- Department of Infection Biology, Institute of Infection and Global Health, University of Liverpool, Liverpool, United Kingdom
Ticks and the diseases they transmit are of huge veterinary, medical and economic importance worldwide. Control of ticks attacking livestock and companion animals is achieved primarily by application of chemical or plant-based acaricides. However, ticks can rapidly develop resistance to any new product brought onto the market, necessitating an ongoing search for novel active compounds and alternative approaches to tick control. Many aspects of tick and tick-borne pathogen research have been facilitated by the application of continuous cell lines derived from some of the most economically important tick species. These include cell lines derived from acaricide-susceptible and resistant ticks, cell sub-lines with in vitro-generated acaricide resistance, and genetically modified tick cells. Although not a replacement for the whole organism, tick cell lines enable studies at the cellular and molecular level and provide a more accessible, more ethical and less expensive in vitro alternative to in vivo tick feeding experiments. Here we review the role played by tick cell lines in studies on acaricide resistance, mode-of-action of acaricides, identification of potential novel control targets through better understanding of tick metabolism, and anti-tick vaccine development, that may lead to new approaches to control ticks and tick-borne diseases.
Introduction
Ticks are obligate, haematophagous ectoparasites. Like mosquitoes, they cause problems to their hosts both from skin irritation and damage during blood feeding and by transmitting numerous pathogens including viruses, bacteria and protozoa. Consequently, ticks and tick-borne pathogens (TBPs) are of huge veterinary, medical and economic importance due to their negative impact on the health of humans and domestic animals (de la Fuente et al., 2008). Therefore, many control strategies have been devised to eliminate or avoid ticks and reduce the spread of TBPs, such as personal protection, landscape management, chemicals, vaccination, and biological control (Stafford et al., 2017). Amongst these, chemical control using a variety of acaricidal compounds is still the main strategy for controlling ticks affecting livestock and companion animals. However, many tick species develop resistance to these chemicals, which has led to attempts to find natural alternatives by studying the effects of some natural products on ticks and TBPs (Rodriguez-Vivas et al., 2018). Globally, the most economically important species, the invasive Asian cattle tick Rhipicephalus (Boophilus) microplus, is also the most notorious for rapid development of resistance to every new acaricidal product brought onto the market (Jonsson and Hope, 2007; Abbas et al., 2014). Acaricide-resistant R. microplus are widespread throughout tropical and sub-tropical Asia, Latin America and Australia (Abbas et al., 2014), and are rapidly spreading in Southern, Central and West Africa (Nyangiwe et al., 2018; Silatsa et al., 2019).
Tick cell lines have an invaluable role to play in many aspects of tick and TBP research including tick biology, host-vector-pathogen relationships, genetic manipulation, genomics and proteomics (Bell-Sakyi et al., 2007). In addition, tick cell lines play a major role as essential tools in in vitro studies to examine and assess the impact of chemical molecules and vaccines against ticks and TBPs, as well as to investigate the mechanisms of resistance and tick metabolism which can lead to the development of novel approaches to control ticks and TBPs (Bell-Sakyi et al., 2018). While >60 continuous cell lines have now been established from 16 ixodid and three argasid tick species (Bell-Sakyi et al., 2018), studies on tick and pathogen control have predominantly centered around cell lines derived from, not surprisingly, R. microplus. A smaller number of studies have used cell lines derived from other tropical Rhipicephalus species of veterinary importance, from Ixodes ricinus, a European tick that feeds on a wide range of domestic and wild animal hosts as well as attacking humans, and from the medically important North American species Ixodes scapularis (Table 1).
This Mini Review focuses on the role of tick cell lines in studies on control of ticks and the few studies dealing with approaches to combined control of ticks and TBPs. While tick cell lines have been used in many studies contributing to aspects of pathogen control per se, these are beyond the scope of our review. Here we review selected studies to highlight how tick cell lines can contribute to tick control research, bearing in mind that results gained in vitro should be validated in vivo.
Tick Cell Lines in Studies on Acaricide Resistance
Tick cell lines have been investigated for their potential as cheap and ethical tools to study acaricide resistance. Some studies focused on establishing drug-resistant cell lines which provide a useful tool to study and understand the mechanisms of resistance, an important step toward improving detection and prevention of tick resistance against acaricides. Tick cell lines are also a useful resource for identifying genes responsible for acaricide resistance and some published studies have focused on this aspect.
