- 1PneumoCardioVascular Laboratory, Hospital Universitário Onofre Lopes, Empresa Brasileira de Serviços Hospitalares (EBSERH), Departamento de Fisioterapia, Universidade Federal do Rio Grande do Norte, Natal, Brazil
- 2Laboratório de Inovação Tecnológica em Reabilitação, Departamento de Fisioterapia, Universidade Federal do Rio Grande do Norte, Natal, Brazil
- 3Dipartimento di Elettronica, Informazione e Bioingegneria, Politecnico di Milano, Milan, Italy
- 4Department of Physical Therapy, University of Toronto, Toronto, ON, Canada
- 5Toronto Rehabilitation Institute, Interdepartmental Division of Critical Care Medicine, University of Toronto, Toronto, ON, Canada
The volumes assessed by optoelectronic plethysmography (OEP) and based on a three-compartmental model provide an accurate breath-by-breath index of expiratory and inspiratory (ribcage muscles and diaphragm) muscle length. Thus, after performing thixotropic maneuvers, OEP may also provide evidence regarding the history-dependent properties of these muscles. We studied the after-effects of different thixotropic conditionings on chest wall (CW) and compartmental operational volumes of 28 healthy subjects (25.5 ± 2.2 years, FVC%pred 94.8 ± 5.5, and FEV1%pred 95.5 ± 8.9) using OEP. Conditionings were composed of inspiratory or expiratory contractions performed from total lung capacity (TLC) or residual volume (RV). The study protocol was composed of three consecutive contractions of the same maneuver, with 60 s of spontaneous breathing in between, and after-effects were studied in the first seven respiratory cycles of each contraction. Cumulative effects were also assessed by comparing the after-effects of each thixotropic maneuver. Inspiratory contractions performed from both TLC and RV acutely increased end-inspiratory (EIV) CW volumes (all p < 0.0001), mainly on both upper and lower ribcage compartments (i.e., non-diaphragmatic inspiratory muscles and diaphragm, respectively); while, expiratory contractions from RV decreased CW volumes (p < 0.0001) by reducing the upper ribcage and abdominal volumes (all p < 0.0001). The response of the thixotropic maneuvers did not present a cumulative effect. In healthy, the use of the three-compartmental model through OEP allows a detailed assessment of the diaphragm, inspiratory and expiratory muscle thixotropy. Furthermore, specific conditioning maneuvers led to thixotropy of the inspiratory ribcage, diaphragm, and expiratory muscles.
Introduction
Skeletal muscle fibers possess complex biophysical properties that make the stiffness and resting tension at a given muscle length dependent on the previous history of movements and contractions. These history-dependent properties, referred in the biomedical literature to as thixotropy (Lakie et al., 1984; Hagbarth et al., 1985; Gregory et al., 1998), have several possible explanations. The most accepted is that proposed by Hill (1968) in which a fraction of the muscle fiber resting tension is generated by stable cross-bridges between actin and myosin filaments. In this sense, the short-range elastic component of the muscle fibers represents the mechanical effects of a small population of slowly cycling cross-bridges, and thixotropic behavior in relaxed muscles reflects a temporary reduction in the number of attached cross-bridges following a mechanical perturbation (Herbst, 1976).
According to Homma and Hagbarth (2000), the rib cage respiratory muscles also possess thixotropic properties. These authors proposed that the history-dependent passive properties of inspiratory muscles are an important component of expiration because expiratory movements need to stretch inspiratory muscles to reduce lung volume. Subsequently, Izumizaki et al. (2004) showed that the intensity and duration of muscle contraction, as well as lung volume and muscle relaxation affects thixotropy behavior. Moreover, the determinant of whether muscles became stiff or slack is muscle length when conditioned, thus lung volume at the time of the muscle contraction can be considered the most influential factor to end-expiratory volume (EEV) changes. Accordingly, a series of studies were performed in both healthy (Izumizaki et al., 2004, 2006c) and COPD patients (Izumizaki et al., 2006b, 2008b) in order to understand the after-effects of different thixotropic conditioning maneuvers on EEV, a measure of functional residual capacity.
The measurement of EEV can be performed indirectly from inspiratory capacity; however, this type of measurement does not follow the breath-by-breath volume variation and may be influenced by the subject’s cooperation. Using optoelectronic plethysmography (OEP), an accurate non-invasive method for measuring total chest wall (CW) volume and ventilation, these problems can be overcome, and changes of both tidal breath and EEV provide an assessment of inspiratory and expiratory muscle length (Dellaca et al., 2001; Aliverti et al., 2004; Wilkens et al., 2010; Fregonezi et al., 2018). In this context, and despite the existence of studies evaluating the presence of thixotropic properties on respiratory muscles especially based on new and more accurate technologies, the present study aimed to analyze the after-effects of different thixotropic conditionings (inspiratory or expiratory contractions) performed from higher (total lung capacity [TLC]) and lower (residual volume [RV]) volumes using OEP. As these after-effects are relatively fast in healthy subjects, the EEV and end-inspiratory (EIV) CW and compartmental volumes, were investigated for the first time on a breath-by-breath basis, thus the variation of both the EEV and EIV could be measured accurately in each single breath. Additionally, as compartmental volumes were analyzed based on a three-compartmental model (Aliverti et al., 2001), the after-effects of the thixotropic maneuvers on the diaphragm (acting on the lower ribcage) and expiratory muscles (acting on abdominal compartment) were also assessed.
Thus, despite the existence of studies evaluating the presence of thixotropic properties on respiratory muscles, the literature is still scarce, especially based on new and more accurate technologies. Accordingly, the present study aimed to analyze the after-effects of different thixotropic conditionings (inspiratory or expiratory contractions) performed from higher (TLC) and lower (RV) volumes using OEP. As these after-effects are relatively fast in healthy subjects, the EEV and EIV CW and compartmental volumes, were investigated for the first time on a breath-by-breath basis, thus the variation of both the EEV and EIV could be measured accurately in each single breath. Additionally, as compartmental volumes were analyzed based on a three-compartmental model (Aliverti et al., 2001), the after-effects of the thixotropic maneuvers on the diaphragm (acting on the lower ribcage) and expiratory muscles (acting on abdominal compartment) were also assessed.
Materials and Methods
Subjects
This is a cross-over study. Twenty-eight self-reported healthy subjects of both genders [14 males and 14 females; age 25.5 ± 2.2 years, with body mass index of 20.6 ± 2 kg/m2, forced vital capacity (FVC) of 4.7 ± 0.4 L and forced expiratory volume in the first second (FEV1) of 3.7 ± 0.5 L] with no history of smoking, without cardiac, neuromuscular or respiratory diseases were studied. Predicted values for pulmonary function were 94.8 ± 5.5% for FVC and 95.5 ± 8.9% for FEV1.
All subjects signed an informed consent form. The study was carried out in accordance with the recommendations of the confines of the World Medical Association Declaration of Helsinki for medical research using human participants and approved by the Research Ethics Committee (Federal University of Rio Grande do Norte, Brazil) under number 1.662.429/2016.
Pulmonary Function
Pulmonary function was assessed by spirometry using a KoKo DigiDoser® (Longmont, CO, United States) spirometer with subjects seated on a standard chair. Assessments were carried out following the acceptability and reproducibility criteria (American Thoracic Society [ATS]/European Respiratory Society [ERS], 2002) and the obtained values were compared to absolute and percentage of predicted spirometric values for the Brazilian population (Pereira et al., 2007).
