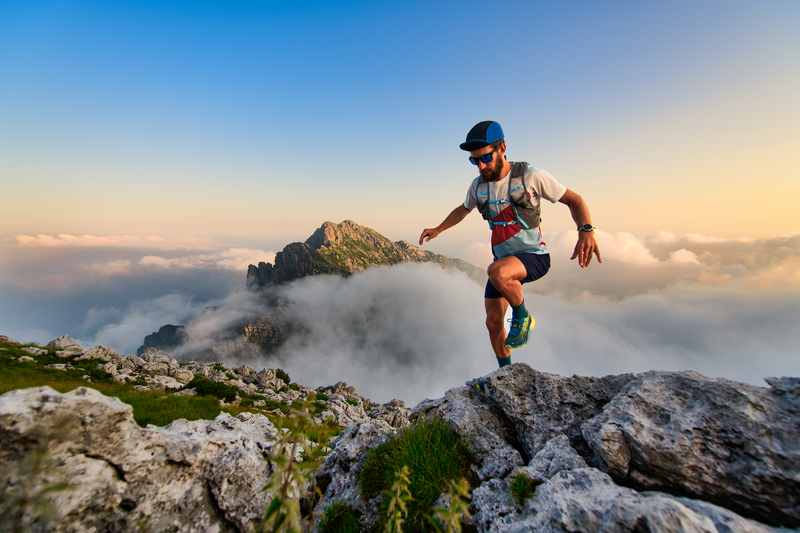
94% of researchers rate our articles as excellent or good
Learn more about the work of our research integrity team to safeguard the quality of each article we publish.
Find out more
ORIGINAL RESEARCH article
Front. Physiol. , 10 July 2019
Sec. Aquatic Physiology
Volume 10 - 2019 | https://doi.org/10.3389/fphys.2019.00840
This article is part of the Research Topic Welfare and Stressors in Fish: Challenges Facing Aquaculture View all 15 articles
Two different O2 levels (normoxia: 75–85% O2 saturation; moderate hypoxia: 42–43% O2 saturation) and stocking densities (LD: 9.5, and HD: 19 kg/m3) were assessed on gilthead sea bream (Sparus aurata) in a 3-week feeding trial. Reduced O2 availability had a negative impact on feed intake and growth rates, which was exacerbated by HD despite of the improvement in feed efficiency. Blood physiological hallmarks disclosed the enhancement in O2-carrying capacity in fish maintained under moderate hypoxia. This feature was related to a hypo-metabolic state to cope with a chronic and widespread environmental O2 reduction, which was accompanied by a differential regulation of circulating cortisol and growth hormone levels. Customized PCR-arrays were used for the simultaneous gene expression profiling of 34–44 selected stress and metabolic markers in liver, white skeletal muscle, heart, and blood cells. The number of differentially expressed genes ranged between 22 and 19 in liver, heart, and white skeletal muscle to 5 in total blood cells. Partial Least-Squares Discriminant Analysis (PLS-DA) explained [R2Y(cum)] and predicted [Q2Y(cum)] up to 95 and 65% of total variance, respectively. The first component (R2Y = 0.2889) gathered fish on the basis of O2 availability, and liver and cardiac genes on the category of energy sensing and oxidative metabolism (cs, hif-1α, pgc1α, pgc1β, sirts 1-2-4-5-6-7), antioxidant defense and tissue repair (prdx5, sod2, mortalin, gpx4, gr, grp-170, and prdx3) and oxidative phosphorylation (nd2, nd5, and coxi) highly contributed to this separation. The second component (R2Y = 0.2927) differentiated normoxic fish at different stocking densities, and the white muscle clearly promoted this separation by a high over-representation of genes related to GH/IGF system (ghr-i, igfbp6b, igfbp5b, insr, igfbp3, and igf-i). The third component (R2Y = 0.2542) discriminated the effect of stocking density in fish exposed to moderate hypoxia by means of hepatic fatty acid desaturases (fads2, scd1a, and scd1b) and muscle markers of fatty acid oxidation (cpt1a). All these findings disclose the different contribution of analyzed tissues (liver ≥ heart > muscle > blood) and specific genes to the hypoxic- and crowding stress-mediated responses. This study will contribute to better explain and understand the different stress resilience of farmed fish across individuals and species.
Several attempts have been made over the course of last years to monitor the ecological and physiological impacts of a reduced O2 availability in aquatic environments (Ekau et al., 2010; Richards, 2011; Zhu et al., 2013; Deutsch et al., 2015). The magnitude and orchestration of adaptive responses from a physiological point of view, including blood hematology and metabolic regulation, reflects the duration and intensity of hypoxic stimuli in different marine species (Martos-Sitcha et al., 2017; Cadiz et al., 2018), being defined the limiting O2 saturation (LOS) as the threshold level where regulatory mechanisms are no longer sufficient to maintain O2 consumption without compromising any physiological function (Remen et al., 2015, 2016). To minimize hypoxia impact, fish reduce feed intake and reorganize its metabolism to limit the tissue O2 demand (Hopkins and Powell, 2001; Bermejo-Nogales et al., 2014b). This allows to preserve aerobic metabolism by means of a restricted mitochondrial respiration and a shift in substrate preferences, as it has been reported in humans and rodents during the metabolic adaption of skeletal muscle to high altitude hypoxia (Murray, 2009). Other adaptive responses include changes in the production and scavenging of reactive oxygen species (ROS) (Lushchak and Bagnyukova, 2006; Bermejo-Nogales et al., 2014a), gill surface functionality (Nilsson, 2007) and hemoglobin (Hb)-O2 binding characteristics (Jensen and Weber, 1982; Nikinmaa, 2001). In most aquaculture scenarios, these adaptive features are commonly associated to increases in temperature and high stocking rearing densities (Person-Le Ruyet et al., 2008; Vikeså et al., 2017), which in turn can compromise water quality resulting in impaired fish growth and immunity (Pickering, 1993; Van Weerd and Komen, 1998; Montero et al., 1999; Ashley, 2007). Indeed, Arctic charr (Salvelinus alpinus) or meager (Argyrosomus regius) kept at high stocking densities evidenced a better growth performance than fish reared at low densities as long as water quality was preserved (Jørgensen et al., 1993; Millán-Cubillo et al., 2016). The impact of crowding stress can also be minimized when O2 levels are not below LOS (Ruer et al., 1987; Araújo-Luna et al., 2018). Unraveling the combined effects of hypoxia and high rearing density are, thereby, crucial to warrant welfare during intensive fish farming.
Progress toward a more sustainable and environmentally friendly aquaculture requires important investments in both conventional and new methodologies for a less invasive and more refined phenotyping of individual farmed fish. Main achievements so far include the use of acoustic telemetry or stand-alone biosensors for the non-disturbing monitoring of feeding behavior or metabolic capabilities (Føre et al., 2017; Martos-Sitcha et al., 2019). In addition to that, major progress has been done with the advent of wide-holistic omics based on functional genomics, proteomics, metabolomics and metagenomics as powerful toolsets for the development of a highly technified aquaculture in different fish species (Yáñez et al., 2015; Martin and Król, 2017; Martínez-Porchas and Vargas-Albores, 2017; Alfaro and Young, 2018; Rodrigues et al., 2018). Such approaches are increasingly used in gilthead sea bream (Sparus aurata), a highly and economically important cultured fish species in the Mediterranean area. Thus, a first draft genome based on genetic-linkage maps (Pauletto et al., 2018) and other current genome initiatives will contribute to have major progress in selective breeding and epigenetic research in gilthead sea bream. Also, in this species, important research efforts have been conducted to define a reference pattern for skin/intestine mucus proteome (Estensoro et al., 2016; Pérez-Sánchez et al., 2017), gut microbiota (Piazzon et al., 2017), or serum metabolome (Gil-Solsona et al., 2019). Moreover, the use of high-density microarrays (Calduch-Giner et al., 2010, 2012, 2014), pathway-focused PCR-arrays (Benedito-Palos et al., 2014, 2016; Bermejo-Nogales et al., 2014b, 2015; Pérez-Sánchez et al., 2015; Magnoni et al., 2017; Martos-Sitcha et al., 2017) and more recently NGS (Piazzon et al., 2019) have contributed to define tissue-specific gene expression patterns in response to nutritional, environmental and parasite challenges. Likewise, the synchronization of the molecular clock of sea bream larvae, involving more than 2,500 genes with a clear circadian rhythmicity, has been proposed as certification of juvenile quality later in life (Yúfera et al., 2017). In the present study, we aim to go further on the definition of criteria of fish welfare and quality, regarding in depth the effect of two different initial stocking densities (9.5 kg/m3, 19 kg/m3) and O2 saturation levels (85%, 42–43% O2 saturation) in a 3-week trial with fast growing juveniles of gilthead sea bream. The analyzed parameters included the gene expression pattern of a set of growth and metabolic markers of liver, skeletal muscle, heart and blood cells in combination with data on growth performance, as well as blood hematology and biochemistry. The working hypothesis is that each tissue contributes differentially to the homeostatic load achievement, helping the generated knowledge to better exploit the plasticity and resilience of gilthead sea bream under stressful conditions.
Gilthead sea bream juveniles of Atlantic origin (Ferme Marine du Douhet, Bordeaux, France) were reared from early life stages in the indoor experimental facilities of Institute of Aquaculture Torre de la Sal (IATS-CSIC, Spain) under natural photoperiod and temperature conditions (40°5′N; 0°10′E). Sea water was pumped ashore (open system) and filtered through a 10-μm filter. The O2 content of water effluents in standard conditions was always higher than 85% saturation, and unionized ammonia remained below 0.02 mg/L.
Juvenile fish (initial body weight 34.08 ± 0.31 g) were randomly distributed in 12 90 L tanks coupled to a recirculation system equipped with physical and biological filters, and programmable temperature and O2 devices (Supplementary Figure 1). Water temperature was daily monitored and maintained at 25-27°C. Fish were arbitrarily allocated to constitute two different initial stocking densities (six tanks per condition) fed once daily to visual satiety with a commercial diet (EFICO Forte 824, BioMar, Palencia, Spain): LD (low density, 25 fish/tank, 9.5 kg/m3) and HD (high density, 50 fish/tank, 19 kg/m3). Fish were allowed to acclimate to these conditions for 12 days before any manipulation. Fish behavior during acclimation was assessed by routine camera monitoring, and also by visual observation regarding to normal feeding performance. After the acclimation period, the water parameters of three tanks of each initial stocking density were kept unchanged, constituting the normoxic (>5.5 ppm O2; >85% O2 saturation) groups of each experimental condition (LDN, low density normoxia; HDN, high density normoxia). Fish maintained in the remaining six tanks experienced a gradual decrease in the water O2 level until reaching 3.0 ppm (42–43% O2 saturation), constituting the hypoxic groups of each experimental condition (LDH, low density hypoxia; HDH, high density hypoxia). The normal range of variation in O2 levels was marked by a rapid drop (3.8–4 ppm normoxic groups; 2.3 ppm hypoxic groups) 15–30 min after feeding, with a rapid restoration of reference values in less than 1 h by the automatic entrance of clean water from the main reservoir tank. This system allowed maintaining unionized ammonia below toxic levels (<0.50 mg/L) in both HDN and HDH groups.
