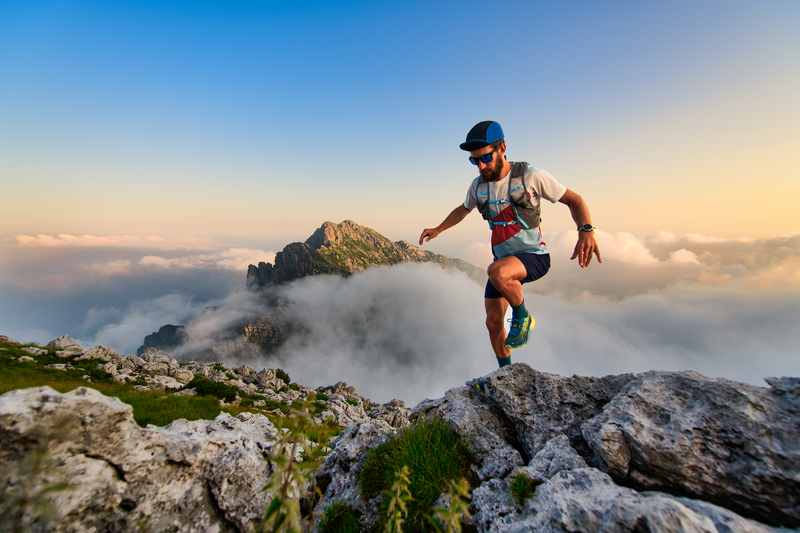
95% of researchers rate our articles as excellent or good
Learn more about the work of our research integrity team to safeguard the quality of each article we publish.
Find out more
ORIGINAL RESEARCH article
Front. Physiol. , 21 July 2020
Sec. Striated Muscle Physiology
Volume 11 - 2020 | https://doi.org/10.3389/fphys.2020.00815
This article is part of the Research Topic Recent Advances on Myocardium Physiology, Volume I View all 17 articles
The chambers of the heart fulfill different hemodynamic functions, which are reflected in their structural and contractile properties. While the atria are highly elastic to allow filling from the venous system, the ventricles need to be able to produce sufficiently high pressures to eject blood into the circulation. The right ventricle (RV) pumps into the low pressure pulmonary circulation, while the left ventricle (LV) needs to overcome the high pressure of the systemic circulation. It is incompletely understood whether these differences can be explained by the contractile differences at the level of the individual cardiomyocytes of the chambers. We addressed this by isolating cardiomyocytes from atria, RV, LV, and interventricular septum (IVS) of five healthy wild-type rats. Using a high-throughput contractility set-up, we measured contractile function of 2,043 cells after overnight culture. Compared to ventricular cardiomyocytes, atrial cells showed a twofold lower contraction amplitude and 1.4- to 1.7-fold slower kinetics of contraction and relaxation. The interventricular differences in contractile function were much smaller; RV cells displayed 12–13% less fractional shortening and 5–9% slower contraction and 3–15% slower relaxation kinetics relative to their LV and IVS counterparts. Aided by a large dataset, we established relationships between contractile parameters and found contraction velocity, fractional shortening and relaxation velocity to be highly correlated. In conclusion, our findings are in line with contractile differences observed at the atrioventricular level, but can only partly explain the interventricular differences that exist at the organ level.
The heart consists of atria and ventricles which at a cellular level are activated through excitation–contraction coupling in a highly coordinated fashion to provide sufficient pressure to maintain perfusion of the body. The structural and contractile properties of each compartment are linked to the hemodynamic function it fulfills. The right ventricle (RV) ejects blood into the low pressure pulmonary vasculature, whereas the left ventricle (LV) pumps blood into the high pressure systemic circulation. As a result, the wall of the RV is considerably thinner and more compliant than the LV wall (Voelkel et al., 2006). The atria function predominantly as a reservoir for venous return and under normal circumstances does not have to overcome high pressures during contraction, which is reflected by highly elastic properties of the atrial myocardium (Blume et al., 2011). Functional differences between the chambers of the heart are also apparent in the differential response to changes in hemodynamics, such as chronic pressure overload (Belin et al., 2011; Rain et al., 2014).
The structural and functional differences between the chambers of the heart may be explained by distinct functional properties of individual cardiomyocytes from each region. Up to date, the contractile function of cardiomyocytes from different regions has not been subject of intensive study. The limited number of studies covering this topic reports inconclusive findings (McMahon et al., 1996; Tanaami et al., 2005; Kondo et al., 2006; Sathish et al., 2006; Belin et al., 2011; Chu et al., 2013), which may be attributed to species differences, methodological differences, selection bias, and small sample sizes (Chung and Campbell, 2013; Sikkel et al., 2017; Clark and Campbell, 2019; Kuster, 2019). Furthermore, regional differences may be small and can therefore only be studied through unbiased, extensive sampling.
We performed large-scale contractility measurements on intact unloaded rat cardiomyocytes isolated from atrial (AT), LV, RV, and interventricular septal (IVS) tissue to assess whether differences in contractile properties exist at the level of single cardiomyocytes.
The animal experiments were performed in accordance with the guidelines from Directive 2010/63/EU of the European Parliament on the protection of animals used for scientific purposes and were approved by the ethics committees of VU University Medical Center, Amsterdam, Netherlands.