Chest Wall and Compartmental Volumes
The variation of the CW volume and its compartments [pulmonary ribcage (RCp), abdominal ribcage (RCa), and abdomen (AB)] were assessed using OEP (BTS Bioengineering, Milan, Italy). Six infrared TV cameras (three in front and three behind the subject), previously calibrated at a frequency of 60 frames s–1, were used to record the movement change of 89 retro-reflexive markers placed on the anterior and posterior surfaces of the thorax and abdomen of the subjects (Cala et al., 1996; Aliverti and Pedotti, 2003). A closed surface of the subject’s total CW was reconstructed by connecting the 3-dimensional coordinates of the markers, and the breath-by-breath volume enclosed by this surface was computed by means of an algorithm based on the Gauss theorem (Cala et al., 1996). This theorem allows the direct computation of the volume enclosed by the thoracoabdominal surface approximated by a closed mesh of triangles. An important feature of the OEP system is that it does not require any calibration based on maneuvers performed by the subject under analysis. In fact, CW volumes are obtained by the direct geometrical measurement of the 3-dimensional position of the points, and the accuracy does not depend upon the subject or the presence of any disease (D’Angelo et al., 2011).
From OEP, the end-inspiratory (EIVCW, EIVRCp, EIVRCa, and EIVAB) and EEV CW and compartmental volume variations (EEVCW, EEVRCp, EEVRCa, and EEVAB) were obtained during the protocol and included into data analysis. Additionally, as an altered breathing pattern may contribute to changes in operational volumes (O’Donnell et al., 2001), inspiratory (Ti), expiratory (Te), and total time of respiratory cycle (Ttot) as well as inspiratory and expiratory flow were also analyzed.
Thixotropy Protocol
After the assessment of pulmonary function, the subjects were instructed to sit in a standard chair without back support and the retro-reflexive markers were positioned in the trunk surface of the subjects. Considering the history-dependency of skeletal muscle mechanical properties as well as to standardize the pre-test condition of the muscles before the experiments (Proske et al., 1993), after the explanation of each thixotropy maneuver, the subjects were instructed to remain seated and relaxed for 5 min before data acquisition.
The study protocol was composed of 1 min of quiet spontaneous breathing (QB) followed by a set of one of the following thixotropic maneuvers: Inspiratory contraction from TLC (ICoTLC); inspiratory contraction from RV (ICoRV); expiratory contraction from TLC (ECoTLC); or expiratory contraction from RV (ECoRV). All maneuvers were performed randomly with an interval of 15 min between them.
Each set was composed of three consecutive contractions of the same maneuver with 60 s of QB in between. Each maneuver consisted of the following steps (Figure 1): (1) maximal voluntary lung inflation (TLC) or deflation (RV); (2) five seconds of maximal sustained effort with the airway closed by a rigid mouthpiece shutter (breathed from a mouthpiece); (3) passive relaxation of 3 s; and (4) passive expiration or inspiration (Homma and Hagbarth, 2000; Izumizaki et al., 2004). After the passive relaxation, the shutter was manually reopened and the subject resumed breathing. During the whole protocol, the subjects were instructed to breathe with a mouthpiece coupled in the mouth.
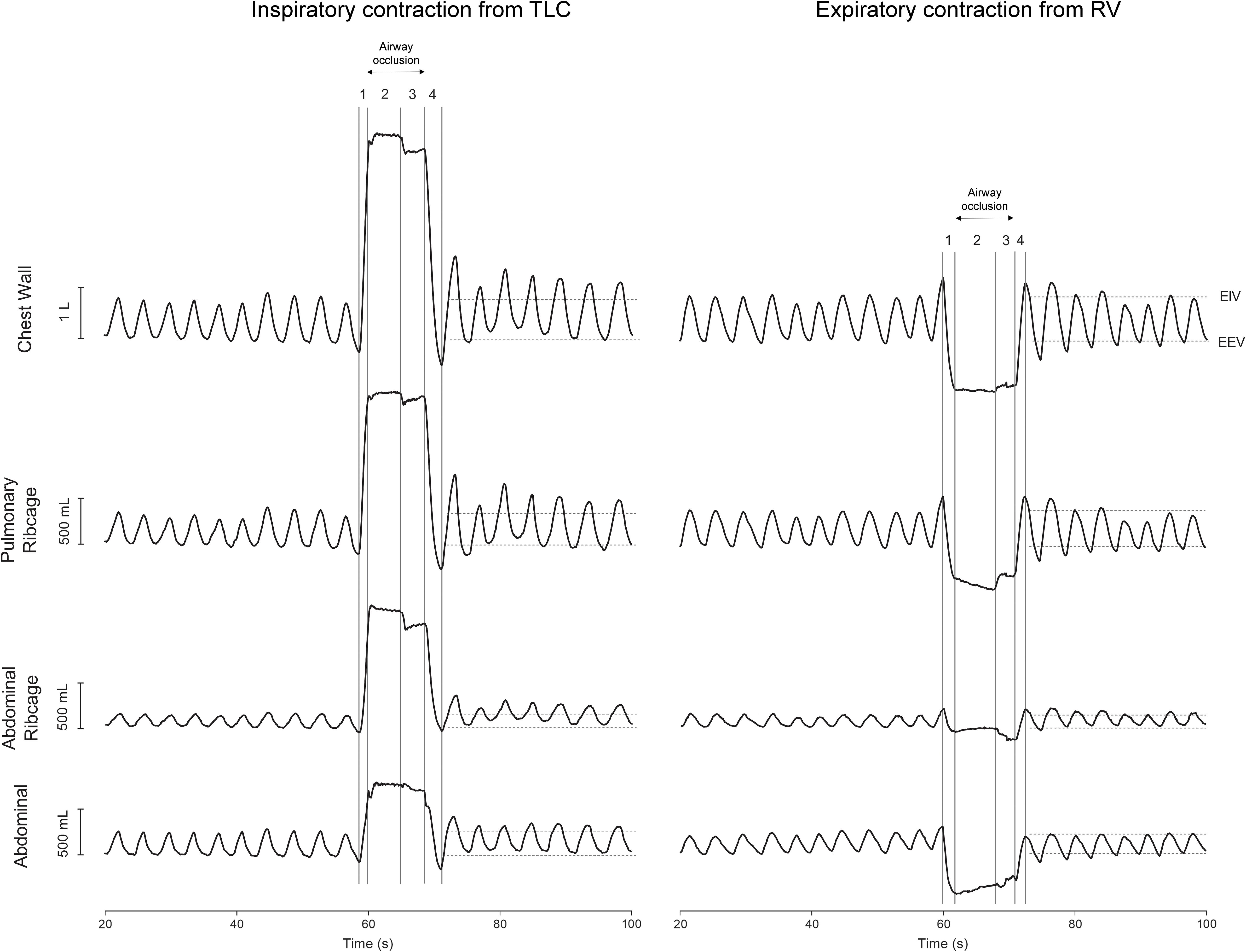
Figure 1. Representative traces of 10 cycles of quiet spontaneous breathing (QB) and the after-effects of inspiratory contraction from total lung capacity (TLC) and expiratory contraction from residual volume (RV) on the first seven cycles of chest wall and compartmental operational volumes. Original resting positions of both end-inspiratory (EIV) and end-expiratory (EEV) volumes obtained during 1 min of QB are indicated by horizontal dashed lines. Left panel: (1) inspiration to TLC, (2) sustained inspiratory effort, (3) voluntary muscle relaxation, and (4) passive expiration. Right panel: (1) expiration to RV, (2) sustained expiratory effort, (3) voluntary muscle relaxation, and (4) passive inspiration. Both records were obtained from the same subject. L, liters; mL, milliliters; s, seconds.