After 22 days under these experimental conditions and following overnight fasting (>20 h after last meal), 12 fish (4 per tank) per experimental condition (LDN, LDH, HDN, and HDH) were anesthetized with 3-aminobenzoic acid ethyl ester (100 mg/L), weighed and blood was taken from caudal vessels with EDTA-treated syringes (less than 5 min for all the fish sampled for each tank). All lethal samples were collected between 10.00 am and 12.00 am to reduce the biologic variability due to circadian rhythms and postprandial-mediated effects, since temperatures used in the present experimental approach (25-27°C) ensured that digestion processes have been completed (Gómez-Requeni et al., 2003). One blood aliquot (25 μL) was directly collected into a microtube containing 500 μL of stabilizing lysis solution (REAL total RNA spin blood kit, Durviz, Valencia, Spain) and stored at -80°C until total RNA extraction. Other aliquots were processed for haematocrit (Ht), Hb, and red blood cells (RBC) counting. The remaining blood was centrifuged at 3,000 × g for 20 min at 4°C, and plasma samples were frozen and stored at -20°C until biochemical and hormonal analyses were performed. Prior to tissue collection, fish were killed by cervical section. Liver and viscera were weighed, and representative biopsies of liver, dorsal skeletal muscle, and complete hearts were immediately snap-frozen in liquid nitrogen and stored at -80°C until extraction of total RNA.
Measures of Ht were conducted using heparinized capillary tubes centrifuged at 1,500 × g for 30 min in a Sigma 1-14 centrifuge (Sigma, Germany). Hb was assessed using a Hemocue Hb 201+ (Hemocue, Sweden). Counts of RBC were made in a Neubauer chamber, using an isotonic solution (1% NaCl). Plasma glucose was analyzed using the glucose oxidase method (Thermo Electron, Louisville, CO, United States). Lactate was measured in deproteinized samples (perchloric acid 8%) by an enzymatic method based on the use of lactate oxidase and peroxidase (SPINREACT S.A., Girona, Spain). Total antioxidant capacity in plasma samples was measured with a commercial kit (Cayman Chemical, Ann Arbor, MI, United States) adapted to 96-well microplates. This assay relies on the ability of antioxidants in the samples to inhibit the oxidation of ABTS [2,2′-azino-di-(3-ethylbenzthiazoline sulphonate)] to ABTS radical cation by metmyoglobin, a derivatized form of myoglobin. The capacity of the sample to prevent ABTS oxidation is compared with that of Trolox (water-soluble tocopherol analog) and is quantified as mM Trolox equivalents. Plasma cortisol levels were analyzed using an EIA kit (Kit RE52061m IBL, International GmbH, Germany). The limit of detection of the assay was 3.01 ng/mL with intra- and inter-assay coefficients of variation lower than 3 and 5%, respectively. Plasma growth hormone (Gh) was determined by a homologous gilthead sea bream RIA as reported elsewhere (Martínez-Barberá et al., 1995). The sensitivity and midrange (ED50) of the assay where 0.15 and 1.8 ng/mL, respectively. Plasma insulin-like growth factors (Igf) were extracted by acid–ethanol cryoprecipitation (Shimizu et al., 2000), and the concentration of Igf-I was measured by means of a generic fish Igf-I RIA validated for Mediterranean perciform fish (Vega-Rubín de Celis et al., 2004). The sensitivity and midrange of the assay were 0.05 and 0.7–0.8 ng/mL, respectively.
Total RNA from liver, white muscle and heart was extracted using a MagMax-96 total RNA isolation kit (Life Technologies, Carlsbad, CA, United States), whereas total RNA from total blood cells was extracted using the REAL total RNA spin blood kit including a DNase step. The RNA yield in all tissues was >3.5 μg, with absorbance measures (A260/280) of 1.9–2.1. Synthesis of cDNA was performed with the High-Capacity cDNA Archive Kit (Applied Biosystems, Foster City, CA, United States) using random decamers and 500 ng of total RNA in a final volume of 100 μL. Reverse transcription (RT) reactions were incubated 10 min at 25°C and 2 h at 37°C. Negative control reactions were run without RT.
The 96-well PCR-array layout was designed for the simultaneous profiling of a panel of 43 (liver), 44 (white muscle and total blood cells) or 34 (heart) genes, including markers of GH/IGF system (13), lipid metabolism (10), energy sensing and oxidative metabolism (12), antioxidant defense and tissue repair (10), muscle growth and cell differentiation (8), respiration uncoupling (3), xenobiotic metabolism (2), nuclear receptors (3), transmembrane translocation (8), mitochondrial dynamics and apoptosis (5), as well as OXPHOS (22) (Table 1). qPCR reactions were performed using an iCycler IQ Real-time Detection System (Bio-Rad, Hercules, CA, United States). Diluted RT reactions were conveniently used for qPCR assays in a 25 μL volume in combination with a SYBR Green Master Mix (Bio-Rad, Hercules, CA, United States) and specific primers at a final concentration of 0.9 μM (Supplementary Table 1). The program used for PCR amplification included an initial denaturation step at 95°C for 3 min, followed by 40 cycles of denaturation for 15 s at 95°C and annealing/extension for 60 s at 60°C. All the pipetting operations were made by means of an EpMotion 5070 Liquid Handling Robot (Eppendorf, Hamburg, Germany) to improve data reproducibility. The efficiency of PCRs (>92%) was checked, and the specificity of reactions was verified by analysis of melting curves (ramping rates of 0.5°C/10 s over a temperature range of 55–95°C) and linearity of serial dilutions of RT reactions (>0.99). Fluorescence data acquired during the extension phase were normalized by the delta-delta CT method (Livak and Schmittgen, 2001) using actb in the liver, white muscle and heart, or cox4a in total blood cells, as the housekeeping gene due to its stability among different experimental conditions (average CT varied less than 0.5 in each tissue). For multi-gene analysis, data on gene expression were in reference to the expression level of cs in the liver, igfr2 in the white muscle, gcr in the heart, and tim8a in total blood cells of LDN fish, for which a value of 1 was arbitrarily assigned (Supplementary Tables 2–5, respectively).
Table 1. Genes included in the liver (L), white muscle (M), heart (H), and total blood cells (B) pathway-focused PCR arrays.
This manuscript follows the ZFIN Zebrafish Nomenclature Guidelines for gene and protein names and symbols1.
Data on growth performance, blood biochemistry, and gene expression were analyzed by two-way analysis of variance (ANOVA) with O2 levels (normoxia and moderate hypoxia) and stocking conditions (low and high stocking densities) as main factors. These analyses were followed by the SNK post hoc test for comparisons among different groups. The significance level was set at P < 0.05. Analyses were performed using SigmaPlot v13 (Systat Software Inc., San Jose, CA, United States). Unsupervised multivariate analysis principal component analysis (PCA) was first performed on data as an unbiased method to observe trends within conditions at different experimental groups, using EZinfo v3.0 (Umetrics, Umeå, Sweden). To achieve the maximum separation among experimental groups, Partial Least-Squares Discriminant Analysis (PLS-DA) was sequentially applied with joint data from liver, heart and white muscle, excluding the results from total blood cells due to its low contribution to the total variance. The quality of the PLS-DA model was evaluated by R2Y(cum) and Q2Y(cum) parameters, which indicated the fitness and prediction ability, respectively. Additionally, 200 random permutation tests to avoid over-fitting of the supervised model were carried out by SIMCA-P+ v15.0 (Umetrics). Cross-validation analysis of variance (CV-ANOVA) was applied (p-value = 0.037). The contribution of differential genes along liver, white muscle and heart tissues was assessed by means of Variable Importance in Projection (VIP) measurements. A VIP score > 1.1 was considered to be an adequate threshold to determine discriminant variables in the PLS-DA model (Wold et al., 2001; Li et al., 2012; Kieffer et al., 2016).
Data on feed intake, growth and somatic indexes (hepatosomatic index, HSI; viscerosomatic index, VSI) are shown in Table 2. Fish reared under moderate hypoxia evidenced lower feed intake, which resulted in reduced weight gain and SGR. This also affected liver and viscera weight as well as HSI. This general impairment of feed intake and growth was further evidenced in fish kept at the highest density, though FE was improved in moderate hypoxia and more especially in fish kept at HD (HDH group).
Table 2. Effects of rearing density and dissolved oxygen level on gilthead sea bream growth performance on a 21-days feeding trial.
Data on blood hematology and biochemistry are shown in Table 3. The results show a significant effect of O2 level, with a generalized increase in Hb, Ht, RBC content, MCH, cortisol and Gh plasma levels, as well as a widespread decrease in MCHC, MCV and plasma lactate levels. Overall this feature was more pronounced in fish maintained under LD conditions. In contrast, the rearing density effect was mostly evidenced in plasma cortisol levels, which showed a pronounced rise in HD fish that was exacerbated by hypoxic conditions. Noticeably, significant O2 level and rearing density interactions were found for cortisol, but also for Ht, MCHC, MCH, and TAA.
Table 3. Effects of rearing density and dissolved oxygen level on blood hematology and plasma levels of metabolites, hormones, and total antioxidant capacity.
All genes selected for PCR-arrays were found at detectable levels in the four tissues analyzed. Results of gene expression profiling in hepatic selected genes are presented in Supplementary Table 2. Among them, 22 out of 43 genes were affected by at least one of the experimental factors or by its interaction (i.e., the combined effect of confinement stress and hypoxia exposure leading to expression changes that could not be attributed to a single parameter), being 11 differentially expressed (DE) in response to O2 level. Relative expression of markers from GH/IGF system (ghr-i), oxidative metabolism (nd2), and antioxidant defense and tissue repair (gpx4, prdx5) was significantly down-regulated by moderate hypoxia in LDH and HDH groups. In addition, several genes of lipid metabolism (elovl1, fads2, and scd1b) were up-regulated in the LD group, whereas markers of oxidative metabolism (nd5), and antioxidant defense and tissue repair (gr, sod2, and grp-75) were down-regulated in fish kept at HD conditions. Stocking density also affected 11 genes related with the GH/IGF system (ghr-i, ghr-ii, and igf-i), lipid metabolism (elovl6, fads2, scd1a, scd1b, and lpl), oxidative metabolism (ucp1, pgc1α) and antioxidant defense and tissue repair (grp-75). A statistically significant interaction of O2 levels and rearing density was found for igf-ii, fads2, scd1a, scd1b, pgc1ß, gr, prdx3, and grp-170 genes.
