- 1Natural Sciences and Science Education, National Institute of Education, Nanyang Technological University, Singapore, Singapore
- 2Department of Biological Sciences, National University of Singapore, Singapore, Singapore
The stinging catfish, Heteropneustes fossilis, can tolerate high concentrations of environmental ammonia. Previously, it was regarded as ureogenic, having a functional ornithine-urea cycle (OUC) that could be up-regulated during ammonia-loading. However, contradictory results indicated that increased urea synthesis and switching to ureotelism could not explain its high ammonia tolerance. Hence, we re-examined the effects of exposure to 30 mmol l–1 NH4Cl on its ammonia and urea excretion rates, and its tissue ammonia and urea concentrations. Our results confirmed that H. fossilis did not increase urea excretion or accumulation during 6 days of ammonia exposure, and lacked detectable carbamoyl phosphate synthetase I or III activity in its liver. However, we discovered that it could actively excrete ammonia during exposure to 8 mmol l–1 NH4Cl. As active ammonia excretion is known to involve Na+/K+-ATPase (Nka) indirectly in several ammonia-tolerant fishes, we also cloned various nkaα-subunit isoforms from the gills of H. fossilis, and determined the effects of ammonia exposure on their branchial transcripts levels and protein abundances. Results obtained revealed the presence of five nkaα-subunit isoforms, with nkaα1b having the highest transcript level. Exposure to 30 mmol l–1 NH4Cl led to significant increases in the transcript levels of nkaα1b (on day 6) and nkaα1c1 (on day 1 and 3) as compared with the control. In addition, the protein abundances of Nkaα1c1, Nkaα1c2, and total NKAα increased significantly on day 6. Therefore, the high environmental ammonia tolerance of H. fossilis is attributable partly to its ability to actively excrete ammonia with the aid of Nka.
Introduction
In fishes, proteins ingested would be first catabolized to amino acids and then further broken down through deamination or transamination with the eventual production of ammonia. Although the production of ammonia can occur in many tissues (Walton and Cowey, 1977), the liver is the main organ where it is produced (Pequin and Serfaty, 1963). Most teleost fishes are ammonotelic, keeping body ammonia levels low by excreting excess ammonia mainly as NH3 (>50% of the total nitrogenous wastes) across the gills (Wright et al., 1993). In aqueous solution, ammonia exists as molecular NH3 and cationic NH4+, based on the reaction NH3 + H3O+ ⇔ NH4+ + H2O with a pK of 9.0–9.5. Hence, acidic environmental conditions augment ammonia excretion, as NH3 diffusing across the branchial epithelium is converted into NH4+ and trapped in the external environment. By contrast, an increase in the pH of the environment would impede the diffusion of NH3 into the external medium leading to the accumulation of endogenous ammonia in the body. When fishes emerge from water, there could be a reduction in the excretion of ammonia due to a lack of water to flush the gills. Furthermore, ammonia concentrations in the ambient water could increase due to the decomposition of organic matters or the addition of fertilizers, and impede ammonia excretion in fishes.
As aquatic hypoxia is a frequent event in tropical waters, air-breathing is a common occurrence (Graham, 1997). With the development of air-breathing abilities, some tropical fishes can leave water and make short excursions on land, while some even burrow into semi-solid mud during drought. When confronted with terrestrial conditions or low levels of environmental (exogenous) ammonia, fishes may have difficulties in excreting ammonia that is endogenously produced. However, when confronted with high environmental ammonia concentrations that result in a reversed PNH3 gradient, fishes would have to detoxify not only endogenous ammonia but also exogenous ammonia that could have penetrated into the body (ammonia-loading). Under all these adverse environmental conditions, ammonia accumulation in the fish’s body would occur, but ammonia is toxic (see Ip et al., 2001a; Chew and Ip, 2014 for reviews) and fishes are generally very susceptible to elevated tissue ammonia levels. Therefore, many tropical fish species have evolved mechanisms to avoid ammonia accumulation and to ameliorate ammonia toxicity during terrestrial or ammonia exposure (see Ip et al., 2001a, 2004a,b; Chew et al., 2006; Ip and Chew, 2010, 2018; Chew and Ip, 2014, 2017 for reviews).
The stinging (or Singhi) catfish, Heteropneustes fossilis, belonging to Family Heteropneustidae (air sac catfishes), can be found in Pakistan, India, Bangladesh, Myanmar, and Thailand (Burgess, 1989; Munshi and Hughes, 1992). Its main habitats include ponds, ditches, swamps, marshes, and muddy rivers (Munshi and Choudhary, 1994). It can survive long periods of emersion and tolerate moderately high concentrations of environmental ammonia (Saha and Ratha, 1990, 1994; Saha et al., 2001). It had been reported that H. fossilis was ureogenic and contained a functional ornithine-urea cycle (OUC) that could be up-regulated during ammonia-loading (Saha and Ratha, 1987, 1989, 1994, 1998; Saha et al., 1997). However, as pointed out by Graham (1997), those reports also comprised intrinsically contradictory evidence, indicating that ureogenesis and shifting from ammonotelism (excreting > 50% of the total waste-N as ammonia) to ureotelism (excreting > 50% of the total waste-N as urea) could not be the “major” contributor to its high ammonia tolerance.
Saha and Ratha (1990) reported that H. fossilis absorbed ammonia from the external environment when exposed to 25, 50, or 75 mmol l–1 NH4Cl for 28 days. There was an increase in urea excretion by 1.5- to 2-fold between day 10 and day 12, which remained high throughout. Thus, they concluded that “prolonged hyper-ammonia stress induced the shift from ammonotelism to ureotelism in H. fossilis.” However, their results did not corroborate such a conclusion. This is because the rate of ammonia−N excretion reported in the control fish fasted for 14 days was 7.82 μmol 48 h–1 g–1 (Table 1 in Saha and Ratha, 1990) and a fish exposed to 75 mmol l–1 NH4Cl for 14 days absorbed 56.37 μmol ammonia−N 48 h–1 g–1. If one assumes that ammonia−N excretion was totally impeded in a medium containing 75 mmol l–1 NH4Cl, the total ammonia−N accrued would be 7.82 + 56.37 = ∼64 μmol 48 h–1 g–1. Yet, the increase in the rate of urea excretion after exposure to 75 mmol l–1 NH4Cl was reported to be only 2.86 (urea excretion rate in 75 mmol l–1 NH4Cl) − 0.96 (urea excretion rate in water) = 1.90 μmol (or 3.80 μmol urea−N) 48 h–1 g–1 (Saha and Ratha, 1990, Table 2). Hence, the increase in urea excretion represented ∼5.93%, which is only a very small fraction, of the total accumulated ammonia−N (∼64 μmol ammonia−N 48 h–1 g–1). Alternatively, it can be interpreted that H. fossilis could have actively excreted a certain amount of ammonia during environmental ammonia exposure; however, this possibility had not been examined. Another enigma was that the rate of ammonia absorption from the external environment increased with increasing concentrations of external NH4Cl (Table 1 in Saha and Ratha, 1990), but the rate of urea excretion was relatively constant during exposure to different concentrations of external NH4Cl (Table 2 in Saha and Ratha, 1990). If indeed increased urea synthesis and shifting from ammonotelism to ureotelism were the “major” strategies adopted by H. fossilis to survive ammonia exposure, an increase in urea excretion rate should have occurred with an increase in the concentration of environmental ammonia. These discrepancies led Graham (1997) to state that “paradoxically, some of the species reported to have the OUC enzymes are not ureotelic; urea, in fact, amounts to a quite a small percentage of the total nitrogen excreted by Heteropneustes (even in 75 mmol l–1 NH4Cl).”
At present, the roles of ureogenesis and ureotelism in defending ammonia toxicity in H. fossilis exposed to environmental ammonia remain controversial. Therefore, this study was undertaken to re-examine the effects of exposure to 30 mmol l–1 NH4Cl on the rates of ammonia and urea excretion, and the tissue ammonia and urea concentrations, in H. fossilis. As results obtained contradicted information in the literature (Saha and Ratha, 1987, 1989, 1990), we decided to re-examine the presence of carbamoyl phosphate synthetase (CPS) III activity in its liver using an established radiometric method (Anderson et al., 1970; Anderson, 1980; Anderson and Walsh, 1995; Chew et al., 2003b, 2004; Tam et al., 2003; Ip et al., 2012a). This is because all the reports that indicated the possible presence of CPS III in the liver of H. fossilis were based on a coupled-enzyme colorimetric assay (Saha and Ratha, 1987; Saha et al., 1997). Subsequently, we obtained results that denoted a lack of detectable CPS III activity in the liver of H. fossilis, demanding an alternative explanation for its high ammonia tolerance. Thus, we made an effort to examine whether H. fossilis could actively excrete ammonia during ammonia exposure (8 mmol l–1 NH4Cl). This is because active ammonia excretion has been reported previously for the highly ammonia-tolerant giant mudskipper, Periophthalmodon schlosseri (Peng et al., 1998; Randall et al., 1999; Ip et al., 2005; Chew et al., 2007), climbing perch, Anabas testudineus (Tay et al., 2006), and African sharptooth catfish, Clarias gariepinus (Ip et al., 2004d). In the case of A. testudineus (Ip et al., 2012b, c; Loong et al., 2012) and P. schlosseri (Chew et al., 2014, 2015), Na+/K+-ATPase (Nka) is known to take part in a multi-component mechanism involved in active ammonia excretion. Therefore, we also cloned and sequenced isoforms of nkaα-subunits from the gills of H. fossilis, and determined the effects of ammonia exposure on their branchial transcript levels and protein abundances, which could serve as an indicator of the ability of H. fossilis to actively excrete ammonia.
Materials and Methods
Catfish and Rearing Condition
Heteropneustes fossilis (approximately 20–60 g) were imported from India through a local fish importer (Qian Hu Fish Farm, Singapore). Its identity was confirmed by the very small dorsal fin that lacked a leading spine and was located in the anterior third of body, as well as the two tubular air sacs that extended from the gill cavity to the caudal peduncle (Burgess, 1989). The fish (without sex differentiation) were acclimated in plastic tanks containing dechlorinated fresh water at a temperature of 25–26°C and under a photoperiod of 12 h illumination and 12 h darkness using artificial light. The fish were fed frozen bloodworms and the water in the tanks was changed daily for a week. Feeding of the fish was halted 2 days before experimentation. Approval of protocol (ARFSBS/NIE-A-0311) for fish maintenance and experimentation was granted by the Nanyang Technological University Institutional Animal Care and Use Committee.
Effects of Exposure to 30 mmol l–1 NH4Cl on Urea Excretion Rate
Fish (20–30 g) were exposed individually in a tank containing 20 vol (volume to the mass of the fish) of either fresh water (five individuals in the control group) or fresh water containing 30 mmol l–1 NH4Cl (five individuals in the experimental group; pH 7.0) for 6 days. The water in the tanks was replaced daily. On day 1, 3, or 6 (after 24 h of water replacement), 2 ml of water was sampled, acidified with 0.2 ml of H2SO4, kept at 4°C, and used for the analysis of urea within a week. The colorimetric method by Jow et al. (1999) was used for the determination of urea. The excretion rate was expressed as μmol urea−N g–1 day–1.
Effects of Exposure to 8 mmol l–1 NH4Cl on Ammonia and Urea Excretion Rate
Fish (20–25 g) were immersed in a small plastic container containing six volumes (volume to the mass of the fish) of fresh water (five individuals) or fresh water with 8 mmol l–1 NH4Cl (five individuals) (pH 7.0). Temperature was kept at 25–26°C. A water sample (1 ml) was taken immediately as the 0 h sample. After 24, 48, and 72 h, 1 ml of water was again sampled. The water sampled was acidified as mentioned above. The water was kept at 4°C and used for both ammonia and urea analyses within a week. A parallel set of containers without fish was set up and water was sampled for ammonia analysis to ensure that the ammonia concentration was not altered significantly by bacterial action. The colorimetric method by Anderson and Little (1986) was used for ammonia analysis. The rate of ammonia excretion was expressed as μmol ammonia−N g–1 day–1.
Effects of Exposure to 30 mmol l–1 NH4Cl on Ammonia and Urea Concentrations in Tissues
Fish (25–40 g) were exposed individually in a tank containing 30 mmol l–1 NH4Cl (pH 7.0) for 1 day (five individuals), 3 days (five individuals), or 6 days (15 individuals) with daily changes of NH4Cl solution. Fish kept individually in fresh water (15 individuals) served as controls. At the respective time interval, fish were sacrificed by immersing in 0.1% phenoxyethanol and then applying a blow to the head. Gills, muscle, and liver were dissected, frozen in liquid nitrogen, and kept in a −80°C freezer. A separate group of fish (30–60 g) used for blood collection was exposed to either fresh water (10 individuals) or fresh water containing 30 mmol l–1 NH4Cl for 6 days (10 individuals). The fish was anesthetized and heparinized capillary tubes were used to collect the blood from the caudal peduncle. The plasma obtained after centrifugation of the blood at 4000 × g for 10 min at 4°C were deproteinized in two volumes of ice-cold 6% trichloroacetic acid. The clear supernatant obtained after centrifugation of the deproteinized samples at 10,000 × g for 15 min at 4°C was used for analyses of ammonia and urea concentrations.
The muscle and liver tissues collected were first ground to a powder in liquid nitrogen, before weighing and homogenizing in five volumes (w/v) of ice-cold 6% trichloroacetic acid using an Ultra-Turrax disperser (Ika-Werke, Staufen, Germany) set to 24,000 r/min for 20 s thrice. The clear supernatant obtained after centrifugation of the homogenate as mentioned above was used for analyses of ammonia and urea concentrations.
