- 1State Research Center of the Russian Federation, Institute of Biomedical Problems, Russian Academy of Sciences, Moscow, Russia
- 2Faculty of Biology, M.V. Lomonosov Moscow State University, Moscow, Russia
- 3Faculty of Basic Medicine, M.V. Lomonosov Moscow State University, Moscow, Russia
- 4Department of Nutrition, Food and Exercise Sciences, College of Human Sciences, Florida State University, Tallahassee, FL, United States
Introduction: Mechanical forces and sympathetic influences are key determinants of vascular structure and function. This study tested the hypothesis that hindlimb unloading (HU) exerts diverse effects on forelimb and hindlimb small arteries of rats in functionally different regions of the skeletal muscle and skin.
Methods: Male Wistar rats were subjected to HU for 2 weeks, then skeletal muscle arteries (deep brachial and sural) and skin arteries (median and saphenous) were examined in vitro using wire myography or isobaric perfusion and glyoxylic acid staining.
Results: HU increased lumen diameter of both forelimb arteries but decreased diameter of the sural artery; the saphenous artery diameter was not affected. Following HU, maximal contractile responses to noradrenaline and serotonin increased in the forelimb but decreased in the hindlimb skeletal muscle feed arteries with no change in skin arteries; all region-specific alterations persisted after endothelium removal. HU increased the sensitivity to vasoconstrictors in the saphenous artery but not in the sural artery. In the saphenous artery, initially high sympathetic innervation density was reduced by HU, sparse innervation in the sural artery was not affected. Electrical stimulation of periarterial sympathetic nerves in isobarically perfused segments of the saphenous artery demonstrated a two-fold decrease of the contractile responses in HU rats compared to that of controls.
Conclusion: HU induces contrasting structural and functional adaptations in forelimb and hindlimb skeletal muscle arteries. Additionally, HU had diverse effects in two hindlimb vascular regions. Hyper-sensitivity of the saphenous artery to vasoconstrictors appears to result from the shortage of trophic sympathetic influence. Importantly, HU impaired sympathetically induced arterial vasoconstriction, consistent with the decreased sympathetic constrictor response in humans following space flight.
Introduction
The vascular system is constantly exposed to mechanical forces such as transmural pressure and shear stress, the latter of which is created by flowing blood on the endothelial cell lining of the vessel. Prolonged changes in these factors govern gross structural remodeling of blood vessels, which in turn affects their functional characteristics. For example, previous work has shown that chronic increases in transmural pressure induce hypertrophy of the vascular wall and increases in vessel contractility, while decreases in pressure result in the opposite changes (Folkow, 1982). Chronic increases in shear stress, in turn, lead to structural increases in maximal diameter, whereas decreases in shear result in a narrowing of the vessel lumen (Langille, 1993; Pourageaud and De Mey, 1997).
The cardiovascular system of all terrestrial inhabitants is adapted to the gravitational field of the Earth and changes significantly in conditions of weightlessness (Watenpaugh and Hargens, 1996). Importantly, redistribution of transmural pressures and flows within the arterial vasculature during space flight or simulated microgravity is known to initiate different adaptations in vessels from different anatomic regions (Zhang, 2001, 2013). In humans, exposure to long term head-down bed rest was shown to reduce intimal-medial thickness and lumen diameter of arterial vessels in lower extremities, in contrast to upper extremity and cranial arteries (Bleeker et al., 2005; Platts et al., 2009).
The model of hindlimb unloading (HU) in rats is commonly used to study the mechanisms of microgravity effects on different body systems, including vasculature (Globus and Morey-Holton, 2016). Even relatively small change in hydrostatic pressure gradient, as observed in the rat body with this model (Colleran et al., 2000), results in different vascular alterations in rostral and caudal parts of the body. Similar to microgravity effects in human vascular system, rat hindlimb arteries commonly demonstrate wall thinning and lumen reduction, in contrast to cranial arteries (Wilkerson et al., 1999; Delp et al., 2000; Sun et al., 2004; Stabley et al., 2013).
Data from the literature also provide the evidence that transmural pressure shifts cannot explain all effects of simulated microgravity on vascular characteristics, because vascular alterations in HU rats may differ in functionally different vascular beds within the same part of the body. This was shown in studies of vascular dimensions and reactivity of arterioles from postural (soleus) and phasic (gastrocnemius) hindlimb muscles (Delp et al., 2000), as well as arterioles from white (glycolytic) and red (oxidative) parts from the same gastrocnemius muscle (Heaps and Bowles, 2002) of HU rats. Obviously, such diverse effects of HU on the arterial vasculature within and among postural and phasic muscles are largely the result of differential alterations in muscle activity and blood flow during the conditions of disuse (McDonald et al., 1992).
Structural and functional changes in the vascular bed could also result from the influence of sympathetic nervous system. In addition to acute control of vessel diameter and blood flow, neurotransmitters from the sympathetic nerves exert a trophic effect on the vasculature (Bevan, 1984; Puzdrova et al., 2014; Adeoye et al., 2015). The severity of trophic sympathetic influence in certain vascular bed may depend on the density of its innervation and/or on the efferent sympathetic traffic it receives. In addition, the state of the vascular neuroeffector apparatus has been shown to depend on the prevailing level of transmural pressure and, therefore, may be affected in the conditions of microgravity (Monos et al., 2001; Zhang, 2001; Puzdrova et al., 2009).
In contrast to the skeletal muscle circulation, the cutaneous circulation is regulated by a smaller contribution from metabolic vasomotor mechanisms and a higher role of sympathetic control, including a greater sympathetic vasoconstriction of small cutaneous arteries relative to that in skeletal muscle (Tarasova et al., 2003). However, to our knowledge, no study has examined the effects of simulated microgravity via HU on the structural and functional properties of cutaneous arteries, nor contrasted these effects in the forelimb and hindlimb circulations. Therefore, this study tested the hypothesis that HU exerts diverse effects on the neurovascular density, structural properties, and functional vasoconstrictor responsiveness of skeletal muscle and cutaneous feed arteries in the rat forelimb and hindlimb regions.
Materials and Methods
All the procedures were conducted in accordance with the European Convention for the Protection of Vertebrate Animals used for experimental and other scientific purposes and conformed to the Guide for the Care and Use of Laboratory Animals published by the US National Institutes of Health (Eighth edition, 2011). The procedures were approved by the Biomedical Ethics Committee of the Institute of Biomedical Problems, Russian Academy of Sciences (protocol N224).
Animals
Male Wistar rats (2.5–3-month-old) were obtained from the breeder of the Institute of Biomedical Problems and housed in laboratory animal unit at the Faculty of Biology, M.V. Lomonosov Moscow State University in controlled conditions of temperature (22–24°C) and light-dark cycle (12–12 h) with ad libitum access to water and standard chow diet (Laboratorkorm, Russia). After 1-week acclimatization to the environment, the rats were randomly assigned to the cage control (n = 10) and HU (n = 10) groups. In HU group, the hindlimbs of the rat were elevated with a hook attached to the proximal third of the tail with adhesive material. The hook was connected by a harness to a swivel apparatus at the top of the cage. The height of the hindquarter elevation was adjusted to prevent the hindlimbs from touching the supporting surfaces, resulting in a suspension angle of 35–40°. The forelimbs maintained the contact with the floor surface, which allowed the animals full range of motion. The animals were housed individually in cages of 50 cm × 50 cm × 50 cm. Animals remained under HU or control conditions for a total of 14 days. This duration of HU was shown to provide the stable changes in muscle weight and blood flow in rats (McDonald et al., 1992). No signs of ischemia or damage of the tail skin were observed during HU.
After 2 weeks of HU, the animals were sacrificed by decapitation under CO2 anesthesia and the arteries were dissected free from surrounding tissue. Two arteries were isolated from the forelimb (deep brachial artery and median artery) and two arteries from the hindlimb (sural artery and saphenous artery). These feed arteries provide blood flow to skeletal muscle (brachial and sural arteries) and predominantly to the skin (median and saphenous arteries). Brachial and median arteries were dissected from the right forelimb for myography experiments. Sural arteries from right and left hindlimbs were used for myography experiments and histochemical examination, respectively. Right saphenous artery from each rat was used for wire myography (distal part) and histochemical examination (proximal part), while the artery from the left hindlimb was studied in perfusion experiments.