In white skeletal muscle, 20 out of 44 DE genes were affected at least by one of the experimental condition or by their interaction (Supplementary Table 3). Markers of the GH/IGF system were mostly affected by stocking density (ghr-i, igf-ii, igfbp3, igfbp5b, igfbp6b, insr, and igfr1) rather than by O2 levels (igfr2). Moderate hypoxia up-regulated myod2 expression as the sole effect on genes related to muscle growth and cell differentiation. In contrast, many genes related to energy sensing, oxidative metabolism, and antioxidant defense and tissue repair were down-regulated by low O2 levels (sirt1, ucp3, hif-1α, prdx5, and sod2) or up-regulated in HD conditions (sirt4, sirt7, coxi, hif-1α, and gpx4). Additionally, a significant interaction between O2 levels and rearing density is reported for cpt1a and grp-170.
In heart, changes in O2 saturation and stocking density triggered significant differences in 19 out of 34 genes presented in the array (Supplementary Table 4). Up to 13 genes, including markers of the GH/IGF system (ghr-i), energy sensing and oxidative metabolism (sirt1, sirt5, sirt6, sirt7, cs, nd5, pgc1α, pgc1ß, and hif-1α) and antioxidant defense and tissue repair (cat, prdx5, and sod2) were down-regulated under moderate hypoxia, especially in HD conditions. The xenobiotic metabolism marker cyp1a1 was up-regulated by hypoxia in both LD and HD. Stocking density also down-regulated the expression of several genes involved in energy sensing and oxidative metabolism (sirt3, sirt5, cs, and nd2) as well as antioxidant defense and tissue repair (gr, prdx3, prdx5, grp-170, and grp-75), preferentially under low O2 levels.
In total blood cells, only 5 out of 44 genes were DE mainly by the interaction among different experimental conditions (Supplementary Table 5), being responsive to the stress challenge enzyme subunits of Complex I (ndufaf2) and Complex IV (coxi, coxii, cox6a2, and cox15) of the mitochondrial respiratory chain.
In order to assess the differential contribution of the DE genes in the physiological response to moderate hypoxia and rearing density, the tissue (liver, white skeletal muscle, and heart) gene expression dataset was analyzed by PLS-DA. The discriminant model was based on six components, which explained [R2Y(cum)] 95% and predicted [Q2Y(cum)] 65% of total variance (Figure 1A). The validity of the PLS-DA model was validated using a permutation test (Supplementary Figure 2). The first three components showed cumulative values for R2Y and Q2Y of 0.836 and 0.493, respectively. A clear separation between normoxic (LDN, HDN) and hypoxic (LDH, HDH) groups was observed along the first component (R2Y = 0.2889) (Figures 1B,C). Component 2 (R2Y = 0.2927) clearly separated LDN and HDN normoxic groups (Figure 1B), whereas component 3 (R2Y = 0.2542) discriminated LDH and HDH hypoxic groups (Figure 1C).
Figure 1. (A) Graphical representation of the goodness-of-fit of the PLS-DA model. (B) Two-dimensional PLS-DA score plot representing the distribution of the samples between the first two components in the model. (C) Two-dimensional PLS-DA score plot representing the distribution of the samples between the first and third components in the model. R2(cum), explained variance; Q2(cum), predicted variance; LDN, low density normoxia; LDH, low density hypoxia; HDN, high density normoxia; HDH, high density hypoxia.
Genes with a contribution to VIP > 1.1 in component 1 were a total of 39, with a main contribution of heart (19) and liver (14) genes involved in energy sensing and oxidative metabolism (14), antioxidant defense and tissue repair (12) and OXPHOS (Figure 2). When the second component was also considered, a total of 44 genes presented VIP values > 1.1 (Figure 3), and 11 out of the 21 new genes (highlighted in yellow) were from white skeletal muscle. Energy sensing and oxidative metabolism (12), antioxidant defense and tissue repair (11), GH/IGF system (11) and OXPHOS (6) were the main categories. Considering the VIP values from the three main components (Figure 4), most of the genes due to component three contribution (highlighted in purple) were related to lipid metabolism.
Figure 2. (A) Graphical representation of the variable importance (VIP) scores after component 1. (B) Ranking list of genes showing VIP score values above 1.1 and their relative gene expression. Liv, liver; WM, white muscle. Values on relative expression are the mean ± SEM of eight fish (2–3 fish per replicate tank). P-values are the result of two-way analysis of variance. Asterisks in each row indicate significant differences with oxygen level for a given rearing density (SNK test, P < 0.05).
Figure 3. (A) Graphical representation of the variable importance (VIP) scores after component 2. (B) Ranking list of genes showing VIP score values above 1.1 and their relative gene expression. Cells shaded in blue highlight genes detected as VIP after component 1; cells shaded in yellow highlight genes detected as VIP after component 2. For further details, see legend on Figure 2.
Figure 4. (A) Graphical representation of the variable importance (VIP) scores after component 3. (B) Ranking list of genes showing VIP score values above 1.1 and their relative gene expression. Cells shaded in blue highlight genes detected as VIP after component 1; cells shaded in yellow highlight genes detected as VIP after component 2; and cells shaded in purple highlight genes detected as VIP after component 3. For further details, see legend on Figure 2.
Hypoxia in aquatic habitats is a common disturbance that is predicted to occur in the future more extensively, more frequently and for longer periods of time (Intergovernmental Panel on Climate Change, 2014), becoming a major aquaculture stressor around the world. This is especially true in the case of intensive fish farming, and unraveling the adaptive hypoxic responses helps to better understand the nature of metabolic disturbances after short- and long-term exposures to challenging O2 levels. Blood physiological landmarks remain mostly unaltered in juveniles of gilthead sea bream exposed over 24 h to moderate hypoxia (40% O2 saturation), whereas changes in Ht and circulating levels of Hb, glucose and lactate are reported few hours after acute hypoxia (20% O2 saturation) (Martos-Sitcha et al., 2017). In the same study, gene expression profiling of total blood cells evidenced a consistent transcriptional response after strong hypoxic challenges, which serve to ensure a reduced but more efficient aerobic ATP production during severe hypoxia. Herein, the combined effects of moderate hypoxia (42–43% O2 saturation) and rearing density (initial density 19 kg/m3, leading up to 30 kg/m3 at the end of experiment) in a 3-week trial highlighted reduced growth and a different contribution of target tissues to the homeostatic load in challenged fish. As discussed below, the ultimate mechanisms for this adaptive stress response remain far to be established, though probably they have a major impact in mitochondrial respiration uncoupling, which varies across life, tissues, individuals and species (Rolfe and Brand, 1996; Hulbert et al., 2002, 2006). Indeed, improved energy efficiency and reduced mitochondrial respiration uncoupling becomes a priority with low food availability (Auer et al., 2015), and the expression of mitochondrial uncoupling proteins (UCP2/UCP3) is differentially regulated by feed restriction in glycolytic (white skeletal muscle) and highly oxidative (heart and skeletal red muscle) tissues of gilthead sea bream (Bermejo-Nogales et al., 2014a).
Growth impairments due to long-term hypoxia exposure have been noticed in a wide-range of farmed fish, including turbot (Scophthalmus maximus), European sea bass (Dicentrarchus labrax), and Atlantic salmon (Salmo salar) (Pichavant et al., 2001; Remen et al., 2016; Cadiz et al., 2017; Vikeså et al., 2017). As reported herein in gilthead sea bream, a primary response is the inhibition of feed intake which would favor a hypo-metabolic state with a reduced ROS production and risk of oxidative stress. This is supported by lowered plasma levels of lactate, which would reflect in hypoxic fish, and in a lower extent in HDN, a low basal metabolism rather than a shift of aerobic to anaerobic metabolism. This metabolic re-adjustment has also been reported in gilthead sea bream juveniles facing multiple sensorial stressors in a model of chronic stress that mimic daily aquaculture operations (Bermejo-Nogales et al., 2014b). Thus, according with the oxystatic theory (Dam and Pauly, 1995; Saravanan et al., 2012), fish finely adjust feed intake and basal metabolism to available O2, prioritizing feed efficiency at the expenses of maximum growth under restricted mitochondrial respiration. As a prove of this, the best FE and hormonal signatures for fast and efficient growth generally occurs before the achievement of maximum growth at the greater ration size (Brett, 1979; Pérez-Sánchez et al., 1995), pointing out a high metabolic plasticity in this euryhaline, eurytherm and euryoxic fish species due to a permissive regulation of feed intake which allows to cope an efficient energy metabolism at slow growth rates. This also applies at the cellular level, where the maximum ATP yield per molecule of O2 (P/O ratio) is highly dependent on ration size, as evidenced the increased P/O ratio of king penguins during periods of food shortage (Monternier et al., 2014) or liver mitochondria of brown trout (Salmo trutta) starved for 2 weeks (Salin et al., 2016).
Most of the hypoxia-mediated effects are accompanied by an enhanced O2-carrying capacity denoted by a swelling, formation and/or release of new erythrocytes together with plasma volume reduction (Gallaugher and Farrell, 1998). A set of different mechanisms operate this complex response in fish. It must be considered that fish RBC are nucleated and have the ability to produce Hb during most of their life span (Speckner et al., 1989). As a general response to stress, erythrocytes from fish spleen reservoirs can be released into the blood (Pearson and Stevens, 1991). In the other hand, the affinity of fish Hb to O2 can be modulated by allosteric regulation (variations in phosphate levels) (Val, 2000). This ability to increase blood O2 affinity as a response to hypoxia is present even in fish living in well-oxygenated environments, as it is the case of the Antarctic fish bald rockcod (Pathogenia borchgrevinki) (Wells et al., 1989), a finding that highlights the general phenotypic plasticity of fish. Hypoxia studies on rainbow trout (Oncorhynchus mykiss) showed an initial increase in Hb concentration mediated by the release of spleen erythrocytes, but under persistent hypoxia conditions the increase of the O2-carrying capacity arose from synthesis of new erythrocytes (Lai et al., 2006). These results suggest a complex and dynamic adaptation of fish to hypoxic conditions, a feature that could be species-specific. From our hematological data in the present work, hypoxia induced a slight increase of Hb content at both rearing densities, although the most evident and significant effects were the increase of the measured Ht, RBC count and corpuscular concentrations of Hb, which were secondly affected by rearing density. A similar enhancement of O2-carrying capacity by means of Ht increase was observed not only in previous short-term acute hypoxia challenges in gilthead sea bream (Magnoni et al., 2017; Martos-Sitcha et al., 2017), but also in eels and rainbow trout (Wood and Johansen, 1972; Soivio et al., 1980). Conversely, changes in HSI, reflecting the amount of lipid and glycogen depots, are more informative of feed intake rather that hypoxic condition, though it is difficult to disclose the main factor. At the hormonal level, this is also inferred from the measurements of circulating levels of cortisol and Gh, which are well-known regulators of metabolic rates by their involvement on mitochondria function (see Mommsen et al., 1999; Bergan-Roller and Sheridan, 2018 for review).