Generation and Application of Acaricide-Resistant Tick Cell Lines
Cossio-Bayugar et al. (2002b) generated three organophosphate-resistant R. microplus cell sub-lines by exposing the R. microplus BmVIII-SCC cell line (Holman, 1981), originally derived from embryos of acaricide-susceptible ticks, to gradually increasing concentrations of the organophosphate compound coumaphos (Table 2). They then assessed the levels of esterase, an enzyme that, when present at high levels, is responsible for causing resistance by modifying the organophosphate target site to be less sensitive. After exposure to coumaphos, there were higher levels of esterase in the resistant sub-lines compared to the original susceptible cell line. These findings, which were in line with previous in vivo studies (Miranda et al., 1995; Rosario-Cruz et al., 1997), validated the use of tick cell lines to study development of resistance in ticks, which could thereafter help to determine a suitable approach to formulating acaricides that would be less likely to induce resistance.
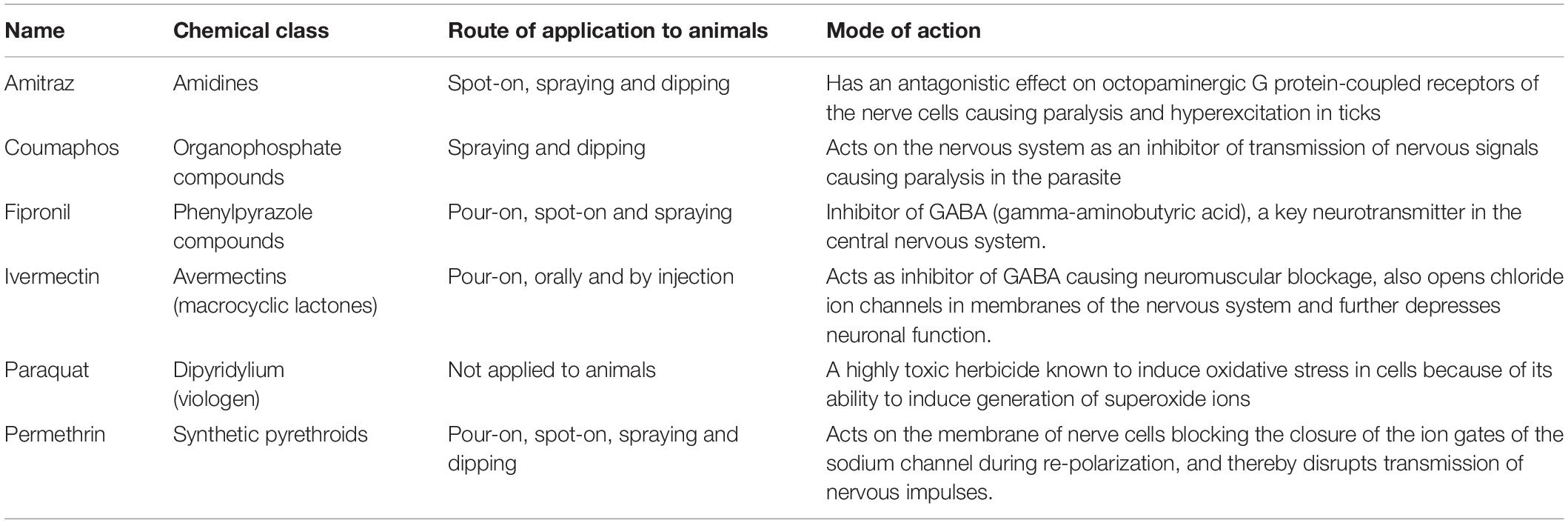
Table 2. Acaricides and pesticides used with tick cell lines in studies on tick control reviewed in this article.
This group then used the coumaphos-resistant R. microplus cell sub-lines to evaluate the mechanisms that may contribute to acaricide resistance by further characterizing them compared to susceptible control cells (Cossio-Bayugar et al., 2002a). In addition to higher esterase levels, they found that the resistant cells exhibited higher levels of intracellular calcium and glutathione, decreased glutathione S-transferase activity, and reduced plasma and mitochondrial membrane potentials. Their results were consistent with the in vivo observations of increased esterase activity in organophosphate-resistant ticks, as well as resistance mechanisms found in other cell systems. One of the coumaphos-resistant R. microplus cell sub-lines played an important role in a further study (Cossio-Bayugar et al., 2005) that identified amino acid substitutions in the protein encoded by the phospholipid hydroperoxide glutathione peroxidase (PHGPx) gene in susceptible and resistant R. microplus, highlighting the possibility of its relationship with acaricide resistance in these tick populations.
A study conducted by Pohl et al. (2014) also focused on understanding the mechanisms of acaricide resistance in R. microplus. In this study, the authors established an ivermectin-resistant sub-line (BME26-IVM) of the R. microplus embryo-derived cell line BME26 (Kurtti et al., 1988; Esteves et al., 2008) by exposing susceptible cells to increasing concentrations of ivermectin (Table 2) over 46 weeks. Using the BME26-IVM sub-line, the contribution of the ATP-binding cassette (ABC) transporter mechanism was investigated by evaluating mRNA expression using quantitative RT-PCR. This study demonstrated increased levels of ABC transporter gene transcripts in the ivermectin-resistant cells compared to the original susceptible BME26 cells.