Cardiomyocytes were isolated from adult Wistar rats (n = 5) weighing 200–250 g. Intact AT (left and right atrium were combined), LV, RV, and IVS rat cardiomyocytes were isolated through collagenase digestion of hearts essentially as described previously (Sequeira et al., 2015; van Deel et al., 2017). In brief, rats were euthanized via isoflurane inhalation, after which hearts were quickly harvested and washed in cold isolation Tyrode solution (130 mM NaCl, 5.4 mM KCl, 3 mM sodium pyruvate, 25 mM HEPES, 0.5 mM MgCl2, 0.33 mM NaH2PO4, 22 mM glucose, pH 7.4) containing 0.2 mM EGTA (Tyrode–EGTA). Hearts were subsequently cannulated to a Langendorff set-up via the aorta and perfused with Tyrode-EGTA for 2 min at 37°C. Next, hearts were perfused for 25–35 min (depending on the integrity of the tissue) with enzyme Tyrode solution composed of Tyrode solution, 50 μM CaCl2, and 1.2 mg/mL collagenase (Type II, 265 U/mg; Worthington Biochemical, NJ, United States). The heart was removed from the cannula and separated into AT, LV, RV, and IVS. Each part was cut into fine pieces, followed by trituration for 3 min with a plastic Pasteur pipette in stopping buffer solution 1 [SB-1; Tyrode solution, 0.6 mg/mL collagenase, 100 μM CaCl2, and 10 mg/mL bovine serum albumin (BSA)]. Cell suspensions were filtered through a 300 μm nylon mesh filter and collected in 50 mL Falcon tubes, followed by centrifugation for 1 min at 27 × g at room temperature. Pellets containing cardiomyocytes were resuspended in SB solution 2 (SB-2; Tyrode solution, 250 μM CaCl2 and 10 mg/mL BSA) and incubated for 10 min at 37°C in order for the cells to settle. After the removal of supernatants, cardiomyocytes were resuspended in SB solution 3 (SB-3; Tyrode solution, 500 μM CaCl2 and 10 mg/mL BSA) and incubated for 10 min at 37°C. Hereafter, cardiomyocytes were suspended in plating medium [Medium 199 (Lonza, Basel, Switzerland), 1% penicillin/streptomycin and 5% fetal bovine serum (FBS)] and transferred to laminin-coated 35 mm2 glass-bottomed dishes (MatTek, Ashland, MA, United States). Following 1 h of incubation at 37°C in humidified air with 5% CO2, unattached cells were washed away by replacing plating medium with culture medium [Medium 199, 1% penicillin/streptomycin, insulin transferrin selenium (ITS; Sigma-Aldrich, composition: insulin, 10 mg/L; transferrin, 5.5 mg/L; selenium 5 μg/L) and 0.5 μM cytochalasin D (Life Technologies)]. The latter two compounds were added in order to prevent dedifferentiation and optimize cell viability (Viero et al., 2008; Tian et al., 2012). Cardiomyocytes were cultured overnight in an incubator at 37°C in humidified air with 5% CO2.
Cardiomyocyte contractility measurements were performed using the CytoCypher Multicell High Throughput System (CytoCypher BV, Wageningen, Netherlands). To measure contractility, culture medium was replaced by experimental Tyrode solution (137 mM NaCl, 5.4 mM KCl, 3 mM sodium pyruvate, 5 mM HEPES, 0.57 mM MgCl2, 0.33 mM NaH2PO4, 1.8 mM CaCl2 and 5.6 mM glucose, pH 7.4). Dishes were placed on a fast x–y–z position programmable scanning microscope, allowing rapid identification and measurements of contracting cardiomyocytes. Cell shortening experiments were performed as described previously (Juni et al., 2019). Contraction was evoked via electrical field stimulation (2 Hz, 4 ms pulse duration, 25 V) at 37°C. Sarcomere shortening and relaxation kinetics were quantified via a video-based sarcomere length (SL) detection system (IonOptix corporation, Milton, MA, United States) at 250 Hz sampling frequency. Each cell was measured for 5 s, in which 8–9 contraction traces could be recorded (see Figure 1 for an example of a full-length trace of a single cell). All contracting cardiomyocytes that could be measured within a 20 min time frame per dish were measured. We observed no significant rundown in contractility during this period. In a subset of cells, cardiomyocyte width and length were assessed via edge detection. Width was measured at three locations along the length of the cell (at 25, 50, and 75%). Contractility assays were performed within 18–24 h after isolation. During this period, no decline in contractility was observed.
Figure 1. Example trace of a full-length recording in a single cell. Cells were paced with a frequency of 2 Hz. Sarcomere length was measured for 5 s, in which 8 or 9 contractions could be recorded.
A schematic overview of data analysis is depicted in Figure 2. The batch of raw contractility data was analyzed using the CytoSolver Transient Analysis Tools package from CytoCypher BV (Wageningen, Netherlands) to yield averaged contractile and kinetic parameters from each cell. With this software, the relaxation phase is fitted with a bi-exponential fit starting at 10% return time. Individual cells were excluded from further analysis if they did not meet all inclusion criteria (Figure 2). Data were subsequently tested for normality and transformed via log or square root transformation when appropriate. Hierarchical clustering analysis (Sikkel et al., 2017) was performed to quantify the amount of clustering for each rat and dish. If the data were tightly grouped, appropriate corrections to the statistical significance test were applied. A student’s t-test or two-way analysis of variance (ANOVA) where appropriate was performed to test for differences between regions, followed by Bonferroni post hoc testing. The significance level was set at p < 0.01.
Figure 2. Schematic overview of data processing and analysis. In total, 2,512 cells were measured, of which 2,043 were included in analysis. If no more than four contraction transients could be fitted by the transient analysis software (i.e., due to disturbances in the sarcomere length signal), cells were excluded from the analysis. Short resting sarcomere length and inaccurate fitting of the contraction transients were additional exclusion criteria.