In fact, cortisol is an established marker of crowding stress in gilthead sea bream (Arends et al., 1999; Skrzynska et al., 2018), as well as under other challenging conditions, and the reduced feed intake as a consequence of the stress challenge also enhanced the responsiveness of the hypothalamic-pituitary-adrenal axis (Sangiao-Alvarellos et al., 2005). Thus, cortisol is the main corticosteroid in teleost fish and its plasma levels can increase dramatically during unfavorable situations activating specific intracellular responses through glucocorticoid receptors present in many fish organs, which in turn alleviate the great energy demand in a systemic way by increasing blood metabolite concentrations and redistributing the energy balance in the organism (reviewed by Mosconi et al., 2006; Wendelaar Bonga, 2011). In fish, high plasma cortisol levels modulate the metabolism of carbohydrates by stimulating gluconeogenesis in liver, and also increase the availability of substrates derived from proteins and fats, although the role of cortisol in fish lipid metabolism has not been clearly established (Vijayan et al., 2010). All this agrees with the observation that the greater circulating concentration of cortisol was achieved herein in the HDH group, which also experienced a higher feed intake inhibition. However, this system cannot be continuously refed, and evidence in rodents points out toward a translocation of cortisol into mitochondria mediated by glucocorticoid receptors to reduce mitochondrial activity and the risk of oxidative stress (Du et al., 2009), a mechanism that could be possibly extended to other animal models including fish, although this fact has still not been documented. Thus, in the absence of a cortisol response, chronic cold-thermal stress up-regulates OXPHOS in gilthead sea bream, whereas the cortisol rise in fish facing multiple aquaculture sensorial stressors is accompanied by a pronounced transcriptional repression of all the hepatic complex units of the mitochondrial respiratory chain (Bermejo-Nogales et al., 2014b). A similar response has been reported after acute hypoxia exposure, though in this case the catalytic and regulatory enzyme subunits of Complex IV (the last electron acceptor of respiratory chain involved in the O2 reduction) were up-regulated, maximizing the use of available O2 for aerobic ATP generation (Martos-Sitcha et al., 2017). The aerobic scope and gene expression profiling of mitochondria is also highly regulated at the nutritional level by synthetic and natural dietary oils (Pérez-Sánchez et al., 2013; Martos-Sitcha et al., 2018), and the suppression of heptanoate effects upon exercise endurance is viewed as a protective measure to counteract disproportionate oxidative metabolic rates in fish fed fast energy-delivery nutrients (short/medium chain fatty acids). In other words, the interplay between stimulatory and inhibitory effects must be envisaged as a response to the energy needs, even if metabolic fuels were available. Accordingly, in the present study, the increased circulating levels of Gh in hypoxic/crowded fish will reflect a reduced feed intake and energy demand rather than a minor capacity to combat oxidative stress, as it is generally referenced in fish and other animal models overexpressing GH (Brown-Borg et al., 1999; Brown-Borg and Rakoczy, 2000; McKenzie et al., 2003; Almeida et al., 2013).
The gene expression profiling of key metabolic biomarkers also contributes to better understand the search of allostatic load in a challenging environment. Thus, the two-way ANOVA revealed the different involvement of tissues and gene categories into the stress-mediated responses. This observation is reinforced by the use of multivariate analysis, which offers the possibility to identify, at a high level of confidence, the most responsive tissues and biomarkers for a given stress stimuli in a factorial stress design. Using such approach, we are able to explain and to predict a high percentage of total variance, being noteworthy that liver, white skeletal muscle and heart remained responsive at long-term to changing O2 and rearing density, whereas the expression pattern of blood cells became mostly unaltered with the imposed stress stimuli of medium intensity, in contrast with the previously reported wide expression change of mitochondrial-related genes in total blood cells in response to a more severe challenge (Martos-Sitcha et al., 2017). For this reason, the transcriptomic analysis of total blood cells during moderate hypoxia challenges was not included in our PLS-DA model to avoid the background noise detected when introduced. In previous studies in gilthead sea bream and other animal models, liver and cardiac muscle are highly responsive to hypoxia (Everett et al., 2012; Hermes-Lima et al., 2015; Magnoni et al., 2017), and genes of these two tissues highly contributed herein to separate normoxic and hypoxic fish along the first component of our PLS-DA (R2Y = 0.2889). One of the most relevant genes participating in this discriminant feature is the hif-1α, a well-documented regulator of O2 homeostasis. This transcriptional factor acts at a multi-regulatory level, managing the hypoxic responsiveness of a vast array of transcribed proteins including antioxidant enzymes (Nikinmaa and Rees, 2005; Lushchak and Bagnyukova, 2006). Concretely, herein, we show a clear down-regulation of hif-1α that was coincident with the repressed expression of other down-stream markers of antioxidant defense and tissue repair (prdx5, sod2, mortalin, gpx4, gr, grp-170, and prdx3). This intriguing result can be cautiously interpreted since Hif-1 is mostly regulated at the post-translational level (Ke and Costa, 2006), though this finding should be understood as a steady-state in which O2 availability is reduced but maintained high enough to preserve aerobic metabolism at a relatively high level. This fact is supported by a reduced expression of cs and associated enzyme subunits of Complex I (nd2, nd5), used successfully in several studies as markers of mitochondria abundance and Krebs cycle activity (Larsen et al., 2012; Magnoni et al., 2017). In addition to that, several sirts (sirt1, 2, 5, 6, and 7) of liver or cardiac muscle were overall down-regulated in hypoxic fish, especially in the case of HDH fish. These NAD+-dependent deacetylases are energy sensors that act in gilthead sea bream as a link between nutrition and energy metabolism in different growth models with nutrients and genetic variables as source of variation (Simó-Mirabet et al., 2017a,b, 2018). This was extended herein to hypoxia/crowding stress, which indicates that most of the envisaged adaptive responses should include changes in the acetylation status of both nuclear histones, and cytoplasmic and mitochondrial metabolic enzymes.
The second component of our PLS-DA (R2Y = 0.2927) differentiates normoxic fish held at different stocking densities. In this case, the white skeletal muscle clearly promotes this separation mainly by the expression pattern of genes related to GH/IGF system (ghr-i, igfbp6b, igfbp5b, insr, igfbp3, igf-i). Components of liver and muscle GH/IGF system are differentially regulated by nutrients and seasonal environmental cues (reviewed by Pérez-Sánchez et al., 2018), but herein this observation becomes specially relevant for muscle ghr-i that highly contributes to discriminate the detrimental growth effects of crowding stress from those more related to hypoxia or water quality. Likewise, genes of igfbp repertoire highly contribute to this differentiation, though the discriminant role of Igfbp counterparts (igfbp6b > igfpb5b > igfpb3) was mostly reduced to skeletal muscle and Igfbp3/5/6 clade. Functional divergence regarding the growth-inhibitory or growth-promoting action of igfbps have been reported across species and physiological context (Garcia de la Serrana and Macqueen, 2018), but herein the overall depressed expression of the muscle Igfbp clade in HD fish is consistent with inhibitory rather than stimulatory growth-promoting effects, which also involves the regulation of insulin and Igfbp receptors with important implications on the final arrangements of carbohydrate, growth, and energy metabolism (reviewed by Reindl and Sheridan, 2012; Vélez et al., 2017). Indeed, fish are the first group in the vertebrate tree in which there is evidence of distinct insulin and Igf receptors, though certain cross-reactivity between ligand and receptors of insulin and Ifgs occurs and the specific-mediated effects are sometimes confounding. However, it is well-recognized that insulin stimulates Hif-1, whereas intermittent hypoxia induces insulin resistance in mice (Treins et al., 2002; Poulain et al., 2017). Likewise, Igfbp1 knock-down alleviates the hypoxia-induced growth retardation in zebrafish (Kajimura et al., 2005), whereas the IGFBP4 expression is induced by hypoxia in U87 glioma cells (Minchenko et al., 2016). From our results it is also conclusive that the muscle expression of igfr1 and igfr2 are specially responsive to hypoxia, but importantly insr in gilthead sea bream seems to be more receptive to crowding stress rather than hypoxic stress stimuli, though it remains to be established the functional relevance of this differential responsiveness to environmental stressors.
Finally, the third component of our multivariate approach (R2Y = 0.2542) discriminates the effect of stocking density in fish exposed to moderate hypoxia, with a marked contribution of hepatic fatty desaturases with Δ6 (fads2) or Δ9 (scd1a, scd1b) activities due to its strong and specific induction in LDH fish. A muscle marker of FA oxidation (cpt1a) was also consistently up-regulated in this group, but this response was opposite to that found in HDH group, which is indicative of the different regulation of muscle lipid catabolism by hypoxia in fish stocked at standard or high densities. Likewise, the major discriminant capacity of other factors related to lipid metabolism (elovl6) was achieved between normoxic fish held at LD and HD. Meanwhile, other elongases (elovl5) with a well-recognized role in the control of hepatic triglyceride storage did not take part of the group separation in the present study, though elovl5 highly contributes to differentiate two gilthead sea bream strains with differences in growth performance and metabolic capacities (Simó-Mirabet et al., 2018). Previous studies, in gilthead sea bream (Benedito-Palos et al., 2013, 2014) and European sea bass (Rimoldi et al., 2016) have also evidenced an important effect of ration size on the hepatic and muscle regulation of most of the lipid biomarkers assessed in the present study, but again it is difficult to disclose what is the main factor (feed intake or the imposed stress condition) due to the logistic limitations of our experiment design that did not include pair-fed groups. However, as a general rule, stressors enhance the demand of specific nutrients and hypoxia in particular promote the cellular uptake of extracellular unsaturated fatty acids in mice cell lines (Ackerman et al., 2018). Moreover, in hypoxic stress, cancer cells enhance lipid synthesis that is important for membrane biosynthesis and energy storage for cell survival and proliferation (Huang et al., 2014), being induced this hypoxia lipogenic phenotype via dependent- and HIF1α-independent pathways (Valli et al., 2015). All this together supports the pronounced stimulation of fads2 and scd desaturases in our stress model, which will promote the increase of the unsaturation index of structural lipids as previously reported during feed restriction in gilthead sea bream (Benedito-Palos et al., 2013). In agreement with this, hypoxia stress on HeLa cells leads to significant changes in their membrane lipid profiles, and polyunsaturated phospholipid species are becoming stronger biomarkers for discriminating the effect of hypoxia treatment on membrane fluidity and further membrane-dependent functions (Yu et al., 2014).
