflammation from lung, heart and blood) who transmit such information to the nucleus of the solitary tract (NST). Activation of NST neurons give origin of the anti-inflammatory response which is generated through two different pathways. The first, known as “hypothalamic-pituitary-adrenal axis,” NST efferents to the hypothalamus (orange arrows) stimulate the release of corticotrophin-releasing hormone (CRH) which stimulates the secretion of adrenocorticotropic hormone (ACTH) from the pituitary gland. ACTH reaches the adrenal glands (purple arrow) where stimulates the production of glucocorticoids (cortisol in humans). Glucocorticoids act on the spleen (red arrow), which leads to reduced cytokine release by acting on cells of the immune system. The second, known as the “cholinergic anti-inflammatory reflex,” NST efferents to DMNV, the dorsal motor nucleus of the vagus nerve (black arrow, green nucleus), stimulate the cholinergic motoneurons that project to the splenic nerve in the celiac ganglion (yellow arrow). Acetylcholine (ACh), released from the preganglionic terminals, excites celiac neurons and provoke the release of norepinephrine in the spleen (NE, green arrow). Then, splenic response inhibits macrophages' cytokines release, decreasing inflammation.
ChAP, anti-inflammatory response is triggered by DMNV neurons that project by the adrenergic splenic nerve to the spleen where T-lymphocytes expressing acetylcholine-transferase get activated (Figure 2). When T-cells travel through the body and identify macrophages, they secrete acetylcholine. Acetylcholine binds to acetylcholine surface receptors of macrophages (and/or other cytokine-producing cells), triggering specific intracellular signal transduction to inhibit the release of inflammatory mediators by macrophages (Wang et al., 2003) and thus leading to suppression of pro-inflammatory cytokines (Inoue et al., 2016; Wang et al., 2016). Additionally, infection-activated NST-DMNV neurons provoke an acetylcholine release at their efferents endings, with the aforementioned anti-inflammatory effects (Borovikova et al., 2000). In HPAA, the vagus nerve input to the NST modulates the membrane potential of group A2 noradrenergic neurons which project to the paraventricular hypothalamic area (Figure 1). Parvocellular paraventricular neurons release the corticotropin-stimulating factor which binds to specific receptors expressed by pituitary gland cells. These cells release adreno-corticotropin, a hormone that modulates the cells in the zona fasciculata in the adrenal glands, who release (strongly anti-inflammatory) glucocorticoids in the body (Bonaz et al., 2013).
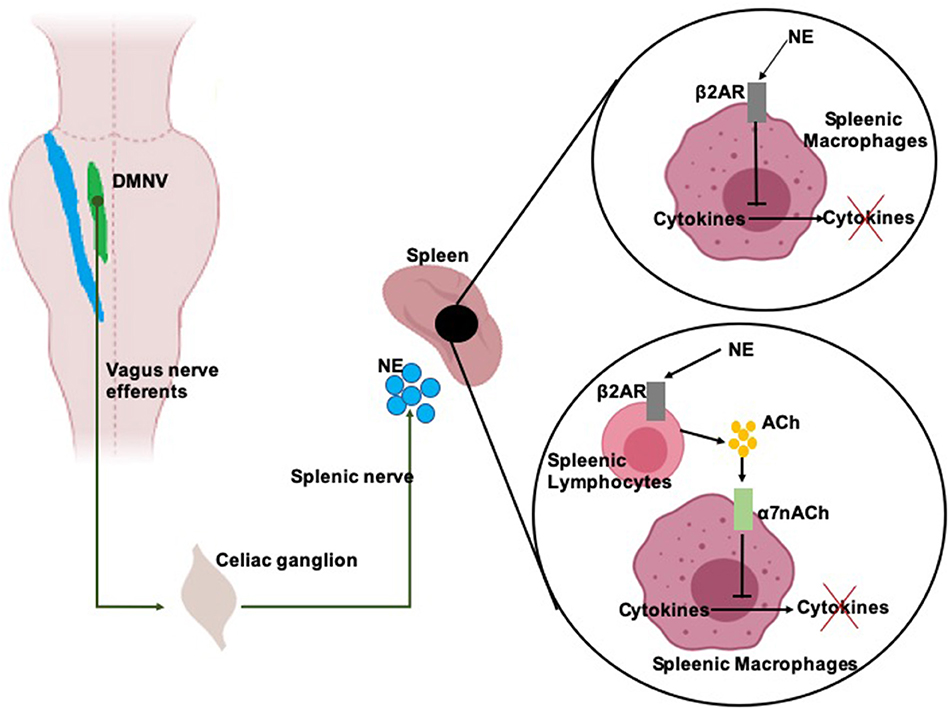
Figure 2. Scheme of the anti-inflammatory activity of the vagal efferents. Vagal efferents arise from the dorsal motor nucleus of the vagus nerve (DMVN) and project to the celiac ganglion, where they synapse with the splenic nerve. DMNV efferents activity provokes stimulates the splenic nerve which release of norepinephrine (NE) over the spleen. NE binds on β2 adrenergic receptors (β2AR) expressed on splenic macrophages and splenic lymphocytes. NE binding on macrophages inhibits the release of pro-inflammatory cytokines of these cells. NE binding on lymphocytes provoke the release of acetylcholine (Ach) which is recognized by α7 acetylcholine receptors (α7nACh) on the membrane of the macrophages. α7nACh activation provokes a disruption of the cytokine release pathway.
Precisely, ARDS is characterized by wide inflammatory processes and very high concentrations of cytokines in the lung, just the natural targets of both anti-inflammatory pathways. Interestingly, all ARDS risk factors, e.g., pneumonia, sepsis, gastric content aspiration, trauma, pancreatitis, inhalation injury, non-cardiogenic shock, drug overdose, etc. worsen ARDS symptoms by increasing cytokines concentration and fostering lung inflammation (Umbrello et al., 2017; Yang et al., 2020).
The Sympatho-Vagal Balance
A homeostasis shift of the autonomic nervous system toward sympathetic predominance (either by severe stress or inflammation) could lead to sympathetic modulation-associated diseases (Stojanovich et al., 2007; Brier et al., 2015). The term “sympatho-vagal balance” denotes the approximate ratio of sympathetic and parasympathetic activity of the autonomic nervous system, frequently given as LF/HF, that means the ratio between low and high frequency components of the power spectrum of the variability of the heart rate, with LF representing both sympathetic and parasympathetic activity and HF representing the parasympathetic activity only (Malik et al., 1996).
In Covid-19, hyperactivity of the sympathetic nervous system may provoke excessive plasma epinephrine and norepinephrine release that lead to pulmonary vasoconstriction and capillary hyperpermeability (see Discussion of Poulat and Couture, 1998; Busl and Bleck, 2015; Liu et al., 2017). At this point an excitatory loop is created in favor to the sympathetic system that provokes an exponential worsening of the symptoms. That is, the acute lung injury provokes a further imbalance in favor to the sympathetic system, with significant plasma IL-6 and IL-10 increases accompanied by considerable hemorrhage, edema, consolidation, atelectasis, neutrophil infiltration, swelling of type I alveolar epithelial and microvascular endothelial cells, mitochondria cavitation, reduction of the mitochondrial crest, vacuolation of the laminar body of type II alveolar epithelial cells, detachment of microvilli, and rarefaction of the basilar membrane (Liu et al., 2017).
Furthermore, the loss of sympatho-vagal equilibrium worsens Covid19-originated inflammation through the renin–angiotensin–aldosterone system, a cascade of vasoactive peptides (Vaduganathan et al., 2020), which has recently been proposed to act as a mediator for ARDS-originated lung injury response (Busse et al., 2020). Indeed, “the activation of the sympathetic nervous system and the renin–angiotensin–aldosterone system appears inextricably, and reciprocally, linked,” at least in the case of hypertension patients (Fisher and Paton, 2012). On the other side, ACE2, the receptor for both, SARS-CoV-1 and SARS-CoV-2, who facilitates the endocytosis of the two viruses, is also an activator enzyme of the renin–angiotensin–aldosterone system (Vaduganathan et al., 2020).
Hypotheses
Physiological Hypothesis
We hypothesize that (1) a sustained increase of the parasympathetic activity of the autonomous nervous system will activate the ChAP and HPAA anti-inflammatory pathways, which will lead to a strong anti-inflammatory response of the immune system and a consequent containment of the associated ARDS and other diseases; (2) the increase of the parasympathetic activity will invert the abnormal sympatho-vagal balance from sympathetic predominance to parasympathetic one, counteracting the course of ARDS and other diseases.