Data Analysis
The averaged of operational (EEV and EIV) CW and compartmental volumes, acquired during 1 min of QB before the first contraction, were defined as baseline levels. In each subject, the after-effects of the maneuvers on operational volumes were studied in detail immediately after each contraction (the first seven respiratory cycles) and compared to baseline. Here, shift values from baseline were signaled as changes in stiffness of respiratory muscles (Homma and Hagbarth, 2000; Izumizaki et al., 2004). Breathing pattern was also studied by analyzing inspiratory time (Ti), expiratory time (Te), and total time of respiratory cycle (Ttot) immediately after each thixotropic maneuver and compared with QB data obtained before the first contraction.
The cumulative effect of the maneuvers (i.e., significantly increases or decreases in CW and compartmental EIV or EEV) was also investigated by comparing each of the seven respiratory cycles after the second and third maneuvers with the after-effects of the first maneuver.
Statistical Analysis
Anthropometric and spirometric data are shown as mean ± SD. Normality and data distribution were verified using Shapiro–Wilk test. Operational volumes, breathing pattern, and cumulative effects were compared using the ANOVA one-way for repeated measures or Friedman’s test followed by Bonferroni’s or Dunn’s post hoc tests, respectively, according to data normality.
Descriptive and inferential analyses were conducted using GraphPad Prism software, version 7.04 (GraphPad Inc., La Jolla, CA, United States). P-values lower than 0.05 (two-sided) were considered statistically significant.
Results
Inspiratory Contraction From TLC
Figure 2 clearly shows that the inspiratory contraction maneuver performed from TLC changes significantly EIV rather than EEV. After the first maneuver, a significant increase in EIVCW, on the first three cycles (p < 0.0001, mean of 0.281 L, see Supplementary Material), was observed. A similar increase was found after the second maneuver, until the fifth respiratory cycle (p < 0.0001, mean of 0.282 L), as well as after the third maneuver until the fourth cycle (p < 0.0001, mean of 305 L). These increases were mainly due to significant changes in EIVRCp (p < 0.0001) and EIVRCa (p < 0.0001) after the first, second, and third maneuvers (see Supplementary Material). Regarding EEVCW, no significant differences were observed when compared with QB values.
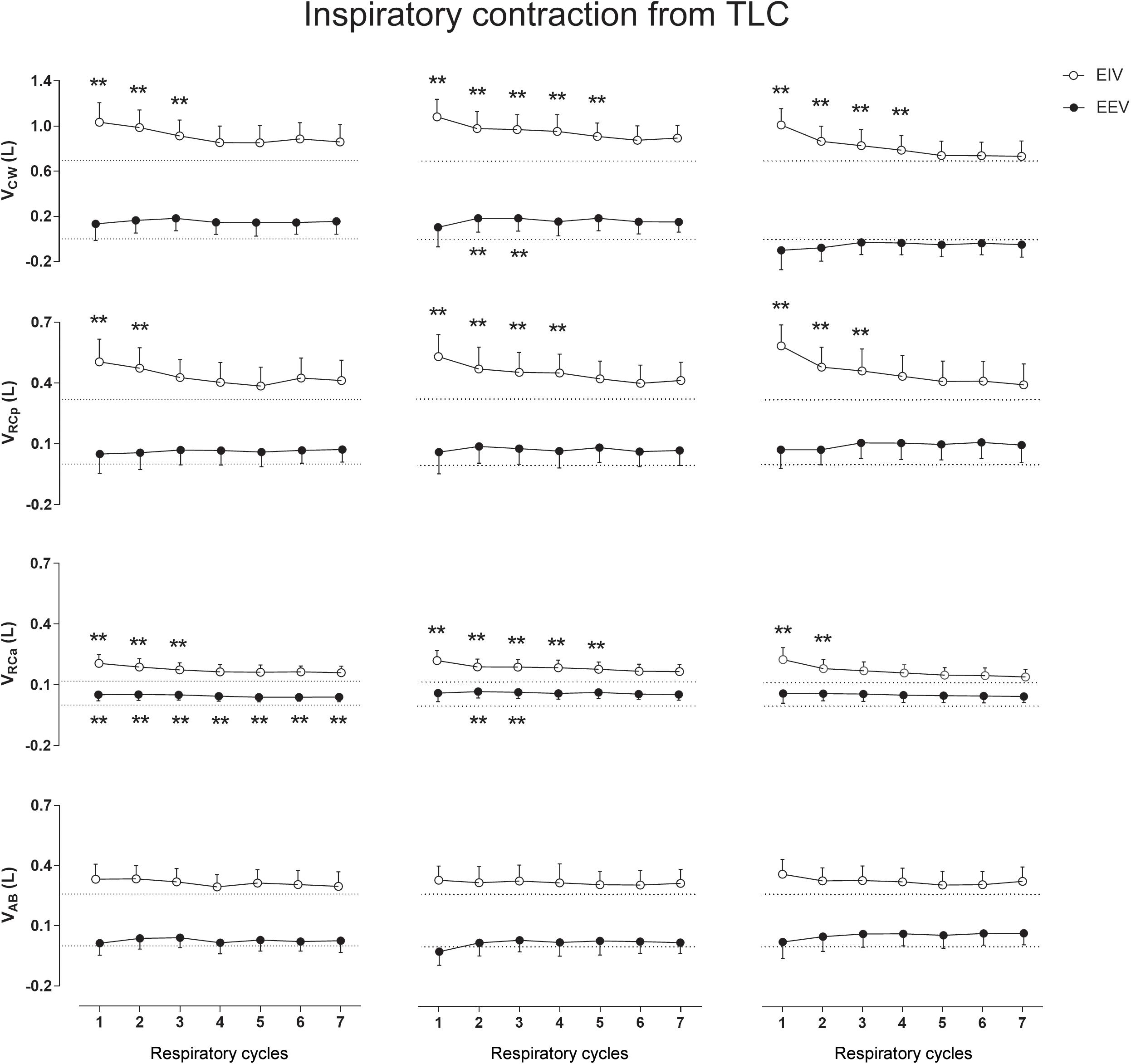
Figure 2. Aftereffects of inspiratory contraction maneuvers performed from total lung capacity (TLC) on end-inspiratory (EIV) and end-expiratory (EEV) volumes of chest wall (CW) and its compartments [pulmonary ribcage (RCp), abdominal ribcage (RCa), and abdominal (AB)]. Data of the first seven respiratory cycles (x-axis) are shown and were compared with mean values (mean of 60 s) of quiet breathing (dotted lines). Left, middle, and right columns shows these variations after the first, second, and third maneuvers, respectively. L, liters; ∗∗p < 0.0001 when compared with quiet breathing.
