- 1Department of Diving and Hyperbaric Medicine, Naval Special Medicine Center, Naval Medical University, Shanghai, China
- 2Naval Diving Medical Discipline, Naval Special Medicine Center, Naval Medical University, Shanghai, China
- 3School of Basic Medicines, Naval Medical University, Shanghai, China
Inflammatory reaction is the crux in various clinical critical diseases including decompression sickness (DCS). Ulinastatin (UTI), a potent anti-inflammatory agent, has been used clinically, including as a substitution for steroids. This study aimed to explore the potential effects of UTI upon DCS in a rabbit model. Eighty-eight rabbits were subjected to simulated diving to 6 atmospheres absolute (ATA) for 60 min with 2.5-minute decompression. Three doses of UTI (15/7.5/3.75 × 105 U/kg) or saline were intravenously administered immediately following decompression. Circulating bubbles were monitored for 3 h following decompression and DCS signs were evaluated for 24 h. Blood was sampled 8 times during 72 h after decompression for inflammatory, endothelial, oxidative and routine blood indices. Lung tissues were also sampled for evaluating endothelial function. Another six rabbits were used as Normal controls. In the high dose UTI group the mortality, general morbidity and incidence of severe DCS was decreased from 31.25 to 9.38% (P = 0.030), 84.38 to 62.50% (P = 0.048) and 46.88 to 21.88% (P = 0.035), respectively. The high dose of UTI significantly postponed the occurrence of DCS (P = 0.030) and prolonged survival time (P = 0.009) compared with the Saline group, and significantly ameliorated inflammation responses, endothelial injuries and oxidative damage. The results strongly suggest the benefit of UTI on DCS, especially for severe cases. Large doses are needed to achieve significant effects. UTI may be a potential ideal pharmacological candidate for the treatment of severe DCS.
Introduction
Urinary trypsin inhibitor (Ulinastatin, UTI) is a nonspecific and multivalent Kunitz-type serine protease inhibitor purified from human urine (Yano et al., 2003). It is capable of suppressing various serine proteases such as trypsin, plasmin, neutrophil elastase and chymotrypsin (Nishiyama et al., 1996; Nakatani et al., 2001), and can also effectively stabilize lysosomal and cellular membranes (Nakahama et al., 1999). UTI has confirmed powerful efficacy in inhibiting the release of inflammatory factors, removing oxygen free radicals, improving microcirculation and tissue perfusion, and alleviating endothelial injuries (Takada et al., 2003; Tanaka et al., 2010; We et al., 2017; Fang et al., 2018). Clinically, UTI has been applied effectively in treating acute pancreatitis, acute circulatory failure, and ischemia-reperfusion injury with few adverse events (Yoo et al., 2008; Li et al., 2017). Recently, UTI was demonstrated to exert protective effects in shock (Li et al., 2019). These clinical indications are similar to those of many steroids.
Decompression sickness (DCS) is one major concern for divers, astronauts and other personnel engaging in barometric operations, and bubble formation in tissue and circulation is the underlying mechanism (Arieli, 2018). As foreign bodies, bubbles can activate inflammatory cascade reactions, and induce cell irritation and tissue injuries (Papadopoulo et al., 2014). In the severe forms of DCS, significant neurological, respiratory and circulatory deficits or failure are frequently involved, and steroids are routinely used due to their potency on inhibiting systemic inflammation, reducing capillary permeability, maintaining cell membrane and reducing tissue edema (Kizer, 1981). However, many studies have shown no significant improvement of DCS when treated with steroids (Dromsk et al., 2003; Montcalm-Smith et al., 2008), and may even worsen the outcome of central nervous system injury due to their adverse effect of elevating blood glucose (Chikani et al., 2017). Hence, there has been a decline in the use of steroids for the treatment of severe DCS as well as other critical diseases due to their adverse effects and uncertainty of outcomes (Dromsk et al., 2003; Felleiter et al., 2012; Gibbison et al., 2017; Walje et al., 2017; Oh et al., 2019). Since 2008, the United States Navy no longer recommends steroids for DCS treatment. In consideration of UTI’s unique pharmacological properties, we speculated that UTI would have therapeutic effects on DCS, and the underlying mechanisms are also explored in the present study.
Materials and Methods
Animals
Ninety-four male New Zealand White rabbits weighing 2.0∼2.3 kg were obtained from Shanghai Shengwang Laboratory Animal Co., Ltd. The current study was performed at the laboratory of Diving and Hyperbaric Medicine at the Naval Medical University. All experimental procedures in this study were reviewed and approved by the Ethics Committee for Animal Experiment of the university. The rabbits were housed, and standard chow and water were given ad libitum in a room with controlled humidity (50∼60%), temperature (24∼26°C) and a 12-hour light-dark cycle.
Experimental Procedure and Design
After acclimation to the laboratory environment for one week, 88 rabbits were randomly divided into 4 groups and subjected to simulated diving and rapid decompression. After surfacing, either a single intravenous injection of UTI (Techpool Biopharma Co., Ltd., Guangdong, China) at 15 × 105 U/kg (High dose, UTI-H, n = 32), 7.5 × 105 U/kg (Median dose, UTI-M, n = 12) and 3.75 × 105 U/kg (Low dose, UTI-L, n = 12) or a same volume of saline (n = 32) were immediately administered. UTI was dissolved in saline (1 ml/kg body weight) and injected via the marginal ear veins. All of the animals were under continuous observation for 24 h after decompression to evaluate DCS signs. Blood was sampled before the simulated diving and at 1, 6, 12, 24, 36, 48, and 72 h following decompression to determine serum levels of biochemical indices. Twelve rabbits in each group underwent bubble detection at 10, 20, 30, 40, 60, 90, 120, and 180 min following decompression, and were then euthanized by intraperitoneal injection of pentobarbital (200 mg/kg) for sampling lung tissues and bronchoalveolar lavage fluid (BALF). Another six rabbits were sham exposed at normobaric air in the chamber as normal controls and were similarly sampled.
Hyperbaric Exposure Protocol
The rabbits were subjected to simulated diving in pairs as designated in Table 1 in an animal hyperbaric chamber (DWC150, Yangyuan, Shanghai, China). The pressure was increased to six atmospheres absolute (ATA) in 5 min, slowly at the beginning to minimize any possible discomfort, and maintained for 60 min before decompressing linearly at two ATA/min to ambient pressure. Our previous research showed this profile could produce an incidence of DCS in rabbits of around 75% (Meng et al., 2018). During exposure, the temperature inside the chamber was maintained between 23 and 25°C, and the chamber was ventilated continuously for timely removal of metabolic generated carbon dioxide (CO2).
DCS Symptoms Observation and Functional Evaluation
Following decompression, DCS was evaluated by two observers who were blinded to the treatments. The appearance and onset time of DCS signs were recorded. Severe DCS was defined as individuals with at least one of the signs including paralysis, dyspnea, seizure and death. Respiratory function was monitored and scored at 10, 20, 30, 40, 60, 90, and 120 min post decompression using a 0–4 grading system as following (Atkins et al., 1988): 0, normal breathing; 1, mild labored breathing; 2, restlessness and labored breathing; 3, severely labored breathing, recumbent posture; 4, collapse, stupor and death. Motor function was evaluated before simulated diving and at 1, 6, 12, and 24 h after surfacing using Tarlov score (Kertmen et al., 2013), and each rabbit was scored 0 to 5 as following: 0, normal hind-limb function; 1, able to hop but uncoordinated; 2, able to sit but unable to hop; 3, active movement but unable to sit without assistance; 4, movement of joints perceptible; and 5, no voluntary hind-limb movement.