The method by Bergmeyer and Beutler (1985) was used for the determination of ammonia concentrations in the tissue samples while the urea concentrations were analyzed as mentioned above.
Determination of CPS Activities in the Liver
The extraction procedure was carried out on liver samples using the method of Ip et al. (2012a). Approximately 300 mg of liver was homogenized in five volumes (w/v) of ice-cold buffer containing 50 mmol l–1 Hepes (pH 7.6), 50 mmol l–1 KCl, 0.5 mmol l–1 EDTA, 0.5 mmol l–1 phenylmethanesulfonyl fluoride (PMSF), and 1 mmol l–1 dithiothreitol as mentioned above. The homogenate was sonicated three times at 110 W, 20 kHz using a Misonix sonicater (Farmingdale, NY, United States) for 20 s each with two 10 s off-intervals. The sonicated homogenate was then centrifuged as mentioned above, and the supernatant collected was desalted using the Econo-Pac 10DG desalting column (Bio-Rad Laboratories, Hercules, CA, United States) equilibrated already with extraction buffer without EDTA and PMSF. The eluent collected was used for CPS assay. The protein concentrations of all samples (before and after elution) were determined using the Bio-Rad Protein assay dye (Bradford, 1976) in order to obtain the dilution factor to be used in the subsequent calculation for CPS activities. CPS activity was determined using the radiometric method described by Anderson and Walsh (1995). Briefly, the reaction mixture consisted of 0.05 mol l–1 Hepes (pH 7.5), 0.05 mol l–1 KCl, 0.024 mol l–1 MgCl2, 0.2 mmol l–1 EDTA, 0.4 mmol l–1 dithiothreitol, 0.02 mol l–1 ATP, and 5 mmol l–1 [14C]bicarbonate. The substrates tested were 10 mmol l–1 glutamine (for CPS III assay) or 100 mmol l–1 NH4Cl (for CPS I assay) or glutamine + NH4Cl (for CPS III + CPS I assay). The inclusion of 0.5 mmol l–1 N-acetylglutamate in the assay medium was used to activate CPS I or III activity, while 1 mmol l–1 UTP was added to inhibit the activity of CPS II. A Wallac 1414 liquid scintillation counter (Wallac Oy, Turku, Finland) was used for radioactivity measurement. CPS activity was expressed as μmol [14C]urea formed min–1 g–1.
Extraction of Total RNA From Gills and cDNA Synthesis
The extraction and purification of total RNA from gill samples and the synthesis of cDNA from the purified RNA were performed following the method described in Chew et al. (2014). Briefly, Tri ReagentTM (Sigma–Aldrich Co., St. Louis, MO, United States) was used for the extraction while RNeasy Plus Mini Kit (Qiagen GmbH, Hilden, Germany) was used for the purification. The quantification of the RNA obtained was performed with a NanoDrop ND-1000 spectrophotometer (Nanodrop Technologies Inc., Wilmington, DE, United States) and the integrity of the RNA was verified using gel electrophoresis. The purified RNA was converted to cDNA with oligo (dT)18 primers using the RevertAidTM First Strand cDNA synthesis kit (Thermo Fisher Scientific Inc., Waltham, MA, United States).
Cloning and Sequencing of nkaα-subunit Isoforms
A set of conserved primers (forward 5′−3′ sequence: CACTTCATCCACATCATCAC; reverse 5′−3′ sequence: ATGGCAGGGAACCATGTC) was designed to obtain the partial nucleotide sequences of nkaα-subunit as described by Chew et al. (2014). Briefly, the polymerase chain reaction (PCR) was performed in a 9902 Veriti 96-well thermal cycler (Applied Biosystems, Carlsbad, CA, United States) using DreamTaqTM polymerase (Thermo Fisher Scientific Inc.). The amplification protocol was as follows: an initial denaturation for 3 min at 94°C, followed by 35 cycles of 94°C for 30 s, 55°C for 30 s, 72°C for 2 min, and one cycle of final elongation at 72°C for 10 min. The products obtained were separated by gel electrophoresis and the bands of the estimated amplicon size were extracted and purified using the Promega Wizard SV gel and PCR cleanup system (Promega Corporation, Madison, WI, United States). These purified products were cloned into pGEM®-T Easy vector (Promega Corporation) following the method used by Chew et al. (2014). The sequencing was carried out using the BigDye® Terminator v3.1 Cycle Sequencing Kit (Applied Biosystems) and the 3130XL Genetic Analyzer (Applied Biosystems). The BioEdit version 7.1.11 (Hall, 1999) software was used to assemble and analyze the sequences obtained. After checking and verifying that the sequences obtained were nkaα-subunit, the full nucleotide sequence of all the isoforms of nkaα-subunit was obtained through Rapid amplification of cDNA ends (RACE-PCR) using RACE-PCR primers (Supplementary Table S1) and the SMARTerTM RACE kit (Clontech Laboratories, Mountain View, CA, United States). The cDNA sequences of the isoforms of nkaα-subunit were identified and deposited into GenBank, and the accession numbers are Nkaα1b (MH427004), Nkaα1c1 (MH427006), Nkaα1c2 (MH427005), Nkaα2 (MH427007), and Nkaα3 (MH427008).
Characterization and Phylogenetic Analysis of the Deduced Nkaα Amino Acid Sequences
The amino acid sequences of the various isoforms of Nkaα were deduced using the ExPASy Proteomic server1 (Gasteiger et al., 2003). The identities of the Nkaα isoforms from H. fossilis were confirmed by aligning and comparing with Nkaα isoforms sequences from selected teleosts retrieved from the Genbank database to generate a sequence identity matrix. The potential phosphorylation sites and transmembrane domains of the Nkaα isoforms were predicted using NetPhos 2.0 and MEMSAT3 and MEMSAT-SVM provided by PSIPRED protein structure prediction server2, respectively (McGuffin et al., 2000).
Selected protein sequences of Nkaα isoforms of teleosts and Saccoglossus kowalevskii NKAα1 (as outgroup) retrieved from GenBank database were used to construct the phenogram. Phylogenetic analysis was conducted using Phylip (Felsentein, 1989) with bootstrapping values taken from 1000 replicates via the neighbor-joining method. The phenogram constructed further confirmed the identities of the Nkaα isoforms.
Determination of nkaα-transcript Levels by Quantitative Real-Time PCR (qPCR)
The cDNA used for quantification of the transcripts levels of various isoforms of nkaα-subunits was synthesized using random hexamer primers provided in the cDNA synthesis kit mentioned above. Absolute quantification of the transcripts levels of nkaα-subunit isoforms was performed as described in Chew et al. (2015). Briefly, the qPCR reaction was accomplished in a volume of 25 μl consisting of 2 × KAPA SYBR® FAST Master Mix ABI Prism® (Kapa Biosystems, Wilmington, MA, United States), 0.3 μmol l–1 forward and reverse qPCR primers (Supplementary Table S1), and 1 ng of cDNA or appropriate standards. Each PCR was carried out in triplicates in a StepOnePlusTM Real-Time PCR System (Applied Biosystems). The amplification protocol consisted of an initial denaturation at 95°C for 3 min followed by 35 cycles at 95°C for 30 s, 60°C for 30 s, 72°C for 30 s, and a final cycle of elongation at 72°C for 10 min. The threshold cycle (Ct) value for each well was collected and a melt curve analysis was conducted to confirm that a single product was obtained. In addition, agarose gel electrophoresis on the product was performed to verify that only one band was present. The amplification efficiencies of the primers for nkaα1b, nkaα1c1, nkaα1c2, nkaα2, and nkaα3 obtained were 84.5, 84.5, 86.2, 89.3, and 92.2%, respectively. The quantity of nkaα-subunit isoforms transcripts in a sample was determined using the standard curve prepared for each nkaα-subunit isoform and expressed as copies of transcripts per ng total RNA.
Antibodies
Two isoform-specific anti-Nkaα antibody against Nkaα1c1 or Nkaα1c2 of H. fossilis were custom-made by GenScript (Piscataway, NJ, United States). They were: (1) a rabbit anti-Nkaα1c1 polyclonal antibody against amino acid residues 19–32 (GNKKSKSKGKKDKD) of Nkaα1c1 and (2) a mouse anti-Nkaα1c2 polyclonal antibody against amino acid residues 469–478 (GGMREKYPKV) of Nkaα1c2. The anti-NKAα antibody (α5), which is known to react comprehensively with multi-Nkaα isoforms (developed by Douglas M. Farmbrough, Johns Hopkins University, Baltimore, MD, United States) was purchased from the Developmental Studies Hybridoma Bank (Department of Biological Sciences, The University of Iowa, Iowa City, IA, United States) while the anti-β-actin antibody (MA5-11869) was purchased from Thermo Fisher Scientific Inc.
Measurement of NKAα Protein Abundance by Western Blotting
The gill samples collected were homogenized thrice in five volumes (w/v) of extraction buffer as described in Chew et al. (2015) in a Precellys homogenizer (Bertin Instruments, Montigny-le-Bretonneux, France) set at 6000 r/min for 20 s. After homogenization, the homogenate was centrifuged and the protein concentration was quantified in the supernatant according to Chew et al. (2015). Protein samples were diluted with Laemmli’s buffer (Laemmli, 1970) so that 20 μg of protein samples was loaded onto the gel (comprising 8% acrylamide for the resolving gel and 4% acrylamide for the stacking gel) in the Mini-PROTEAN® Tetra Vertical Electrophoresis Cell apparatus (Bio-Rad Laboratories). Subsequently, the separated proteins were transferred onto nitrocellulose membranes using a mini Transblot Cell (Bio-Rad Laboratories). The separated proteins were detected using the Pierce Fast Western Blot kit, SuperSignal® West Pico Substrate (Thermo Fisher Scientific Inc.). The membranes were incubated with anti-Nkaα1c1 antibody (1:5000 dilution), or anti-Nkaα1c2 antibody (1:3000 dilution) or anti-α5 (1:8000 dilution). To detect the reference protein, the membranes were incubated with pan-anti-actin antibody (1:15,000 dilution) for 1 h at 25°C. Subsequently, the membranes were incubated with either anti-rabbit or anti-mouse horseradish peroxidase-conjugated secondary antibody for 15 min at 25°C. The imaging of the blots was performed using the ChemiDoc Imager (Bio-Rad Laboratories). The intensity of the bands were quantified using the ImageJ software (version 1.40, NIH), calibrated with a 37-step reflection scanner scale (1” x 8”; Stouffer #R3705-1C). The protein abundance was expressed as relative protein abundance of Nka per μg protein normalized with pan-actin. A peptide competition assay was performed on the anti-Nkaα1c1 and anti-Nkaα1c2 antibodies to confirm the identity of the band of interest for each antibody. The anti-Nkaα1c1 (1 μg) and anti-Nkaα1c2 (3.33 μg) antibodies were pre-incubated with their corresponding immunizing peptide (4 and 33.33 μg, respectively) from Genscript at 25°C for 1 h before performing the peptide competition assay.
Statistical Analysis
The SPSS software version 25 (IBM Corp., Armonk, NY, United States) was used for all statistical analysis. Measured or generated data were presented as means ± standard errors of means (SEM). All data were evaluated for equality of variance using the Levene’s test. For testing the difference between the freshwater (control) group and the ammonia-exposed group, student’s t-test was applied while for testing among the freshwater (control) group and/or among the ammonia-exposed group at different time interval, one-way analysis of variance was applied. When a significant difference was detected for the means among the groups, multiple comparisons of the means using Dunnett’s T3 were performed when the variance was unequal while Tukey’s test was applied when the variance was equal. The means were considered as statistically different with P < 0.05.
Results
Effects of 30 mmol l–1 NH4Cl on Urea Excretion Rate
The daily urea excretion rate of H. fossilis kept in fresh water was relatively low, ranging from 1.24 to 1.76 μmol urea−N g–1, and it remained statistically unchanged during 6 days of exposure to 30 mmol l–1 NH4Cl (Figure 1). It was technically not possible to determine the rate of ammonia excretion due to the presence of such a high concentration of ammonia (30 mmol l–1 NH4Cl) initially in the water.
