- 1Research Institute of Applied Biology, Shanxi University, Taiyuan, China
- 2College of Life Science, Shanxi University, Taiyuan, China
- 3Modern Research Center for Traditional Chinese Medicine, Shanxi University, Taiyuan, China
- 4Interfaculty Institute of Cell Biology, University of Tübingen, Tübingen, Germany
- 5Université Côte d’Azur, CNRS, Inserm, iBV, Nice, France
Apolipophorins are carrier proteins that bind lipids and mediate their transport from tissue to tissue in animals. Apolipophorin I and II (apoLp-II/I) are the major apolipophorins in insects. The implication of apoLp-II/I in cuticle lipid-barrier formation in insects has not been addressed to date. In the present study, we investigated the function of apoLp-II/I in the migratory locust Locusta migratoria (LmapoLp-II/I). During the development of fifth instar nymphs, LmapoLp-II/I transcript levels increased until mid-instar, and then decreased gradually until molting to the adult stage. We found that LmapoLp-II/I was predominately expressed in the fat body and the integument including oenocytes and epidermal cells. Immunodetection experiments revealed that LmapoLp-I mainly localized in the cytoplasm of oenocytes and epidermal cells. Silencing of LmapoLp-II/I caused molting defects in nymphs. Importantly, RNA interference against LmapoLp-II/I resulted in a significant decrease in the content of cuticle surface lipids including alkanes and methyl alkanes. Cuticular permeability was significantly enhanced in these nymphs in Eosin Y penetration assays. By consequence, desiccation resistance and insecticide tolerance of dsLmapoLp-II/I-treated locusts were reduced. Taken together, our results indicate that LmapoLp-II/I is involved in the transport and deposition of surface-cuticular lipids that are crucial for maintaining normal cuticle barrier function in L. migratoria.
Introduction
Apolipoproteins are carrier proteins that bind lipids to form lipoprotein particles called lipophorins. In mammals, the major apolipoproteins (apo) are generally divided into four categories including apoA, apoB, apoC, and apoE (Mahley et al., 1984; Liu et al., 2019). These proteins are important components of the blood plasma, and mediate trafficking of various lipids between producing and consuming organs (Su and Peng, 2020). In insects, apolipoproteins were referred to as apolipophorins (apoLp) (Beenakkers et al., 1981). Apolipophorins are the main components of insect lipophorin particles. Lipophorin (Lp) was isolated and purified from the hemolymph of various insects such as Hyalophora cecropia, Philosamia cynthia, Periplaneta americana and Locusta migratoria (Thomas and Gilbert, 1968; Chino et al., 1969, 1981; Chino and Kitazawa, 1981), as a reusable shuttle, whose major role is transport of lipids including diacylglycerol, phospholipids, sterols, and hydrocarbons between tissues (Ryan and Van Der Horst, 2000).
The insect lipophorin is composed of two non-exchangeable apolipophorins, apolipophorin I (apoLp-I, ∼240 kDa) and apolipophorin II (apoLp-II, ∼80 kDa), and may additionally contain an exchangeable protein, apolipophorin III (apoLp-III, ∼18 kDa) (Ryan and Van Der Horst, 2000). ApoLp-I and apoLp-II are derived from a common precursor protein, apolipophorin II/I (apoLp-II/I) through post-translational cleavage (Weers et al., 1993). ApoLp-II/I is a homolog of the mammalian apoB and belongs to the same superfamily of large lipid transfer proteins (LLTP) (Van Der Horst and Rodenburg, 2010), while apoLp-III is homologous to mammalian apoE (Weers and Ryan, 2006).
Molecular work on apolipophorins was initiated in Manduca sexta and L. migratoria. The cDNA sequences of apoLp-III were first cloned from these two insect species (Cole et al., 1987; Kanost et al., 1988). The biochemical and molecular properties of apoLp-III and apoLp-II/I were explored in locusts (Van Antwerpen et al., 1988; Van Der Horst et al., 1991; Weers et al., 1993), which are involved in purification of apoLp-III, origination of apoLp-II and I and immunocytochemical localization of all of them. Subsequently, it was reported that locust apoLp-II/I was strongly expressed in pigmented glial cells of the lamina underlying the locust retina (Bogerd et al., 2000). It localized to the basement membrane suggesting an implication of apoLp-II/I in the transport of retinoids and/or fatty acids to the insect retina. Interestingly, it was shown that apoLp-III replaced apoLp-II/I in high density lipophorin (HDLp) to recruit more DAGs, resulting in the transformation of HDLp to low density lipophorin (LDLp) particles during the prolonged flight of locusts (Van Der Horst and Rodenburg, 2010). In addition, apoLp-III also participated at an innate immunity response to microorganism infection in many insects (Zdybicka-Barabas and Cytryńska, 2013). Compared to apoLp-III, there are fewer studies on the function of apoLp-II/I. In the tsetse fly Glossina morsitans morsitans, RNA interference (RNAi) against GmmapoLp-II/I resulted in decreased hemolymph lipid levels in females and delayed oocyte development (Benoit et al., 2011). In the fruit fly Drosophila melanogaster, DmapoLp-II/I was shown to be the major lipid transport protein in the larval hemolymph (Palm et al., 2012). It was expressed in the fat body, secreted to the hemolymph and tethered to the gut, where it took up lipids and delivered them to consuming tissues such as imaginal discs and the brain. Although the roles of apoLp as circulating components of the hemolymph are well established, their functions in lipid trafficking to the cuticle surface in insects are largely unknown.
Cuticular lipids of insects are usually found on the cuticle surface and are composed of hydrocarbons (saturated alkanes and unsaturated alkenes), fatty acids, fatty alcohols, and wax esters (Chapman, 2013). In insects, cuticular lipids provide protection against water loss and prevent penetration of xenobiotics (Howard and Blomquist, 2005; Balabanidou et al., 2016, 2019; Wen et al., 2016). As reported, Lp serves as a carrier for the transport of hydrocarbons from the site of synthesis (oenocyte) to the site of deposition (cuticle) in P. americana and L. migratoria (Katase and Chino, 1982, 1984). It is speculated that after synthesis in the oenocytes, the lipids bind to apoLps and release into the hemolymph, subsequently shuttle to the epidermis where they bind to lipoprotein receptors, and finally transported to the cuticle surface via pore canals (Chapman, 2013). However, our understanding of how apoLps affect the molecular processes of the deposition of surface-cuticular lipids as well as the cuticular lipids dependent cuticle barrier construction in insects remains fragmentary.
In the present study, we analyzed the function of apoLp-II/I in L. migratoria (LmapoLp-II/I) in an RNAi-based approach. We found that LmapoLp-II/I was essential for the molting of locusts. We also showed that the deposition of cuticular lipids was dependent on LmapoLp-II/I. Both the inward and the outward barrier functions of the cuticle were compromised in LmapoLp-II/I-knockdown animals. The migratory locust L. migratoria is an important worldwide agricultural pest and has strong adaptability to high temperature and desiccation. This work adds new knowledge to the current understanding of apoLp-II/I function in insects and identifies apoLp-II/I as a potential target for pest management.
Materials and Methods
Insect Rearing
The eggs of L. migratoria were purchased from Insect Protein Co., Ltd., Cangzhou City, China. They were incubated in a climate chamber at 30 ± 2°C and 40 ± 10% relative humidity (RH). After hatching, the first instar nymphs were transferred to a gauze cage and fed with fresh wheat sprouts in a 14:10-h light: dark photoperiod. Fresh wheat sprouts were added daily until the nymphs grew to the third instar, thereafter, fresh wheat was supplemented with wheat bran.
Bioinformatics Analysis of LmapoLp-II/I
The cDNA sequence of LmapoLp-II/I was obtained from the NCBI database. It is identical with one identified previously by Bogerd et al. (2000). The amino acid sequence of LmapoLp-II/I was translated from the cDNA sequence by the translation tools at ExPASy1. Protein domains were analyzed using SMART2. Protein domain composition was drawn using the Adobe Illustrator CS6 software (Adobe, United States). The molecular weight and isoelectric point were predicted using software at the EXPASY proteomics server3.
Construction of Phylogenetic Tree
A phylogenetic analysis of apoLp-II/I was performed by using the full-length amino acid sequences of representative apoLp-II/I proteins in different insects such as Blattella germanica, D. Melanogaster, and Zootermopsis nevadensis retrieved from GenBank database. As described previously (Zhao et al., 2018), the phylogenetic tree was constructed with the MEGA 5.1 software based on a multiple alignment of the amino acid sequences performed by Clustal W, and by adopting the neighbor-joining (NJ) method. A bootstrap analysis of 1000 replications was performed, and 50% cut-off bootstrap values were used to condense the tree. The GenBank accession numbers of apoLp-II/I from the different species used to construct the phylogenetic tree are listed in Supplementary Table S1.
Tissue-Specific and Developmental Expression Analysis
To analyze the tissue-specific and developmental expression patterns of LmapoLp-II/I, eleven tissues including integument, wing pads, foregut, gastric caeca, midgut, hindgut, Malpighian tubules, hemolymph, fat body, testis and ovary were dissected from 2-day-old fifth-instar nymphs, and the integument from 1-day-old fifth-instar nymphs (N5D1) to 8-day-old fifth-instar nymphs (N5D8) were collected. All samples were collected with four biological replicates, each with three nymphs. RNAiso Plus (TaKaRa, Japan) was used to extract total RNA of each samples according to the manufacturer’s recommendation. As described previously (Yu R. et al., 2016), one microgram of the total RNA was used to synthesize the first-strand cDNA using M-MLV reverse transcriptase (TaKaRa, Japan). Each cDNA sample was diluted 10-fold for reverse-transcription quantitative PCR (RT-qPCR) analysis. RT-qPCR was performed using SYBR® Premix ExTaqTM II (TaKaRa, Japan) on the ABI 7300 Real-Time PCR System (Applied Biosystems, United States). Two technical repetitions were made for each sample. Relative mRNA levels of target genes were calculated with the 2–Δ Ct method, and the target gene expression level was normalized to the expression of the internal marker gene EF1-α that exhibited the most stable expression at different stages and in different tissues (Yang et al., 2014).
RNA Interference
Specific primers for synthesizing double-stranded RNA (dsRNA) of LmapoLp-II/I (dsLmapoLp-II/I) and GFP (dsGFP) were designed at the E-RNAi web service4, which are listed in Supplementary Table S2. The dsLmapoLp-II/I and dsGFP were synthesized in vitro using T7 RiboMAXTM Express RNAi System (Promega, United States) as described previously (Zhao et al., 2018). Thirty nymphs from 2-day-old fourth-instar (N4D2) were randomly selected for dsRNA injection. Each nymph was injected with 10 μg dsRNA, and the control group was injected with the same amount of dsGFP. At least three repetitions were performed. The injected locusts were raised under the same feeding condition. To determine silencing efficiency, different phenotypes of dsLmapoLp-II/I- or dsGFP-injected nymphs were collected for RT-qPCR analysis.
