- Plastic and Reconstructive Surgery Service, Department of Surgery, Memorial Sloan Kettering Cancer Center, New York, NY, United States
The lymphatic system has many functions, including macromolecules transport, fat absorption, regulation and modulation of adaptive immune responses, clearance of inflammatory cytokines, and cholesterol metabolism. Thus, it is evident that lymphatic function can play a key role in the regulation of a wide array of biologic phenomenon, and that physiologic changes that alter lymphatic function may have profound pathologic effects. Recent studies have shown that obesity can markedly impair lymphatic function. Obesity-induced pathologic changes in the lymphatic system result, at least in part, from the accumulation of inflammatory cells around lymphatic vessel leading to impaired lymphatic collecting vessel pumping capacity, leaky initial and collecting lymphatics, alterations in lymphatic endothelial cell (LEC) gene expression, and degradation of junctional proteins. These changes are important since impaired lymphatic function in obesity may contribute to the pathology of obesity in other organ systems in a feed-forward manner by increasing low-grade tissue inflammation and the accumulation of inflammatory cytokines. More importantly, recent studies have suggested that interventions that inhibit inflammatory responses, either pharmacologically or by lifestyle modifications such as aerobic exercise and weight loss, improve lymphatic function and metabolic parameters in obese mice. The purpose of this review is to summarize the pathologic effects of obesity on the lymphatic system, the cellular mechanisms that regulate these responses, the effects of impaired lymphatic function on metabolic syndrome in obesity, and the interventions that may improve lymphatic function in obesity.
Introduction
Lymphatic Anatomy and Physiology
The lymphatics are a component of the vascular system and are present only in vertebrate animals. The lymphatic system is comprised of open-ended initial or capillary lymphatic vessels that drain successively into larger collecting lymphatics. Capillary lymphatic vessels comprise of single layer of overlapping oak-leaf shaped lymphatic endothelial cells (LECs) with interendothelial gaps, discontinuous button-like junctions that enable interstitial fluid absorption and leukocyte entry. Collecting lymphatic vessels (CLVs) are surrounded by basement membrane, covered with lymphatic muscle cells (LMC) and contain bileaflet intraluminal valves that prevent lymph back flow. Unlike capillary lymphatic vessels CLVs exhibit zipper-like interendothelial junctions to prevent lymph leakage. The contractile activity of LMCs of CLVs help actively pump interstitial fluid and drain into lymph nodes that then filter the fluid for antigens and bacteria (Alitalo et al., 2005; Baluk et al., 2007; Tammela and Alitalo, 2010; Wang et al., 2017). Efferent lymphatics drain from lymph nodes into larger CLVs that eventually return the interstitial fluid back into the blood circulation (Petrova and Koh, 2018; Liu and Oliver, 2019). In this manner, the lymphatic system plays a key role in regulating fluid homeostasis, immune cell migration and antigen presentation, resolution of inflammatory responses, and regulation of peripheral tolerance to self-antigens (Angeli and Randolph, 2006; Kim et al., 2012; Betterman and Harvey, 2016; Randolph et al., 2017).
The earliest observation of lymphatic vessels was made based on the ability of intestinal lymphatic vessels to uptake lipids after a fat-rich meal. In 300 B.C., ancient Greeks observed milk-filled intestinal lymphatics and provided the first anatomic description of the lymphatic system (Lord, 1968). In the 17th Century, Gaspero Aselli identified these milk-filled vessels as components of the vascular system (Asellius, 1627). In the last few decades, the discovery of lymphatic-specific markers helped to differentiate lymphatic vessels from the blood vasculature, and to identify the vital role of the lymphatic system in absorption of dietary fat and cholesterol transport. The intestinal lymphatics are known as lacteals and are located centrally within each intestinal villus. Dietary fats are converted by enterocytes into triglyceride-rich lipoproteins enveloped by proteins and cholesterol, which are called chylomicrons, and absorbed and transported by the intestinal lacteals by both passive and active contractile mechanisms (Choe et al., 2015; Escobedo and Oliver, 2017; Suh et al., 2018; Cifarelli and Eichmann, 2019; Hokkanen et al., 2019). Intestinal lacteals can also absorb fat-soluble vitamins. Once taken up by the lacteals, chylomicrons are transported by lymphatic vessels and drained into the systemic venous circulation, bypassing the portal venous system. This is important since bypassing the liver decreases first-pass metabolism of dietary compounds and is, as a result, an active area of research in the pharmaceutical industry.
Peripheral lymphatics also play a role in cholesterol transport by a mechanism known as reverse cholesterol transport (RCT). In this process, cholesterol molecules deposited in peripheral tissues are released by cells facilitated by lipid free apolipoprotein A1 (APOA1) or lipidated high-density lipoprotein (HDL). This extracellular cholesterol is transported by the lymphatic system back to the blood stream and liver, where they are excreted or processed. This mechanism is thought to play an important role in regulating pathological processes such as atherosclerosis. Thus, understanding how the lymphatics regulate this process may facilitate development of novel therapies for treatment of cardiovascular diseases (Lim et al., 2013; Martel et al., 2013; Huang et al., 2015).
Defects in lymphatic development or function resulting from a variety of genetic defects result in adipose deposition, changes in cholesterol metabolism, metabolic changes, and, in some cases, adult-onset obesity depending on the degree of lymphatic structural and functional abnormality (Karkkainen et al., 2001; Gale et al., 2002; Harvey et al., 2005; Dellinger et al., 2007, 2008). These findings suggest that the lymphatic system and obesity are related, and that this interaction is bi-directional.
Previous reviews covered several aspects of obesity and its implication on lymphatic vessels in relation to different pathologies like lymphedema and metabolic disorders (Escobedo and Oliver, 2017; Jiang et al., 2019; Ho and Srinivasan, 2020). This article emphasizes on the bidirectional interaction between obesity and lymphatic function. We highlighted how inflammatory pathologic changes of obesity in the form of peri-lymphatic inflammation adversely affect lymphatic structure, function and how these changes predispose obese individuals to lymphedema and metabolic complications. We also discussed how life style and pharmacological interventions mitigate inflammation to improve lymphatic function in obesity.
Obesity Causes Lymphatic Dysfunction and Increases Risk of Lymphedema
The etiology of obesity is, in most cases, related to excess calorie consumption and limited caloric expenditure, however, in rare cases, obesity may also be caused by genetic abnormalities. Obesity is a systemic disease affecting virtually every organ system and increases the risk of developing metabolic syndrome and a variety of malignancies (Barton et al., 2012; Barton, 2013; Flegal et al., 2013; Segula, 2014; Kim et al., 2016).
Recent studies have also shown that obesity and adipose related disorders have significant negative effects on the lymphatic system. For example, clinical studies have demonstrated that obese individuals have impaired subcutaneous clearance of macromolecules (Arngrim et al., 2013). Patients with Dercum’s disease, a poorly understood disorder characterized by accumulation of subcutaneous adipose tissue and painful lipomas, have structural and functional lymphatic defects (Rasmussen et al., 2014). Lipedema, another subcutaneous adipose tissue disorder with excessive fat deposition under skin, exhibits lymphatic vessel microaneurysms causing leakage of lipids from damaged and leaky lymphatic vessels causing excessive adipogenesis (Stallworth et al., 1974; Herbst, 2000; Amann-Vesti et al., 2001; Al-Ghadban et al., 2019). Reports indicate that lymphatic transport defects are more prominent in late stage lipedema compared to early stages (Gould et al., 2020). In similar lines, research indicates that lymphedema a chronic, morbid disease characterized by tissue swelling, accumulation of fluids, fibro-adipose tissue spontaneously develops in severely obese individuals. Early research showed the occurrence of massive localized lymphedema in morbidly obese individuals (Farshid and Weiss, 1998). Furthermore, several studies show that obesity is a well-recognized risk factor and body mass index (BMI) has a direct correlation with development of spontaneous as well as secondary lymphedema following lymph node dissection for cancer treatment (Swenson et al., 2009; Greene et al., 2012; Greene and Maclellan, 2013; Mehrara and Greene, 2014; Maclellan et al., 2017). This is supported and likely related to the fact that baseline lymphatic function is a predictor of lymphedema development and severity of disease (Hespe et al., 2019). As a result, obese patients are 2- to 3-fold more likely to develop lymphedema after surgery (Clark et al., 2005; Arrault and Vignes, 2006; Helyer et al., 2010). Even postoperative weight gain (and, presumably, the altered lymphatic function that ensues) increases the risk of lymphedema development (McLaughlin et al., 2008). Finally, recent studies have demonstrated that weight loss is an effective means of treating lymphedema and decreasing its symptoms (Shaw et al., 2007; Ridner et al., 2011), however, not in the case of dercum’s disease, in which lipomas still exist despite weight loss (Herbst and Asare-Bediako, 2007).
Animal Models Reveal That Obesity Results in Structural and Functional Changes in the Lymphatic System
Animal models of obesity have been used by several groups to study the cellular mechanisms that regulate development of obesity-induced lymphatic dysfunction. A commonly used mouse model is high fat diet (HFD)-induced obesity. This model is thought to reflect changes that occur in spontaneous obesity (in contrast to genetically induced models of obesity) and requires feeding adult animals a diet in which 60–70% of the total calories are derived from fat. Typically, adult male C57BL/6J mice are maintained on an HFD for 10–12 weeks and are compared to littermates fed a normal chow diet (30% calories from fat) (Savetsky et al., 2014). Male mice are much more prone to HFD-induced obesity and are used by most researchers. Female mice also gain weight following prolonged exposure to HFD, but to a lesser extent than male mice with over all less body fat levels; however, this process can be accelerated by ovariectomy. Ovariectomized female mice gained weight similar to male mice indicating that ovarian hormones might have a protective effect on female mice in weight gain (Hong et al., 2009). Report indicates that, 10–12 weeks of HFD feeding regime showed higher the blood glucose, insulin, liver enzymes, plasma lipids, cholesterol, adipose tissue macrophages, and circulating leukocytes in male mice compared to female (Singer et al., 2015; Ingvorsen et al., 2017). However, long term HFD regime (24 weeks) results in no differences between male and female in any of the parameters mentioned above indicating duration of high fat feeding is critical in exhibition of sexual dimorphism in HFD induced obesity (Bruder-Nascimento et al., 2017). Most common mice strains like C57BL/6J, FVB/N, 129 × 1, DBA/2 are prone for obesity upon HFD feeding but BALB/c mice strain is found to show certain degree of resistance to obesity even after HFD feeding (Montgomery et al., 2013). Other studies have used genetically induced models of obesity such as leptin deficient mice (Zhang et al., 1994). These animals become obese spontaneously, regardless of the diet, but are less representative of obesity clinically, since only a small percentage of obese patients have genetic abnormalities.
Studies in HFD-induced obese mice have consistently shown that obese animals have decreased lymphatic density in subcutaneous tissues, reduced LEC proliferation, increased lymphatic leakiness of both initial and collecting lymphatics, decreased collecting-vessel pumping capacity, and impaired clearance of macromolecules (Weitman et al., 2013; Blum et al., 2014; Garcia Nores et al., 2016; Torrisi et al., 2016). Not surprisingly, obese mice have significantly decreased trafficking of dendritic cells (DCs) from tissues to regional lymph nodes, have structurally abnormal and dilated lymphatic vessels, and have lymph node abnormalities consisting of decreased lymph node size, irregular architecture and loss of chemokine gradients (Weitman et al., 2013; Blum et al., 2014). Reports also indicated that obese mice lymph nodes showed loss of follicular pattern, T-cell zones and impaired ex vivo T-cell recall ability after in vivo sensitization with 1-fluoro-2,4-dinitrobenzene (DNFB) (Savetsky et al., 2015a; Hespe et al., 2016).