Methodological Hypothesis
We hypothesize that the sustained increase of the parasympathetic activity (required for the inversion of the sympatho-vagal balance) can be achieved by a non-invasive electrical vagus nerve stimulation (VNS), namely, by the stimulation of the auricular branch of the vagus nerve (aVNS).
These hypotheses assume that the severity of Covid19-originated ARDS and other diseases can be reduced by an “endogenous systemic brake” which will be naturally given by the parasympathetic part of the autonomous nervous system.
Methods
Pathways and Processes
Our hypotheses on the therapeutic effects of VNS-induced parasympathetic activity are supported by a wide range of state-of-the-art clinical and experimental data: decrease of pro-inflammatory cytokines and modulation of lung injuries by activation of the anti-inflammatory pathways, improvement of pulmonary and cardiac functions by adjusting the sympatho-vagal imbalance, etc. (Dos Santos et al., 2011; Krzyzaniak et al., 2011; Kessler et al., 2012; Kox et al., 2012; Levy et al., 2012; Reys et al., 2013; Tarras et al., 2013; Shinlapawittayatorn et al., 2014; Akella and Deshpande, 2015; Chen et al., 2016; Stavrakis and Po, 2016; Liu et al., 2017; Huang et al., 2019).
Electrical stimulation of the vagus nerve can be clinically effective in counteracting Covid-19 complications
• by activating the ChAP,
• by activating the HPAA anti-inflammatory pathway,
• by restoring the sympatho-vagal balance while increasing parasympathetic activity and
◦ improving oxygenation
◦ favoring modulation of local cardiovascular effects and
◦ improving respiratory control.
Mechanisms by Which Therapeutic Effects of aVNS Will Be Achieved
Covid-19 viral particles invading the lung mucosa, trigger a series of responses of the immune system that lead to the generation of cytokine storms in the body1. Covid-19 patients present increased levels of IL-1, IL-2, IL-4, IL-7, IL-10, IL-12, IL-13, IL-17, GCSF, MCSF, IP-10, MCP-1, MIP-1α, HGF, IFN-γ, and TNF-α (Guo et al., 2020). Vagal afferents in the lungs detect inflammatory tissue-damaging stimuli (Carr and Undem, 2003), while VNS reduces the storm of pro-inflammatory cytokines through ChAP and HPAA activation, without leading to immunosuppression (Dos Santos et al., 2011; Krzyzaniak et al., 2011; Akella and Deshpande, 2015; Huang et al., 2019). Consequently, we expect VNS to reduce the severity of Covid-19 inflammation-related complications and fatalities while preventing strong and deteriorating immune responses.
VNS regularizes the sympatho-vagal imbalance and increases parasympathetic activity (Pavlov and Tracey, 2012; Kampusch et al., 2015; Deuchars et al., 2018). We expect it to be especially effective in elderly Covid-19 patients with age-related decline in their natural parasympathetic responses to endogenous and exogenous disturbances and consequent severe complications leading to fatalities. A regularization of the sympatho-vagal balance will decrease the sympathetic activity which in turn will provoke vasodilation and, consequently, improve oxygenation. Additionally, VNS-based release of vasodilating NO (Sator-Katzenschlager et al., 2004; Olshansky et al., 2008; Brack et al., 2009)-combined with VNS anti-inflammatory effects (Chapleau et al., 2016)-mediates cardiovascular responses, thus potentially leading to further improvement of tissues oxygenation (Kaniusas et al., 2015).
Vagus nerve is the major sensory channel from lung/airways to the brain; it controls pulmonary function and it regulates respiration, including normal breathing and respiratory defense mechanisms and provide sensory feedback from the lungs to the brain (Chang et al., 2015). Both, sensory feedback and respiration processes may be significantly impaired by inflammatory conditions: “It is clear that the function of afferent fibers can be substantively influenced by airway inflammation and remodeling. The perturbations and perversions of afferent nerve function that occur during these states almost certainly contributes to many of the signs and symptoms of inflammatory airway disease” (Carr and Undem, 2003). In this light, we expect that VNS-provided respiratory feedback will favor lung inflammation control. Furthermore, since the sympathetic-vagal imbalance worsens ARDS symptoms, we expect that VNS balancing function will improve treatment of a wider spectrum of respiratory diseases.
Finally, VNS favorably modulates numerous cardiovascular parameters, yielding to a reduction of blood pressure (Annoni et al., 2015; Mahadi et al., 2019), reduction of arrhythmias (Vanoli et al., 1991; Annoni et al., 2015), and suppression of atrial fibrillation (Stavrakis et al., 2015). VNS blunts sympathetic activity in heart failure (Li et al., 2004; Zhang et al., 2009), and reverses cardiac remodeling after myocardial infarction (Buchholz et al., 2015; Wang et al., 2015), also reviewed in Kaniusas et al. (2019a,b). Thus, VNS could favorably modulate cardiovascular complications in Covid-19 patients, especially in comorbid ones, and reduce the percentage of fatal outcomes.
Results
Neurophysiological and Therapeutic Effects of VNS and aVNS
VNS and aVNS have comparable physiological effects (Kaniusas et al., 2019a,b). aVNS-induced cerebral activity patterns alike the activity patterns induced by cervical VNS (Beekwilder and Beems, 2010) and alike favorable healing results (Mercante et al., 2018). aVNS generates far-field brainstem potentials (Berthoud and Neuhuber, 2000; Fallgatter et al., 2003; Polak et al., 2009) were similar to cervical VNS ones (Babygirija et al., 2017; Nonis et al., 2017), while both VNS and aVNS increase brain norepinephrine levels (Lockard et al., 1990; Oleson, 2002; Lehtimäki et al., 2013; Han et al., 2015).
VNS Therapeutic Effects in ARDS, Lung Injury, and Sepsis
Laboratory research demonstrated VNS lung protective effects (Krzyzaniak et al., 2011). VNS protected against Mesobuthus tamulus venom-induced ARDS in rats, improving parameters like respiratory alterations, hypoxemia, pulmonary edema and histopathological changes (Akella and Deshpande, 2015). It attenuated acute lung injury following burn via the anti-inflammatory pathways (Krzyzaniak et al., 2011) and improved the pulmonary function of acute lung injury while counterbalancing the predominance of the sympathetic nervous system (Liu et al., 2017). VNS was effective to limit pulmonary dendritic cells recruitment within the lung and prevented acute lung injury after burn injury (Lowry et al., 2014).
VNS is a serious candidate for ventilator-induced lung injury, frequently being the consequence of serious lung infections (Johnson and Wilson, 2018). Both, ARDS and acute lung injury, either alone or accompanied by sepsis, can also result in pronounced pulmonary inflammation. Vagus nerve is critical in pulmonary inflammation, whereas vagotomy-induced ChAP interrupt vagotomy leads to a deterioration of the ventilator-induced lung injuries (Dos Santos et al., 2011). Authors also report increased injuries to the alveoli of mechanically-ventilated vagotomized animals as well as hemorrhage and increased levels of IL-6. VNS attenuated the ventilator-induced lung injury by diminishing pro-apoptotic and pro-inflammatory reactions. In lung injury caused by hemorrhagic shock, VNS has been shown to prevent failures of the intestinal barrier and lung injuries (Reys et al., 2013). Interestingly, in vitro studies of pulmonary endothelial cells injuries provided evidence that VNS prevents lung injury through activation of the ChAP (Tarras et al., 2013). Furthermore, hemorrhagic shock-caused lung injury can be relieved by VNS which provokes a fall of gut permeability (Levy et al., 2012). VNS showed clear therapeutic effects for hemorrhagic shock mainly due to its anti-inflammatory properties while recovering lung permeability and mitigating lung injury (Powell et al., 2019). Thirty minutes-long VNS trains diminish TNFα and IL-6 the expression in the respiratory brainstem nuclei of developing rats, who control breathing activity of the animal (Johnson et al., 2016). Johnson and Wilson summarize the importance of vagus nerve activity for normal lung function, and the possibility of using VNS to improve outcome in lung injury is very promising (Johnson and Wilson, 2018). Only Kox et al. question the applicability of VNS in systemic and pulmonary inflammation after a two-hit rat model with mechanical ventilation (Kox et al., 2012).
Kessler and co-authors showed in a mice model that interrupt of vagus nerve sensory afferents can deprove the physiological conditions in polymicrobial sepsis (Kessler et al., 2012), while Huang and co-authors showed that VNS mitigates inflammation by re-equilibrating the sympathetic-parasympathetic balance and by reducing the sympathetic activity, with a consequent and thus slowing down the progression of the sepsis (Huang et al., 2010). Patients with vagotomy had no more sepsis or ARDS but more septicemia (Peterson et al., 2012). VNS anti-inflammatory effects counteract lethal endotoxemia or polymicrobial sepsis in mice while reducing systemic TNF (Huston et al., 2007). VNS prevented postoperative ileus and endotoxemia in mice (Hong et al., 2019).