A growing effort is devoted in fish to define a “stressome,” or a catalog of genes expressed when an organism is challenged with a given stress, particularly those that comprise a common response to diverse stressful scenarios (as reviewed in Balasch and Tort, 2019). Our work follows a similar approach in order to determine not only the most consistent and reliable biomarkers for welfare assessment, but also the most (or least) convenient tissues for these analyses.
The findings described herein evidence the re-adjustment of several biological functions in a factorial model of chronic stress, where most of the hypoxia-mediated effects on growth performance and energy metabolism were exacerbated in fish held at HD. The integrated data on blood hematology, biochemistry and hormonal profiling highlights a hypo-metabolic state with the enhancement of O2-carrying capacity, being this metabolic feature accompanied by a reduction in voluntary feed intake and a more efficient energy metabolism at the expenses of slow growth rates. This notion was supported at the transcriptional level by global changes of tissue-gene expression profiles, which also evidenced tissue-specific orchestration of stress response reflecting the nature and intensity of stress stimuli, but also the different metabolic capacities of targeted tissues. Thus, the number of DE in response to a given stress stimuli varies across the targeted tissues (liver ≥ heart > muscle > blood), but importantly PLS-DA analysis also informs of the different tissue contribution to the allostatic load. Thus, liver and heart mostly contribute to cope with a global hypoxic response involving changes in energy sensing and production as well as antioxidant defense and tissue repair. In contrast, metabolic markers of skeletal muscle with a high over-representation of GH/IGF system mostly contribute to disclose the effects of rearing density not necessarily mediated by low O2 levels. Likewise, lipid metabolism and hepatic fatty acid desaturases are becoming strong biomarkers of crowding stress in hypoxic fish, which reveals the complexity and metabolic plasticity of gilthead sea bream to cope with stress resilience under intensive fish farming. These results evidence the potential of the identified biomarkers for a reliable assessment of fish welfare, although for some tissues such as blood cells, responsiveness is highly dependent on the intensity of the challenge. Overall, this new knowledge will contribute to better explain and understand the different stress resilience of farmed fish across individuals and species.
All procedures decribed here were approved by the Ethics and Animal Welfare Committee of Institute of Aquaculture Torre de la Sal and carried out according to national (Royal Decree RD53/2013), and the EU legislation (2010/63/EU) on the handling of animals for experiments.
JM-S, JC-G, and JP-S conceived and designed the study. JM-S, PS-M, and VdlH carried out the experimental procedures. JM-S and JP-S wrote the original draft. All authors analyzed and interpreted the data, reviewed, edited, and approved the final manuscript.
This work has been carried out with financial support from the European Commission of the European Union, under the Horizon 2020 research infrastructure project AQUAEXCEL2020 (652831) to JP-S. This publication reflects the views only of the authors, and the European Commission cannot be held responsible for any use which may be made of the information contained therein. JM-S was funded by a Postdoctoral Research Fellowship (Juan de la Cierva-Formación, Reference FJCI-2014-20161) and belongs to the Fish Welfare and Stress Network (AGL2016-81808-REDT) from the MINECO.
The authors declare that the research was conducted in the absence of any commercial or financial relationships that could be construed as a potential conflict of interest.
The authors are grateful to M. A. González for her excellent technical assistance in samplings and PCR analyses.
The Supplementary Material for this article can be found online at: https://www.frontiersin.org/articles/10.3389/fphys.2019.00840/full#supplementary-material
Ackerman, D., Tumanov, S., Qiu, B., Michalopoulou, E., Spata, M., Azzam, A., et al. (2018). Triglycerides promote lipid homeostasis during hypoxic stress by balancing fatty acid saturation. Cell Rep. 24, 2596–2605. doi: 10.1016/j.celrep.2018.08.015
Alfaro, A. C., and Young, T. (2018). Showcasing metabolomics research in aquaculture: a review. Rev. Aquac. 10, 135–152. doi: 10.1111/raq.12152
Almeida, D. V., Bianchini, A., and Marins, L. F. (2013). Growth hormone overexpression generates an unfavorable phenotype in juvenile transgenic zebrafish under hypoxic conditions. Gen. Comp. Endocrinol. 194, 102–109. doi: 10.1016/j.ygcen.2013.08.017
Araújo-Luna, R., Ribeiro, L., Bergheim, A., and Pousão-Ferreira, P. (2018). The impact of different rearing condition on gilthead seabream welfare: dissolved oxygen levels and stocking densities. Aquacult. Res. 49, 3845–3855. doi: 10.1111/are.13851
Arends, R. J., Mancera, J. M., Munoz, J. L., Bonga, S. W., and Flik, G. (1999). The stress response of the gilthead sea bream (Sparus aurata L.) to air exposure and confinement. J. Endocrinol. 163, 149–157. doi: 10.1677/joe.0.1630149
Ashley, P. J. (2007). Fish welfare: current issues in aquaculture. Appl. Anim. Behav. Sci. 104, 199–235. doi: 10.1016/j.applanim.2006.09.001
Auer, S. K., Salin, K., Rudolf, A. M., Anderson, G. J., and Metcalfe, N. B. (2015). The optimal combination of standard metabolic rate and aerobic scope for somatic growth depends on food availability. Funct. Ecol. 29, 479–486. doi: 10.1111/1365-2435.12396
Balasch, J. C., and Tort, L. (2019). Netting the stress responses in fish. Front. Endocrinol. 10:62. doi: 10.3389/fendo.2019.00062
Benedito-Palos, L., Ballester-Lozano, G., and Pérez-Sánchez, J. (2014). Wide-gene expression analysis of lipid-relevant genes in nutritionally challenged gilthead sea bream (Sparus aurata). Gene 547, 34–42. doi: 10.1016/j.gene.2014.05.073
Benedito-Palos, L., Ballester-Lozano, G. F., Simó, P., Karalazos, V., Ortiz, A., Calduch-Giner, J. A., et al. (2016). Lasting effects of butyrate and low FM/FO diets on growth performance, blood haematology/biochemistry and molecular growth-related markers in gilthead sea bream (Sparus aurata). Aquaculture 454, 8–18. doi: 10.1016/j.aquaculture.2015.12.008
Benedito-Palos, L., Calduch-Giner, J. A., Ballester-Lozano, G. F., and Pérez-Sánchez, J. (2013). Effect of ration size on fillet fatty acid composition, phospholipid allostasis and mRNA expression patterns of lipid regulatory genes in gilthead sea bream (Sparus aurata). Br. J. Nutr. 109, 1175–1187. doi: 10.1017/S000711451200311X
Bergan-Roller, H. E., and Sheridan, M. A. (2018). The growth hormone signaling system: insights into coordinating the anabolic and catabolic actions of growth hormone. Gen. Comp. Endocrinol. 258, 119–133. doi: 10.1016/j.ygcen.2017.07.028
Bermejo-Nogales, A., Calduch-Giner, J. A., and Pérez-Sánchez, J. (2015). Unraveling the molecular signatures of oxidative phosphorylation to cope with the nutritionally changing metabolic capabilities of liver and muscle tissues in farmed fish. PLoS One 10:e0122889. doi: 10.1371/journal.pone.0122889
Bermejo-Nogales, A., Nederlof, M., Benedito-Palos, L., Ballester-Lozano, G. F., Folkedal, O., Olsen, R. E., et al. (2014b). Metabolic and transcriptional responses of gilthead sea bream (Sparus aurata L.) to environmental stress: new insights in fish mitochondrial phenotypingGen. Comp. Endocrinol. 205, 305–315. doi: 10.1016/j.ygcen.2014.04.016
Bermejo-Nogales, A., Calduch-Giner, J. A., and Pérez-Sánchez, J. (2014a). Tissue-specific gene expression and functional regulation of uncoupling protein 2 (UCP2) by hypoxia and nutrient availability in gilthead sea bream (Sparus aurata): implications on the physiological significance of UCP1-3 variants. Fish Physiol. Biochem. 40, 751–762. doi: 10.1007/s10695-013-9882-7
Brett, J. R. (1979). “Environmental factors and growth,” in Fish Physiology, Vol. VIII, eds W. S. Hoar, D. J. Randall, and J. R. Brett (London: Academic Press), 599–675. doi: 10.1016/s1546-5098(08)60033-3
Brown-Borg, H. M., Bode, A. M., and Bartke, A. (1999). Antioxidative mechanisms and plasma growth hormone levels. Endocrine 11, 41–48. doi: 10.1385/ENDO:11:1:41
Brown-Borg, H. M., and Rakoczy, S. G. (2000). Catalase expression in delayed and premature aging mouse models. Exp. Gerontol. 35, 199–212. doi: 10.1016/S0531-5565(00)00079-6
Cadiz, L., Servili, A., Quazuguel, P., Madec, L., Zambonino-Infante, J. L., and Mazurais, D. (2017). Early exposure to chronic hypoxia induces short-and long-term regulation of hemoglobin gene expression in European sea bass (Dicentrarchus labrax). J. Exp. Biol. 220, 3119–3126. doi: 10.1242/jeb.160713