In total, 2,512 cardiomyocytes from five healthy rats were measured, of which 2,043 met the inclusion criteria for further analysis (Figure 2). Representative cells from each region are shown in Figure 3. Averaged contraction and velocity traces of each region are depicted in Figures 4A–C. These traces indicate markedly different contraction characteristics in AT cells compared to cells isolated from ventricular regions. A principal component analysis (PCA) was carried out to assess if cardiomyocytes from AT, LV, RV, and IVS display clustering based on their respective origin (Figure 4D). Hereto, redundant variables (e.g., “Time to Peak 10%,” “Time to Peak 20%”) were omitted to avoid overrepresentation of similar variables in the dimension axes. This confirmed that only cells from AT were clearly distinct from cells from the ventricular regions. To elucidate, the main purpose of a PCA is to reduce the number of variables in a dataset, especially when many variables are correlated with each other, while retaining the variation and differences in the dataset (Jolliffe and Cadima, 2016). Hence, the variables are “combined” mathematically into several dimensions that indicate the percentage of differences retained. In our case, Dim 1 represents 37% of the difference in the dataset, while Dim 2 represents 35%. In Supplementary Figure S1, the length of the arrow indicates how strongly the parameter affects the dimension. Thus, fractional shortening, relaxation velocity, contraction velocity, and time to peak all contribute substantially to the difference between AT and ventricular cells. Excluding the AT cells from the analysis showed that ventricular cells do not display clustering based on regional origin (Figure 4E).
Figure 3. Representative cells from each cardiac region. Cells from atrium (AT) were markedly thinner and shorter than cells from left ventricle (LV) and right ventricle (RV) and interventricular septum (IVS).
Figure 4. Large atrioventricular and small interventricular differences in contractile function. Contractile function was compared between cardiomyocytes isolated from atria (AT; n = 272), left (LV; n = 553), and right ventricle (RV; n = 711) and interventricular septum (IVS; n = 507) from five rats. (A) Averaged and (B) normalized contraction traces and (C) averaged velocity traces for each cardiac region. Shading indicates SEM. (D) Principal component analysis displays clear clustering of AT cells. (E) Principal component analysis with AT cells omitted shows no separate clusters at the ventricular level.
To further address how contractility of AT cells differ from ventricular cells, data from LV, RV, and IVS cells were pooled (labeled “VT”) and tested for significance against AT cells after correcting for normality and variation arising from rat and dish differences (Table 1). Cells from AT tissue were found to differ strongly from VT cells for every parameter tested except 80% relaxation time and relaxation coefficient tau. Most notably, fractional shortening in AT cells was twofold lower compared to VT cells. Velocities of contraction and relaxation were substantially lower in AT cells compared to values observed in VT cardiomyocytes. Despite lower contraction velocity, AT cells reached peak earlier than VT cells. Similarly, partial relaxation is achieved earlier in AT cells than VT cells, in spite of lower maximal relaxation velocity in AT cells. In terms of cell dimensions, AT cells were found to be both shorter and smaller compared to VT cells, indicating that, as anticipated, atrial cardiomyocytes are both structurally and functionally distinct from ventricular cardiomyocytes.
To define if differences between RV and LV structure and function arise from inherent changes in the cardiomyocytes that make up the ventricles, we compared dimensions and contractile properties of RV, LV, and IVS cardiomyocytes (Table 2). RV cells were found to have a lower amplitude of contraction than LV and IVS when expressed both in absolute terms (peak height) as well as relative terms (fractional shortening). With respect to contraction kinetics, RV cardiomyocytes showed a slightly lower velocity of shortening compared to LV and IVS. Relaxation velocity in IVS cells was higher compared to RV and LV cells. Compared to LV, a trend toward lower relaxation velocity was observed in RV (p = 0.02). RV cells moreover reached peak earlier than LV and IVS cells. Lastly, with respect to cell dimensions, LV cardiomyocytes were larger both in terms of width and length compared to RV. The analysis on regional differences in fractional shortening and contraction and relaxation velocities were performed on cardiomyocytes from five animals taken together, but can also be observed when looking at individual animals (Supplementary Figure S2).
The size of our dataset allowed us to establish correlations between contractility parameters (Figure 5). Contraction velocity strongly correlates with relaxation velocity (R2 = 0.68). Fractional shortening displays robust correlation with both contraction velocity (R2 = 0.55) and relaxation velocity (R2 = 0.59), but correlates poorly with time to peak (R2 = 0.06). No regional differences were observed in correlations between these parameters when RV, LV, and IVS cells were analyzed separately (data not shown). Fractional shortening shows no correlation with relaxation time (50% relaxation time; R2 = 0.004), and relaxation coefficient (tau; R2 = 0.06). These findings imply that contraction velocity is the most important determinant of the degree of contraction and the velocity of subsequent relaxation.
Figure 5. Correlations between contractility parameters. Correlation analysis was performed on ventricular cardiomyocytes (n = 1,771). Distribution plots and individual cardiomyocytes are colored based on their origin: RV (orange), LV (purple), and IVS (blue). All correlations with an R2 larger than 0.01 are statistically significant at the level of p < 0.001.
The large-scale dataset obtained in this study provides an accurate estimation of the variation that is present among ventricular cardiomyocytes. Therefore, the standard deviations of the parameters reported in this study (Tables 1, 2) can be used to calculate the sample size that is needed to uncover an anticipated effect size. Calculating the sample size for a range of effect sizes generates an exponential function, which is exemplified in Figure 6 for fractional shortening, contraction velocity, and relaxation velocity. When subtle differences are subject of study, such as the regional differences reported here, a large sample size is required, in particular when kinetics of contraction are addressed. To elucidate, fractional shortening in RV cells was 7.8%, and in IVS cells, it was 8.8%. Hence, this constitutes an effect size of 13% and requires a sample size of 140 per group in order to be detected with statistical significance at the level of p < 0.01. In contrast, β-adrenergic receptor stimulation with isoproterenol typically induces effect sizes of more than 50% (Harding et al., 1988; Najafi et al., 2016) and hence can be studied using relatively small sample sizes (n = 10–20). This may serve as guidance to future studies investigating contractility differences between (experimental) conditions.