Immunohistochemistry
To analyze the localization of LmapoLp-II/I protein, immunohistochemistry was performed as described previously (Yu R. et al., 2016). In brief, paraffin sections (5 microns thick) of the third abdominal integuments from 2-day-old fifth-instar nymphs after injection of dsGFP and dsLmapoLp-II/I were prepared. The polyclonal antibody against LmapoLp-I (LVAKRDIKSPDDYELK) was produced in mouse by the China Peptides Co., Ltd. (Shanghai, China). The mouse antiserum against LmapoLp-I (1:200) is used as a primary antibody at 4°C overnight to detect LmapoLp-I protein. After incubation with antibody, the sections were washed with PBS three times for 5 min each and then incubated with the Anti-mouse Alexa Fluor® 594 (1:200, Thermo Fisher Scientific, United States) as secondary antibody for 2 h at 37°C. After washing three times for 5 min each with PBS, sections were incubated with Fluorescent Brightener 28 (FB28, 1 mg/ml, Sigma) for 5 s to detect chitin. The sample sections were then washed with PBS three times followed by incubated with SYTOXTM Green Nucleic Acid Stain (Thermo Fisher Scientific, United States), which was diluted in PBS to a concentration of 0.025 μg/μl, for 15 min at room temperature to detect nucleus. The preimmune mouse serum was used as negative control. Images were captured using an LSM 880 confocal laser-scanning microscope (Zeiss, Germany) with excitation at 405 nm (FB28), 594 nm (Anti-mouse Alexa Fluor® 594) and 488 nm (SYTOXTM Green).
Cryo-Sectioning and BODIPY Staining
The 3rd–5th abdominal segments of nymphs with different phenotypes (before ecdysis, during ecdysis and after ecdysis) after dsLmapoLp-II/I or dsGFP injection in 2-day-old fourth-instar nymphs were prepared for cryo-sectioning, respectively. The samples were dehydrated in a PBS containing increasing sucrose concentrations of 10, 20, and 30% (w/v), and then were embedded in the optimal cutting temperature compound (SAKURA, United States). Sections of 20 microns were prepared on a cryostat microtome (Leica, CM1950) at −25°C and blotted on SuperFrost Plus adhesion slides. The samples were baked on slide drier at 45°C for 3–4 h for tissue adhesion. The Bodipy staining was performed according to Yu et al. (2017). In brief, the sections were washed with PBS three times for 5 min each and then stained with Bodipy505/515 diluted in dimethyl sulfoxide to a concentration of 0.025 μg/μl (GE Healthcare, Germany) by incubation at 37°C for 30–45 min. After washing with PBS three times for 5 min each, the sections were stained with DAPI for 5 min at room temperature to label the nuclei. Images were observed and captured using an LSM 880 confocal laser-scanning microscope (Zeiss, Germany).
Extraction and Quantification of Cuticular Hydrocarbons and Internal Hydrocarbons
After injection of dsLmapoLp-II/I or dsGFP in 2-day-old fourth-instar nymphs (N4D2), the nymphs at 48 h after dsRNA treatment (N4D4) and at 5 h after molting to the next stage (N5D1) were collected to quantify cuticular hydrocarbons (CHCs) and internal hydrocarbons (IHCs). Each nymph was immersed in 3 ml of n-hexane for 2 min before transferring the solvent to another chromatography bottle. This step was repeated twice. The three hexane extracts were combined and subjected to CHC purification and analysis by gas chromatography-mass spectrometry (GC-MS) on a TRACE 1310 coupled to an ISQ single-quadrupole MS detector with Xcalibur 2.2 software (Thermo Fisher Scientific, United States) as described previously (Yu Z. et al., 2016). The nymphs after the extraction of the CHCs were subjected to the extraction of IHCs. The wing pads, legs, guts and gonads were removed before extraction to exclude the effects of other factors, followed by extraction of IHCs with a mixture of n-hexane, methanol and water (2:1:1). After centrifugation, the n-hexane phase (supernatant) was recovered and purified as described previously (Yu Z. et al., 2016). Some of the alkanes were identified by their retention times compared to those of known standards (C7–C40 saturated alkanes Std, SUPELCO). The remaining alkanes were identified by Kovats retention index (I), which was calculated by an equation using retention time. These alkanes were quantified by their peak areas compared to that of the internal standard.
Eosin Y Staining
Nymphs with different phenotypes (before ecdysis, during ecdysis and after ecdysis) after dsLmapoLp-II/I or dsGFP injection in 2-day-old fourth-instar nymphs were collected for Eosin Y staining to investigate the effect of cuticular lipids on cuticle permeability. The nymphs were transferred to a 2 ml microcentrifuge tube containing 1.5 ml of dye solution [0.5% eosin (W/V, Sigma, a red water-soluble dye with a molecular mass of 691.86 Da)], incubated at 45°C for 30 min, and then washed three times with water as described previously (Yu et al., 2017). Finally, the locusts were photographed with an Epson perfection V700 photo using the Epson Scan software.
Desiccation Experiment
After injection of dsLmapoLp-II/I or dsGFP to 2-day-old fourth-instar nymphs (N4D2) for 24 h, nymphs were used for the desiccation experiment which were randomly divided into three groups and separately placed in a 50 ml bottle that covered with gauze and contained 2 ± 0.5 g of dry wheat bran at the bottom of the bottle for feeding. All test bottles were then placed in constant climate chamber (HPP110, Memmert, Germany) to control RH at 10 ± 2% for desiccation treatment and 50 ± 2% for control. The number of surviving insects was recorded every 12 h after desiccation treatment and the survival rate was calculated. The median lethal times (LT50) were obtained from a regression-probit value by using the SPSS program (SPSS Inc., Chicago, IL, United States). The corrected data were calculated by subtracting the effect at 50% RH from the effect at 10% RH. Overall survival was analyzed by the Kaplan–Meier method combined with the log-rank test. Three biological replicates were set up, and ten locusts were taken for each biological replicate.
Bioassays With Insecticides After RNAi
To determine the effect of lipid changes on insecticide susceptibility, three different insecticide classes, organophosphate (malathion), carbamate (carbaryl), and pyrethroid (deltamethrin) were applied with topical application method (Zhang et al., 2017, 2019). The three insecticides were separately dissolved in acetone, and 3 μL of the solution (malathion for 120 μg/mL, carbaryl for 70 μg/mL, and deltamethrin for 8 μg/mL) was dropped on the abdomen of the locusts at 24 h after dsLmapoLp-II/I or dsGFP injection into 2-day-old third-instar nymphs that have the appropriate size for toxicology experiments. Mortality was determined at 24 h after insecticide exposure. Mean and standard errors for each group were performed in five independent bioassays, each with 11–13 nymphs.
Expression Levels of Lipid Synthesis Related Genes After LmapoLp-II/I RNAi
To determine the effect of LmapoLp-II/I RNAi on lipid synthesis, the expression of lipid synthesis related genes, including acetyl-CoA Carboxylase (LmACC), fatty acid synthases (LmFAS1, LmFAS2, and LmFAS3), fatty acid elongases (LmELO1-7), and Cytochrome P450s (LmCYP4G62 and LmCYP4G102) identified in our lab, was determined in the integument at 24 h after injection of dsLmapoLp-II/I or dsGFP on 2-day-old fourth-instar nymphs by RT-qPCR as described above. The primers used for RT-qPCR are listed in Supplementary Table S2.
Statistical Analysis
The one-way analysis of variance test of the SPSS software (SPSS Inc., Chicago, IL, United States) was applied to analyze differences between different developmental stages and different tissues followed by the Tukey’s test. The Student’s t-test was carried out for silencing efficiency, CHCs quantity, LT50 and survival time with desiccation treatment, and mortality rate with insecticide treatment after RNAi. Asterisks indicate significant differences (∗P < 0.05; ∗∗P < 0.01; ∗∗∗P < 0.001).
Results
Bioinformatics and Phylogenetic Analysis of LmapoLp-II/I
The coding sequence of LmapoLp-II/I is 10,143 bp long and encodes a protein of 3380 amino acids containing a signal peptide, a lipoprotein N-terminal domain (LPD_N), a von Willebrand factor (vWF) type D (VWD) domain, a C8 domain and two domains (DUF1943 and DUF1081) with unknown function (Supplementary Figure S1A). This protein has a theoretical molecular weight of 371.8 kD and a pI of 6.18. Phylogenetic analysis using full-length amino acid sequences from other insect species revealed that LmapoLp-II/I clustered with the orthologs of Blattaria (Supplementary Figure S1B).
Tissue-Specific and Developmental Expression Analysis of LmapoLp-II/I
To assess the expression characteristics of LmapoLp-II/I, we analyzed the transcript levels of LmapoLp-II/I in different tissues and different developmental stages of fifth instar nymphs by RT-qPCR (Figure 1). LmapoLp-II/I transcripts were predominately detected in the integument and the fat body of 2 days old fifth instar nymphs (Figure 1A). Analysis of the LmapoLp-II/I temporal expression profile in the integument of fifth instar nymphs revealed that LmapoLp-II/I transcript levels increased in the first two days of this stage (N5D1-N5D2), reached a peak in 3 and 4 days old fifth instar nymphs (N5D3–N5D4), then decreased gradually until molting to the next stage (N5D5–N5D8) (Figure 1B).