Bubble Detection
After surfacing, rabbits were placed in a supine position for bubble detection using a 10 MHz transducer connected to an ultrasound scanner (Mylab 30CV, Esaote, Italy). Bubbles were recognized as bright spots in heart chambers, and were scored by optimized Eftedal-Brubakk (EB) grading scale (Møllerløkken et al., 2016), with the modification shown in Table 2.
Blood Routine Examination
Half milliliter venous blood samples were taken from the marginal ear veins and collected into EDTA-anticoagulated tubes for blood routine examination using an automatic hematology analyzer (BC-2800Vet, Mindray, China).
Determination of Serum Biochemical Indices
Another 2 ml venous blood was obtained and centrifuged at 4°C and 2500 rpm for 10 min. Serum levels of intercellular cell adhesion molecule-1 (ICAM-1), endothelin-1 (ET-1), monocyte chemoattractant protein-1 (MCP-1) and interleukin-1beta (IL-1β) were determined by respective enzyme-linked immunosorbent assay (ELISA) kits (Jiancheng Bioengineering Institute, Nanjing, China). Levels of malondialdehyde (MDA) and myeloperoxidase (MPO) were determined by ELISA using respective assay kits (Melian Biological Technology Co., Shanghai, China). All assays were performed according to the manufacturers’ instructions.
Lung Wet/Dry Weight Ratio Assay and BALF Analysis
After euthanasia at 24 h following decompression, fresh specimens of the right lung lobes were removed and weighed as wet weight and placed in an oven at 60°C for 72 h to measure dry weight. Lung water content was assayed by Wet/Dry (W/D) weight ratio. A plastic tube was tied into the trachea and the left lung lavage was performed by flushing the airways with 3 × 10 ml 0.9% saline, and BALF recovery was approximately 80%. Total BALF protein was measured by bicinchoninic acid (BCA) using enhanced BCA protein assay kit (Beyotime Institute of Biotechology, Nantong, China).
Statistical Analysis
Where applicable, parametric data are expressed as mean ± s.d. Morbidity and mortality from different treatments were compared by Chi-square test. Normal distribution was determined by Shapiro-Wilk test. Comparisons of latency to DCS and survival curves were performed with the log-rank test. Bubble loads were analyzed by the generalized estimation equation. The mean differences between groups were assessed using an LSD test or Dunnett’s test. Respiratory and Tarlov scores were compared between UTI and Saline groups by Mann-Whitney U test. P values less than 0.05 were considered statistically significant.
Results
Morbidity and Mortality of DCS
The rabbits weighted 2.16 ± 0.12 kg before simulated diving, and there was no significant difference between groups. In 95% animals, DCS occurred within 30 min following decompression. The morbidity of DCS and severe DCS in the UTI-H group were significantly lower than in the Saline group (62.50% vs. 84.38%, χ2 = 3.925, P = 0.048 and 21.88% vs. 46.88%, χ2 = 4.433, P = 0.035; Figure 1A), and the occurrence of DCS was significantly postponed (χ2 = 4.724, P = 0.030, Figure 1B). The majority of deaths occurred following ∼1 min severe dyspnea and a transient convulsion. The high dose of UTI significantly decreased mortality from 31.25 to 9.38% (χ2 = 4.730, P = 0.030, Figure 1A), and survival time was also obviously extended (χ2 = 6.838, P = 0.009, Figure 1C). No statistical significance was found in DCS signs between UTI-M, UTI-L and Saline groups.
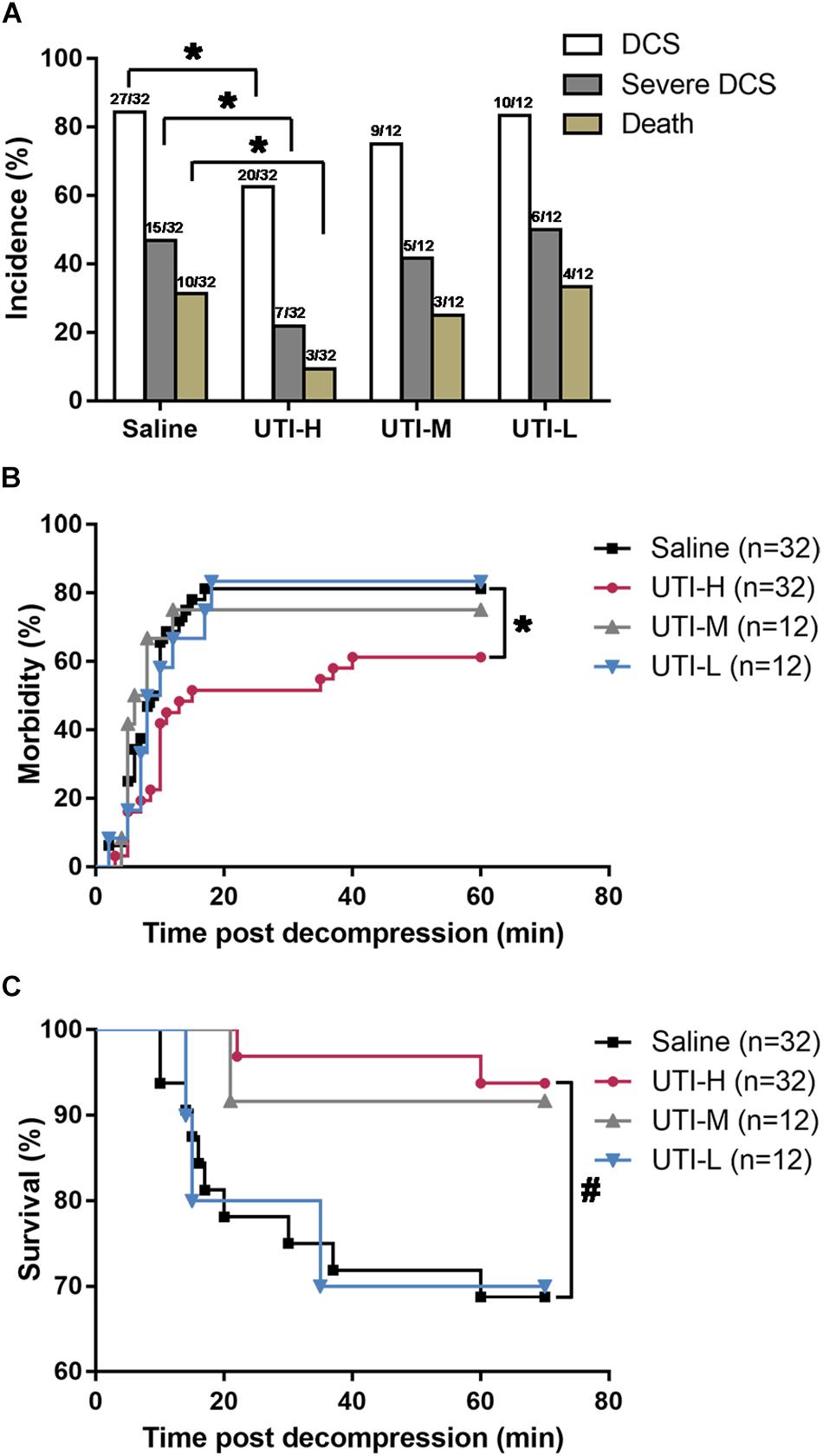
Figure 1. Effects of different doses of UTI on DCS morbidity and mortality in rabbits. Rabbits were subjected to a simulated dive to six atmospheres absolute (ATA) for 60 min with 2.5-minute decompression, and received intravenous injection of different doses of UTI or saline immediately following decompression. (A) The mortality, general morbidity and incidence of severe DCS. (B) The incidence of DCS with time. (C) Survival curve of rapid decompressed rabbits. * P < 0.05, #P < 0.01 vs. Saline group.