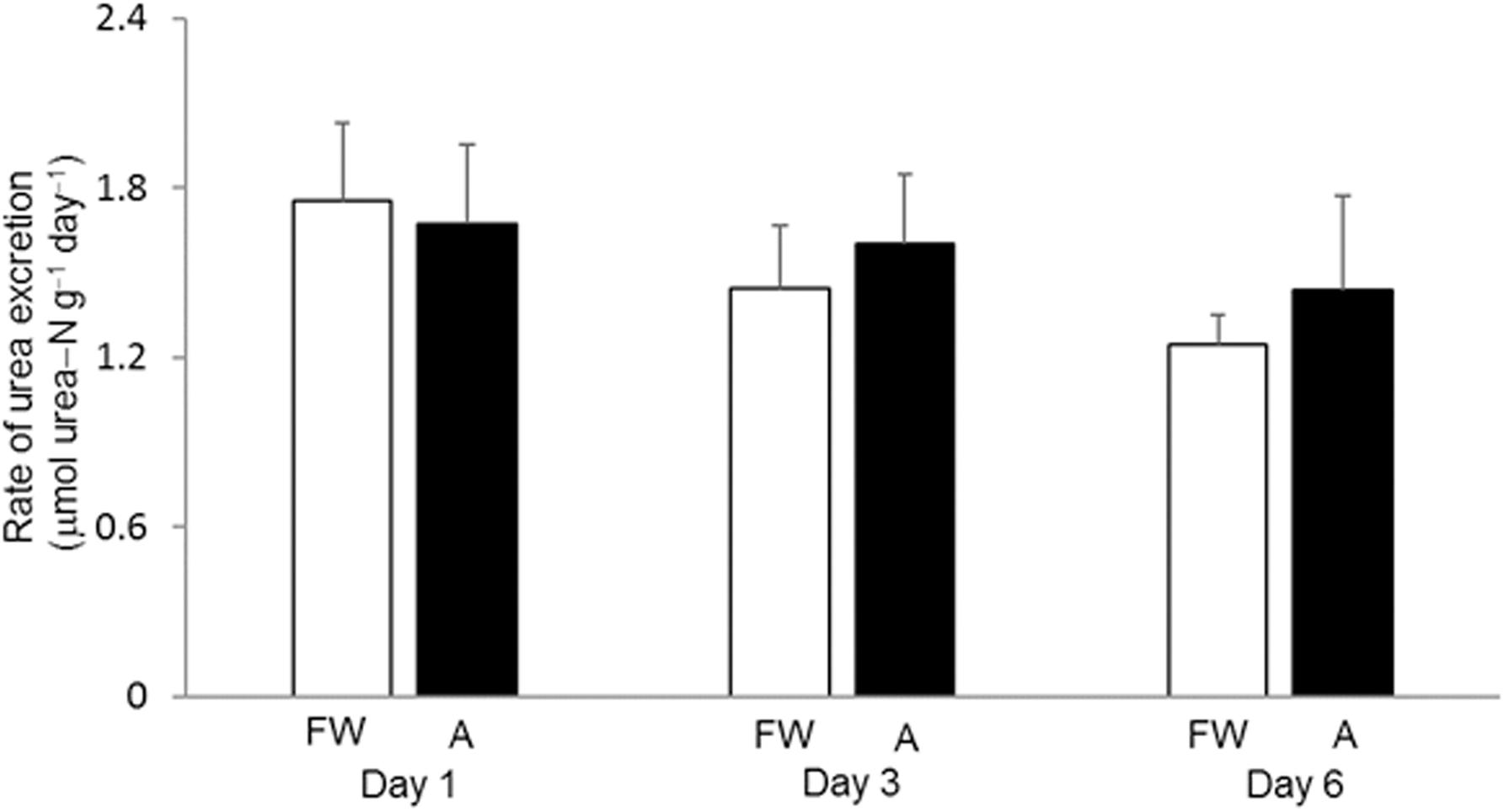
Figure 1. Effects of exposure to 30 mmol l–1 NH4Cl on the rate of urea excretion in Heteropneustes fossilis. Rate of urea excretion (μmol urea−N g–1 day–1) in H. fossilis kept in fresh water (FW; open bar) or exposed to 30 mmol l–1 NH4Cl (A; closed bar) for 1, 3, or 6 days. Values are mean + SEM (N = 5).
Effects of 30 mmol l–1 NH4Cl on Tissue Ammonia and Urea Concentrations
Generally, the muscle and liver of H. fossilis contained much more ammonia than urea (Figures 2A,B). Exposure to 30 mmol l–1 NH4Cl for 6 days led to significant increases in ammonia contents in the muscle (∼twofold) and plasma (∼fivefold) but not the liver (Figure 2A). Surprisingly, the urea content in the muscle, liver, and plasma decreased significantly (by ∼65%) after exposure to 30 mmol l–1 NH4Cl for 6 days (Figure 2B).
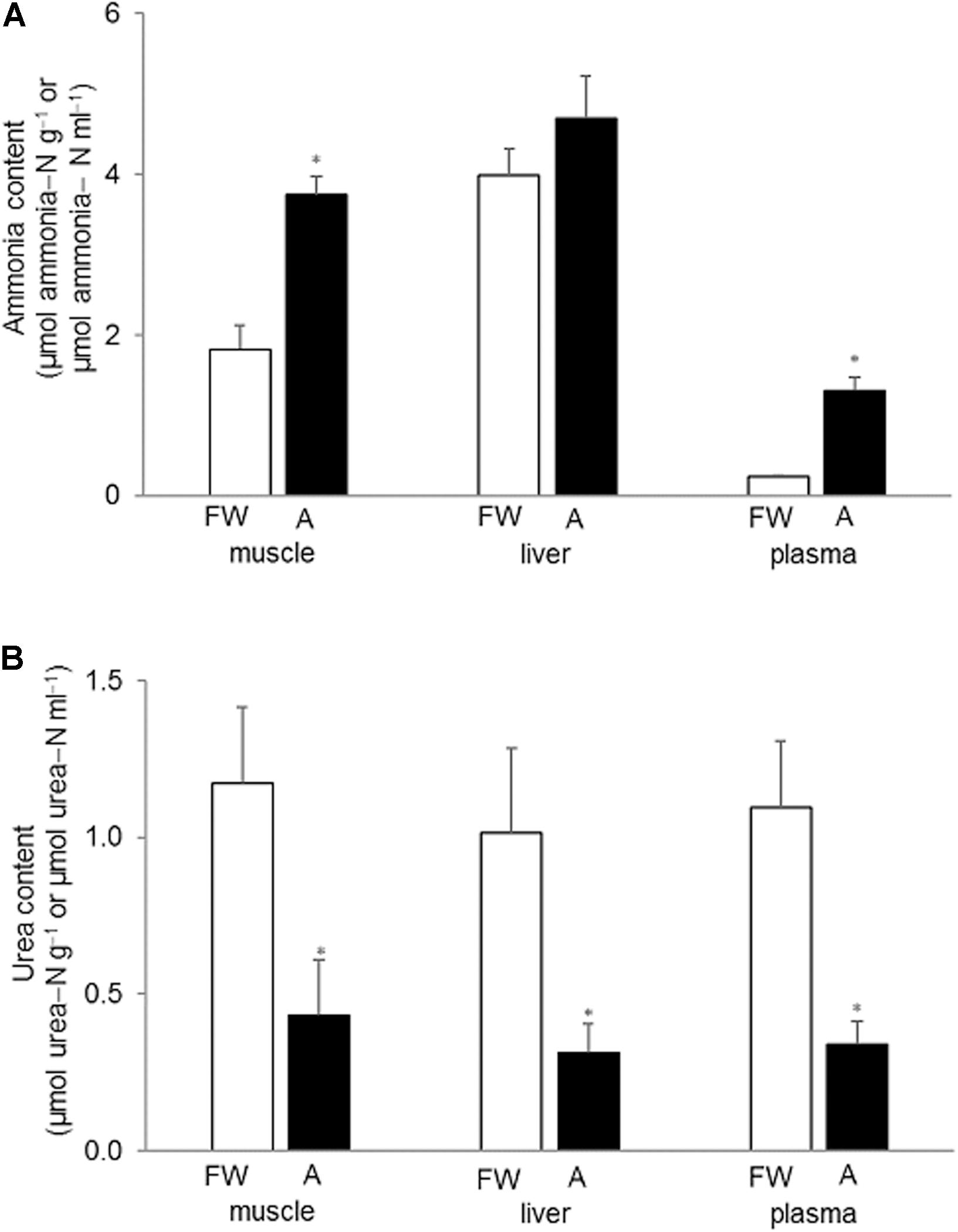
Figure 2. Effects of exposure to 30 mmol l–1 NH4Cl on ammonia and urea concentrations in the tissues of Heteropneustes fossilis. Concentrations (μmol ammonia−N or urea−N g–1 tissue or μmol ammonia−N or urea−N ml–1 plasma) of (A) ammonia and (B) urea in the muscle liver and plasma of H. fossilis kept in fresh water (FW; open bar) or exposed to 30 mmol l–1 NH4Cl (A; closed bar) for 6 days. Values are mean + SEM (N = 5). *Significantly different from the corresponding tissue or plasma of the FW fish, P < 0.05.
Effects of 8 mmol l–1 NH4Cl on Rates of Ammonia and Urea Excretion
When H. fossilis was exposed to water containing 8 mmol l–1 NH4Cl, there was a progressive and significant increase in the ammonia concentration, from 8 to 15 mmol ammonia−N l–1, in the ambient water during a 3-day period (Figure 3A). This indicated that H. fossilis was able to continuously excrete endogenously and metabolically produced ammonia during exposure to 8 mmol l–1 NH4Cl. For the control fish kept in fresh water for 3 days, the daily rate of ammonia−N excretion ranged between 12.7 and 14.7 μmol g–1 day–1 (Figure 3B). In comparison, fish exposed to 8 mmol l–1 NH4Cl had a positive ammonia−N excretion rate ranging from 6.2 to 9.8 μmol g–1 day–1. Although the rate of ammonia excretion was significantly lower than the freshwater control on day 1 of ammonia exposure, it recovered to the control level on day 2 and day 3, indicating the possible up-regulation of certain mechanisms of active ammonia excretion (Figure 3B). By contrast, the daily rate of urea−N excretion in control fish kept in fresh water was very low (ranging from 1.2 to 2.0 μmol g–1 day–1, and it remained unchanged during 3 days of exposure to 8 mmol l–1 NH4Cl (ranged from 0.68 to 1.46 μmol g–1 day–1) (Figure 3C).
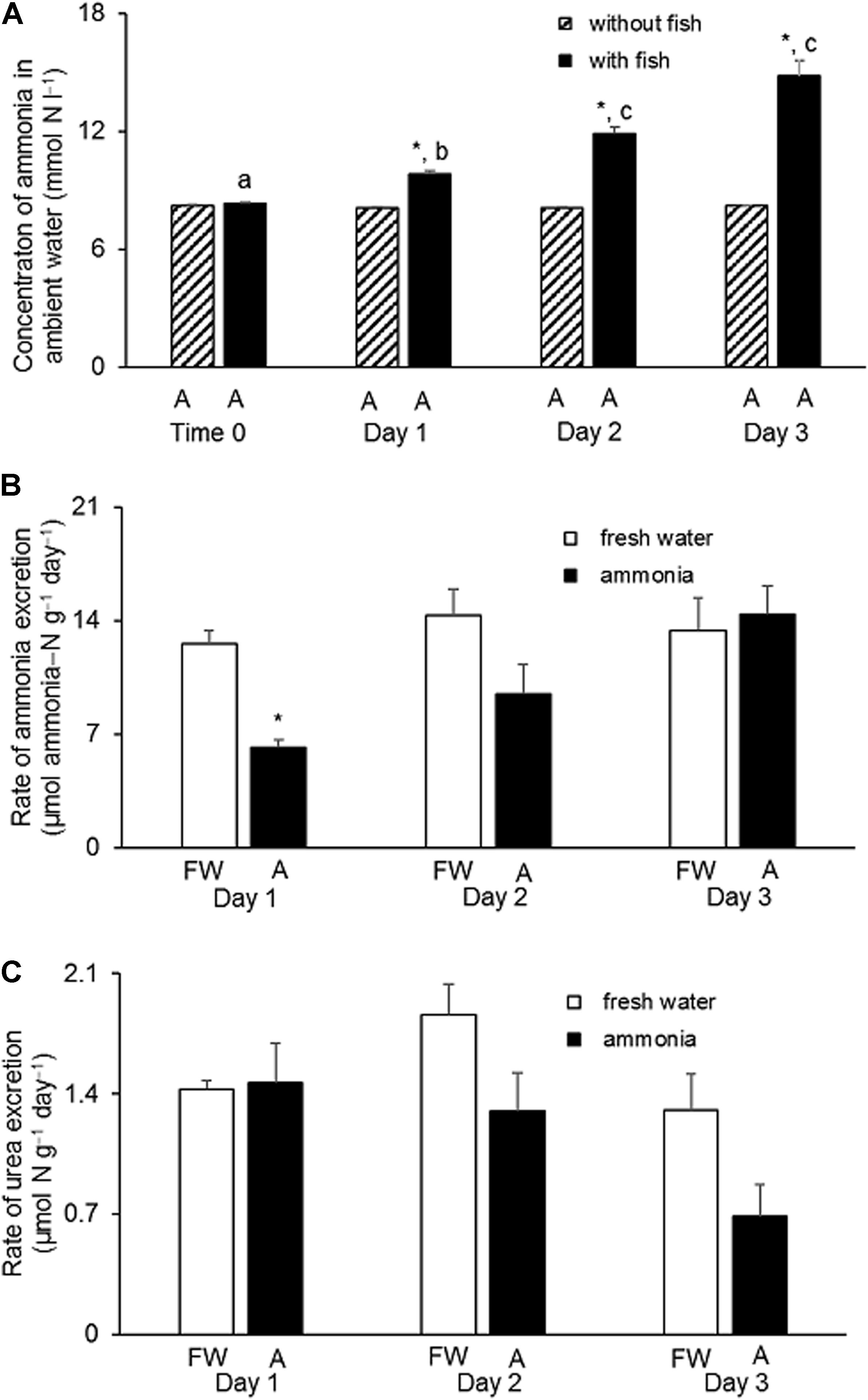
Figure 3. Effects of exposure to 8 mmol l–1 NH4Cl on rates of ammonia and urea excretion in Heteropneustes fossilis. (A) Concentrations of ammonia in the external medium containing 8 mmol l–1 NH4Cl at time 0 or on day 1, 2, or 3 without (shaded bar) or with (closed bar) the incubation of H. fossilis. Rates (μmol ammonia−N or urea−N g–1 day–1) of (B) ammonia and (C) urea excretion in H. fossilis exposed to the same external medium of fresh water (FW; open bar) or fresh water containing 8 mmol l–1 NH4Cl (A; closed bar) on day 1, 2, or 3. Values are mean + SEM (N = 5). ∗Significantly different from that of fish kept in fresh water, P < 0.05. Means not sharing the same letter are significantly different (P < 0.05).