Therapeutic Effects of aVNS
aVNS effects are systemic: the auricular branch of the vagus nerve projects directly to the NST and through that it excites DMNV neurons that modulate the parasympathetic division of the autonomic nervous system (Figure 1), an in particular the cardiovascular, respiratory and immunological processes. aVNS therapeutic effects (Kaniusas et al., 2019a,b) in inflammation, respiratory dysfunction and cardiovascular diseases include reduction of pro-inflammatory cytokines (Tracey, 2007; Stavrakis et al., 2015), decrease of inflammation level in chronic inflammatory processes like rheumatoid arthritis, postoperative ileus, inflammatory bowel disease (Crohn's disease, ulcerative colitis), systemic inflammation and attenuation of the acute postsurgical inflammatory response after lung lobectomy (Barone et al., 2007; Bernateck et al., 2008; Chakravarthy et al., 2015; Kox et al., 2015; Marshall et al., 2015; Bonaz et al., 2016; Koopman et al., 2016; Kwan et al., 2016; French et al., 2017; Hoover, 2017; Salama et al., 2017). aVNS resulted in an increase of the middle cerebral and supratrochlear artery blood flow velocity in human subjects (Széles and Litscher, 2004) and a decrease of the velocity of the carotid-femoral flow (Hackl et al., 2017). aVNS diminishes systolic blood pressure in subjects with coronary artery diseases as well as in subjects with dysfunctions of glucose metabolism (Zamotrinsky et al., 2001; Huang et al., 2014; Stavrakis et al., 2015) it suppresses atrial fibrillation in patients with paroxysmal atrial fibrillation (Afanasiev et al., 2016) and reliefs anginal symptoms in coronary artery disease patients (Zamotrinsky et al., 1997). aVNS improves cardiac baroreflex sensitivity (Antonino et al., 2017), increases capillary-venous oxygenation in deep tissues in diabetic patients (Kaniusas et al., 2015), increases skin temperature in human subjects with disfunctions of the peripheral arteries and chronic diabetic wounds (Szeles et al., 2013) and improves symptoms in peripheral arterial occlusive diseases (Payrits et al., 2011).
Systemic effects of aVNS are additionally supported by improvement of metabolic processes (Richards and Marley, 1998; Cabýoglu et al., 2006; Huang et al., 2014; Ju et al., 2014; Schukro et al., 2014), attenuation of neurological disorders (Kraus et al., 2007; Hein et al., 2013; Lehtimäki et al., 2013; Kampusch et al., 2015; Bauer et al., 2016; Fang et al., 2016; Rong et al., 2016; Capone et al., 2017; Ylikoski et al., 2017) and enhancement of cognitive performances (Rong et al., 2012; Jacobs et al., 2015) as well as by pain relief effects (Sator-Katzenschlager et al., 2003, 2004; Napadow et al., 2012; Chakravarthy et al., 2015; Kox et al., 2015; Kwan et al., 2016; French et al., 2017; Hoover, 2017; Kovacic et al., 2017; Kaniusas et al., 2019a,b).
Possible Collateral Effects and Contraindications of aVNS
aVNS can be performed either transcutaneously, in a non-invasive way using surface skin electrodes on the outer ear (Ellrich, 2011; Straube et al., 2015), or percutaneously, in a minimally-invasive way using needle electrodes (Kampusch et al., 2013).
Transcutaneous aVNS employs large surface electrodes which generate diffuse electrical fields that stimulate both, vagal and non-vagal nerve terminals in the ear. Stimulation is safe with minor side effects like headache, dizziness, skin irritation or pain (Badran et al., 2018; Mertens et al., 2018). Stimulation is usually intermittently activated (e.g., NEMOS, Cerbomed GmbH with 3–4 sessions per day, each session lasting at least 1 h) with a total stimulation duration of about 4–5 h per day.
Percutaneous aVNS employs small needle electrodes by which one can achieve a spatially focused electric field that favors precise and specific stimulation of local nerve endings. Electrode contact impedances are lower, allowing an energy-efficient stimulation, with minor side effects (Kaniusas et al., 2019a,b). Skin irritation incidence (<10%) and inadvertent bleeding (<1%) can be reduced to <0.05% if transillumination of the outer ear is employed to visualize auricular vascularization and to prevent erroneous needles placement (Kampusch et al., 2016; Roberts et al., 2016) (Szeles, unpublished clinical data). Also this stimulation is intermittently activated (e.g., AuriStim, SzeleSTIM GmbH with 3 h on and 3 h off). However, it is active during day and night with a much longer effective stimulation duration of 12 h per 24 h than in the case of the transcutaneous aVNS. It is important to underlay that despite such electrodes must be maintained in their place for several days, >80% of the patients expressed very high satisfaction with the cure in terms of quality of life and they reported minor side effects or even total absence of them (Kampusch et al., 2016). The suggested stimulation is 3 h on and 3 h off, with periodic bursts at 1 Hz, and bursts composed out of triphasic patterns with pulse width of 500 μs.
Very light aVNS adverse effects have been reported: Arnold's ear-cough reflex, ear-gag, vaso-vagal reflex, ear-lacrimation, and auriculo-cardiac reflex, all of them being indirect effects of afferent-efferent vagal reflexes, have been observed in only a few percent of the cases (Tekdemir et al., 1998; Ellrich, 2011; Napadow et al., 2012) while headache, syncope, vertigo, alterations of the heart rate and paresthesia have a <1% incidence (Szeles, unpublished clinical data).
aVNS is contraindicated for persons with vagal hypersensitivity, hemophilia, psoriasis vulgaris at application site. aVNS is also contraindicated for patients with active implantable devices, like pacemakers, for their possible interference with the stimulation device.
To our knowledge, there are no reports on special adverse events and contraindications of aVNS in viral infections such as Covid-19.
Discussion
Based on biophysical principles, we introduce a hypothesis that the severity of Covid-19 complications, especially ARDS, can be reduced by triggering an “endogenous systemic brake” of the inflammatory response. This brake is naturally given by the parasympathetic nervous system and can be accelerated by the electrical stimulation of the vagus nerve.
A large amount of experimental data provides evidence that aVNS activates the parasympathetic anti-inflammatory pathways, equilibrates the sympatho-vagal balance, and improves respiratory and cardiac (Fudim et al., 2020) ailments, making aVNS an excellent candidate for clinical treatment of Covid19-related ARDS and other co-morbidities, including apparently less related diseases, as for example Kawasaki disease, an acute childhood vasculitis of unknown etiology, but associated with viral respiratory infections [infants with classic Kawasaki disease displayed also Covid19-positive symptoms (Jones et al., 2020)].
Furthermore, it has been postulated that the employment of renin–angiotensin–aldosterone system inhibitors could alter ACE2 expression, which in turn could contribute to the expansion of Covid-19 (Diaz, 2020; Fang et al., 2020). The dysregulation of renin–angiotensin–aldosterone system may have detrimental effects on cardiovascular regulation and parasympathetic tone (Fudim et al., 2020), so that aVNS re-equilibrium of the sympatho-vagal balance could possibly counteract these negative effects. Furthermore, hyperactivation of the vagus nerve downregulated the expression or activity of ACE2 which could even potentially prevent viral infection via the ACE2 entry point (Fudim et al., 2020) (see also Osterziel et al., 1994; Duprez et al., 1995; Cody, 1997; Karas et al., 2005; Zucker et al., 2014; Liu et al., 2015).
Last but not least, auricular VNS is an already available, CE-certified, and FDA-approved neuromodulatory therapy for several diseases, safe in application and with absence, or with only minimal, collateral effects.
Physiological data are needed to validate the proposed aVNS therapeutic procedure, to determine the stimulation parameters and to evaluate to which extent it could be used for the treatment of Covid-19 and other virus-originated ARDS. Furthermore, it will be very important to investigate the potential protective functions of aVNS, especially in the high-risk elderly and co-morbid population (Yang et al., 2020). If aVNS is effective, it will help to reduce lung inflammation and, consequently, to reduce the need for hospitalization and for mechanical ventilation, which are the major determinants of healthcare collapse and if fatal outcomes in Covid-19 infection.
Finally, we should investigate if also early aVNS -before ARDS' outbreak- is effective, having a potentially protective function, especially in the elderly and co-morbid population.