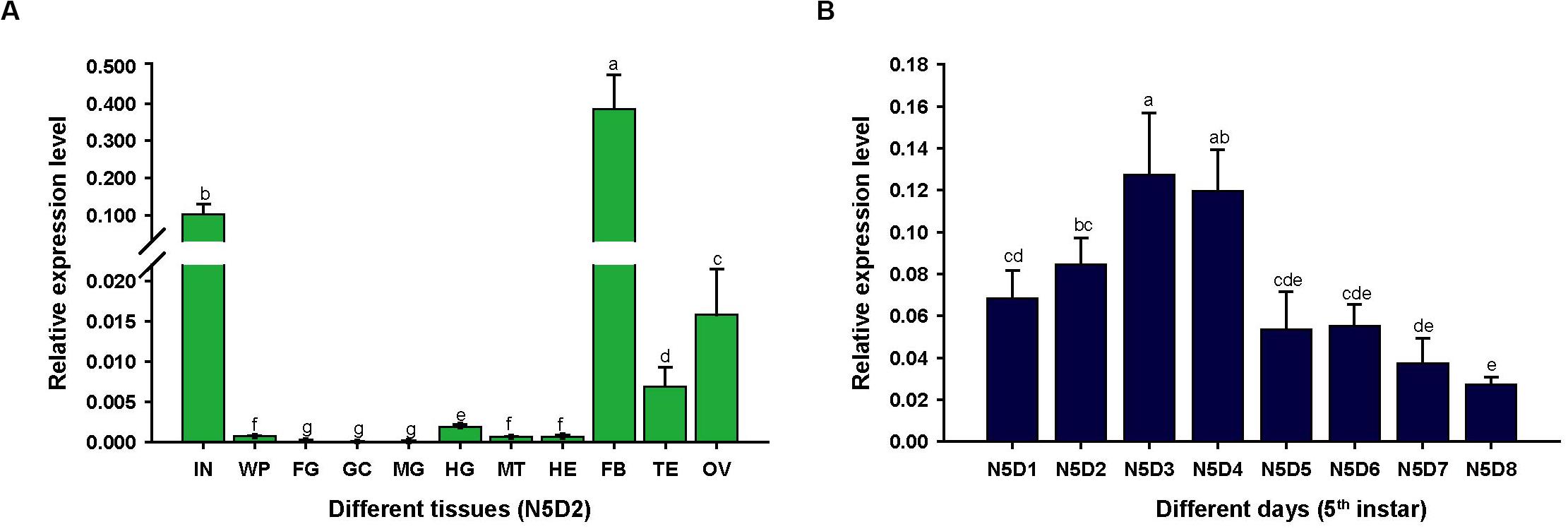
Figure 1. The relative expression levels of LmapoLp-II/I in different tissues and stages of the fifth instar nymphs in L. migratoria by RT-qPCR. (A) Expression of LmapoLp-II/I in different tissues of 2-day-old fifth-instar nymphs (N5D2) detected by RT-qPCR. The tissues are integument (IN), wing pads (WP), foregut (FG), gastric caeca (GC), midgut (MG), hindgut (HG), Malpighian tubules (MT), hemolymph (HE), fat body (FB), testis (TE), and ovary (OV). (B) Developmental expression profile of LmapoLp-II/I in the integument of N5D1-N5D8; EF1-α was used as the reference control. All data are reported as means ± SD of four independent biological replications. Different letters on the bars indicate significant difference among different samples (P < 0.05, Tukey’s HSD test; n = 4).
LmapoLp-I Localized to Epidermal Cells and Oenocytes
To scrutinize the biological function of LmapoLp-II/I, we sought to analyze its localization by immunodetection in the integument using a LmapoLp-I specific antibody (Figure 2). A positive signal was detected in the cytoplasm of epidermal cells and oenocytes in the dsGFP-injected control nymphs, whereas only a weak signal was detected in the dsLmapoLp-II/I-injected nymphs. This indicates that injection of dsLmapoLp-II/I suppressed LmapoLp-I protein accumulation. The localization of LmapoLp-I in the cytoplasm of epidermal cells and oenocytes further suggests that LmapoLp-I accumulated in those cells that express it.
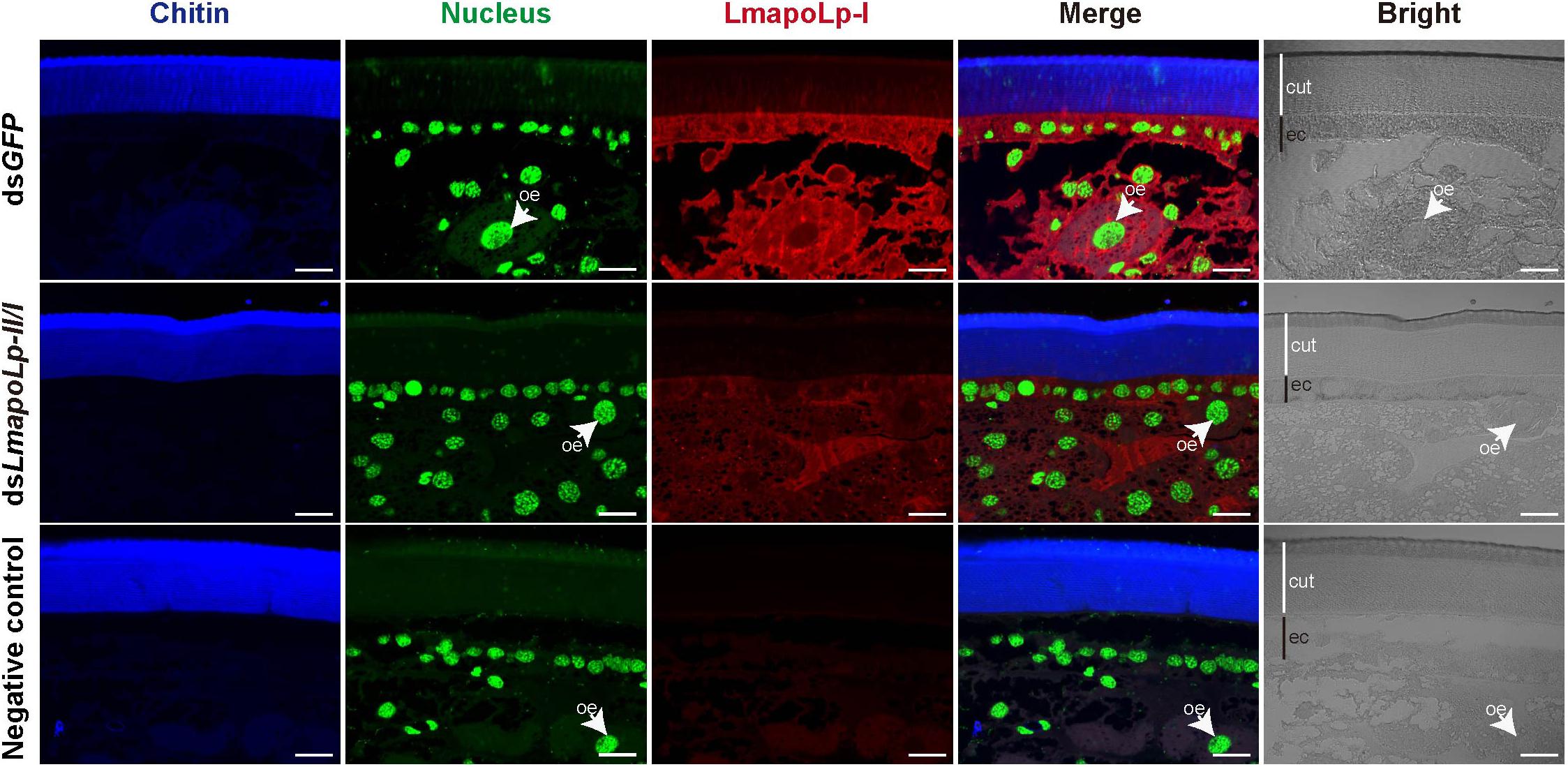
Figure 2. Localization of LmapoLp-I by immunohistochemistry. Chitin, nucleus and apolipophorins were detected by a blue, green, and red signal, respectively. A positive signal was detected in the epidermal cells and oenocytes of the dsGFP-injected nymphs. Whereas only a weak signal was detected in the dsLmapoLp-II/I-injected nymphs and almost no signal in the negative control. cut, cuticle; ec, epidermal cells; oe, oenocytes. Scale bar = 20 μm.
LmapoLp-II/I Is Essential for Locust Molting and Development
To investigate the role of LmapoLp-II/I during growth and development, dsRNAs directed against LmapoLp-II/I (dsLmapoLp-II/I) and GFP (dsGFP, control) transcripts were injected into fourth-instar nymphs (2-days-old, N4D2). Compared to the control, three different phenotypes at different stages (before ecdysis, during ecdysis, and after ecdysis) were observed in the dsLmapoLp-II/I-injected nymphs with a silencing efficiency of 45, 80, and 18%, respectively (Figure 3A). In dsGFP-injected control cohort, all nymphs molted normally to the next stage (fifth-instar nymphs), whereas 83% of the locusts injected with dsLmapoLp-II/I failed to molt to the next stage. These nymphs died either before ecdysis (30%) or during ecdysis (53%). The remaining 17% of the dsLmapoLp-II/I-injected nymphs molted to the next stage but died before molting to adults (Figure 3B). These results indicate that LmapoLp-II/I is essential for locust molting and development.
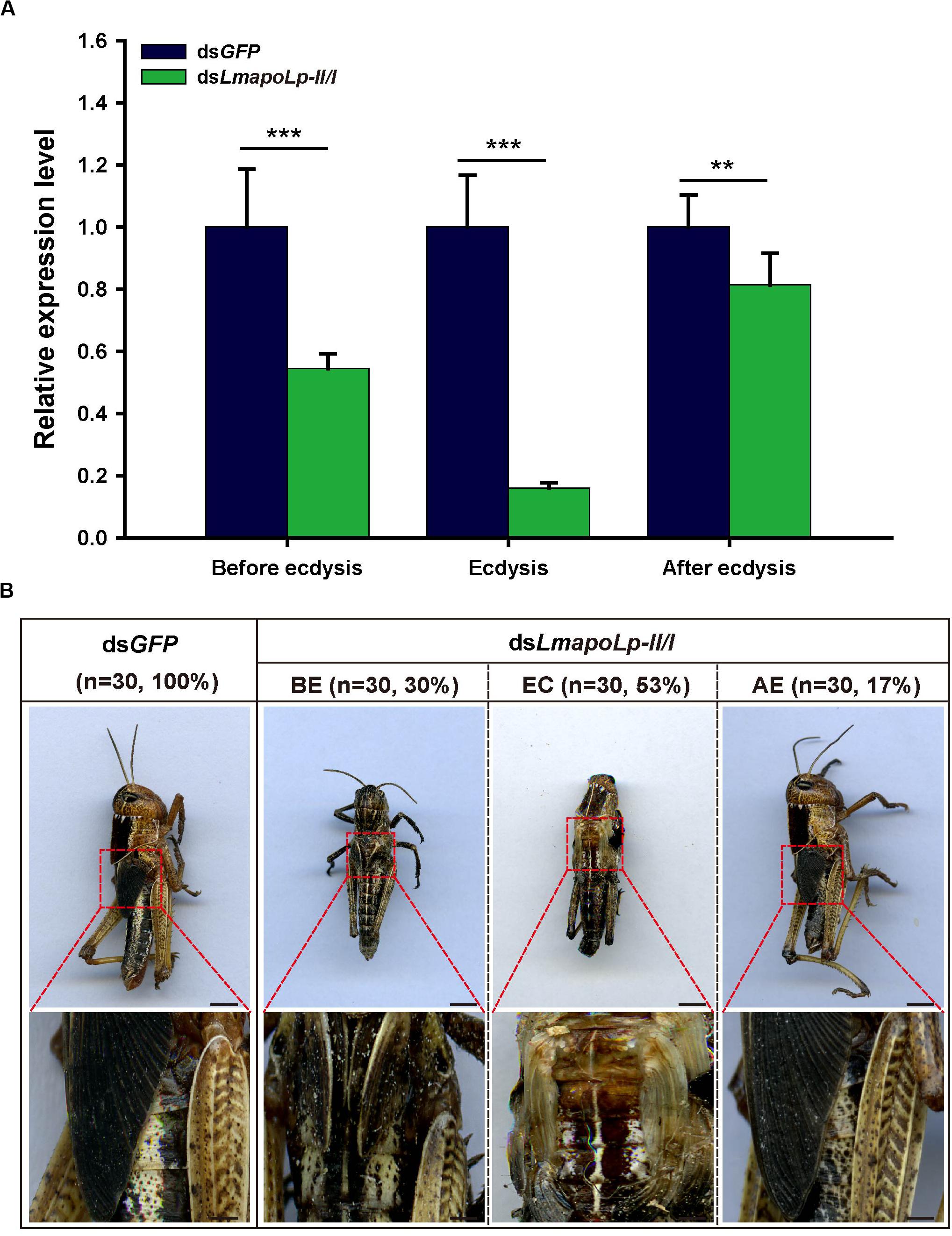
Figure 3. LmapoLp-II/I is required for locust molting. (A) Relative expression levels of LmapoLp-II/I in integuments after the dsGFP and dsLmapoLp-II/I injection was detected by RT-qPCR. EF1-α was used as the reference control. Data are shown as means ± SD from three independent experiments. Statistical significance was analyzed with Student’s t-test. Asterisks indicate significant differences (**P < 0.01; ***P < 0.001). (B) Phenotype of the nymphs after dsGFP and dsLmapoLp-II/I injection (n = 30). Nymphs injected with dsGFP were able to molt to fifth-instar normally. After injection of dsLmapoLp-II/I, 30% of nymphs died before ecdysis (BE), and 53% of nymphs died during ecdysis (EC). The remaining 17% of the dsLmapoLp-II/I-injected nymphs could molt to the fifth-instar nymphs, but died before molting to adults (after ecdysis, AE). Scale bar = 5 mm.