Obese Animals Have Decreased Expression of Lymphatic Genes by Isolated LECs
How does obesity cause lymphatic vessel abnormalities and decrease LEC proliferation? Several lines of evidence suggest that HFD-induced obesity results in marked alterations in LEC gene expression. Using fluorescence-activated cell sorting to isolate LECs from dermal samples, studies in our lab have shown that obese mice have downregulated expression of LEC genes including lymphatic vessel endothelial hyaluronan receptor 1 (LYVE-1), prospero-related homeobox 1 (PROX-1), podoplanin, and vascular endothelial growth factor receptor 3 (VEGFR-3) (Garcia Nores et al., 2016; Hespe et al., 2016; Nitti et al., 2016). It is well known that, the transcription factor PROX-1 is the master regulator of LEC differentiation and VEGFR-3 is the main receptor for vascular endothelial growth factor C (VEGF-C) and vascular endothelial growth factor D (VEGF-D), the most important lymphangiogenic growth factors. Binding of VEGFR-3 by VEGF-C/D results in activation of downstream signaling pathways that are key regulators of LEC proliferation, differentiation, and protection from apoptosis. Thus, decreases in VEGFR-3 transcription and cell surface expression in LECs of obese animals are likely important mechanisms by which obesity decreases lymphatic function. This hypothesis is supported by the fact that expression of VEGF-C is increased in the tissues and serum of obese mice and patients (Silha et al., 2005; Garcia Nores et al., 2016; Zafar et al., 2018). This increased expression of the receptor ligand VEGF-C may represent a homeostatic effect to maintain VEGFR-3 activation. Such a homeostatic regulation of insulin secretion by pancreatic β-cells in response to decreased insulin receptors or vice versa is observed in diabetic individuals (Zick, 2005; Rhodes et al., 2013). Additional research is clearly needed to determine how obesity modulates the VEGFR-3/VEGF-C signaling axis, and how these changes translate to lymphatic abnormalities in vivo.
Obesity-Induced Inflammation and Lymphatic Function
Chronic, low-grade inflammation is a key cellular mechanism that regulates the pathophysiology of obesity in a variety of organ systems. This phenomenon also appears to hold true for the effects of obesity on the lymphatic system. For example, several studies from our lab have shown that HFD-induced obesity results in peri-lymphatic accumulation of leukocytes (CD45+ cells) (Garcia Nores et al., 2016; Torrisi et al., 2016). Interestingly, we found that not only do obese mice have increased generalized low-grade inflammation in their subcutaneous fat, but that these inflammatory cells tended to accumulate around lymphatic vessels (both capillary and collecting lymphatics) (Savetsky et al., 2015a; Torrisi et al., 2016; Ariyagunarajah and Chen, 2017). This peri-lymphatic inflammatory response was mixed in nature consisting of CD11b+/inducible nitric oxide synthase (iNOS)+ macrophages and CD4+ T-lymphocytes. Although the mechanisms regulating the peri-lymphatic clustering of inflammatory cells remain unknown, some authors have shown changes in expression patterns of chemokines such as C-C motif chemokine ligand 21 (CCL21) (Weitman et al., 2013). Gradients of CCL21 regulate inflammatory cell migration into lymphatics, suggesting that the loss of these gradients in obese mice may result in trapping of inflammatory cells in tissues (Johnson and Jackson, 2010; Tal et al., 2011; Russo et al., 2016). In addition, CLV permeability is reported to facilitate peri-nodal adipose tissue inflammation. CLVs leak lymph born antigens enabling sampling by adipose tissue DCs and T cell recruitment during recall responses (Kuan et al., 2015). Furthermore, leaky CLVs facilitate severe adipose deposition, chronic inflammation and tertiary lymphoid organ formation in mesentery of Crohn’s disease patients aggravating the disease pathology (Randolph et al., 2016). Another mechanism that may contribute to white adipose tissue inflammation is increased expression of growth factors such as vascular endothelial growth factor A (VEGF-A) and VEGF-C in obese individuals (Miyazawa-Hoshimoto et al., 2003; Tinahones et al., 2012; Zafar et al., 2018). VEGF-C is highly chemotactic for myeloid-derived cells such as macrophages (Skobe et al., 2001; Nurmi et al., 2015; Shew et al., 2018). High levels of VEGF-C can also increase blood and lymphatic leakiness, macrophage infiltration and may impair lymphatic function in a feed forward manner (Jeon et al., 2008; Gousopoulos et al., 2017). An interesting study demonstrated that blockade of VEGF-C is an effective means of preventing development of insulin resistance by decreasing macrophage chemotaxis to subcutaneous tissues (Karaman et al., 2015).
A recent study reported that enhancing adipose tissue-specific lymphangiogenesis not only improves adipose tissue lymphatic function but also decreases metabolic abnormalities (Chakraborty et al., 2019). In this study, a novel mouse model of adipose tissue-specific overexpression of VEGF-D (Adipo-VD mice) was created, resulting in abundant functional lymphangiogenesis in white adipose tissue. Adipo-VD mice fed an HFD had improved glucose clearance, lower insulin levels, and reduced triglycerides from the liver as compared to controls. Functionally, these mice exhibited higher glycerol flux from adipose tissue and decreased number of macrophage-positive crown-like structures as compared to controls suggesting that these animals had improved immune cell trafficking from adipose tissue. This study therefore suggests that the effects of lymphangiogenic growth factor expression are context dependent and variable depending on the target cell (i.e., adipocyte versus inflammatory cells).
Several lines of evidence suggest that peri-lymphatic inflammatory responses play a role in the development of obesity-induced lymphatic dysfunction. For example, mice deficient in CD4+ cells (CD4 knockout), athymic nude mice (deficient in T cells), or Rag-1 mice (deficient in both T and B cells) have decreased peri-lymphatic inflammation after prolonged exposure to HFD and have improved lymphatic function as compared with similarly fed wild-type controls (Weitman et al., 2013; Torrisi et al., 2016). Topical application of tacrolimus, an IL-2 inhibitor that decreases T cell proliferation and differentiation, results in decreased lymphatic leakiness and improved collecting lymphatic pumping capacity in obese mice (Torrisi et al., 2016). Tacrolimus applied topically poorly absorbed into systemic circulation and the improvements in lymphatic function were only limited to the site of drug delivery, suggesting that local changes in inflammation rather than systemic effects of obesity were responsible for obesity-induced lymphatic leakiness and abnormal pumping. This hypothesis is supported by the fact that there was no difference in total body weight or adipose tissue weights in animals treated with topical tacrolimus versus controls. However, topical tacrolimus treatment significantly reduced the number of CD4+ T cells and related inflammation locally, correlating with the increased lymphatic function. Thus, neutralizing T cell inflammation seems to have a strong therapeutic value to enhance lymphatic function in obesity. In fact, previous research demonstrated that neutralization of CD3+ T cells with systemic antibody therapy decreases metabolic syndrome and insulin resistance in obese mice (Winer et al., 2009). It will be interesting to see how systemic CD3+ cell neutralization effects lymphatic function in obesity.
Behavioral modifications that decrease inflammation also improve lymphatic function. It is well established that lifestyle modifications such as caloric restriction or aerobic exercise training significantly decrease white adipose tissue, skeletal muscle inflammation, iNOS, and release of inflammatory cytokines (Bruun et al., 2006; Bradley et al., 2008; Kawanishi et al., 2010; Baynard et al., 2012; Kawanishi et al., 2013). These interventions also improve glucose intolerance and insulin sensitivity, decrease adipose tissue inflammation and adipokine production, and improve cardiac function (Sjostrom et al., 2000; Poirier et al., 2003; Clement et al., 2004; Sjostrom et al., 2004; Moschen et al., 2010). More recently, it has become clear that behavioral modifications also improve lymphatic function. For example, HFD-induced obese mice that underwent a 6-week course of aerobic exercise training that did not cause weight loss but did decrease white adipose tissue inflammation had significantly improved lymphatic function (decreased leakiness, improved pumping) as compared with sedentary obese controls (Hespe et al., 2016). These findings are interesting, as they suggest that lymphatic dysfunction in obesity is regulated by paracrine responses rather than direct effects of dietary toxins or physical compression of lymphatic vessels by enlarged adipocytes. In another study, caloric restriction of obese mice not only resulted in weight loss but also decreased white adipose tissue inflammation and restored lymphatic function to near normal levels (Nitti et al., 2016). This study also demonstrated a threshold effect from weight gain (weight > 40 g) beyond which lymphatic functional deficits become more pronounced and measurable. These findings are important because they suggest that obesity-induced lymphatic dysfunction is reversible (at least partially), and that behavioral modifications or pharmaceutical interventions that decrease inflammation may aid in this process.
Peri-lymphatic inflammatory cells, particularly macrophages, strongly express iNOS (Mattila et al., 2013; Xue et al., 2018). It has been reported that, collecting lymphatic pumping is regulated by gradients of NO and, under normal circumstances, NO production around lymphatic vessels is secondary to the expression of endothelial nitric oxide synthase (eNOS) (Berg and Scherer, 2005; Lahdenranta et al., 2009; Liao et al., 2011; Mauricio et al., 2013; Iantorno et al., 2014). Valvular and tubular lymphatic segments increase NOS expression during phasic contractions in turn regulate lymphatic contraction and relaxation (Bohlen et al., 2009). These findings suggest that the loss of NO gradients around collecting lymphatics due to high levels of iNOS expression by perilymphatic inflammatory cells impairs lymphatic pumping and results in collecting lymphatic dilatation (a phenotype that is commonly observed). This conclusion is supported by the fact that in vivo inhibition of iNOS in obese mice using a small molecule inhibitor, 1400 W, significantly improves lymphatic pumping and overall lymphatic function (Torrisi et al., 2016), and increased levels of iNOS are known to cause abnormalities in lymphatic contractile function in a variety of pathologic settings (Liao et al., 2011; Scallan et al., 2016). On the other hand, ex vivo experimental reports suggests basal NO causes decreased contractile function and lymph flow (Scallan and Davis, 2013).
High concentrations of NO decrease lymphatic contraction frequency and amplitude of contractions (Gashev et al., 2002; Gasheva et al., 2006, 2007). High levels of iNOS and NO may also regulate lymphatic function by other mechanisms. For example, NO is an oxygen donor, and high local concentrations of this molecule may result in generation of free oxygen and free nitrogen radicals and LECs are sensitive to free radical injury (Kasuya et al., 2014). Free radicals and iNOS itself also regulate inflammatory cell migration and may promote chronic inflammatory reactions (Liang et al., 2016). Obese iNOS knockout mice have improved metabolic parameters, decreased insulin resistance, and, most importantly, decreased tissue inflammation (Perreault and Marette, 2001). Whether or not these animals also have improved lymphatic function remains to be seen, and this is a topic of active study in our laboratory.
Inflammatory cells are also a major source of cytokines that may have important effects on LECs. For example, T cells are major sources of cytokines including interferon gamma [IFN-γ, interleukin 4 (IL-4), IL-13, and transforming growth factor beta (TGF-β)]. These cytokines have potent anti-lymphatic effects, and downregulate LEC proliferation and function in vivo and in vitro (Clavin et al., 2008; Kataru et al., 2011; Savetsky et al., 2015b; Shin et al., 2015). Thus, subcutaneous tissue inflammatory responses may directly inhibit lymphangiogenesis and lymphatic function by increasing the expression of anti-lymphangiogenic cytokines.
Is It Obesity or HFD?
Is it possible that some of the lymphatic abnormalities noted in HFD-induced obese mice may be related to toxic compounds in their diet or perhaps generated by high levels of dietary fat? To study this question, we compared lymphatic function in genetically obesity-resistant [myostatin (MSTN) knockout and BALB/c mice] and obesity-prone (C57BL/6J) mice that were fed on a HFD for prolonged periods of time (Garcia Nores et al., 2016). Not surprisingly, obesity-prone mice fed an HFD progressively increased in weight and became obese after 12–14 weeks of HFD feeds. In contrast, obesity-resistant mice gained only modest amounts of weight and did not become obese. Obesity-prone mice fed an HFD had severely abnormal lymphatic function, leaky lymphatics, impaired collecting vessel pumping, and decreased LEC mRNA expression of lymphatic-specific markers, including VEGFR-3. These mice also had abnormal metabolic parameters, including hyperinsulinemia and increased serum leptin as well as accumulation of crown-like structures (macrophages engulfing enlarged adipocytes) (Boi et al., 2016). In contrast, obesity-resistant mice fed an HFD had essentially normal lymphatic function, preserved lymphatic architecture/anatomy, and normal metabolic parameters. More importantly, these mice had limited white adipose tissue inflammation and very few peri-lymphatic inflammatory cells. Taken together, these findings suggest that obesity and inflammation are both important regulators of lymphatic dysfunction, and that dietary modifications alone are insufficient to induce this effect.