Expiratory Contraction From TLC
No significant differences were observed in CW and compartmental EIV and EEV during this type of thixotropic contraction (Figure 3).
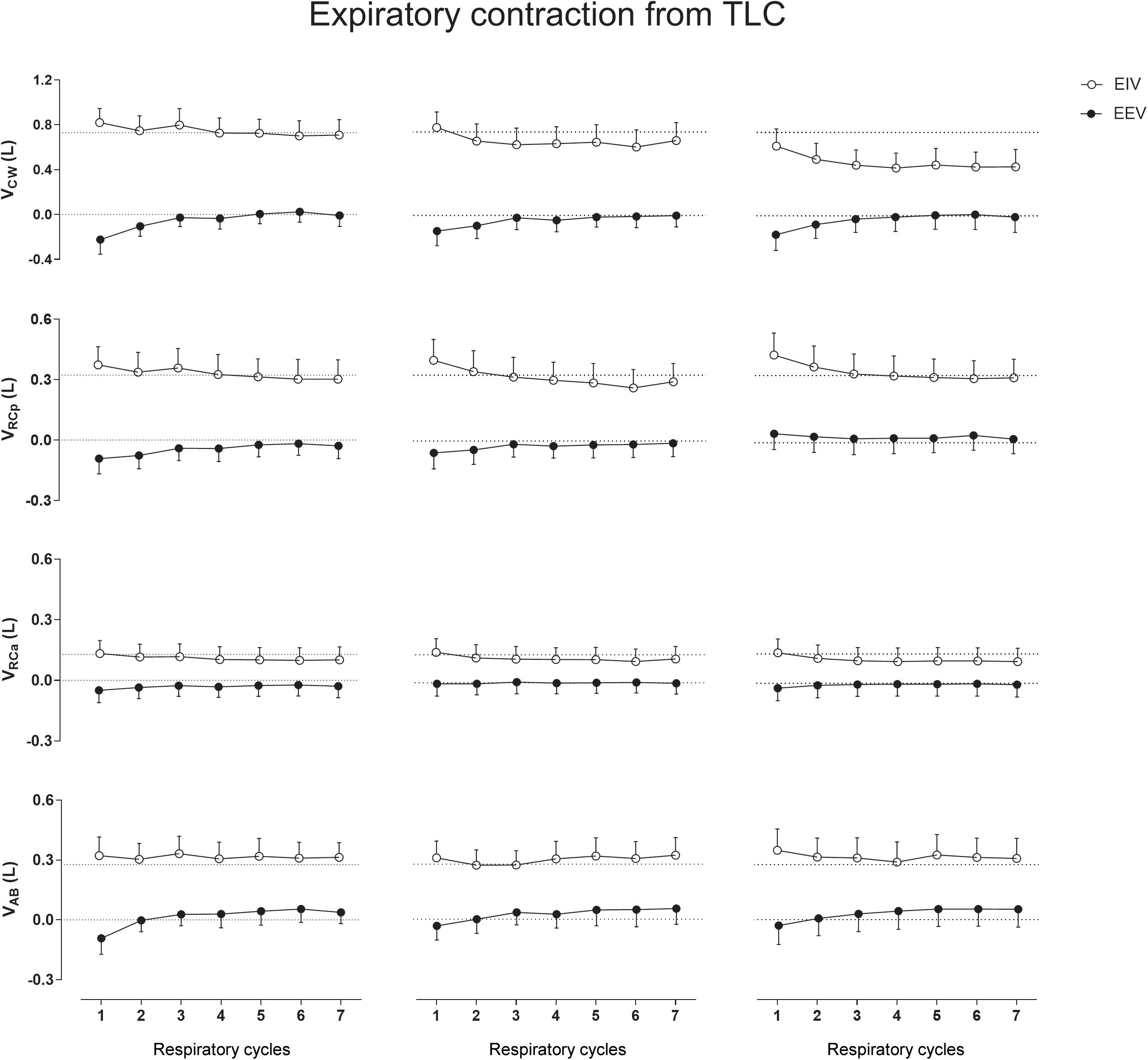
Figure 3. Aftereffects of expiratory contraction maneuvers performed from total lung capacity (TLC) on end-inspiratory (EIV) and end-expiratory (EEV) volumes of chest wall (CW) and its compartments [pulmonary ribcage (RCp), abdominal ribcage (RCa), and abdominal (AB)]. Data of the first seven respiratory cycles (x-axis) are shown and were compared with mean values (mean of 60 s) of quiet breathing (dotted lines). Left, middle, and right columns shows these variations after the first, second, and third maneuvers, respectively. L, liters.
Inspiratory Contraction From RV
Figure 4 shows that, when performed from RV, the inspiratory contractions lead to significant increases in EIV with no significant effects on EEV. Again, the RCp and RCa compartments were the main contributors to the significant increases in EIVCW (p < 0.001) after each maneuver. Neither on RV nor on TLC the AB compartment participated significantly to changes in operational volumes.
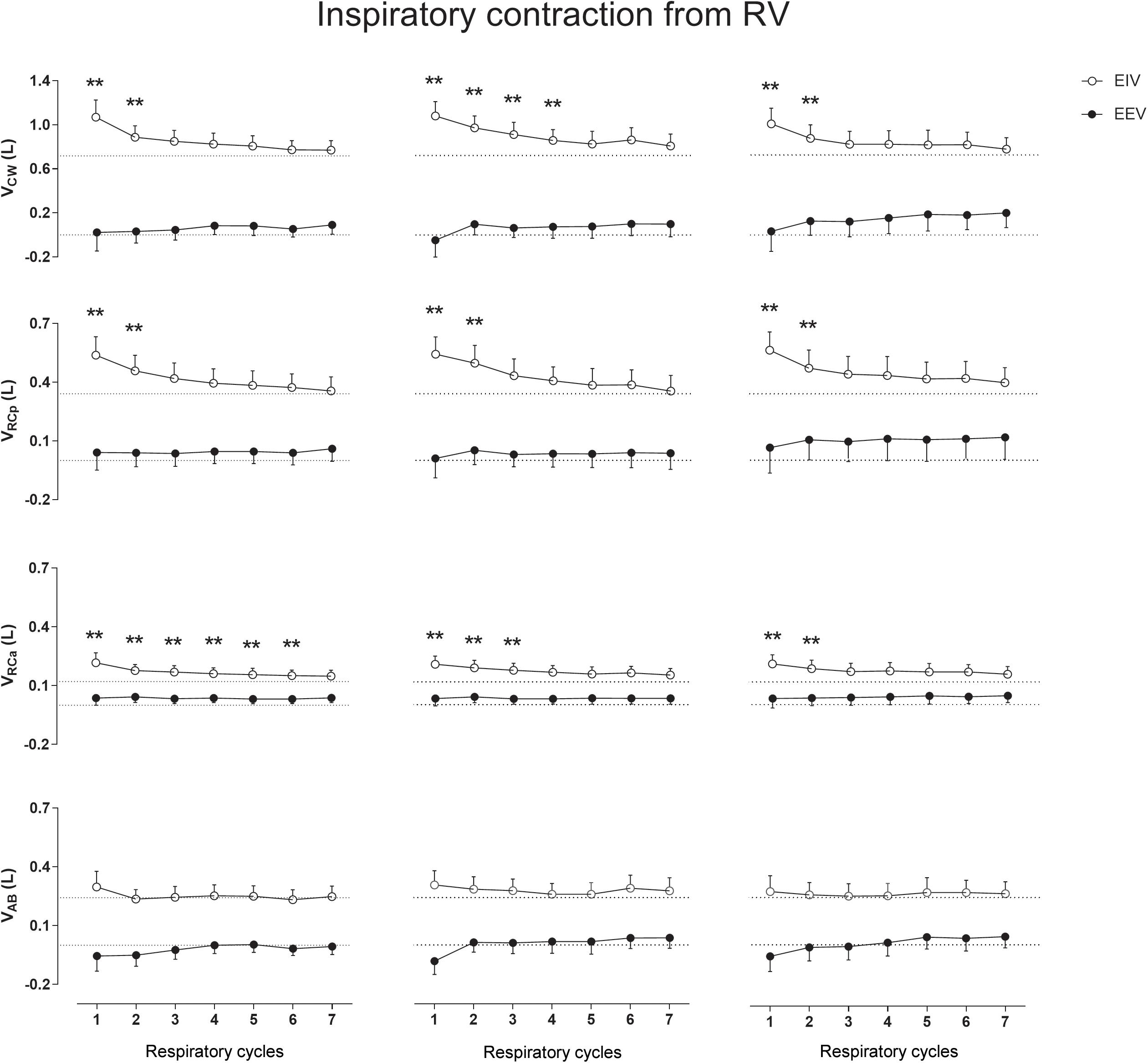
Figure 4. Aftereffects of inspiratory contraction maneuvers performed from residual volume (RV) on end-inspiratory (EIV) and end-expiratory (EEV) volumes of chest wall (CW) and its compartments [pulmonary ribcage (RCp), abdominal ribcage (RCa), and abdominal (AB)]. Data of the first seven respiratory cycles (x-axis) are shown and were compared with mean values (mean of 60 s) of quiet breathing (dotted lines). Left, middle, and right columns shows these variations after the first, second, and third maneuvers, respectively. L, liters; ∗∗p < 0.0001 when compared with quiet breathing.