Wire Myography
Two neighboring segments with a length of 2 mm were cut from each type of artery and mounted in wire myograph system (DMT, Denmark, 410A or 620 model) for isometric force recoding at well-defined internal circumferences. Endothelium was gently removed from one of the segments by rubbing with a rat whisker. The preparations were kept at 37°C in physiological salt solution containing (in mM): 120 NaCl, 26 NaHCO3, 4.5 KCl, 1.2 NaH2PO4, 1.0 MgSO4, 1.6 CaCl2, 5.5 D-glucose, 0.025 EDTA, and 5 HEPES, equilibrated with gas mixture 5% CO2 + 95% O2 to maintain pH 7.4. Force readings were continuously recorded at 10 Hz sampling rate using E14-140 analog-to-digital data converter (L-Card, Russia) and PowerGraph 3.3 software (DISoft, Russia). Fully relaxed arterial segments were gradually stretched to d100, the inner diameter equivalent to a transmural pressure of 100 mmHg, and then set to 0.9d100, where maximal active force is developed (Mulvany and Halpern, 1977).
The arteries were activated three times with noradrenaline (10−6 M, Sigma). Functional integrity of the endothelium was checked by application of acetylcholine (10−6 M, Sigma) on top of noradrenaline-induced contraction (3 × 10−7 M). Endothelium-intact preparations relaxed in response to acetylcholine by at least 50% of the precontraction level and the relaxation response of endothelium-denuded preparations was not larger than 10%. The experimental protocol included consecutively performed concentration-response relationships to noradrenaline (10−8 – 3 × 10−5 М, Sigma) and serotonin (3 × 10−8 – 3 × 10−5 М, Sigma) with 30-min washout interval between them. The agonists were given cumulatively in half-log increments. The responses to noradrenaline were studied in the presence of propranolol (10−6 M, Sigma), to prevent relaxation mediated by β-adrenoceptors.
During data analysis, active force values were calculated by subtracting the passive force value (recorded in the preparation with fully relaxed smooth muscle) from all recorded force values (before the first and at each noradrenaline or serotonin concentration). Then, respective values of active wall tension were calculated as , where T is tension, F is active force and l is the segment length. The concentration-response relationships were fitted to a sigmoidal function with variable slope using GraphPad Prism 7.0 software (La Jolla, CA, USA) to calculate pD2 values (the negative logarithm of EC50). Inner diameter of each artery (d100) was estimated from its passive length-tension relationship.
Constant-Pressure Perfusion
A segment of the saphenous artery was isolated, placed into the tissue bath, and cannulated at both ends. The vessel fragment length between the cannulae was about 5–6 mm. Krebs-Henseleit physiological salt solution was used for perfusion and superfusion (NaCl 119 mM, KCl 4.7 mM, CaCl2 2.5 mM, MgSO4 1.17 mM, NaHCO3 25 mM, KH2PO4 1.18 mM, D-glucose 5.5 mM, EDTA 0.026 mM, 37°C, and 95% O2 + 5% CO2). Artery was perfused under a constant pressure; input and output pressures were measured continuously using two pressure transducers (BLPR2, World Precision Instruments, USA) and kept at 60 and 50 mmHg, respectively. Volume flow rate was measured with 1N type transducer connected to transit-time flowmeter (Transonic Systems Inc., USA). All parameters were permanently recorded at 20 Hz sampling rate using E14-140 analog-to-digital data converter (L-Card, Russia) and PowerGraph 3.3 software (DISoft, Russia).
Intramural nerves were stimulated with rectangular electric pulses of changing polarity, with amplitude of 200–300 mA and 0.2 ms duration. All the effects of electrical stimulation could be blocked by tetrodotoxin (3 μM, Sigma), thus indicating the neurogenic origin of the response. Electric pulse frequency was 4, 8, or 12 Hz, the stimulation lasted for 30 s, and 3-min intervals were applied between the stimulations. Data analysis was performed using a custom program working under MATLAB (MathWorks Inc., USA). The constrictor response was evaluated as a relative change of the intralumen diameter (D), which was calculated by Poiseuille equation: , l, length of the vessel segment between canulae; Q, volume flow rate; and ΔP, the difference between input and output pressures.
Glyoxylic Acid Staining for Periarterial Adrenergic Innervation
Segments of saphenous and sural arteries were cut lengthwise and placed in 0.1 M PBS (pH 7.2) supplemented with 2% glyoxylic acid, 10% sucrose, and 0.03% Pontamine Sky Blue (Puzdrova et al., 2009). After a 30-min incubation, the preparation was flattened on the slide with the adventitia upward, dried (30 min in jet of warm air and 5 min at 100°C), and overlaid with mineral oil and covered with a cover glass.
A LUMAM R3 microscope (LOMO, USSR; eyepiece ×7, objective lens ×40) was used for visualization. The exciting light wavelength was 380–440 nm; the luminescence wavelength was 480–700 nm. The plexus density was estimated with a grid that covered a field of 300 × 300 μm on the preparation and consisted of 24 rows, each containing 22–23 round markers (the ratio of marker diameter to space between markers was 1:2.5). Counting was performed in three randomly selected fields and the results were averaged.
Statistical Data Analysis
Statistical analysis was performed in GraphPad Prism 7.0. The normality of the data distribution was confirmed using D’Agostino-Pearson test. Unpaired Student’s t-test or Repeated Measures ANOVA with Bonferroni post hoc test was used, as appropriate. Statistical significance was reached at p < 0.05. All data are given as mean ± S.E.M. and n represents the number of animals.
Results
Body Weight
At the beginning of the experiment, body weights of rats from control and HU groups were 316 ± 6 g (n = 10) and 310 ± 9 g (n = 10), respectively (p > 0.05). Within 2 weeks, control group increased body weight to 376 ± 14 g (n = 10) and HU group to 353 ± 6 g (n = 10). No difference in body weight was observed between the two experimental groups at the end of the experiment (p > 0.05, control vs. HU).
Inner Diameter of the Arteries
Both forelimb arteries from HU rats had larger inner diameters compared to the respective arteries from control rats (Table 1). However, when calculated as percentage of the mean value in control group, the increment of diameter in the brachial artery was more prominent compared to the median artery: 22.5 ± 3.0 and 10.2 ± 3.8%, respectively (p < 0.05).
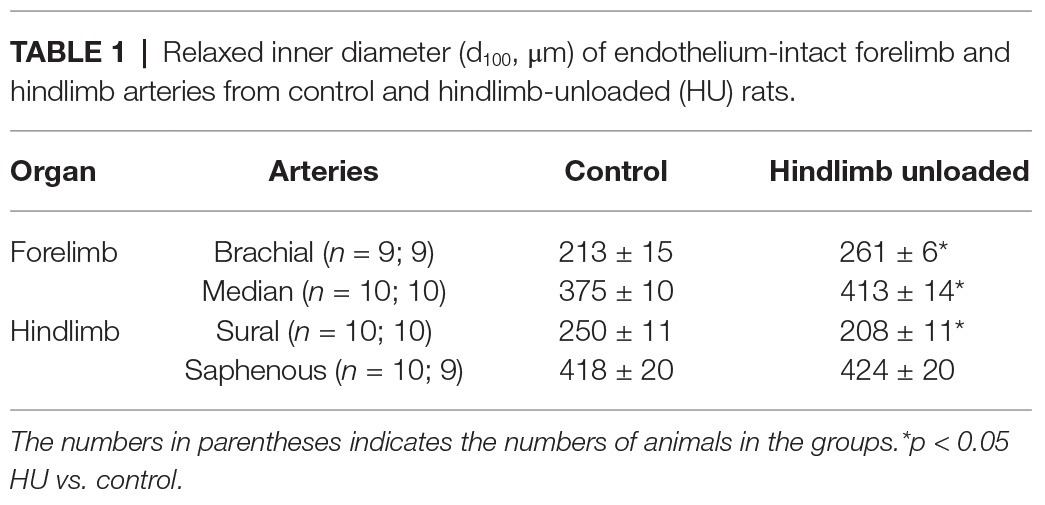
Table 1. Relaxed inner diameter (d100, μm) of endothelium-intact forelimb and hindlimb arteries from control and hindlimb-unloaded (HU) rats.
In contrast, sural arteries of HU rats demonstrated a decrease of inner diameter (by 17.9 ± 4.7%) compared to the arteries of control rats (Table 1). The diameter of saphenous artery was not affected by HU (Table 1).