Role of Adipokines and Free Fatty Acids
In addition to inflammation, obesity induces the expression of a variety of adipokines and accumulation of free fatty acids (FFAs) in adipose tissues, and several studies have suggested that these products can negatively impact lymphatic function. For example, high levels of leptin, as noted in obese individuals, strongly inhibits LEC tubule formation/proliferation, and leptin treatment results in aberrant morphological changes in human lymphatic ducts (Leal-Cerro et al., 1996; Rose et al., 2002; Sato et al., 2016). Adiponectin, a cytokine with a positive effect on obesity, is reported to be found in low levels in obese patients (Shibata et al., 2009; Nigro et al., 2014). Interestingly, adiponectin is found to have a protective effect on lymphatic vessels by LEC differentiation and viability. This is also supported by the finding that adiponectin-knockout mice show reduced lymphangiogenesis and exacerbated lymphedema phenotype (Shimizu et al., 2013).
FFAs are abundant in tissues of obese subjects, and their role in insulin resistance and inflammation in target tissues is well studied (Boden, 2008). Reports indicate that FFAs, especially stearic acid, induced significantly more blood endothelial cell apoptosis and necrosis (Harvey et al., 2010). Treatment with oleic acid caused hyperpermeability of LECs in vitro (Sawane et al., 2013). Recent studies on cultured LECs show that some FFAs are highly toxic to LECs even in low concentrations by causing dose-dependent increase in apoptosis, decreased proliferation and downregulation of PROX-1 and VEGFR-3 expression, (Garcia Nores et al., 2016). These reports indicate that increased tissue concentration of FFAs in obese subjects can directly injure the lymphatic endothelium. In addition to direct toxic effects on LECs, FFAs may interact with other molecules that regulate lymphatic vessel stability. For example, apelin, a well-known endogenous ligand orphan G protein-coupled receptor (APJ), is an important regulator of lymphatic vessel stability and integrity (Sawane et al., 2011). Apelin-knockout mice exhibit hyperpermeability and abnormal skin lymphatic function under HFD-fed conditions, due to elevated plasma FFAs and their negative effects on LECs. The lymphatic defective phenotype in Apelin-knockout mice is reversed in Apelin-transgenic mice with skin-specific overexpression of apelin (K14-Apelin), indicating the protective role of apelin against FFA-induced damage (Sawane et al., 2013).
Lymphatic Dysfunction Causes Adipose Deposition
Lymphatic Fluid Causes Adipocyte Maturation and Lipid Accumulation
While it is clear that obesity causes lymphatic dysfunction, it is equally clear that lymphatic dysfunction can cause alterations in adipocyte biology and contribute to local adiposity (Figure 1). Indirect evidence for this hypothesis can be derived from adipose deposition in patients with lymphedema. Lymphedema is a disease that occurs as a result of genetic or acquired abnormalities of the lymphatic system secondary to infections, trauma, or cancer treatment. While early stage lymphedema is characterized by accumulation of interstitial fluid and pitting edema, late stages of the disease result in chronic and progressive adipose tissue deposition, often resulting in massive tissue distortion (Rockson, 2001, 2018; Warren et al., 2007; Mehrara and Greene, 2014). Lipedema, a less well-defined disease resulting in abnormal adipose deposition, has also been shown to be associated with functional alterations in the lymphatic system (Harwood et al., 1996). Lymphatic injury results in expression of IL-6, a broad inflammation marker and an important regulator of adipose tissue homeostasis in lymphedema (Cuzzone et al., 2014). Lymphatic injury in mouse models results in remodeling of adipose tissues and increased expression of adipocyte differentiation genes such as CCAAT/enhancer-binding protein-alpha (CEBP-α), adiponectin, peroxisome proliferator-activated receptor-gamma (PPAR-γ) (Rutkowski et al., 2010; Aschen et al., 2012; Zampell et al., 2012; Tashiro et al., 2017). Other experiments using a rat model in which collecting lymphatics are ligated have shown that insufficient lymphatic drainage results in abnormal lipid accumulation along the vein walls, causing chronic venous insufficiency (Tanaka et al., 2016). These findings are supported by in vitro experiments demonstrating that addition of lymphatic fluid (chylous fluid) to culture media of preadipocytes results in cellular differentiation, mature adipocyte gene expression (e.g., PPAR-γ, GLUT-4), and intracellular lipid accumulation (Harvey et al., 2005). More recent studies suggest that FFAs including oleic acid, α-linoleic acid, and palmitic acid are the components of lymphatic fluid that induce adipocyte maturation and differentiation (Escobedo et al., 2016). Taken together, these studies suggest that lymphatic injury causes activation of adipocytes and accumulation of adipose tissues.
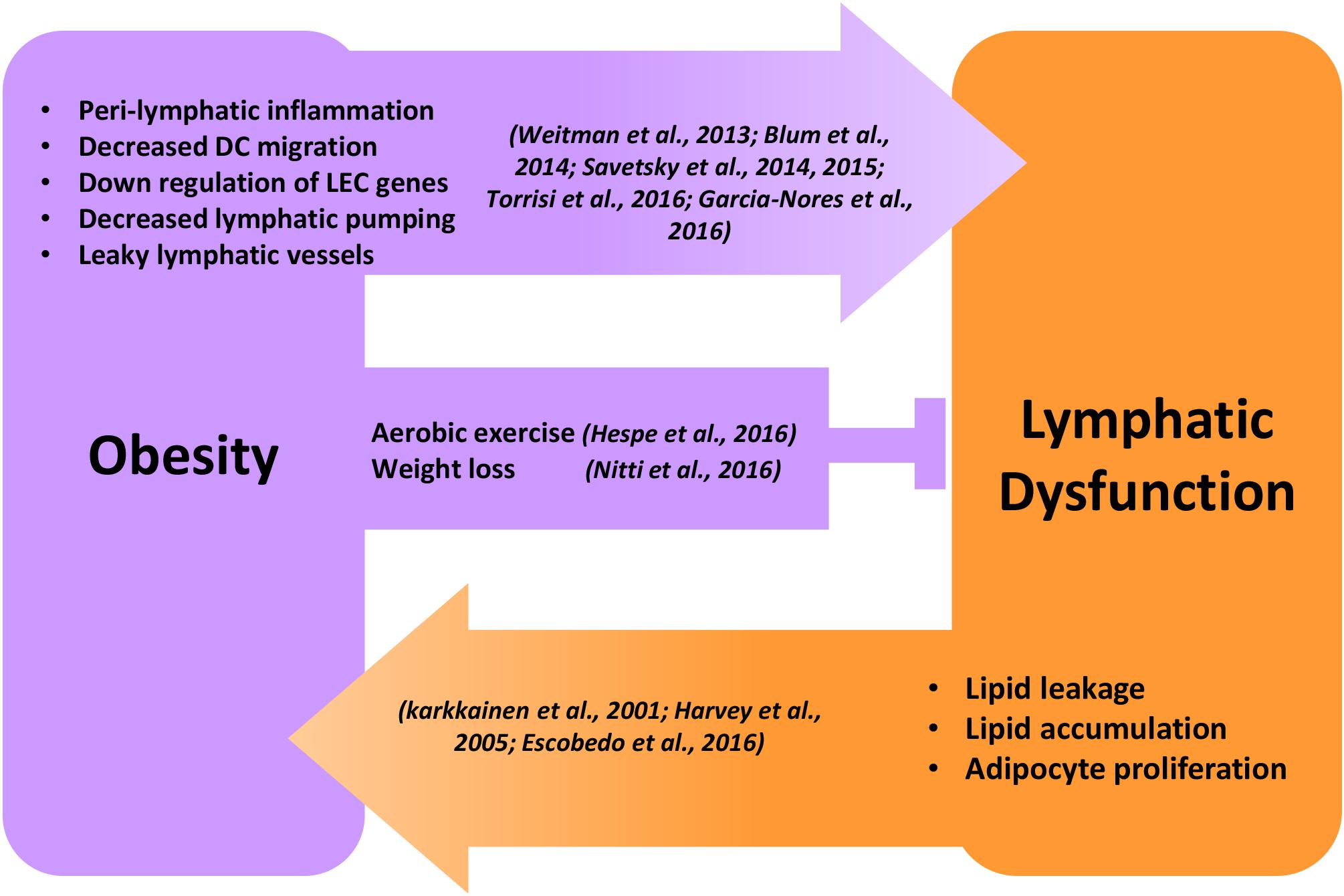
Figure 1. Reciprocal regulation of obesity and lymphatic dysfunction. Schematic illustration showing the reciprocal regulation of obesity and lymphatic dysfunction. Peri-lymphatic inflammation in obesity causes decreased lymphatic pumping, DC migration, LEC gene expression, and increased lymphatic leakage leading to lymphatic dysfunction. Behavioral interventions like aerobic exercise and weight loss inhibit and reverse obesity-induced lymphatic dysfunction. Genetic or surgical lymphatic damage or dysfunction causes malabsorption of lipids, adipocyte proliferation, and progressive accumulation of adipose tissue, leading to obesity.
Lymphatics Are Anatomically Co-localized With Adipose Tissues
Anatomic considerations also suggest that the interaction between the lymphatics and adipose tissues is bidirectional. Throughout the body, lymphatic structures are physically located in close proximity to adipose tissues. The open-ended initial dermal lymphatic vessels are located just above the adipose tissue layer in the skin. Major CLVs and trunks are always surrounded by adipose tissues, and lymph nodes are maintained in thick fat pads even in highly lean individuals (Harvey, 2008). These fat pads serve as an energy reservoir that helps to sustain immune responses in lymph nodes (Pond and Mattacks, 1995, 1998, 2002). Surgical removal of lymph nodes postnatally causes failure of fat pad development, suggesting that there is a 2-way communication between adipocytes and the lymphatic system during development (Eberl et al., 2004).
Congenital Abnormalities of the Lymphatic System Result in Adult-Onset Obesity in Mice
Experiments using transgenic animals with lymphatic defects provide even more direct evidence that lymphatic function can regulate adipose tissue deposition. The homeobox transcription factor Prox-1 is the master regulator of lymphatic specification and identity (Wigle et al., 2002). Prox-1 null mutations are lethal, with death occurring around embryonic day 14.5. However, heterozygous mutations (Prox-1±) as well as conditional Prox-1 knockouts survive to adulthood despite anatomic and functional lymphatic abnormalities. These mice have leaky lymphatics and chylous ascites that partially resolve (Harvey et al., 2005). Interestingly, mice with heterozygous Prox-1 mutations become obese as adults (by 4 months of age) even when fed a normal chow diet. These mice display no differences in food intake but nevertheless gain significantly more weight, accumulate adipose tissues in both intra-abdominal and subcutaneous tissues, and develop metabolic abnormalities, including insulin resistance when compared with littermate controls. Restoration of lymphatic Prox-1 signaling not only rescues lymphatic function but also prevents abnormal adult-onset weight gain and metabolic abnormalities (Escobedo et al., 2016). Interestingly, although the most common clinical cause of obesity is over-nutrition rather than genetic abnormalities, recent genome-wide association studies have identified single-nucleotide polymorphisms in the Prox-1 that are linked with increased waist circumferences and obesity (Kim et al., 2013; Lecompte et al., 2013). However, more in-depth research is warranted attributing these results to lymphatic phenotype as Prox-1 is also expressed by liver and skeletal muscle which significantly contributes to metabolism and energy homeostasis.