Effect of Acaricide Treatment of “Susceptible” Tick Cell Lines on Expression of Genes Associated With Resistance
Taking a different approach and using a cell line expected to be susceptible to ivermectin, Mangia et al. (2016) evaluated the expression of selected members of the ABC transporter subfamily B genes encoding P-glycoproteins (PgPs) in the I. ricinus embryo-derived cell line IRE/CTVM19 following treatment with ivermectin. The authors showed that ABC pump expression was not significantly modulated by ivermectin treatment, and expression of one of the subfamily genes was not detected. Interestingly, this study revealed that the IRE/CTVM19 cell line was able to tolerate a much higher concentration of ivermectin (30 μg/ml) than the R. microplus-derived line BME26 that did not survive after exposure to 12.5 μg/ml ivermectin (Pohl et al., 2014). This could have been due to biological differences between the two tick species from which the cell lines were derived, differences in the phenotypic composition of the cell lines and/or in the culture conditions used. This should be considered when conducting such in vitro experiments using tick cell lines.
In a further study, Mangia et al. (2018) treated IRE/CTVM19 cultures with different concentrations of the acaricides permethrin, amitraz and fipronil (Table 2). They assessed cell viability, morphology, metabolic activity and expression of ABC genes at day 10 post-treatment. In general, the results showed that fipronil had the greatest significant negative effects on cell viability and metabolic activity, followed by permethrin, while amitraz only caused a significant decrease in these parameters at the highest concentration tested. The negative effects of fipronil and permethrin were confirmed by their effects on ABC gene expression with upregulation of ABCB6, ABCB8, and ABCB10, while amitraz had little or no effect on expression of any of the ABC genes tested.
Exploiting Tick Cell Lines Derived From Susceptible and Resistant Ticks to Identify Genes Involved in Acaricide Resistance
For some of the currently available R. microplus cell lines, the acaricide resistance status of the parent ticks from which the cultures were derived is known. As already mentioned, the parent BmVIII-SCC and BME26 cells used by, respectively, Cossio-Bayugar et al. (2002b) and Pohl et al. (2014) to derive acaricide-resistant sub-lines in vitro were originally susceptible to the compounds used, implying that the parent ticks of both lines, originally from Mexico, were also acaricide-susceptible. Two additional R. microplus cell lines, BME/CTVM2 (Bell-Sakyi, 2004) and BME/CTVM4 (Bell-Sakyi et al., 2007), were derived from known acaricide-susceptible ticks from Costa Rica, while two other cell lines, BME/CTVM5 (Bell-Sakyi et al., 2007) and BME/CTVM6 (Bell-Sakyi, 2004) were derived from Colombian ticks known to be resistant to organophosphates, organochlorines and amitraz (Koh-Tan et al., 2016). While these cells whose natural resistance status derives from their parent ticks offer a potentially very interesting complement to the in vitro-derived resistant R. microplus cell lines of Cossio-Bayugar et al. (2002b) and Pohl et al. (2014), to date they have only been exploited in a single study. Koh-Tan et al. (2016) investigated the expression of the β-adrenergic octopamine receptor (βAOR) gene, associated with amitraz resistance, and the ATP-binding cassette B10 (ABCB10) gene, associated with macrocyclic lactone resistance, in tick cell lines derived from R. microplus, including BmVIII-SCC, BME/CTVM2, BME/CTVM5 and BME/CTVM6, Rhipicephalus appendiculatus, Rhipicephalus sanguineus, and Rhipicephalus evertsi (Table 1). Expression of the βAOR gene was detected in all the Rhipicephalus spp. cell lines, but in BME/CTVM6, derived from amitraz-resistant ticks, a novel larger amplicon was detected. This was found to encode a 12-amino acid insertion identified as a duplication of the immediately upstream sequence; the insertion was also present in genomic DNA from BME/CTVM5 cells, similarly derived from amitraz-resistant ticks, but transcription of the novel amplicon was not detectable. Expression of the ABC10 gene was approximately seven-fold higher in BME/CTVM6 cells compared to all other cell lines examined including BME/CTVM5. These findings suggest that BME/CTVM6 cells in particular could be a useful model system for studying resistance to multiple acaricide groups in R. microplus; further experiments are needed to determine whether additional genes are mutated in this cell line.