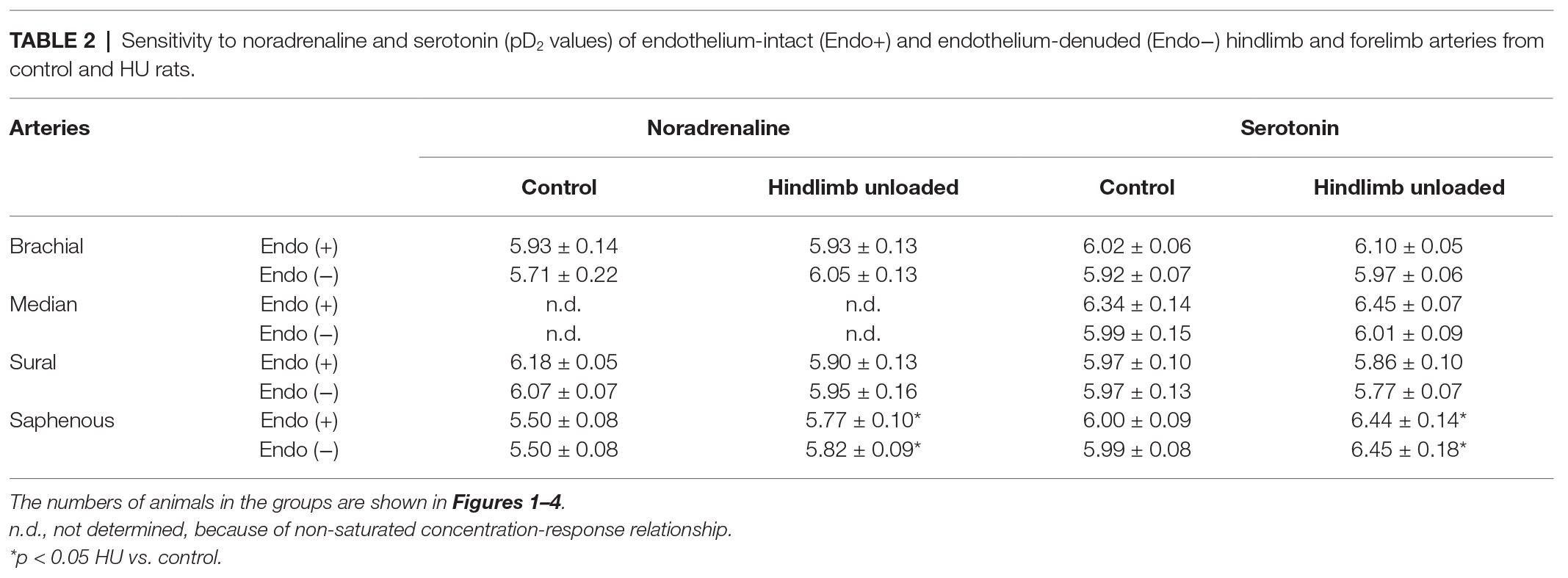
Table 2. Sensitivity to noradrenaline and serotonin (pD2 values) of endothelium-intact (Endo+) and endothelium-denuded (Endo−) hindlimb and forelimb arteries from control and HU rats.
Contractile Responses of Forelimb Arteries
Contractile responses of brachial arteries were greater in HU rats compared to that in control rats. This was observed when endothelium-intact segments of brachial arteries were exposed to noradrenaline (Figure 1A) or serotonin (Figure 1B). Endothelium removal did not change the differences in brachial artery contractile responses between HU and control rats (Figures 1C,D). Most prominent effects of HU on brachial artery contractility were observed when the agonists were applied in near-maximal to maximal concentrations. The sensitivity of endothelium-intact and endothelium-denuded brachial artery preparations to vasoconstrictors was not altered by HU (Table 2).
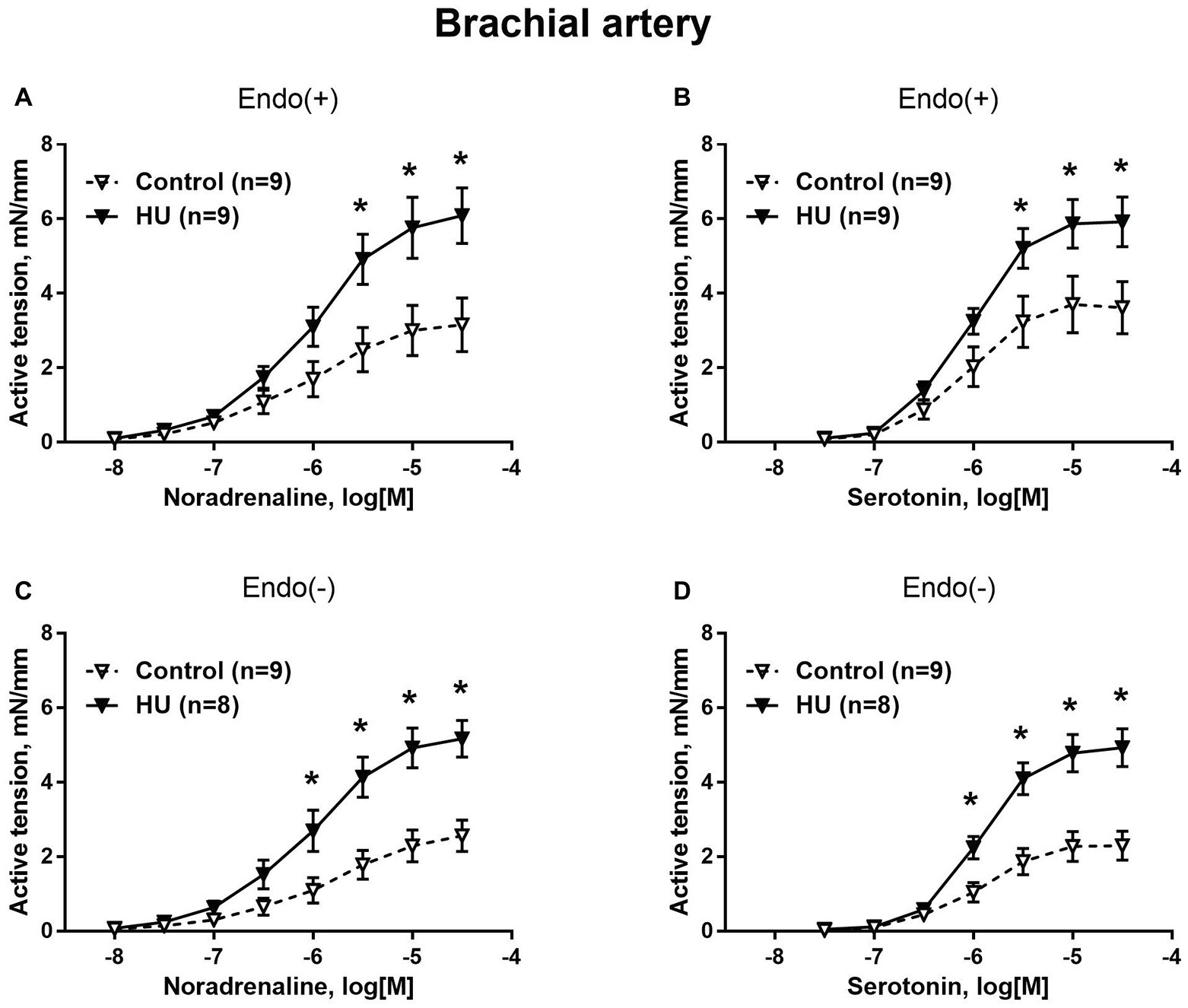
Figure 1. HU increases contractile responses of skeletal muscle feed arteries from the rat forelimb. Concentration-response relationships to noradrenaline (A,C) and serotonin (B,D) of endothelium-intact (A,B) and endothelium-denuded (C,D) deep brachial arteries in control and HU rats. The number in parentheses indicates the number of animals in the group. * p < 0.05 HU vs. control.
Contractile responses of median artery segments to noradrenaline and serotonin were not different in HU rats compared to control rats regardless of the presence of the endothelium (Figure 2). The sensitivity of median artery segments to both vasoconstrictors was also similar in control and HU rats (Table 2).