Bubble Load Following Decompression
Abundant bubbles were detected as bright spots in the right heart chambers. Bubbles peaked within 30 min following decompression, and diminished gradually thereafter (Figure 2). There was no obvious difference between bubble scores in rabbits treated with UTI and saline (F = 0.201 and P = 0.895).
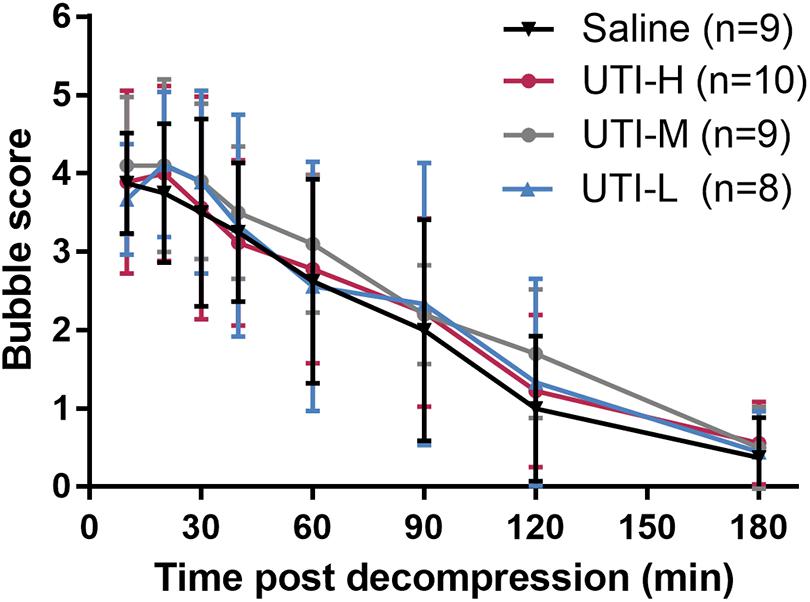
Figure 2. Bubble load in rabbits after simulated diving and UTI treatments. After a simulated dive, rabbits immediately received an intravenous injection of different doses of UTI or saline. Bubbles were detected by ultrasound at multiple time points following decompression and were scored using the modified Eftedal-Brubakk grading scale. No statistical difference existed between groups (P = 0.895).
Protective Effects of UTI Against Lung Injuries and Neurological Disorders
In the Saline group, 37.50% of individuals underwent a short period of fast or difficult breathing following decompression. Though no obvious difference existed in UTI treated animals when compared with those treated with saline, the high dose of UTI showed a tendency to decrease maximum respiratory scores observed during the 2 h following decompression (Z = −1.684, P = 0.092, Figure 3A). Protein content in BALF and lung W/D weight ratio were significantly decreased in the UTI-H group compared with the Saline group (P = 0.025, Figure 3C and P = 0.042, Figure 3D), and detailed statistical results are shown in Table 3. There was no statistical difference in UTI-M and UTI-L with regard to BALF protein (P = 0.592 and P = 0.727, Figure 3C) or lung W/D weight ratio (P = 0.402 and P = 0.781, Figure 3D). 46.88% of individuals in the Saline group developed neurological disorders, which were accompanied with different degrees of paralysis. The high dose of UTI significantly lowered maximal observed Tarlov scores and scores assessed at 24 h following decompression (Z = −2.220, P = 0.026, and Z = −2.592, P = 0.010, Figure 3B). The median or low doses of UTI did not show a statistical difference.
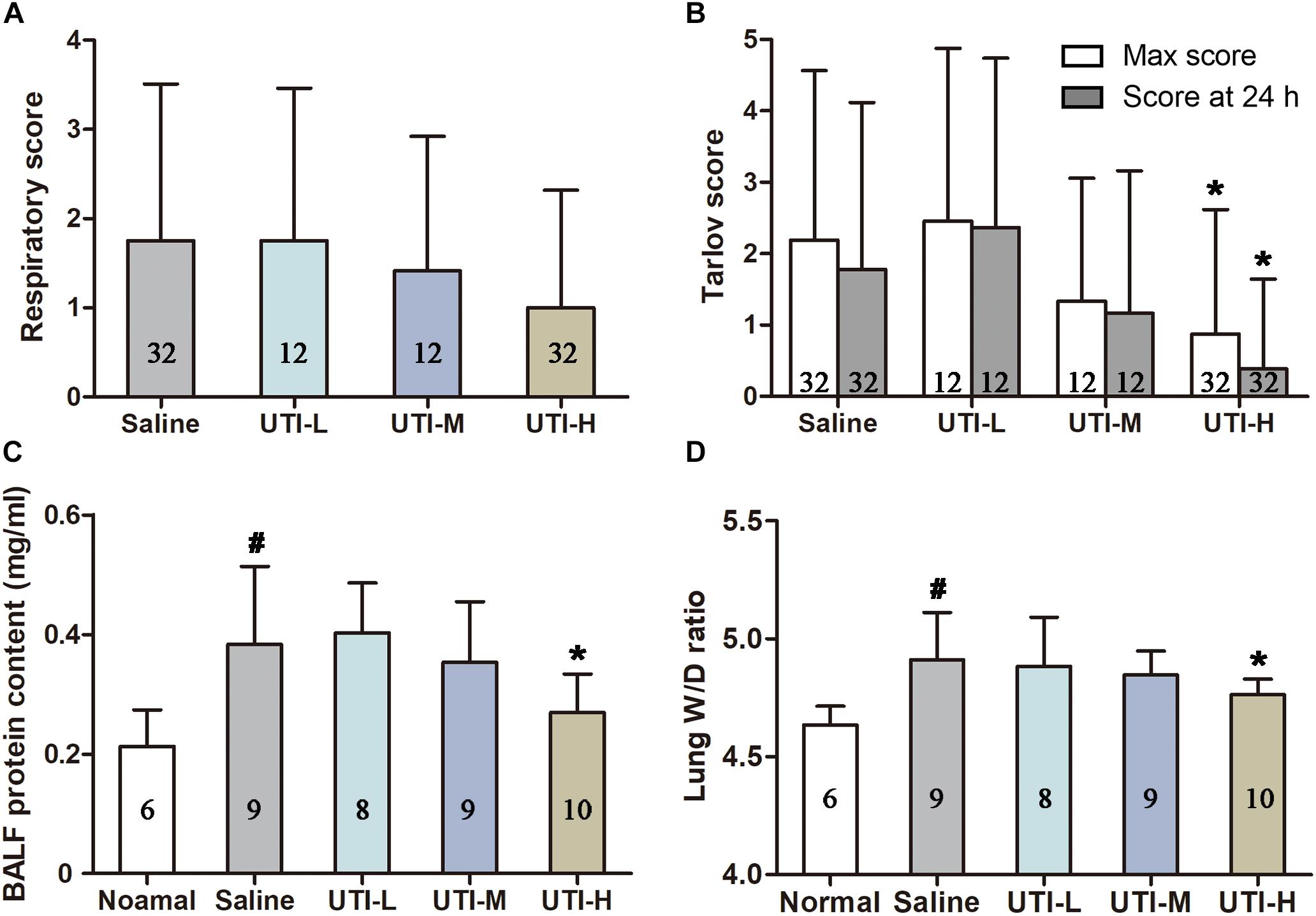
Figure 3. Protective effects of UTI against lung injuries and neurological disorders. Respiratory function was monitored and scored for 2 h following simulated diving. The maximum observed respiratory scores are shown in panel (A). Motor function was evaluated using Tarlov score for 24 h, and UTI-H significantly lowered both the maximal observed scores and scores assessed at 24 h after surfacing (B). The high dose of UTI reduced the elevated BALF protein content and lung W/D ratio (C,D). #P < 0.01 vs. Normal controls, * P < 0.05 vs. Saline group.