Other mouse models with genetic abnormalities of the lymphatic system that cause lymphatic dysfunction also display abnormal adipose deposition. For example, mice with heterozygous inactivating mutations in Vegfr-3 (also known as Chy mice) develop chylous ascites post-natally that resolve spontaneously. However, these mice develop swollen feet and increased subcutaneous adipose tissue deposition as compared to wild-type mice (Karkkainen et al., 2001; Dellinger et al., 2007; Rutkowski et al., 2010). Similar findings have been reported in angiopoietin-2 (Ang-2) knockout mice. These mice have abnormal embryonic lymphatic patterning and develop adult-onset foot pad swelling due to adipose deposition (Dellinger et al., 2008).
Regulation of Metabolic Abnormalities by the Lymphatic System
Lymphatic vessel function plays a key role in regulation of wide variety of biological phenomenon, and, as a result, lymphatic abnormalities are associated with many pathologic conditions, including cancer, autoimmune disorders, transplant rejection, atherosclerosis, diabetes, myocardial infarction (MI), and hypertension, to name a few. However, in the context of this review, we will briefly discuss those pathologies that are concerned with lymphatic function and metabolic disorders.
Lymphatic Function in Hypercholesterolemia and MI
Obesity is frequently associated with hypercholesterolemia. However, many obese patients exhibit normal blood lipids, and not all hypercholesterolemia patients are obese (Miettinen, 1971; Guerrero-Romero and Rodriguez-Moran, 2006). These irregularities indicate that some other common factor might play a key role in hypercholesterolemia pathology. Accumulation of lipids in the extracellular tissues is the key feature of hypercholesterolemia. Recent studies have shown that the lymphatic system plays an important role in cholesterol metabolism by transporting cholesterol from the peripheral tissues to the liver via a process called RCT (Lim et al., 2013; Martel et al., 2013; Veronique and Ying, 2013; Huang et al., 2015). Studies using Chy mice or surgical ligation of lymphatic channels results in decreased RCT and cholesterol clearance from the tissues (Lim et al., 2013; Martel et al., 2013). The efflux of cholesterol from peripheral tissues to systemic circulation through lymphatic vessels is not passive, but an active process could dependent on uptake and transcytosis of HDL by scavenger receptor class B type 1 (SR-B1) expressed on LECs (Lim et al., 2013). This concept is supported by the fact that patients with primary lymphedema often display xanthomas (cholesterol-rich deposits in the skin) (Berger et al., 1972; Romani et al., 2012). Poor lymphatic function in primary lymphedema causing increased cholesterol accumulation might contribute to fat deposition, a major symptom of lymphedema. Impaired lymphatic function is also linked to lipoprotein metabolism, increased plasma cholesterol levels, and enhanced atherogenesis (Vuorio et al., 2014). Other studies using apolipoprotein E knockout (ApoE–/–), an important protein in fat metabolism, or low-density lipoprotein receptor knockout (Ldlr–/–) models of hypercholesterolemia have shown that these mice also have severe lymphatic dysfunction, suggesting the interaction between elevated cholesterol levels and lymphatic function may be bidirectional (Lim et al., 2009; Milasan et al., 2019). Based on these findings, RCT by lymphatic vessels from the arterial wall or atherosclerotic plaques has been discussed as a potential anti-atherogenic therapeutic target in patients with cardiovascular diseases (Milasan et al., 2015; Zheng et al., 2018; Csanyi and Singla, 2019).
The most common complication of obesity and atherosclerosis is MI (Zhu et al., 2014). It is caused by obstruction of coronary circulation, leading to cardiomyocyte loss and scar tissue accumulation culminating in heart failure. The primary event post MI is a potent innate inflammatory reaction for phagocytosis of the dead cell debris and clearance. Reports indicate that after an MI incident, there is a profound remodeling of cardiac lymphatic vessels, and that augmentation of this response by VEGF-C treatment can lead to improved cardiac function (Klotz et al., 2015). These findings are also supported by data from soluble VEGFR-3 decoy transgenic mice (sVEGFR-3) and Chy mice, which reveal that downregulation of VEGFR-3 signaling structurally alters the cardiac lymphatic vasculature and increases mortality after MI (Vuorio et al., 2018). In addition to VEGF-C, adrenomedullin, a known cardioprotective peptide, is also shown to induce cardiac lymphatic growth and function after MI (Trincot et al., 2019). Mechanistically, after MI, cardiac lymphatic vessels clear acute inflammatory immune response, help in resolution of cardiac inflammation, and inhibit scaring and fibrosis and this process is dependent on cardiac lymphatic vessel expression of LYVE-1 (Vieira et al., 2018). Taken together, improving both cardiac lymphatic regeneration and function has the promise to be a therapeutic strategy for infracted myocardium recovery.
Lymphatic Function and Diabetes
Obesity is highly associated with diabetes and chronic hyperglycemia (Al-Goblan et al., 2014). The role of lymphatic function on diabetes, and how hyperglycemia regulates lymphatic vessels, is still an active topic of investigation. Impaired wound healing is a severe complication of diabetes, and lymphatic regeneration is an integral part of wound healing; therefore, it is imperative to understand how lymphatic function influences diabetes and vice versa (Saaristo et al., 2006; Brem and Tomic-Canic, 2007; Clavin et al., 2008). Using a mouse corneal suture model, it is reported that lymphangiogenesis is significantly suppressed in a diabetic mouse model of leptin receptor deficiency (db/db). These mice also showed impaired wound healing due to defective lymphatic vessel proliferation and collateral lymphatic vessel formation (Maruyama et al., 2007). Uptake of dextran tracer from peripheral tissues to lymph nodes was decreased in alloxan-induced diabetic rats, and insulin treatment rescued this phenomenon, indicating possible decreased lymphatic function in diabetes (Moriguchi et al., 2005). Other studies analyzing gene expression pathways in LECs isolated from patients with diabetes have shown that pro-inflammatory, pro-lymphangiogenic, and lipid-shuttling gene expression pathways are increased in diabetes, while genes related to immune defense, apoptosis mediation, and small-compound transporters are downregulated (Haemmerle et al., 2013). Using cultured LECs, Lee et al. showed that prolonged hyperglycemia induced insulin resistance and these LECs showed disruption of adherent junction proteins, indicating negative effects of hyperglycemia on LECs (Lee et al., 2018). Using lymphatic muscle cells (LMCs), the same group found that hyperglycemia strongly inhibits LMC contractile function by alteration of cellular bioenergetics and activation of inflammatory signaling in lymphatic muscle. These results were supported by an elegant ex vivo study by Scallan et al. (2015) that used db/db diabetic mice to study CLV function. They found that collecting lymphatic vascular integrity is disrupted in Type 2 diabetes due to low NO bioavailability. Scallan et al. (2015) further showed that inhibition of the phosphodiesterase 3 (PDE3) enzyme, that is normally inhibited by NO signaling, restored lymphatic vascular integrity and improved lymphatic function (Alexander and Becker, 2015; Scallan et al., 2015). Taken together, these findings indicate that diabetes impairs lymphangiogenesis, lymphatic permeability, and contractile function. However, the molecular events leading to impaired lymphangiogenesis during diabetes were unknown known recently. Using a streptozotocin-induced model of diabetes in mice fed an HFD, Wu et al. (2018) found that lymphangiogenesis and lymphatic function were impaired in diabetes due to downregulation of VEGFR-3 expression on LECs. This VEGFR-3 downregulation is mediated by epsin-mediated endocytosis (Wu et al., 2018). The specific deletion of epsin in LECs not only improved lymphatic growth but also enhanced lymphatic function in HFD-fed diabetic mice. These findings reveal that diabetes-induced lymphatic dysfunction is mainly mediated by endocytosis of VEGFR-3 by epsins and depletion of epsins in a potential therapeutic target to improve lymphatic function in diabetes.
Intestinal Lacteal Vascular Endothelial-Cadherin (VE-Cadherin) Zippering
Lymphatic leakiness causes chylus leakage and obesity. Lacteals are the intestinal villi lymphatic vessels with button-like VE-cadherin junctions. Vascular factors such as VEGF-C, angiopoietin-2, and DLL4 are reported to maintain VE-cadherin junctional integrity and regeneration of lacteals (Zheng et al., 2014; Bernier-Latmani et al., 2015; Suh et al., 2019). These VE-cadherin intercellular button-like junctions allow fluid and chylomicrons into lacteals and, eventually, into blood circulation (Cifarelli and Eichmann, 2019; Hokkanen et al., 2019). Converting the lacteal LEC button junctions to zipper-like junctions prevents chylomicron entry into lacteals thus reducing lipid absorption and protection against diet-induced obesity. A study by Zhang et al. (2018) reported that endothelial-specific genetic deletion of Neurophilin-1 (Nrp-1) and vascular endothelial growth factor receptor 1 (Vegfr-1) induced lacteal VE-cadherin junction zippering and chylomicron malabsorption. Deletion of Nrp-1 and Vegfr-1 on endothelial cells increased signaling through VEGFR-2 due to increased VEGF-A bioavailability, causing zippering of the lacteal junctions. This resulted in malabsorption of the chylomicrons in these transgenic mice and turning them resistant to diet-induced obesity. As an alternative pharmacologic approach, Rho-associated protein kinase (ROCK) inhibitor Y27632 was used to induce straightening lacteal junctions, causing reduced chylomicron absorption into lacteals of wild-type mice. This novel intervention of closing lymphatic junctions by transformation of buttons to zippers is quite intriguing. However, detailed research is needed to determine the adverse effects of lacteal zippering on overall intestinal health.
Future Directions
Although considerable progress has been made in understanding the reciprocal relationship between obesity and lymphatic function, many questions still remain. One of the major topics that requires addressing is identification of the mechanisms by which obesity injures the lymphatic system. While it is clear that inflammation plays a key role in this process, the cellular pathways that mediate lymphatic dysfunction in obesity, both clinically and in mouse models, remain largely unknown. Equally important in this discussion are studies analyzing the reversibility of these changes clinically. Thus, while preclinical models indicate that anti-inflammatory medications and behavioral modifications can reverse the pathologic effects of obesity on the lymphatic system, it remains to be seen whether these abnormalities can be reversed in patients with longstanding obesity. Understanding the cellular mechanisms that regulate lymphatic function in obesity and understanding how these effects are normalized with targeted treatments, will have a profound impact on a variety of metabolic diseases, and is an urgent biomedical need.
Author Contributions
RK, BM, HP, JB, and CL performed the literature search, compiled and wrote the manuscript. HP, JB, and JS proofed the manuscript. RK and HP created the figures. All authors contributed to manuscript revision and read and approved the submitted version.
Funding
This review was supported by the NIH Grant No. RO1 HL111130-01, and the preparation of this review was supported in part by the NIH/NCI Cancer Center Support Grant No. P30CA008748 to Memorial Sloan Kettering Cancer Center. CL was supported in part by the NCI Grant No. T32CA009501.
Conflict of Interest
The authors declare that the research was conducted in the absence of any commercial or financial relationships that could be construed as a potential conflict of interest.
References
Alexander, J. S., and Becker, F. (2015). Dual signals underlying diabetic lymphatic barrier dysregulation. Cardiovasc. Res. 107, 3–4. doi: 10.1093/cvr/cvv158
Al-Ghadban, S., Cromer, W., Allen, M., Ussery, C., Badowski, M., Harris, D., et al. (2019). Dilated blood and lymphatic microvessels, angiogenesis, increased macrophages, and adipocyte hypertrophy in lipedema thigh skin and fat tissue. J. Obes. 2019:8747461. doi: 10.1155/2019/8747461
Al-Goblan, A. S., Al-Alfi, M. A., and Khan, M. Z. (2014). Mechanism linking diabetes mellitus and obesity. Diabetes Metab. Syndr. Obes. 7, 587–591. doi: 10.2147/DMSO.S67400
Alitalo, K., Tammela, T., and Petrova, T. V. (2005). Lymphangiogenesis in development and human disease. Nature 438, 946–953.