Effects of LmapoLp-II/I RNAi on Neutral Lipid Accumulation in the Cuticle
To investigate the effect of LmapoLp-II/I on lipid accumulation in the cuticle, we performed histochemical experiments using fluorescent Bodipy505/515 that detects neutral lipids. Compared with the nymphs injected with dsGFP (Figures 4A–C,A’–C’), the green fluorescence on the surface of the post-molting cuticle was significantly reduced in the nymphs treated with dsLmapoLp-II/I (Figures 4D–F,D’–F’). The intensity of the Bodipy signal was similar in the epidermis of dsGFP- and dsLmapoLp-II/I-injected animals before and during ecdysis (Figures 4A,B,D,E), while it was obviously reduced in the epidermis of dsLmapoLp-II/I-injected nymphs after ecdysis (Figures 4C,F). The signal was comparable in the oenocytes and the fat body between dsLmapoLp-II/I- and dsGFP-injected nymphs, indicating that silencing of LmapoLp-II/I only affects the distribution and content of neutral lipids on the cuticle surface, but has no effect on the accumulation of internal neutral lipids.
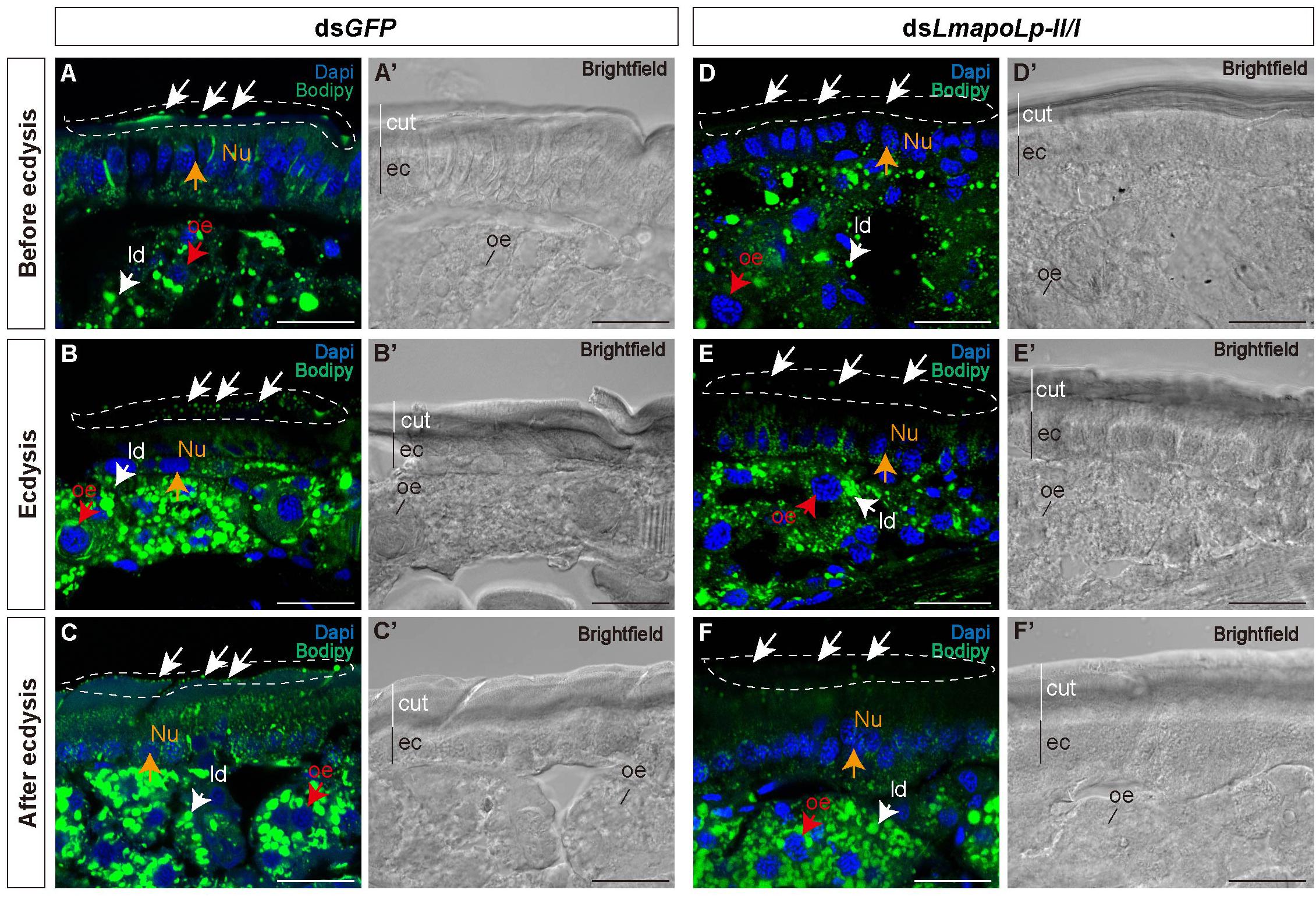
Figure 4. The effect of LmapoLp-II/I suppression on the content of neutral lipids in the cuticle. The cryosections (20 μm) of the abdominal cuticles from the nymphs after the injection of dsGFP (A’–C’) or dsLmapoLp-II/I (D’–F’) in 2-day-old fourth-instar nymphs were prepared and stained with Bodipy505/515 and DAPI to mark neutral lipid (green) and the nucleus (blue) of epidermal cells (ec), respectively. Compared to the dsGFP-injected group, the lipid droplets (ld) on the surface of the new cuticle (cut) were significantly reduced after injection with dsLmapoLp-II/I either before ecdysis (D), during ecdysis (E) or after ecdysis (F) (white dashed box). While the Bodipy signal intensity seemed to be similar in the epidermis of control (A,B) and dsLmapoLp-II/I (D,E) nymphs before ecdysis and during ecdysis, it was clearly weaker in the epidermis of dsLmapoLp-II/I treated nymphs (F) than in the epidermis of control nymphs (C) after ecdysis. White arrows point to lipid droplets. Red arrows mark the oenocytes (oe). Orange arrows point to the nucleus (Nu). Scale bar = 20 μm.
Analysis of Cuticular Hydrocarbons and Internal Hydrocarbons
The cuticular lipids of locusts mainly consist of long chain fatty acids, methyl and ethyl esters and hydrocarbons that account for about 60% of cuticular lipids (Cerkowniak et al., 2013). The main components of the locust CHCs are n-alkanes and methyl alkanes (Pedrini et al., 2007). To detect the effects of LmapoLp-II/I RNAi on cuticular lipid deposition, the CHCs and IHCs from dsGFP- and dsLmapoLp-II/I-injected nymphs were extracted before ecdysis and after ecdysis and analyzed by GC-MS. The results showed that, compared to the dsGFP-injected group, the total alkane content of CHC was significantly decreased by 24% in the dsLmapoLp-II/I-injected nymphs before ecdysis (Figure 5A), and by 84% after ecdysis (Figure 5C). The extent of reduction after ecdysis is much higher than that of before ecdysis. This reduction was systematically affecting almost all surface-cuticular alkanes from C25 to C33 and methyl alkanes from MeC25 to MeC31 (Supplementary Figure S2). However, there were no significant differences of alkane contents in the IHC extracts between the dsLmapoLp-II/I- and dsGFP-injected groups (Figures 5B,D and Supplementary Figure S3) except for two methyl alkanes, 3-MeC27 and 12-MeC30, that were slightly decreased in the dsLmapoLp-II/I-injected group after ecdysis (Supplementary Figure S3D). Because the CHC amounts depend on the lipid synthesis process, we monitored the expression levels of genes related to lipid synthesis after silencing of LmapoLp-II/I. The results showed that, compared to the control, the mRNA levels of LmFAS1-3, LmELO1, and LmELO7 were significantly decreased after deficiency of LmapoLp-II/I (Supplementary Figure S4). This effect may reduce the synthesis of hydrocarbons and subsequently result in reduction of CHCs in dsLmapoLp-II/I-injected animals. Taken together, we conclude that LmapoLp-II/I is involved in the deposition of CHCs, but has almost no effect on IHCs.