Figure 6. Relationships between effect and sample size. Sample sizes needed to detect a range of effect sizes were calculated and plotted, using the variability of ventricular cells in: (A) fractional shortening, (B) contraction velocity, and (C) relaxation velocity. Solid line shows effect size at significance level of p < 0.05, dashed line of p < 0.01. Red arrows indicate sample size required for uncovering effect sizes at the magnitude of the regional differences identified in this study.
In the present study, we deployed a high-throughput system to measure contractile properties and cell dimensions in AT, LV, RV, and IVS cardiomyocytes isolated from healthy rats. We observed major differences between AT and ventricular cells. Moreover, we report relatively small, but significant contractile and dimensional differences between cardiomyocytes from ventricular regions, most notably lower contraction amplitude and kinetics in RV cardiomyocytes compared with LV and IVS. Differences between atrial and ventricular cardiomyocytes are in keeping with the respective functions of atria and ventricles. The much smaller differences between RV, IVS, and LV cardiomyocytes can only partly explain the differences between RV and LV that exist at the organ level. Our large dataset enabled us to detect small differences despite the remarkable heterogeneity we demonstrate to be present among cells. We could also correlate contractile parameters, revealing a strong correlation between contraction velocity, fractional shortening, and relaxation velocity. Taken together, our study highlights the importance of extensive, unbiased sampling when performing studies on cardiomyocyte contractility.
To the best of our knowledge, unloaded shortening in AT cardiomyocytes relative to ventricular cells has not been comprehensively assessed. A study by Tanaami et al. (2005) reports no contractile differences between rat AT and VT cardiomyocytes in terms of fractional shortening. However, this was based on experiments in a small number of cardiomyocytes (n = 12–30) in which a large standard error was observed. Under loaded conditions, Luss et al. (1999) demonstrated AT cells to reach peak force development in a shorter time period than LV cells, which is in good agreement with our observation that AT cells achieve peak shortening earlier than ventricular cells (Table 1). This might in part be explained by higher expression of the fast α-isoform of cardiac myosin heavy chain (MHC) and faster cross-bridge kinetics in atrial relative to ventricular tissue (Reiser and Kline, 1998; Andruchov et al., 2006), although in the present study contraction velocity was lower in atrial than in ventricular cardiomyocytes. Relative to ventricular cells, AT cells display shorter action potentials, more heterogeneous intracellular Ca2+ transient propagation, and higher sarcoplasmic reticulum (SR) Ca2+-ATPase (SERCA2) levels (Minajeva et al., 1997; Luss et al., 1999; Walden et al., 2009; Greiser et al., 2014); hence, Ca2+-release from the SR and subsequent cross-bridge activation may be less uniformly orchestrated, which combined with faster Ca2+ re-uptake may give rise to short-lived, relatively slow contractions (Brandenburg et al., 2016). This would explain the low amplitude of fractional shortening and slow kinetics of contraction observed in our study. Faster Ca2+ re-uptake may also in part explain why in our study AT cells required less time to re-lengthen during the early phase of relaxation, although lower relaxation velocity indicates overall slower relengthening than ventricular cells.
Our findings predominantly suggest that RV cardiomyocytes show reduced contraction amplitude and kinetics of contraction and relaxation compared to LV and IVS. Hence, under unloaded conditions cardiomyocytes display similarity to RV and LV contraction in vivo, i.e., less shortening and slower relaxation as assessed by tricuspid and mitral valve inflow in RV versus LV (Zoghbi et al., 1990; Pela et al., 2004; Hudsmith et al., 2005). Comparing shortening velocity under unloaded conditions to tissue contraction velocity is complicated due to differences in fiber orientation, which is more longitudinal in the RV and more circular in the LV (Naito et al., 1995). Accordingly, in the RV, longitudinal shortening is the main contributor to contraction, as opposed to circumferential shortening in the LV.
Our general finding of lower contractility in the RV versus LV is consistent with other studies reporting interventricular differences in unloaded shortening in rodent cardiomyocytes (Kondo et al., 2006; Sathish et al., 2006; Chu et al., 2013). However, the percent difference in shortening between LV and RV cells in these studies is considerably larger (ranging from 25 to 80%) than the difference we report here (12%). A similar trend is seen for kinetics of contraction, where we measured a 5% difference in contraction velocity between RV and LV, compared to 44% in the study by Kondo et al. (2006). Importantly, we measured shortening at a tightly controlled physiological temperature (37°C), whereas comparable experiments were carried out at less optimally controlled room temperature (19–22°C) (Kondo et al., 2006; Sathish et al., 2006; Chu et al., 2013). Cross-bridge cycling and concomitant cardiomyocyte shortening are highly temperature sensitive (de Tombe and Stienen, 2007; Chung and Campbell, 2013); thus, any differences that may exist between RV and LV cells could be magnified when measuring at non-physiological temperatures. Also, we detected no LV versus RV difference in relaxation as defined by the time constant tau, dissimilarly to Chu et al. (2013) who report a twofold higher value in RV versus LV. This discrepancy might be explained by different experimental temperatures, as this heavily influences cardiomyocyte relaxation (Chung and Campbell, 2013). A study by McMahon et al. (1996) addressing unloaded shortening in large numbers of porcine cardiomyocytes reported higher contractility in RV compared to LV cells, which is in contrast to findings in rodents. As these data were gathered under experimental conditions identical to our study, their findings likely reflect a species difference.