Amann-Vesti, B. R., Franzeck, U. K., and Bollinger, A. (2001). Microlymphatic aneurysms in patients with lipedema. Lymphology 34, 170–175.
Angeli, V., and Randolph, G. J. (2006). Inflammation, lymphatic function, and dendritic cell migration. Lymphat. Res. Biol. 4, 217–228. doi: 10.1089/lrb.2006.4406
Ariyagunarajah, R., and Chen, H. H. (2017). To be or not to be obese: impact of obesity on lymphatic function. J. Physiol. 595, 1449–1450. doi: 10.1113/JP273743
Arngrim, N., Simonsen, L., Holst, J. J., and Bulow, J. (2013). Reduced adipose tissue lymphatic drainage of macromolecules in obese subjects: a possible link between obesity and local tissue inflammation? Int. J. Obes. 37, 748–750. doi: 10.1038/ijo.2012.98
Arrault, M., and Vignes, S. (2006). Risk factors for developing upper limb lymphedema after breast cancer treatment. Bull. Cancer 93, 1001–1006.
Aschen, S., Zampell, J. C., Elhadad, S., Weitman, E., De Brot, M., and Mehrara, B. J. (2012). Regulation of adipogenesis by lymphatic fluid stasis: part II. Expression of adipose differentiation genes. Plast. Reconstr. Surg. 129, 838–847. doi: 10.1097/PRS.0b013e3182450b47
Baluk, P., Fuxe, J., Hashizume, H., Romano, T., Lashnits, E., Butz, S., et al. (2007). Functionally specialized junctions between endothelial cells of lymphatic vessels. J. Exp. Med. 204, 2349–2362. doi: 10.1084/jem.20062596
Barton, M. (2013). Prevention and endothelial therapy of coronary artery disease. Curr. Opin. Pharmacol. 13, 226–241. doi: 10.1016/j.coph.2013.05.005
Barton, M., Baretella, O., and Meyer, M. R. (2012). Obesity and risk of vascular disease: importance of endothelium-dependent vasoconstriction. Br. J. Pharmacol. 165, 591–602. doi: 10.1111/j.1476-5381.2011.01472.x
Baynard, T., Vieira-Potter, V. J., Valentine, R. J., and Woods, J. A. (2012). Exercise training effects on inflammatory gene expression in white adipose tissue of young mice. Mediators Inflamm. 2012:767953. doi: 10.1155/2012/767953
Berg, A. H., and Scherer, P. E. (2005). Adipose tissue, inflammation, and cardiovascular disease. Circ. Res. 96, 939–949. doi: 10.1089/met.2007.0034
Berger, B. W., Kantor, I., and Maier, H. S. (1972). Xanthomatosis and lymphedema. Arch. Dermatol. 105, 730–731.
Bernier-Latmani, J., Cisarovsky, C., Demir, C. S., Bruand, M., Jaquet, M., Davanture, S., et al. (2015). DLL4 promotes continuous adult intestinal lacteal regeneration and dietary fat transport. J. Clin. Invest. 125, 4572–4586. doi: 10.1172/JCI82045
Betterman, K. L., and Harvey, N. L. (2016). The lymphatic vasculature: development and role in shaping immunity. Immunol. Rev. 271, 276–292. doi: 10.1111/imr.12413
Blum, K. S., Karaman, S., Proulx, S. T., Ochsenbein, A. M., Luciani, P., Leroux, J. C., et al. (2014). Chronic high-fat diet impairs collecting lymphatic vessel function in mice. PLoS One 9:e94713. doi: 10.1371/journal.pone.0094713
Bohlen, H. G., Wang, W., Gashev, A., Gasheva, O., and Zawieja, D. (2009). Phasic contractions of rat mesenteric lymphatics increase basal and phasic nitric oxide generation in vivo. Am. J. Physiol. Heart Circ. Physiol. 297, H1319–H1328. doi: 10.1152/ajpheart.00039.2009
Boi, S. K., Buchta, C. M., Pearson, N. A., Francis, M. B., Meyerholz, D. K., Grobe, J. L., et al. (2016). Obesity alters immune and metabolic profiles: New insight from obese-resistant mice on high-fat diet. Obesity 24, 2140–2149. doi: 10.1002/oby.21620
Bradley, R. L., Jeon, J. Y., Liu, F. F., and Maratos-Flier, E. (2008). Voluntary exercise improves insulin sensitivity and adipose tissue inflammation in diet-induced obese mice. Am. J. Physiol. Endocrinol. Metab. 295, E586–E594. doi: 10.1152/ajpendo.00309.2007
Brem, H., and Tomic-Canic, M. (2007). Cellular and molecular basis of wound healing in diabetes. J. Clin. Invest. 117, 1219–1222. doi: 10.1172/JCI32169
Bruder-Nascimento, T., Ekeledo, O. J., Anderson, R., Le, H. B., Belin, and De Chantemèle, E. J. (2017). Long term high fat diet treatment: an appropriate approach to study the sex-specificity of the autonomic and cardiovascular responses to obesity in mice. Front. Physiol. 8:32. doi: 10.3389/fphys.2017.00032
Bruun, J. M., Helge, J. W., Richelsen, B., and Stallknecht, B. (2006). Diet and exercise reduce low-grade inflammation and macrophage infiltration in adipose tissue but not in skeletal muscle in severely obese subjects. Am. J. Physiol. Endocrinol. Metab. 290, E961–E967. doi: 10.1152/ajpendo.00506.2005
Chakraborty, A., Barajas, S., Lammoglia, G. M., Reyna, A. J., Morley, T. S., Johnson, J. A., et al. (2019). Vascular endothelial growth factor-D (VEGF-D) overexpression and lymphatic expansion in murine adipose tissue improves metabolism in obesity. Am. J. Pathol. 189, 924–939. doi: 10.1016/j.ajpath.2018.12.008
Choe, K., Jang, J. Y., Park, I., Kim, Y., Ahn, S., Park, D. Y., et al. (2015). Intravital imaging of intestinal lacteals unveils lipid drainage through contractility. J. Clin. Invest. 125, 4042–4052. doi: 10.1172/JCI76509
Cifarelli, V., and Eichmann, A. (2019). The intestinal lymphatic system: functions and metabolic implications. Cell Mol. Gastroenterol. Hepatol. 7, 503–513. doi: 10.1016/j.jcmgh.2018.12.002
Clark, B., Sitzia, J., and Harlow, W. (2005). Incidence and risk of arm oedema following treatment for breast cancer: a three-year follow-up study. QJM 98, 343–348. doi: 10.1093/qjmed/hci053
Clavin, N. W., Avraham, T., Fernandez, J., Daluvoy, S. V., Soares, M. A., Chaudhry, A., et al. (2008). TGF-beta1 is a negative regulator of lymphatic regeneration during wound repair. Am. J. Physiol. Heart Circ. Physiol. 295, H2113–H2127. doi: 10.1152/ajpheart.00879.2008
Clement, K., Viguerie, N., Poitou, C., Carette, C., Pelloux, V., Curat, C. A., et al. (2004). Weight loss regulates inflammation-related genes in white adipose tissue of obese subjects. FASEB J. 18, 1657–1669. doi: 10.1096/fj.04-2204com
Csanyi, G., and Singla, B. (2019). Arterial lymphatics in atherosclerosis: old questions, new insights, and remaining challenges. J. Clin. Med. 8:495. doi: 10.3390/jcm8040495
Cuzzone, D. A., Weitman, E. S., Albano, N. J., Ghanta, S., Savetsky, I. L., Gardenier, J. C., et al. (2014). IL-6 regulates adipose deposition and homeostasis in lymphedema. Am. J. Physiol. Heart Circ. Physiol. 306, H1426–H1434. doi: 10.1152/ajpheart.01019.2013
Dellinger, M., Hunter, R., Bernas, M., Gale, N., Yancopoulos, G., Erickson, R., et al. (2008). Defective remodeling and maturation of the lymphatic vasculature in Angiopoietin-2 deficient mice. Dev. Biol. 319, 309–320. doi: 10.1016/j.ydbio.2008.04.024
Dellinger, M. T., Hunter, R. J., Bernas, M. J., Witte, M. H., and Erickson, R. P. (2007). Chy-3 mice are Vegfc haploinsufficient and exhibit defective dermal superficial to deep lymphatic transition and dermal lymphatic hypoplasia. Dev. Dyn. 236, 2346–2355. doi: 10.1089/lrb.2007.5308
Eberl, G., Marmon, S., Sunshine, M. J., Rennert, P. D., Choi, Y., and Littman, D. R. (2004). An essential function for the nuclear receptor RORgamma(t) in the generation of fetal lymphoid tissue inducer cells. Nat. Immunol. 5, 64–73. doi: 10.1038/ni1022
Escobedo, N., and Oliver, G. (2017). The lymphatic vasculature: its role in adipose metabolism and obesity. Cell Metab. 26, 598–609. doi: 10.1016/j.cmet.2017.07.020
Escobedo, N., Proulx, S. T., Karaman, S., Dillard, M. E., Johnson, N., Detmar, M., et al. (2016). Restoration of lymphatic function rescues obesity in Prox1-haploinsufficient mice. JCI Insight 1:e85096. doi: 10.1172/jci.insight.85096.
Farshid, G., and Weiss, S. W. (1998). Massive localized lymphedema in the morbidly obese: a histologically distinct reactive lesion simulating liposarcoma. Am. J. Surg. Pathol. 22, 1277–1283. doi: 10.1097/00000478-199810000-00013
Flegal, K. M., Kit, B. K., Orpana, H., and Graubard, B. I. (2013). Association of all-cause mortality with overweight and obesity using standard body mass index categories: a systematic review and meta-analysis. JAMA 309, 71–82. doi: 10.1001/jama.2012.113905
Gale, N. W., Thurston, G., Hackett, S. F., Renard, R., Wang, Q., Mcclain, J., et al. (2002). Angiopoietin-2 is required for postnatal angiogenesis and lymphatic patterning, and only the latter role is rescued by angiopoietin-1. Dev. Cell 3, 411–423. doi: 10.1016/s1534-5807(02)00217-4
Garcia Nores, G. D., Cuzzone, D. A., Albano, N. J., Hespe, G. E., Kataru, R. P., Torrisi, J. S., et al. (2016). Obesity but not high-fat diet impairs lymphatic function. Int. J. Obes. 40, 1582–1590. doi: 10.1038/ijo.2016.96
Gashev, A. A., Davis, M. J., and Zawieja, D. C. (2002). Inhibition of the active lymph pump by flow in rat mesenteric lymphatics and thoracic duct. J. Physiol. 540, 1023–1037. doi: 10.1113/jphysiol.2001.016642
Gasheva, O. Y., Knippa, K., Nepiushchikh, Z. V., Muthuchamy, M., and Gashev, A. A. (2007). Age-related alterations of active pumping mechanisms in rat thoracic duct. Microcirculation 14, 827–839. doi: 10.1080/10739680701444065
Gasheva, O. Y., Zawieja, D. C., and Gashev, A. A. (2006). Contraction-initiated NO-dependent lymphatic relaxation: a self-regulatory mechanism in rat thoracic duct. J. Physiol. 575, 821–832. doi: 10.1113/jphysiol.2006.115212
Gould, D. J., El-Sabawi, B., Goel, P., Badash, I., Colletti, P., and Patel, K. M. (2020). Uncovering lymphatic transport abnormalities in patients with primary lipedema. J. Reconstr. Microsurg. 36, 136–141. doi: 10.1055/s-0039-1697904
Gousopoulos, E., Proulx, S. T., Bachmann, S. B., Dieterich, L. C., Scholl, J., Karaman, S., et al. (2017). An important role of VEGF-C in promoting lymphedema development. J. Invest. Dermatol. 137, 1995–2004. doi: 10.1016/j.jid.2017.04.033
Greene, A. K., Grant, F. D., and Slavin, S. A. (2012). Lower-extremity lymphedema and elevated body-mass index. N. Engl. J. Med. 366, 2136–2137. doi: 10.1056/NEJMc1201684
Greene, A. K., and Maclellan, R. A. (2013). Obesity-induced Upper Extremity Lymphedema. Plast. Reconstr. Surg. Glob. Open 1:e59. doi: 10.1097/GOX.0b013e3182a96359
Guerrero-Romero, F., and Rodriguez-Moran, M. (2006). Prevalence of dyslipidemia in non-obese prepubertal children and its association with family history of diabetes, high blood pressure, and obesity. Arch. Med. Res. 37, 1015–1021. doi: 10.1016/j.arcmed.2006.06.003
Haemmerle, M., Keller, T., Egger, G., Schachner, H., Steiner, C. W., Stokic, D., et al. (2013). Enhanced lymph vessel density, remodeling, and inflammation are reflected by gene expression signatures in dermal lymphatic endothelial cells in type 2 diabetes. Diabetes Metab. Res. Rev 62, 2509–2529. doi: 10.2337/db12-0844
Harvey, K. A., Walker, C. L., Pavlina, T. M., Xu, Z., Zaloga, G. P., and Siddiqui, R. A. (2010). Long-chain saturated fatty acids induce pro-inflammatory responses and impact endothelial cell growth. Clin. Nutr. 29, 492–500. doi: 10.1016/j.clnu.2009.10.008
Harvey, N. L. (2008). The link between lymphatic function and adipose biology. Ann. N. Y. Acad. Sci. 1131, 82–88. doi: 10.1196/annals.1413.007
Harvey, N. L., Srinivasan, R. S., Dillard, M. E., Johnson, N. C., Witte, M. H., Boyd, K., et al. (2005). Lymphatic vascular defects promoted by Prox1 haploinsufficiency cause adult-onset obesity. Nat. Genet. 37, 1072–1081. doi: 10.1038/ng1642
Harwood, C. A., Bull, R. H., Evans, J., and Mortimer, P. S. (1996). Lymphatic and venous function in lipoedema. Br. J. Dermatol. 134, 1–6.