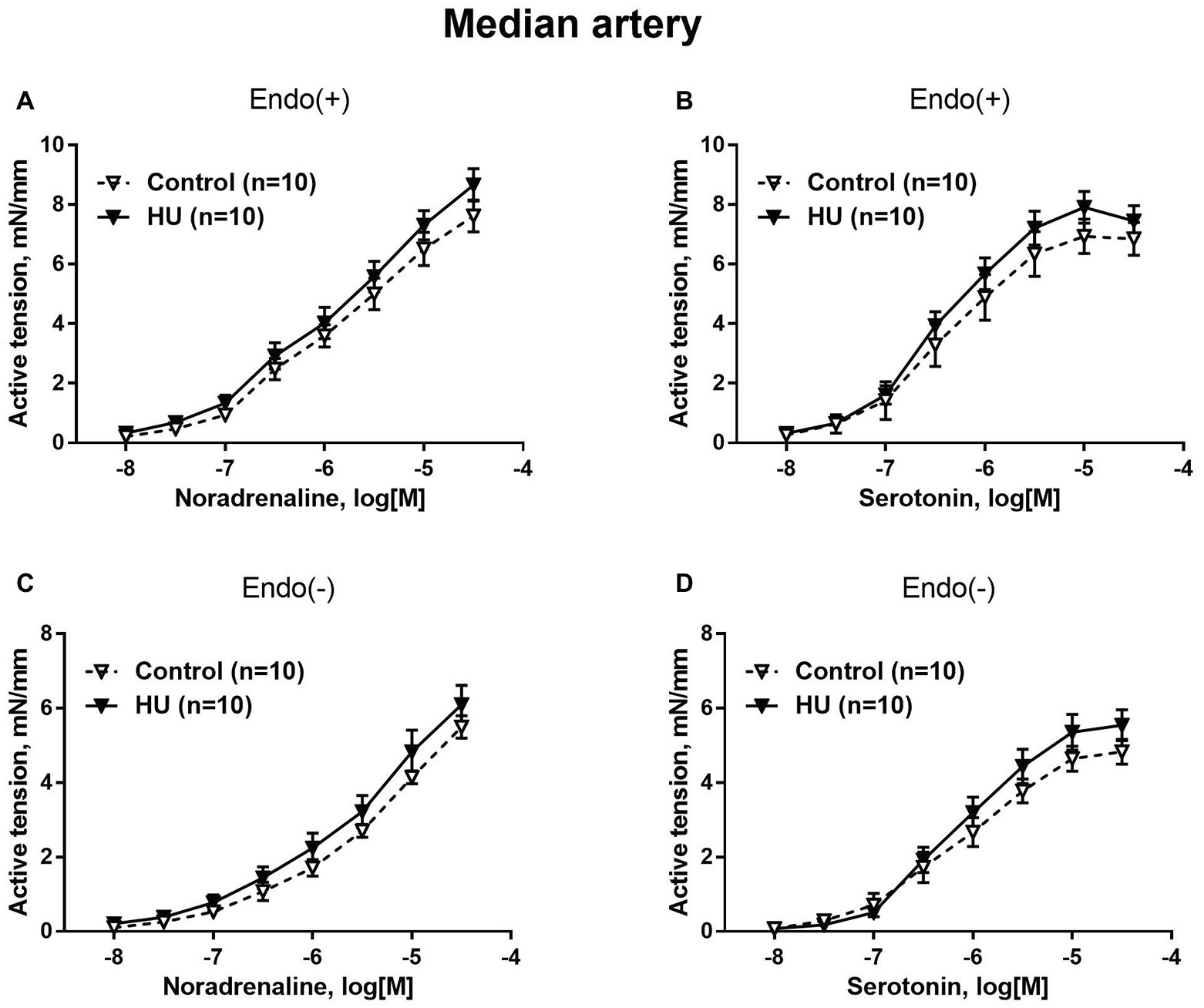
Figure 2. HU does not change contractile responses of skin feed arteries from the rat forelimb. Concentration-response relationships to noradrenaline (A,C) and serotonin (B,D) of endothelium-intact (A,B) and endothelium-denuded (C,D) median arteries in control and HU rats. The number in parentheses indicates the number of animals in the group.
Contractile Responses of Hindlimb Arteries
Sural arteries of HU rats developed less contractile tension to noradrenaline and serotonin. This decrease was observed in both endothelium-intact (Figures 3A,B) and endothelium-denuded (Figures 3C,D) segments of sural artery from HU rats compared to control rats. Again, most prominent effect of HU on contractile responses was observed with the higher concentrations of the agonists, while the sensitivity of sural arteries to contractile stimuli was not altered by HU (Table 2).
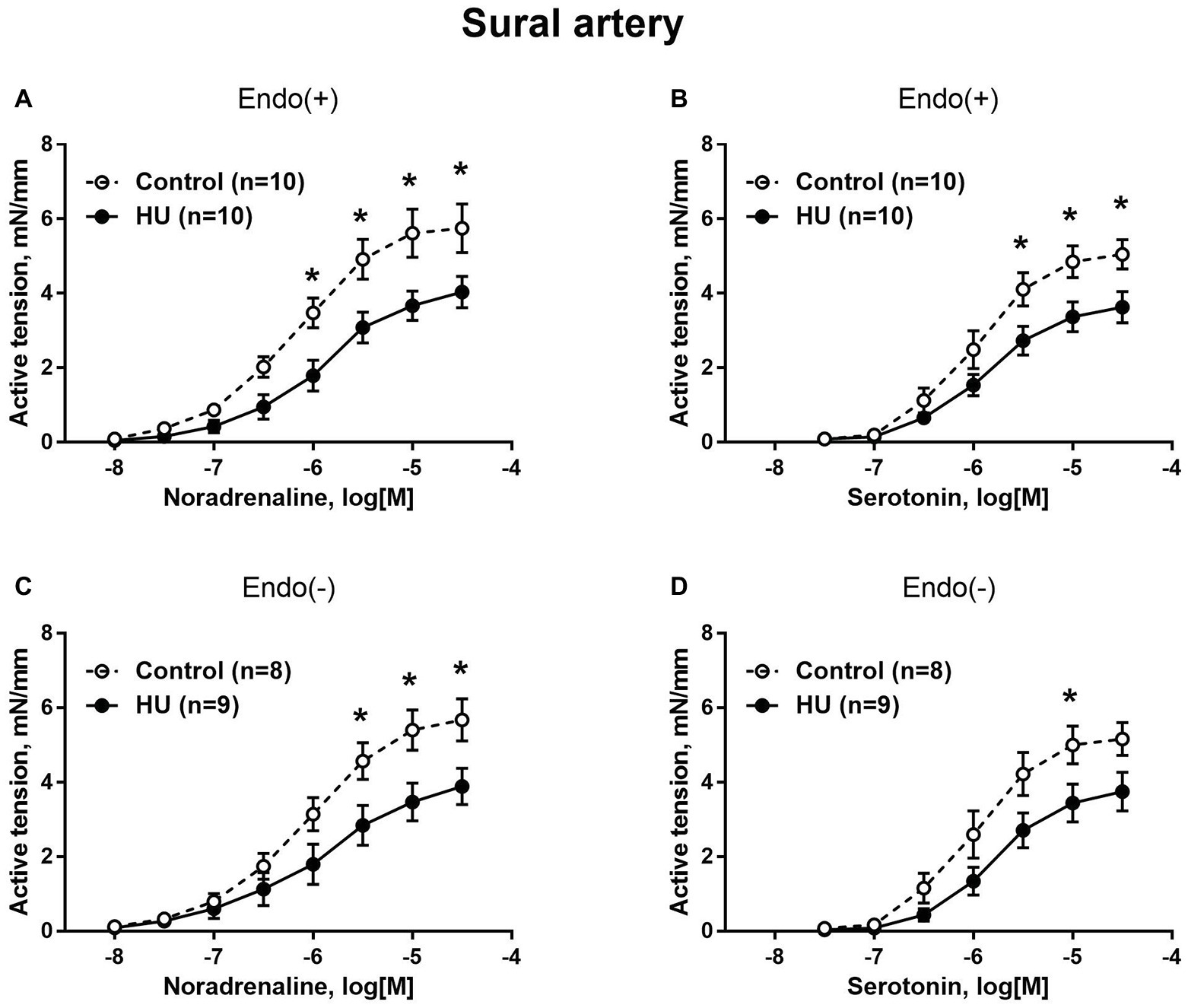
Figure 3. HU decreases contractile responses of skeletal muscle feed arteries from the rat hindlimb. Concentration-response relationships to noradrenaline (A,C) and serotonin (B,D) of endothelium-intact (A,B) and endothelium-denuded (C,D) sural arteries in control and HU rats. The number in parentheses indicates the number of animals in the group. * p < 0.05 HU vs. control.
In contrast to sural arteries, saphenous arteries from HU rats developed stronger contractile responses compared to the arteries from control rats, regardless of the type of agonist and the presence of the endothelium (Figure 4). HU did not alter saphenous artery maximum contractile responses to noradrenaline and serotonin (Figure 4), but rather elevated the sensitivity of this artery to vasoconstrictors (Table 2).