The exact mechanisms underlying the interventricular differences reported here remain largely enigmatic. The RV has a different embryological origin than the LV (Cai et al., 2003); hence, contractile differences may simply be a reflection thereof. Additionally, the LV and RV may adapt to loading conditions not only structurally via cardiomyocyte count but also at the level of individual cells. The latter is also reflected in the cell dimension difference we observed, with LV cells being on average larger than RV cells. Findings from proteomic studies indicate LV versus RV differences in protein expression levels of sarcomere proteins such as myosin light chain 2, α-MHC and cardiac myosin binding protein C, and tropomyosin- and titin isoforms, i.e., more stiff titin in the LV (Comunian et al., 2011; Cadete et al., 2012; Peng et al., 2013). These differences may partly contribute to the contraction properties of LV and RV myofilaments described by Belin et al. (2011), in addition to the differences in myofilament phosphorylation they observed. Titin isoform composition could play a role in the kinetics and extent of contraction, as we recently showed (Najafi et al., 2019). Furthermore, LV versus RV differences have been found in terms of ion channel make-up and regulation (Gaborit et al., 2007; Molina et al., 2016; Zaitsev et al., 2019). As the IVS separates the LV and RV and is formed from the cell populations of both LV and RV embryological origin in rodents (Franco et al., 2006), we expected IVS cells to have contractile parameters intermediate between RV and LV. However, there were very few significant differences between LV and IVS, but almost all measured parameters were different between IVS and RV. This indicates that with respect to contraction/relaxation, IVS and LV are more similar to each other than the RV. Taken together, LV and RV are distinct from one another in multiple cellular aspects, which may provide a substrate for contractile differences and represents an interesting avenue for future research.
We observed remarkable intraregional variation in cardiomyocyte contractility, which may stem from several factors. For example, Clark and Campbell (2019) recently demonstrated that variation in unloaded rat cardiomyocytes decreases if cells are sampled from a smaller area, implying that local stretch and strain and paracrine signaling impact on cellular contractility (Kuster, 2019). Heterogeneity may also arise from differences between transmural regions. Transmural differences have been described for numerous properties, such as length-dependent activation, action potential waveforms, calcium homeostasis, mitochondrial respiration, ion channel make-up, and myofilament protein phosphorylation (Gaborit et al., 2007; Cazorla and Lacampagne, 2011; Gregorich et al., 2015; Kindo et al., 2016), which may all influence contractility. Experiments in a limited number of unloaded cardiomyocytes from subepicardial, midmyocardial and subendocardial regions suggest the existence of subtle contractile differences (Chung and Campbell, 2013), which would be an interesting subject for more extensive study.
The large scale of our dataset enabled us to look for correlations between different parameters. We found a strong correlation between contraction velocity, fractional shortening, and relaxation velocity. The correlation between relaxation velocity and amplitude of shortening was previously described to occur in canine (Tsutsui et al., 1993) and porcine cardiomyocytes (McMahon et al., 1996). Also in a recent paper by Clark and Campbell (2019), a correlation was observed between cell shortening and time to 50% relaxation in rat cardiomyocytes. Cells that showed a greater amplitude of cell shortening had a shorter relaxation time. In the current study, the velocity of relaxation correlated much better to shortening than time to 50% relaxation. This might be expected as relaxation time is more dependent on the amplitude of contraction than velocity. The observation that there is a robust correlation between contraction and relaxation velocity was shown in an elegant paper by Janssen (2010). Here he compiled data from isometric contractions of mouse trabeculae that his group had gathered over the years and showed that under a wide variety of conditions, contraction and relaxation velocities correlated very strongly. The relationship between kinetics of contraction and relaxation seemed to be a property of the myofilaments, as perturbations to calcium transients did not influence the correlation (Janssen, 2010). The exact mechanism that determines this relationship is not well understood, but could involve cardiac myosin binding protein C (Janssen, 2010), which is an important mediator of cross-bridge cycling (Previs et al., 2014; Sequeira et al., 2014).
We acknowledge several methodological limitations in this study. We combined cardiomyocytes from the left and right atrium in order to obtain a sufficient number of atrial cells, thus obscuring contractile differences that may exist between cardiomyocytes from these two regions. Furthermore, measurements were performed after overnight culture, allowing cells to recover from stress and damage induced during isolation (Mitcheson et al., 1998) and enabling us to perform all individual animal measurements on a single day. However, during this culturing period, regional contractile differences may become less pronounced compared to that directly after isolation.
Here, we report large atrioventricular contractile differences that are in line with differences at the organ level. The interventricular differences we found are more subtle and reflect only in part the differences observed in vivo. Through extensive sampling we furthermore identified strong correlations between contraction velocity, fractional shortening and relaxation velocity. Lastly, our large-scale dataset demonstrates notable intraregional heterogeneity, providing valuable methodological insights for future cardiomyocyte shortening studies.
All datasets generated for this study are available upon request.
The animal study was reviewed and approved by the ethics committees of the VU University Medical Center, Amsterdam, Netherlands.
EN, EM, MH, and DK designed the study, wrote the manuscript, and performed the experiments and data analysis. MG, VJ, CB, and JO helped performing the experiments and edited the manuscript. JV helped drafting and revising the manuscript. All authors contributed to the article and approved the submitted version.
We acknowledge support from the Netherlands Cardiovascular Research Initiative, an initiative with support from the Dutch Heart Foundation, CVON2014-40 DOSIS & CVON2017−18 ARENA−PRIME, and NWO (NWO-ZonMW; 91818602 VICI) grant to JV. The project is co-funded by the PPP Allowance made available by Health∼Holland, Top Sector Life Sciences & Health, to stimulate public–private partnerships, DK and MH.