Conclusions
aVNS activates the parasympathetic anti-inflammatory pathways, equilibrates the sympatho-vagal balance, and improves respiratory and cardiac ailments.
aVNS is a safe clinical procedure and it could be an effective treatment for ARDS originated by Covid-19 and similar viruses.
Data Availability Statement
The original contributions presented in the study are included in the article/supplementary material, further inquiries can be directed to the corresponding author/s.
Author Contributions
EK and FP contributed to the conceptualization, methodology, investigation, and the writing of the original draft. JS and CN contributed to the supervision. SK, NA-L, DY-C, and XL contributed to the investigation. JM and MP contributed to the writing, review, and editing. All authors contributed to the article and approved the submitted version.
Funding
EK, JS, and SK have received funding from the European Union's Horizon 2020 Research and Innovation Programme under grant agreement no. 880603.
Conflict of Interest
EK and SK are employed by company SzeleSTIM GmbH. JS received honoraria from SzeleSTIM GmbH and owns patents in the field of the auricular vagus nerve stimulation. EK, SK, and JS are shareholders of SzeleSTIM GmbH.
The remaining authors declare that the research was conducted in the absence of any commercial or financial relationships that could be construed as a potential conflict of interest.
Acknowledgments
The authors would like to acknowledge Dr. Robert Spitz, Medical Director of the ICON Government and Public Health Solutions, USA, for the initiative and support in the application of Vagus Nerve Stimulation in COVID-19 infected patients. The authors would also like to acknowledge the networking support from the COST (European Cooperation in Science and Technology) Action EMF-MED.
Footnote
1. ^The S proteins of the viral particles of COVID-19 bind to the cells of the pulmonary epithelium by ACE2, fusing with the membrane and releasing their RNA into the cell interior. This RNA, as a pathogen-associated molecular standard (PAMP), is detected by pattern recognition receptors. Generally, they are the Toll-like receptors: TLR3, TLR7, TLR8, and TLR9. Then, RNA is recognized in the cytoplasm by viral RNA receptor I (RIG-I) retinoic acid, gene 5 associated with differentiation of cytosolic receptor melanoma (MDA5), and cyclic nucleotidyltransferase synthase GMP-AMP (cGAS). These signaling adapter complexes, which include the TIR domain-containing adapter protein, including IFN-β (TRIF), mitochondrial antiviral signaling protein (MAVS), and the interferon gene protein stimulator (STING), activate a downstream molecular cascade involving the adapter molecule MyD88. This leads to activation of interferon regulatory factor 3 (IRF3) and of nuclear transcription factor κB (NF-κB), as well as to the production of type I interferons (IFN-α/β) and of several types of inflammatory pro-cytokines.
References
Afanasiev, S. A., Pavliukova, E. N., Kuzmichkina, M. A., Rebrova, T. Y., Anfinogenova, Y., Likhomanov, K. S., et al. (2016). Nonpharmacological correction of hypersympatheticotonia in patients with chronic coronary insufficiency and severe left ventricular dysfunction. Ann. Noninvasive Electrocardiol. 21, 548–556. doi: 10.1111/anec.12349
Akella, A., and Deshpande, S. B. (2015). Vagal efferent stimulation protects against Mesobuthus tamulus venom-induced acute respiratory distress syndrome in rats. Toxicon 108, 189–201. doi: 10.1016/j.toxicon.2015.10.013
Annoni, E. M., Xie, X., Lee, S. W., Libbus, I., KenKnight, B. H., Osborn, J. W., et al. (2015). Intermittent electrical stimulation of the right cervical vagus nerve in salt-sensitive hypertensive rats: effects on blood pressure, arrhythmias, and ventricular electrophysiology. Physiol. Rep. 3:e12476. doi: 10.14814/phy2.12476
Antonino, D., Teixeira, A. L., Maia-Lopes, P. M., Souza, M. C., Sabino-Carvalho, J. L., Murray, A. R., et al. (2017). Non-invasive vagus nerve stimulation acutely improves spontaneous cardiac baroreflex sensitivity in healthy young men: a randomized placebo-controlled trial. Brain Stimul. 10, 875–881. doi: 10.1016/j.brs.2017.05.006
Babygirija, R., Sood, M., Kannampalli, P., Sengupta, J. N., and Miranda, A. (2017). Percutaneous electrical nerve field stimulation modulates central pain pathways and attenuates post-inflammatory visceral and somatic hyperalgesia in rats. Neuroscience 356, 11–21. doi: 10.1016/j.neuroscience.2017.05.012
Badran, B. W., Mithoefer, O. J., Summer, C. E., LaBate, N. T., Glusman, C. E., Badran, A. W., et al. (2018). Short trains of transcutaneous auricular vagus nerve stimulation (taVNS) have parameter-specific effects on heart rate. Brain Stimul. 11, 699–708. doi: 10.1016/j.brs.2018.04.004
Barone, L., Colicchio, G., Policicchio, D., Di Clemente, F., Di Monaco, A., Meglio, M., et al. (2007). Effect of vagal nerve stimulation on systemic inflammation and cardiac autonomic function in patients with refractory epilepsy. Neuroimmunomodulation 14, 331–336. doi: 10.1159/000127360
Bauer, S., Baier, H., Baumgartner, C., Bohlmann, K., Fauser, S., Graf, W., et al. (2016). Transcutaneous Vagus Nerve Stimulation (tVNS) for treatment of drug-resistant epilepsy: a randomized, double-blind clinical trial (cMPsE02). Brain Stimul. 9, 356–363. doi: 10.1016/j.brs.2015.11.003
Beekwilder, J. P., and Beems, T. (2010). Overview of the clinical applications of vagus nerve stimulation. J. Clin. Neurophysiol. 27, 130–138. doi: 10.1097/WNP.0b013e3181d64d8a
Bernateck, M., Becker, M., Schwake, C., Hoy, L., Passie, T., Parlesak, A., et al. (2008). Adjuvant auricular electroacupuncture and autogenic training in rheumatoid arthritis: a randomized controlled trial - auricular acupuncture and autogenic training in rheumatoid arthritis. Forsch. Komplementarmed. 15, 187–193. doi: 10.1159/000141929
Berthoud, H. R., and Neuhuber, W. L. (2000). Functional and chemical anatomy of the afferent vagal system. Auton. Neurosci. Basic Clin. 20, 1–17. doi: 10.1016/S1566-0702(00)00215-0
Bonaz, B., Picq, C., Sinniger, V., Mayol, J. F., and Clarençon, D. (2013). Vagus nerve stimulation: from epilepsy to the cholinergic anti-inflammatory pathway. Neurogastroenterol. Motil. 25, 208–221. doi: 10.1111/nmo.12076
Bonaz, B., Sinniger, V., and Pellissier, S. (2016). Anti-inflammatory properties of the vagus nerve: potential therapeutic implications of vagus nerve stimulation. J. Physiol. 594, 5781–5790. doi: 10.1113/JP271539
Bonaz, B., Sinniger, V., and Pellissier, S. (2017). Vagus nerve stimulation: a new promising therapeutic tool in inflammatory bowel disease. J. Intern. Med. 282, 46–63. doi: 10.1111/joim.12611
Borovikova, L. V., Ivanova, S., Zhang, M., Yang, H., Botchkina, G. I., Watkins, L. R., et al. (2000). Vagus nerve stimulation attenuates the systemic inflammatory response to endotoxin. Nature 405, 458–462. doi: 10.1038/35013070
Brack, K. E., Patel, V. H., Mantravardi, R., Coote, J. H., and Ng, G. A. (2009). Direct evidence of nitric oxide release from neuronal nitric oxide synthase activation in the left ventricle as a result of cervical vagus nerve stimulation. J. Physiol. 587, 3045–3054. doi: 10.1113/jphysiol.2009.169417
Brier, T. J., Jain, A. K., and Lobo, M. D. (2015). Central arteriovenous anastomosis for hypertension: it is not all about sympathomodulation. Fut. Cardiol. 11, 503–506. doi: 10.2217/fca.15.34
Buchholz, B., Donato, M., Perez, V., Deutsch, A. C. R., Höcht, C., Del Mauro, J. S., et al. (2015). Changes in the loading conditions induced by vagal stimulation modify the myocardial infarct size through sympathetic-parasympathetic interactions. Pflugers Arch. Eur. J. Physiol. 467, 1509–1522. doi: 10.1007/s00424-014-1591-2
Busl, K. M., and Bleck, T. P. (2015). Neurogenic pulmonary edema. Crit. Care Med. 43, 1710–1715. doi: 10.1097/CCM.0000000000001101
Busse, L. W., Chow, J. H., McCurdy, M. T., and Khanna, A. K. (2020). COVID-19 and the RAAS-a potential role for angiotensin II? Crit. Care 24:136. doi: 10.1186/s13054-020-02862-1
Cabýoglu, M. T., Ergene, N., and Tan, U. (2006). The treatment of obesity by acupuncture. Int. J. Neurosci. 116, 165–175. doi: 10.1080/00207450500341522
Capone, F., Miccinilli, S., Pellegrino, G., Zollo, L., Simonetti, D., Bressi, F., et al. (2017). Transcutaneous vagus nerve stimulation combined with robotic rehabilitation improves upper limb function after stroke. Neural Plast. 2017, 1–6. doi: 10.1155/2017/7876507