Tick Cell Lines Elucidate Novel Aspects of Tick Cell Metabolism to Identify Potential Control Targets
Tick cell lines have also played a valuable role in the study of tick cell metabolism which can help in developing novel control methods. De Abreu et al. (2013) investigated the role of protein kinase B (AKT) and glycogen synthase kinase 3 (GSK3) in glycogen metabolism and cell viability using the R. microplus cell line BME26, and the results revealed an antagonistic role for AKT and GSK3. A reduction in AKT caused a decrease in glycogen content, cell viability and altered cell membrane permeability, whereas the inhibition of GSK3 promoted an increase in glycogen content.
To better understand energy metabolism during embryonic development in ticks, Da Silva et al. (2015) used the BME26 cell line to evaluate several genes involved in gluconeogenesis, glycolysis and glycogen metabolism, the major pathways for carbohydrate catabolism and anabolism. Their results showed that several genes displayed mutual regulation in response to glucose treatment, and the transcription of gluconeogenic genes in tick cells is controlled by GSK3.
The BME26 cell line also played an essential and effective role in the first report on characterization of a cell cycle protein in arachnids, and the reversal of its functions with an inhibitor (Gomes et al., 2013). The BME26 cell line was used in an in vitro inhibition assay to determine the inhibitory effects of roscovitine, a purine derivative, on tick cyclin-dependent kinases (CDKs), which are essential for cell cycle progression, and the results suggested that CDKs may present an alternative strategy for designing a novel acaricide that targets both oogenesis and embryogenesis processes.
The I. scapularis embryo-derived tick cell line ISE6 (Kurtti et al., 1996) is the one of the most widely used tick cell lines due to its susceptibility to a wide range of TBPs (Oliver et al., 2015). ISE6 cells were used by Cabezas-Cruz et al. (2017) to study possible control of infection with the obligate intracellular bacterium Anaplasma phagocytophilum, causative agent of disease in humans, ruminants, horses and dogs, by increasing the synthesis of phosphoenolpyruvate from tyrosine. Ferritins (FER) are iron-storage proteins that act to store excess iron available in the cellular iron pool by the binding and oxidation of the ferrous ion (Fe2+) and the formation of ferric ion (Fe3+) in the FER core cavity (Galay et al., 2015). Hernandez et al. (2018) were able to induce intracellular ferritin (FER1) protein expression by exposing ISE6 cells to various concentrations of ferrous sulfate. They showed that silencing of FER1 expression by RNA interference led to a decrease in ISE6 cell proliferation and an increase in mortality, concluding that ISE6 cells could be a good tool to further understanding of the mechanism of FER1 action. Thereafter, Hernandez et al. (2019) characterized the activity of an iron-inducible tick promoter derived from the tick Haemaphysalis longicornis, previously identified by Kusakisako et al. (2018b), in ISE6 cells, which could be a valuable tool in the development of a gene-manipulation system to control ticks and TBPs. Kusakisako et al. (2018a) established methods to visualize H2O2 in ISE6 cells using an intracellular H2O2 probe and observed that paraquat, known to induce oxidative stress in mammalian cells (Table 2), was also effective in this respect in ISE6 tick cells. Iron derived from host blood may react with oxygen in the tick’s body and lead to high levels of H2O2, causing oxidative stress such as DNA strand breaks, lipid peroxidation and degradation of other molecules (Kusakisako et al., 2018a; Hernandez et al., 2019).
Genetic Manipulation of Tick Cell Lines
The development of new strategies for control of ticks could be greatly enhanced by exploring genetic manipulation of ticks and tick cell lines. Demonstrating proof of concept, Kurtti et al. (2006) first reported stable transfection of the I. scapularis cell line ISE6 with a gene encoding a fluorescent protein using the Sleeping Beauty transposon system. In a different approach, Cossio-Bayugar and Miranda-Miranda (2007) reported retroviral transfection of primary R. microplus cell cultures with green fluorescent protein; fluorescence was detectable for up to 2 weeks following retroviral transfection. Using yet another approach, Machado-Ferreira et al. (2015) achieved the expression of transgenes in R. microplus ticks and the cell line BME/CTVM2 using Agrobacterium tumefaciens T-DNA constructs; evidence of transcription and protein synthesis were detectable for 2–3 weeks by fluorescence microscopy, western blot analyses and RT-PCR. These studies demonstrate that genetic manipulation approaches used with other animal cell systems can equally be applied to tick cell lines, and raise the possibility of modifying ticks to render them incapable of developing acaricide resistance.