CPS III and CPS I Activities Are Undetectable in the Liver
Using the radiometric method employed in this study, no CPS I activity was detected in the liver of H. fossilis when NH4+ was used as the substrate (Table 1). Although a very low activity was detected when glutamine was used as the substrate, it could not be CPS III activity as it was refractory to N-acetyl-glutamate activation (Table 1). The results of CPS activities from the mouse, Mus musculus, and the stingray, Taeniura lymma, presented in Table 1 were extracted from Ip et al. (2012a), which were obtained using the same method in the same laboratory.
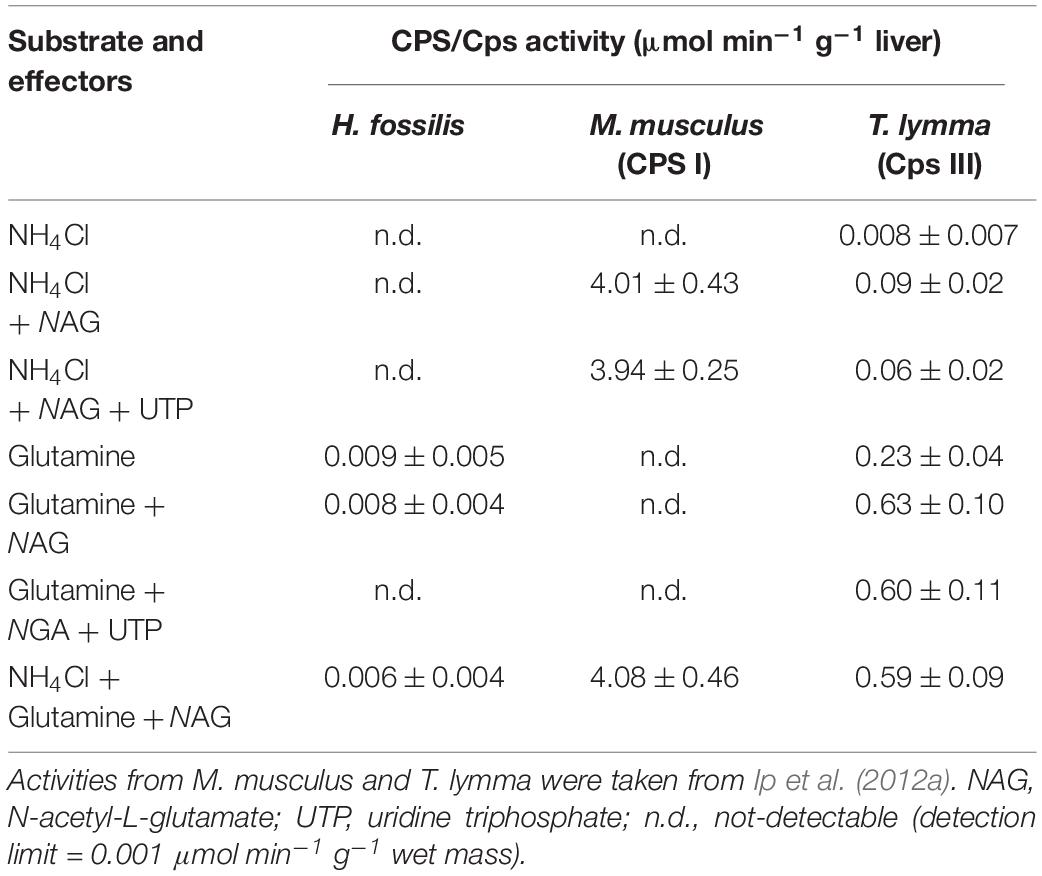
TABLE 1 Activities (μmol [14C]urea min–1 g–1 liver; N = 4) of carbamoyl phosphate synthetase (CPS/Cps), determined in the presence of various substrates and effectors, from the liver of Heteropneustes fossilis, Mus musculus (mouse; with CPS I), and Taeniura lymma (stingray; with CPS III).
Nucleotide Sequences, Translated Amino Acid Sequences, and Phylogenetic Analysis of nkaα/Nkaα in the Gills
Five complete coding cDNA sequences of nkaα-subunit were obtained from the gills of H. fossilis. They were deposited into GenBank with accession numbers presented in Table 2. The cDNA sequences ranged from 3063 to 3078 bp, and the translated amino acids sequences ranged from 1021 to 1026 residues. The molecular mass of these nkaα-subunit isoforms ranged from 112.6 to 113.2 kDa (Table 2).
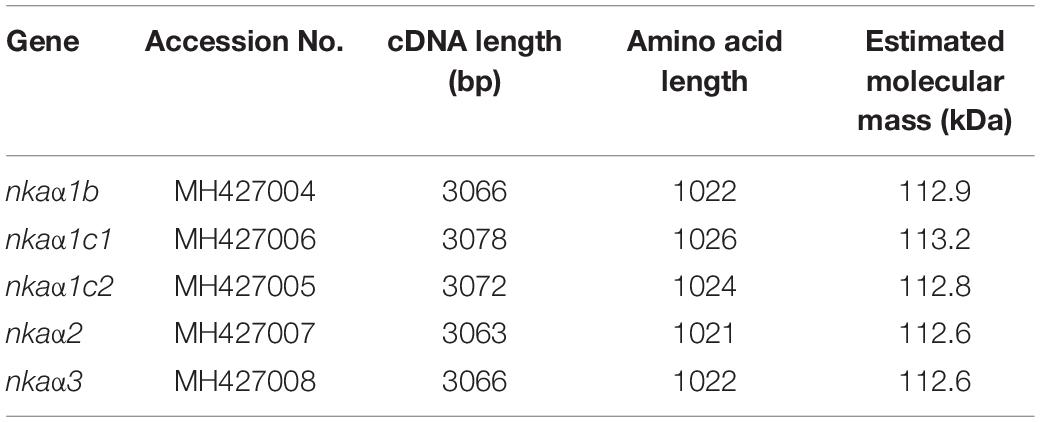
Table 2. cDNA length (bp), amino acid length, and estimated molecular mass (kDa) of Na+/K+-ATPase (nka) α-subunit isoforms.
A phenogramic analysis revealed that three isoforms of Nkaα1 were present in H. fossilis as they were grouped together with Nkaα1 isoforms of other teleosts (Figure 4). As one isoform of Nkaα1 was highly similar to the Nkaα1c1 and another was highly similar to the Nkaα1c2 of the electric eel, Electrophorus electricus, they were identified as Nkaα1c1 and Nkaα1c2, respectively (Figure 4). In addition, one isoform of Nkaα1 was clustered closely with Nkaα1b of A. testudineus, thus it was identified as Nkaα1b. The other two Nkaα isoforms were identified as Nkaα2 and Nkaα3 as they were grouped under the same clade as Nkaα2 of E. electricus, Oncorhynchus mykiss, and Fundulus heteroclitus and Nkaα3 of E. electricus, Carassius auratus, O. mykiss, Oreochromis mossambicus, and P. schlosseri, respectively (Figure 4).
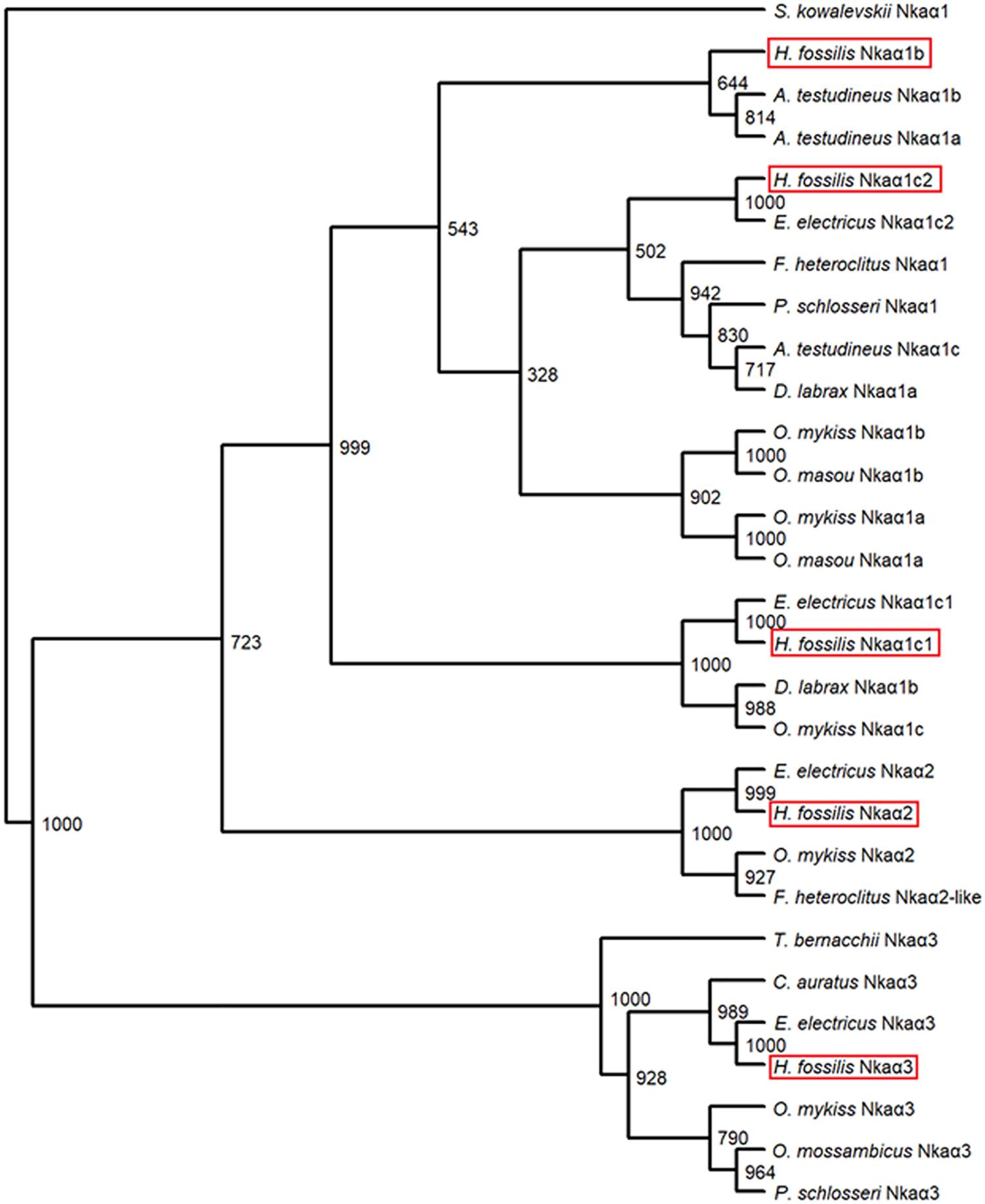
FIGURE 4 A phenogramic analysis of Na+/K+-ATPase α (Nkaα) isoforms from the gills of Heteropneustes fossilis. A neighbor-joining tree was constructed to illustrate the relationship between Nkaα isoforms from the gills of H. fossilis and Nkaα/NKAα of selected teleost species. The number at each node represents the bootstrap value obtained from 1000 replicates. NKAα1 of Saccoglossus kowalevskii was selected as an outgroup. The accession numbers of the amino acid sequences of Nkaα-subunit isoforms obtained from Genbank database were as follows: Anabas testudineus Nkaα1a (JN180940.1), A. testudineus Nkaα1b (JN180941.1), A. testudineus Nkaα1c (JN180942.1), Carassius auratus Nkaα3 (BAB60722.1), Dicentrarchus labrax Nkaα1a (AKQ12834.1), D. labrax Nkaα1b (AKQ12835.1), Electrophorus electricus Nkaα3 (AJR20273), E. electricus Nkaα2 (AJR20272), E. electricus Nkaα1c1 (AJR20270), E. electricus Nkaα1c2 (AJR20271), Fundulus heteroclitus Nkaα1 (AAL18002.1), F. heteroclitus Nkaα2 (AAL18003.1), Oncorhynchus mykiss Nkaα1a (NP_001117933.1), O. mykiss Nkaα1b (NP_001117932.1), O. mykiss Nkaα1c, (NP_001117931.1), O. mykiss Nkaα2 (NP_001117930.1), O. mykiss Nkaα3 (NP_001118102.1), O. masou Nkaα1a (BAJ13363.1), O. masou Nkaα1b (BAJ13362.1), Oreochromis mossambicus Nkaα3 (AAD11455.2), Periophthalmodon schlosseri Nkaα1 (AGR87393.1), P. schlosseri Nkaα3 (AGR87394.1), and Trematomus bernacchii Nkaα3 (AAY30258.1).
The deduced amino acid sequences of all five Nkaα isoforms from the gills of H. fossilis had 10 predicted transmembrane domains (TM1-TM10). An alignment of these five amino acid sequences revealed the presence of some highly conserved regions of Nkaα. These include: (1) the threonine-glycine-glutamic acid-serine (TGES) motif that is critical for the catalytic cycle; (2) the proline-glutamic acid-glycine-leucine (PEGL) signature motif in TM4 that is needed for ion binding and transport together with TM5 and TM6; (3) the aspartic acid-lysine-threonine-glycine-threonine (DKTGT) motif that is important for phosphorylation; and (4) the glycine-aspartic acid-glycine-valine-asparagine-aspartic acid-serine-proline (GDGVNDSP) motif that is associated with the phosphorylation site and stabilization of phosphoenzyme intermediates. A highly conserved lysine-rich region that serves as the ion-selective gate important for cation binding and occlusion (Shull et al., 1986) was also present in all five Nkaα isoforms of H. fossilis, with Nkaα1c2 containing the greatest number of lysine residues followed by Nkaα1c1, Nkaα1b, Nkaα2, and Nkaα3 (Figure 5).