EM is an employee of CytoCypher BV. MH is CEO of CytoCypher BV.
The remaining authors declare that the research was conducted in the absence of any commercial or financial relationships that could be construed as a potential conflict of interest.
The Supplementary Material for this article can be found online at: https://www.frontiersin.org/articles/10.3389/fphys.2020.00815/full#supplementary-material
Andruchov, O., Andruchova, O., and Galler, S. (2006). Fine-tuning of cross-bridge kinetics in cardiac muscle of rat and mouse by myosin light chain isoforms. Pflugers Arch. 452, 667–673. doi: 10.1007/s00424-006-0080-7
Belin, R. J., Sumandea, M. P., Sievert, G. A., Harvey, L. A., Geenen, D. L., Solaro, R. J., et al. (2011). Interventricular differences in myofilament function in experimental congestive heart failure. Pflugers Arch. 462, 795–809. doi: 10.1007/s00424-011-1024-4
Blume, G. G., McLeod, C. J., Barnes, M. E., Seward, J. B., Pellikka, P. A., Bastiansen, P. M., et al. (2011). Left atrial function: physiology, assessment, and clinical implications. Eur. J. Echocardiogr. 12, 421–430. doi: 10.1093/ejechocard/jeq175
Brandenburg, S., Arakel, E. C., Schwappach, B., and Lehnart, S. E. (2016). The molecular and functional identities of atrial cardiomyocytes in health and disease. Biochim. Biophys. Acta 1863(7 Pt B), 1882–1893. doi: 10.1016/j.bbamcr.2015.11.025
Cadete, V. J., Lin, H. B., Sawicka, J., Wozniak, M., and Sawicki, G. (2012). Proteomic analysis of right and left cardiac ventricles under aerobic conditions and after ischemia/reperfusion. Proteomics 12, 2366–2377. doi: 10.1002/pmic.201100604
Cai, C. L., Liang, X., Shi, Y., Chu, P. H., Pfaff, S. L., Chen, J., et al. (2003). Isl1 identifies a cardiac progenitor population that proliferates prior to differentiation and contributes a majority of cells to the heart. Dev. Cell 5, 877–889. doi: 10.1016/s1534-5807(03)00363-0
Cazorla, O., and Lacampagne, A. (2011). Regional variation in myofilament length-dependent activation. Pflugers Arch. 462, 15–28. doi: 10.1007/s00424-011-0933-6
Chu, C., Thai, K., Park, K. W., Wang, P., Makwana, O., Lovett, D. H., et al. (2013). Intraventricular and interventricular cellular heterogeneity of inotropic responses to alpha(1)-adrenergic stimulation. Am. J. Physiol. Heart Circ. Physiol. 304, H946–H953.
Chung, C. S., and Campbell, K. S. (2013). Temperature and transmural region influence functional measurements in unloaded left ventricular cardiomyocytes. Physiol. Rep. 1:e00158. doi: 10.1002/phy2.158
Clark, J. A., and Campbell, S. G. (2019). Diverse relaxation rates exist among rat cardiomyocytes isolated from a single myocardial region. J. Physiol. 597, 711–722. doi: 10.1113/jp276718
Comunian, C., Rusconi, F., De Palma, A., Brunetti, P., Catalucci, D., and Mauri, P. L. (2011). A comparative MudPIT analysis identifies different expression profiles in heart compartments. Proteomics 11, 2320–2328. doi: 10.1002/pmic.201000479
de Tombe, P. P., and Stienen, G. J. (2007). Impact of temperature on cross-bridge cycling kinetics in rat myocardium. J. Physiol. 584(Pt 2), 591–600. doi: 10.1113/jphysiol.2007.138693
Franco, D., Meilhac, S. M., Christoffels, V. M., Kispert, A., Buckingham, M., and Kelly, R. G. (2006). Left and right ventricular contributions to the formation of the interventricular septum in the mouse heart. Dev. Biol. 294, 366–375. doi: 10.1016/j.ydbio.2006.02.045
Gaborit, N., Le Bouter, S., Szuts, V., Varro, A., Escande, D., Nattel, S., et al. (2007). Regional and tissue specific transcript signatures of ion channel genes in the non-diseased human heart. J. Physiol. 582(Pt 2), 675–693. doi: 10.1113/jphysiol.2006.126714
Gregorich, Z. R., Peng, Y., Lane, N. M., Wolff, J. J., Wang, S., Guo, W., et al. (2015). Comprehensive assessment of chamber-specific and transmural heterogeneity in myofilament protein phosphorylation by top-down mass spectrometry. J. Mol. Cell Cardiol. 87, 102–112. doi: 10.1016/j.yjmcc.2015.08.007
Greiser, M., Kerfant, B. G., Williams, G. S., Voigt, N., Harks, E., Dibb, K. M., et al. (2014). Tachycardia-induced silencing of subcellular Ca2+ signaling in atrial myocytes. J. Clin. Invest. 124, 4759–4772. doi: 10.1172/jci70102
Harding, S. E., Vescovo, G., Kirby, M., Jones, S. M., Gurden, J., and Poole-Wilson, P. A. (1988). Contractile responses of isolated adult rat and rabbit cardiac myocytes to isoproterenol and calcium. J. Mol. Cell Cardiol. 20, 635–647. doi: 10.1016/s0022-2828(88)80121-4
Hudsmith, L. E., Petersen, S. E., Francis, J. M., Robson, M. D., and Neubauer, S. (2005). Normal human left and right ventricular and left atrial dimensions using steady state free precession magnetic resonance imaging. J Cardiovasc. Magn. Reson. 7, 775–782. doi: 10.1080/10976640500295516
Janssen, P. M. (2010). Kinetics of cardiac muscle contraction and relaxation are linked and determined by properties of the cardiac sarcomere. Am. J. Physiol. Heart Circ. Physiol. 299, H1092–H1099.