Helyer, L. K., Varnic, M., Le, L. W., Leong, W., and Mccready, D. (2010). Obesity is a risk factor for developing postoperative lymphedema in breast cancer patients. Breast J. 16, 48–54. doi: 10.1111/j.1524-4741.2009.00855.x
Herbst, K. L. (2000). “Subcutaneous adipose tissue diseases: dercum disease, lipedema, familial multiple lipomatosis, and madelung disease,” in Endotext, eds K. R. Feingold, B. Anawalt, A. Boyce, G. Chrousos, K. Dungan, A. Grossman, et al. (South Dartmouth, MA: MDText Inc).
Herbst, K. L., and Asare-Bediako, S. (2007). Adiposis dolorosa is more than painful fat. Endocrinologist 17, 326–334.
Hespe, G. E., Kataru, R. P., Savetsky, I. L., Garcia Nores, G. D., Torrisi, J. S., Nitti, M. D., et al. (2016). Exercise training improves obesity-related lymphatic dysfunction. J. Physiol. 594, 4267–4282. doi: 10.1113/JP271757
Hespe, G. E., Ly, C. L., Kataru, R. P., and Mehrara, B. J. (2019). Baseline lymphatic dysfunction amplifies the negative effects of lymphatic injury. Plast. Reconstr. Surg. 143, 77e–87e. doi: 10.1097/PRS.0000000000005091
Ho, Y. C., and Srinivasan, R. S. (2020). Lymphatic vasculature in energy homeostasis and obesity. Front. Physiol. 11:3. doi: 10.3389/fphys.2017.0003
Hokkanen, K., Tirronen, A., and Yla-Herttuala, S. (2019). Intestinal lymphatic vessels and their role in chylomicron absorption and lipid homeostasis. Curr. Opin. Lipidol. 30, 370–376. doi: 10.1097/MOL.0000000000000626
Hong, J., Stubbins, R. E., Smith, R. R., Harvey, A. E., and Nunez, N. P. (2009). Differential susceptibility to obesity between male, female and ovariectomized female mice. Nutr. J. 8:11. doi: 10.1186/1475-2891-8-11
Huang, L. H., Elvington, A., and Randolph, G. J. (2015). The role of the lymphatic system in cholesterol transport. Front. Pharmacol. 6:182. doi: 10.3389/fphys.2017.000182
Iantorno, M., Campia, U., Di Daniele, N., Nistico, S., Forleo, G. B., Cardillo, C., et al. (2014). Obesity, inflammation and endothelial dysfunction. J. Biol. Regul. Homeost. Agents 28, 169–176.
Ingvorsen, C., Karp, N. A., and Lelliott, C. J. (2017). The role of sex and body weight on the metabolic effects of high-fat diet in C57BL/6N mice. Nutr. Diabetes 7:e261. doi: 10.1038/nutd.2017.6
Jeon, B. H., Jang, C., Han, J., Kataru, R. P., Piao, L., Jung, K., et al. (2008). Profound but dysfunctional lymphangiogenesis via vascular endothelial growth factor ligands from CD11b+ macrophages in advanced ovarian cancer. Cancer Res. 68, 1100–1109. doi: 10.1158/0008-5472.CAN-07-2572
Jiang, X., Tian, W., Nicolls, M. R., and Rockson, S. G. (2019). The lymphatic system in obesity, insulin resistance, and cardiovascular diseases. Front. Physiol. 10:1402. doi: 10.3389/fphys.2019.01402
Johnson, L. A., and Jackson, D. G. (2010). Inflammation-induced secretion of CCL21 in lymphatic endothelium is a key regulator of integrin-mediated dendritic cell transmigration. Int. Immunol. 22, 839–849. doi: 10.1093/intimm/dxq435
Karaman, S., Hollmen, M., Robciuc, M. R., Alitalo, A., Nurmi, H., Morf, B., et al. (2015). Blockade of VEGF-C and VEGF-D modulates adipose tissue inflammation and improves metabolic parameters under high-fat diet. Mol. Metab. 4, 93–105. doi: 10.1016/j.molmet.2014.11.006
Karkkainen, M. J., Saaristo, A., Jussila, L., Karila, K. A., Lawrence, E. C., Pajusola, K., et al. (2001). A model for gene therapy of human hereditary lymphedema. Proc. Natl. Acad. Sci. U.S.A. 98, 12677–12682. doi: 10.1073/pnas.221449198
Kasuya, A., Sakabe, J., and Tokura, Y. (2014). Potential application of in vivo imaging of impaired lymphatic duct to evaluate the severity of pressure ulcer in mouse model. Sci. Rep. 4:4173. doi: 10.1038/srep04173
Kataru, R. P., Kim, H., Jang, C., Choi, D. K., Koh, B. I., Kim, M., et al. (2011). T lymphocytes negatively regulate lymph node lymphatic vessel formation. Immunity 34, 96–107. doi: 10.1016/j.immuni.2010.12.016
Kawanishi, N., Mizokami, T., Yano, H., and Suzuki, K. (2013). Exercise attenuates M1 macrophages and CD8+ T cells in the adipose tissue of obese mice. Med. Sci. Sports Exerc. 45, 1684–1693. doi: 10.1249/MSS.0b013e31828ff9c6
Kawanishi, N., Yano, H., Yokogawa, Y., and Suzuki, K. (2010). Exercise training inhibits inflammation in adipose tissue via both suppression of macrophage infiltration and acceleration of phenotypic switching from M1 to M2 macrophages in high-fat-diet-induced obese mice. Exerc. Immunol. Rev. 16, 105–118.
Kim, B., Chung, M. J., Park, S. W., Park, J. Y., Bang, S., Park, S. W., et al. (2016). Visceral obesity is associated with poor prognosis in pancreatic adenocarcinoma. Nutr. Cancer 68, 201–207. doi: 10.1080/01635581.2016.1134600
Kim, H., Kataru, R. P., and Koh, G. Y. (2012). Regulation and implications of inflammatory lymphangiogenesis. Trends Immunol. 33, 350–356. doi: 10.1016/j.it.2012.03.006
Kim, H. J., Yoo, Y. J., Ju, Y. S., Lee, S., Cho, S. I., Sung, J., et al. (2013). Combined linkage and association analyses identify a novel locus for obesity near PROX1 in Asians. Obesity 21, 2405–2412. doi: 10.1002/oby.20153
Klotz, L., Norman, S., Vieira, J. M., Masters, M., Rohling, M., Dube, K. N., et al. (2015). Cardiac lymphatics are heterogeneous in origin and respond to injury. Nature 522, 62–67. doi: 10.1038/nature14483
Kuan, E. L., Ivanov, S., Bridenbaugh, E. A., Victora, G., Wang, W., Childs, E. W., et al. (2015). Collecting lymphatic vessel permeability facilitates adipose tissue inflammation and distribution of antigen to lymph node-homing adipose tissue dendritic cells. J. Immunol. 194, 5200–5210. doi: 10.4049/jimmunol.1500221
Lahdenranta, J., Hagendoorn, J., Padera, T. P., Hoshida, T., Nelson, G., Kashiwagi, S., et al. (2009). Endothelial nitric oxide synthase mediates lymphangiogenesis and lymphatic metastasis. Cancer Res. 69, 2801–2808. doi: 10.1158/0008-5472.CAN-08-4051
Leal-Cerro, A., Considine, R. V., Peino, R., Venegas, E., Astorga, R., Casanueva, F. F., et al. (1996). Serum immunoreactive-leptin levels are increased in patients with Cushing’s syndrome. Horm. Metab. Res. 28, 711–713. doi: 10.1055/s-2007-979884
Lecompte, S., Pasquetti, G., Hermant, X., Grenier-Boley, B., Gonzalez-Gross, M., De Henauw, S., et al. (2013). Genetic and molecular insights into the role of PROX1 in glucose metabolism. Diabetes Metab. Res. Rev 62, 1738–1745. doi: 10.2337/db12-0864
Lee, Y., Chakraborty, S., Meininger, C. J., and Muthuchamy, M. (2018). Insulin resistance disrupts cell integrity, mitochondrial function, and inflammatory signaling in lymphatic endothelium. Microcirculation 25:e12492. doi: 10.1111/micc.12492
Liang, Q., Ju, Y., Chen, Y., Wang, W., Li, J., Zhang, L., et al. (2016). Lymphatic endothelial cells efferent to inflamed joints produce iNOS and inhibit lymphatic vessel contraction and drainage in TNF-induced arthritis in mice. Arthritis Res. Ther. 18:62. doi: 10.1186/s13075-016-0963-8
Liao, S., Cheng, G., Conner, D. A., Huang, Y., Kucherlapati, R. S., Munn, L. L., et al. (2011). Impaired lymphatic contraction associated with immunosuppression. Proc. Natl. Acad. Sci. U.S.A. 108, 18784–18789. doi: 10.1073/pnas.1614689113
Lim, H. Y., Rutkowski, J. M., Helft, J., Reddy, S. T., Swartz, M. A., Randolph, G. J., et al. (2009). Hypercholesterolemic mice exhibit lymphatic vessel dysfunction and degeneration. Am. J. Pathol. 175, 1328–1337. doi: 10.2353/ajpath.2009.080963
Lim, H. Y., Thiam, C. H., Yeo, K. P., Bisoendial, R., Hii, C. S., Mcgrath, K. C., et al. (2013). Lymphatic vessels are essential for the removal of cholesterol from peripheral tissues by SR-BI-mediated transport of HDL. Cell Metab. 17, 671–684. doi: 10.1016/j.cmet.2013.04.002
Liu, X., and Oliver, G. (2019). New insights about the lymphatic vasculature in cardiovascular diseases. F1000Research 8:F1000 Faculty Rev-1811. doi: 10.12688/f1000research.20107.1
Lord, R. S. (1968). The white veins: conceptual difficulties in the history of the lymphatics. Med. Hist. 12, 174–184. doi: 10.1017/s0025727300013053
Maclellan, R. A., Zurakowski, D., Grant, F. D., and Greene, A. K. (2017). Massive localized lymphedema: a case-control study. J. Am. Coll. Surg. 224, 212–216. doi: 10.1016/j.jamcollsurg.2016.10.047
Martel, C., Li, W., Fulp, B., Platt, A. M., Gautier, E. L., Westerterp, M., et al. (2013). Lymphatic vasculature mediates macrophage reverse cholesterol transport in mice. J. Clin. Invest. 123, 1571–1579. doi: 10.1172/JCI63685
Maruyama, K., Asai, J., Ii, M., Thorne, T., Losordo, D. W., and D’amore, P. A. (2007). Decreased macrophage number and activation lead to reduced lymphatic vessel formation and contribute to impaired diabetic wound healing. Am. J. Pathol. 170, 1178–1191. doi: 10.2353/ajpath.2007.060018
Mattila, J. T., Ojo, O. O., Kepka-Lenhart, D., Marino, S., Kim, J. H., Eum, S. Y., et al. (2013). Microenvironments in tuberculous granulomas are delineated by distinct populations of macrophage subsets and expression of nitric oxide synthase and arginase isoforms. J. Immunol. 191, 773–784. doi: 10.4049/jimmunol.1300113
Mauricio, M. D., Aldasoro, M., Ortega, J., and Vila, J. M. (2013). Endothelial dysfunction in morbid obesity. Curr. Pharm. Des. 19, 5718–5729.