Tick Cell Lines Contribute to Anti-Tick Vaccine Development
Vaccination with recombinant tick antigens represents a promising tick control strategy. Vaccines containing recombinant tick antigens demonstrated advantages including reduction in acaricide application, cost-effectiveness and reducing the prevalence of tick-borne diseases through reducing exposure of cattle to infected ticks. However, the efficacies of these vaccines vary considerably between tick species and geographic location (de la Fuente et al., 2007). Tick cell lines have played a central role in identifying tick protective antigens to produce a variety of vaccines that contribute to the prevention and control of tick infestations and TBP infections. For example, three embryo-derived tick cell lines, R. microplus BME/CTVM2 and I. scapularis IDE8 (Munderloh et al., 1994) and ISE6, were used in a study conducted by Antunes et al. (2014) to identify tick proteins involved in tick-pathogen interactions. The aim was to identify candidate protective antigens that could be used as vaccines for the control of cattle infestations with R. microplus ticks and infection with the TBPs Anaplasma marginale and Babesia bigemina transmitted by R. microplus. As an important part of the experiment, antibodies produced against the R. microplus protein subolesin were tested using immunofluorescence and showed positive reactions in the three cell lines, but no effect was observed on pathogen DNA levels. In contrast, using the I. scapularis cell line ISE6 and the I. ricinus cell line IRE/CTVM20 (Bell-Sakyi et al., 2007), Contreras et al. (2017) determined that two candidate tick-protective antigens – I. scapularis lipocalin (ISCW005600) and lectin pathway inhibitor (AAY66632), and their I. ricinus homologs – constitute candidate protective antigens for the control of tick infestations as well as A. phagocytophilum infection. They showed that anti ISCW005600 and AAY66632 IgG significantly increased the percentage of apoptotic tick cells and decreased pathogen infection, which could help in the development of effective anti-tick and anti-pathogen vaccines.
Conclusion
Ticks and TBPs have huge negative impacts on global livestock and public health and national economies. Scientific research has focused on creating and developing a variety of strategies to prevent and control ticks and TBPs. Tick cell lines represent essential tools in this area of research: in vitro-generated acaricide-resistant cell lines, cell lines derived from acaricide-resistant and susceptible ticks, genetically modified cell lines and cell lines supporting growth of intracellular TBPs all have a role to play. Experimental parameters can be easily controlled, effects at the cellular and molecular level can be determined, and cell lines reduce, while not ultimately replacing, the need for expensive in vivo studies involving maintenance of tick colonies and feeding on laboratory animals. As the numbers of cell lines derived from economically important tick species increase, so do the opportunities for exploiting them in a wide range of studies. For example, the potential for developing high-throughput preliminary screens of novel, potentially acaricidal compounds using cell lines from multiple tick species remains unexplored; such screens could greatly increase the number of active compounds identified for further testing at relatively low cost, and could be applied both to compound libraries held by pharmaceutical companies and to natural plant products. It is anticipated that gene editing of tick cell lines by technologies such as CRISPR will add another facet to the control portfolio. The Tick Cell Biobank (Bell-Sakyi et al., 2018), which houses all the cell lines mentioned in this review as well as cell lines derived from many other species of veterinary and medical concern, will continue to underpin research on tick and TBP control.
Author Contributions
AA-R drafted the manuscript, LB-S edited the draft and both authors produced and agreed the final version.
Funding
AA-R is supported by the Council for At-Risk Academics (CARA) and the University of Liverpool. LB-S is supported by the United Kingdom Biotechnology and Biological Sciences Research Council grant number BB/P024270/1.
Conflict of Interest
The authors declare that the research was conducted in the absence of any commercial or financial relationships that could be construed as a potential conflict of interest.
The reviewer UM declared a past co-authorship with one of the authors LB-S to the handling Editor.
Acknowledgments
We would like to thank staff of the Council for At-Risk Academics (CARA) and Dr. Benjamin Makepeace for their instrumental roles in bringing AA-R to the University of Liverpool.