Carr, M. J., and Undem, B. J. (2003). Bronchopulmonary afferent nerves. Respirology 8, 291–301. doi: 10.1046/j.1440-1843.2003.00473.x
Chakravarthy, K., Chaudhry, H., Williams, K., and Christo, P. J. (2015). Review of the uses of vagal nerve stimulation in chronic pain management. Curr. Pain Headache Rep. 19:54. doi: 10.1007/s11916-015-0528-6
Chang, R. B., Strochlic, D. E., Williams, E. K., Umans, B. D., and Liberles, S. D. (2015). Vagal sensory neuron subtypes that differentially control breathing. Cell 161, 622–633. doi: 10.1016/j.cell.2015.03.022
Chapleau, M. W., Rotella, D. L., Reho, J. J., Rahmouni, K., and Stauss, H. M. (2016). Chronic vagal nerve stimulation prevents high-salt diet-induced endothelial dysfunction and aortic stiffening in stroke-prone spontaneously hypertensive rats. Am. J. Physiol. Heart Circ. Physiol. 311, H276–H285. doi: 10.1152/ajpheart.00043.2016
Chen, I. C., Kuo, J., Ko, W. J., Shih, H. C., and Kuo, C. D. (2016). Increased flow resistance and decreased flow rate in patients with acute respiratory distress syndrome: the role of autonomic nervous modulation. J. Chin. Med. Assoc. 79, 17–24. doi: 10.1016/j.jcma.2015.10.001
Cody, R. J. (1997). The sympathetic nervous system and the renin-angiotensin-aldosterone system in cardiovascular disease. Am. J. Cardiol. 80, 9–14. doi: 10.1016/S0002-9149(97)00832-1
Deuchars, S. A., Lall, V. K., Clancy, J., Mahadi, M., Murray, A., Peers, L., et al. (2018). Mechanisms underpinning sympathetic nervous activity and its modulation using transcutaneous vagus nerve stimulation. Exp. Physiol. 103, 326–331. doi: 10.1113/EP086433
Diaz, J. H. (2020). Hypothesis: angiotensin-converting enzyme inhibitors and angiotensin receptor blockers may increase the risk of severe COVID-19. J. Travel Med. 27:taaa041. doi: 10.1093/jtm/taaa041
Dos Santos, C. C., Shan, Y., Akram, A., Slutsky, A. S., and Haitsma, J. J. (2011). Neuroimmune regulation of ventilator-induced lung injury. Am. J. Respir. Crit. Care Med. 183, 471–482. doi: 10.1164/rccm.201002-0314OC
Duprez, D., De Buyzere, M., Rietzschel, E., Rimbout, S., Kaufman, J. M., Van Hoecke, M. J., et al. (1995). Renin-angiotensin-aldosterone system, RR-interval and blood pressure variability during postural changes after myocardial infarction. Eur. Heart J. 16, 1050–1056. doi: 10.1093/oxfordjournals.eurheartj.a061046
Ellrich, J. (2011). Transcutaneous vagus nerve stimulation. Eur. Neurol. Rev. 6, 254–256. doi: 10.17925/ENR.2011.06.04.254
Fallgatter, A. J., Neuhauser, B., Herrmann, M. J., Ehlis, A. C., Wagener, A., Scheuerpflug, P., et al. (2003). Far field potentials from the brain stem after transcutaneous vagus nerve stimulation. J. Neural Transm. 110, 1437–1443. doi: 10.1007/s00702-003-0087-6
Fang, J., Rong, P., Hong, Y., Fan, Y., Liu, J., Wang, H., et al. (2016). Transcutaneous vagus nerve stimulation modulates default mode network in major depressive disorder. Biol. Psychiatry 79, 266–273. doi: 10.1016/j.biopsych.2015.03.025
Fang, L., Karakiulakis, G., and Roth, M. (2020). Are patients with hypertension and diabetes mellitus at increased risk for COVID-19 infection? Lancet Respir. Med. 8:e21. doi: 10.1016/S2213-2600(20)30116-8
Fisher, J. P., and Paton, J. F. R. (2012). The sympathetic nervous system and blood pressure in humans: implications for hypertension. J. Hum. Hypertens. 26, 463–475. doi: 10.1038/jhh.2011.66
French, J. A., Koepp, M., Naegelin, Y., Vigevano, F., Auvin, S., Rho, J. M., et al. (2017). Clinical studies and anti-inflammatory mechanisms of treatments. Epilepsia 58, 69–82. doi: 10.1111/epi.13779
Fudim, M., Qadri, Y. J., Ghadimi, K., MacLeod, D. B., Molinger, J., Piccini, J. P., et al. (2020). Implications for neuromodulation therapy to control inflammation and related organ dysfunction in COVID-19. J. Cardiovasc. Transl. Res. doi: 10.1007/s12265-020-10031-6. [Epub ahead of print].
Guan, W., Ni, Z., Hu, Y., Liang, W., Ou, C., He, J., et al. (2020). Clinical characteristics of Coronavirus Disease 2019 in China. N. Engl. J. Med. 382, 1708–1720. doi: 10.1056/NEJMoa2002032
Guo, Y. R., Cao, Q. D., Hong, Z. S., Tan, Y. Y., Chen, S. D., Jin, H. J., et al. (2020). The origin, transmission and clinical therapies on coronavirus disease 2019 (COVID-19) outbreak - an update on the status. Mil. Med. Res. 7:11. doi: 10.1186/s40779-020-00240-0
Hackl, G., Prenner, A., Jud, P., Hafner, F., Rief, P., Seinost, G., et al. (2017). Auricular vagal nerve stimulation in peripheral arterial disease patients. Vasa Eur. J. Vasc. Med. 46, 462–470. doi: 10.1024/0301-1526/a000660
Han, L., Jian-Bin, Z., Chen, X., Qing-Qing, T., Wei-Xing, S., Jing-Zhu, Z., et al. (2015). Effects and mechanisms of auricular vagus nerve stimulation on high-fat-diet-induced obese rats. Nutrition 31, 1416–1422. doi: 10.1016/j.nut.2015.05.007
Hein, E., Nowak, M., Kiess, O., Biermann, T., Bayerlein, K., Kornhuber, J., et al. (2013). Auricular transcutaneous electrical nerve stimulation in depressed patients: a randomized controlled pilot study. J. Neural Transm. 120, 821–827. doi: 10.1007/s00702-012-0908-6
Hoffmann, M., Kleine-Weber, H., Schroeder, S., Krüger, N., Herrler, T., Erichsen, S., et al. (2020). SARS-CoV-2 cell entry depends on ACE2 and TMPRSS2 and is blocked by a clinically proven protease inhibitor. Cell 181, 271–280.e8. doi: 10.1016/j.cell.2020.02.052
Hong, G. S., Zillekens, A., Schneiker, B., Pantelis, D., de Jonge, W. J., Schaefer, N., et al. (2019). Non-invasive transcutaneous auricular vagus nerve stimulation prevents postoperative ileus and endotoxemia in mice. Neurogastroenterol. Motil. 31:e13501. doi: 10.1111/nmo.13501
Hoover, D. B. (2017). Cholinergic modulation of the immune system presents new approaches for treating inflammation. Pharmacol. Ther. 179, 1–16. doi: 10.1016/j.pharmthera.2017.05.002
Huang, F., Dong, J., Kong, J., Wang, H., Meng, H., Spaeth, R. B., et al. (2014). Effect of transcutaneous auricular vagus nerve stimulation on impaired glucose tolerance: a pilot randomized study. BMC Complement. Altern. Med. 16:218. doi: 10.1186/s12906-016-1190-1
Huang, J., Wang, Y., Jiang, D., Zhou, J., and Huang, X. (2010). The sympathetic-vagal balance against endotoxemia. J. Neural Transm. 117, 729–735. doi: 10.1007/s00702-010-0407-6
Huang, Y., Zhao, C., and Su, X. (2019). Neuroimmune regulation of lung infection and inflammation. QJM 112, 483–487. doi: 10.1093/qjmed/hcy154
Huston, J. M., Gallowitsch-Puerta, M., Ochani, M., Ochani, K., Yuan, R., Rosas-Ballina, M., et al. (2007). Transcutaneous vagus nerve stimulation reduces serum high mobility group box 1 levels and improves survival in murine sepsis. Crit. Care Med. 35, 2762–2768. doi: 10.1097/01.CCM.0000288102.15975.BA
Inoue, T., Abe, C., Sung, S. S. J., Moscalu, S., Jankowski, J., Huang, L., et al. (2016). Vagus nerve stimulation mediates protection from kidney ischemia-reperfusion injury through α7nAChR+ splenocytes. J. Clin. Invest. 126, 1939–1952. doi: 10.1172/JCI83658
Jacobs, H. I. L., Riphagen, J. M., Razat, C. M., Wiese, S., and Sack, A. T. (2015). Transcutaneous vagus nerve stimulation boosts associative memory in older individuals. Neurobiol. Aging 36, 1860–1867. doi: 10.1016/j.neurobiolaging.2015.02.023
Johnson, R. L., Murray, S. T., Camacho, D. K., and Wilson, C. G. (2016). Vagal nerve stimulation attenuates IL-6 and TNFα expression in respiratory regions of the developing rat brainstem. Respir. Physiol. Neurobiol. 229, 1–4. doi: 10.1016/j.resp.2016.03.014
Johnson, R. L., and Wilson, C. G. (2018). A review of vagus nerve stimulation as a therapeutic intervention. J. Inflamm. Res. 11, 203–213. doi: 10.2147/JIR.S163248
Jones, V. G., Mills, M., Suarez, D., Hogan, C. A., Yeh, D., Bradley Segal, J., et al. (2020). COVID-19 and Kawasaki disease: novel virus and novel case. Hosp. Pediatr. 10, 537–540. doi: 10.1542/hpeds.2020-0123
Ju, Y., Zhang, H., Chen, M., Chi, X., Lan, W., Zhang, H., et al. (2014). Effects of auricular stimulation in the cavum conchae on glucometabolism in patients with type 2 diabetes mellitus. Complement. Ther. Med. 22, 858–863. doi: 10.1016/j.ctim.2014.09.002
Kampusch, S., Kaniusas, E., and Szeles, J. C. (2013). “New approaches in multi-punctual percutaneous stimulation of the auricular vagus nerve,” in 2013 6th International IEEE/EMBS Conference on Neural Engineering (NER) (San Diego, CA), 263–266.