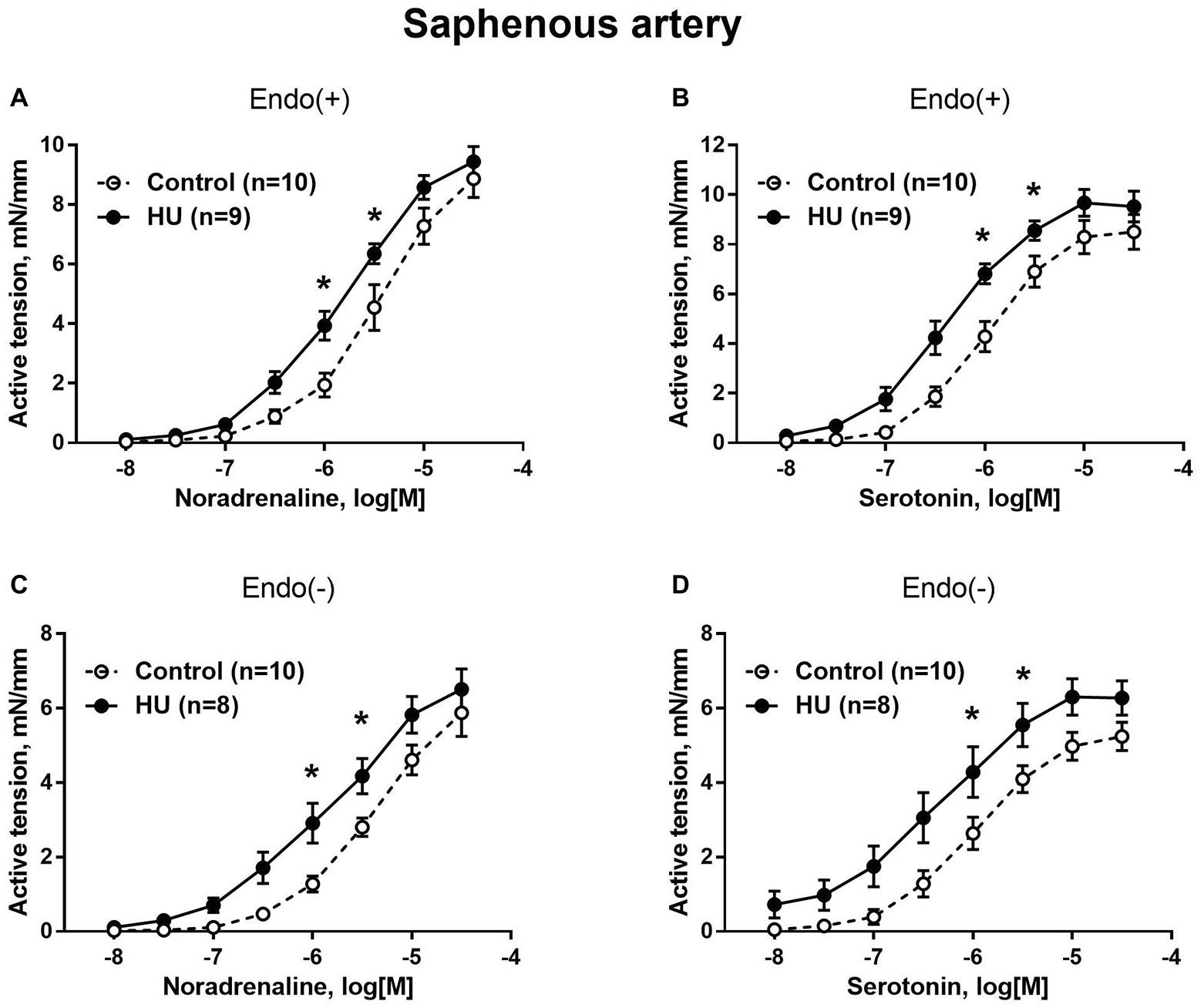
Figure 4. HU increases contractile responses of skin feed arteries from the rat hindlimb. Concentration-response relationships to noradrenaline (A,C) and serotonin (B,D) of endothelium-intact (A,B) and endothelium-denuded (C,D) sural arteries in control and HU rats. The number in parentheses indicates the number of animals in the group. * p < 0.05 HU vs. control.
Sympathetic Neurotransmission in Saphenous Artery
In control rats, adrenergic fiber plexus was dense in the saphenous artery, but sparse in the sural artery (Figure 5A). HU had no effect on innervation density in the sural artery (Figure 5A) but decreased it by about 20% in the saphenous artery (Figures 5A,B).
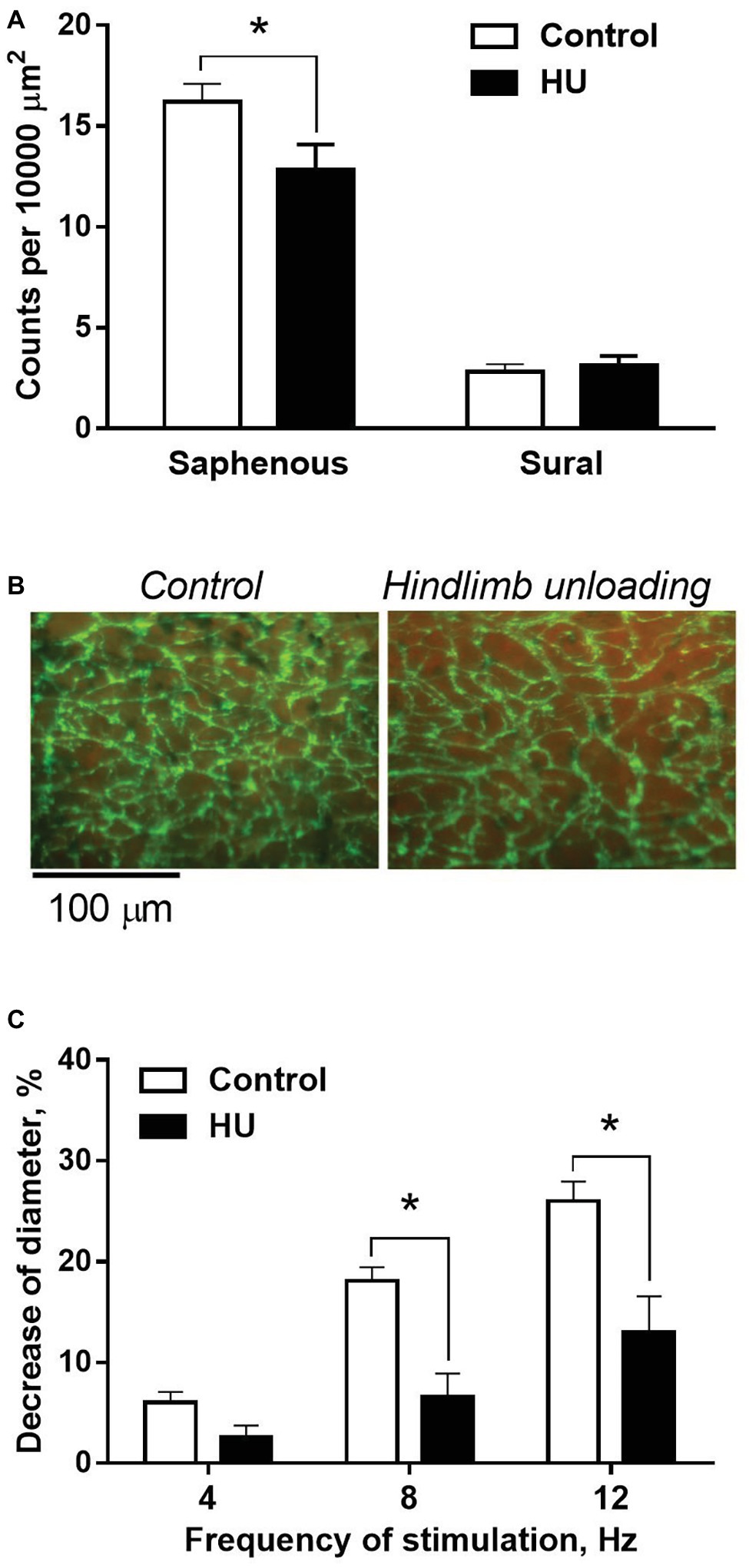
Figure 5. HU worsens sympathetic control in skin but not skeletal muscle feed arteries from rat hindlimb. (A) The density of periarterial adrenergic plexus in saphenous and sural arteries from control (n = 10; 10) and HU (n = 8; 7) rats. (B) Representative staining for adrenergic nerves with glyoxylic acid in whole-mount preparations of saphenous arteries. (C) Constrictor responses of isobarically perfused saphenous arteries from control (n = 5) and HU (n = 5) rats to electrical field stimulation of intramural nerves with graded frequencies. * p < 0.05 HU vs. control.