Expiratory Contraction From RV
When compared with QB no significant differences were observed on CW and compartmental EIV after the ECRV maneuver (Figure 5). On the other hand, the ECRV was by far the most effective maneuver in decreasing EEV. Changes in EEVCW were observed immediately after the first, second, and third expiratory contractions (mean of 0.220, 0.215, and 0.182 L, respectively) and were caused mainly by significant decreases in EEVRCp (p < 0.0001) and EEVAB (p < 0.0001) with no participation of the RCa compartment (see Supplementary Material and Zenodo Repository)1.
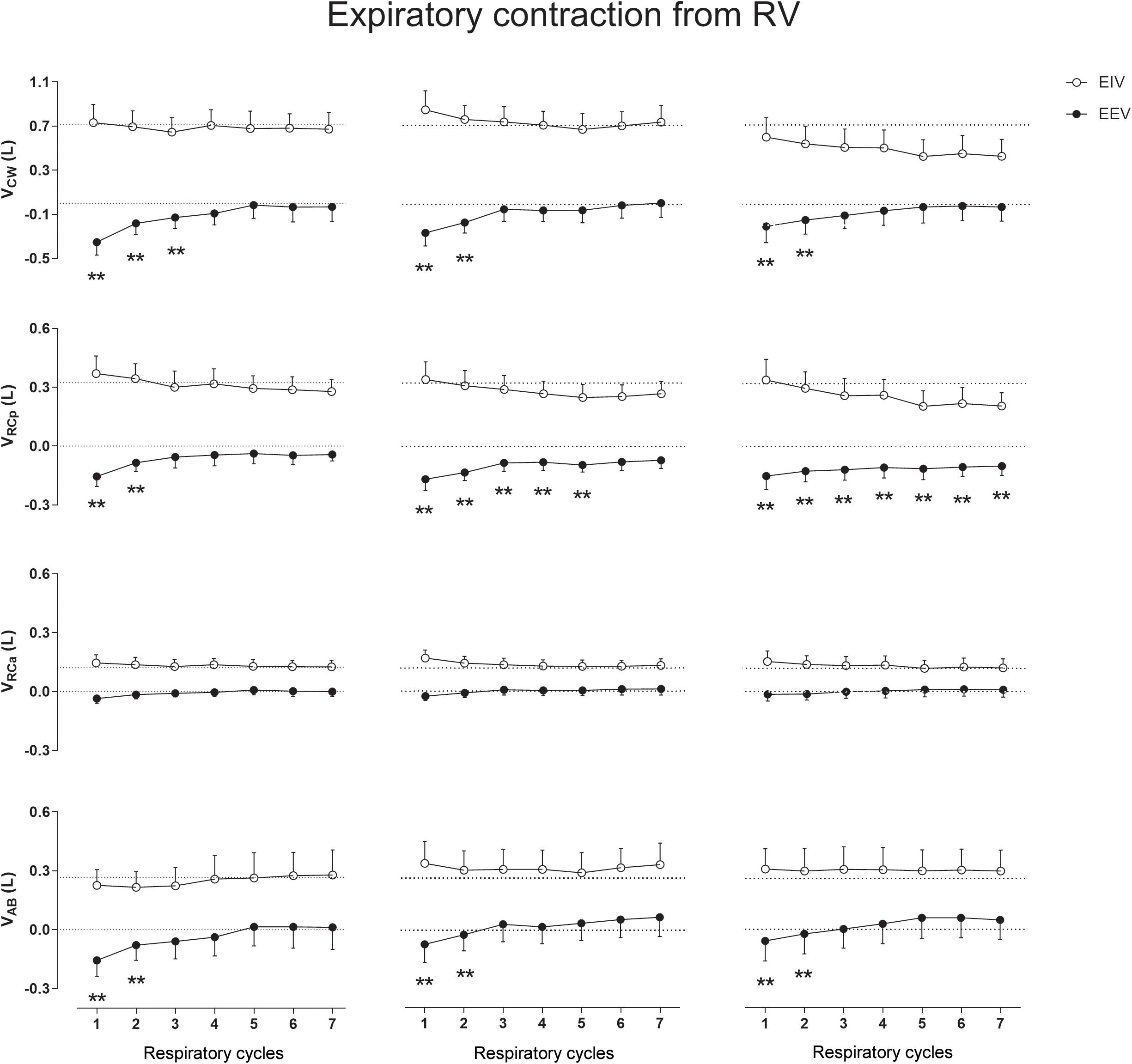
Figure 5. Aftereffects of expiratory contraction maneuvers performed from residual volume (RV) on end-inspiratory (EIV) and end-expiratory (EEV) volumes of chest wall (CW) and its compartments [pulmonary ribcage (RCp), abdominal ribcage (RCa), and abdominal (AB)]. Data of the first seven respiratory cycles (x-axis) are shown and were compared with mean values (mean of 60 s) of quiet breathing (dotted lines). Left, middle, and right columns shows these variations after the first, second, and third maneuvers, respectively. L, liters; ∗∗p < 0.0001 when compared with quiet breathing.
Cumulative Effect Analysis
In all types of thixotropy contractions performed, no cumulative effects were observed between the three sets of maneuvers in both CW and compartmental EIV and EEV.