References
Abbas, R. Z., Zaman, M. A., Colwell, D. D., Gilleard, J., and Iqbal, Z. (2014). Acaricide resistance in cattle ticks and approaches to its management: the state of play. Vet. Parasitol. 203, 6–20. doi: 10.1016/j.vetpar.2014.03.006
Alberdi, M. P., Dalby, M. J., Rodriguez-Andres, J., Fazakerley, J. K., Kohl, A., and Bell-Sakyi, L. (2012). Detection and identification of putative bacterial endosymbionts and endogenous viruses in tick cell lines. Ticks Tick Borne Dis. 3, 137–146. doi: 10.1016/j.ttbdis.2012.05.002
Antunes, S., Merino, O., Mosqueda, J., Moreno-Cid, J. A., Bell-Sakyi, L., Fragkoudis, R., et al. (2014). Tick capillary feeding for the study of proteins involved in tick-pathogen interactions as potential antigens for the control of tick infestation and pathogen infection. Parasit. Vectors 7:42. doi: 10.1186/1756-3305-7-42
Bekker, C. P. J., Bell-Sakyi, L., Paxton, E. A., Martinez, D., Bensaid, A., and Jongejan, F. (2002). Transcriptional analysis of the major antigenic protein 1 multigene family of Cowdria ruminantium. Gene 285, 193–201. doi: 10.1016/s0378-1119(02)00408-0
Bell-Sakyi, L. (2004). Ehrlichia ruminantium grows in cell lines from four ixodid tick genera. J. Comp. Pathol. 130, 285–293. doi: 10.1016/j.jcpa.2003.12.002
Bell-Sakyi, L., Darby, A., Baylis, M., and Makepeace, B. L. (2018). The tick cell biobank: a global resource for in vitro research on ticks, other arthropods and the pathogens they transmit. Ticks Tick Borne Dis. 9, 1364–1371. doi: 10.1016/j.ttbdis.2018.05.015
Bell-Sakyi, L., Palomar, A., Bradford, E. L., and Shkap, V. (2015). Propagation of the Israeli vaccine strain of Anaplasma centrale in tick cell lines. Vet. Microbiol. 179, 270–276. doi: 10.1016/j.vetmic.2015.07.008
Bell-Sakyi, L., Zweygarth, E., Blouin, E. F., Gould, E. A., and Jongejan, F. (2007). Tick cell lines: tools for tick and tick-borne disease research. Trends Parasitol. 23, 450–457. doi: 10.1016/j.pt.2007.07.009
Cabezas-Cruz, A., Espinosa, P. J., Obregon, D. A., Alberdi, P., and de la Fuente, J. (2017). Ixodes scapularis tick cells control Anaplasma phagocytophilum infection by increasing the synthesis of phosphoenolpyruvate from tyrosine. Front. Cell. Infect. Microbiol. 7:375. doi: 10.3389/fcimb.2017.00375
Contreras, M., Alberdi, P., Fernandez de Mera, I. G., Krull, C., Nijhof, A., Villar, M., et al. (2017). Vaccinomics approach to the identification of candidate protective antigens for the control of tick vector infestations and Anaplasma phagocytophilum infection. Front. Cell. Infect. Microbiol. 7:360. doi: 10.3389/fcimb.2017.00360
Cossio-Bayugar, R., Barhoumi, R., Burghardt, R. C., Wagner, G. G., and Holman, P. J. (2002a). Basal cellular alterations of esterase, glutathione, glutathione S-transferase, intracellular calcium, and membrane potentials in coumaphos-resistant Boophilus microplus (Acari: Ixodidae) cell lines. Pesticide Biochem. Physiol. 72, 1–9. doi: 10.1006/pest.2001.2578
Cossio-Bayugar, R., Miranda, E., and Holman, P. J. (2005). Molecular cloning of a phospholipid-hydroperoxide glutathione peroxidase gene from the tick Boophilus microplus (Acari: Ixodidae). Insect Biochem. Molec. Biol. 35, 1378–1387. doi: 10.1016/j.ibmb.2005.08.008
Cossio-Bayugar, R., and Miranda-Miranda, E. (2007). Heterologus gene expression in a cattle tick Rhipicephalus microplus embryonic cell culture. J. Anim. Vet. Adv. 6, 1214–1218.
Cossio-Bayugar, R., Wagner, G. G., and Holman, P. J. (2002b). In vitro generation of organophosphate resistant Boophilus microplus (Acari: Ixodidae) cell lines. J. Med. Entomol. 39, 278–284. doi: 10.1603/0022-2585-39.2.278
Da Silva, R. M., Noce, B. D., Waltero, C. F., Costa, E. P., de Abreu, L. A., Githaka, N. W., et al. (2015). Non-classical gluconeogenesis-dependent glucose metabolism in Rhipicephalus microplus embryonic cell line BME26. Int. J. Mol. Sci. 16, 1821–1839. doi: 10.3390/ijms16011821
De Abreu, L. A., Calixto, C., Waltero, C. F., Della Noce, B. P., Githaka, N. W., Seixas, A., et al. (2013). The conserved role of the AKT/GSK3 axis in cell survival and glycogen metabolism in Rhipicephalus Boophilus microplus embryo tick cell line BME26. Biochim. Biophys. Acta 1830, 2574–2582. doi: 10.1016/j.bbagen.2012.12.016
de la Fuente, J., Almazán, C., Canales, M., Pérez de la Lastra, J. M., Kocan, K. M., and Willadsen, P. (2007). A ten-year review of commercial vaccine performance for control of tick infestations on cattle. Anim. Health Res. Rev. 8, 23–28. doi: 10.1017/s1466252307001193