Jolliffe, I. T., and Cadima, J. (2016). Principal component analysis: a review and recent developments. Philos. Trans. A Math. Phys. Eng. Sci. 374:20150202. doi: 10.1098/rsta.2015.0202
Juni, R. P., Kuster, D. W. D., Goebel, M., Helmes, M., Musters, R. J. P., van der Velden, J., et al. (2019). Cardiac microvascular endothelial enhancement of cardiomyocyte function is impaired by inflammation and restored by empagliflozin. JACC Basic Transl. Sci. 4, 575–591. doi: 10.1016/j.jacbts.2019.04.003
Kindo, M., Gerelli, S., Bouitbir, J., Hoang Minh, T., Charles, A. L., Mazzucotelli, J. P., et al. (2016). Left ventricular transmural gradient in mitochondrial respiration is associated with increased sub-endocardium nitric oxide and reactive oxygen species productions. Front. Physiol. 7:331. doi: 10.3389/fphys.2016.00331
Kondo, R. P., Dederko, D. A., Teutsch, C., Chrast, J., Catalucci, D., Chien, K. R., et al. (2006). Comparison of contraction and calcium handling between right and left ventricular myocytes from adult mouse heart: a role for repolarization waveform. J. Physiol. 571(Pt 1), 131–146. doi: 10.1113/jphysiol.2005.101428
Kuster, D. W. D. (2019). What grows together, goes together: assessing variability in cardiomyocyte function. J. Physiol. 597, 665–666. doi: 10.1113/jp277287
Luss, I., Boknik, P., Jones, L. R., Kirchhefer, U., Knapp, J., Linck, B., et al. (1999). Expression of cardiac calcium regulatory proteins in atrium v ventricle in different species. J. Mol. Cell Cardiol. 31, 1299–1314. doi: 10.1006/jmcc.1999.0962
McMahon, W. S., Mukherjee, R., Gillette, P. C., Crawford, F. A., and Spinale, F. G. (1996). Right and left ventricular geometry and myocyte contractile processes with dilated cardiomyopathy: myocyte growth and beta-adrenergic responsiveness. Cardiovasc. Res. 31, 314–323. doi: 10.1016/0008-6363(95)00212-x
Minajeva, A., Kaasik, A., Paju, K., Seppet, E., Lompre, A. M., Veksler, V., et al. (1997). Sarcoplasmic reticulum function in determining atrioventricular contractile differences in rat heart. Am. J. Physiol. 273, H2498–H2507.
Mitcheson, J. S., Hancox, J. C., and Levi, A. J. (1998). Cultured adult cardiac myocytes: future applications, culture methods, morphological and electrophysiological properties. Cardiovasc. Res. 39, 280–300. doi: 10.1016/s0008-6363(98)00128-x
Molina, C. E., Heijman, J., and Dobrev, D. (2016). Differences in left versus right ventricular electrophysiological properties in cardiac dysfunction and arrhythmogenesis. Arrhythm. Electrophysiol. Rev. 5, 14–19.
Naito, H., Arisawa, J., Harada, K., Yamagami, H., Kozuka, T., and Tamura, S. (1995). Assessment of right ventricular regional contraction and comparison with the left ventricle in normal humans: a cine magnetic resonance study with presaturation myocardial tagging. Br. Heart J. 74, 186–191. doi: 10.1136/hrt.74.2.186
Najafi, A., Sequeira, V., Helmes, M., Bollen, I. A., Goebel, M., Regan, J. A., et al. (2016). Selective phosphorylation of PKA targets after beta-adrenergic receptor stimulation impairs myofilament function in Mybpc3-targeted HCM mouse model. Cardiovasc. Res. 110, 200–214. doi: 10.1093/cvr/cvw026
Najafi, A., van de Locht, M., Schuldt, M., Schonleitner, P., van Willigenburg, M., Bollen, I., et al. (2019). End-diastolic force pre-activates cardiomyocytes and determines contractile force: role of titin and calcium. J. Physiol. 597, 4521–4531. doi: 10.1113/jp277985
Pela, G., Regolisti, G., Coghi, P., Cabassi, A., Basile, A., Cavatorta, A., et al. (2004). Effects of the reduction of preload on left and right ventricular myocardial velocities analyzed by dopplzer tissue echocardiography in healthy subjects. Eur. J. Echocardiogr. 5, 262–271. doi: 10.1016/j.euje.2003.10.001
Peng, Y., Yu, D., Gregorich, Z., Chen, X., Beyer, A. M., Gutterman, D. D., et al. (2013). In-depth proteomic analysis of human tropomyosin by top-down mass spectrometry. J. Muscle Res. Cell Motil. 34, 199–210. doi: 10.1007/s10974-013-9352-y
Previs, M. J., Michalek, A. J., and Warshaw, D. M. (2014). Molecular modulation of actomyosin function by cardiac myosin-binding protein C. Pflugers Arch. 466, 439–444. doi: 10.1007/s00424-013-1433-7
Rain, S., Handoko, M. L., Vonk Noordegraaf, A., Bogaard, H. J., van der Velden, J., and de Man, F. S. (2014). Pressure-overload-induced right heart failure. Pflugers Arch. 466, 1055–1063.
Reiser, P. J., and Kline, W. O. (1998). Electrophoretic separation and quantitation of cardiac myosin heavy chain isoforms in eight mammalian species. Am. J. Physiol. 274, H1048–H1053.