Breathing Pattern
No significant differences in Ti, Te, and Ttot (see Supplementary Material) as well as inspiratory and expiratory flow (data not shown) were found after the conditioning maneuvers.
Discussion
This is the first study to report the detailed after-effects of thixotropic maneuvers on the three-compartmental CW and compartmental operational volumes using OEP. The main findings of this study were that ICoTLC and ICoRV thixotropic conditionings affect equally the operational volumes of healthy subjects; i.e., increases EIVCW. More specifically, the after-effects of these two thixotropic conditionings were particularly pronounced on both RCp and RCa compartments. On the other hand, only the ECoRV thixotropic conditioning was able to decrease EEVRCp and EEVAB with no influences on EIV.
The precise mechanisms responsible for the history-dependent thixotropic behavior of skeletal muscle fibers are still controversial (Axelson and Hagbarth, 2003). This history-dependent fraction of muscular resting tension may be partly explicable in terms of several hypotheses, namely, (1) cross-bridge formation between actin and myosin filaments, (2) the titin-actin, and (3) the Ca2+ interactions (Kellermayer et al., 1997) [surrounding passive tissues as well as pressure and viscosity of the intra- and inter-cellular fluids may also play a role. For review see Roberts (2016)]. According to Hill (1968), those cross-bridges formed in the resting muscle disrupt during stretch and only reform if the muscles are left undisturbed. Additionally, the reformation of those cross-bridges is not instantaneous, but may continue for several seconds and, during this interval, the resting tension gradually changes as a result of the built-up tension by newly formed cross-bridges (Campbell and Lakie, 1998). Regarding titin, a spring filament whose characteristic length and stiffness changes with the unfolding of molecular bonds (Kellermayer et al., 1997), its individual molecules are thixotropic by its nature since they display pronounced history-dependent viscoelastic behavior during an active stretch that may lead to exponential enhance in passive force production of the muscle (Kellermayer et al., 2001; Powers et al., 2014). Evidence also point out to the Ca2+ dependence in both cross-bridge (Campbell and Lakie, 1998; Campbell and Moss, 2000) and titin mechanisms (Labeit et al., 2003) (i.e., increasing the number of cross-bridges in the former, and changing the passive force levels in the latter) (Moss et al., 1976; Campbell and Moss, 2000; Tatsumi et al., 2001).
The results of previous studies (Homma and Hagbarth, 2000; Izumizaki et al., 2008a) showed that the after-effects of thixotropic conditionings on EEV were much more prominent when combining deep inspirations or expirations with strong isometric contractions of the respiratory muscles, being of minor importance the direction of the forced efforts (toward inspiration of expiration). This indicates that the after-effects of conditioning primarily depend on changes in passive properties attributable to cross-bridge kinetics. Although no significant effects were observed in our study when expiratory contractions were performed from TLC, a more detailed breath-by-breath analysis of the after-effects presented in Figure 3 shows a decrease in the first EEVCW respiratory cycle (due to decreases in EEVRCp and EEVAB) suggesting a pattern similar to that observed in Homma and Hagbarth’s (2000) study. On the other hand, after ICoTLC and ECoRV conditionings, it was observed that specific operational CW volumes change with the direction of contraction to the shortened muscle. At inflated lung volumes, the inspiratory muscles are shortened and expiratory muscles are lengthened, while the opposite occurs during lung deflation. In this sense, inspiratory and expiratory muscle stiffness could increase after ICoTLC and ECoRV conditioning, respectively, which could counteract the effects of the ensuing inspiratory muscle lengthening during lung deflation (Izumizaki et al., 2004) (and inflation, in case of ECoRV), thus increasing EIVCW and EEVCW. Moreover, according to Homma and Hagbarth (2000), thixotropy of the antagonistic respiratory muscles also may occur and would work together with thixotropy of the contracted muscles. For example, if voluntary co-contraction of expiratory muscles occurs at an inflated lung position slackness will be introduced in the expiratory muscles favoring operational volume elevation.
The after-effects of both ICoTLC and ICoRV conditionings were clearly evidenced in the upper ribcage (RCp and RCa) and are in accordance with previous studies (Izumizaki et al., 2004, 2006a,b) performed using respiratory inductance plethysmography. Although measurements errors and artifactual EEV changes may be presented while using this system (American Thoracic Society [ATS]/European Respiratory Society [ERS], 2002), our results using OEP strongly supports the hypothesis that thixotropy of ribcage inspiratory muscles plays an important part in CW inflation (Homma and Hagbarth, 2000; Izumizaki et al., 2006c). In this context, and in the absence of such study using diaphragmatic fibers as well as assuming that diaphragm muscle is similar to limb muscles, the short-range elastic component of diaphragm fibers may also contribute to thixotropy of lengthening–shortening cycles and affect the mechanics of breathing (Sieck et al., 2013). Indeed, our findings suggest that specific conditioning maneuvers may result in diaphragm thixotropy behavior since significant increases in EIVRCa were observed (Konno and Mead, 1967) during inspiratory contractions of both ICTLC and ICRV conditionings. These observations were feasible due to the high accuracy of the breath-by-breath OEP volume measurements as well as the use of the three-compartmental model (which provides an index of overall and compartmental inspiratory and expiratory muscle length and shortening), and may be of great interest in clinical rehabilitation for increasing lung volume in a population with pulmonary restrictive pattern.
Expiratory muscle activation following conditioning might contribute to thoracic-volume deflation, thus possibly reducing lung hyperinflation (Izumizaki et al., 2006b). In our study, it is possible that the AB compartment did not participate actively in the variation of operational volumes during ICoTLC, ICoRV, and ECoTLC due to its function in stabilizing the CW (Loring and Mead, 1982). However, during the ECoRV maneuver EEVAB decreased significantly and, although changes in EEVRCp accounted the most for the decrease in EEVCW (see Supplementary Material) as also showed in a previous study (Homma and Hagbarth, 2000), these findings support the hypothesis that the expiratory muscles present thixotropy behavior (Izumizaki et al., 2006a, 2008a). Apart from being relatively fast (Izumizaki et al., 2006a), this transient effect may be of particularly importance in clinical settings, mainly in those COPD patients that present hyperinflation since contributes do abdominal deflation and consequently decrease in functional RV.
In our study using OEP, no lingering effects were observed. This result is in line with those reported by Campbell and Moss (2000) which showed that intrafilamentary movement of actively contracting muscles reduces the number of cross-bridges bound between the muscle filaments with a high cross-bridge reformation rate. As ribcage respiratory muscles are, by default, in constant contraction/relaxation activity during QB, the influence of both intrafilamentary movement of respiratory muscles and the time taken to the next thixotropy conditioning may have influenced the results. However, we believe that this was not the only factor affecting the results. Straining against a closed mouthpiece may also not affect the moles of gas in the lungs, but it will have considerable effects on the configuration of the thorax and also on lung volume via gas compression and rarefaction (which was not calculated due to lack of airway pressure measurements). This means that the various tissues of the thorax (ligaments, tendons, and connective in addition to muscle) will all be stretched, and since these tissues are all viscoelastic they will exhibit stress recovery afterward. The fact that expiratory contractions tended to affect EEV exclusively (Figures 3, 5), and vice versa for EIV (Figures 2, 4), supports the idea that viscoelastic structures were being stretched past their normal operating points for maximal expiration and inspiration, respectively.