The effects of HU on sympathetic control of the saphenous artery were studied with the use of perfusion technique. In the absence of nerve stimulation, solution flow rate values did not differ in control and HU rats: 3.9 ± 0.18 and 4.1 ± 0.18 ml/min, respectively. The relative decreases of arterial inner diameter caused by 8 and 12 Hz stimulation were lower in HU rats compared to that in controls (Figure 5C).
Discussion
The purpose of this study was to compare the effects of 2 weeks of simulated microgravity on skeletal muscle and skin feed arteries from the rat forelimb and hindlimb regions. We hypothesized that HU exerts diverse effects on the sympathetic innervation density, structural properties, and functional vasoconstrictor responsiveness of skeletal muscle and cutaneous feed arteries. The data indicate that HU induces increases in maximal lumen diameter and vasoconstrictor responsiveness in forelimb skeletal muscle arteries, but decreases in these structural and functional properties in hindlimb skeletal muscle arteries. In the cutaneous vasculature of the forelimbs and hindlimbs, alterations in the structure and vasoconstrictor properties either did not occur or were of smaller magnitude than that occurring in the skeletal muscle arteries from the same region of the body. Exposure to HU also diminished sympathetic nerve density and impaired vasoconstriction induced by sympathetic nerve stimulation in hindlimb cutaneous, but not skeletal muscle arteries. However, vasoconstrictor responses of hindlimb cutaneous arteries to direct smooth muscle stimulation by vasoconstrictor agonists exhibited greater sensitivity in the HU rats. Collectively, these results demonstrate that the complexity of vascular adaptations to simulated microgravity is much greater than previously described. Vascular alterations not only differentially occur between regions of the body, but also within those regions, and can be functionally related to physical alterations in sympathetic nerve density and gross structural remodeling of resistance arteries.
Both the forelimbs and hindlimbs of the rat perform functions of support and locomotion, which suggest these two different anatomical regions of the body share similar inherent characteristics that include skeletal muscle and cutaneous circulations. Additionally, systemic humoral influences would not differ in forelimb and hindlimb arteries of the same rat. Alterations in nerve traffic during HU exposure would also likely be equalized between the same-type arteries in the forelimbs and hindlimbs, since functional specialization of efferent sympathetic pathways is organotypic rather than somatotopic (Jänig and McLachlan, 1992; Jänig and Häbler, 2003). Therefore, the design of this study allowed us to separate adaptive arterial responses to the anti-orthostatic body position and redistribution of support and muscle activity from other effects associated with the HU model, including social isolation, restraint, and the development of neuroendocrine stress (Tsvirkun et al., 2012).
Hindlimb Unloading Induces Contrasting Structural and Functional Adaptations in Forelimb and Hindlimb Skeletal Muscle Arteries
HU exposure resulted in divergent alterations of forelimb and hindlimb skeletal muscle arteries, i.e., lumen diameter and wall contractility (maximal active tension) increased in the forelimb but decreased in the hindlimb. The changes in contractility were independent of the vascular endothelium and not associated with the change in smooth muscle sensitivity to contractile stimulation by noradrenaline or serotonin. These data point to non-specific amplification of smooth muscle contractile responses in forelimb arteries, presumably resulting from smooth muscle hypertrophy and media wall thickening. In contrast, non-specific attenuation of smooth muscle contractile responses takes place in hindlimb arteries, presumably resulting from smooth muscle atrophy and a thinning of the medial wall (Delp et al., 2000).
Previous work has shown that HU in rats is associated with ~10 mmHg gradient between aortic and hindlimb arterial pressures (Colleran et al., 2000). Therefore, arterial transmural pressure in HU rats is elevated in the forelimb region and reduced in the hindlimbs; this pressure differential presumably governs the divergent alterations in media thickness (Wilkerson et al., 1999; Stabley et al., 2012). The mechanisms which mediate the effects of transmural pressure on media remodeling may include the secretion of angiotensin II from vascular smooth muscle cells with further stimulation of MAPK cascades (Eskildsen-Helmond and Mulvany, 2003). Importantly, HU was shown to stimulate the activity of vascular renin-angiotensin system in cranial arteries, but depress it in femoral arteries (Bao et al., 2007; Zhang, 2013). In support of the present data, wall thickness and medial cross-section area were shown to be diminished in resistance arteries associated with the anterior tibial (Sun et al., 2004) and gastrocnemius (Delp et al., 2000) muscles in the hindlimbs of HU rats. In addition, chronic increases in blood flow/shear stress in loaded forelimb muscles and chronic decreases in unloaded hindlimb muscles induce divergent changes in maximal passive diameter of arteries (Delp et al., 2000; Stabley et al., 2013). Similar results have also been found in human models used to simulate microgravity. For instance, long-duration (up to 60 days) head-down bed rest has been reported to reduce intimal-medial thickness in the anterior tibial artery (Platts et al., 2009), as well as lumen diameter of the femoral artery and its branch (Bleeker et al., 2005). Therefore, these results indicate that the gross structural adaptations of skeletal muscle arteries in HU rats and humans undergoing head-down bed rest are mainly induced by the influence of mechanical forces.
In both the forelimbs and hindlimbs, cutaneous arteries were less affected by HU, as compared to skeletal muscle arteries from the same region of the body. These results may be attributed to several factors. First, cutaneous blood flow would not be as greatly affected by changes in postural support and low level physical activity as that of the skeletal muscle, particularly given the rapid and profound changes in skeletal muscle vascular resistance associated with increases and decreases in muscle activity (Delp, 1999). Second, the profile of blood pressure changes within the cutaneous vasculature may differ from that within the skeletal muscle vascular tree. A prominent constriction of proximal cutaneous arteries during sympathetic activation (Tarasova et al., 2003) would decrease transmural pressure in more distal arteries (Abboud and Eckstein, 1966) and protect them from redistribution of transmural pressure during HU.
Hindlimb Unloading Had Diverse Effects in Two Functionally Different Vascular Beds of the Rat Hindlimb
In the hindlimb, 2-week exposure to HU impaired sympathetic nerve-mediated vasoconstriction while enhancing the sensitivity of smooth muscle to the adrenergic agonist noradrenaline in cutaneous arteries. The higher constrictor sensitivity of cutaneous arteries was also observed after endothelium removal and with the application of the non-adrenergic agonist serotonin, pointing to its independence of the type of activated receptors in smooth muscle cells. These data suggest the development of non-specific hyper-sensitivity of the saphenous artery to constrictor stimulation, which may result from the deficiency in its sympathetic control. This suggestion is based on several observations from the present and earlier published studies: (1) the higher sensitivity to constrictors was observed in densely innervated saphenous artery but not in the sparsely innervated sural artery; (2) HU reduced the density of adrenergic plexus in saphenous arteries; and (3) denervated vessels are well-known to become hyper-sensitive to various constrictor agonists and high-K+ depolarization (Bevan, 1984; Bentzer et al., 1997; Tarasova et al., 2006). Post-denervation hyper-sensitivity occurs without changes in total density or affinity of post-junctional α1-adrenoceptors (Dalessandri et al., 1991; Taki et al., 2004), indicating its mechanisms of effect are downstream to the receptors and may include depolarization of smooth muscle cells (Fleming, 1999), facilitation of L-type Ca2+-channel-dependent signaling (Heumann et al., 2016), Ca2+-sensitization of smooth muscle contractile machinery (Puzdrova et al., 2014), and altered cell-to-cell coupling (Slovut et al., 2004). Taken together, these observations allow the conclusion that the shortage of sympathetic influences may govern functional remodeling of arteries subjected to gravitational unloading.
In our opinion, the effect of HU on saphenous artery sympathetic innervation is due in part to a drop in transmural pressure in hindlimb arteries during long-term anti-orthostasis (Colleran et al., 2000). It is well-known that the functional state of postganglionic nerve fibers and the density of sympathetic innervation is controlled by target tissue-derived trophic factors, including nerve growth factor (NGF) (Creedon and Tuttle, 1991; Thrasivoulou and Cowen, 1995). For example, it has been shown that NGF secretion by vascular smooth muscle cells in cell culture increases with stretch (Clemow et al., 2000). Presumably, the production of NGF under in vivo conditions is also dependent on the stretch induced by the transmural pressure. A chronic decrease in transmural pressure in the saphenous artery of HU rats could suppress NGF production and, therefore, result in the decrease of adrenergic nerve plexus density. We have shown previously that chronic hindquarter hypotension (induced by partial occlusion of the abdominal aorta distal to the renal arteries) has negative effects on sympathetic innervation of the rat saphenous artery (Tarasova et al., 2006). Interestingly, an opposite change of innervation density was demonstrated in the rat saphenous artery after the 2-week experimental orthostatic body position (Monos et al., 2001). Results from the present study thus indicate that chronic decreases in transmural pressure worsens sympathetic vasomotor control at the prejunctional level, but at the same time, increases vessel reactivity to noradrenaline, a principal transmitter of the sympathetic nerves. Further work should be conducted to determine whether noradrenaline release during activation of the sympathetic nerves in skin arteries differs between control and HU rats to better characterize how sympathetic neural control of peripheral resistance is altered.