Kampusch, S., Kaniusas, E., and Széles, J. C. (2015). Modulation of muscle tone and sympathovagal balance in cervical dystonia using percutaneous stimulation of the auricular vagus nerve. Artif. Organs 39, E202–E212. doi: 10.1111/aor.12621
Kampusch, S., Kaniusas, E., Thürk, F., Felten, D., Hofmann, I., and Széles, J. C. (2016). Device development guided by user satisfaction survey on auricular vagus nerve stimulation. Curr. Dir. Biomed. Eng. 2, 593–597. doi: 10.1515/cdbme-2016-0131
Kaniusas, E., Kampusch, S., and Szeles, J. C. (2015). “Depth profiles of the peripheral blood oxygenation in diabetics and healthy subjects in response to auricular electrical stimulation: auricular vagus nerve stimulation as a potential treatment for chronic wounds,” in SAS 2015 - 2015 IEEE Sensors Applications Symposium (Zadar).
Kaniusas, E., Kampusch, S., Tittgemeyer, M., Panetsos, F., Gines, R. F., Papa, M., et al. (2019a). Current directions in the auricular vagus nerve stimulation I – a physiological perspective. Front. Neurosci. 13:854. doi: 10.3389/fnins.2019.00854
Kaniusas, E., Kampusch, S., Tittgemeyer, M., Panetsos, F., Gines, R. F., Papa, M., et al. (2019b). Current directions in the auricular vagus nerve stimulation II - an engineering perspective. Front. Neurosci. 13:772. doi: 10.3389/fnins.2019.00772
Karas, M., Lacourcière, Y., LeBlanc, A. R., Nadeau, R., Dubé, B., Florescu, M., et al. (2005). Effect of the renin-angiotensin system or calcium channel blockade on the circadian variation of heart rate variability, blood pressure and circulating catecholamines in hypertensive patients. J. Hypertens. 23, 1251–1260. doi: 10.1097/01.hjh.0000170389.69202.53
Kessler, W., Diedrich, S., Menges, P., Ebker, T., Nielson, M., Partecke, L. I., et al. (2012). The role of the nerve: modulation of the inflammatory reaction in murine polymicrobial sepsis. Mediat. Inflamm. 2012:467620. doi: 10.1155/2012/467620
Koopman, F. A., Chavan, S. S., Miljko, S., Grazio, S., Sokolovic, S., Schuurman, P. R., et al. (2016). Vagus nerve stimulation inhibits cytokine production and attenuates disease severity in Rheumatoid arthritis. Proc. Natl. Acad. Sci. U.S.A. 113, 8284–8289. doi: 10.1073/pnas.1605635113
Kovacic, K., Hainsworth, K., Sood, M., Chelimsky, G., Unteutsch, R., Nugent, M., et al. (2017). Neurostimulation for abdominal pain-related functional gastrointestinal disorders in adolescents: a randomised, double-blind, sham-controlled trial. Lancet Gastroenterol. Hepatol. 2, 727–737. doi: 10.1016/S2468-1253(17)30253-4
Kox, M., van Eijk, L. T., Verhaak, T., Frenzel, T., Kiers, H. D., Gerretsen, J., et al. (2015). Transvenous vagus nerve stimulation does not modulate the innate immune response during experimental human endotoxemia: a randomized controlled study. Arthritis Res. Ther. 17:150. doi: 10.1186/s13075-015-0667-5
Kox, M., Vaneker, M., van der Hoeven, J. G., Scheffer, G. J., Hoedemaekers, C. W., and Pickkers, P. (2012). Effects of vagus nerve stimulation and vagotomy on systemic and pulmonary inflammation in a two-hit model in rats. PLoS ONE 7:e34431. doi: 10.1371/journal.pone.0034431
Kraus, T., Hösl, K., Kiess, O., Schanze, A., Kornhuber, J., and Forster, C. (2007). BOLD fMRI deactivation of limbic and temporal brain structures and mood enhancing effect by transcutaneous vagus nerve stimulation. J. Neural Transm. 114, 1485–1493. doi: 10.1007/s00702-007-0755-z
Krzyzaniak, M. J., Peterson, C. Y., Cheadle, G., Loomis, W., Wolf, P., Kennedy, V., et al. (2011). Efferent vagal nerve stimulation attenuates acute lung injury following burn: the importance of the gut-lung axis. Surgery 150, 379–389. doi: 10.1016/j.surg.2011.06.008
Kwan, H., Garzoni, L., Liu, H. L., Cao, M., Desrochers, A., Fecteau, G., et al. (2016). Vagus nerve stimulation for treatment of inflammation: systematic review of animal models and clinical studies. Bioelectron. Med. 3, 1–6. doi: 10.15424/bioelectronmed.2016.00005
Lehtimäki, J., Hyvärinen, P., Ylikoski, M., Bergholm, M., Mäkelä, J. P., Aarnisalo, A., et al. (2013). Transcutaneous vagus nerve stimulation in tinnitus: a pilot study. Acta Otolaryngol. 133, 378–382. doi: 10.3109/00016489.2012.750736
Levy, G., Fishman, J. E., Xu, D. Z., Dong, W., Palange, D., Vida, G., et al. (2012). Vagal nerve stimulation modulates gut injury and lung permeability in trauma-hemorrhagic shock. J. Trauma Acute Care Surg. 73, 338–342. doi: 10.1097/TA.0b013e31825debd3
Li, M., Zheng, C., Sato, T., Kawada, T., Sugimachi, M., and Sunagawa, K. (2004). Vagal nerve stimulation markedly improves long-term survival after chronic heart failure in rats. Circulation 109, 120–124. doi: 10.1161/01.CIR.0000105721.71640.DA
Liu, J. J., Huang, N., Lu, Y., Zhao, M., Yu, X. J., Yang, Y., et al. (2015). Improving vagal activity ameliorates cardiac fibrosis induced by angiotensin II: in vivo and in vitro. Sci. Rep. 5:17108. doi: 10.1038/srep17108
Liu, Y., Tao, T., Li, W., and Bo, Y. (2017). Regulating autonomic nervous system homeostasis improves pulmonary function in rabbits with acute lung injury. BMC Pulm. Med. 17:98. doi: 10.1186/s12890-017-0436-0
Lockard, J. S., Congdon, W. C., and DuCharme, L. L. (1990). Feasibility and safety of vagal stimulation in monkey model. Epilepsia 31, S20–S26. doi: 10.1111/j.1528-1157.1990.tb05844.x
Lowry, D. M., Morishita, K., Eliceiri, B. P., Bansal, V., Coimbra, R., and Costantini, T. W. (2014). The vagus nerve alters the pulmonary dendritic cell response to injury. J. Surg. Res. 192, 12–18. doi: 10.1016/j.jss.2014.06.012
Mahadi, K. M., Lall, V. K., Deuchars, S. A., and Deuchars, J. (2019). Cardiovascular autonomic effects of transcutaneous auricular nerve stimulation via the tragus in the rat involve spinal cervical sensory afferent pathways. Brain Stimul. 12, 1151–1158. doi: 10.1016/j.brs.2019.05.002
Malik, M., Camm, A. J., Bigger, J. T., Breithardt, G., Cerutti, S., Cohen, R. J., et al. (1996). Heart rate variability. Standards of measurement, physiological interpretation, and clinical use. Eur. Heart J. 17, 354–381. doi: 10.1093/oxfordjournals.eurheartj.a014868
Marshall, R., Taylor, I., Lahr, C., Abell, T. L., Espinoza, I., Gupta, N. K., et al. (2015). Bioelectrical stimulation for the reduction of inflammation in inflammatory bowel disease. Clin. Med. Insights Gastroenterol. 8, 55–59. doi: 10.4137/CGast.S31779
Mercante, B., Ginatempo, F., Manca, A., Melis, F., Enrico, P., and Deriu, F. (2018). Anatomo-physiologic basis for auricular stimulation. Med. Acupunct. 30, 141–150. doi: 10.1089/acu.2017.1254
Mertens, A., Raedt, R., Gadeyne, S., Carrette, E., Boon, P., and Vonck, K. (2018). Recent advances in devices for vagus nerve stimulation. Expert Rev. Med. Devices 15, 527–539. doi: 10.1080/17434440.2018.1507732