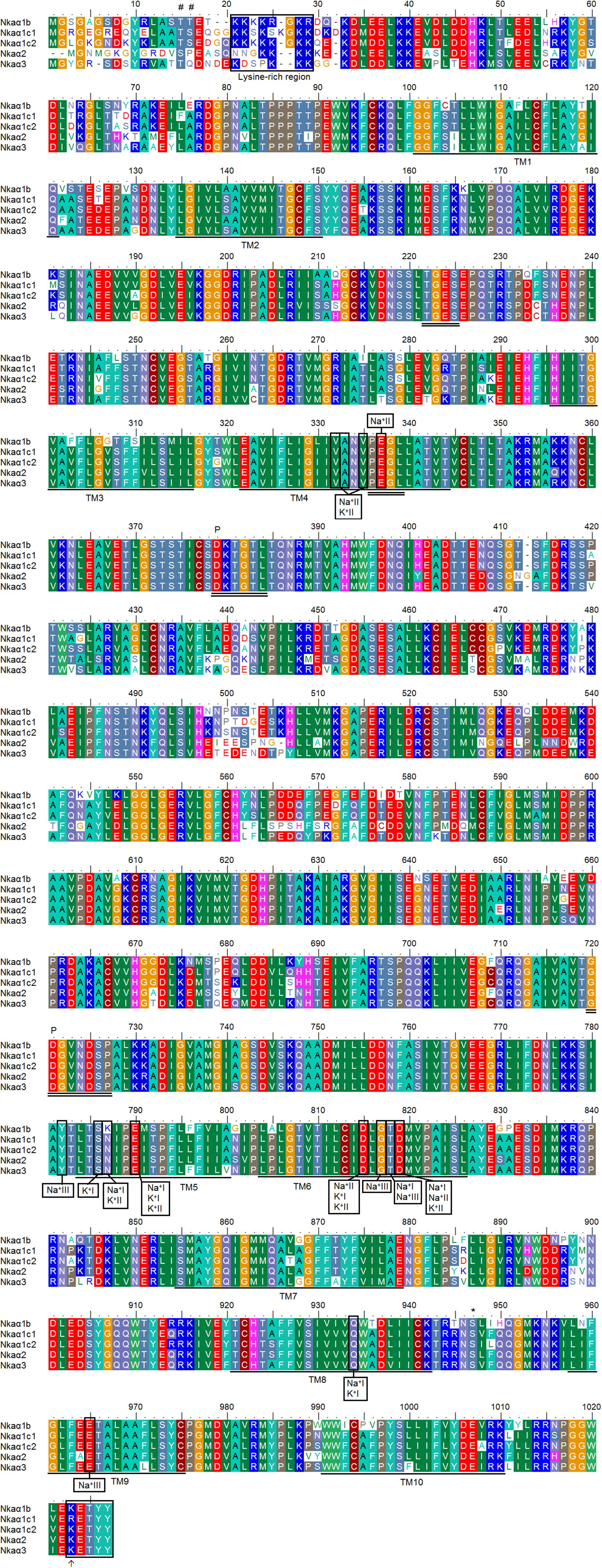
FIGURE 5 A multiple alignment of the deduced amino acid sequences of the five Na+/K+-ATPase α (Nkaα) isoforms obtained from the gills of Heteropneustes fossilis. Identical or similar amino acid residues are indicated by shaded residues. The 10 predicted transmembrane regions (TM1–TM10) of Nkaα1b, Nkaα1c1, Nkaα1c2, Nkaα2, and Nkaα3 are underlined. Vertical boxes represent coordinating residues for Na+ or K+ binding. The conserved regions containing the TGES, PEGL, DKTGT, and GDGVNDSP sequence motifs are double underlined and the phosphorylation sites are indicated by a “P.” The asterisk and hash marks denote the amino acid residues phosphorylated by protein kinase A and protein kinase C, respectively. The lysine-rich region is indicated with a box. The KETYY motif is indicated with a box and an arrow indicates the amino acid residue that replaces arginine. The transmembrane domains were predicted using MEMSAT3 and MEMSAT-SVM provided by PSIPRED protein structure prediction server.
Three Na+ (denoted as Na+I, Na+II, and Na+III) and two K+ (denoted as K+I and K+II) binding sites are known to be present in TM4, TM5, and TM6 of NKAα (Ogawa and Toyoshima, 2002). These binding sites were fully conserved in all five Nkaα isoforms of H. fossilis, except that asparagine (N) was replaced by lysine (K) at residue 787 in TM5 of Nkaα1b (Figure 5). When the Nkaα1 isoforms (Nkaα1b, Nkaα1c1, and Nkaα1c2) from the gills of H. fossilis were aligned and compared with those (Nkaα1a, Nkaα1b, and Nkaα1c) of A. testudineus at amino residues 761–800, the amino acid residue (asparagine) constituting the K+ binding sites of Nkaα1c1 and Nkaα1c2 of H. fossilis was identical to that of Nkaα1c of A. testudineus (Figure 6).
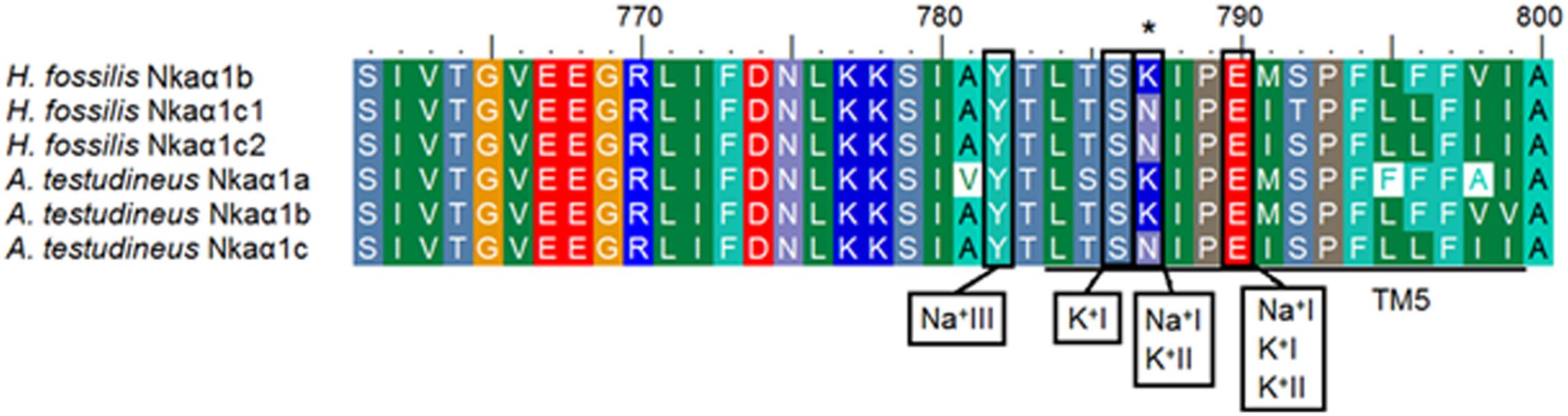
Figure 6. Analysis of Na+/K+ binding site of the three isoforms of Na+/K+-ATPase α1 (Nkaα1) from the gills of Heteropneustes fossilis. A multiple amino acid sequence alignment of a region of Nkaα1b, Nkaα1c1, and Nkaα1c2 from the gills of H. fossilis, with Nkaα1a (GenBank: AFK29492.1), Nkaα1b (GenBank: AFK29493.1), and Nkaα1c (GenBank: AFK29494.1) from the gills of Anabas testudineus. Identical or similar amino acid residues are indicated by shaded residues. A vertical box represents the coordinating residue for Na+ or K+ binding. An asterisk indicates the amino acid residue that is similar to Nkaα1c but different from Nkaα1a and Nkaα1b.
Effects of Exposure to 30 mmol l–1 NH4Cl on the Transcript Levels of nkaα-subunit Isoforms in the Gills
In the gills of H. fossilis kept in fresh water (control), the transcript level of nkaα1b was the highest (∼60,000 copies per ng of total RNA; Figure 7A), followed by nkaα1c1 (∼12,000 copies per ng of total RNA; Figure 7B), nkaα1c2 (∼11,000 copies per ng of total RNA; Figure 7C), nkaα3 (∼2600 copies per ng of total RNA; Figure 7E), and nkaα2 (∼800 copies per ng of total RNA; Figure 7D). After 1 day of exposure to 30 mmol l–1 NH4Cl, the transcript level of nkaα1b decreased by 38.5%, but this change was transient and the transcript returned to a level comparable to that of the control after 3 days of ammonia exposure (Figure 7A). Interestingly, exposure of H. fossilis to 30 mmol l–1 NH4Cl for 6 days resulted in ∼2.1-fold increase in the transcript level of nkaα1b in its gills, as compared with that of the freshwater control (Figure 7A). There was also a significant increase (∼1.4-fold) in the transcript level of nkaα1c1 after 1 or 3 days of exposure to ammonia (Figure 7B). On the other hand, exposure to 30 mmol l–1 NH4Cl had no significant effects on the transcript level of nkaα1c2 in the gills of H. fossilis (Figure 7C). Exposure to ammonia also did not lead to any significant changes in the transcript levels of nkaα2 (Figure 7D) and nkaα3 (Figure 7E) in the gills of H. fossilis.
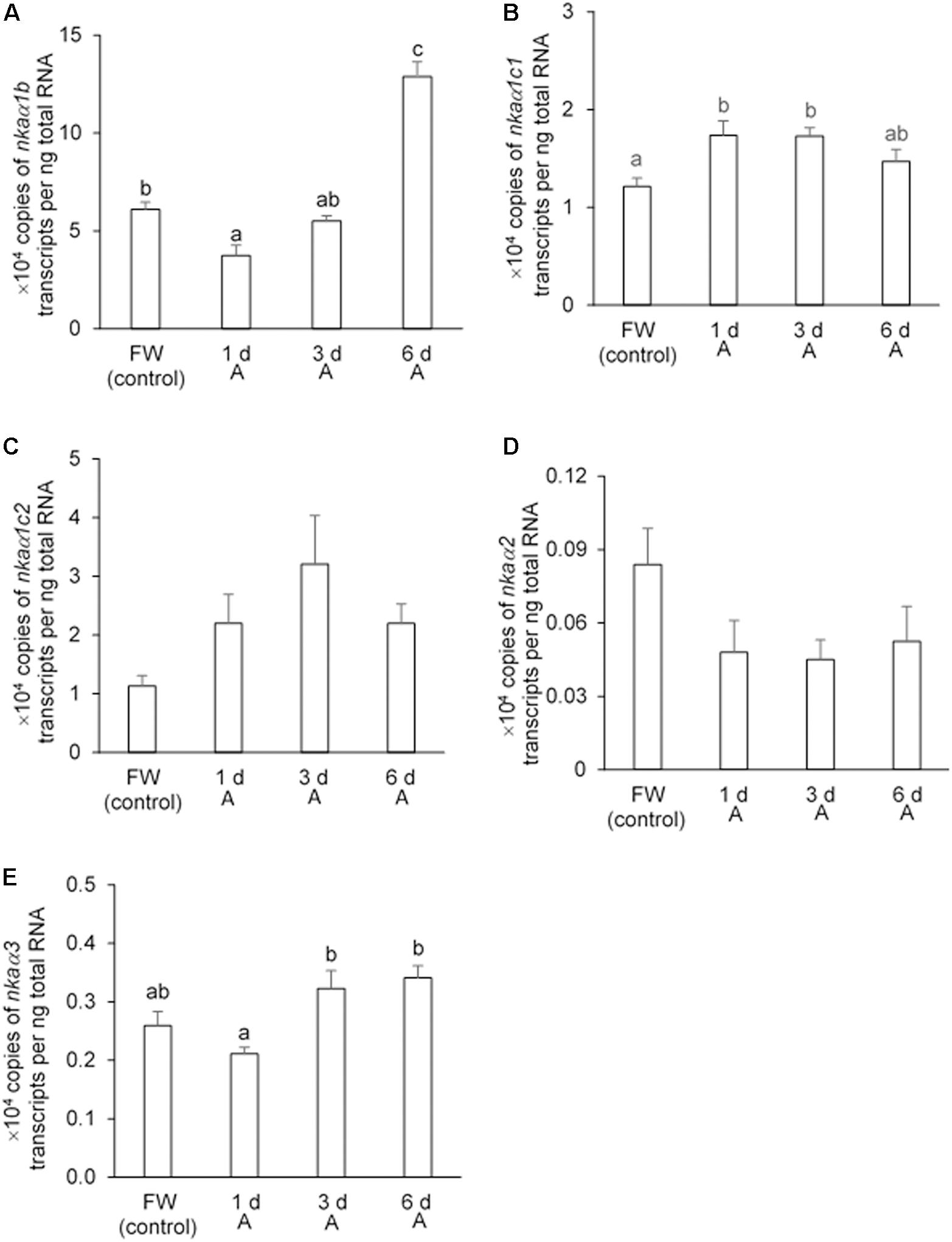
Figure 7. Effects of exposure to 30 mmol l–1 NH4Cl on the transcript levels of Na+/K+-ATPase α-subunit (nkaα) isoforms in the gills of Heteropneustes fossilis. Absolute quantification (copies of transcript per ng of total RNA) of (A) nkaα1b, (B) nkaα1c1, (C) nkaα1c2, (D) nkaα2, and (E) nkaα3 in the gills of H. fossilis kept in fresh water (FW; control) or exposed to 30 mmol l–1 NH4Cl (A) for 1, 3, or 6 days (d). Values are mean + SEM (N = 5). Means not sharing the same letter are significantly different (P < 0.05).