It was also showed that in healthy subjects breathing pattern is not influenced by the conditioning maneuvers. The increases in EIV after ICoTLC and ICoRV could be simply a result of an increased inspiratory airflow since Ti remained constant. However, neither inspiratory nor expiratory flow was changed after these conditionings. Thus, the results support the assumption that breathing pattern is of minor importance to the thixotropic behavior of respiratory muscles in healthy subjects (Izumizaki et al., 2004).
A major limitation of our study was the lack of mouth pressure acquisition during the maneuvers. Nevertheless, a strong contraction was requested to all subjects during all procedures. The lack of EMG acquisition is also a major limitation since the findings regarding EIV could be a result of an increase in inspiratory muscle activation after a period of apnea. Additionally, it cannot be maintained that ribcage respiratory muscles alone were responsible for the after-effects alone. Activation of muscles other than those of the ribcage itself may have affected the results and, if these muscles also possess thixotropic properties, they may contribute to the observed after-effects (Homma and Hagbarth, 2000). Plastic properties of other structures in the CW and lung hysteresis may also play a role in changing the relaxed volume of the respiratory system. However, albeit we did not measure the after-effects on thoracoabdominal asynchrony, the use of the OEP and breath-by-breath analysis of the three-compartmental model of the CW clarify some questions raised by Izumizaki et al. (2006c) and provides new information about the influence of thixotropic conditionings on the muscles acting on RCp (non-diaphragmatic inspiratory muscles), RCa (diaphragm), and AB (expiratory muscles) compartments.
Conclusion
The present study shows that both EIV and EEV CW and compartmental volumes change with different thixotropic conditionings. Moreover, the use of the three-compartmental model through OEP allowed the demonstration of the diaphragm and expiratory muscle thixotropy evidence in healthy subjects. The results can also be of great interest in clinical rehabilitation since specific thixotropic conditionings may be useful in reducing functional residual capacity as well as increase EIV.
Data Availability Statement
All datasets generated for this study are included in the article/Supplementary Material.
Ethics Statement
The studies involving human participants were reviewed and approved by the Research Ethics Committee (Federal University of Rio Grande do Norte, Brazil) under number 1.662.429/2016. The patients/participants provided their written informed consent to participate in this study.
Author Contributions
IL, AA, and GF designed and supervised the study. IL, AS, MG, and AL collected the data. IL, AS, AA, EM, and AL analyzed and interpreted the data. IL wrote the article. WR, AA, and GF revised the article.
Funding
This work was supported by the Conselho Nacional de Desenvolvimento Científico e Tecnológico (CNPq) Number: 214699/2013-7, Guilherme Augusto de Freitas Fregonezi received a grant from CNPq Number: 312876/2018-1, and Coordenação de Aperfeiçoamento de Pessoal de Nível Superior (CAPES) Programa Geral de Cooperação Internacional (PGCI) Number: 23038.007514/2014-78.
Conflict of Interest
The authors declare that the research was conducted in the absence of any commercial or financial relationships that could be construed as a potential conflict of interest.
Supplementary Material
The Supplementary Material for this article can be found online at: https://www.frontiersin.org/articles/10.3389/fphys.2019.01376/full#supplementary-material
Footnotes
References
Aliverti, A., Dellaca, R., Pelosi, P., Chiumello, D., Gatihnoni, L., and Pedoti, A. (2001). Compartmental analysis of breathing in the supine and prone positions by optoelectronic plethysmography. Ann. Biomed. Eng. 29, 60–70. doi: 10.1114/1.1332084
Aliverti, A., and Pedotti, A. (2003). Opto-electronic plethysmography. Monaldi Arch. Chest Dis. 59, 12–16.
Aliverti, A., Stevenson, N., Dellaca, R. L., Lo Mauro, A., Pedotti, A., and Calverley, P. M. (2004). Regional chest wall volumes during exercise in chronic obstructive pulmonary disease. Thorax 59, 210–216. doi: 10.1136/thorax.2003.011494
American Thoracic Society [ATS]/European Respiratory Society [ERS] (2002). ATS/ERS statement on respiratory muscle testing. Am. J. Respir. Crit. Care Med. 166, 518–624. doi: 10.1164/rccm.166.4.518
Axelson, H. W., and Hagbarth, K. E. (2003). Human motor compensations for thixotropy-dependent changes in resting wrist joint position after large joint movements. Acta Physiol. Scand. 179, 389–398. doi: 10.1046/j.0001-6772.2003.01217.x
Cala, S. J., Kenyon, C. M., Ferrigno, G., Carnevali, P., Aliverti, A., Pedotti, A., et al. (1996). Chest wall and lung volume estimation by optical reflectance motion analysis. J. Appl. Physiol. 81, 2680–2689. doi: 10.1152/jappl.1996.81.6.2680
Campbell, K. S., and Lakie, M. (1998). A cross-bridge mechanism can explain the thixotropic short-range elastic component of relaxed frog skeletal muscle. J. Physiol. 510(Pt 3), 941–962. doi: 10.1111/j.1469-7793.1998.941bj.x
Campbell, K. S., and Moss, R. L. (2000). A thixotropic effect in contracting rabbit psoas muscle: prior movement reduces the initial tension response to stretch. J. Physiol. 525(Pt 2), 531–548. doi: 10.1111/j.1469-7793.2000.00531.x
D’Angelo, M. G., Romei, M., Lo Mauro, A., Marchi, E., Gandossini, S., Bonato, S., et al. (2011). Respiratory pattern in an adult population of dystrophic patients. J. Neurol. Sci. 306, 54–61. doi: 10.1016/j.jns.2011.03.045
Dellaca, R. L., Aliverti, A., Pelosi, P., Carlesso, E., Chiumello, D., Pedotti, A., et al. (2001). Estimation of end-expiratory lung volume variations by optoelectronic plethysmography. Crit. Care Med. 29, 1807–1811. doi: 10.1097/00003246-200109000-00026
Fregonezi, G., Sarmento, A., Pinto, J., Lomauro, A., Resqueti, V., and Aliverti, A. (2018). Thoracoabdominal asynchrony contributes to exercise limitation in mild asthmatic subjects. Front. Physiol. 9:719. doi: 10.3389/fphys.2018.00719
Gregory, J. E., Wise, A. K., Wood, S. A., Prochazka, A., and Proske, U. (1998). Muscle history, fusimotor activity and the human stretch reflex. J. Physiol. 513(Pt 3), 927–934. doi: 10.1111/j.1469-7793.1998.927ba.x
Hagbarth, K. E., Hagglund, J. V., Nordin, M., and Wallin, E. U. (1985). Thixotropic behaviour of human finger flexor muscles with accompanying changes in spindle and reflex responses to stretch. J. Physiol. 368, 323–342. doi: 10.1113/jphysiol.1985.sp015860
Herbst, M. (1976). Studies on the relation between latency relaxation and resting cross-bridges of frog skeletal muscle. Pflügers Arch. 364, 71–76. doi: 10.1007/bf01062914
Hill, D. K. (1968). Tension due to interaction between the sliding filaments in resting striated muscle. The effect of stimulation. J. Physiol. 199, 637–684. doi: 10.1113/jphysiol.1968.sp008672
Homma, I., and Hagbarth, K. E. (2000). Thixotropy of rib cage respiratory muscles in normal subjects. J. Appl. Physiol. 89, 1753–1758. doi: 10.1152/jappl.2000.89.5.1753
Izumizaki, M., Iwase, M., Ohshima, Y., and Homma, I. (2006a). Acute effects of thixotropy conditioning of inspiratory muscles on end-expiratory chest wall and lung volumes in normal humans. J. Appl. Physiol. 101, 298–306. doi: 10.1152/japplphysiol.01598.2005
Izumizaki, M., Kakizaki, F., Tanaka, K., and Homma, I. (2006b). Immediate effects of thixotropy conditioning of inspiratory muscles on chest-wall volume in chronic obstructive pulmonary disease. Respir. Care 51, 750–757.