Nanchal, R. S., and Truwit, J. D. (2018). Recent advances in understanding and treating acute respiratory distress syndrome [version 1; referees: 2 approved]. F1000 Res. 7:1322. doi: 10.12688/f1000research.15493.1
Napadow, V., Edwards, R. R., Cahalan, C. M., Mensing, G., Greenbaum, S., Valovska, A., et al. (2012). Evoked pain analgesia in chronic pelvic pain patients using respiratory-gated auricular vagal afferent nerve stimulation. Pain Med. 13, 777–789. doi: 10.1111/j.1526-4637.2012.01385.x
Nonis, R., D'Ostilio, K., Schoenen, J., and Magis, D. (2017). Evidence of activation of vagal afferents by non-invasive vagus nerve stimulation: an electrophysiological study in healthy volunteers. Cephalalgia 37, 1285–1293. doi: 10.1177/0333102417717470
Oleson, T. (2002). Auriculotherapy stimulation for neuro-rehabilitation. NeuroRehabilitation 17, 49–62. doi: 10.3233/NRE-2002-17107
Olshansky, B., Sabbah, H. N., Hauptman, P. J., and Colucci, W. S. (2008). Parasympathetic nervous system and heart failure pathophysiology and potential implications for therapy. Circulation 118, 863–871. doi: 10.1161/CIRCULATIONAHA.107.760405
Osterziel, K. J., Dankward Hänlein, R., and Dietz (1994). Interactions between the renin-angiotensin system and the parasympathetic nervous system in heart failure. J. Cardiovasc. Pharmacol. 24, S70–S74.
Pavlov, V. A., and Tracey, K. J. (2012). The vagus nerve and the inflammatory reflex - linking immunity and metabolism. Nat. Rev. Endocrinol. 8, 743–754. doi: 10.1038/nrendo.2012.189
Payrits, T., Ernst, A., Ladits, E., Pokorny, H., Viragos, I., and Längle, F. (2011). Vagal stimulation -a new possibility for conservative treatment of peripheral arterial occlusion disease. Zentralblatt Chir. Zeitschr Allg. Visz. Gefasschir. 136, 431–435. doi: 10.1055/s-0031-1283739
Peterson, C. Y., Krzyzaniak, M., Coimbra, R., and Chang, D. C. (2012). Vagus nerve and postinjury inflammatory response. Arch. Surg. 147, 76–80. doi: 10.1001/archsurg.2011.237
Polak, T., Markulin, F., Ehlis, A. C., Langer, J. B. M., Ringel, T. M., and Fallgatter, A. J. (2009). Far field potentials from brain stem after transcutaneous Vagus nerve stimulation: optimization of stimulation and recording parameters. J. Neural Transm. 116, 116. doi: 10.1007/s00702-009-0282-1
Poulat, P., and Couture, R. (1998). Increased pulmonary vascular permeability and oedema induced by intrathecally injected endothelins in rat. Eur. J. Pharmacol. 344, 251–259. doi: 10.1016/S0014-2999(97)01569-0
Powell, K., Shah, K., Hao, C., Wu, Y.-C., John, A., Narayan, R. K., et al. (2019). Neuromodulation as a new avenue for resuscitation in hemorrhagic shock. Bioelectron. Med. 5:17. doi: 10.1186/s42234-019-0033-z
Reys, L. G., Ortiz-Pomales, Y. T., Lopez, N., Cheadle, G., De Oliveira, P. G., Eliceiri, B., et al. (2013). Uncovering the neuroenteric-pulmonary axis: vagal nerve stimulation prevents acute lung injury following hemorrhagic shock. Life Sci. 92, 783–792. doi: 10.1016/j.lfs.2013.02.009
Richards, D., and Marley, J. (1998). Stimulation of auricular acupuncture points in weight loss. Aust. Fam. Phys. 27, S73–S77.
Roberts, A., Sithole, A., Sedghi, M., Walker, C. A., and Quinn, T. M. (2016). Minimal adverse effects profile following implantation of periauricular percutaneous electrical nerve field stimulators: a retrospective cohort study. Med. Devices Evid. Res. 3, 389–393. doi: 10.2147/MDER.S107426
Rong, P., Liu, J., Wang, L., Liu, R., Fang, J., Zhao, J., et al. (2016). Effect of transcutaneous auricular vagus nerve stimulation on major depressive disorder: a nonrandomized controlled pilot study. J. Affect. Disord. 195, 172–179. doi: 10.1016/j.jad.2016.02.031
Rong, P. J., Fang, J. L., Wang, L. P., Meng, H., Liu, J., Ma, Y., et al. (2012). Transcutaneous vagus nerve stimulation for the treatment of depression: a study protocol for a double blinded randomized clinical trial. BMC Complement. Altern. Med. 27, S73–S77. doi: 10.1186/1472-6882-12-255
Salama, M., Akan, A., and Mueller, M. (2017). B-005 Vagus nerve stimulation attenuates the acute postsurgical inflammatory response after lung lobectomy. Interact. Cardiovasc. Thorac. Surg. 25:ivx280.005. doi: 10.1093/icvts/ivx280.005
Sator-Katzenschlager, S. M., Scharbert, G., Kozek-Langenecker, S. A., Szeles, J. C., Finster, G., Schiesser, A. W., et al. (2004). The short- and long-term benefit in chronic low back pain through adjuvant electrical versus manual auricular acupuncture. Anesth. Analg. 98, 1359–1364. doi: 10.1213/01.ANE.0000107941.16173.F7
Sator-Katzenschlager, S. M., Szeles, J. C., Scharbert, G., Michalek-Sauberer, A., Kober, A., Heinze, G., et al. (2003). Electrical stimulation of auricular acupuncture points is more effective than conventional manual auricular acupuncture in chronic cervical pain: a pilot study. Anesth. Analg. 97, 1469–1473. doi: 10.1213/01.ANE.0000082246.67897.0B
Schukro, R. P., Heiserer, C., Michalek-Sauberer, A., Gleiss, A., and Sator-Katzenschlager, S. (2014). The effects of auricular electroacupuncture on obesity in female patients - a prospective randomized placebo-controlled pilot study. Complement. Ther. Med. 22, 21–25. doi: 10.1016/j.ctim.2013.10.002
Shinlapawittayatorn, K., Chinda, K., Palee, S., Surinkaew, S., Kumfu, S., Kumphune, S., et al. (2014). Vagus nerve stimulation initiated late during ischemia, but not reperfusion, exerts cardioprotection via amelioration of cardiac mitochondrial dysfunction. Hear. Rhythm 11, 2278–2287. doi: 10.1016/j.hrthm.2014.08.001
Stavrakis, S., Humphrey, M. B., Scherlag, B. J., Hu, Y., Jackman, W. M., Nakagawa, H., et al. (2015). Low-level transcutaneous electrical vagus nerve stimulation suppresses atrial fibrillation. J. Am. Coll. Cardiol. 65, 867–875. doi: 10.1016/j.jacc.2014.12.026
Stavrakis, S., and Po, S. S. (2016). Neuroimmunomodulation: a new frontier of treating cardiovascular diseases. Trends Cardiovasc. Med. 26, 12–13. doi: 10.1016/j.tcm.2015.04.007
Stevens, J. P., Law, A., and Giannakoulis, J. (2018). Acute respiratory distress syndrome. J. Am. Med. Assoc. 319:732. doi: 10.1001/jama.2018.0483
Stojanovich, L., Milovanovich, B., De Luka, S. R., Popovich-Kuzmanovich, D., Bisenich, V., Djukanovich, B., et al. (2007). Cardiovascular autonomic dysfunction in systemic lupus, rheumatoid arthritis, primary Sjögren syndrome and other autoimmune diseases. Lupus 16, 181–185. doi: 10.1177/0961203306076223
Straube, A., Ellrich, J., Eren, O., Blum, B., and Ruscheweyh, R. (2015). Treatment of chronic migraine with transcutaneous stimulation of the auricular branch of the vagal nerve (auricular t-VNS): a randomized, monocentric clinical trial. J. Headache Pain 16:63. doi: 10.1186/s10194-015-0543-3
Szeles, J. C., Kampusch, S., and Kaniusas, E. (2013). “Peripheral blood perfusion controlled by auricular vagus nerve stimulation,” in Proceedings of 17th International Conference on Biomedical Engineering (Kaunas), 73–77.