Protective Effects of UTI on Blood Routine
As shown in Figure 4, counts of white blood cells (WBC) and platelet (PLT) decreased rapidly 1 h following decompression, and returned to normal gradually around 24 h following decompression. The high dose of UTI significantly inhibited the reduction of WBC and PLT (P = 0.006, Figure 4B and P = 0.005, Figure 4D). No statistical difference existed in UTI-M and UTI-L in terms of WBC (P = 0.066 and P = 0.134, Figure 4B) and PLT (P = 0.065 and P = 0.160, Figure 4D).
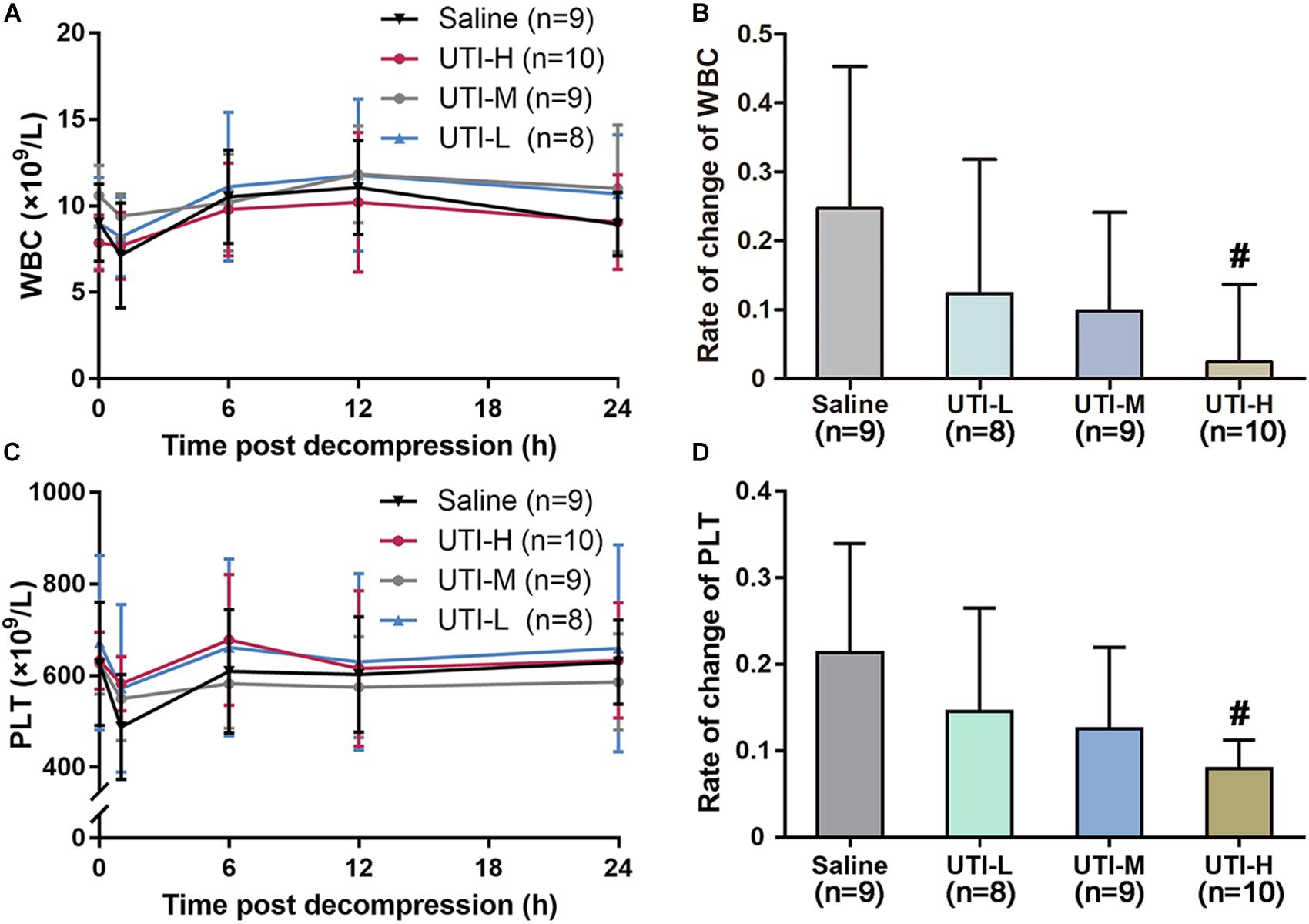
Figure 4. Protective effects of different doses of UTI on blood routine. Blood was sampled from marginal ear veins in rabbits treated with saline or UTI before and after a simulated dive. Changes of WBC and PLT with time are shown (A,C), and respective changes rates of different groups at 1 h following decompression are shown in panels (B,D). The change rates of WBC and PLT were compared between UTI and Saline groups, respectively. #P < 0.01 vs. Saline group.
Anti-inflammatory, Anti-oxidative, Endothelial Protective Effects of UTI Against DCS
Levels of IL-1β, MCP-1, ET-1, ICAM-1, MDA, and MPO increased following decompression, peaked at 6, 12, 12, 12, 6, and 1 h, and gradually decreased to normal around 72, 72, 48, 48, 24, and 24 h, respectively (Figures 5A1–A3). As shown in Figures 5B1–B3, both the high and median doses of UTI reduced the peak levels of all the determined biochemical indices (P < 0.05 or P < 0.01) except median dose of UTI on MCP-1, MDA and MPO, which did not reach statistical significance. The low dose of UTI decreased MCP-1, ET-1, ICAM-1 and MPO levels, but only reached statistical significance on ICAM-1 (P < 0.05). Statistical results in detail are shown in Table 3.