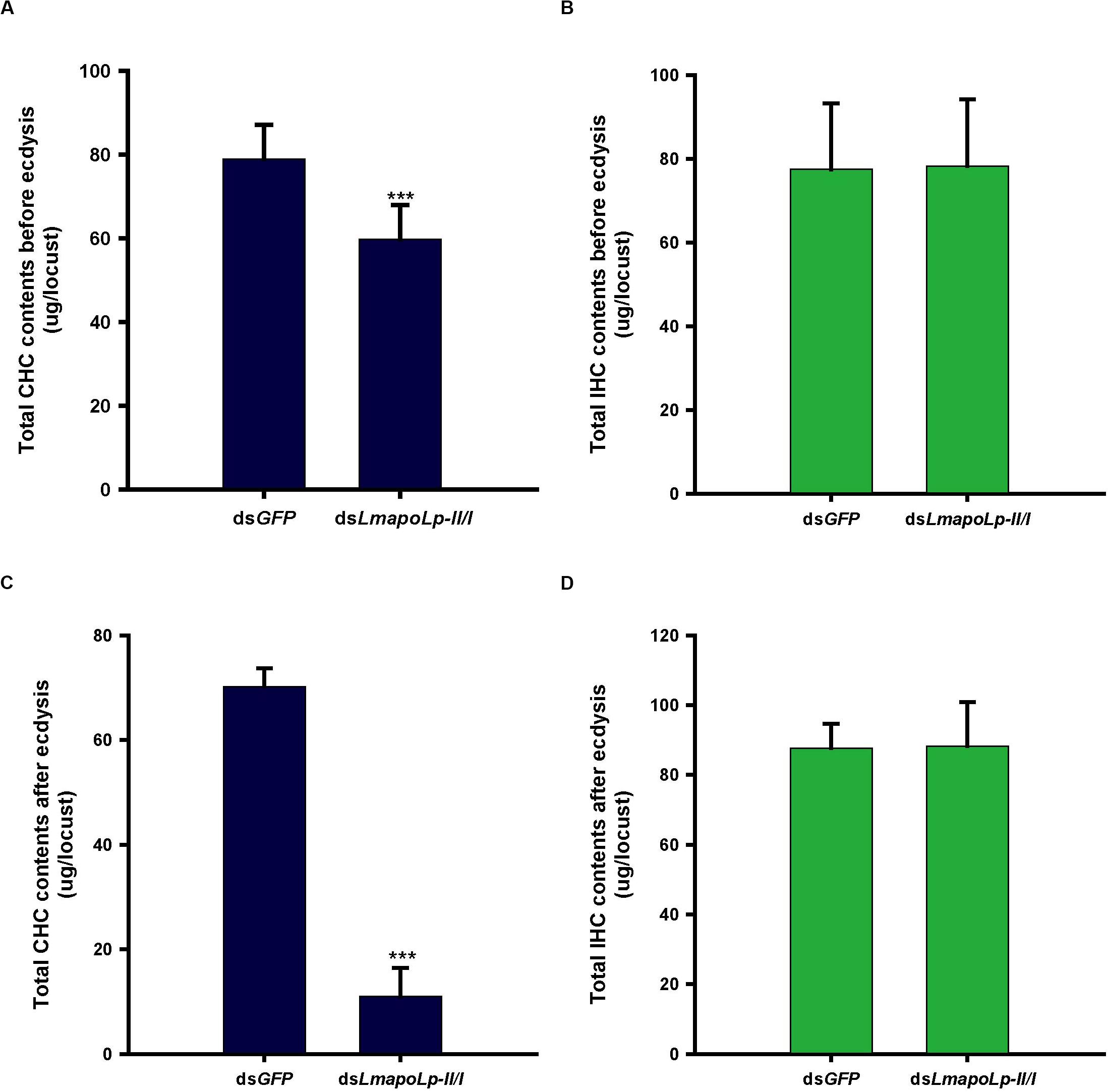
Figure 5. Effects of LmapoLp-II/I RNAi on the total CHC and IHC contents in L. migratoria. Total hydrocarbons contents extracted from the nymphs 48 h after dsGFP and dsLmapoLp-II/I injection (before ecdysis, A,B) and 5 h after ecdysis (C,D), respectively. In dsLmapoLp-II/I-injected group, the total CHCs before ecdysis (A) and after ecdysis (C) decreased by 24 and 84% respectively, compared to the dsGFP control group. However, there was no significant difference in IHCs contents between the dsLmapoLp-II/I- and dsGFP-injected group nymphs (B,D). The hydrocarbons are represented as micrograms (μg) per locust, and the data are shown as means ± SD. Statistical significance was analyzed with Student’s t-test. ***P < 0.001. N = 15–20 nymphs per treatment.
LmapoLp-II/I RNAi Affected the Sensitivity of Locusts to Desiccation
The water-retention capacity of the cuticle depends on the lipids deposited on its surface (Gibbs, 1998; Howard and Blomquist, 2005). In order to determine whether knockdown of LmapoLp-II/I affects the adaptability of L. migratoria to drought, a desiccation experiment was conducted with nymphs at 24 h after dsLmapoLp-II/I injection. The results showed that the survival rate of the nymphs injected with dsLmapoLp-II/I declined significantly compared to the dsGFP-treated control after desiccation treatment (10% RH) (P = 7.94e-7, Figure 6A and Supplementary Figure S5), the median lethal time (LT50 ± SEM) strongly decreased from 129.7 ± 2.9 h to 85.3 ± 10.8 h after injection of dsLmapoLp-II/I (Figure 6B). Similar results were obtained after correction of the survival rate by the subtraction of the values determined at 50% RH from those determined at 10% RH (Supplementary Figure S6). In addition, the survival time of dsLmapoLp-II/I-treated nymphs decreased by 33% compared with the dsGFP control nymphs (Figure 6C). However, there was no significant difference in LT50 between dsLmapoLp-II/I- and dsGFP-injected locusts at 50% RH, but the survival time decreased by 11% (Figures 6B,C). These results indicate that LmapoLp-II/I is able to modulate desiccation resistance of L. migratoria.
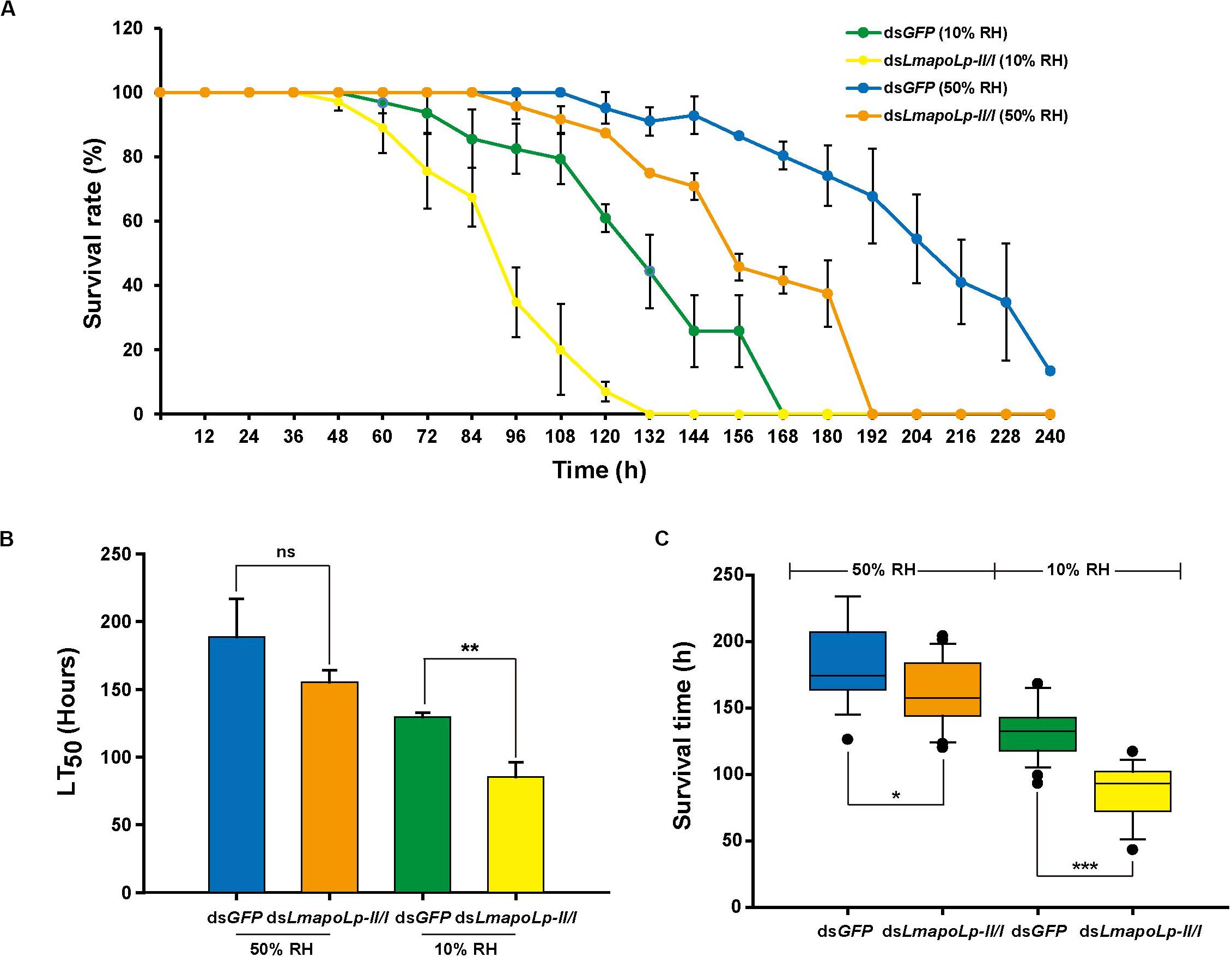
Figure 6. LmapoLp-II/I is essential for desiccation resistance of L. migratoria. (A) Temporal profile of survival rate of dsGFP- and dsLmapoLp-II/I-injected nymphs at different stages after desiccation treatment. Green line: dsGFP-injected nymphs at 10% relative humidity. Yellow line: dsLmapoLp-II/I-injected nymphs at 10% relative humidity. Blue line: dsGFP-injected nymphs at 50% relative humidity. Orange line: dsLmapoLp-II/I-injected nymphs at 50% relative humidity. (B) The median lethal time (LT50) calculated from the time-dependent survival rates. (C) Survival time of dsGFP- and dsLmapoLp-II/I-injected nymphs after desiccation treatment. All data are reported as means ± SD of three independent biological replications. Statistical significance was analyzed with Student’s t-test. Asterisks indicate significant differences (*P < 0.05; **P < 0.01; ***P < 0.001).
Suppression of LmapoLp-II/I Enhanced Cuticle Penetration and Increases Its Susceptibility to Insecticides
To investigate whether suppression of LmapoLp-II/I affects the inward barrier function of the cuticle, Eosin Y staining was applied to examine cuticle permeability. As shown in Figure 7, Eosin Y did not penetrate into the cuticle of dsGFP-injected nymphs after incubation for 30 min at 45°C at any stage of ecdysis (Figures 7A–C,A’–C’). In contrast, the dorsum and abdomen of dsLmapoLp-II/I-injected nymphs before ecdysis and during ecdysis were stained by Eosin Y under the same conditions (Figures 7D,E,D’,E’). Nymphs that did not die immediately after ecdysis were stained slightly by Eosin Y (Figures 7F,F’). Together, these data suggested that suppression of LmapoLp-II/I enhances the permeability of the cuticle.