Izumizaki, M., Ohshima, Y., Iwase, M., and Homma, I. (2006c). Chest wall motion after thixotropy conditioning of inspiratory muscles in healthy humans. J. Physiol. Sci. 56, 433–440. doi: 10.2170/physiolsci.rp012406
Izumizaki, M., Nakajima, T., Iwase, M., Ohshima, Y., and Homma, I. (2008a). Effect of thixotropy conditioning of inspiratory muscles on the chest wall response to CPAP. Respirology 13, 379–386. doi: 10.1111/j.1440-1843.2007.01228.x
Izumizaki, M., Satake, M., Takahashi, H., Sugawara, K., Shioya, T., and Homma, I. (2008b). Effects of inspiratory muscle thixotropy on the 6-min walk distance in COPD. Respir. Med. 102, 970–977. doi: 10.1016/j.rmed.2008.02.007
Izumizaki, M., Shibata, M., and Homma, I. (2004). Factors contributing to thixotropy of inspiratory muscles. Respir. Physiol. Neurobiol. 140, 257–264. doi: 10.1016/j.resp.2004.03.001
Kellermayer, M. S., Smith, S. B., Bustamante, C., and Granzier, H. L. (2001). Mechanical fatigue in repetitively stretched single molecules of titin. Biophys. J. 80, 852–863. doi: 10.1016/s0006-3495(01)76064-x
Kellermayer, M. S., Smith, S. B., Granzier, H. L., and Bustamante, C. (1997). Folding-unfolding transitions in single titin molecules characterized with laser tweezers. Science 276, 1112–1116. doi: 10.1126/science.276.5315.1112
Konno, K., and Mead, J. (1967). Measurement of the separate volume changes of rib cage and abdomen during breathing. J. Appl. Physiol. 22, 407–422. doi: 10.1152/jappl.1967.22.3.407
Labeit, D., Watanabe, K., Witt, C., Fujita, H., Wu, Y., Lahmers, S., et al. (2003). Calcium-dependent molecular spring elements in the giant protein titin. Proc. Natl. Acad. Sci. U.S.A. 100, 13716–13721. doi: 10.1073/pnas.2235652100
Lakie, M., Walsh, E. G., and Wright, G. W. (1984). Resonance at the wrist demonstrated by the use of a torque motor: an instrumental analysis of muscle tone in man. J. Physiol. 353, 265–285. doi: 10.1113/jphysiol.1984.sp015335
Loring, S. H., and Mead, J. (1982). Action of the diaphragm on the rib cage inferred from a force-balance analysis. J. Appl. Physiol. Respir. Environ. Exerc. Physiol. 53, 756–760. doi: 10.1152/jappl.1982.53.3.756
Moss, R. L., Sollins, M. R., and Julian, F. J. (1976). Calcium activation produces a characteristic response to stretch in both skeletal and cardiac muscle. Nature 260, 619–621. doi: 10.1038/260619a0
O’Donnell, D. E., D’arsigny, C., and Webb, K. A. (2001). Effects of hyperoxia on ventilatory limitation during exercise in advanced chronic obstructive pulmonary disease. Am. J. Respir. Crit. Care Med. 163, 892–898. doi: 10.1164/ajrccm.163.4.2007026
Pereira, C. A. D. C., Sato, T., and Rodrigues, S. C. (2007). Novos valores de referência para espirometria forçada em brasileiros adultos de raça branca. J. Bras. Pneumol. 33, 397–406. doi: 10.1590/s1806-37132007000400008
Powers, K., Schappacher-Tilp, G., Jinha, A., Leonard, T., Nishikawa, K., and Herzog, W. (2014). Titin force is enhanced in actively stretched skeletal muscle. J. Exp. Biol. 217, 3629–3636. doi: 10.1242/jeb.105361
Proske, U., Morgan, D. L., and Gregory, J. E. (1993). Thixotropy in skeletal muscle and in muscle spindles: a review. Prog. Neurobiol. 41, 705–721. doi: 10.1016/0301-0082(93)90032-n
Roberts, T. J. (2016). Contribution of elastic tissues to the mechanics and energetics of muscle function during movement. J. Exp. Biol. 219, 266–275. doi: 10.1242/jeb.124446
Sieck, G. C., Ferreira, L. F., Reid, M. B., and Mantilla, C. B. (2013). Mechanical properties of respiratory muscles. Compr. Physiol. 3, 1553–1567. doi: 10.1002/cphy.c130003
Tatsumi, R., Maeda, K., Hattori, A., and Takahashi, K. (2001). Calcium binding to an elastic portion of connectin/titin filaments. J. Muscle Res. Cell Motil. 22, 149–162.
Wilkens, H., Weingard, B., Lo Mauro, A., Schena, E., Pedotti, A., Sybrecht, G. W., et al. (2010). Breathing pattern and chest wall volumes during exercise in patients with cystic fibrosis, pulmonary fibrosis and COPD before and after lung transplantation. Thorax 65, 808–814. doi: 10.1136/thx.2009.131409
Keywords: optoelectronic plethysmography, lung volume measurements, lung capacities, muscle thixotropy, respiratory muscles
Citation: Lima INDF, Sarmento A, Goes MC, Mazzuca E, Lomauro A, Reid WD, Aliverti A and Fregonezi GADF (2019) After-Effects of Thixotropic Maneuvers on Chest Wall and Compartmental Operational Volumes of Healthy Subjects Using Optoelectronic Plethysmography. Front. Physiol. 10:1376. doi: 10.3389/fphys.2019.01376
Received: 26 May 2019; Accepted: 18 October 2019;
Published: 01 November 2019.
Edited by:
Thomas Similowski, INSERM U1158 Neurophysiologie Respiratoire Expérimentale et Clinique, FranceReviewed by:
Bela Suki, Boston University, United StatesBruno-Pierre Dubé, Centre Hospitalier de l’Université de Montréal (CHUM), Canada
Copyright © 2019 Lima, Sarmento, Goes, Mazzuca, Lomauro, Reid, Aliverti and Fregonezi. This is an open-access article distributed under the terms of the Creative Commons Attribution License (CC BY). The use, distribution or reproduction in other forums is permitted, provided the original author(s) and the copyright owner(s) are credited and that the original publication in this journal is cited, in accordance with accepted academic practice. No use, distribution or reproduction is permitted which does not comply with these terms.
*Correspondence: Illia Nadinne Dantas Florentino Lima, aWxsaWFsaW1hQHlhaG9vLmNvbS5icg==