Cadiz, L., Zambonino-Infante, J.-L., Quazuguel, P., Madec, L., Le Delliou, H., and Mazurais, D. (2018). Metabolic response to hypoxia in European sea bass (Dicentrarchus labrax) displays developmental plasticity. Comp. Biochem. Physiol. 215, 1–9. doi: 10.1016/j.cbpb.2017.09.005
Calduch-Giner, J. A., Davey, G., Saera-Vila, A., Houeix, B., Talbot, A., Prunet, P., et al. (2010). Use of microarray technology to assess the time course of liver stress response after confinement exposure in gilthead sea bream (Sparus aurata L.). BMC Genomics 11:193. doi: 10.1186/1471-2164-11-193
Calduch-Giner, J. A., Echasseriau, Y., Crespo, D., Baron, D., Planas, J. V., Prunet, P., et al. (2014). Transcriptional assessment by microarray analysis and large-scale meta-analysis of the metabolic capacity of cardiac and skeletal muscle tissues to cope with reduced nutrient availability in gilthead sea bream (Sparus aurata L.). Mar. Biotechnol. 16, 423–435. doi: 10.1007/s10126-014-9562-3
Calduch-Giner, J. A., Sitjà-Bobadilla, A., Davey, G. C., Cairns, M. T., Kaushik, S., and Pérez-Sánchez, J. (2012). Dietary vegetable oils do not alter the intestine transcriptome of gilthead sea bream (Sparus aurata), but modulate the transcriptomic response to infection with Enteromyxum leei. BMC Genomics 13:470. doi: 10.1186/1471-2164-13-470
Dam, A. V., and Pauly, D. (1995). Simulation of the effects of oxygen on food consumption and growth of Nile tilapia, Oreochromis niloticus (L.). Aquacult. Res. 26, 427–440. doi: 10.1111/j.1365-2109.1995.tb00932.x
Deutsch, C., Ferrel, A., Seibel, B., Pörtner, H.-O., and Huey, R. (2015). Climate change tightens a metabolic constraint on marine habitats. Science 384, 1132–1135. doi: 10.1126/science.aaa1605
Du, J., Wang, Y., Hunter, R., Wei, Y., Blumenthal, R., Falke, C., et al. (2009). Dynamic regulation of mitochondrial function by glucocorticoids. Proc. Natl. Acad. Sci. U.S.A. 106, 3543–3548. doi: 10.1073/pnas.0812671106
Ekau, W., Auel, H., Pörtner, H. O., and Gilbert, D. (2010). Impacts of hypoxia on the structure and processes in pelagic communities (zooplankton, macro-invertebrates and fish). Biogeosciences 7, 1669–1699. doi: 10.5194/bg-7-1669-2010
Estensoro, I., Ballester-Lozano, G. F., Benedito-Palos, L., Grammes, F., Martos-Sitcha, J. A., Mydland, L.-T., et al. (2016). Dietary butyrate helps to restore the intestinal status of a marine teleost (Sparus aurata) fed extreme diets low in fish meal and fish oil. PLoS One 11:e0166564. doi: 10.1371/journal.pone.0166564
Everett, M. V., Antal, C. E., and Crawford, D. L. (2012). The effect of short-term hypoxic exposure on metabolic gene expression. J. Exp. Zool. A Ecol. Genet. Physiol. 317, 9–23. doi: 10.1002/jez.717
Føre, M., Frank, K., Norton, T., Svendsen, E., Alfredsen, J. A., Dempster, T., et al. (2017). Precision fish farming: a new framework to improve production in aquaculture. Biosyst. Eng. 173, 176–193. doi: 10.1016/j.biosystemseng.2017.10.014
Gallaugher, P., and Farrell, A. P. (1998). “Hematocrit and blood oxygen-carrying capacity,” in Fish Physiology, Vol. 17, eds S. F. Perry and B. L. Tufts (New York, NY: Academic Press), 185–227. doi: 10.1016/s1546-5098(08)60262-9
Garcia de la Serrana, D., and Macqueen, D. J. (2018). Insulin-like growth factor-binding proteins of teleost fishes. Front. Endocrinol. 9:80. doi: 10.3389/fendo.2018.00080
Gil-Solsona, R., Calduch-Giner, J. A., Nácher-Mestre, J., Lacalle-Bergeron, L., Sancho, J. V., Hernández, F., et al. (2019). Contributions of MS metabolomics to gilthead sea bream (Sparus aurata) nutrition. Serum fingerprinting of fish fed low fish meal and fish oil diets. Aquaculture 498, 503–512. doi: 10.1016/j.aquaculture.2018.08.080
Gómez-Requeni, P., Mingarro, M., Kirchner, S., Calduch-Giner, J., Medale, F., Corraze, G., et al. (2003). Effects of dietary amino acid profile on growth performance, key metabolic enzymes and somatotropic axis responsiveness of gilthead sea bream (Sparus aurata). Aquaculture 220, 749–763. doi: 10.1016/S0044-8486(02)00654-3
Hermes-Lima, M., Moreira, D. C., Rivera-Ingraham, G. A., Giraud-Billoud, M., Genaro-Mattos, T. C., and Campos, ÉG. (2015). Preparation for oxidative stress under hypoxia and metabolic depression: revisiting the proposal two decades later. Free Radic. Biol. Med. 89, 1122–1143. doi: 10.1016/j.freeradbiomed.2015.07.156
Hopkins, S. R., and Powell, F. L. (2001). “Common themes of adaptation to hypoxia,” in Hypoxia, Vol. 502, eds R. C. Roach, P. D. Wagner, and P. H. Hackett (Boston, MA: Springer), 153–167. doi: 10.1007/978-1-4757-3401-0_11
Huang, D., Li, C., and Zhang, H. (2014). Hypoxia and cancer cell metabolism. Acta Biochim. Biophys. Sin. 46, 214–219. doi: 10.1093/abbs/gmt148
Hulbert, A. J., Else, P. L., Manolis, S. C., and Brand, M. D. (2002). Proton leak in hepatocytes and liver mitochondria from archosaurs (crocodiles) and allometric relationships for ectotherms. J. Comp. Physiol. B 172, 387–397. doi: 10.1007/s00360-002-0264-1
Hulbert, A. J., Turner, N., Hinde, J., Else, P., and Guderley, H. (2006). How might you compare mitochondria from different tissues and different species? J. Comp. Biochem. B 176, 93–105. doi: 10.1007/s00360-005-0025-z
Intergovernmental Panel on Climate Change (2014). Climate change 2014-Impacts, Adaptation and Vulnerability: Part A: Global And Sectoral Aspects: Working group II contribution to the IPCC fifth assessment report. Cambridge: Cambridge University Press, doi: 10.1017/CBO9781107415379
Jensen, F. B., and Weber, R. E. (1982). Respiratory properties of tench blood and hemoglobin. Adaptation to hypoxic-hypercapnic water. Mol. Physiol. 2, 235–250.
Jørgensen, E. H., Christiansen, J. S., and Jobling, M. (1993). Effects of stocking density on food intake, growth performance and oxygen consumption in Arctic charr (Salvelinus alpinus). Aquaculture 110, 191–204. doi: 10.1016/0044-8486(93)90272-Z
Kajimura, S., Aida, K., and Duan, C. (2005). Insulin-like growth factor-binding protein-1 (IGFBP-1) mediates hypoxia-induced embryonic growth and developmental retardation. Proc. Natl. Acad. Sci. U.S.A. 102, 1240–1245. doi: 10.1073/pnas.0407443102
Ke, Q., and Costa, M. (2006). Hypoxia-inducible factor-1 (HIF-1). Mol. Pharmacol. 70, 1469–1480. doi: 10.1124/mol.106.027029
Kieffer, D. A., Piccolo, B. D., Vaziri, N. D., Liu, S., Lau, W. L., Khazaeli, M., et al. (2016). Resistant starch alters gut microbiome and metabolomic profiles concurrent with amelioration of chronic kidney disease in rats. Am. J. Physiol. Renal Physiol. 310, F857–F871. doi: 10.1152/ajprenal.00513.2015
Lai, J. C., Kakuta, I., Mok, H. O., Rummer, J. L., and Randall, D. (2006). Effects of moderate and substantial hypoxia on erythropoietin levels in rainbow trout kidney and spleen. J. Exp. Biol. 209, 2734–2738. doi: 10.1242/jeb.02279
Larsen, S., Nielsen, J., Hansen, C. N., Nielsen, L. B., Wibrand, F., Stride, N., et al. (2012). Biomarkers of mitochondrial content in skeletal muscle of healthy young human subjects. J. Physiol. 590, 3349–3360. doi: 10.1113/jphysiol.2012.230185
Li, H., Ma, M. L., Luo, S., Zhang, R. M., Han, P., and Hu, W. (2012). Metabolic responses to ethanol in Saccharomyces cerevisiae using a gas chromatography tandem mass spectrometry-based metabolomics approach. Int. J. Biochem. Cell Biol. 44, 1087–1096. doi: 10.1016/j.biocel.2012.03.017
Livak, K. J., and Schmittgen, T. D. (2001). Analysis of relative gene expression data using real-time quantitative PCR and the 2-ΔΔCT method. Methods 25, 402–408. doi: 10.1006/meth.2001.1262
Lushchak, V. I., and Bagnyukova, T. V. (2006). Effects of different environmental oxygen levels on free radical processes in fish. Comp. Biochem. Physiol. B Biochem. Mol. Biol. 144, 283–289. doi: 10.1016/j.cbpb.2006.02.014
Magnoni, L. J., Martos-Sitcha, J. A., Queiroz, A., Calduch-Giner, J. A., Magalhàes Gonçalves, J. F., Rocha, C. M. R., et al. (2017). Dietary supplementation of heat-treated Gracilaria and Ulva seaweeds enhanced acute hypoxia tolerance in gilthead seabream (Sparus aurata). Biol. Open 6, 897–908. doi: 10.1242/bio.024299
Martin, S. A. M., and Król, E. (2017). Nutrigenomics and immune function in fish: new insights from omics technologies. Dev. Comp. Immunol. 75, 86–98. doi: 10.1016/j.dci.2017.02.024
Martínez-Barberá, J. P., Pendón, C., Martí-Palanca, H., Calduch-Giner, J. A., Rodríguez, R. B., Valdivia, M. M., et al. (1995). The use of recombinant gilthead sea bream (Sparus aurata) growth hormone for radioiodination and standard preparation in radioimmunoassay. Comp. Biochem. Physiol. A Physiol. 110, 335–340. doi: 10.1016/0300-9629(94)00178-V
Martínez-Porchas, M., and Vargas-Albores, F. (2017). Microbial metagenomics in aquaculture: a potential tool for a deeper insight into the activity. Rev. Aquac. 9, 42–56. doi: 10.1111/raq.12102