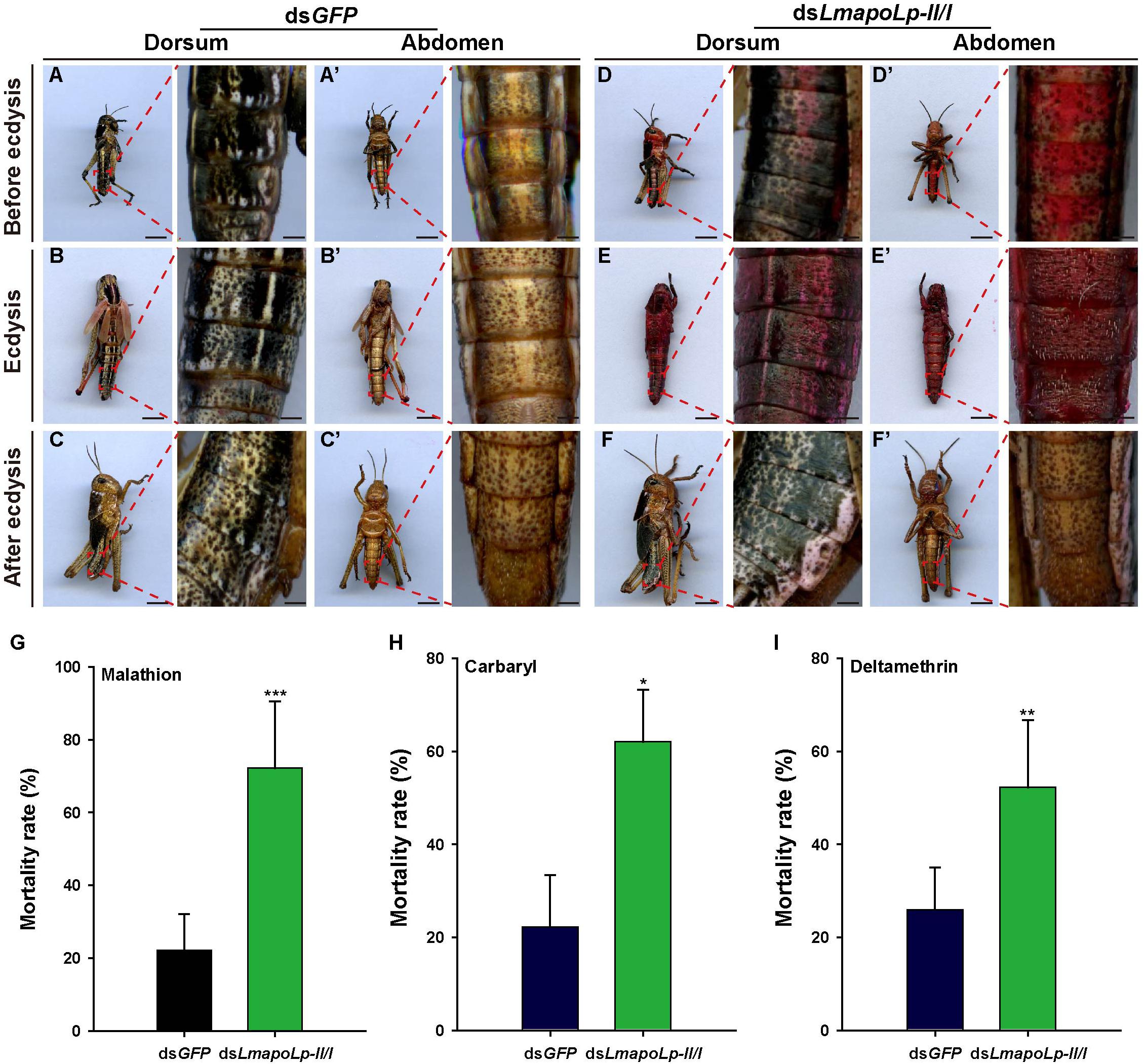
Figure 7. Suppression of LmapoLp-II/I enhances cuticle permeability and susceptibility to insecticides. Dorsal and ventral views of the nymphs with different phenotypes (before ecdysis, during ecdysis, and after ecdysis) after dsGFP and dsLmapoLp-II/I injection in 2-day-old fourth-instar nymphs are presented. Nymphs injected with dsGFP (A–C,A’–C’) were not stained by Eosin Y at 45°C, while both the dorsal and ventral sides of dsLmapoLp-II/I-injected (D–F,D’–F’) group nymphs were stained by Eosin Y. (G–I) Mortality rate of the third-instar nymphs injected with dsGFP (blue black column) or dsLmapoLp-II/I (green column) after insecticide exposure for 24 h. Asterisks indicate significant differences (*P < 0.05; **P < 0.01; ***P < 0.001). Data is represented as means ± SD of the five biological repeats. Statistical significance was analyzed with Student’s t-test.
To further investigate the inward barrier function of locusts with dysfunction of LmapoLp-II/I, three types of insecticides (malathion, carbaryl and deltamethrin) were applied to dsLmapoLp-II/I-treated third-instar nymphs. Our results showed that knockdown of LmapoLp-II/I significantly increased sensitivity of nymphs to the three insecticides with a mortality of 72.2, 62, and 52.2% compared to 22.2, 22.2, and 25.9% in the control group, respectively (Figures 7G–I). These results indicate that silencing of LmapoLp-II/I significantly increases the susceptibility of L. migratoria to insecticides by enhancing the permeability of the cuticle.
Discussion
ApoLps were characterized for their roles in lipid transportation between organs such as fat body, guts, wing discs, and oocytes (Benoit et al., 2011; Palm et al., 2012). They have also been implicated in innate immune responses (Zdybicka-Barabas and Cytryńska, 2013; Wen et al., 2017). ApoLp-II/I arise from a common precursor protein apoLp, which are invariably present in a 1:1 molar ratio (Weers et al., 1993). In the present study, by using the orthopteran insect L. migratoria as a model animal, we analyzed the characteristics and functions of LmapoLp-II/I in the deposition of lipids on the cuticle surface. To address different aspects of LmapoLp-II/I function, different nymphal stages were chosen depending the suitability of the size of the nymph for the respective experiment. Most experiments including immunohistochemistry, RT-qPCR analyses and mass spectrometry were performed on 4th and 5th instar nymphs whereas for insecticides tests third instar nymphs were used. We believe that the data are transferable between stages and will test this possibility in the next future.
LmapoLp-II/I Is Conserved Among Insect Species
Many apoLp-II/I proteins have been identified from different insect species including ApapoLp-II/I, BmapoLp-II/I, DmapoLp-II/I, and LmapoLp-II/I. They all contain LPD_N, DUF1943, DUF1081, and VWD domains (Bogerd et al., 2000; Hanada et al., 2011; Wen et al., 2017), indicating that apoLp-II/I proteins are conserved among insect species. As reported by Bogerd et al. (2000), the transcript of LmapoLp-II/I was mainly detected in the fat body and in the brain. In our work, besides in the fat body, we also found that LmapoLp-II/I was predominantly expressed in the integument, but showed low expression levels in the other tested tissues. Similarly, the transcript of apoLp-II/I in Antheraea pernyi was mainly expressed in the fat body and the integument, but weakly in other tissues (Wen et al., 2017). Thus, the fat body and the integument might be the main tissues expressing apoLp-II/I in insects. In addition, using a LmapoLp-I antibody, we found that the LmapoLp-I protein is located in epidermal cells and oenocytes. This finding suggests that LmapoLp-II/I is a carrier between oenocytes and epidermal cells in the integument. It is well possible that the actual sites of apoLp-II/I expression in the integument are not the epidermal cells themselves but the associated oenocytes that are hepatocyte-like cells involved in lipid production and circulation in insects (Schal et al., 1998; Gutierrez et al., 2007; Makki et al., 2014). Thus, both the expression pattern of apoLp-II/I and its encoded protein are conserved among insect species.
LmapoLp-II/I Is Required for Deposition of Cuticular Lipids
The transport of cuticular lipids in insects mainly occurs during molting, and a number of lipids are secreted by oenocytes and transported to the cuticle for deposition immediately after formation of the new cuticle (Qiu et al., 2012; Flaven-Pouchon et al., 2016). The expression profiles of LmapoLp-II/I during developmental stages showed that the transcript levels of LmapoLp-II/I increased after ecdysis and reached a peak on day 3 and 4 before decreasing gradually. In the fruit fly D. melanogaster, initial studies have shown that apoLp-II/I-RNAi caused accumulation of neutral lipids in the gut and reduction of lipid droplets in fat body cells and imaginal discs, suggesting that apoLp-II/I is required for the delivery of lipids from the gut to other tissues (Panakova et al., 2005). Subsequent studies confirmed that apoLp-II/I is an important lipid carrier in the hemolymph, and that apoLp-II/I produced in the fat body is recruited to the gut to mobilize lipids taken up by the midgut (Palm et al., 2012). By Bodipy staining, we showed that the amounts of surface lipids in the abdomen of the dsLmapoLp-II/I-injected nymphs were significantly reduced compared to those of dsGFP-injected nymphs. We confirmed this observation by GC-MS analyses of CHC amounts including normal and methyl-branched alkanes in control and dsLmapoLp-II/I-injected nymphs. The reduction of CHC amounts before ecdysis was modest, conceivably because the bulk of hydrocarbons had been synthesized and transported to the cuticle before dsLmapoLp-II/I injection. By contrast, the reduction of CHC amounts after ecdysis was strong in dsLmapoLp-II/I-treated nymphs. Obviously, LmapoLp-II/I is needed for the deposition of large amounts of CHCs on the surface of the newly formed cuticle. Incidentally, the process of CHC reconstitution after ecdysis involving LmapoLp-II/I seems to be rather fast in the locust. Indeed, the amounts of CHCs before and immediately after ecdysis are similar. By contrast, in the fruit fly D. melanogaster, the CHC levels reach their peak several days after eclosion (Ferveur et al., 1997; Savarit and Ferveur, 2002). An interesting observation was that the levels of most of the IHCs tested were stable in the dsLmapoLp-II/I-injected group before and after ecdysis. The exceptions were the levels of 3-MeC27 and 12-MeC30 that decreased by 27 and 35%, respectively, after ecdysis. Overall, we reckon that a possible explanation for the stability of the levels of most of the IHCs is feedback regulation at the transcriptional level. The concentrations of hydrocarbons inside the cell presumably control the abundance of transcripts of enzymes involved in hydrocarbon production. Indeed, this kind of feedback regulation is recurrent in lipid metabolism (Jacquemyn et al., 2017; Tsai et al., 2019). This explanation obviously does not apply to the levels of 3-MeC27 and 12-MeC30. Identification and characterization of the enzymes responsible for 3-MeC27 and 12-MeC30 production should shed light on this problem. In summary, our data indicate that LmapoLp-II/I expressed in the fat body and the integument is needed for CHC deposition on the surface of the locust cuticle during molting.