Effects of Exposure to 30 mmol l–1 NH4Cl on the Protein Abundances of Nkaα Isoforms in the Gills
The protein abundances of Nkaα1c1 and Nkaα1c2 increased significantly (∼7.4-fold and ∼3.5-fold, respectively) in the gills of fish exposed to 30 mmol l–1 NH4Cl for 6 days (Figure 8). Overall, there was a significant increase in the protein abundance (∼6.4-fold) of total NKAα (as reflected by immunostaining with the α5 anti-NKAα antibody) in the gills of these experimental fishes as compared with the controls (Figure 8). The peptide competition assay conducted for the anti-Nkaα1c1 and anti-Nkaα1c2 antibodies confirmed the identity of the band of interest obtained for the western blotting results of both Nkaα1c1 and Nkaα1c2 (Supplementary Figure S1).
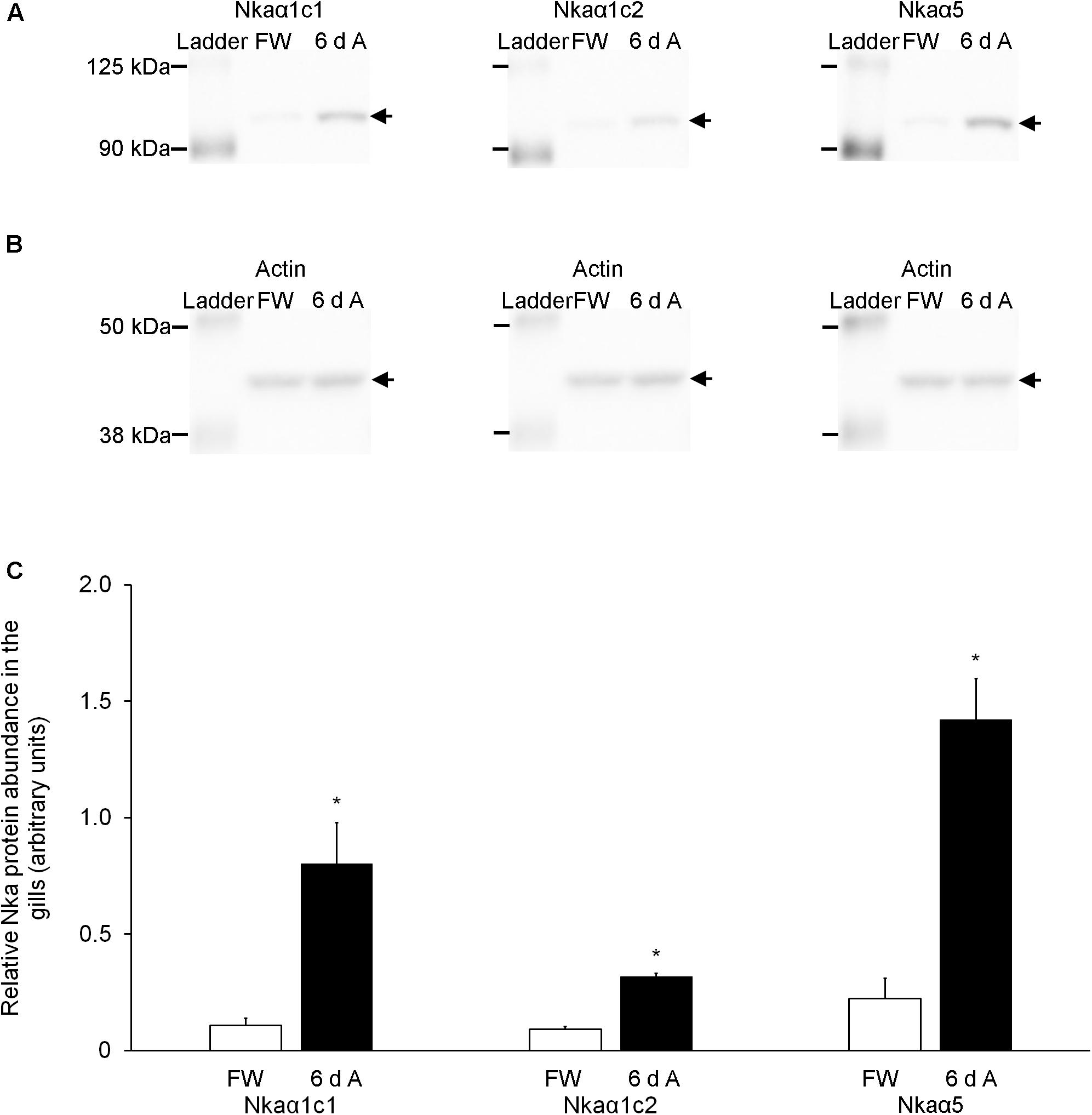
Figure 8. Effects of exposure to 30 mmol l–1 NH4Cl on the protein abundance of Na+/K+-ATPase α-subunit (Nkaα) isoforms in the gills of Heteropneustes fossilis. Protein abundances of Nkaα1c1, Nkaα1c2, and total Nkaα (as reflected by immunostaining with the α5 comprehensive anti-Nkaα antibody) in the gills of H. fossilis kept in fresh water (control; FW) or exposed to 30 mmol l–1 NH4Cl for 6 days (6 d A). (A) An example of the immunoblot of Nkaα1c1, Nkaα1c2, and total Nkaα. (B) An example of the immunoblot of actin. (C) The protein abundance of the various Nka bands normalized with respect to that of actin. Results represent mean + SEM (N = 4). *Significance difference between the NH4Cl-exposed group (6 d A; closed bar) and the corresponding freshwater group (FW; open bar) (P < 0.05).
Discussion
A ureotelic animal may or may not be able to synthesize urea through the OUC (ureogenesis), because urea can also be produced through the degradation of arginine (argininolysis) or uric acid (uricolysis) (Campbell, 1991; Anderson, 2001). Even if a fish can conduct ureogenesis, it is imperative to examine whether increased urea synthesis is the “major strategy” employed by the fish to detoxify the accumulating ammonia under adverse conditions, and whether the fish also employs other strategies to ameliorate ammonia toxicity. To date, there are very few ureotelic teleosts, and most adult tropical teleosts do not rely on ureogenesis as a major strategy to detoxify ammonia produced internally or infiltrated from the external environment (see Ip et al., 2001a, 2004a, 2004b; Chew et al., 2006; Ip and Chew, 2010, 2018; Chew and Ip, 2014, 2017 for reviews). Even though Lim et al. (2001) detected a complete OUC in the liver of P. schlosseri, the CPS activity present was extremely low. Hence, P. schlosseri does not depend on increased urea synthesis to detoxify ammonia when it is in a terrestrial environment (Ip et al., 1993, 2001b), high ammonia environment (Peng et al., 1998; Randall et al., 1999), or alkaline medium (Chew et al., 2003a). Instead, it resorts to excreting NH4+ actively (Randall et al., 1999; Chew et al., 2007, 2014, 2015) and increasing H+ excretion (Ip et al., 2004c) through its gills to ameliorate ammonia toxicity during ammonia or terrestrial exposure. Here, we report that likewise, H. fossilis is non-ureotelic and non-ureogenic, but capable of active ammonia excretion.
No Increases in Urea Excretion or Accumulation in H. fossilis Exposed to Ammonia
Our results confirmed that H. fossilis was non-ureotelic in fresh water or during 6 days of exposure to 30 mmol l–1 NH4Cl. It did not accumulate urea in its liver, muscle, or plasma during ammonia exposure. In fact, the muscle urea concentration decreased to 0.431 μmol urea−N g–1 (compared to the control level of 1.17 μmol urea−N g–1) after 6 days of exposure to 30 mmol l–1 NH4Cl. Hence, it can be concluded that ammonia exposure does not induce ureogensis in H. fossilis. By contrast, after exposure to 30 mmol l–1 NH4Cl for 6 days, the muscle ammonia concentration increased from 1.81 to 3.74 μmol ammonia−N g–1, indicating that high tissue ammonia tolerance could be an important strategy to deal with ammonia toxicity in H. fossilis. Notably, high tissue ammonia tolerance can accommodate high concentrations of ammonia in the plasma, which could lower the environment-to-body NH3 and/or NH4+ gradients and reduce the rate of exogenous ammonia influx. By contrast, detoxifying the exogenous ammonia that has entered the body into urea through the OUC would maintain an inwardly driven NH3 gradient and a continuous influx of NH3. That could be one of the reasons why ureogenesis is not commonly utilized by adult teleost fishes to defend against environmental ammonia (see reviews by Ip et al., 2001a, 2004a, 2004b; Chew et al., 2006; Ip and Chew, 2010, 2018; Chew and Ip, 2014, 2017).
No Detectable CPS III or I Activities in the Liver of H. fossilis
Based on the radiometric assay employed in this study, H. fossilis lacked detectable CPS I and III activities in its liver. Hence, it does not possess a functional hepatic OUC, and is non-ureogenic. The discrepancy between the results obtained in this study and those of Saha and Ratha (1989, 1994) and Saha et al. (1997) could be due to differences in the CPS assay methods employed. The CPS assay adopted by Saha and Ratha (1989, 1994) and Saha et al. (1997) was a colorimetric method involving a coupled enzyme system whereby commercially available ornithine transcarbamoylase was added to the assay medium. The accuracy and sensitivity of such a coupled enzyme system can be affected by the purity and source of the enzymes used. Such a discrepancy of the activities of CPS had also been reported by Ip et al. (2004a) and Tay et al. (2006), who were unable to detect activities of CPS I or III in the livers of the Asian walking catfish, Clarias batrachus, and A. testudineus using the radiometric method, although the presence of CPS activities in these fishes has been reported by Saha and Ratha (1989) and Saha et al. (1999) based on the colorimetric method. Taken together, our results explain why freshwater H. fossilis is ammonotelic, excreting more than 80% of its nitrogenous wastes (ammonia−N + urea−N) as ammonia−N. A priori, the small quantity of urea excreted by H. fossilis could originate from argininolysis and/or uricolysis.
Active Excretion of Ammonia in H. fossilis Against 8 mmol l–1 NH4Cl
Active excretion of ammonia is the most effective way for fishes to defend against ammonia toxicity during terrestrial or ammonia exposure, as it would result in low internal ammonia concentrations and prevent ammonia intoxication in the brain (Peng et al., 1998; Ip et al., 2005). We report for the first time that H. fossilis could excrete ammonia continuously in spite of being immersed in water containing 8 mmol l–1 NH4Cl. The external water containing 8 mmol l–1 NH4Cl (pH 7.0) would have a NH3 concentration of 0.036 mmol l–1 and NH4+ concentration of 7.96 mmol l–1 calculated according to the Henderson–Hasselbalch equation. In comparison, the NH3 and NH4+ concentrations in the blood of H. fossilis were calculated to be 0.0028 and 0.239 mmol l–1, respectively, based on a blood pH of 7.41 (Ip and Chew, unpublished data) and a blood ammonia concentration of 0.242 mmol l–1 (Figure 2A). Therefore, it can be concluded that H. fossilis has the ability to actively excrete ammonia (possibly NH4+) despite being confronted by inwardly-directed NH3 and NH4+ gradients. To date, there are three tropical air-breathing teleosts, P. schlosseri (Peng et al., 1998; Randall et al., 1999; Ip et al., 2005; Chew et al., 2007), A. testudineus (Tay et al., 2006), and C. gariepinus (Ip et al., 2004d), which can actively excrete NH4+. These three fishes can survive for at least 6 days in 75–150 mmol l–1 NH4Cl, which is far greater than the ammonia concentration of less than 10 mmol l–1 NH4Cl tolerated by other fishes in the literature. It has been reported that active NH4+ excretion in the gills of both A. testudineus (Ip et al., 2012b) and P. schlosseri (Chew et al., 2014) involves NKA as part of the NH4+ transport mechanism.
Molecular Characterization of Nkaα Isoforms From the Gills of H. fossilis
NKA/Nka is a universal membrane bound enzyme that serves to actively transport three Na+ out of the cell and two K+ into the cell, supported by energy from ATP hydrolysis (Sweader, 1989). The functioning of this pump is critical for normal cellular functions, such as maintaining the osmotic balance and membrane potential across cells, and providing the driving force for transport of molecules such as glucose and amino acids (Therien and Bolstein, 2000). NKA comprises two major subunits, α and β, which function as a αβ heterodimer. The α-subunit is a large (110–120 kDa) protein with all the functional sites that serve for the catalytic functioning of the enzyme (Blanco and Mercer, 1998). We have identified five different Nkaα isoforms (Nkaα1b, Nkaα1c1, Nkaα1c2, Nkaα2, and Nkaα3) from the gills of H. fossilis. Since Nkaα is responsible for the catalytic and transport functions of Nka, various Nkaα isoforms expressed by a fish may exhibit specific differences in their affinities to Na+ and K+ (see reviews by Hwang and Lee, 2007; Hwang et al., 2011). These differential affinities of branchial Nkaα isoforms toward Na+ and/or K+ are important in euryhaline teleosts, such as the freshwater- and seawater-acclimated tilapia, pufferfish, and milkfish for both hypoosmotic and hyperosmotic osmoregulation, respectively (Lin and Lee, 2005).