Martos-Sitcha, J. A., Bermejo-Nogales, A., Calduch-Giner, J. A., and Pérez-Sánchez, J. (2017). Gene expression profiling of whole blood cells supports a more efficient mitochondrial respiration in hypoxia-challenged gilthead sea bream (Sparus aurata). Front. Zool. 14:34. doi: 10.1186/s12983-017-0220-2
Martos-Sitcha, J. A., Simó-Mirabet, P., Piazzon, M. C., de las Heras, V., Calduch-Giner, J. A., Puyalto, M., et al. (2018). Dietary sodium heptanoate helps to improve feed efficiency, growth hormone status and swimming performance in gilthead sea bream (Sparus aurata). Aquac. Nutr. 24, 1638–1651. doi: 10.1111/anu.12799
Martos-Sitcha, J. A., Sosa, J., Ramos-Valido, D., Bravo, F. J., Carmona-Duarte, C., Gomes, H. L., et al. (2019). Ultra-low power sensor devices for monitoring physical activity and respiratory frequency in farmed fish. Front. Physiol. 10:667. doi: 10.3389/fphys.2019.00667
McKenzie, D. J., Martinez, R., Morales, A., Acosta, J., Morales, R., Taylor, E. W., et al. (2003). Effects of growth hormone transgenesis on metabolic rate, exercise performance and hypoxia tolerance in tilapia hybrids. J. Fish Biol. 63, 398–409. doi: 10.1046/j.1095-8649.2003.00162.x
Millán-Cubillo, A. F., Martos-Sitcha, J. A., Ruiz-Jarabo, I., Cárdenas, S., and Mancera, J. M. (2016). Low stocking density negatively affects growth, metabolism and stress pathways in juvenile specimens of meagre (Argyrosomus regius, Asso 1801). Aquaculture 451, 87–92. doi: 10.1016/j.aquaculture.2015.08.034
Minchenko, D. O., Kharkova, A. P., Halkin, O. V., Karbovskyi, L. L., and Minchenko, O. H. (2016). Effect of hypoxia on the expression of genes encoding insulin-like growth factors and some related proteins in U87 glioma cells without IRE1 function. Endocr. Regul. 50, 43–54. doi: 10.1515/enr-2016-0008
Mommsen, T. P., Vijayan, M. M., and Moon, T. W. (1999). Cortisol in teleosts: dynamics, mechanisms of action, and metabolic regulation. Rev. Fish Biol. Fish. 9, 211–268. doi: 10.1023/A:1008924418720
Monternier, P. A., Marmillot, V., Rouanet, J. L., and Roussel, D. (2014). Mitochondrial phenotypic flexibility enhances energy savings during winter fast in king penguin chicks. J. Exp. Biol. 217, 2691–2697. doi: 10.1242/jeb.122671
Montero, D., Izquierdo, M. S., Tort, L., Robaina, L., and Vergara, J. M. (1999). High stocking density produces crowding stress altering some physiological and biochemical parameters in gilthead seabream, Sparus aurata, juveniles. Fish Physiol. Biochem. 20, 53–60. doi: 10.1023/A:1007719928905
Mosconi, G., Cardinaletti, G., Carotti, M., Palermo, F., Soverchia, L., and Polzonetti-Magni, A. M. (2006). “Neuroendocrine mechanisms regulating stress response in cultured teleost species,” in Fish Endocrinology, Vol. 2, eds M. Reinecke, G. Zaccone, and B. G. Kapoor (New Hampshire Enfield: Science Publishers), 693–720. doi: 10.1201/b10745-30
Murray, A. J. (2009). Metabolic adaptation of skeletal muscle to high altitude hypoxia: how new technologies could resolve the controversies. Genome Med. 1:117. doi: 10.1186/gm117
Nikinmaa, M. (2001). Haemoglobin function in vertebrates: evolutionary changes in cellular regulation in hypoxia. Respir. Physiol. 128, 317–329. doi: 10.1016/S0034-5687(01)00309-7
Nikinmaa, M., and Rees, B. B. (2005). Oxygen-dependent gene expression in fishes. Am. J. Physiol. Regul. Integr. Comp. Physiol. 288, R1079–R1090. doi: 10.1152/ajpregu.00626.2004
Nilsson, G. E. (2007). Gill remodeling in fish–a new fashion or an ancient secret? J. Exp. Biol. 210, 2403–2409. doi: 10.1242/jeb.000281
Pauletto, M., Manousaki, T., Ferraresso, S., Babbucci, M., Tsakogiannis, A., Louro, B., et al. (2018). Genomic analysis of Sparus aurata reveals the evolutionary dynamics of sex-biased genes in a sequential hermaphrodite fish. Commun. Biol. 1:119. doi: 10.1038/s42003-018-0122-7
Pearson, M. P., and Stevens, E. D. (1991). Size and haematological impact of the splenic erythrocyte reservoir in rainbow trout, Oncorhynchus mykiss. Fish Physiol. Biochem. 9, 39–50. doi: 10.1007/BF01987610
Pérez-Sánchez, J., Benedito-Palos, L., Estensoro, I., Petropoulos, Y., Calduch-Giner, J. A., Browdy, C. L., et al. (2015). Effects of dietary NEXT ENHANCE®150 on growth performance and expression of immune and intestinal integrity related genes in gilthead sea bream (Sparus aurata L.). Fish Shellfish Immunol. 44, 117–128. doi: 10.1016/j.fsi.2015.01.039
Pérez-Sánchez, J., Borrel, M., Bermejo-Nogales, A., Benedito-Palos, L., Saera-Vila, A., Calduch-Giner, J. A., et al. (2013). Dietary oils mediate cortisol kinetics and the hepatic expression profile of stress responsive genes in juveniles of gilthead sea bream (Sparus aurata) exposed to crowding stress. Comp. Biochem. Physiol. Part D 8, 123–130. doi: 10.1016/j.cbd.2013.02.001
Pérez-Sánchez, J., Martí-Palanca, H., and Kaushik, S. (1995). Ration size and protein intake affect growth hormone (GH) levels, hepatic GH-binding and plasma insulin-like growth factor-I immunoreactivity in a marine teleost, gilthead sea bream (Sparus aurata). J. Nutr. 125, 546–552. doi: 10.1093/jn/125.3.546
Pérez-Sánchez, J., Simó-Mirabet, P., Naya, F., Martos-Sitcha, J. A., Perera, E., Bermejo-Nogales, A., et al. (2018). Somatotropic axis regulation unravels the differential effect of nutritional and environmental factors in growth performance of marine farmed fish. Front. Endocrinol. 9:687. doi: 10.3389/fendo.2018.00687
Pérez-Sánchez, J., Terova, G., Simó-Mirabet, P., Rimoldi, S., Folkedal, O., Calduch-Giner, J. A., et al. (2017). Skin mucus of gilthead sea bream (Sparus aurata L.). Protein mapping and regulation in chronically stressed fish. Front. Physiol. 8:34. doi: 10.3389/fphys.2017.00034
Person-Le Ruyet, J., Labbé, L., Le Bayon, N., Sévère, A., Le Roux, A., Le Delliou, H., et al. (2008). Combined effects of water quality and stocking density on welfare and growth of rainbow trout (Oncorhynchus mykiss). Aquat. Living Resour. 21, 185–195. doi: 10.1051/alr:2008024
Piazzon, M. C., Calduch-Giner, J. A., Fouz, B., Estensoro, I., Simó-Mirabet, P., Puyalto, M., et al. (2017). Under control: how a dietary additive can restore the gut microbiome and proteomic profile, and improve disease resilience in a marine teleostean fish fed vegetable diets. Microbiome 5:164. doi: 10.1186/s40168-017-0390-3
Piazzon, M. C., Mladineo, I., Naya-Català, F., Dirks, R. P., Jong-Raadsen, S., Vrbatoviæ, A., et al. (2019). Acting locally - affecting globally: RNA sequencing of gilthead sea bream with a mild Sparicotyle chrysophrii infection reveals effects on apoptosis, immune and hypoxia related genes. BMC Genomics 20:200. doi: 10.1186/s12864-019-5581-9
Pichavant, K., Person-Le-Ruyet, J., Bayon, N. L., Severe, A., Roux, A. L., and Boeuf, G. (2001). Comparative effects of long-term hypoxia on growth, feeding and oxygen consumption in juvenile turbot and European sea bass. J. Fish Biol. 59, 875–883. doi: 10.1006/jfbi.2001.1702
Pickering, A. D. (1993). “Growth and stress in fish production,” in Genetics in Aquaculture, Vol. 111, eds A. E. Graham Gall and H. Chen (Amsterdam: Elsevier Science Publishers B.V.), 51–63. doi: 10.1016/b978-0-444-81527-9.50010-5
Poulain, L., Mathieu, H., Thomas, A., Borel, A. L., Remy, C., Levy, P., et al. (2017). Intermittent hypoxia-induced insulin resistance is associated with alterations in white fat distribution. Sci. Rep. 7:11180. doi: 10.1038/s41598-017-11782-0
Reindl, K. M., and Sheridan, M. A. (2012). Peripheral regulation of the growth hormone-insulin-like growth factor system in fish and other vertebrates. Comp. Biochem. Physiol. A Mol. Integr. Physiol. 163, 231–245. doi: 10.1016/j.cbpa.2012.08.003
Remen, M., Nederlof, M. A. J., Folkedal, O., Thorsheim, G., Sitjà-Bobadilla, A., Pérez-Sánchez, J., et al. (2015). Effect of temperature on the metabolism, behavior and oxygen requirements of Sparus aurata. Aquacult. Environ. Interac. 7, 115–123. doi: 10.3354/aei00141
Remen, M., Sievers, M., Torgersen, T., and Oppedal, F. (2016). The oxygen threshold for maximal feed intake of Atlantic salmon post-smolts is highly temperature-dependent. Aquaculture 464, 582–592. doi: 10.1016/j.aquaculture.2016.07.037
Richards, J. G. (2011). Physiological, behavioral and biochemical adaptations of intertidal fishes to hypoxia. J. Exp. Biol. 214, 191–199. doi: 10.1242/jeb.047951
Rimoldi, S., Benedito-Palos, L., Terova, G., and Pérez-Sánchez, J. (2016). Wide-targeted gene expression infers tissue-specific molecular signatures of lipid metabolism in fed and fasted fish. Rev. Fish Biol. Fish. 26, 93–108. doi: 10.1007/s11160-015-9408-8
Rodrigues, P. M., Martin, S. A. M., Silva, T. S., Boonanuntanasarn, S., Schrama, D., Moreira, M., et al. (2018). “Proteomics in fish and aquaculture research,” in Proteomics in Domestic Animals: from Farm to Systems Biology, eds A. de Almeida, D. Eckersall, and I. Miller (Cham: Springer), 311–338. doi: 10.1007/978-3-319-69682-9_16