de la Fuente, J., Estrada-Pena, A., Venzal, J. M., Kocan, K. M., and Sonenshine, D. E. (2008). Overview: ticks as vectors of pathogens that cause disease in humans and animals. Front. Biosci. 13:6938–6946. doi: 10.2741/3200
Esteves, E., Lara, F. A., Lorenzini, D. M., Costa, G. H. N., Fukuzawa, A. H., Pressinotti, L. N., et al. (2008). Cellular and molecular characterization of an embryonic cell line (BME26) from the tick Rhipicephalus (Boophilus) microplus. Insect Biochem. Mol. Biol. 38, 568–580. doi: 10.1016/j.ibmb.2008.01.006
Galay, R. L., Umemiya-Shirafuji, R., Mochizuki, M., Fujisaki, K., and Tanaka, T. (2015). Iron metabolism in hard ticks (Acari: Ixodidae): the antidote to their toxic diet. Parasitol. Int. 64, 182–189. doi: 10.1016/j.parint.2014.12.005
Gomes, H., Romeiro, N. C., Braz, G. R. C., de Oliveira, E. A. G., Rodrigues, C., da Fonseca, R. N., et al. (2013). Identification and structural-functional analysis of cyclin-dependent kinases of the cattle tick Rhipicephalus (Boophilus) microplus. PLoS One 8:e76128. doi: 10.1371/journal.pone.0076128
Hernandez, E. P., Kusakisako, K., Hatta, T., and Tanaka, T. (2019). Characterization of an iron-inducible Haemaphysalis longicornis tick-derived promoter in an Ixodes scapularis-derived tick cell line (ISE6). Parasit. Vectors 12:321. doi: 10.1186/s13071-019-3574-9
Hernandez, E. P., Kusakisako, K., Talactac, M. R., Galay, R. L., Yoshii, K., and Tanaka, T. (2018). Induction of intracellular ferritin expression in embryo-derived Ixodes scapularis cell line (ISE6). Sci. Rep. 8:16566. doi: 10.1038/s41598-018-34860-3
Holman, P. J. (1981). Partial characterization of a unique female diploid cell strain from the tick Boophilus microplus (Acari: Ixodidae). J. Med. Entomol. 18, 84–88. doi: 10.1093/jmedent/18.1.84
Jonsson, N. N., and Hope, M. (2007). Progress in the epidemiology and diagnosis of amitraz resistance in the cattle tick Boophilus microplus. Vet. Parasitol. 146, 193–198. doi: 10.1016/j.vetpar.2007.03.006
Koh-Tan, H. H. C., Strachan, E., Cooper, K., Bell-Sakyi, L., and Jonsson, N. N. (2016). Identification of a novel β-adrenergic octopamine receptor-like gene (βAOR-like) and increased ATP-binding cassette B10 (ABCB10) expression in a Rhipicephalus microplus cell line derived from acaricide-resistant ticks. Parasit. Vectors 9:425.
Kurtti, T. J., Felsheim, R. F., Mattila, J. T., Baldridge, G. D., Burkhardt, N. Y., and Munderloh, U. G. (2006). Stable transformation of a tick (Ixodes scapularis) cell line with the Sleeping Beauty transposon system. Soc. Vitro Biol. J. 42:32.
Kurtti, T. J., Munderloh, U. G., and Ahlstrand, G. G. (1988). Tick tissue and cell culture in vector research. Adv. Dis. Vector Res. 5, 87–109.
Kurtti, T. J., Munderloh, U. G., Andreadis, T. G., Magnarelli, L. A., and Mather, T. N. (1996). Tick cell culture isolation of an intracellular prokaryote from the tick Ixodes scapularis. J. Invert. Pathol. 67, 318–321. doi: 10.1006/jipa.1996.0050
Kusakisako, K., Hernandez, E. P., Talactac, M. R., Yoshii, K., Umemiya-Shirafuji, R., Fujisaki, K., et al. (2018a). Peroxiredoxins are important for the regulation of hydrogen peroxide concentrations in ticks and tick cell line. Ticks Tick Borne Dis. 9, 872–881. doi: 10.1016/j.ttbdis.2018.03.016
Kusakisako, K., Ido, A., Masatan, T., Morokuma, H., Hernandez, E. P., Talactac, M. R., et al. (2018b). Transcriptional activities of two newly identified Haemaphysalis longicornis tick-derived promoter regions in the Ixodes scapularis tick cell line (ISE6). Insect Mol. Biol. 27, 590–602. doi: 10.1111/imb.12497
Machado-Ferreira, E., Balsemão-Pires, E., Dietrich, G., Hojgaard, A., Vizzoni, V. F., Scoles, G., et al. (2015). Transgene expression in tick cells using Agrobacterium tumefaciens. Exp. Appl. Acarol. 67, 269–287. doi: 10.1007/s10493-015-9949-5
Mangia, C., Vismarra, A., Genchi, M., Epis, S., Bandi, C., Grandi, G., et al. (2018). Exposure to amitraz, fipronil and permethrin affects cell viability and ABC transporter gene expression in an Ixodes ricinus cell line. Parasit. Vectors 11:437. doi: 10.1186/s13071-018-3020-4
Mangia, C., Vismarra, A., Kramer, L., Bell-Sakyi, L., Porretta, D., Otranto, M., et al. (2016). Evaluation of the in vitro expression of ATP binding-cassette (ABC) proteins in an Ixodes ricinus cell line exposed to ivermectin. Parasit. Vectors 9:215. doi: 10.1186/s13071-016-1497-2
Miranda, E., Cossio-Bayugar, R., Tellez-Alanis, M. R., Garcia-Vazquez, Z., Rosario-Cruz, R., and Ortiz-Estrada, M. (1995). An enzymatic marker for ixodicide resistance detection in the cattle tick Boophilus microplus. Adv. Agric. Res. 4, 1–8.