McLaughlin, S. A., Wright, M. J., Morris, K. T., Giron, G. L., Sampson, M. R., Brockway, J. P., et al. (2008). Prevalence of lymphedema in women with breast cancer 5 years after sentinel lymph node biopsy or axillary dissection: objective measurements. J. Clin. Oncol. 26, 5213–5219. doi: 10.1200/JCO.2008.16.3725
Mehrara, B. J., and Greene, A. K. (2014). Lymphedema and obesity: is there a link? Plast. Reconstr. Surg. 134, 154–160. doi: 10.1097/PRS.0000000000000268
Milasan, A., Ledoux, J., and Martel, C. (2015). Lymphatic network in atherosclerosis: the underestimated path. Future Sci. OA 1:Fso61. doi: 10.4155/fso.15.61
Milasan, A., Smaani, A., and Martel, C. (2019). Early rescue of lymphatic function limits atherosclerosis progression in Ldlr(-/-) mice. Atherosclerosis 283, 106–119. doi: 10.1016/j.atherosclerosis.2019.01.031
Miyazawa-Hoshimoto, S., Takahashi, K., Bujo, H., Hashimoto, N., and Saito, Y. (2003). Elevated serum vascular endothelial growth factor is associated with visceral fat accumulation in human obese subjects. Diabetologia 46, 1483–1488. doi: 10.1007/s00125-003-1221-6
Montgomery, M. K., Hallahan, N. L., Brown, S. H., Liu, M., Mitchell, T. W., Cooney, G. J., et al. (2013). Mouse strain-dependent variation in obesity and glucose homeostasis in response to high-fat feeding. Diabetologia 56, 1129–1139. doi: 10.1007/s00125-013-2846-8
Moriguchi, P., Sannomiya, P., Lara, P. F., Oliveira-Filho, R. M., Greco, K. V., and Sudo-Hayashi, L. S. (2005). Lymphatic system changes in diabetes mellitus: role of insulin and hyperglycemia. Diabetes Metab. Res. Rev. 21, 150–157. doi: 10.1002/dmrr.500
Moschen, A. R., Molnar, C., Geiger, S., Graziadei, I., Ebenbichler, C. F., Weiss, H., et al. (2010). Anti-inflammatory effects of excessive weight loss: potent suppression of adipose interleukin 6 and tumour necrosis factor alpha expression. Gut 59, 1259–1264. doi: 10.1136/gut.2010.214577
Nigro, E., Scudiero, O., Monaco, M. L., Palmieri, A., Mazzarella, G., Costagliola, C., et al. (2014). New insight into adiponectin role in obesity and obesity-related diseases. Biomed. Res. Int. 2014:658913. doi: 10.1155/2014/658913
Nitti, M. D., Hespe, G. E., Kataru, R. P., Garcia Nores, G. D., Savetsky, I. L., Torrisi, J. S., et al. (2016). Obesity-induced lymphatic dysfunction is reversible with weight loss. J. Physiol. 594, 7073–7087. doi: 10.1113/JP273061
Nurmi, H., Saharinen, P., Zarkada, G., Zheng, W., Robciuc, M. R., and Alitalo, K. (2015). VEGF-C is required for intestinal lymphatic vessel maintenance and lipid absorption. EMBO Mol. Med. 7, 1418–1425. doi: 10.15252/emmm.201505731
Perreault, M., and Marette, A. (2001). Targeted disruption of inducible nitric oxide synthase protects against obesity-linked insulin resistance in muscle. Nat. Med. 7, 1138–1143. doi: 10.1038/nm1001-1138
Petrova, T. V., and Koh, G. Y. (2018). Organ-specific lymphatic vasculature: From development to pathophysiology. J. Exp. Med. 215, 35–49. doi: 10.1084/jem.20171868
Poirier, P., Hernandez, T. L., Weil, K. M., Shepard, T. J., and Eckel, R. H. (2003). Impact of diet-induced weight loss on the cardiac autonomic nervous system in severe obesity. Obes. Res. 11, 1040–1047. doi: 10.1038/oby.2003.143
Pond, C. M., and Mattacks, C. A. (1995). Interactions between adipose tissue around lymph nodes and lymphoid cells in vitro. J. Lipid Res. 36, 2219–2231.
Pond, C. M., and Mattacks, C. A. (1998). In vivo evidence for the involvement of the adipose tissue surrounding lymph nodes in immune responses. Immunol. Lett. 63, 159–167. doi: 10.1016/s0165-2478(98)00074-1
Pond, C. M., and Mattacks, C. A. (2002). The activation of the adipose tissue associated with lymph nodes during the early stages of an immune response. Cytokine 17, 131–139. doi: 10.1006/cyto.2001.0999
Randolph, G. J., Bala, S., Rahier, J. F., Johnson, M. W., Wang, P. L., Nalbantoglu, I., et al. (2016). Lymphoid aggregates remodel lymphatic collecting vessels that serve mesenteric lymph nodes in crohn disease. Am. J. Pathol. 186, 3066–3073. doi: 10.1016/j.ajpath.2016.07.026
Randolph, G. J., Ivanov, S., Zinselmeyer, B. H., and Scallan, J. P. (2017). The Lymphatic System: Integral Roles in Immunity. Annu. Rev. Immunol. 35, 31–52. doi: 10.1146/annurev-immunol-041015-055354
Rasmussen, J. C., Herbst, K. L., Aldrich, M. B., Darne, C. D., Tan, I. C., Zhu, B., et al. (2014). An abnormal lymphatic phenotype is associated with subcutaneous adipose tissue deposits in Dercum’s disease. Obesity 22, 2186–2192. doi: 10.1002/oby.20836
Rhodes, C. J., White, M. F., Leahy, J. L., and Kahn, S. E. (2013). Direct autocrine action of insulin on beta-cells: does it make physiological sense? Diabetes 62, 2157–2163. doi: 10.2337/db13-0246
Ridner, S. H., Dietrich, M. S., Stewart, B. R., and Armer, J. M. (2011). Body mass index and breast cancer treatment-related lymphedema. Support Care Cancer 19, 853–857. doi: 10.1007/s00520-011-1089-9
Romani, J., Luelmo, J., Saez, A., Yebenes, M., Sabat, M., Fernandez-Chico, N., et al. (2012). Localized xanthomas associated with primary lymphedema. Pediatr. Dermatol. 29, 113–114. doi: 10.1111/j.1525-1470.2011.01686.x
Rose, D. P., Gilhooly, E. M., and Nixon, D. W. (2002). Adverse effects of obesity on breast cancer prognosis, and the biological actions of leptin (review). Int. J. Oncol. 21, 1285–1292.
Russo, E., Teijeira, A., Vaahtomeri, K., Willrodt, A. H., Bloch, J. S., Nitschke, M., et al. (2016). Intralymphatic CCL21 promotes tissue egress of dendritic cells through afferent lymphatic vessels. Cell Rep. 14, 1723–1734. doi: 10.1016/j.celrep.2016.01.048
Rutkowski, J. M., Markhus, C. E., Gyenge, C. C., Alitalo, K., Wiig, H., and Swartz, M. A. (2010). Dermal collagen and lipid deposition correlate with tissue swelling and hydraulic conductivity in murine primary lymphedema. Am. J. Pathol. 176, 1122–1129. doi: 10.2353/ajpath.2010.090733
Saaristo, A., Tammela, T., Farkkila, A., Karkkainen, M., Suominen, E., Yla-Herttuala, S., et al. (2006). Vascular endothelial growth factor-C accelerates diabetic wound healing. Am. J. Pathol. 169, 1080–1087. doi: 10.2353/ajpath.2006.051251
Sato, A., Kamekura, R., Kawata, K., Kawada, M., Jitsukawa, S., Yamashita, K., et al. (2016). Novel mechanisms of compromised lymphatic endothelial cell homeostasis in obesity: the role of leptin in lymphatic endothelial cell tube formation and proliferation. PLoS One 11:e0158408. doi: 10.1371/journal.pone.00158408
Savetsky, I. L., Albano, N. J., Cuzzone, D. A., Gardenier, J. C., Torrisi, J. S., Garcia Nores, G. D., et al. (2015a). Lymphatic function regulates contact hypersensitivity dermatitis in obesity. J. Invest. Dermatol. 135, 2742–2752. doi: 10.1038/jid.2015.283
Savetsky, I. L., Ghanta, S., Gardenier, J. C., Torrisi, J. S., Garcia Nores, G. D., Hespe, G. E., et al. (2015b). Th2 cytokines inhibit lymphangiogenesis. PLoS One 10:e0126908. doi: 10.1371/journal.pone.00126908
Savetsky, I. L., Torrisi, J. S., Cuzzone, D. A., Ghanta, S., Albano, N. J., Gardenier, J. C., et al. (2014). Obesity increases inflammation and impairs lymphatic function in a mouse model of lymphedema. Am. J. Physiol. Heart Circ. Physiol. 307, H165–H172. doi: 10.1152/ajpheart.00244.2014
Sawane, M., Kajiya, K., Kidoya, H., Takagi, M., Muramatsu, F., and Takakura, N. (2013). Apelin inhibits diet-induced obesity by enhancing lymphatic and blood vessel integrity. Diabetes Metab. Res. Rev 62, 1970–1980. doi: 10.2337/db12-0604
Sawane, M., Kidoya, H., Muramatsu, F., Takakura, N., and Kajiya, K. (2011). Apelin attenuates UVB-induced edema and inflammation by promoting vessel function. Am. J. Pathol. 179, 2691–2697. doi: 10.1016/j.ajpath.2011.08.024
Scallan, J. P., and Davis, M. J. (2013). Genetic removal of basal nitric oxide enhances contractile activity in isolated murine collecting lymphatic vessels. J. Physiol. 591, 2139–2156. doi: 10.1113/jphysiol.2012.250662
Scallan, J. P., Hill, M. A., and Davis, M. J. (2015). Lymphatic vascular integrity is disrupted in type 2 diabetes due to impaired nitric oxide signalling. Cardiovasc. Res. 107, 89–97. doi: 10.1093/cvr/cvv117
Scallan, J. P., Zawieja, S. D., Castorena-Gonzalez, J. A., and Davis, M. J. (2016). Lymphatic pumping: mechanics, mechanisms and malfunction. J. Physiol. 594, 5749–5768. doi: 10.1113/JP272088
Segula, D. (2014). Complications of obesity in adults: a short review of the literature. Malawi Med. J. 26, 20–24.