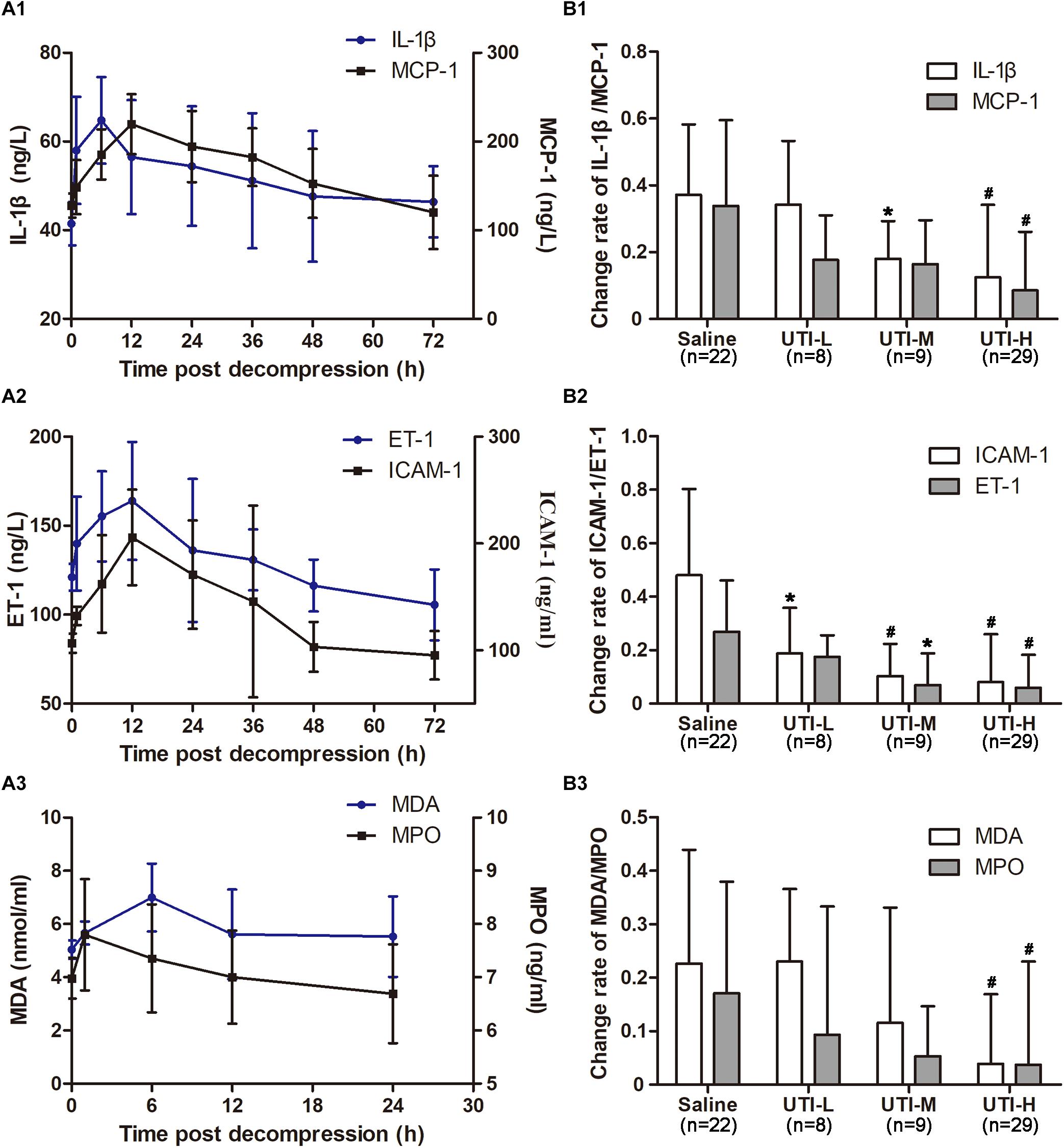
Figure 5. Effects of different doses of UTI on inflammatory, endothelial and oxidative indicators. Before simulated diving and 1, 6, 12, 24, 36, 48, and 72 h following decompression, blood was sampled via marginal ear veins in rabbits treated with different doses of UTI or saline. Six rabbits in the Saline group were used to monitor changes in biochemical indices over time to determine their respective peak time points (A1–A3), and the change rates were compared between UTI and Saline groups at the respective peak time points (B1–B3). ∗P < 0.05 and #P < 0.01 vs. Saline group.
Discussion
Inflammation is an adaptive and evolving process, which can be triggered by pernicious stimuli and conditions, such as infection and tissue injury (Medzhitov, 2008). Generally, it is supposed to be beneficial when it’s under control, such as by exerting protective effects against infection. However, it becomes detrimental when out of control and may progress into a vicious cycle. Decompression bubbles formed in tissues and the circulatory system trigger inflammatory cascades, and play an extremely important role in the pathophysiology of DCS, especially in severe cases, in which systemic inflammatory responses continue despite the disappearance of bubbles, and further worsen the outcomes of DCS (Levin et al., 1981; Dennison et al., 2012). The inhibition of inflammation cascade responses is of great importance to stop the progression of DCS (Papadopoulo et al., 2014).
Ulinastatin is a multivalent serine protease inhibitor extracted from human urine. It exerts protective effects via suppressing various serine proteases (Nishiyama et al., 1996; Park et al., 2010) and stabilizing lysosomes and cellular membranes (Kanayama et al., 1997). Various clinical and animal experiments have demonstrated its anti-inflammatory, anti-oxidative, anticoagulant properties, and it can also improve the microcirculatory environment and reduce tissue damage (Tsujimura et al., 2008; Xu et al., 2012; Li et al., 2017; Liu et al., 2018). Clinically, it has been adopted successfully in the treatment of life-threatening inflammation such as acute pancreatitis and sepsis (Tsujino et al., 2005; Zhang et al., 2008). Previously, steroids were routinely recommended and administered for severe DCS considering their benefits of decreasing tissue edema, reducing inflammation, alleviating leakage of blood vessels, and preventing histamine release (Kizer, 1981; Halpern et al., 1982; Dutka et al., 1992; Montcalm-Smith et al., 2008). However, even a high dose of steroids showed no improvement (Francis and Dutka, 1989; Montcalm-Smith et al., 2008) and even worsened the outcomes of DCS in animals (Dromsk et al., 2003). Side effects including the elevation of blood sugar were observed and steroids are no longer recommended for treating DCS in divers (Moon, 2009). Since UTI is regarded as an exciting alternative for steroids clinically (Linder and Russell, 2014), research into the potential protective effects of UTI on DCS is warranted.
In this study, the median dose of 7.5 × 105 U/kg was derived from the effective dosage commonly used in experimental acute pancreatitis (Ohbishi et al., 1984), and a dose gradient of 3.75/7.5/15 × 105 U/kg was adopted to explore possible dose-effect responses. Based on our preliminary experiments, the morbidity of DCS was around 75% for the saline treated rabbits and 40% for high dose of UTI treated ones, and a sample size of 32 for the two groups would provide 80% power to reach statistical significance concerning DCS morbidity based on a two-tailed significance level of 0.05. To minimize the use of animals, 12 rabbits were chosen for the other two dosage groups, which were designed to compare mainly biochemical indices with a concurrent observation of morbidity and mortality.
Autochthonous or circulating bubbles formed after rapid decompression play an important role in the progress of DCS (Qing et al., 2017; Zhang et al., 2017). The bubbles can not only cause mechanical damages and blockage, but also act as foreign bodies that trigger a cascade of inflammatory responses (Levett and Millar, 2008). As a powerful serine protease inhibitor, UTI is capable of reducing the release of inflammatory factors (Liu et al., 2017). IL-1β is one proximal factor responsible for downstream cytokine production and vascular injuries induced by decompression (Thom et al., 2018). MCP-1 is another proinflammatory cytokine in the process of disease resistance, which is related to decompression stress (Qing et al., 2018). UTI treatment significantly decreased the elevated serum levels of IL-1β and MCP-1 and, hence, in this study relieved inflammation cascade reactions induced by DCS.
In addition to its anti-inflammation property, UTI also effectively relieves endothelial injuries in DCS, probably through protecting endothelial glycocalyx (Wang et al., 2017). Besides, UTI can reduce the permeability of pulmonary capillary endothelial cells through the protection of endothelial junctional proteins (Fang et al., 2018). UTI may also protect endothelial cells from neutrophil-mediated injury not only by inactivation of the extracellular elastase excreted from neutrophils, but also by suppressing the production of activated elastase (Nakatani et al., 2001). These pathways ultimately lowered the degrees of endothelial injuries and high permeability. Since the endothelial system is one of the vulnerable organs of DCS, UTI may hence alleviate DCS injuries. The effectiveness on reducing BALF protein content and lung W/D weight ratio, and serum levels of ET-1 and ICAM-1 provide evidence that endothelial injuries and microvascular permeability were ameliorated by UTI.