Széles, J. C., and Litscher, G. (2004). Objectivation of cerebral effects with a new continuous electrical auricular stimulation technique for pain management. Neurol. Res. 26, 797–800. doi: 10.1179/016164104225016100
Tarras, S. L., Diebel, L. N., Liberati, D. M., and Ginnebaugh, K. (2013). Pharmacologic stimulation of the nicotinic anti-inflammatory pathway modulates gut and lung injury after hypoxia-reoxygenation injury. Surgery 154, 841–848. doi: 10.1016/j.surg.2013.07.018
Tekdemir, I., Asian, A., and Elhan, A. (1998). A clinico-anatomic study of the auricular branch of the vagus nerve and Arnold's ear-cough reflex. Surg. Radiol. Anat. 20, 253–257. doi: 10.1007/s00276-998-0253-5
Tracey, K. J. (2007). Physiology and immunology of the cholinergic antiinflammatory pathway. J. Clin. Invest. 117, 289–296. doi: 10.1172/JCI30555
Umbrello, M., Formenti, P., Bolgiaghi, L., and Chiumello, D. (2017). Current concepts of ARDS: a narrative review. Int. J. Mol. Sci. 18:64. doi: 10.3390/ijms18010064
Vaduganathan, M., Vardeny, O., Michel, T., McMurray, J. J. V., Pfeffer, M. A., and Solomon, S. D. (2020). Renin–angiotensin–aldosterone system inhibitors in patients with Covid-19. N. Engl. J. Med. 382, 1653–1659. doi: 10.1056/NEJMsr2005760
Vanoli, E., De Ferrari, G. M., Stramba-Badiale, M., Hull, S. S., Foreman, R. D., and Schwartz, P. J. (1991). Vagal stimulation and prevention of sudden death in conscious dogs with a healed myocardial infarction. Circ. Res. 68, 1471–1481. doi: 10.1161/01.RES.68.5.1471
Vellingiri, B., Jayaramayya, K., Iyer, M., Narayanasamy, A., Govindasamy, V., Giridharan, B., et al. (2020). COVID-19: a promising cure for the global panic. Sci. Total Environ. 725:138277. doi: 10.1016/j.scitotenv.2020.138277
Wang, D.-W., Yin, Y.-M., and Yao, Y.-M. (2016). Vagal Modulation of the Inflammatory Response in Sepsis. Int. Rev. Immunol. 35, 415–433. doi: 10.3109/08830185.2015.1127369
Wang, H., Yu, M., Ochani, M., Amelia, C. A., Tanovic, M., Susarla, S., et al. (2003). Nicotinic acetylcholine receptor α7 subunit is an essential regulator of inflammation. Nature 421, 384–388. doi: 10.1038/nature01339
Wang, Z., Yu, L., Huang, B., Wang, S., Liao, K., Saren, G., et al. (2015). Low-level transcutaneous electrical stimulation of the auricular branch of vagus nerve ameliorates left ventricular remodeling and dysfunction by downregulation of matrix metalloproteinase 9 and transforming growth factor β1. J. Cardiovasc. Pharmacol. 65, 342–348. doi: 10.1097/FJC.0000000000000201
Yang, J., Zheng, Y., Gou, X., Pu, K., Chen, Z., Guo, Q., et al. (2020). Prevalence of comorbidities in the novel Wuhan coronavirus (COVID-19) infection: a systematic review and meta-analysis. Int. J. Infect. Dis. 127:104371. doi: 10.1016/j.ijid.2020.03.017
Ylikoski, J., Lehtimäki, J., Pirvola, U., Mäkitie, A., Aarnisalo, A., Hyvärinen, P., et al. (2017). Non-invasive vagus nerve stimulation reduces sympathetic preponderance in patients with tinnitus. Acta Otolaryngol. 137, 426–431. doi: 10.1080/00016489.2016.1269197
Zamotrinsky, A., Afanasiev, S., Karpov, R. S., and Cherniavsky, A. (1997). Effects of electrostimulation of the vagus afferent endings in patients with coronary artery disease. Coron. Artery Dis. 8, 551–557.
Zamotrinsky, A. V., Kondratiev, B., and De Jong, J. W. (2001). Vagal neurostimulation in patients with coronary artery disease. Auton. Neurosci. Basic Clin. 12, 109–116. doi: 10.1016/S1566-0702(01)00227-2
Zhang, Y., Popović, Z. B., Bibevski, S., Fakhry, I., Sica, D. A., Van Wagoner, D. R., et al. (2009). Chronic vagus nerve stimulation improves autonomic control and attenuates systemic inflammation and heart failure progression in a canine high-rate pacing model. Circ. Hear. Fail. 2, 692–699. doi: 10.1161/CIRCHEARTFAILURE.109.873968
Zheng, Y. Y., Ma, Y. T., Zhang, J. Y., and Xie, X. (2020). COVID-19 and the cardiovascular system. Nat. Rev. Cardiol. 17, 259–260. doi: 10.1038/s41569-020-0360-5
Zhou, F., Yu, T., Du, R., Fan, G., Liu, Y., Liu, Z., et al. (2020). Clinical course and risk factors for mortality of adult inpatients with COVID-19 in Wuhan, China: a retrospective cohort study. Lancet 395, 1054–1062. doi: 10.1016/S0140-6736(20)30566-3
Keywords: parasympathetic system, cholinergic anti-inflammatory pathway, hypothalamic pituitary adrenal axis, sympatho-vagal balance, lung inflammation
Citation: Kaniusas E, Szeles JC, Kampusch S, Alfageme-Lopez N, Yucuma-Conde D, Li X, Mayol J, Neumayer C, Papa M and Panetsos F (2020) Non-invasive Auricular Vagus Nerve Stimulation as a Potential Treatment for Covid19-Originated Acute Respiratory Distress Syndrome. Front. Physiol. 11:890. doi: 10.3389/fphys.2020.00890
Received: 17 May 2020; Accepted: 30 June 2020;
Published: 28 July 2020.
Edited by:
Yu Ru Kou, National Yang-Ming University, TaiwanReviewed by:
Jian Kong, Massachusetts General Hospital and Harvard Medical School, United StatesChing Jung Lai, Tzu Chi University, Taiwan
Copyright © 2020 Kaniusas, Szeles, Kampusch, Alfageme-Lopez, Yucuma-Conde, Li, Mayol, Neumayer, Papa and Panetsos. This is an open-access article distributed under the terms of the Creative Commons Attribution License (CC BY). The use, distribution or reproduction in other forums is permitted, provided the original author(s) and the copyright owner(s) are credited and that the original publication in this journal is cited, in accordance with accepted academic practice. No use, distribution or reproduction is permitted which does not comply with these terms.
*Correspondence: Fivos Panetsos, fivos@ucm.es