LmapoLp-II/I Is Essential for the Barrier Function of the Cuticle
In this study, after silencing of LmapoLp-II/I by dsRNA injection, about 83% of the nymphs failed to molt and died. As a protein involved in the fundamental process of lipid trafficking, there may be many reasons of dsLmapoLp-II/I-induced lethality. Conceivably, a major reason for dsLmapoLp-II/I-induced lethality is a weakened cuticle barrier function due to reduction of surface lipids. It is commonly agreed that surface lipids including CHCs are crucial components of the cuticle barrier against desiccation and xenobiotics penetration (Howard and Blomquist, 2005; Balabanidou et al., 2016, 2019; Wen et al., 2016). Indeed, we found that dsLmapoLp-II/I-injected nymphs are sensitive to low air humidity, insecticide application and Eosin Y penetration. Thus, LmapoLp-II/I contributes to the construction and function of both inward and outward cuticular barriers.
Our findings, however, suggest an unexpected complexity in barrier formation in the locust. Just after ecdysis, CHC levels strongly dropped in dsLmapoLp-II/I-injected nymphs, whereas, contra-intuitively, Eosin Y penetration was weaker at this stage compared to the situation before ecdysis. In a simple view, there is, hence, a contradiction to the assumption that CHCs constitute an inward barrier. We speculate that compensatory mechanisms in survived nymphs alleviate failure in CHC deposition. A possible compensatory mechanisms is melanization that according to the melanism-desiccation hypothesis by Kalmus (1941) prevents desiccation. In a recent work, it was shown that the melanization degree of the fruit fly cuticle correlates with CHC amounts suggesting the melanization and CHC production pathways interact (Massey et al., 2019). In line with this argument, CHC reduction in dsLmapoLp-II/I-injected nymphs may induce cuticle melanization and by consequence increase cuticle barrier function. To substantiate this possibility, the melanization degree of the cuticle of dsLmapoLp-II/I-injected nymphs should be determined.
With this work, we add LmapoLp-II/I to a row or cascade of proteins and enzymes that have been demonstrated to be needed for CHC production, transport and deposition in the locust. In oenocytes or the fat body, fatty acids are decarbonylated to produce CHCs by LmCYP4G102 (Yu Z. et al., 2016). Subsequently, LmapoLp-II/I delivers these CHCs to the epidermal cells where they are transported to the surface probably by the ABC transporter ABCH-9C (Yu et al., 2017). Taking work done in other species into account, we are close to a comprehensive view on these processes in insects. The fatty acid synthesis initiating enzymes acetyl-CoA Carboxylase (ACC) and fatty acid synthase 3 (FASN3) and the lipophorin receptors LpR1 and LpR2 in D. melanogaster, for instance, have been reported to be essential for CHC production or trafficking and against water-loss (Wicker-Thomas et al., 2015). In addition, in the brown plant hopper Nilaparvata lugens, some fatty acid elongases (ELOs) and reductases (FARs) have been shown to be crucial for correct CHC amounts and desiccation resistance in this rice pest (Li et al., 2019, 2020). Taken together, these proteins needed for CHC production or trafficking are crucial for water-loss and insecticide-penetration, thereby modulate the ecological adaptability of insects. In conclusion, the susceptibility of this pathway to perturbation, assigns its proteins and enzymes as perfect targets for intelligent pest control in the future.
Data Availability Statement
The datasets presented in this study can be found in online repositories. The names of the repository/repositories and accession number(s) can be found in the article/Supplementary Material.
Ethics Statement
Ethical review and approval was not required for the animal study because no vertebrates and higher invertebrates studies and no potentially identifiable human images or data are presented in this study. The locusts used in this study are lower invertebrates and are not regulated animals (i.e., all live vertebrates and higher invertebrates) which are exempt from ethics approval.
Author Contributions
JZZ conceived and designed the study. YZ, HG, and YY performed the experiments. YZ, WL, XZ, ZY, and BM analyzed the data. YZ, WL, and XZ drafted the manuscript and prepared the figures. All authors interpreted the results, edited and revised the manuscript, and approved the final version of the manuscript.
Funding
This work was supported by the NSFC-DFG (Grant No. 31761133021), the National Natural Science Foundation of China (Grant Nos. 31730074, 31672364, 31970469, and 31702067), the Program for Top Young Academic Leaders of Higher Learning Institutions of Shanxi (TYAL) 2017, Key Research and Development Program of Shanxi Province (201803D221004-5), Scientific and Technological Innovation Programs of Higher Education Institutions in Shanxi (2019L0033 and 2017104), and the Natural Science Foundation of Shanxi Province, China (No. 201601D202057). BM’s contribution was supported by the German Research Foundation (DFG grant MO1714/9-1).
Conflict of Interest
The authors declare that the research was conducted in the absence of any commercial or financial relationships that could be construed as a potential conflict of interest.
Supplementary Material
The Supplementary Material for this article can be found online at: https://www.frontiersin.org/articles/10.3389/fphys.2020.00790/full#supplementary-material
Footnotes
- ^ http://www.expasy.org/tools/dna.html
- ^ http://smart.embl.de/
- ^ http://www.expasy.org
- ^ http://www.dkfz.de/signaling/e-rnai3//
References
Balabanidou, V., Kampouraki, A., Maclean, M., Blomquist, G. J., Tittiger, C., Juárez, M. P., et al. (2016). Cytochrome P450 associated with insecticide resistance catalyzes cuticular hydrocarbon production in Anopheles gambiae. Proc. Natl. Acad. Sci. U.S.A. 113, 9268–9273. doi: 10.1073/pnas.1608295113
Balabanidou, V., Kefi, M., Aivaliotis, M., Koidou, V., Girotti, J. R., Mijailovsky, S. J., et al. (2019). Mosquitoes cloak their legs to resist insecticides. Proc. Biol. Sci. 286:20191091. doi: 10.1098/rspb.2019.1091
Beenakkers, A. M. T., Chino, H., and Law, J. H. (1981). Lipophorin nomenclature. Insect Biochem. 18, 1–2. doi: 10.1016/0020-1790(88)90029-7
Benoit, J. B., Yang, G., Krause, T. B., Patrick, K. R., Aksoy, S., and Attardo, G. M. (2011). Lipophorin acts as a shuttle of lipids to the milk gland during tsetse fly pregnancy. J. Insect Physiol. 57, 1553–1561. doi: 10.1016/j.jinsphys.2011.08.009
Bogerd, J., Babin, P. J., Kooiman, F. P., André, M., Ballagny, C., Van Marrewijk, W. J., et al. (2000). Molecular characterization and gene expression in the eye of the apolipophorin II/I precursor from Locusta migratoria. J. Comp. Neurol. 427, 546–558.
Cerkowniak, M., Puckowski, A., Stepnowski, P., and Golebiowski, M. (2013). The use of chromatographic techniques for the separation and the identification of insect lipids. J. Chromatogr. B Analyt. Technol. Biomed. Life Sci. 937, 67–78. doi: 10.1016/j.jchromb.2013.08.023
Chapman, R. F. (2013). The Insects: Structure and Function, 5th Edn. New York, NY: Cambridge University Press, 463–500.
Chino, H., Katase, H., Downer, R. G., and Takahashi, K. (1981). Diacylglycerol-carrying lipoprotein of hemolymph of the American cockroach purification, characterization, and function. J. Lipid Res. 22, 7–15.
Chino, H., and Kitazawa, K. (1981). Diacylglycerol-carrying lipoprotein of hemolymph of the locust and some insects. J. Lipid Res. 22, 1042–1052.
Chino, H., Murakami, S., and Harashima, K. (1969). Diglyceride-carrying lipoproteins in insect hemolymph isolation, purification and properties. Biochim. Biophys. Acta 176, 1–26. doi: 10.1016/0005-2760(69)90068-x
Cole, K. D., Fernando-Warnakulasuriya, G. P., Boguski, M. S., Freeman, M., Gordon, J. I., Clark, W. A., et al. (1987). Primary structure and comparative sequence analysis of an insect apolipoprotein. Apolipophorin-III from Manduca sexta. J. Biol. Chem. 262, 11794–11800.
Ferveur, J. F., Savarit, F., O’kane, C. J., Sureau, G., Greenspan, R. J., and Jallon, J. M. (1997). Genetic feminization of pheromones and its behavioral consequences in Drosophila males. Science 276, 1555–1558. doi: 10.1126/science.276.5318.1555
Flaven-Pouchon, J., Farine, J. P., Ewer, J., and Ferveur, J. F. (2016). Regulation of cuticular hydrocarbon profile maturation by Drosophila tanning hormone, bursicon, and its interaction with desaturase activity. Insect Biochem. Mol. Biol. 79, 87–96. doi: 10.1016/j.ibmb.2016.10.007
Gibbs, A. G. (1998). Water-proofing properties of cuticular lipids. Am. Zool. 38, 471–482. doi: 10.1093/icb/38.3.471
Gutierrez, E., Wiggins, D., Fielding, B., and Gould, A. P. (2007). Specialized hepatocyte-like cells regulate Drosophila lipid metabolism. Nature 445, 275–280. doi: 10.1038/nature05382
Hanada, Y., Sekimizu, K., and Kaito, C. (2011). Silkworm apolipophorin protein inhibits Staphylococcus aureus virulence. J. Biol. Chem. 286, 39360–39369. doi: 10.1074/jbc.M111.278416
Howard, R. W., and Blomquist, G. J. (2005). Ecological, behavioral, and biochemical aspects of insect hydrocarbons. Annu. Rev. Entomol. 50, 371–393. doi: 10.1146/annurev.ento.50.071803.130359
Jacquemyn, J., Cascalho, A., and Goodchild, R. E. (2017). The ins and outs of endoplasmic reticulum-controlled lipid biosynthesis. EMBO Rep. 18, 1905–1921. doi: 10.15252/embr.201643426
Kalmus, H. (1941). The resistance to desiccation of Drosophila mutants affecting body colour. Proc. R. Soc. Lond. B Biol. Sci. 130, 185–201. doi: 10.1098/rspb.1941.0011
Kanost, M. R., Boguski, M. S., Freeman, M., Gordon, J. I., Wyatt, G. R., and Wells, M. A. (1988). Primary structure of apolipophorin-III from the migratory locust, Locusta migratoria. Potential amphipathic structures and molecular evolution of an insect apolipoprotein. J. Biol. Chem. 263, 10568–10573.