Bubbles interact with blood cells and plasma proteins, which may cause platelet activation and deposition, as well as leucocyte-endothelial adhesion, which are further enhanced by the increased expression of ICAM-1 (Aosasa et al., 1998; Levett and Millar, 2008; Vann et al., 2011). UTI has been confirmed to be effective in inhibiting neutrophil accumulation caused by the ischemia/reperfusion injury (Okuhama et al., 1999), and suppressing reduction of PLT induced by lipopolysaccharide (Inoue and Takano, 2010). Similarly, in this study UTI effectively counteracted the reduction of WBC and PLT post decompression. Apart from the properties mentioned above, UTI has also been demonstrated to inhibit oxidative stress and acts as a potent reactive oxygen scavenger (Du and Ko, 2005). Levels of oxidative stress, which were correlated with the severity of decompression (Lambrechts et al., 2015), were significantly lowered in UTI-H as exhibited by the changes of MDA and MPO. As oxidative stress is likely to trigger endothelial responses (Eftedal et al., 2012), endothelial injuries may be relieved in turn due to alleviated oxidative stress.
The inflammatory, endothelial and oxidative indices in this study were mainly selected based on our previous studies in rat and swine DCS models (Zhang et al., 2017; Qing et al., 2018, 2019). In accordance with our swine model, all biochemical indices showed similar changes to those seen in rats except for E-selectin, which showed no change in the rabbit and swine DCS model. Considering the time course following decompression of these indices between different species, there was a general trend showing that the peak time points in rabbits were earlier than that of swine, then that of rats. These time courses supply indispensable information to determine the severity of DCS at different times following decompression. Another trend observed was that the change rates of indices were contrary to animal size.
The present results also confirm the protective effects of UTI against lung injuries and neurological disorders induced by DCS. There is strong evidence indicating protective effects of UTI against sepsis-induced multiorgan injuries, such as acute lung injury (Fang et al., 2018). It has been demonstrated that UTI relieves TNF-α induced hyperpermeability of vascular endothelial cells (We et al., 2017). In addition, UTI can effectively inhibit inflammation and reduce protein expression of AQP-4 (Cui and Zhu, 2015), which helps to attenuate capillary permeability and, as a result, alleviates lung and neural injuries.
The results of the present study show that a high dose of UTI (15 × 105 U/kg) significantly lowered the morbidity and mortality of DCS. Because no obvious difference in circulating bubbles between groups were found, the protective effects of UTI relied mainly on its anti-inflammatory, anti-oxidative, endothelial protective properties, as well as hematology stabilization. These features led to the alleviation of lung injuries and neurologic disorders, and significant improvement of DCS prognosis.
Clinically, UTI has already been adopted in treating diseases such as acute pancreatitis, circulatory shock, and systemic inflammatory response syndrome conditions including burns and acute respiratory distress syndrome (ARDS) in China and Japan (Nakahama et al., 1999; Tsujimura et al., 2008; Wang et al., 2016). The results in the present study not only confirm the protective effects of UTI in treating DCS, especially for severe cases, but also prove UTI is powerful in coping with the inflammation cascade response and endothelium related injuries. These evidences provide additional support for replacing steroids with UTI, at least partially, in the treatment of critical diseases characterized by inflammatory responses. No obvious side effect has been observed in past observations, either clinically or experimentally. All these properties favor applying UTI in treating various critical diseases.
In conclusion, this is the first study to explore the potential effects of UTI on DCS using a rabbit model. A single intravenous injection of UTI immediately following decompression exerted significant protective effects against DCS in a dose-response manner, especially for severe cases. The beneficial effects of UTI on DCS were attributed to its anti-inflammatory, anti-oxidative, endothelial protective properties, as well as inhibition of cell adhesion. Depending on the clinical routine in using UTI, it is thought repeated administrations will likely gain further benefits. Regarding that steroids are no longer recommended for the treatment of DCS, UTI may be an ideal substitute. Further explorations in large animals and divers are encouraged.
Data Availability Statement
The raw data supporting the conclusions of this manuscript will be made available by the authors, without undue reservation, to any qualified researcher.
Ethics Statement
The animal study was reviewed and approved by the Ethics Committee for Animal Experiment of the Naval Medical University.
Author Contributions
WX, WM, and LQ designed the experiments. WM, LQ, CL, and HY conducted the experiments. WM, LQ, and XZ contributed to data analyses and interpretation of the results. WX, WM, LQ, and CL wrote the manuscript and prepared all the figures and tables. KZ revised the manuscript.
Funding
The study was partly supported by the Natural Science Foundation of China (grant number: 81571846).
Conflict of Interest
The authors declare that the research was conducted in the absence of any commercial or financial relationships that could be construed as a potential conflict of interest.
Acknowledgments
The authors thank Dr. Peter Buzzacott for language polishing.
References
Aosasa, S., Ono, S., Seki, S., Takayama, E., Tadakuma, T., Hirdide, H., et al. (1998). Inhibitory effect of protease inhibitor on endothelial cell activation. J. Surg. Res. 80, 182–187. doi: 10.1006/jsre.1998.5474
Arieli, R. (2018). Extravascular hydrophobic surfaces, fat droplets, and the connection with decompression illness spinal, joint pain, and dysbaric osteonecrosis. Front. Physiol. 9:305. doi: 10.3389/fphys.2018.00305
Atkins, C. E., Lehner, C. E., Beck, K. A., Dubielzig, R. R., Nordheim, E. V., and Lanphier, E. H. (1988). Experimental respiratory decompression sickness in sheep. J. Appl. Physiol. 65, 1163–1171. doi: 10.1152/jappl.1988.65.3.1163
Chikani, U. N., Ibekwe, M. U., Oguonu, T., Mungai, L., Bisi-Onyemaechi, A. I., Ugege, O. M., et al. (2017). Steroid-induced impairment of glucose tolerance: prevalence among pediatric patients on long-term steroid use in Nigeria. Pediatr Diabetes. 18, 942–946. doi: 10.1111/pedi.12514
Cui, T., and Zhu, G. (2015). Ulinastatin attenuates brain edema after traumatic brain injury in rats. Cell Biochem. Biophys. 71, 595–600. doi: 10.1007/s12013-014-0239-3
Dennison, S., Moore, M., Fahlman, A., Moore, K., Sharp, S., Harry, C. T., et al. (2012). Bubbles in live-stranded dolphins. Proc. Biol. Sci. 279, 1396–1404. doi: 10.1098/rspb.2011.1754
Dromsk, D. M., Weathersby, P. K., and Fahlman, A. (2003). Prophylactic high dose methylprednisolone fails to treat severe decompression sickness in swine. Aviat Space Environ. Med. 74, 21–28.
Du, Y., and Ko, K. M. (2005). Effects of emodin treatment on mitochondrial ATP generation capacity and antioxidant components as well as susceptibility to ischemia-reperfusion injury in rat hearts: single versus multiple doses and gender difference. Life Sci. 77, 2770–2782. doi: 10.1016/j.lfs.2005.03.027
Dutka, A. J., Mink, R. B., Pearson, R. R., and Hallenbeck, J. M. (1992). Effects of treatment with dexamethasone on recovery from experimental cerebral arterial gas embolism. Undersea Biomed. Res. 19, 131–141.