Munderloh, U. G., Liu, Y., Wang, M., Chen, C., and Kurtti, T. J. (1994). Establishment, maintenance and description of cell lines from the tick Ixodes scapularis. J. Parasitol. 80, 533–543.
Nyangiwe, N., Yawa, M., and Muchenje, V. (2018). Driving forces for changes in geographic range of cattle ticks (Acari: Ixodidae) in Africa: a review. South Afr. J. Anim. Sci. 48, 829–841.
Oliver, J. D., Chavez, A. S., Felsheim, R. F., Kurtti, T. J., and Munderloh, U. G. (2015). An Ixodes scapularis cell line with a predominantly neuron-like phenotype. Exp. Appl. Acarol. 66, 427–442. doi: 10.1007/s10493-015-9908-1
Pohl, P. C., Carvalho, D. D., Daffre, S., da Silva Vaz, I., and Masuda, A. (2014). In vitro establishment of ivermectin-resistant Rhipicephalus microplus cell line and the contribution of ABC transporters on the resistance mechanism. Vet. Parasitol. 204, 316–322. doi: 10.1016/j.vetpar.2014.05.042
Rodriguez-Vivas, R. I., Jonsson, N. N., and Bhushan, C. (2018). Strategies for the control of Rhipicephalus microplus ticks in a world of conventional acaricide and macrocyclic lactone resistance. Parasitol. Res. 117, 3–29. doi: 10.1007/s00436-017-5677-5676
Rosario-Cruz, R., Miranda-Miranda, E., Garcia-Vazquez, Z., and Ortiz-Estrada, M. (1997). Detection of esterase activity in susceptible and organophosphate resistant strains of the cattle tick Boophilus microplus. Bull. Entomol. Res. 87, 197–202. doi: 10.1017/s0007485300027358
Silatsa, B. A., Simo, G., Githaka, N., Mwaura, S., Kamga, R. M., Oumarou, F., et al. (2019). A comprehensive survey of the prevalence and spatial distribution of ticks infesting cattle in different agro-ecological zones of Cameroon. Parasit. Vectors 12:489. doi: 10.1186/s13071-019-3738-7
Stafford, K. C., Williams, S. C., and Molaei, G. (2017). Integrated pest management in controlling ticks and tick-associated diseases. J. Integr. Pest Manag. 8:28. doi: 10.1093/jipm/pmx018
Varma, M. G. R., Pudney, M., and Leake, C. J. (1975). The establishment of three cell lines from the tick Rhipicephalus appendiculatus (Acari: Ixodidae) and their infection with some arboviruses. J. Med. Entomol. 11, 698–706. doi: 10.1093/jmedent/11.6.698
Keywords: Rhipicephalus microplus, Ixodes spp., control, tick cell line, acaricide, resistance, metabolism, anti-tick vaccine
Citation: Al-Rofaai A and Bell-Sakyi L (2020) Tick Cell Lines in Research on Tick Control. Front. Physiol. 11:152. doi: 10.3389/fphys.2020.00152
Received: 16 December 2019; Accepted: 12 February 2020;
Published: 25 February 2020.
Edited by:
Itabajara Silva Vaz Jr., Federal University of Rio Grande do Sul, BrazilReviewed by:
Eliane Esteves, University of São Paulo, BrazilUlrike G. Munderloh, University of Minnesota Twin Cities, United States
Copyright © 2020 Al-Rofaai and Bell-Sakyi. This is an open-access article distributed under the terms of the Creative Commons Attribution License (CC BY). The use, distribution or reproduction in other forums is permitted, provided the original author(s) and the copyright owner(s) are credited and that the original publication in this journal is cited, in accordance with accepted academic practice. No use, distribution or reproduction is permitted which does not comply with these terms.
*Correspondence: Lesley Bell-Sakyi, TC5CZWxsLVNha3lpQGxpdmVycG9vbC5hYy51aw==