Based on comparison with the human NKAα (Ogawa and Toyoshima, 2002), amino acid residues involved in binding of cations in all five Nkaα isoforms of H. fossilis were conserved except for amino acid residue 787 of Nkaα1b where asparagine was substituted by lysine in the Na+ binding site I and K+ binding site II (Figure 6). Mutational studies have demonstrated the importance of asparagine for both Na+ and K+ binding (Pedersen et al., 1998). Substitution of asparagine with alanine severely reduced Na+ and K+ binding, while substitution with glutamine only reduced Na+ binding. Hence, the Na+ and K+ affinities of Nkaα1b could be different from those of Nkaα1c1 and Nkaα1c2 in H. fossilis and NKA/Nka of other animal species. According to Jorgensen (2008), the presence of a lysine residue instead of asparagine residue at amino acid position 787 in the Nkaα1b of the rainbow trout and Atlantic salmon would reduce the Na+:ATP ratio and the work done in one Na+/K+ pump cycle of active Na+ transport. This would render Nkaα1b energetically suitable for Na+ transport against extreme electrochemical gradients during iono-regulation in fresh water. It is therefore logical to deduce that Nkaα1b in the gills of H. fossilis could be involved in ion uptake in fresh water.
The amino acid residues constituting the K+ binding sites of Nkaα1c1 and Nkaα1c2 from H. fossilis are identical to those of Nkaα1c, but different from those of Nkaα1a and Nkaα1b, from the gills of A. testudineus (Figure 6). Ip et al. (2012b) identified the Nkaα1a and Nkaα1b from the gills of A. testudineus as the freshwater isoform and the seawater isoform, respectively, and demonstrated the possible involvement of Nkaα1c in active ammonia excretion when the fish is confronted with environmental ammonia. They (Ip et al., 2012b) concluded that there was a reduction in the effectiveness of NH4+ to substitute for K+ in the activation of Nka during ammonia exposure, and the up-regulation of Nkaα1c expression functions to remove excess Na+ from, and to transport K+ in preference to NH4+ into, the ionocytes so as to maintain intracellular Na+/K+ homeostasis. High similarities in K+ binding sites between Nkaα1c1/Nkaα1c2 of H. fossilis and Nkaα1c of A. testudineus denote that they may exhibit high substrate specificity for K+ and could participate in active ammonia excretion during ammonia exposure.
Ammonia Exposure Increases the Protein Abundances of Nkaα1c Isoforms and Total Nkaα in the Gills of H. fossilis
It is apparent that the expression of Nkaα isoforms in the gills of H. fossilis could be regulated at transcriptional and translational levels. Although molecular characterization revealed that Nkaα1b was unlikely to play a part in the multi-component mechanism of active ammonia excretion in H. fossilis, its transcript level increased significantly on day 6 of exposure to 30 mmol l–1 NH4Cl. However, our attempt to develop a specific anti-NKAα1b to determine the protein abundance of NKAα1b in the gills of the control and experimental fish was unsuccessful. Hence, it remains inconclusive whether NKAα1b is a part of the mechanism involved in active ammonia excretion in H. fossilis. Importantly, there were significant increases in the transcript levels and/or protein abundances of Nkaα1c1, Nkaα1c2, and total Nkaα in the gills of H. fossilis exposed to ammonia. These results indirectly support the proposition that H. fossilis can actively excrete ammonia, with reference to our knowledge on A. testudineus and P. schlosseri. They also corroborate the deduction based on molecular characterization that Nkaα1c1 and Nkaα1c2 could be essential parts of the mechanism that partake in active ammonia excretion in H. fossilis. In the case of A. testudineus, active ammonia excretion through its gills involves a type of Nka-immunoreactive ionocyte which co-expresses a basolateral Na+:K+:2Cl– cotransporter 1a (NKCC1a) and an apical cystic fibrosis transmembrane conductance regulator Cl– channel (Ip et al., 2012b, c; Loong et al., 2012). NH4+ can enter this ionocyte through the basolateral Nkcc1a before being actively transported across the apical membrane. The operation of Nkcc1a in the gills of A. testudineus during active ammonia excretion would lead to an increase in the intracellular Na+ concentration of the ionocyte, and therefore an up-regulation of Nka activity would be necessary to remove the excess Na+. Specifically, exposure to environmental ammonia leads to significant increases in the mRNA expression of nkaα1c, the overall Nka protein abundance, the Nka activity, and the Km for NH4+ relative to K+ in the gills of A. testudineus. Hence, the up-regulation of nkaα1c expression in the gills of ammonia-exposed A. testudineus may serve to remove excess Na+ from, and to transport K+ in preference to NH4+ into, the cell in order to maintain intracellular Na+ and K+ homeostasis (Ip et al., 2012b). Similarly, the increased protein abundances of Nkaα1c1 and Nkaα1c2 in the gills of H. fossilis exposed to ammonia indicate the possible involvement of NKCC and the needs to maintain both the intracellular Na+ and K+ concentrations in the branchial ionocytes involved in active ammonia excretion. Experiments are being performed in the authors’ laboratories to verify the validity of this proposition.
Conclusion
Our results confirmed that H. fossilis is ammonotelic and does not switch to ureotelism when exposed to environmental ammonia. In fact, it does not possess detectable CPSIII or CPSI activities in its liver, and is therefore non-ureogenic. However, it can actively excrete ammonia during ammonia exposure, which also leads to increases in the protein abundances of Nkaα1c1, Nkaα1c2, and total NKAα in its gills. Hence, it can be concluded that active ammonia excretion through the gills with the indirect aid of branchial NKA, but not increased urea synthesis and excretion, engenders the high environmental ammonia tolerance of H. fossilis.
Data Availability Statement
The datasets generated for this study can be found in the GenBank: nkaα1b (MH427004), nkaα1c1 (MH427006), nka1αc2 (MH427005), nkaα2 (MH427007), and nkaα3 (MH427008).
Ethics Statement
The animal study was reviewed and approved by the Nanyang Technological University Institutional Animal Care and Use Committee which gave the approval for fish maintenance and experimentation under the protocol ARFSBS/NIE-A-0311.
Author Contributions
SC and YI designed the experiments and wrote the manuscript. ST, SI, and CP performed the experiments and analyzed the data. SC, SI, and KH participated in animal subjection and sample collection. SC, KH, and YI were involved in the analysis of data and approval of the manuscript.
Funding
This study was supported in part by the Singapore Ministry of Education through grants to SC [through National Institute of Education (NIE) Academic Research Fund RI 3/15 CSF and the NIE Research Support for Senior Academic Administrator Grant RS 5/19 CSF] and to YI (R154-000-470-112).
Conflict of Interest
The authors declare that the research was conducted in the absence of any commercial or financial relationships that could be construed as a potential conflict of interest.
Supplementary Material
The Supplementary Material for this article can be found online at: https://www.frontiersin.org/articles/10.3389/fphys.2019.01615/full#supplementary-material
FIGURE S1 | Protein abundances of Nkaα1c1 and Nkaα1c2 in the gills of H. fossilis exposed to 30 mmol l–1 NH4Cl for 6 days (6 d A). (A) An example of the immunoblot of Nkaα1c1, (left) and Nkaα1c1 pre-incubated with immunizing peptide for the peptide competition assay (PCA; right). (B) An example of the immunoblot of Nkaα1c2 (left) and Nkaα1c2 pre-incubated with immunizing peptide for the peptide competition assay (PCA; right).
TABLE S1 | Primer sequences used for RACE-PCR and qPCR of Na+/K+-ATPase (nka) α-subunit isoforms.
Footnotes
References
Anderson, P. M. (1980). Glutamine- and N-acetylglutamate dependent carbamoyl phosphate synthetase in elasmobranchs. Science 208, 291–293. doi: 10.1126/science.6245445
Anderson, P. M. (2001). “Urea and glutamine synthesis: environmental influences on nitrogen excretion,” in Fish Physiology, eds P. A. Wright and P. M. Anderson (New York, NY: Academic Press), 239–277. doi: 10.1016/s1546-5098(01)20008-9
Anderson, P. M., and Little, R. M. (1986). Kinetic properties of cyanase. Biochemistry 25, 1621–1626. doi: 10.1021/bi00355a026
Anderson, P. M., and Walsh, P. J. (1995). Subcellular localization and biochemical properties of the enzyme carbamoyl phosphate synthetase and urea synthesis in the batrachoidid fishes, Opsanus beta, Opsanus tau and Porichthys notatus. J. Exp. Biol. 198, 755–766.
Anderson, P. M., Wellner, V. P., Rosenthal, G. A., and Meister, A. (1970). Carbamyl phosphate synthetase. Methods Enzymol. 17A, 235–243.
Bergmeyer, H. U., and Beutler, H. O. (1985). “Ammonia,” in Methods of enzymatic analysis, eds H. U. Bergmeyer, J. Bergmeyer, and M. Grabl (New York, NY: Academic Press), 454–461.
Blanco, G., and Mercer, R. W. (1998). Isozymes of the Na, K-ATPase: heterogeneity in structure, diversity and function. Am. J. Physiol. 257, F633–F650. doi: 10.1152/ajprenal.1998.275.5.F633
Bradford, M. M. (1976). A rapid and sensitive method for quantitation of microgram quantities of protein utilising the principle of protein-dye binding. Anal. Biochem. 72, 248–254. doi: 10.1006/abio.1976.9999
Burgess, W. E. (1989). An Atlas of Freshwater and Marine Catfishes: A Preliminary Survey of the Siluriformes. New Jersey: TFH Publications.
Campbell, J. W. (1991). “Excretory nitrogen metabolism,” in Environmental and Metabolic Animal Physiology. Comparative Animal Physiology, ed. C. L. Prosser (New York, NY: Wiley-Interscience), 277–324.
Chew, S. F., Chan, N. K. Y., Loong, A. M., Hiong, K. C., Tam, W. L., and Ip, Y. K. (2004). Nitrogen metabolism in the African lungfish (Protopterus dolloi) aestivating in a mucus cocoon on land. J. Exp. Biol. 207, 777–786. doi: 10.1242/jeb.00813
Chew, S. F., Hiong, K. C., Lam, S. P., Chen, X. L., Ching, B., and Ip, Y. K. (2015). Ammonia exposure increases the expression of Na+:K+:2Cl- cotransporter 1a in the gills of the giant mudskipper, Periophthalmodon schlosseri. J. Comp. Physiol. B 185, 57–72. doi: 10.1007/s00360-014-0867-3
Chew, S. F., Hiong, K. C., Lam, S. P., Ong, S. W., Wee, W. L., Wong, W. P., et al. (2014). Functional roles of Na+/K+-ATPase in active ammonia excretion and seawater acclimation in the giant mudskipper, Periophthalmodon schlosseri. Front. Physiol. 5:158. doi: 10.3389/fphys.2014.00158
Chew, S. F., Hong, L. N., Wilson, J. M., Randall, D. J., and Ip, Y. K. (2003a). Alkaline environmental pH has no effect on the excretion of ammonia in the mudskipper Periophthalmodon schlosseri but inhibits ammonia excretion in the related species Boleophthalmus boddaerti. Physiol. Biochem. Zool. 76, 204–214. doi: 10.1086/374281
Chew, S. F., Ong, T. F., Ho, L., Tam, W. L., Loong, A. M., Hiong, K. C., et al. (2003b). Urea synthesis in the African lungfish Protopterus dolloi – hepatic carbamoyl phosphate synthetase III and glutamine synthetase are upregulated by 6⋅days of aerial exposure. J. Exp. Biol. 206, 3615–3624. doi: 10.1242/jeb.00619
Chew, S. F., and Ip, Y. K. (2014). Excretory nitrogen metabolism and defense against ammonia toxicity in air-breathing fishes. J. Fish Biol. 84, 603–638. doi: 10.1111/jfb.12279
Chew, S. F., and Ip, Y. K. (2017). “Nitrogen metabolism and nitrogenous wastes excretion,” in Fishes Out of Water: The Biology and Ecology of Mudskippers, eds E. Murdy and J. Zeehan (Boca Raton, FL: CRC Press), 167–194. doi: 10.1201/9781315119861-7
Chew, S. F., Sim, M. Y., Phua, Z. C., Wong, W. P., and Ip, Y. K. (2007). Active ammonia excretion in the giant mudskipper, Periophthalmodon schlosseri (Pallas), during emersion. J. Exp. Zool. 307A, 357–369. doi: 10.1002/jez.385
Chew, S. F., Wilson, J. M., Ip, Y. K., and Randall, D. J. (2006). “Nitrogen excretion and defence against ammonia toxicity,” in Fish Physiology The Physiology of Tropical Fishes, eds A. Val, V. Almedia-Val, and D. J. Randall (New York, NY: Academic Press), 307–395. doi: 10.1016/s1546-5098(05)21008-7
Gasteiger, E., Gattiker, A., Hoogland, C., Ivanyi, I., Appel, R. D., and Bairoch, A. (2003). ExPASy: the proteomics server for in-depth protein knowledge and analysis. Nucleic Acids Res. 31, 3784–3788. doi: 10.1093/nar/gkg563
Hall, T. A. (1999). BioEdit: a user-friendly biological sequence editor and analysis program for Windows 95/98/NT. Nucleic Acids Symp. Ser. 41, 95–98.