Eftedal, I., Jørgensen, A., Røsbjørgen, R., Flatberg, A., and Brubakk, A. O. (2012). Early genetic responses in rat vascular tissue after simulated diving. Physiol. Genomics. 44, 1201–1207. doi: 10.1152/physiolgenomics.00073.2012
Fang, M., Zhong, W. H., Song, W. L., Deng, Y. Y., Yang, D. M., Xiong, B., et al. (2018). Ulinastatin ameliorates pulmonary capillary endothelial permeability induced by sepsis through protection of tight junctions via inhibition of TNF-α and related pathways. Front. Pharmacol. 9:823. doi: 10.3389/fphar.2018.00823
Felleiter, P., Müller, N., Schumann, F., Felix, O., and Lierz, P. (2012). Changes in the use of the methylprednisolone protocol for traumatic spinal cord injury in Switzerland. Spine 37, 953–956. doi: 10.1097/BRS.0b013e31823a07a2
Francis, T., and Dutka, A. (1989). Methyl prednisolone in the treatment of acute spinal cord decompression sickness. Undersea Biomed. Res. 16, 165–174.
Gibbison, B., Lopez-Lopez, J. A., Higgins, J. P., Miller, T., Angelini, G. D., Lightman, S. L., et al. (2017). Corticosteroids in septic shock: a systematic review and network meta-analysis. Crit Care 21:78. doi: 10.1186/s13054-017-1659-4
Halpern, P., Greenstein, A., Melamed, Y., Margulies, J. Y., and Robin, G. C. (1982). Spinal decompression sickness with delayed onset, delayed treatment, and full recovery. Br. Med. J. 284, 1014. doi: 10.1136/bmj.284.6321.1014
Inoue, K., and Takano, H. (2010). Urinary trypsin inhibitor as a therapeutic option for endotoxin-related inflammatory disorders. Expert Opin. Investig Drugs. 19, 513–520. doi: 10.1517/13543781003649533
Kanayama, N., Mashara, K., Suzuki, M., Fujise, Y., and Terao, T. (1997). The role of chondroitin sulfate chains of urinary trypsin inhibitor in inhibition of LPS-induced increase of cytosolic free Ca2+ in HL60 cells and HUVEC cells. Biochem. Biophys. Res. Commun. 238, 560–564. doi: 10.1006/bbrc.1997.7344
Kertmen, H., Gürer, B., Yılmaz, E. R., Sanlı, A. M., Sorar, M., Arıkök, A. T., et al. (2013). The protective effect of low-dose methotrexate on ischemia-reperfusion injury of the rabbit spinal cord. Eur. J. Pharmacol. 714, 148–156. doi: 10.1016/j.ejphar.2013.05.012
Kizer, K. W. (1981). Corticosteroids in treatment of serious decompression sickness. Ann. Emerg. Med. 10, 485–488. doi: 10.1016/s0196-0644(81)80285-5
Lambrechts, K., Pontier, J. M., Mazur, A., Theron, M., Buzzacott, P., Wang, Q., et al. (2015). Mechanism of action of antiplatelet drugs on decompression sickness in rats: a protective effect of anti-GPIIbIIIa therapy. J. Appl. Physiol. 118, 1234–1239. doi: 10.1152/japplphysiol.00125.2015
Levett, D. Z., and Millar, I. L. (2008). Bubble trouble: a review of diving physiology and disease. Postgrad Med. J. 84, 571–578. doi: 10.1136/pgmj.2008.068320
Levin, L. L., Stewart, G. J., Lynch, P. R., and Bove, A. A. (1981). Blood and blood vessel wall changes induced by decompression sickness in dogs. J. Appl. Physiol 50, 944–949. doi: 10.1152/jappl.1981.50.5.944
Li, M., Shen, J., Zou, F., Li, B., and Fan, M. (2017). Effect of ulinastatin on the permeability of the blood-brain barrier on rats with global cerebral ischemia/reperfusion injury as assessed by MRI. Biomed. Pharmacother. 85, 412–417. doi: 10.1016/j.biopha.2016.11.044
Li, S., Yang, W., Zhou, L., Nie, D., and Yu, H. (2019). Vascular permeability and hemodynamic effects of ulinastatin on organs affected by shock during early burn injury. Am. J. Emerg. Med. 37, 249–253. doi: 10.1016/j.ajem.2018.05.038
Linder, A., and Russell, J. A. (2014). An exciting candidate therapy for sepsis: ulinastatin, a urinary protease inhibitor. Intensive Care Med. 40, 1164–1167. doi: 10.1007/s00134-014-3366-9
Liu, S., Xu, J., Gao, Y., Shen, P., Xia, S., Li, Z., et al. (2018). Multi-organ protection of ulinastatin in traumatic cardiac arrest model. World J. Emerg Surg. 13:51. doi: 10.1186/s13017-018-0212-3
Liu, W., Pang, G., Wang, S., and Sun, A. (2017). Protective effect of ulinastatin on severe pulmonary infection under immunosuppression and its molecular mechanism. Exp. Ther. Med. 14, 3583–3588. doi: 10.3892/etm.2017.4993
Medzhitov, R. (2008). Origin and physiological roles of inflammation. Nature 454, 428–435. doi: 10.1038/nature07201
Meng, W. T., Qing, L., Yi, H. J., and Xu, W. G. (2018). Establishment of a rabbit decompression sickness model and its evaluation system. Acad. J. Second Milit. Med. Univ. 39, 33–39.
Møllerløkken, A., Blogg, S. L., Doolette, D. J., Nishi, R. Y., and Pollock, N. W. (2016). Consensus guidelines for the use of ultrasound for diving research. Diving Hyperb. Med. 46, 26–32.
Montcalm-Smith, E. A., Fahlman, A., and Kayar, S. R. (2008). Pharmacological interventions to decompression sickness in rats: comparison of five agents. Aviat Space Environ. Med. 79, 7–13. doi: 10.3357/asem.2071.2008
Moon, R. E. (2009). Adjunctive therapy for decompression illness: a review and update. Diving Hyperb. Med. 39, 81–87.