Shaw, C., Mortimer, P., and Judd, P. A. (2007). A randomized controlled trial of weight reduction as a treatment for breast cancer-related lymphedema. Cancer 110, 1868–1874. doi: 10.1002/cncr.22994
Shew, T., Wolins, N. E., and Cifarelli, V. (2018). VEGFR-3 signaling regulates triglyceride retention and absorption in the intestine. Front. Physiol. 9:1783. doi: 10.3389/fphys.2017.001783
Shibata, R., Ouchi, N., and Murohara, T. (2009). Adiponectin and cardiovascular disease. Circ. J. 73, 608–614.
Shimizu, Y., Shibata, R., Ishii, M., Ohashi, K., Kambara, T., Uemura, Y., et al. (2013). Adiponectin-mediated modulation of lymphatic vessel formation and lymphedema. J. Am Heart Assoc. 2:e0004938. doi: 10.1161/JAHA.113.000438
Shin, K., Kataru, R. P., Park, H. J., Kwon, B. I., Kim, T. W., Hong, Y. K., et al. (2015). TH2 cells and their cytokines regulate formation and function of lymphatic vessels. Nat. Commun. 6:6196. doi: 10.1038/ncomms7196
Silha, J. V., Krsek, M., Sucharda, P., and Murphy, L. J. (2005). Angiogenic factors are elevated in overweight and obese individuals. Int. J. Obes. 29, 1308–1314. doi: 10.1038/sj.ijo.0802987
Singer, K., Maley, N., Mergian, T., Delproposto, J., Cho, K. W., Zamarron, B. F., et al. (2015). Differences in hematopoietic stem cells contribute to sexually dimorphic inflammatory responses to high fat diet-induced obesity. J. Biol. Chem. 290, 13250–13262. doi: 10.1074/jbc.M114.634568
Sjostrom, C. D., Peltonen, M., Wedel, H., and Sjostrom, L. (2000). Differentiated long-term effects of intentional weight loss on diabetes and hypertension. Hypertension 36, 20–25. doi: 10.1161/01.hyp.36.1.20
Sjostrom, L., Lindroos, A. K., Peltonen, M., Torgerson, J., Bouchard, C., Carlsson, B., et al. (2004). Lifestyle, diabetes, and cardiovascular risk factors 10 years after bariatric surgery. N. Engl. J. Med. 351, 2683–2693. doi: 10.1056/NEJMoa035622
Skobe, M., Hamberg, L. M., Hawighorst, T., Schirner, M., Wolf, G. L., Alitalo, K., et al. (2001). Concurrent induction of lymphangiogenesis, angiogenesis, and macrophage recruitment by vascular endothelial growth factor-C in melanoma. Am. J. Pathol. 159, 893–903. doi: 10.1016/S0002-9440(10)61765-8
Stallworth, J. M., Hennigar, G. R., Jonsson, H. T. Jr., and Rodriguez, O. (1974). The chronically swollen painful extremity. A detailed study for possible etiological factors. JAMA 228, 1656–1659.
Suh, S. H., Choe, K., Hong, S. P., Jeong, S. H., Makinen, T., Kim, K. S., et al. (2019). Gut microbiota regulates lacteal integrity by inducing VEGF-C in intestinal villus macrophages. EMBO Rep. 20:e46927. doi: 10.15252/embr.201846927
Suh, S. H., Hong, S. P., Park, I., Song, J. H., and Koh, G. Y. (2018). Morphological Analysis of Lacteal Structure in the Small Intestine of Adult Mice. Methods Mol. Biol. 1846, 131–139. doi: 10.1007/978-1-4939-8712-2_8
Swenson, K. K., Nissen, M. J., Leach, J. W., and Post-White, J. (2009). Case-control study to evaluate predictors of lymphedema after breast cancer surgery. Oncol. Nurs. Forum 36, 185–193. doi: 10.1188/09.ONF.185-193
Tal, O., Lim, H. Y., Gurevich, I., Milo, I., Shipony, Z., Ng, L. G., et al. (2011). DC mobilization from the skin requires docking to immobilized CCL21 on lymphatic endothelium and intralymphatic crawling. J. Exp. Med. 208, 2141–2153. doi: 10.1084/jem.20102392
Tammela, T., and Alitalo, K. (2010). Lymphangiogenesis: Molecular mechanisms and future promise. Cell 140, 460–476. doi: 10.1016/j.cell.2010.01.045
Tanaka, H., Yamamoto, N., Suzuki, M., Mano, Y., Sano, M., Zaima, N., et al. (2016). Insufficient lymph drainage causes abnormal lipid accumulation and vein wall degeneration. Ann. Vasc. Dis. 9, 277–284. doi: 10.3400/avd.oa.16-00122
Tashiro, K., Feng, J., Wu, S. H., Mashiko, T., Kanayama, K., Narushima, M., et al. (2017). Pathological changes of adipose tissue in secondary lymphoedema. Br. J. Dermatol. 177, 158–167. doi: 10.1111/bjd.15238
Tinahones, F. J., Coin-Araguez, L., Mayas, M. D., Garcia-Fuentes, E., Hurtado-Del-Pozo, C., Vendrell, J., et al. (2012). Obesity-associated insulin resistance is correlated to adipose tissue vascular endothelial growth factors and metalloproteinase levels. BMC Physiol. 12:4. doi: 10.1186/1472-6793-12-4
Torrisi, J. S., Hespe, G. E., Cuzzone, D. A., Savetsky, I. L., Nitti, M. D., Gardenier, J. C., et al. (2016). Inhibition of inflammation and iNOS improves lymphatic function in obesity. Sci. Rep. 6:19817. doi: 10.1038/srep19817
Trincot, C. E., Xu, W., Zhang, H., Kulikauskas, M. R., Caranasos, T. G., Jensen, B. C., et al. (2019). Adrenomedullin induces cardiac lymphangiogenesis after myocardial infarction and regulates cardiac edema via connexin 43. Circ. Res. 124, 101–113. doi: 10.1161/CIRCRESAHA.118.313835
Veronique, A., and Ying, L. H. (2013). Emerging role of lymphatic vessels in reverse cholesterol transport. Aging 5, 390–391. doi: 10.18632/aging.100570
Vieira, J. M., Norman, S., Villa Del, Campo, C., Cahill, T. J., Barnette, D. N., et al. (2018). The cardiac lymphatic system stimulates resolution of inflammation following myocardial infarction. J. Clin. Invest. 128, 3402–3412. doi: 10.1172/JCI97192
Vuorio, T., Nurmi, H., Moulton, K., Kurkipuro, J., Robciuc, M. R., Ohman, M., et al. (2014). Lymphatic vessel insufficiency in hypercholesterolemic mice alters lipoprotein levels and promotes atherogenesis. Arterioscler. Thromb. Vasc. Biol. 34, 1162–1170. doi: 10.1161/ATVBAHA.114.302528
Vuorio, T., Yla-Herttuala, E., Laakkonen, J. P., Laidinen, S., Liimatainen, T., and Yla-Herttuala, S. (2018). Downregulation of VEGFR3 signaling alters cardiac lymphatic vessel organization and leads to a higher mortality after acute myocardial infarction. Sci. Rep. 8:16709. doi: 10.1038/s41598-018-34770-4
Wang, Y., Jin, Y., Mae, M. A., Zhang, Y., Ortsater, H., Betsholtz, C., et al. (2017). Smooth muscle cell recruitment to lymphatic vessels requires PDGFB and impacts vessel size but not identity. Development 144, 3590–3601. doi: 10.1242/dev.147967
Warren, A. G., Brorson, H., Borud, L. J., and Slavin, S. A. (2007). Lymphedema: a comprehensive review. Ann. Plast. Surg. 59, 464–472.
Weitman, E. S., Aschen, S. Z., Farias-Eisner, G., Albano, N., Cuzzone, D. A., Ghanta, S., et al. (2013). Obesity impairs lymphatic fluid transport and dendritic cell migration to lymph nodes. PLoS One 8:e70703. doi: 10.1371/journal.pone.0070703
Wigle, J. T., Harvey, N., Detmar, M., Lagutina, I., Grosveld, G., Gunn, M. D., et al. (2002). An essential role for Prox1 in the induction of the lymphatic endothelial cell phenotype. EMBO J. 21, 1505–1513. doi: 10.1093/emboj/21.7.1505
Winer, S., Chan, Y., Paltser, G., Truong, D., Tsui, H., Bahrami, J., et al. (2009). Normalization of obesity-associated insulin resistance through immunotherapy. Nat. Med. 15, 921–929. doi: 10.1038/nm.2001
Wu, H., Rahman, H. N. A., Dong, Y., Liu, X., Lee, Y., Wen, A., et al. (2018). Epsin deficiency promotes lymphangiogenesis through regulation of VEGFR3 degradation in diabetes. J. Clin. Invest. 128, 4025–4043. doi: 10.1172/JCI96063
Xue, Q., Yan, Y., Zhang, R., and Xiong, H. (2018). Regulation of iNOS on immune cells and its role in diseases. Int. J. Mol. Sci. 19:E3805. doi: 10.3390/ijms19123805
Zafar, M. I., Mills, K., Ye, X., Blakely, B., Min, J., Kong, W., et al. (2018). Association between the expression of vascular endothelial growth factors and metabolic syndrome or its components: a systematic review and meta-analysis. Diabetol. Metab. Syndr. 10:62. doi: 10.1186/s13098-018-0363-0
Zampell, J. C., Aschen, S., Weitman, E. S., Yan, A., Elhadad, S., De Brot, M., et al. (2012). Regulation of adipogenesis by lymphatic fluid stasis: part I. Adipogenesis, fibrosis, and inflammation. Plast. Reconstr. Surg. 129, 825–834. doi: 10.1097/PRS.0b013e3182450b7a
Zhang, F., Zarkada, G., Han, J., Li, J., Dubrac, A., Ola, R., et al. (2018). Lacteal junction zippering protects against diet-induced obesity. Science 361, 599–603. doi: 10.1126/science.aap9331
Zhang, Y., Proenca, R., Maffei, M., Barone, M., Leopold, L., and Friedman, J. M. (1994). Positional cloning of the mouse obese gene and its human homologue. Nature 372, 425–432. doi: 10.1038/372425a0
Zheng, W., Nurmi, H., Appak, S., Sabine, A., Bovay, E., Korhonen, E. A., et al. (2014). Angiopoietin 2 regulates the transformation and integrity of lymphatic endothelial cell junctions. Genes Dev. 28, 1592–1603. doi: 10.1101/gad.237677.114
Zheng, Z., Ren, K., Peng, X., Zhu, X., and Yi, G. (2018). Lymphatic vessels: a potential approach to the treatment of atherosclerosis?. Lymphat. Res. Biol. 16, 498–506. doi: 10.1089/lrb.2018.0015
Zhu, J., Su, X., Li, G., Chen, J., Tang, B., and Yang, Y. (2014). The incidence of acute myocardial infarction in relation to overweight and obesity: a meta-analysis. Arch. Med. Sci. 10, 855–862. doi: 10.5114/aoms.2014.46206
Keywords: lymphatic vessels, lymphatic function, obesity, inflammation, metabolic syndrome
Citation: Kataru RP, Park HJ, Baik JE, Li C, Shin J and Mehrara BJ (2020) Regulation of Lymphatic Function in Obesity. Front. Physiol. 11:459. doi: 10.3389/fphys.2020.00459
Received: 30 January 2020; Accepted: 16 April 2020;
Published: 15 May 2020.
Edited by:
Joshua Scallan, University of South Florida, United StatesReviewed by:
Catherine Martel, Université de Montréal, CanadaScott D. Zawieja, University of Missouri, United States
Jorge A. Castorena-Gonzalez, University of Missouri, United States
Copyright © 2020 Kataru, Park, Baik, Li, Shin and Mehrara. This is an open-access article distributed under the terms of the Creative Commons Attribution License (CC BY). The use, distribution or reproduction in other forums is permitted, provided the original author(s) and the copyright owner(s) are credited and that the original publication in this journal is cited, in accordance with accepted academic practice. No use, distribution or reproduction is permitted which does not comply with these terms.
*Correspondence: Babak J. Mehrara, mehrarab@mskcc.org