In contrast to cutaneous arteries, we did not find any effect of transmural pressure changes on the adrenergic nerve plexus in the sural artery, probably due to the already low density of its innervation in control rats. However, we suggest that such effects of pressure to decrease the innervation density at distal part of skeletal muscle arterial tree may occur, since the more distal resistance arteries have a higher density of innervation. Indeed, a decrease in the innervation density was observed in hindlimb muscle arterioles in rats after a 4-week HU (Zhang, 2001). This suggestion is supported by our findings of a reduced integral response of perfused rat hindlimb vascular bed to the stimulation of sympathetic nerves (Rodionov et al., 1999).
Our findings indicate that the precision in which the sympathetic nervous system controls peripheral resistance and cutaneous perfusion is compromised in HU rats. Importantly, a decreased vascular sensitivity to sympathetic influences has been observed in humans following space flight (Fu et al., 2002). A smaller increase in lower limb arterial resistance was observed in cosmonauts during infight and postflight lower body negative pressure (LBNP) test (Herault et al., 2000). In contrast, forelimb subcutaneous vascular response to LBNP was more pronounced after 10-day-long space flight than during preflight (Gabrielsen et al., 1995). Although the present study is limited to an animal model used to simulate spaceflight, it provides a mechanistic explanation of the link between the state of sympathetic vascular control with the prevailing changes in transmural pressure thought to occur in astronauts and cosmonauts.
Conclusion
A novel result of this study is that simulated microgravity differentially affects the skin and skeletal muscle arteries in the hindlimb. Functional alterations in the vasoconstrictor responses of sparsely innervated skeletal muscle arteries appear to primarily result from the direct effects of physical forces acting on smooth muscle or endothelial cells to induce a gross structural remodeling of these arteries. However, functional changes in the densely innervated cutaneous arteries appear to be mainly governed by the effect of transmural pressure on periarterial sympathetic innervation. If similar adaptations occur in the peripheral vasculature of human cosmonauts and astronauts, such changes could underlie the weaker arterial vasoconstriction during activation of the sympathetic nerves, as well as serve as a mechanism of reduced vascular resistance and orthostatic intolerance following a return to Earth.
Data Availability Statement
The datasets generated for this study are available on request to the corresponding author.
Ethics Statement
The animal study was reviewed and approved by Biomedical Ethics Committee of the Institute of Biomedical Problems, Russian Academy of Sciences.
Author Contributions
OT, AB, VG, MD, and OV conceived and designed the study. OT, VK, AB, and VG were involved in laboratory work, data collection, and analysis. OT, AB, MD, and OV drafted the manuscript. All the authors contributed in the final writing.
Funding
The study was performed according to the Plan for Fundamental research of State Research Center of the Russian Federation, Institute of Biomedical Problems, Russian Academy of Sciences (N 64.1).
Conflict of Interest
The authors declare that the research was conducted in the absence of any commercial or financial relationships that could be construed as a potential conflict of interest.
References
Abboud, F. M., and Eckstein, J. W. (1966). Comparative changes in segmental vascular resistance in response to nerve stimulation and to norepinephrine. Circ. Res. 18, 263–277. doi: 10.1161/01.RES.18.3.263
Adeoye, O. O., Silpanisong, J., Williams, J. M., and Pearce, W. J. (2015). Role of the sympathetic autonomic nervous system in hypoxic remodeling of the fetal cerebral vasculature. J. Cardiovasc. Pharmacol. 65, 308–316. doi: 10.1097/FJC.0000000000000192
Bao, J.-X., Zhang, L.-F., and Ma, J. (2007). Angiotensinogen and AT1R expression in cerebral and femoral arteries during hindlimb unloading in rats. Aviat. Space Environ. Med. 78, 852–858.
Bentzer, P., Nielsen, N., Arner, M., Danielsen, N., Ekblad, E., Lundborg, G., et al. (1997). Supersensitivity in rat micro-arteries after short-term denervation. Acta Physiol. Scand. 161, 125–133. doi: 10.1046/j.1365-201X.1997.00177.x
Bevan, R. D. (1984). Trophic effects of peripheral adrenergic nerves on vascular structure. Hypertension 6, 19–26. doi: 10.1161/01.hyp.6.6_pt_2.iii19
Bleeker, M. W. P., De Groot, P. C. E., Rongen, G. A., Rittweger, J., Felsenberg, D., Smits, P., et al. (2005). Vascular adaptation to deconditioning and the effect of an exercise countermeasure: results of the Berlin Bed Rest study. J. Appl. Physiol. 99, 1293–1300. doi: 10.1152/japplphysiol.00118.2005
Clemow, D. B., Steers, W. D., and Tuttle, J. B. (2000). Stretch-activated signaling of nerve growth factor secretion in bladder and vascular smooth muscle cells from hypertensive and hyperactive rats. J. Cell. Physiol. 183, 289–300. doi: 10.1002/(SICI)1097-4652(200006)183:3<289::AID-JCP1>3.0.CO;2-6
Colleran, P. N., Wilkerson, M. K., Bloomfield, S. A., Suva, L. J., Turner, R. T., and Delp, M. D. (2000). Alterations in skeletal perfusion with simulated microgravity: a possible mechanism for bone remodeling. J. Appl. Physiol. 89, 1046–1054. doi: 10.1152/jappl.2000.89.3.1046
Creedon, D., and Tuttle, J. B. (1991). Nerve growth factor synthesis in vascular smooth muscle. Hypertension 18, 730–741. doi: 10.1161/01.hyp.18.6.730
Dalessandri, K. M., Giri, S. R., Robison, T. W., Hayashi, H. H., and Talken, L. (1991). No change in alpha 1 adrenoceptors in canine femoral arteries after lumbar sympathectomy. J. Investig. Surg. 4, 137–140. doi: 10.3109/08941939109140773
Delp, M. D. (1999). Control of skeletal muscle perfusion at the onset of dynamic exercise. Med. Sci. Sports Exerc. 31, 1011–1018. doi: 10.1097/00005768-199907000-00014
Delp, M. D., Colleran, P. N., Wilkerson, M. K., McCurdy, M. R., and Muller-Delp, J. (2000). Structural and functional remodeling of skeletal muscle microvasculature is induced by simulated microgravity. Am. J. Physiol. Heart Circ. Physiol. 278, H1866–H1873. doi: 10.1152/ajpheart.2000.278.6.H1866
Eskildsen-Helmond, Y. E. G., and Mulvany, M. J. (2003). Pressure-induced activation of extracellular signal-regulated kinase 1/2 in small arteries. Hypertension 41, 891–897. doi: 10.1161/01.HYP.0000058701.11991.C4
Fleming, W. W. (1999). Cellular adaptation: journey from smooth muscle cells to neurons. J. Pharmacol. Exp. Ther. 291, 925–931.