Hwang, P. P., and Lee, T. H. (2007). New insights into fish ion regulation and mitochondrion-rich cells. Comp. Biochem. Physiol. A 148, 479–497. doi: 10.1016/j.cbpa.2007.06.416
Hwang, P. P., Lee, T. H., and Lin, L. Y. (2011). Ion regulation in fish gills: recent progress in the cellular and molecular mechanisms. Am. J. Physiol. Regul. Integr. Comp. Physiol. 301, R28–R47. doi: 10.1152/ajpregu.00047.2011
Ip, Y. K., and Chew, S. F. (2010). Ammonia production, excretion, toxicity, and defense in fish: a review. Front. Physiol. 1:134. doi: 10.3389/fphys.2010.00134
Ip, Y. K., and Chew, S. F. (2018). Air-breathing and excretory nitrogen metabolism in fishes. Acta Histochem. 120, 680–690. doi: 10.1016/j.acthis.2018.08.013
Ip, Y. K., Chew, S. F., and Randall, D. J. (2001a). “Ammonia toxicity, tolerance and excretion,” in Fish Physiology Nitrogen Excretion, eds P. M. Anderson and P. A. Wright (New York, NY: Academic Press), 109–148. doi: 10.1016/s1546-5098(01)20005-3
Ip, Y. K., Lim, C. B., Chew, S. F., Wilson, J. M., and Randall, D. J. (2001b). Partial amino acid catabolism leading to the formation of alanine in Periophthalmodon schlosseri (mudskipper): a strategy that facilitates the use of amino acids as an energy source during locomotory activity on land. J. Exp. Biol. 204, 1615–1624.
Ip, Y. K., Chew, S. F., and Randall, D. J. (2004a). Five tropical fishes, six different strategies to defend against ammonia toxicity on land. Physiol. Biochem. Zool. 77, 768–782. doi: 10.1086/422057
Ip, Y. K., Chew, S. F., Wilson, J. M., and Randall, D. J. (2004b). Defenses against ammonia toxicity in tropical air breathing fishes exposed to high concentration of environmental ammonia: a review. J. Comp. Physiol. B 174, 565–575.
Ip, Y. K., Randall, D. J., Kok, T. K. T., Barzaghi, C., Wright, P. A., Ballantyne, J. S., et al. (2004c). The giant mudskipper Periophthalmodon schlosseri facilitates active NH4+ excretion by increasing acid excretion and decreasing NH3 permeability in the skin. J. Exp. Biol. 207, 787–801. doi: 10.1242/jeb.00788
Ip, Y. K., Zubaidah, R. M., Liew, P. C., Loong, A. M., Hiong, K. C., Wong, W. P., et al. (2004d). African sharptooth catfish Clarias gariepinus does not detoxify ammonia to urea or amino acids but actively excretes ammonia during exposure to environmental ammonia. Physiol. Biochem. Zool. 77, 242–254. doi: 10.1086/383499
Ip, Y. K., Lee, C. Y., Chew, S. F., Low, W. P., and Peng, K. W. (1993). Differences in the responses of two mudskippers to terrestrial exposure. Zool. Sci. 10, 511–519.
Ip, Y. K., Leong, M. W. F., Sim, M. Y., Goh, G. S., and Chew, S. F. (2005). Chronic and acute ammonia toxicity in mudskippers, Periophthalmodon schlosseri and Boleophthalmus boddaerti: brain ammonia and glutamine contents, and effects of methionine sulfoximine and MK801. J. Exp. Biol. 208, 1993–2004. doi: 10.1242/jeb.01586
Ip, Y. K., Loong, A. M., Chng, Y. R., Hiong, K. C., and Chew, S. F. (2012a). Hepatic carbamoyl phosphate synthetase (CPS) I and urea contents in the hylid tree frog, Litoria caerulea: transition from CPS III to CPS I. J. Comp. Physiol. B 182, 1081–1094. doi: 10.1007/s00360-012-0682-7
Ip, Y. K., Loong, A. M., Kuah, J. S., Sim, E. W. L., Chen, X. L., Wong, W. P., et al. (2012b). The roles of three branchial Na+/K+-ATPase α-subunit isoforms in freshwater adaptation, seawater acclimation and active ammonia excretion in Anabas testudineus. Am. J. Physiol. Regul. Integ. Comp. Physiol. 303, R112–R125. doi: 10.1152/ajpregu.00618.2011
Ip, Y. K., Wilson, J. M., Loong, A. M., Chen, X. L., Wong, W. P., Delgado, I. L. S., et al. (2012c). Cystic fibrosis transmembrane conductance regulator-like Cl- channel in the gills of the climbing perch, Anabas testudineus, is involved in both hypoosmotic regulation during seawater acclimation and active ammonia excretion during ammonia exposure. J. Comp. Physiol. B 182, 793–812. doi: 10.1007/s00360-012-0664-9
Jorgensen, P. L. (2008). Importance for absorption of Na+ from freshwater of lysine, valine and serine substitutions in the α1a-isoform of Na,K-ATPase in the gills of rainbow trout (Oncorhynchus mykiss) and Atlantic salmon (Salmo salar). J. Membrane Biol. 223, 37–47. doi: 10.1007/s00232-008-9111-y
Jow, L. Y., Chew, S. F., Lim, C. B., Anderson, P. M., and Ip, Y. K. (1999). The marble goby Oxyeleotris marmoratus activates hepatic glutamine synthetase and detoxifies ammonia to glutamine during air exposure. J. Exp. Biol. 202, 237–245.
Laemmli, U. K. (1970). Cleavage of structural proteins during the assembly of the head bacteriophage T4. Nature 227, 680–685. doi: 10.1038/227680a0
Lim, C. B., Chew, S. F., Anderson, P. M., and Ip, Y. K. (2001). Reduction in the rates of protein and amino acid catabolism to slow down the accumulation of endogenous ammonia: a strategy potentially adopted by mudskippers (Periophthalmodon schlosseri and Boleophthalmus boddaerti) during aerial exposure in constant darkness. J. Exp. Biol. 204, 1605–1614.
Lin, C. H., and Lee, T. H. (2005). Sodium or potassium ions activate different kinetics of gill Na, K-ATPase in three seawater- and freshwater-acclimated euryhaline teleosts. J. Exp. Zool. 303A, 57–65. doi: 10.1002/jez.a.130
Loong, A. M., Chew, S. F., Wong, W. P., Lam, S. H., and Ip, Y. K. (2012). Both seawater acclimation and environmental ammonia exposure lead to increases in mRNA expression and protein abundance of Na+:K+:2Cl– cotransporter in the gills of the climbing perch, Anabas testudineus. J. Comp. Physiol. B 182, 491–506. doi: 10.1007/s00360-011-0634-7
McGuffin, L. J., Bryson, K., and Jones, D. T. (2000). The PSIPRED protein structure prediction server. Bioinformatics 16, 404–405. doi: 10.1093/bioinformatics/16.4.404
Munshi, J. S. D., and Choudhary, S. (1994). Ecology of Heteropneustes fossilis (Bloch): An Air-Breathing Catfish of South East Asia. Bhagalpur: Freshwater Biological Association of India.
Munshi, J. S. D., and Hughes, G. M. (1992). Air-Breathing Fishes: Their Structure and Life History. New Delhi: Oxford Publication.
Ogawa, H., and Toyoshima, C. (2002). Homology modeling of the cation binding sites of Na+,K+-ATPase. Proc. Natl. Acad. Sci. U.S.A. 99, 15977–15982. doi: 10.1073/pnas.202622299
Pedersen, P. A., Nielsen, J. M., Rasmussen, J. H., and Jorgensen, P. L. (1998). Contribution of Tl+, K+, and Na+ binding of Asn776, Ser775, Thr774, Thr772, and Tyr771 in cytoplasmic part of fifth transmembrane segment in α-subunit of renal Na,K-ATPase. Biochemistry 37, 17818–17827. doi: 10.1021/bi981898w
Peng, K. W., Chew, S. F., Lim, C. B., Kuah, S. S. L., Kok, T. W. K., and Ip, Y. K. (1998). The mudskippers Periophthalmodon schlosseri and Boleophthalmus boddaerti can tolerate environmental NH3 concentration of 446 and 36 μM, respectively. Fish Physiol. Biochem. 19, 59–69.
Pequin, L., and Serfaty, A. (1963). L’excretion ammoniacale chez un Teleosteen dulcicole Cyprinius carpio. L. Comp. Biochem. Physiol. 10, 315–324. doi: 10.1016/0010-406x(63)90230-5
Randall, D. J., Wilson, J. M., Peng, K. W., Kok, T. W. K., Kuah, S. S. L., Chew, S. F., et al. (1999). The mudskipper, Periophthalmodon schlosseri, actively transports NH4+ against a concentration gradient. Am. J. Physiol. Regul. Integr. Comp. Physiol. 277, R1562–R1567. doi: 10.1152/ajpregu.1999.277.6.R1562
Saha, N., Das, L., and Dutta, S. (1999). Types of carbamyl phosphate synthetases and subcellular localization of urea cycle and related enzymes in air-breathing walking catfish, Clarias batrachus, infused with ammonium chloride: a strategy to adapt under hyperammonia stress. J. Exp. Zool. 283, 121–130. doi: 10.1002/(sici)1097-010x(19990201)283:2<121::aid-jez2>3.0.co;2-5
Saha, N., Das, L., Dutta, S., and Goswami, U. C. (2001). Role of ureogenesis in the mud-dwelled Singhi catfish (Heteropneustes fossilis) under condition of water shortage. Comp. Biochem. Physiol. A. 128, 137–146. doi: 10.1016/s1095-6433(00)00282-8
Saha, N., Dkhar, J., Anderson, P. M., and Ratha, B. K. (1997). Carbamyl phosphate synthetases in an air-breathing teleost, Heteropneustes fossilis. Comp. Biochem. Physiol. 116B, 57–63. doi: 10.1016/s0305-0491(96)00193-9
Saha, N., and Ratha, B. K. (1987). Active ureogenesis in a freshwater airbreathing teleost, Heteropneustes fossilis. J. Exp. Zool. 241, 137–141. doi: 10.1002/jez.1402410117
Saha, N., and Ratha, B. K. (1989). A comparative study of ureogenesis in freshwater air-breathing teleosts. J. Exp. Zool. 252, 1–8. doi: 10.1002/jez.1402520102
Saha, N., and Ratha, B. K. (1990). Alterations in excretion pattern of ammonia and urea in a fresh-water air breathing teleost, Heteropneustes fossilis (Bloch) during hyper-ammonia stress. Ind. J. Exp. Biol. 28, 597–599.
Saha, N., and Ratha, B. K. (1994). Induction of ornithine-urea cycle in a freshwater teleost, Heteropneustes fossilis, exposed to high concentrations of ammonium chloride. Comp. Biochem. Physiol. 108B, 315–325. doi: 10.1016/0305-0491(94)90083-3
Saha, N., and Ratha, B. K. (1998). Ureogenesis in Indian air-breathing teleosts: adaptation to environmental constraints. Comp. Biochem. Physiol. 120A, 195–208. doi: 10.1016/s1095-6433(98)00026-9
Shull, G. E., Greeb, J., and Lingrel, J. B. (1986). Molecular cloning of three distinct forms of the Na+,K+-ATPase α-Subunit from rat brain. Biochemistry 25, 8125–8132. doi: 10.1021/bi00373a001
Tam, W. L., Wong, W. P., Loong, A. M., Hiong, K. C., Chew, S. F., Ballantyne, J. S., et al. (2003). The osmotic response of the Asian freshwater stingray (Himantura signifer) to increased salinity: a comparison with marine (Taeniura lymma) and Amazonian freshwater (Potamotrygon motoro) stingrays. J. Exp. Biol. 206, 2931–2940. doi: 10.1242/jeb.00510
Tay, Y. L., Loong, A. M., Hiong, K. C., Lee, S. J., Tng, Y. Y. M., Wee, N. L. J., et al. (2006). Active ammonia transport and excretory nitrogen metabolism in the climbing perch, Anabas testudineus, during 4 days of emersion or 10 minutes of forced exercise on land. J. Exp. Biol. 209, 4475–4489. doi: 10.1242/jeb.02557
Therien, A. G., and Bolstein, R. (2000). Mechanisms of sodium pump regulation. Am. J. Physiol. Cell. Physiol. 279, C541–C566.
Walton, M. J., and Cowey, C. B. (1977). Aspects of ammoniagenesis in rainbow trout, Salmo gairdneri. Comp. Biochem. Physiol. 57, 143–149.
Keywords: air-breathing fishes, carbamoyl phosphate synthetase, nitrogen metabolism, ornithine-urea cycle, Singhi catfish, ureogenesis
Citation: Chew SF, Tan SZL, Ip SCY, Pang CZ, Hiong KC and Ip YK (2020) The Non-ureogenic Stinging Catfish, Heteropneustes fossilis, Actively Excretes Ammonia With the Help of Na+/K+-ATPase When Exposed to Environmental Ammonia. Front. Physiol. 10:1615. doi: 10.3389/fphys.2019.01615
Received: 24 October 2019; Accepted: 23 December 2019;
Published: 22 January 2020.
Edited by:
Pung P. Hwang, Academia Sinica, TaiwanReviewed by:
Sian-Tai Liu, National Taiwan Normal University, TaiwanShigehisa Hirose, Tokyo Institute of Technology, Japan
Alex M. Zimmer, University of Ottawa, Canada
Copyright © 2020 Chew, Tan, Ip, Pang, Hiong and Ip. This is an open-access article distributed under the terms of the Creative Commons Attribution License (CC BY). The use, distribution or reproduction in other forums is permitted, provided the original author(s) and the copyright owner(s) are credited and that the original publication in this journal is cited, in accordance with accepted academic practice. No use, distribution or reproduction is permitted which does not comply with these terms.
*Correspondence: Shit F. Chew, c2Z1bi5jaGV3QG5pZS5lZHUuc2c=