Nakahama, H., Kuribayashi, K., Sugita, M., and Shinkawa, T. (1999). Cytoprotective effect of ulinastatin, a Kunitz-type protease inhibitor, on hypoxic injury in L2 cells treated with antimycin A via stabilization of lysosomal fragility. Pulm. Pharmacol. Ther. 12, 1–6. doi: 10.1006/pupt.1998.9998
Nakatani, K., Takeshita, S., Tsujimoto, H., Kawamura, Y., and Sekine, I. (2001). Inhibitory effect of serine protease inhibitors on neutrophil-mediated endothelial cell injury. J. Leukoc Biolo. 69, 241–247. doi: 10.1189/jlb.69.2.241
Nishiyama, T., Aibike, M., and Hanaoka, K. (1996). The effect of ulinastatin, a human protease inhibitor, on the transfusion-induced increase of plasma polymorphonuclear granulocyte elastase. Anesth Analg. 82, 108–112. doi: 10.1097/00000539-199601000-00019
Oh, T. K., Song, I. A., Lee, J. H., Lim, C., Jeon, Y. T., Bae, H. J., et al. (2019). Effect of preadmission glucocorticoid therapy on 30-day mortality in critically ill patients: a retrospective study of a mixed ICU population in a tertiary hospital. Ann. Intensive Care 9:8. doi: 10.1186/s13613-019-0489-8
Ohbishi, H., Kosuzume, H., Ashida, Y., Kato, K., and Honjo, I. (1984). Effects of urinary trypsin inhibitor on pancreatic enzymes and experimental acute pancreatitis. Dig Dis Sci. 29, 26–32. doi: 10.1007/bf01296858
Okuhama, Y., Shiraishi, M., Higa, T., Tomori, H., Taira, K., Mamadi, T., et al. (1999). Protective effects of ulinastatin against ischemia-reperfusion injury. J. Surg Res. 82, 34–42. doi: 10.1006/jsre.1998.5496
Papadopoulo, P., Tan, M. X., Balestra, C., Eckersley, R. J., and Karapantsios, T. D. (2014). Circulatory bubble dynamics: from physical to biological aspects. Adv. Colloid. Interface Sci. 206, 239–249. doi: 10.1016/j.cis.2014.01.017
Park, K. H., Lee, K. H., Kim, H., and Hwang, S. O. (2010). The anti-inflammatory effects of ulinastatin in trauma patients with hemorrh shock. J. Korean Med. Sci. 25, 128–134. doi: 10.3346/jkms.2010.25.1.128
Qing, L., Ariyadewa, D. K., Yi, H., Wang, Y., Zhou, Q., and Xu, W. (2017). Skin lesions in swine with decompression sickness: clinical appearance and pathogenesis. Front. Physiol. 8:540. doi: 10.3389/fphys.2017.00540
Qing, L., Meng, W., Zhang, W., Yi, H. J., Zhang, K., Ariyadewa, D. K., et al. (2019). Benefits of escin for decompression sickness in bama pigs by endothelial-targeting protection. Front. Physiol. 10:605. doi: 10.3389/fphys.2019.00605
Qing, L., Yi, H. J., Wang, Y. W., Zhou, Q., Ariyadewa, D. K., and Xu, W. G. (2018). Benefits of hyperbaric oxygen pretreatment for decompression sickness in Bama pigs. J. Exp. Biol. 221:jeb171066. doi: 10.1242/jeb.171066
Takada, K., Komori, M., Notoya, A., Tomizawa, Y., and Ozaki, M. (2003). Effect of ulinastatin on microcirculation during excessive hemorrhage using fluid therapy. In vivo 17, 129–135. doi: 10.1159/000346205
Tanaka, R., Fujita, M., Tsuruta, R., Fujimoto, K., Aki, H. S., Kumagai, K., et al. (2010). Urinary trypsin inhibitor suppresses excessive generation of superoxide anion radical, systemic inflammation, oxidative stress, and endothelial injury in endotoxemic rats. Inflamm Res. 59, 597–596. doi: 10.1007/s00011-010-0166-8
Thom, S. R., Bhopale, V. M., Yu, K., and Yang, M. (2018). Provocative decompression causes diffuse vascular injury in mice mediated by microparticles containing interleukin-1β. J. Appl. Physiol. [Epub ahead of print].
Tsujimura, S., Saito, K., Nakayamada, S., and Tanaka, Y. (2008). Bolus infusion of human urinary trypsin inhibitor improves intractable interstitial pneumonia in patients with connective tissue diseases. Rheumatology 47, 907–913. doi: 10.1093/rheumatology/ken067
Tsujino, T., Komatsu, Y., Isayama, H., Hirano, K., Sasahira, N., Yamamoto, N., et al. (2005). Ulinastatin for pancreatitis after endoscopic retrograde cholangiopancreatography: a randomized, controlled trial. Clin. Gastroenterol. Hepatol. 3, 376–383. doi: 10.1016/s1542-3565(04)00671-8
Vann, R. D., Butler, F. K., Mitchell, S. J., and Moon, R. E. (2011). Decompression illness. Lancet 377, 153–164. doi: 10.1016/S0140-6736(10)61085-9
Walje, A. K., Rogers, M. A., Lin, P., Singal, A. G., Stein, J. D., Marks, R. M., et al. (2017). Short term use of oral corticosteroids and related harms among adults in the United States: population based cohort study. BMJ 357:j1415. doi: 10.1136/bmj.j1415
Wang, J., Wu, A., and Wu, Y. (2017). Endothelial glycocalyx layer: a possible therapeutic target for acute lung injury during lung resection. Biomed. Res. Int. 2017:5969657. doi: 10.1155/2017/5969657
Wang, L. Z., Luo, M. Y., Zhang, J. S., Ge, F. G., Chen, J. L., and Zheng, C. Q. (2016). Effect of ulinastatin on serum inflammatory factors in Asian patients with acute pancreatitis before and after treatment: a meta-analysis. Int. J. Clin. Pharmacol. Ther. 54, 890–898. doi: 10.5414/CP202454
We, F., Liu, S., Luo, L., Gu, N., Zeng, Y., Chen, X., et al. (2017). Anti-inflammatory mechanism of ulinastatin: Inhibiting the hyperpermeability of vascular endothelial cells induced by TNF-α via the RhoA/ROCK signal pathway. Int. Immunopharmacol. 46, 220–227. doi: 10.1016/j.intimp.2017.03.007
Xu, C. E., Zhang, M. Y., Zou, C. W., and Guo, L. (2012). Evaluation of the pharmacological function of ulinastatin in experimental animals. Molecules 17, 9070–9080. doi: 10.3390/molecules17089070
Yano, T., Anraku, S., Nakayama, R., and Ushijima, K. (2003). Neuroprotective effect of urinary trypsin inhibitor against focal cerebral ischemia-reperfusion injury in rats. Anesthesiology 98, 465–473. doi: 10.1097/00000542-200302000-00028
Yoo, J. W., Ryu, J. K., Lee, S. H., Woo, S. M., Park, J. K., Yoon, W. J., et al. (2008). Preventive effects of ulinastatin on post-endoscopic retrograde cholangiopancreatography pancreatitis in high-risk patients: a prospective, randomized, placebo-controlled trial. Pancreas 37, 366–370. doi: 10.1097/MPA.0b013e31817f528f
Zhang, K., Wang, M., Wang, H., Liu, Y., Buzzacott, P., and Xu, W. (2017). Time course of endothelial dysfunction induced by decompression bubbles in rats. Front. Physiol. 8:181. doi: 10.3389/fphys.2017.00181
Keywords: ulinastatin, decompression sickness, inflammation, endothelial injury, rabbit
Citation: Meng W, Qing L, Li C, Zhang K, Yi H, Zhao X and Xu W (2020) Ulinastatin: A Potential Alternative to Glucocorticoid in the Treatment of Severe Decompression Sickness. Front. Physiol. 11:273. doi: 10.3389/fphys.2020.00273
Received: 01 February 2020; Accepted: 10 March 2020;
Published: 26 March 2020.
Edited by:
François Guerrero, Université de Bretagne Occidentale, FranceReviewed by:
Jacek Kot, Medical University of Gdańsk, PolandJacky Lautridou, Norwegian University of Science and Technology, Norway
Copyright © 2020 Meng, Qing, Li, Zhang, Yi, Zhao and Xu. This is an open-access article distributed under the terms of the Creative Commons Attribution License (CC BY). The use, distribution or reproduction in other forums is permitted, provided the original author(s) and the copyright owner(s) are credited and that the original publication in this journal is cited, in accordance with accepted academic practice. No use, distribution or reproduction is permitted which does not comply with these terms.
*Correspondence: Wei-gang Xu, d2dfaHN1QDE2My5jb20=
†These authors have contributed equally to this work