Sathish, V., Xu, A., Karmazyn, M., Sims, S. M., and Narayanan, N. (2006). Mechanistic basis of differences in Ca2+ -handling properties of sarcoplasmic reticulum in right and left ventricles of normal rat myocardium. Am. J. Physiol. Heart Circ. Physiol. 291, H88–H96.
Sequeira, V., Najafi, A., McConnell, M., Fowler, E. D., Bollen, I. A., Wust, R. C., et al. (2015). Synergistic role of ADP and Ca(2+) in diastolic myocardial stiffness. J. Physiol. 593, 3899–3916. doi: 10.1113/jp270354
Sequeira, V., Witjas-Paalberends, E. R., Kuster, D. W., and van der Velden, J. (2014). Cardiac myosin-binding protein C: hypertrophic cardiomyopathy mutations and structure-function relationships. Pflugers Arch. 466, 201–206. doi: 10.1007/s00424-013-1400-3
Sikkel, M. B., Francis, D. P., Howard, J., Gordon, F., Rowlands, C., Peters, N. S., et al. (2017). Hierarchical statistical techniques are necessary to draw reliable conclusions from analysis of isolated cardiomyocyte studies. Cardiovasc. Res. 113, 1743–1752. doi: 10.1093/cvr/cvx151
Tanaami, T., Ishida, H., Seguchi, H., Hirota, Y., Kadono, T., Genka, C., et al. (2005). Difference in propagation of Ca2+ release in atrial and ventricular myocytes. JPN J. Physiol. 55, 81–91. doi: 10.2170/jjphysiol.r2077
Tian, Q., Pahlavan, S., Oleinikow, K., Jung, J., Ruppenthal, S., Scholz, A., et al. (2012). Functional and morphological preservation of adult ventricular myocytes in culture by sub-micromolar cytochalasin D supplement. J. Mol. Cell Cardiol. 52, 113–124. doi: 10.1016/j.yjmcc.2011.09.001
Tsutsui, H., Urabe, Y., Mann, D. L., Tagawa, H., Carabello, B. A., Cooper, G. T., et al. (1993). Effects of chronic mitral regurgitation on diastolic function in isolated cardiocytes. Circ. Res. 72, 1110–1123. doi: 10.1161/01.res.72.5.1110
van Deel, E. D., Najafi, A., Fontoura, D., Valent, E., Goebel, M., Kardux, K., et al. (2017). In vitro model to study the effects of matrix stiffening on Ca(2+) handling and myofilament function in isolated adult rat cardiomyocytes. J. Physiol. 595, 4597–4610. doi: 10.1113/jp274460
Viero, C., Kraushaar, U., Ruppenthal, S., Kaestner, L., and Lipp, P. (2008). A primary culture system for sustained expression of a calcium sensor in preserved adult rat ventricular myocytes. Cell Calcium. 43, 59–71. doi: 10.1016/j.ceca.2007.04.001
Voelkel, N. F., Quaife, R. A., Leinwand, L. A., Barst, R. J., McGoon, M. D., Meldrum, D. R., et al. (2006). Right ventricular function and failure: report of a National Heart, Lung, and Blood Institute working group on cellular and molecular mechanisms of right heart failure. Circulation 114, 1883–1891. doi: 10.1161/circulationaha.106.632208
Walden, A. P., Dibb, K. M., and Trafford, A. W. (2009). Differences in intracellular calcium homeostasis between atrial and ventricular myocytes. J. Mol. Cell Cardiol. 46, 463–473. doi: 10.1016/j.yjmcc.2008.11.003
Zaitsev, A. V., Torres, N. S., Cawley, K. M., Sabry, A. D., Warren, J. S., and Warren, M. (2019). Conduction in the right and left ventricle is differentially regulated by protein kinases and phosphatases: implications for arrhythmogenesis. Am. J. Physiol. Heart Circ. Physiol. 316, H1507–H1527.
Keywords: cardiomyocyte, contractility, large-scale, atria, ventricles, regional differences
Citation: Nollet EE, Manders EM, Goebel M, Jansen V, Brockmann C, Osinga J, van der Velden J, Helmes M and Kuster DWD (2020) Large-Scale Contractility Measurements Reveal Large Atrioventricular and Subtle Interventricular Differences in Cultured Unloaded Rat Cardiomyocytes. Front. Physiol. 11:815. doi: 10.3389/fphys.2020.00815
Received: 20 December 2019; Accepted: 18 June 2020;
Published: 21 July 2020.
Edited by:
Henk Granzier, The University of Arizona, United StatesReviewed by:
Mei Methawasin, The University of Arizona, United StatesCopyright © 2020 Nollet, Manders, Goebel, Jansen, Brockmann, Osinga, van der Velden, Helmes and Kuster. This is an open-access article distributed under the terms of the Creative Commons Attribution License (CC BY). The use, distribution or reproduction in other forums is permitted, provided the original author(s) and the copyright owner(s) are credited and that the original publication in this journal is cited, in accordance with accepted academic practice. No use, distribution or reproduction is permitted which does not comply with these terms.
*Correspondence: Diederik W. D. Kuster, ZC5rdXN0ZXJAYW1zdGVyZGFtdW1jLm5s
Disclaimer: All claims expressed in this article are solely those of the authors and do not necessarily represent those of their affiliated organizations, or those of the publisher, the editors and the reviewers. Any product that may be evaluated in this article or claim that may be made by its manufacturer is not guaranteed or endorsed by the publisher.
Research integrity at Frontiers
Learn more about the work of our research integrity team to safeguard the quality of each article we publish.