Rolfe, D. F., and Brand, M. D. (1996). Contribution of mitochondrial proton leak to skeletal muscle respiration and to standard metabolic rate. Am. J. Physiol. Regul. Integr. Comp. Physiol. 271, C1380–C1389. doi: 10.1152/ajpcell.1996.271.4.C1380
Ruer, P. M., Cech, J. J. Jr., and Doroshov, S. I. (1987). Routine metabolism of the white sturgeon, Acipenser transmontanus: effect of population density and hypoxia. Aquaculture 62, 45–52. doi: 10.1016/0044-8486(87)90183-9
Salin, K., Villasevil, E. M., Auer, S. K., Anderson, G. J., Selman, C., Metcalfe, N. B., et al. (2016). Simultaneous measurement of mitochondrial respiration and ATP production in tissue homogenates and calculation of effective P/O ratios. Phys. Rep. 4:e13007. doi: 10.14814/phy2.13007
Sangiao-Alvarellos, S., Guzmán, J. M., Láiz-Carrión, R., Míguez, J. M., Martín Del Río, M. P., Mancera, J. M., et al. (2005). Interactive effects of high stocking density and food deprivation on carbohydrate metabolism in several tissues of gilthead sea bream Sparus auratus. J. Exp. Zool. A Comp. Exp. Biol. 303, 761–775. doi: 10.1002/jez.a.203
Saravanan, S., Geurden, I., Figueiredo-Silva, A. C., Kaushik, S. J., Haidar, M. N., Verreth, J. A., et al. (2012). Control of voluntary feed intake in fish: a role for dietary oxygen demand in Nile tilapia (Oreochromis niloticus) fed diets with different macronutrient profiles. Br. J. Nutr. 108, 1519–1529. doi: 10.1017/S0007114511006842
Shimizu, M., Swanson, P., Fukada, H., Hara, A., and Dickhoff, W. W. (2000). Comparison of extraction methods and assay validation for salmon insulin-like growth factor-I using commercially available components. Gen. Comp. Endocrinol. 119, 26–36. doi: 10.1006/gcen.2000.7498
Simó-Mirabet, P., Bermejo-Nogales, A., Calduch-Giner, J. A., and Pérez-Sánchez, J. (2017a). Tissue-specific gene expression and fasting regulation of sirtuin family in gilthead sea bream (Sparus aurata). J. Comp. Physiol. B 187, 153–163. doi: 10.1007/s00360-016-1014-0
Simó-Mirabet, P., Piazzon, M. C., Calduch-Giner, J. A., Ortiz,Á, Puyalto, M., Sitjà-Bobadilla, A., et al. (2017b). Sodium salt medium-chain fatty acids and Bacillus-based probiotic strategies to improve growth and intestinal health of gilthead sea bream (Sparus aurata). PeerJ. 5:e4001. doi: 10.7717/peerj.4001
Simó-Mirabet, P., Perera, E., Calduch-Giner, J. A., Afonso, J. M., and Pérez-Sánchez, J. (2018). Co-expression analysis of sirtuins and related metabolic biomarkers in juveniles of gilthead sea bream (Sparus aurata) with differences in growth performance. Front. Physiol. 9:608. doi: 10.3389/fphys.2018.00608
Skrzynska, A. K., Martos-Sitcha, J. A., Martínez-Rodríguez, G., and Mancera, J. M. (2018). Unraveling vasotocinergic, isotocinergic and stress pathways after food deprivation and high stocking density in the gilthead sea bream. Comp. Biochem. Physiol. A Mol. Integr. Physiol. 215, 35–44. doi: 10.1016/j.cbpa.2017.10.012
Soivio, A., Nikinmaa, M., and Westman, K. (1980). The blood oxygen binding properties of hypoxic Salmo gairdneri. J. Comp. Physiol. 136, 83–87. doi: 10.1007/BF00688627
Speckner, W., Schindler, J. F., and Albers, C. (1989). Age-dependent changes in volume and haemoglobin content of erythrocytes in the carp (Cyprinus carpio). J. Exp. Biol. 141, 133–149.
Treins, C., Giorgetti, S., Murdaca, J., Semenza, G. L., and Van Obberghen, E. (2002). Insulin stimulates hypoxia-inducible factor 1 through a phosphatidylinositol 3-kinase/target of rapamycin-dependent signaling pathway. J. Biol. Chem. 277, 27975–27981. doi: 10.1074/jbc.M204152200
Val, A. L. (2000). Organic phosphates in the red blood cells of fish. Comp. Biochem. Physiol. 125, 417–435. doi: 10.1016/S1095-6433(00)00184-7
Valli, A., Rodriguez, M., Moutsianas, L., Fischer, R., Fedele, V., Huang, H.-L., et al. (2015). Hypoxia induces a lipogenic cancer cell phenotype via HIF1α-dependent and -independent pathways. Oncotarget 6, 1920–1941. doi: 10.18632/oncotarget.3058
Van Weerd, J. H., and Komen, J. (1998). The effects of chronic stress on growth in fish: a critical appraisal. Comp. Biochem. Physiol. Part A Mol. Integr. Physiol. 120, 107–112. doi: 10.1016/S1095-6433(98)10017-X
Vega-Rubín de Celis, S., Gómez-Requeni, P., and Pérez-Sánchez, J. (2004). Production and characterization of recombinantly derived peptides and antibodies for accurate determinations of somatolactin, growth hormone and insulin-like growth factor-I in European sea bass (Dicentrarchus labrax). Gen. Comp. Endocrinol. 139, 266–277. doi: 10.1016/j.ygcen.2004.09.017
Vélez, E. J., Lutfi, E., Azizi, S., Perelló, M., Salmerón, C., Riera-Codina, M., et al. (2017). Understanding fish muscle growth regulation to optimize aquaculture production. Aquaculture 467, 28–40. doi: 10.1016/j.aquaculture.2016.07.004
Vijayan, M. M., Aluru, N., and Leatherhand, J. F. (2010). “Stress response and the role of cortisol,” in Fish diseases and Disorders, Vol. 2, eds J. F. Leatherhand and P. T. K. Woo (Oxfordshire: CAB International), 182–201. doi: 10.1079/9781845935535.0182
Vikeså, V., Nankervis, L., and Hevrøy, E. M. (2017). High dietary energy level stimulates growth hormone receptor and feed utilization in large Atlantic salmon (Salmo salar L.) under hypoxic conditions. Aquac. Nutr. 23, 1193–1203. doi: 10.1111/anu.12488
Wells, R. M. G., Grigg, G. C., Beard, L. A., and Summers, G. (1989). Hypoxic responses in a fish from a stable environment: blood oxygen transport in the Antarctic fish Pagothenia borchgrevinki. J. Exp. Biol. 141, 97–111.
Wendelaar Bonga, S. E. (2011). “Hormonal responses to stress,” in Encyclopaedia of Fish Physiology, ed. A. P. Farrell (San Diego, CA: Academic Press), 1515–1523. doi: 10.1016/B978-0-12-374553-8.00183-0
Wold, S., Sjöström, M., and Eriksson, L. (2001). PLS-regression: a basic tool of chemometrics. Chemometr. Intell. Lab. Syst. 58, 109–130. doi: 10.1016/S0169-7439(01)00155-1
Wood, S. C., and Johansen, K. (1972). Adaptation to hypoxia by increased HbO2 affinity and decreased red cell ATP concentration. Nat. New Biol. 237, 278–279. doi: 10.1038/newbio237278a0
Yáñez, J. M., Newman, S., and Houston, R. D. (2015). Genomics in aquaculture to better understand species biology and accelerate genetic progress. Front. Genet. 6:128. doi: 10.3389/fgene.2015.00128
Yu, Y., Vidalino, L., Anesi, A., Macchi, P., and Guella, G. (2014). A lipidomics investigation of the induced hypoxia stress on HeLa cells by using MS and NMR techniques. Mol. Biosyst. 10, 878–890. doi: 10.1039/C3MB70540D
Yúfera, M., Perera, E., Mata-Sotres, J. A., Calduch-Giner, J., Martínez-Rodríguez, G., and Pérez-Sánchez, J. (2017). The circadian transcriptome of marine fish (Sparus aurata) larvae reveals highly synchronized biological processes at the whole organism level. Sci. Rep. 7:12943. doi: 10.1038/s41598-017-13514-w
Keywords: hematology, hypometabolism, hypoxia, limiting oxygen saturation, Sparus aurata, stocking density, tissue-specific transcriptomics
Citation: Martos-Sitcha JA, Simó-Mirabet P, de las Heras V, Calduch-Giner JÀ and Pérez-Sánchez J (2019) Tissue-Specific Orchestration of Gilthead Sea Bream Resilience to Hypoxia and High Stocking Density. Front. Physiol. 10:840. doi: 10.3389/fphys.2019.00840
Received: 01 January 2019; Accepted: 19 June 2019;
Published: 10 July 2019.
Edited by:
José Luis Soengas, University of Vigo, SpainReviewed by:
Ivan Viegas, University of Coimbra, PortugalCopyright © 2019 Martos-Sitcha, Simó-Mirabet, de las Heras, Calduch-Giner and Pérez-Sánchez. This is an open-access article distributed under the terms of the Creative Commons Attribution License (CC BY). The use, distribution or reproduction in other forums is permitted, provided the original author(s) and the copyright owner(s) are credited and that the original publication in this journal is cited, in accordance with accepted academic practice. No use, distribution or reproduction is permitted which does not comply with these terms.
*Correspondence: Juan Antonio Martos-Sitcha, anVhbmFudG9uaW8uc2l0Y2hhQHVjYS5lcw==; anVhbmFudG9uaW8uc2l0Y2hhQGljbWFuLmNzaWMuZXM=; Jaume Pérez-Sánchez, amFpbWUucGVyZXouc2FuY2hlekBjc2ljLmVz
†Present address: Juan Antonio Martos-Sitcha, Department of Biology, Faculty of Marine and Environmental Sciences, Instituto Universitario de Investigación Marina (INMAR), Campus de Excelencia Internacional del Mar (CEI-MAR), University of Cádiz, Cádiz, Spain Verónica de las Heras, Futuna Blue España S.L., El Puerto de Santa María, Cádiz, Spain
Disclaimer: All claims expressed in this article are solely those of the authors and do not necessarily represent those of their affiliated organizations, or those of the publisher, the editors and the reviewers. Any product that may be evaluated in this article or claim that may be made by its manufacturer is not guaranteed or endorsed by the publisher.
Research integrity at Frontiers
Learn more about the work of our research integrity team to safeguard the quality of each article we publish.