Folkow, B. (1982). Physiological aspects of primary hypertension. Physiol. Rev. 62, 347–504. doi: 10.1152/physrev.1982.62.2.347
Fu, Q., Levine, B. D., Pawelczyk, J. A., Ertl, A. C., Diedrich, A., Cox, J. F., et al. (2002). Cardiovascular and sympathetic neural responses to handgrip and cold pressor stimuli in humans before, during and after spaceflight. J. Physiol. 544, 653–664. doi: 10.1113/jphysiol.2002.025098
Gabrielsen, A., Norsk, P., Videbaek, R., and Henriksen, O. (1995). Effect of microgravity on forearm subcutaneous vascular resistance in humans. J. Appl. Physiol. 79, 434–438. doi: 10.1152/jappl.1995.79.2.434
Globus, R. K., and Morey-Holton, E. (2016). Hindlimb unloading: rodent analog for microgravity. J. Appl. Physiol. 120, 1196–1206. doi: 10.1152/japplphysiol.00997.2015
Heaps, C. L., and Bowles, D. K. (2002). Nonuniform changes in arteriolar myogenic tone within skeletal muscle following hindlimb unweighting. J. Appl. Physiol. 92, 1145–1151. doi: 10.1152/japplphysiol.01031.2000
Herault, S., Fomina, G., Alferova, I., Kotovskaya, A., Poliakov, V., and Arbeille, P. (2000). Cardiac, arterial and venous adaptation to weightlessness during 6-month MIR spaceflights with and without thigh cuffs (bracelets). Eur. J. Appl. Physiol. 81, 384–390. doi: 10.1007/s004210050058
Heumann, P., Koenen, A., Zavaritskaya, O., Schütze, K., Ramm, A., Schlüter, T., et al. (2016). Sympathetic denervation facilitates L-type Ca2+ channel activation in renal but not in mesenteric resistance arteries. J. Hypertens. 34, 692–703. doi: 10.1097/HJH.0000000000000856
Jänig, W., and Häbler, H.-J. (2003). Neurophysiological analysis of target-related sympathetic pathways--from animal to human: similarities and differences. Acta Physiol. Scand. 177, 255–274. doi: 10.1046/j.1365-201X.2003.01088.x
Jänig, W., and McLachlan, E. M. (1992). Specialized functional pathways are the building blocks of the autonomic nervous system. J. Auton. Nerv. Syst. 41, 3–13. doi: 10.1097/00005344-199321001-00003
Langille, B. L. (1993). Remodeling of developing and mature arteries: endothelium, smooth muscle, and matrix. J. Cardiovasc. Pharmacol. 21, S11–S17. doi: 10.1097/00005344-199321001-00003
McDonald, K. S., Delp, M. D., and Fitts, R. H. (1992). Effect of hindlimb unweighting on tissue blood flow in the rat. J. Appl. Physiol. 72, 2210–2218. doi: 10.1152/jappl.1992.72.6.2210
Monos, E., Lóránt, M., and Fehér, E. (2001). Influence of long-term experimental orthostatic body position on innervation density in extremity vessels. Am. J. Physiol. Heart Circ. Physiol. 281, H1606–H1612. doi: 10.1152/ajpheart.2001.281.4.H1606
Mulvany, M. J., and Halpern, W. (1977). Contractile properties of small arterial resistance vessels in spontaneously hypertensive and normotensive rats. Circ. Res. 41, 19–26. doi: 10.1161/01.RES.41.1.19
Platts, S. H., Martin, D. S., Stenger, M. B., Perez, S. A., Ribeiro, L. C., Summers, R., et al. (2009). Cardiovascular adaptations to long-duration head-down bed rest. Aviat. Space Environ. Med. 80, A29–A36. doi: 10.3357/asem.br03.2009
Pourageaud, F., and De Mey, J. G. R. (1997). Structural properties of rat mesenteric small arteries after 4-wk exposure to elevated or reduced blood flow. Am. J. Physiol. Heart Circ. Physiol. 273, H1699–H1706. doi: 10.1152/ajpheart.1997.273.4.h1699
Puzdrova, V. A., Kargina-Terent’eva, R. A., and Tarasova, O. S. (2009). Effects of chronic hypotension on the adrenergic nervous plexus of the saphenous artery in rats and its regeneration after femoral nerve injury. Neurosci. Behav. Physiol. 39, 757–761. doi: 10.1007/s11055-009-9194-7
Puzdrova, V. A., Kudryashova, T. V., Gaynullina, D. K., Mochalov, S. V., Aalkjaer, C., Nilsson, H., et al. (2014). Trophic action of sympathetic nerves reduces arterial smooth muscle Ca(2+) sensitivity during early post-natal development in rats. Acta Physiol. 212, 128–141. doi: 10.1111/apha.12331
Rodionov, I. M., Timin, E. N., Matchkov, V. V., Tarasova, O. S., and Vinogradova, O. L. (1999). An experimental study and mathematical simulation of adrenergic control of hindlimb vessels in rats after 3-week tail suspension. Environ. Med. 43, 1–9.
Slovut, D. P., Mehta, S. H., Dorrance, A. M., Brosius, F. C., Watts, S. W., and Webb, R. C. (2004). Increased vascular sensitivity and connexin43 expression after sympathetic denervation. Cardiovasc. Res. 62, 388–396. doi: 10.1016/j.cardiores.2003.12.024
Stabley, J. N., Dominguez, J. M., Dominguez, C. E., Mora Solis, F. R., Ahlgren, J., Behnke, B. J., et al. (2012). Spaceflight reduces vasoconstrictor responsiveness of skeletal muscle resistance arteries in mice. J. Appl. Physiol. 113, 1439–1445. doi: 10.1152/japplphysiol.00772.2012
Stabley, J. N., Prisby, R. D., Behnke, B. J., and Delp, M. D. (2013). Chronic skeletal unloading of the rat femur: mechanisms and functional consequences of vascular remodeling. Bone 57, 355–360. doi: 10.1016/j.bone.2013.09.003
Sun, B., Zhang, L.-F., Gao, F., Ma, X.-W., Zhang, M.-L., Liu, J., et al. (2004). Daily short-period gravitation can prevent functional and structural changes in arteries of simulated microgravity rats. J. Appl. Physiol. 97, 1022–1031. doi: 10.1152/japplphysiol.00188.2004
Taki, N., Tanaka, T., Zhang, L., Suzuki, F., Israilova, M., Taniguchi, T., et al. (2004). Alpha-1D adrenoceptors are involved in reserpine-induced supersensitivity of rat tail artery. Br. J. Pharmacol. 142, 647–656. doi: 10.1038/sj.bjp.0705817
Tarasova, O. S., Puzdrova, V. A., Kalenchuk, V. U., and Koshelev, V. B. (2006). Increase in the sensitivity of the vascular smooth muscle to constrictor factors after denervation and with a decrease in blood pressure. Biophysics 51, 805–810. doi: 10.1134/S000635090605023X
Tarasova, O., Sjöblom-Widfeldt, N., and Nilsson, H. (2003). Transmitter characteristics of cutaneous, renal and skeletal muscle small arteries in the rat. Acta Physiol. Scand. 177, 157–166. doi: 10.1046/j.1365-201X.2003.01057.x
Thrasivoulou, C., and Cowen, T. (1995). Regulation of rat sympathetic nerve density by target tissues and NGF in maturity and old age. Eur. J. Neurosci. 7, 381–387. doi: 10.1111/j.1460-9568.1995.tb00333.x
Tsvirkun, D., Bourreau, J., Mieuset, A., Garo, F., Vinogradova, O., Larina, I., et al. (2012). Contribution of social isolation, restraint, and hindlimb unloading to changes in hemodynamic parameters and motion activity in rats. PLoS One 7:e39923. doi: 10.1371/journal.pone.0039923
Watenpaugh, D., and Hargens, A. (1996). “The cardiovascular system in microgravity” in Handbook of physiology vol. environmental physiology. eds. M. J. Fregly and C. M. Blatteis (Bethesda, MD: American Physiological Society), 631–674.
Wilkerson, M. K., Muller-Delp, J., Colleran, P. N., and Delp, M. D. (1999). Effects of hindlimb unloading on rat cerebral, splenic, and mesenteric resistance artery morphology. J. Appl. Physiol. 87, 2115–2121. doi: 10.1152/jappl.1999.87.6.2115
Zhang, L. F. (2001). Vascular adaptation to microgravity: what have we learned? J. Appl. Physiol. 91, 2415–2430. doi: 10.1152/jappl.2001.91.6.2415
Keywords: microgravity, hindlimb unloading, rat, small arteries, remodeling, sympathetic innervation, noradrenaline, serotonin
Citation: Tarasova OS, Kalenchuk VU, Borovik AS, Golubinskaya VO, Delp MD and Vinogradova OL (2020) Simulated Microgravity Induces Regionally Distinct Neurovascular and Structural Remodeling of Skeletal Muscle and Cutaneous Arteries in the Rat. Front. Physiol. 11:675. doi: 10.3389/fphys.2020.00675
Edited by:
Dieter Blottner, Charité – Universitätsmedizin Berlin, GermanyReviewed by:
Nicholas Gregory Jendzjowsky, University of Calgary, CanadaSatoshi Iwase, Aichi Medical University, Japan
Copyright © 2020 Tarasova, Kalenchuk, Borovik, Golubinskaya, Delp and Vinogradova. This is an open-access article distributed under the terms of the Creative Commons Attribution License (CC BY). The use, distribution or reproduction in other forums is permitted, provided the original author(s) and the copyright owner(s) are credited and that the original publication in this journal is cited, in accordance with accepted academic practice. No use, distribution or reproduction is permitted which does not comply with these terms.
*Correspondence: Olga S. Tarasova, b3N0Lm1zdUBnbWFpbC5jb20=
†Present address: Veronika O. Golubinskaya, Department of Physiology, Institute of Neuroscience and Physiology, The Sahlgrenska Academy, University of Gothenburg, Gothenburg, Sweden