Katase, H., and Chino, H. (1982). Transport of hydrocarbons by the lipophorin of incect hemolymph. Biochim. Biophys. Acta 710, 341–348. doi: 10.1016/0005-2760(82)90117-5
Katase, H., and Chino, H. (1984). Transport of hydrocarbons by haemolymph lipophorin in Locusta migratoria. Insect Biochem. 14, 1–6. doi: 10.1016/0020-1790(84)90076-3
Li, D. T., Chen, X., Wang, X. Q., Moussian, B., and Zhang, C. X. (2019). The fatty acid elongase gene family in the brown planthopper, Nilaparvata lugens. Insect Biochem. Mol. Biol. 108, 32–43. doi: 10.1016/j.ibmb.2019.03.005
Li, D. T., Dai, Y. T., Chen, X., Wang, X. Q., Li, Z. D., Moussian, B., et al. (2020). Ten fatty acyl-CoA reductase family genes were essential for the survival of the destructive rice pest, Nilaparvata lugens. Pest Manag. Sci. 76, 2304–2315. doi: 10.1002/ps.5765
Liu, J. Q., Li, W. X., Zheng, J. J., Tian, Q. N., Huang, J. F., and Dai, S. X. (2019). Gain and loss events in the evolution of the apolipoprotein family in vertebrata. BMC Evol. Biol. 19:209. doi: 10.1186/s12862-019-1519-8
Mahley, R. W., Innerarity, T. L., Rall, S. C., and Weisgraber, K. H. (1984). Plasma lipoproteins: apolipoprotein structure and function. J. Lipid Res. 25, 1277–1294.
Makki, R., Cinnamon, E., and Gould, A. P. (2014). The development and functions of oenocytes. Annu. Rev. Entomol. 59, 405–425. doi: 10.1146/annurev-ento-011613-162056
Massey, J. H., Akiyama, N., Bien, T., Dreisewerd, K., Wittkopp, P. J., Yew, J. Y., et al. (2019). Pleiotropic Effects of ebony and tan on pigmentation and cuticular hydrocarbon composition in Drosophila melanogaster. Front. Physiol. 10:518. doi: 10.3389/fphys.2019.00518
Palm, W., Sampaio, J. L., Brankatschk, M., Carvalho, M., Mahmoud, A., Shevchenko, A., et al. (2012). Lipoproteins in Drosophila melanogaster—assembly, function, and influence on tissue lipid composition. PLoS Genet. 8:e1002828. doi: 10.1371/journal.pgen.1002828
Panakova, D., Sprong, H., Marois, E., Thiele, C., and Eaton, S. (2005). Lipoprotein particles are required for Hedgehog and Wingless signalling. Nature 435, 58–65. doi: 10.1038/nature03504
Pedrini, N., Crespo, R., and Juárez, M. P. (2007). Biochemistry of insect epicuticle degradation by entomopathogenic fungi. Comp. Biochem. Physiol. C Toxicol. Pharmacol. 146, 124–137. doi: 10.1016/j.cbpc.2006.08.003
Qiu, Y., Tittiger, C., Wicker-Thomas, C., Le Goff, G., Young, S., Wajnberg, E., et al. (2012). An insect-specific P450 oxidative decarbonylase for cuticular hydrocarbon biosynthesis. Proc. Natl. Acad. Sci. U.S.A. 109, 14858–14863. doi: 10.1073/pnas.1208650109
Ryan, R. O., and Van Der Horst, D. J. (2000). Lipid transport biochemistry and its role in energy production. Annu. Rev. Entomol. 45, 233–260. doi: 10.1146/annurev.ento.45.1.233
Savarit, F., and Ferveur, J. F. (2002). Temperature affects the ontogeny of sexually dimorphic cuticular hydrocarbons in Drosophila melanogaster. J. Exp. Biol. 205, 3241–3249.
Schal, C., Sevala, V. L., Young, H. P., and Bachmann, J. A. S. (1998). Sites of synthesis and transport pathways of insect hydrocarbons: cuticle and ovary as target tissues. Am. Zool. 38, 382–393. doi: 10.1093/icb/38.2.382
Su, X., and Peng, D. (2020). The exchangeable apolipoproteins in lipid metabolism and obesity. Clin. Chim. Acta 503, 128–135. doi: 10.1016/j.cca.2020.01.015
Thomas, K. K., and Gilbert, L. I. (1968). Isolation and characterization of the hemolymph lipoproteins of the American silkmoth, Hyalophora cecropia. Arch. Biochem. Biophys. 127, 512–521. doi: 10.1016/0003-9861(68)90256-7
Tsai, J. W., Kostyleva, R., Chen, P. L., Rivas-Serna, I. M., Clandinin, M. T., Meinertzhagen, I. A., et al. (2019). Transcriptional feedback links lipid synthesis to synaptic vesicle pools in drosophila photoreceptors. Neuron 101, 721–737.e4. doi: 10.1016/j.neuron.2019.01.015
Van Antwerpen, R., Linnemans, W. A., Van Der Horst, D. J., and Beenakkers, A. M. (1988). Immunocytochemical localization of lipophorins in the flight muscles of the migratory locust (Locusta migratoria) at rest and during flight. Cell Tissue Res. 252, 661–668. doi: 10.1007/bf00216654
Van Der Horst, D. J., and Rodenburg, K. W. (2010). Lipoprotein assembly and function in an evolutionary perspective. Biomol. Concepts 1, 165–183. doi: 10.1515/bmc.2010.012
Van Der Horst, D. J., Van Doorn, J. M., Voshol, H., Kanost, M. R., Ziegler, R., and Beenakkers, A. M. (1991). Different isoforms of an apoprotein (apolipophorin III) associate with lipoproteins in Locusta migratoria. Eur. J. Biochem. 196, 509–517. doi: 10.1111/j.1432-1033.1991.tb15843.x
Weers, P. M., and Ryan, R. O. (2006). Apolipophorin III: role model apolipoprotein. Insect Biochem. Mol. Biol. 36, 231–240. doi: 10.1016/j.ibmb.2006.01.001
Weers, P. M., Van Marrewijk, W. J., Beenakkers, A. M., and Van Der Horst, D. J. (1993). Biosynthesis of locust lipophorin. Apolipophorins I and II originate from a common precursor. J. Biol. Chem. 268, 4300–4303.
Wen, D., Luo, H., Li, T., Wu, C., Zhang, J., Wang, X., et al. (2017). Cloning and characterization of an insect apolipoprotein (apolipophorin-II/I) involved in the host immune response of Antheraea pernyi. Dev. Comp. Immunol. 77, 221–228. doi: 10.1016/j.dci.2017.08.010
Wen, D., Wang, X., Shang, L., Huang, Y., Li, T., Wu, C., et al. (2016). Involvement of a versatile pattern recognition receptor, apolipophorin-III in prophenoloxidase activation and antibacterial defense of the Chinese oak silkworm, Antheraea pernyi. Dev. Comp. Immunol. 65, 124–131. doi: 10.1016/j.dci.2016.07.001
Wicker-Thomas, C., Garrido, D., Bontonou, G., Napal, L., Mazuras, N., Denis, B., et al. (2015). Flexible origin of hydrocarbon/pheromone precursors in Drosophila melanogaster. J. Lipid Res. 56, 2094–2101. doi: 10.1194/jlr.M060368
Yang, Q., Li, Z., Cao, J., Zhang, S., Zhang, H., Wu, X., et al. (2014). Selection and assessment of reference genes for quantitative PCR normalization in migratory locust Locusta migratoria (Orthoptera: Acrididae). PLoS One 9:e98164. doi: 10.1371/journal.pone.0098164
Yu, R., Liu, W., Li, D., Zhao, X., Ding, G., Zhang, M., et al. (2016). Helicoidal organization of Chitin in the cuticle of the migratory locust requires the function of the chitin Deacetylase2 enzyme (LmCDA2). J. Biol. Chem. 291, 24352–24363. doi: 10.1074/jbc.M116.720581
Yu, Z., Wang, Y., Zhao, X., Liu, X., Ma, E., Moussian, B., et al. (2017). The ABC transporter ABCH-9C is needed for cuticle barrier construction in Locusta migratoria. Insect Biochem. Mol. Biol. 87, 90–99. doi: 10.1016/j.ibmb.2017.06.005
Yu, Z., Zhang, X., Wang, Y., Moussian, B., Zhu, K., Li, S., et al. (2016). LmCYP4G102: An oenocyte-specific cytochrome P450 gene required for cuticular waterproofing in the migratory locust, Locusta migratoria. Sci. Rep. 6:29980. doi: 10.1038/srep29980
Zdybicka-Barabas, A., and Cytryńska, M. (2013). Apolipophorins and insects immune response. Invertebrate Surviv. J. 10, 58–68.
Zhang, X., Jie, D., Liu, J., Zhang, J., Zhang, T., Zhang, J., et al. (2019). Aryl hydrocarbon receptor regulates the expression of LmGSTd7 and is associated with chlorpyrifos susceptibility in Locusta migratoria. Pest Manag. Sci. 75, 2916–2924. doi: 10.1002/ps.5600
Zhang, X., Wang, J., Liu, J., Li, Y., Liu, X., Wu, H., et al. (2017). Knockdown of NADPH-cytochrome P450 reductase increases the susceptibility to carbaryl in the migratory locust, Locusta migratoria. Chemosphere 188, 517–524. doi: 10.1016/j.chemosphere
Keywords: Locusta migratoria, cuticular lipids, apolipophorin, cuticular hydrocarbons, RNAi
Citation: Zhao Y, Liu W, Zhao X, Yu Z, Guo H, Yang Y, Zhang J, Moussian B and Zhang J (2020) Apolipophorin-II/I Contributes to Cuticular Hydrocarbon Transport and Cuticle Barrier Construction in Locusta migratoria. Front. Physiol. 11:790. doi: 10.3389/fphys.2020.00790
Received: 20 March 2020; Accepted: 15 June 2020;
Published: 08 July 2020.
Edited by:
Bin Tang, Hangzhou Normal University, ChinaReviewed by:
Wen-Jia Yang, Guiyang University, ChinaRui Pang, Guangdong Academy of Science, China
Yuxian Xia, Chongqing University, China
Copyright © 2020 Zhao, Liu, Zhao, Yu, Guo, Yang, Zhang, Moussian and Zhang. This is an open-access article distributed under the terms of the Creative Commons Attribution License (CC BY). The use, distribution or reproduction in other forums is permitted, provided the original author(s) and the copyright owner(s) are credited and that the original publication in this journal is cited, in accordance with accepted academic practice. No use, distribution or reproduction is permitted which does not comply with these terms.
*Correspondence: Bernard Moussian, YmVybmFyZC5tb3Vzc2lhbkB1bmktdHVlYmluZ2VuLmRl; Jianzhen Zhang, emp6QHN4dS5lZHUuY24=
†These authors have contributed equally to this work